- 1Cornell Atkinson Center for Sustainability, Cornell University, Ithaca, NY, United States
- 2Department of Public and Ecosystem Health, College of Veterinary Medicine, Cornell University, Ithaca, NY, United States
- 3Department of Population Medicine and Diagnostic Sciences, College of Veterinary Medicine, Cornell University, Ithaca, NY, United States
Introduction: Antimicrobial resistance (AMR) is a growing and complex One Health concern worldwide, threatening the practice of human and veterinary medicine. Although dogs are a potential reservoir of multidrug-resistant bacteria, there are very few surveillance studies on AMR from the canine population in the United States. Here, we assessed the antimicrobial susceptibility patterns, identified temporal resistance and minimum inhibitory concentration trends, and described associations between resistance phenotypes among canine clinical enterococci in the northeastern United States.
Methods: Through a large-scale retrospective study design, we collected species identification, minimum inhibitory concentration, and clinical data from 3,659 canine enterococci isolated at the Cornell University Animal Health Diagnostic Center between 2007 and 2020. We used the Mann-Kendall test, Sen’s slope, multivariable logistic regression, and survival analysis models to detect the presence of a significant trend in resistance over the study period.
Results: Enterococcus faecalis was the most prevalent species (67.1% of isolates), followed by Enterococcus faecium (20.4%). We found high levels of AMR among enterococci to almost all the tested antimicrobials, particularly E. faecium. The lowest percentage of resistance was to vancomycin and chloramphenicol. Multidrug resistance was common (80% of E. faecium and 33% of E. faecalis) and 31 isolates were extensively drug resistant. Multidrug resistance among E. faecium increased over time, but not in E. faecalis. Resistance to penicillins, enrofloxacin, and rifampin increased during the study period, but resistance to tetracyclines is on a downward trajectory compared to AMR data from the last decade. Emerging vancomycin-resistant E. faecalis (0.3%) and E. faecium (0.8%) infections in the canine population are of great concern to both human and animal health. One E. faecium isolate with acquired vancomycin resistance was identified in 2017 and four vancomycin-resistant enterococci isolates were identified in 2020.
Conclusion: There is a crucial need to make rational prescribing decisions on the prudent use of antimicrobials and improve the quality of care for patients, especially when empirical antimicrobial treatment for enterococcal infection is common.
1. Introduction
Antimicrobial resistance (AMR) has become one of the leading global public health challenges facing humanity, posing a major threat to human and animal health around the globe (Murray et al., 2022). Although AMR is a complex issue with many contributing factors, excessive use of antimicrobials in humans and animals represents the most important driving force toward the selection of bacteria with acquired resistance and subsequently the emergence and dissemination of AMR determinants (Holmes et al., 2016).
During the last few decades, the number of companion animals (e.g., dogs, cats, horses) in the United States has substantially increased and a change in their social role has occurred; the pet dog population has been recently estimated at nearly 77 million in the country, with approximately 38% of households having a dog (Overgaauw et al., 2020). Pet-associated bacterial infections represent a relatively neglected area compared with food-producing animal infections. Household pets live in close contact with humans and pose a substantial risk for transmission of illnesses and drug-resistant pathogens to susceptible owners, pet shop employees, veterinarians, as well as other animals (Rees et al., 2021). Novel resistance determinants continue to emerge in zoonotic pathogens and commensal bacteria isolated from household pets, mostly dogs and cats (Jackson et al., 2009; Leonard et al., 2011; Cummings et al., 2015; KuKanich and Lubbers, 2015; Zhang et al., 2016; Bourély et al., 2019; Li et al., 2021; Hata et al., 2022; Tóth et al., 2022). Dogs are increasingly recognized as a potential reservoir and a relevant transmission pathway of commensal and pathogenic bacteria or their resistance genes (Harada et al., 2012; Damborg et al., 2016; Francois Watkins et al., 2021).
Narrow- and broad-spectrum antimicrobial agents are used widely in veterinary medicine for therapeutic and prophylactic purposes in companion animals. Many of the antimicrobials are the same as or similar to those used in human medicine (Joosten et al., 2020). Prescriptions for antimicrobials important in human medicine to companion animals in the United States do not have to be reported, though they are common among veterinarians and legal (Papich, 2021). A direct relationship exists between excessive use of antimicrobials and the spread of drug-resistant bacteria, increasing the risk of antimicrobial treatment failure in both animals and humans (Llor and Bjerrum, 2014).
Among the animal commensal flora, enterococcal species have been commonly considered as a potential source of infections and resistance genes among humans. Enterococcus spp. are Gram-positive, catalase-negative, facultative anaerobic commensal bacteria that exist in chains or pairs and do not form spores, with the ability to grow in 6.5% NaCl broth and a particular resistance to drying and bile (Švec and Devriese, 2015). These natural inhabitants of the gastrointestinal tract and oral environment of mammals can cause opportunistic infections in humans and dogs and constitute a frequent reason for antimicrobial prescription (Komiyama et al., 2016; Ramos et al., 2020). Enterococcal species are a common cause of urinary tract and skin and soft tissue infections but also a major pathogen of concern responsible for life-threatening infections such as endocarditis, abscesses, meningitis, and bacteremia (Mercuro et al., 2018).
Enterococcus spp. are known to be intrinsically resistant to a number of antimicrobial agents, including cephalosporins, clindamycin, and colistin, and exhibit low-level resistance to β-lactams and aminoglycosides (Zaheer et al., 2020). The minimal inhibitory concentration (MIC) of enterococci to gentamicin typically ranges from 6 mg/l to as high as 48 mg/l. The facultative anaerobic metabolism of enterococci is most likely the reason of their intrinsic resistance to all aminoglycosides by reducing the transmembrane potential and thereby limiting drug uptake into the cell (Chow, 2000). The use of trimethoprim-sulfamethoxazole against enterococci is not appropriate and associated with adverse effects. Although enterococci appear sensitive in vitro, the antimicrobial is not effective in vivo and not recommended clinically (Wisell et al., 2008; Sykes, 2014). Additionally, enterococci are remarkable in their ability to survive their hosts (Tyne et al., 2019), acquire AMR determinants, and horizontally transfer antimicrobial-resistant determinants via genetic mobile elements to other enterococcal strains or different species such as Staphylococcus aureus and Listeria monocytogenes (Leclercq et al., 1989; Johnson and Woodford, 2002; González-Zorn and Courvalin, 2003; de Niederhäusern et al., 2004; Ahmed and Baptiste, 2018). Enterococcus faecalis and Enterococcus faecium, the most prevalent enterococci species encountered in human and animal infections, have become of increasing importance over recent decades (Barlow et al., 2017). Dogs have been described as potential reservoirs of drug-resistant enterococci in animals worldwide, but available data on resistant enterococci remain scarce in the United States. We aim to assess the antimicrobial susceptibility patterns, identify trends in resistance, and describe associations between resistance phenotypes among canine clinical enterococci isolates in the northeastern United States. Understanding the prevalence and temporal trends of AMR among dogs is critical to understand the One Health risk associated with antimicrobial use and AMR in companion animals.
2. Materials and methods
2.1. Study design, data source, and management
Retrospective clinical and minimum inhibitory concentration (MIC) records from Enterococcus spp. isolated from canines between July 19, 2007, and December 31, 2020 were analyzed in the present study. The data were provided by the Cornell University Animal Health Diagnostic Center (AHDC) in Ithaca, New York. The records were analyzed using R software (R Core team, version 4.1.0; R Studio, version 1.4.1106). The database was imported for cleaning, variable coding, and analysis. Descriptive analysis, models, and illustrations were done on all variables using several R packages (e.g., stringr, summarytools, prettyR, ggplot2, hrbrthemes, stats, Kendall, survival, icenReg). All code necessary to replicate the analysis is publicly available (DOI: 10.5281/zenodo.7126369).
The database was assessed for duplicates and missing information. According to the Clinical and Laboratory Standards Institute (CLSI) guideline regarding cumulative antibiograms reports, only one Enterococcus isolate per culture (our dataset lacked unique patient identifiers) was included in our investigation, regardless of the body site and antimicrobial susceptibility pattern. Subsequent isolates were identified and removed from the database. Variables collected from the laboratory information system included the species identification, date of the isolation, origin of clinical sample (body site), and MIC value for each antimicrobial agent. All enterococcal isolates were recovered from patients with clinically significant infections, including urinary tract, skin and soft tissues, reproductive system, and invasive infections.
2.2. Microbiological analysis and antimicrobial susceptibility testing
Microbiological identification at species level was performed using either the Sensititre Automated Microbiology System (TREK Diagnostic Systems, Cleveland, Ohio, USA) or Matrix-Assisted Laser Desorption/Ionization Time-Of-Flight mass spectrometry (MALDI Biotyper; Bruker, Bellerica, MA, USA). All procedures at the Cornell University AHDC were performed in accordance with accreditation by the American Association of Veterinary Laboratory Diagnosticians (AAVLD). Antimicrobial susceptibility testing of Enterococcus isolates was carried out using the broth microdilution method as previously described (Cummings et al., 2015). The Sensititre™ Gram Positive MIC Plates, panel CMV1BURF and COMPGP1F, were used for canine urinary and non-urinary Enterococcus spp. isolates, respectively. Quality control was performed weekly using E. coli ATCC 25922, S. aureus 29213, E. faecalis 29212, and Pseudomonas aeruginosa 27853. The MIC ranges for quality control recommended by the CLSI were used, and results were accepted if the MIC values were within expected ranges for these bacterial strains.
The MIC values were interpreted according to the interpretive criteria (i.e., breakpoints) recommended by the CLSI guidelines (CLSI-VET01S ED5: 2020; Human breakpoints were used as there are no animal enterococci breakpoints; Weinstein and Lewis, 2020) and, if a CLSI breakpoint was not available, veterinary antibiogram committee of the French Society for Microbiology (CA-SFM; www.sfm-microbiologie.fr) to classify isolates as susceptible or non-susceptible to each agent. The drugs selected for this study (Table 1) have pharmacologic activity against Enterococcus spp. and are clinically relevant to canine medicine, either through therapeutic use or as markers for susceptibility to commonly used antimicrobial agents. No clinical breakpoints are available in the CLSI/CA-SFM guidelines for enrofloxacin; thus, we adopted those from the veterinary CA-SFM guidelines for Streptococcus. Regardless of isolation year, all MIC values were interpreted using the same set of current guidelines. We excluded the rare cases of historical MIC values that could not be interpreted with the current CLSI or CA-SFM clinical breakpoints. The few isolates with intermediate susceptibility were categorized as being non-susceptible.
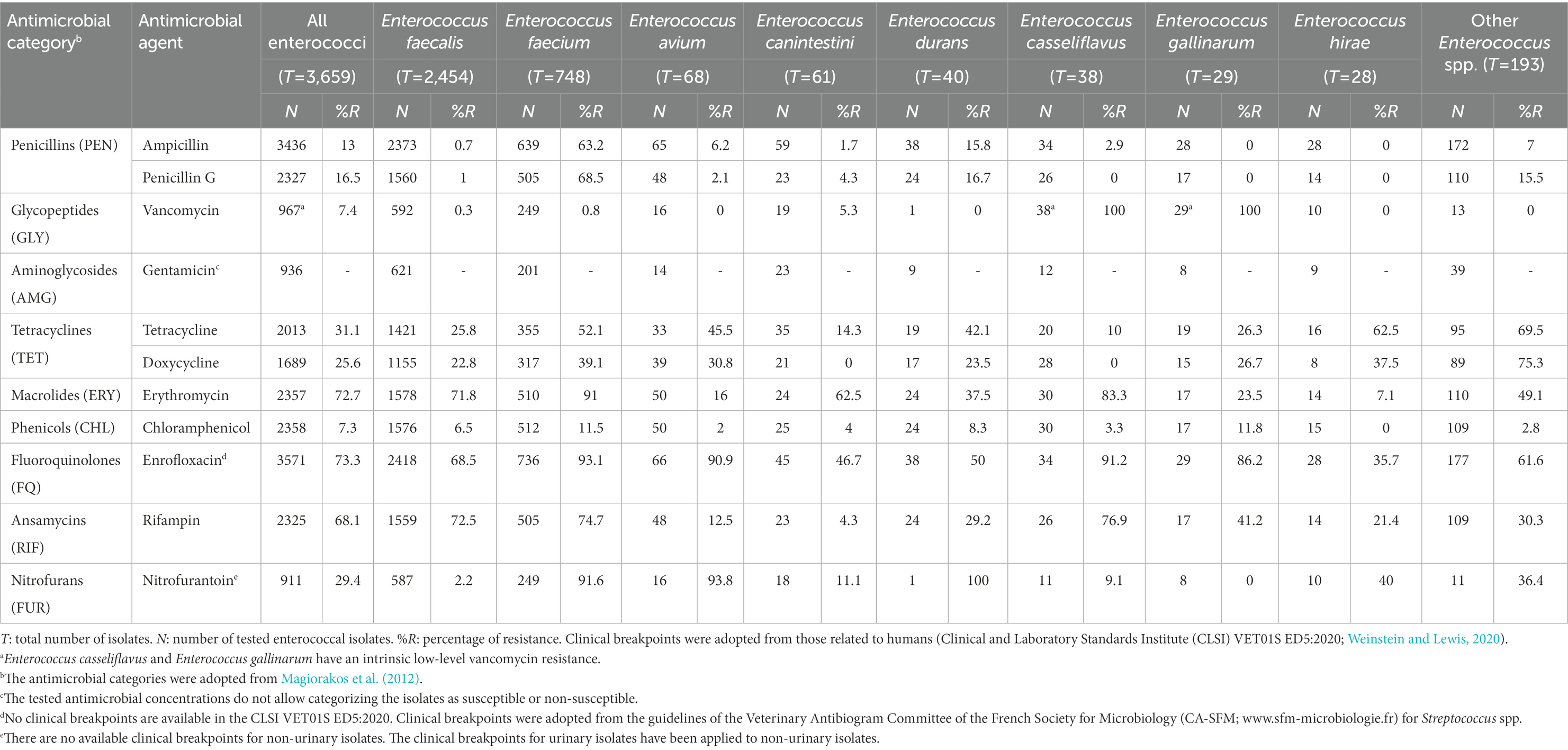
Table 1. Prevalence of antimicrobial resistance among Enterococcus spp. clinical isolates from dogs stratified by species, from canine clinical infections at the Cornell University Animal Health Diagnostic Center (AHDC), 2007–2020.
Although 11 antimicrobials (penicillin G, ampicillin, vancomycin, gentamicin, tetracycline, doxycycline, erythromycin, chloramphenicol, enrofloxacin, rifampicin, and nitrofurantoin) were tested throughout the study period, only ampicillin (n = 3,589 isolates tested out of 3,659) and enrofloxacin (n = 3571 isolates tested out of 3,659) were used on almost all Enterococcus spp. isolates. Vancomycin and nitrofurantoin were only consistently used after 2017. The susceptibility of urinary isolates was systematically assessed using a narrow antimicrobial susceptibility testing panel (CMV1BURF Sensititre plate), including ampicillin, tetracycline, and enrofloxacin. In the case of non-urinary isolates, the antimicrobial susceptibility testing panel was extended to the full list of antimicrobials, except tetracycline which was rarely tested for non-urinary isolates. On the other hand, in few specific cases (e.g., multidrug-resistant (MDR) isolates) of Enterococcus urinary tract infections, the susceptibility was assessed using the larger non-urinary panel. We did not report the percentage of resistance against these antimicrobials among urinary isolates when fewer than 5% of the isolates representing a species were tested (Table 2). Given that Enterococcus gallinarum and Enterococcus casseliflavus have intrinsic low-level vancomycin resistance (Monticelli et al., 2018), we categorized the respective isolates as resistant to vancomycin regardless of their MIC values.
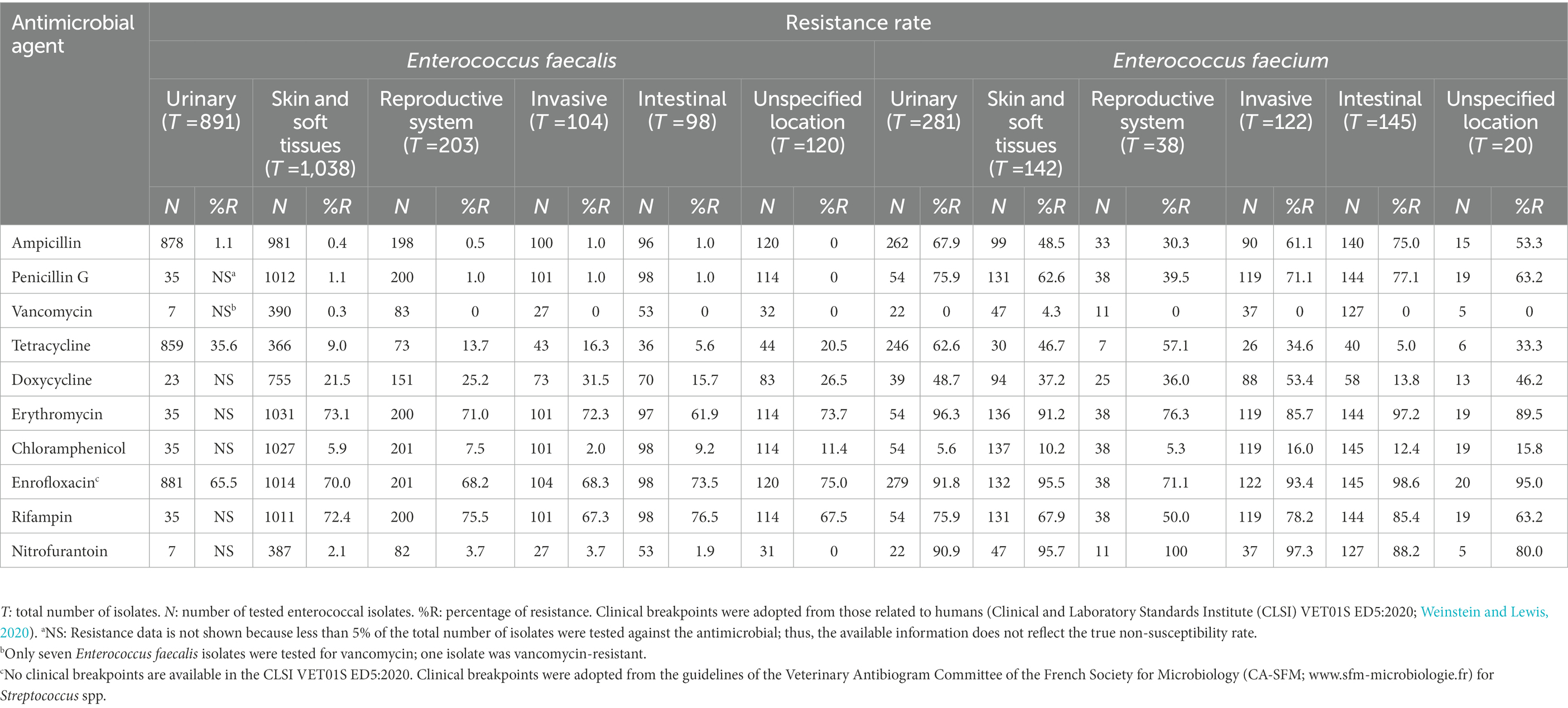
Table 2. Prevalence of antimicrobial resistance among Enterococcus spp. clinical isolates from dogs stratified by sample source, from canine clinical infections at the Cornell University Animal Health Diagnostic Center (AHDC), 2007–2020.
2.3. Definition of multidrug resistant isolates
We divided our isolates into two main groups, E. faecalis and E. faecium isolates. MDR isolates were defined as acquired non-susceptibility to at least one agent in three or more antimicrobial categories (Magiorakos et al., 2012). Extremely drug resistant (XDR) isolates were defined as in vitro acquired non-susceptibility to at least one antimicrobial drug in all but two or fewer antimicrobial categories (Magiorakos et al., 2012). We defined nine categories: penicillins (PEN; penicillin G and ampicillin), glycopeptides (GLY; vancomycin), tetracyclines (TET; tetracycline and doxycycline), macrolides (ERY; erythromycin), phenicols (CHL; chloramphenicol), fluoroquinolones (FQ; enrofloxacin), ansamycins (RIF; rifampin), and nitrofurans (FUR; nitrofurantoin; Table 1). The tested MIC values for gentamicin did not allow us to interpret isolates as susceptible or resistant with the current breakpoint; thus, we excluded the aminoglycoside category in our MDR definition.
2.4. Statistical analysis
Descriptive and statistical analysis were performed using the R software. The mean, standard deviation, and range of Enterococcus isolates per year was calculated. The categorical data was presented as frequencies and associated proportions. For each antimicrobial agent, the differences in resistance trends across E. faecalis and E. faecium were initially compared using the chi-squared test. The Mann–Kendall test (MKT) and Sen’s slope were used to detect temporal trends of antimicrobial monoresistance and multidrug resistance among E. faecalis and E. faecium isolates over the study period (2007–2020). Subsequently, using multivariable logistic regression (MLR), we modeled resistance to antimicrobials for E. faecalis and E. faecium accounting for both body site and time, divided into four periods: (1) 2007–2010, (2) 2011–2014, (3) 2015–2017, and (4) 2018–2020. Resistance to the antimicrobial was the outcome and body site and study period were the explanatory variables. We analyzed MIC distributions with Cox proportional hazards regression models for all 12 tested antimicrobials. Briefly, the inhibition of bacterial growth was considered as the event; thus, we analyzed the concentration of antimicrobial required to achieve the event (i.e., MIC), instead of time to event. In this context, resistance trends can be analyzed over an entire range of concentrations and no specific breakpoint value for resistance has to be determined. A separate model was created for each tested antimicrobial with species identification, body site, and study period as the explanatory variables. A Hazard Ratio (HR) has been calculated indicating a higher (HR > 1) or lower (HR < 1) likelihood of growth inhibition of the studied Enterococcus group at each antimicrobial concentration compared to a reference Enterococcus group (Spruance et al., 2004; Combescure et al., 2014; Osman et al., 2022). We assessed the assumption of proportional hazards visually by examining the survival curves. MLR models were also used to predict resistance to each of the regularly used antimicrobials with co-resistant and cross-resistant agents among Enterococcus spp. and the E. faecalis and E. faecium subpopulations. Antimicrobials within the same category were removed from the statistical models (e.g., penicillin was excluded from models to predict ampicillin resistance). All statistical tests were two-sided, with a type I error set at α = 0.05. Backward stepwise model selection was used to better identify the associations of covariates with the outcome antimicrobial in MLR models. To decrease the false discovery rate in our statistical analyses, we performed the Benjamini-Hochberg method to adjust the calculated p-values in each table, with a false discovery rate of 0.05 (Benjamini and Hochberg, 1995).
3. Results
A total of 3,659 canine Enterococcus spp. unique isolates (one isolate per culture) were collected at the Cornell University AHDC during a 14-year period (2007–2020). These isolates were mostly obtained from urine (N = 1,344; 36.7%), followed by skin and soft tissues (N = 1,324; 36.2%), reproductive system (N = 319; 8.7%), invasive locations (N = 261; 7.1%), intestinal tract (N = 252; 6.9%), and other locations (N = 159; 4.3%). Eleven different Enterococcus spp. were isolated from canine clinical specimens. The predominant species identified was E. faecalis (N = 2,454; 67.1%), followed by E. faecium (N = 748; 20.4%), Enterococcus avium (N = 68; 1.9%), Enterococcus canintestini (N = 61; 1.7%), Enterococcus durans (N = 40; 1.1%), E. casseliflavus (N = 38; 1.0%), E. gallinarum (N = 29; 0.8%), Enterococcus hirae (N = 28; 0.8%), Enterococcus canis (N = 5; 0.1%), Enterococcus raffinosus (N = 5; 0.1%), and Enterococcus mundtii (N = 1; 0.0%). The remaining isolates (N = 182, 5.0%) were not identified at species level. Overall, the mean number of Enterococcus spp. isolated per year was 261 (standard deviation [SD]: 77, range: 87–369), with 175 (SD: 49, range: 67–248) E. faecalis isolates and 53 (SD: 24, range: 11–98) E. faecium isolates per year.
Enterococcus faecalis isolates were mainly obtained from skin and soft tissues (N = 1038, 42.3%) and urine (N = 891, 36.3%). However, E. faecium was isolated from broader specimen types including urine (N = 281, 37.6%), intestinal tract (N = 145; 19.4%), skin and soft tissues (N = 142, 19.0%), and invasive locations (N = 122, 16.3%; Table 2).
The prevalence of resistance to each antimicrobial across the study period, stratified by species, is summarized in Table 1. Antimicrobial susceptibility testing showed a relatively low resistance rate to chloramphenicol (7.3% resistant), vancomycin (7.4%), and penicillins (13%–16.5%) among Enterococcus spp. isolates. Higher percentages of resistance were observed against tetracyclines (25.6% resistant to doxycycline and 31.1% to tetracycline), nitrofurantoin (29.4%), rifampin (68.1%), erythromycin (72.7%), and enrofloxacin (73.3%).
Of note, only three antimicrobials were consistently tested on E. faecalis and E. faecium urinary isolates: ampicillin, tetracycline, and enrofloxacin (Table 2). After dividing the study years into four periods and accounting for year of isolation, MLR analysis demonstrated that Enterococcus spp. non-urinary isolates were significantly less likely than urinary isolates to present in vitro resistance to tetracycline (odds ratio (OR) = 0.10–0.39; p < 0.05; Table 3). However, intestinal Enterococcus isolates showed the highest rates of resistance to ampicillin (OR = 3.51; 95% confidence interval (95% CI) = 2.58 to 4.77; p < 0.001) and enrofloxacin (OR = 2.70; 95% CI = 1.84 to 4.06; p < 0.001) compared to urinary isolates. In addition, invasive isolates were more likely to be resistant to the abovementioned antimicrobials (p < 0.01) compared to urinary isolates (Table 3). In contrast, after accounting for species, body site, and study period, we only found higher MICs against enrofloxacin among intestinal and invasive isolates compared to urinary isolates (Supplementary Table S1). Compared to E. faecalis, isolates from E. faecium were more resistant to all the tested antimicrobials, particularly penicillins (63.2% ampicillin resistant and 68.5% penicillin resistant in E. faecium versus 0.75 and 1.0% in E. faecalis, p ≤ 0.001), vancomycin (0.8% versus 0.3%, p = 0.729), tetracyclines (39.1% doxycycline resistant and 52.1% tetracycline resistant versus 22.8and 25.8%, p ≤ 0.001), erythromycin (91.0% versus 71.8%, p ≤ 0.001), enrofloxacin (93.1% versus 68.5%, p ≤ 0.001), and nitrofurantoin (91.6% versus 2.2%, p ≤ 0.001). Survival analysis models concurred with changes in the percent of resistant isolates. Enterococcus other than faecalis and faecium showed a decrease in MIC values for penicillin (p ≤ 0.001), gentamicin (p ≤ 0.001), erythromycin (i ≤ 0.001), chloramphenicol (p ≤ 0.001), and rifampin (p ≤ 0.001) but an increase in MIC values for enrofloxacin (p ≤ 0.001) compared to the reference E. faecalis (Supplementary Table S1).
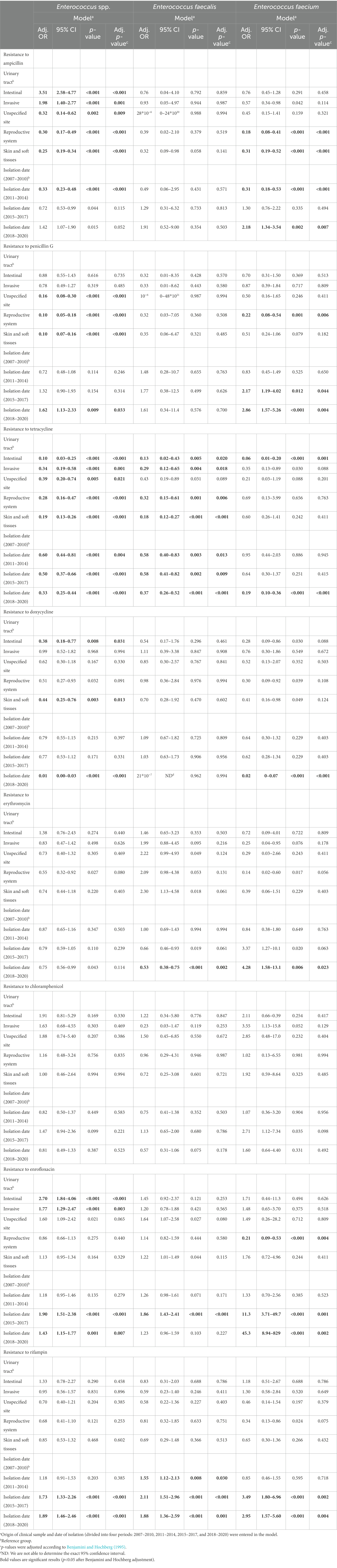
Table 3. Determinants of resistance to the common antimicrobials including specimen source and study period among Enterococcus spp. isolates using multivariable logistic regression models, in canine clinical infections at the Cornell University Animal Health Diagnostic Center (AHDC), 2007–2020.
Our data showed that the pan-susceptible pattern was uncommon among both E. faecalis (N = 229/2454, 9.3%; Figure 1A) and E. faecium (N = 27/748, 3.6%; Figure 2A). The rates of monoresistance (29.5%) and biresistance (30.8%) patterns were higher in E. faecalis compared to those in E. faecium (7.9% monoresistance, 12.4% biresistance). MDR, defined as in vitro acquired non-susceptibility to at least one drug in three or more antimicrobial categories (Magiorakos et al., 2012), was more frequently observed among E. faecium (76.1%) compared to E. faecalis (30.4%). Most MDR E. faecium isolates (82.6%) showed resistance to penicillins, but penicillin resistance was rare among MDR E. faecalis (2.9%). The most common multidrug resistance pattern among MDR E. faecalis isolates was erythromycin-fluoroquinolones-rifampin (57.4%, 428/746), followed by the same resistance pattern with an additional resistance to tetracycline (13.8%, 103/746; Figure 1B). Enterococcus faecium isolates were resistant to more antimicrobial classes (Figure 2B): penicillins-erythromycin-fluoroquinolones-rifampin-nitrofurantoin (24.1%, 137/569) was predominant, followed by penicillins-tetracycline-fluoroquinolones (21.3%, 121/569) and penicillins-tetracycline-erythromycin-fluoroquinolones-rifampin (10.5%, 60/569). XDR pattern, defined as in vitro acquired non-susceptibility to at least one antimicrobial drug in all but two or fewer antimicrobial categories (Magiorakos et al., 2012), was observed in E. faecium (N = 41) and E. faecalis (N = 1) isolates.
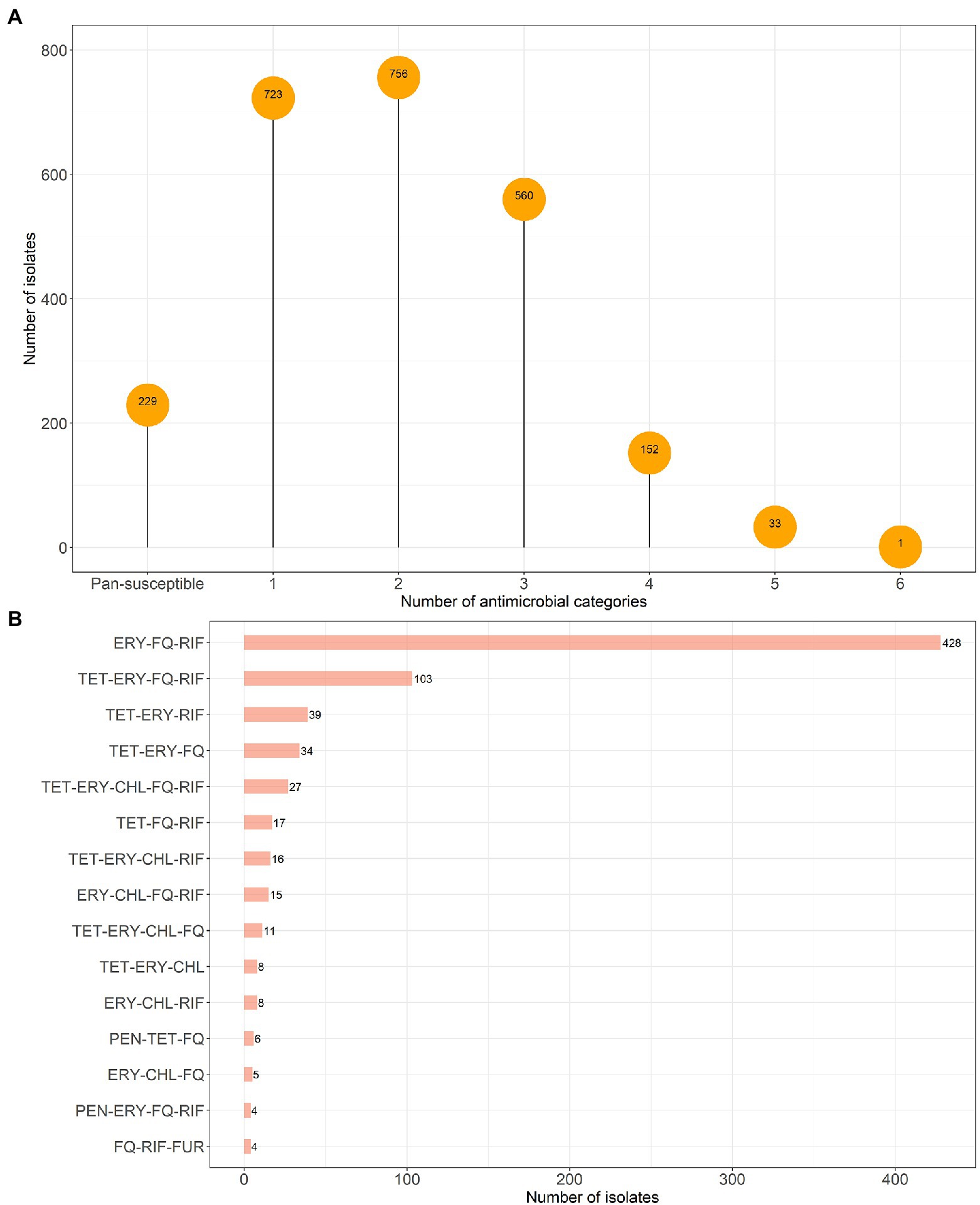
Figure 1. Distribution of resistance by number of antimicrobial categories (A) and most 15 common multidrug resistance patterns among Enterococcus faecalis isolates (B), in canine clinical infections at the Cornell University Animal Health Diagnostic Center (AHDC), 2007–2020. Antimicrobial category abbreviations are listed in Table 1.
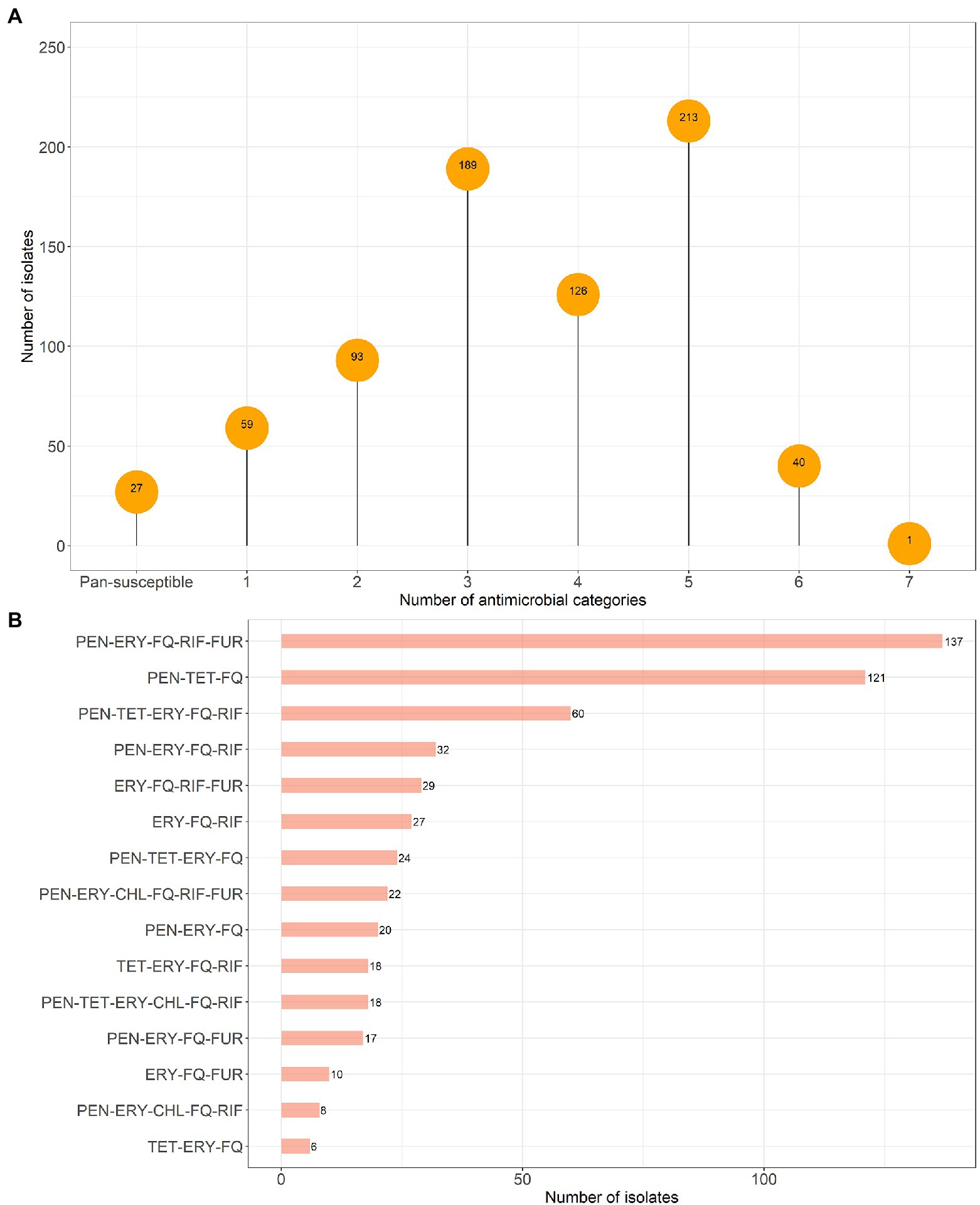
Figure 2. Distribution of resistance by number of antimicrobial categories (A) and most 15 common multidrug resistance patterns among Enterococcus faecium isolates (B), in canine clinical infections at the Cornell University Animal Health Diagnostic Center (AHDC), 2007–2020. Antimicrobial category abbreviations are listed in Table 1.
The proportion of E. faecalis that were MDR increased, generally, by 0.2 percentage points each year but the trend was not statistically significant (Z = 0.77, Sen’s = 0.002, p-value = 0.443). Similarly, E. faecium MDR proportion increased by 2 percentage points each year, but the trend was not statistically significant by the MKT (Z = 1.75, Sen’s = 0.021, p-value = 0.080). There was a statistically significant increase in the percent of isolates resistant to enrofloxacin [E. faecalis: increase of 1.1 percentage point per year (Z = 2.19, Sen’s = 1.064, p-value = 0.029), E. faecium: increase of 1.4 percentage points per year (Z = 3.20, Sen’s = 1.379, p-value = 0.001)] and rifampin [E. faecalis: increase of 1.5 percentage points per year (Z = 2.52, Sen’s = 1.510, p-value = 0.011), E. faecium: increase of 2.3 percentage points per year (Z = 2.47, Sen’s = 2.300, p-value = 0.014)]. However, the MKT and Sen’s slope showed a significant decreasing temporal resistance trend to tetracyclines among both E. faecalis (decrease of 1.5 percentage points per year for tetracycline, Z = −2.08, Sen’s = −1.487, p-value = 0.038) and E. faecium (decrease of 7.1% points per year for doxycycline, Z = −2.55, Sen’s = −7.109, p-value = 0.011). Moreover, erythromycin resistance is decreasing over time among E. faecalis isolates (decrease of 1.4 percentage points per year, Z = −3.18, Sen’s = −1.436, p-value = 0.001; Figure 3).
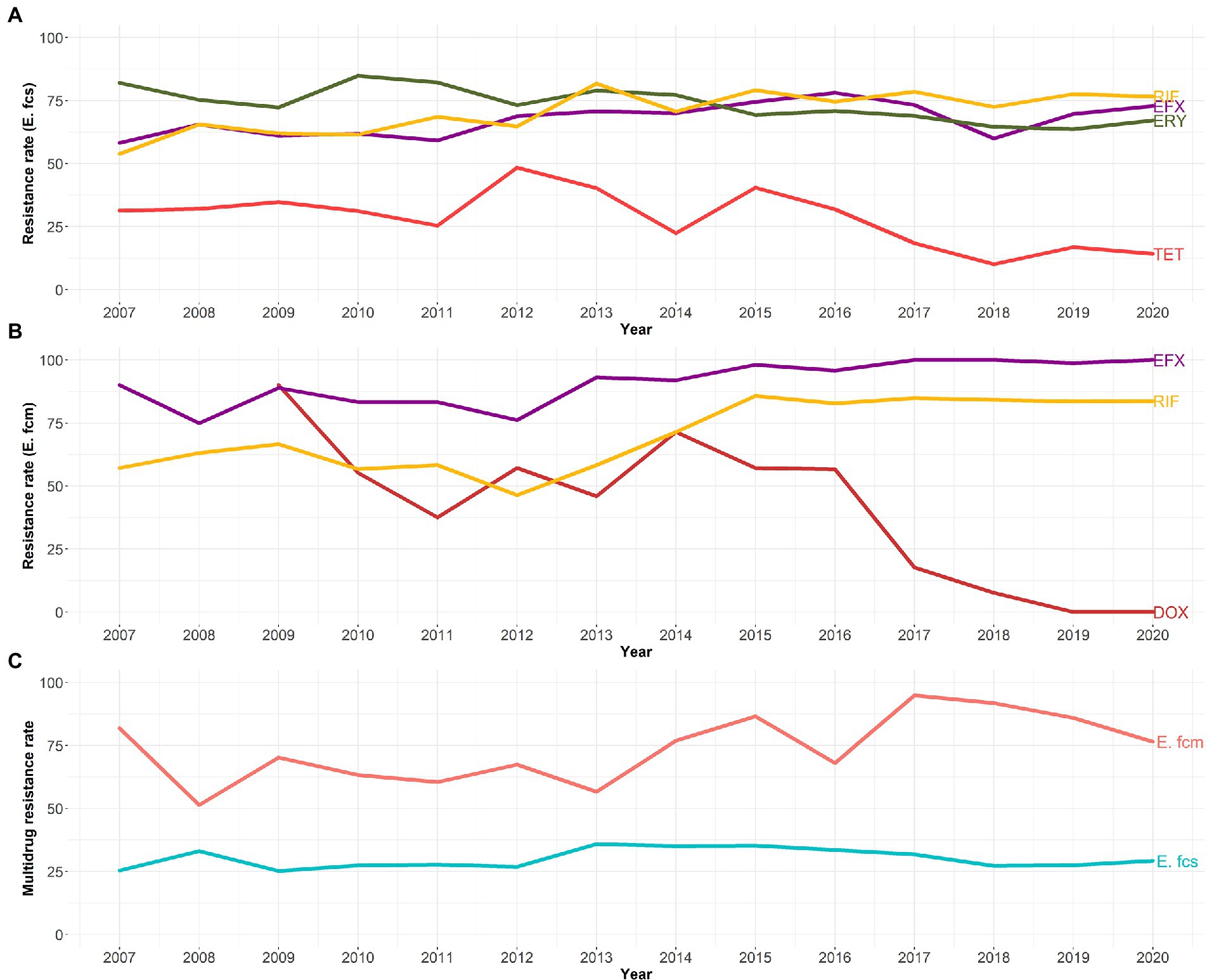
Figure 3. Temporal trends in the prevalence of resistance to tetracycline (TET), doxycycline (DOX), erythromycin, enrofloxacin (EFX), and/or rifampin (RIF; A, B), and multidrug resistance (C) among canine Enterococcus faecalis (E. fcs) and Enterococcus faecium (E. fcm) isolates during the study period (2007–2020), in canine clinical infections at the Cornell University Animal Health Diagnostic Center (AHDC), 2007–2020.
MLR analysis concurred with the MKT and Sen’s slope findings for both E. faecalis and E. faecium, identifying a decrease in resistance to tetracycline and increase in resistance to enrofloxacin and rifampin over time was observed after accounting for body site isolates (Table 3). Regarding E. faecium, MLR analysis has not only confirmed the MKT and Sen’s slope results, but also showed a significant increase in the level of resistance to ampicillin (OR = 2.18; 95% CI = 1.34 to 3.54; p = 0.007), penicillin (OR = 2.86; 95% CI = 1.57 to 5.26; p = 0.004), and erythromycin (OR = 4.28; 95% CI = 1.58 to 13.1; p = 0.023) among the circulating isolates in the 2018–2020 period compared to those isolated between 2007 and 2010. As for tetracycline, E. faecium isolates from 2018 to 2020 showed a lower resistance rate to doxycycline compared to peers isolated between 2007 and 2010 (OR = 0.02; 95% CI = 0.00 to 0.07; p < 0.001). Compared to the reference period (2007–2010), survival analysis models concurred with MLR findings among E. faecium isolates from 2018 to 2020. In addition, survival analysis confirmed MLR findings for rifampin, showing an increase in MIC values for this antimicrobial among E. faecalis isolated from 2018 to 2020 (HR = 0.69; CI = 0.57–0.84; p = 0.002), and also revealed an increase in MIC values for ampicillin (HR = 0.71; CI = 0.58–0.88; p = 0.011) and penicillin (HR = 0.58; CI = 0.46–0.74; p ≤ 0.001) and a decrease in MIC values for erythromycin (HR = 1.30; CI = 1.10–1.54; p = 0.017; Table 4).
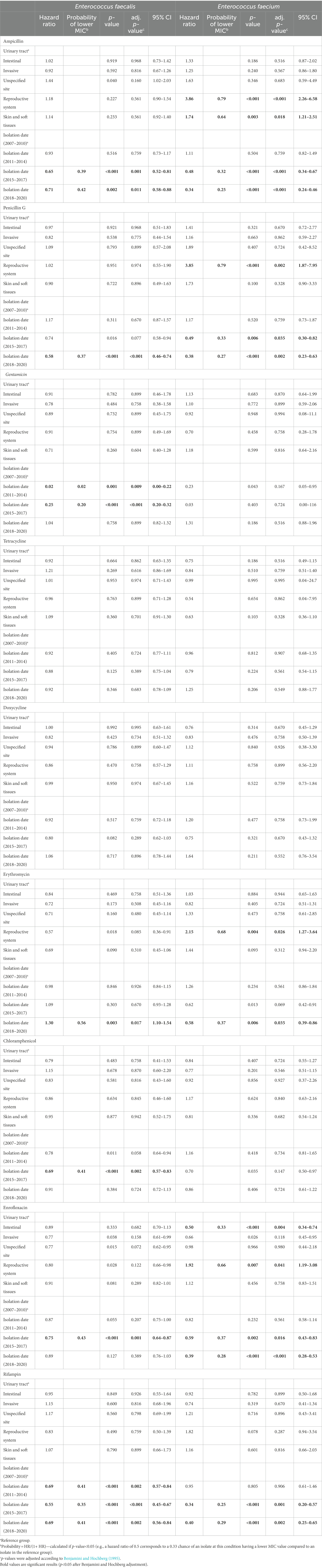
Table 4. Multivariable Cox proportional hazard regression model representing minimum inhibitory concentration trends of Enterococcus faecalis and Enterococcus faecium to different antimicrobials in this study, in canine clinical infections at the Cornell University Animal Health Diagnostic Center (AHDC), 2007–2020.
MLR models revealed several potential associations between drug resistances. Among all Enterococcus isolates, ampicillin was a strong predictor of erythromycin resistance (and vice versa; OR = 8.77; 95% CI = 3.85 to 25.3; p < 0.001), tetracycline (OR = 5.67; 95% CI = 2.99 to 10.8; p < 0.001) and enrofloxacin (OR = 21.1; 95% CI = 6.28 to 132; p < 0.001; Table 5). Tetracycline resistance was associated with resistance to erythromycin (OR = 2.98; 95% CI = 1.57 to 6.10; p = 0.004) and chloramphenicol (OR = 62.6; 95% CI = 19.5 to 282; p < 0.001), but was found to be associated with a decrease in the probability of resistance to enrofloxacin (OR = 0.30; 95% CI = 0.17 to 0.52; p < 0.001) and rifampin (OR = 0.31; 95% CI = 0.19 to 0.52; p < 0.001) among all Enterococcus spp. (and vice versa). Some species differences in associations between resistances were observed. Enrofloxacin-resistance among E. faecalis isolates was predicted by resistance to rifampin (OR = 2.82; 95% CI = 1.86 to 4.29; p < 0.001) and tetracycline (OR = 0.23; 95% CI = 0.12–0.45; p < 0.001) and enrofloxacin-resistant E. faecium was only predicted by resistance to erythromycin (OR = 26.4; 95% CI = 3.10 to 581; p = 0.015). Resistance to erythromycin is only associated with resistance to tetracycline among E. faecalis (OR = 3.35; 95% CI = 1.47 to 9.04; p = 0.015) and to enrofloxacin among E. faecium (OR = 17.5; 95% CI = 2.62 to 162; p = 0.010) isolates.
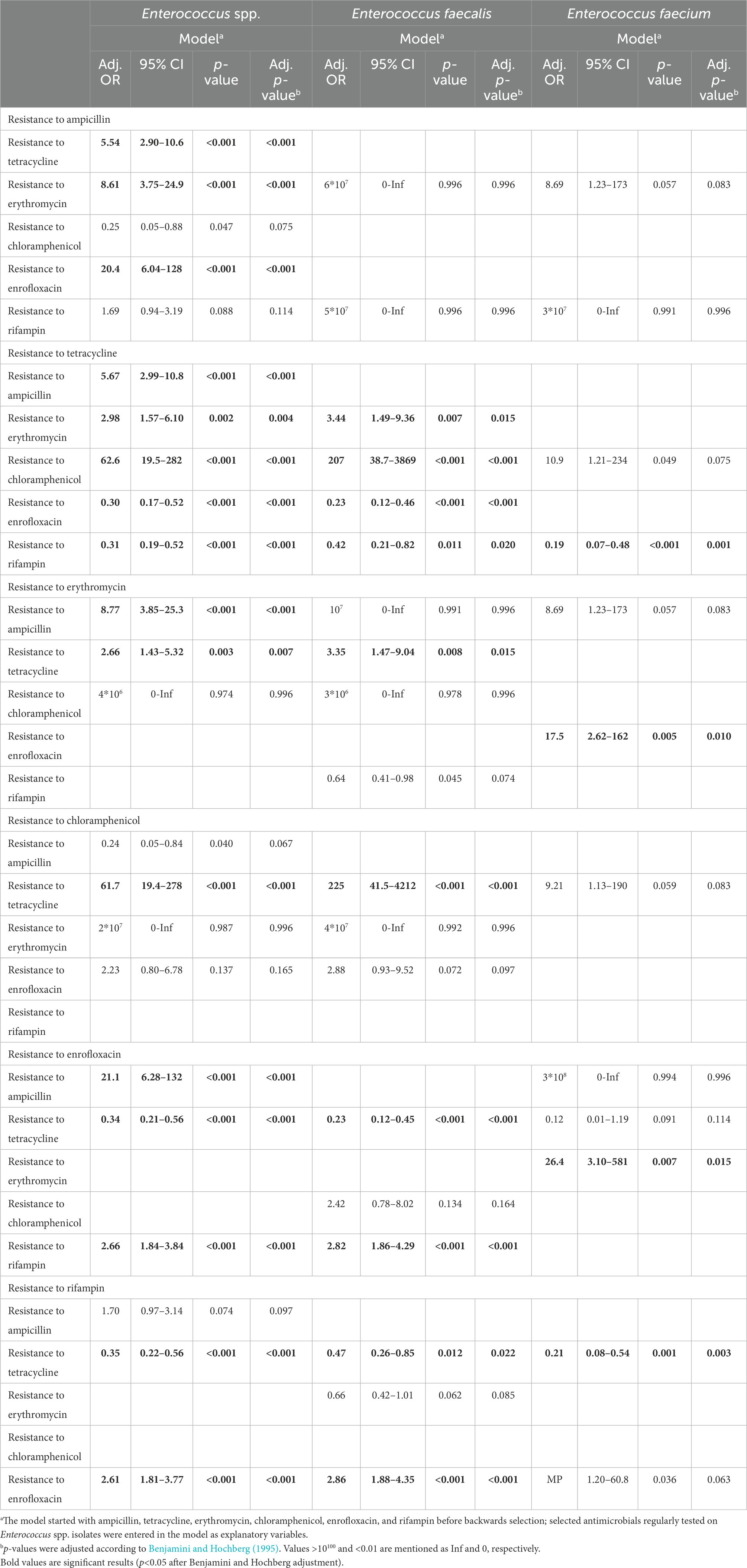
Table 5. Association between resistance to ampicillin, tetracycline, erythromycin, chloramphenicol, enrofloxacin, or rifampin and other antimicrobial compounds among Enterococcus spp. isolates using multivariable logistic regression models.
4. Discussion
The present study provided updated data on the most frequently isolated Enterococcus spp. from canine infections and their associated AMR patterns and trends in the northeastern United States. Antimicrobial-resistant enterococcal infections have become a major public health concern to modern health care, representing a growing global threat to human and animal health (Ahmed and Baptiste, 2018; Wada et al., 2021; Murray et al., 2022). Enterococcus faecalis was the most prevalent species (67.1%) encountered in dog enterococcal infections, followed by E. faecium (20.4%). This distribution is consistent with previous data showing that E. faecalis was the most commonly cultured enterococcal species (38%–77.4%) from dogs followed by E. faecium (12.9%–21%) in the United States (Jackson et al., 2009; KuKanich and Lubbers, 2015), as well as in other countries such as Spain (90.2 and 7.8%, respectively; Li et al., 2021) and Portugal (95.8% and 4.2%, respectively; Oliveira et al., 2016). However, an older study at the Michigan State University Veterinary Teaching Hospital performed between 1996 and 1998, including a low number of canine enterococcal isolates (N = 35), described a predominance of E. faecium (37.1%), followed by E. gallinarum (31.4%) and E. faecalis (20%; Simjee et al., 2002). E. gallinarum, which is a dominant bacterium in poultry gastrointestinal tracts, was rarely found in our study (0.8%). Similarly, E. casseliflavus was rarely reported (1%), and E. flavescens was not found. In contrast to previous data from dogs in Athens, Georgia, United States (Jackson et al., 2009) and Eastern Slovakia (Kubašová et al., 2017), the zoonotic pathogen commonly found in animals, E. hirae, was rarely observed (0.8%) in this study.
Due to limited therapeutic options, enterococci are hard to treat with antimicrobial agents, even when relatively susceptible isolates are involved. Although uncomplicated urinary infections are easily treated empirically with a first-line antimicrobial, typically a penicillin, cephalosporin, or folate-pathway antagonist (Weese et al., 2019), Enterococcus spp. possess inherent resistance to cephalosporins (e.g., cephalexin, cefazolin, cefovecin, cefpodoxime, ceftiofur) through the expression of low-affinity penicillin binding proteins (PBP4 in E. faecalis and PBP5 in E. faecium) that bind weakly to these antimicrobials (Hollenbeck and Rice, 2012). Enterococcus faecium isolates also possess an inherent resistance to penicillins and carbapenems. The activity of fluoroquinolones (e.g., enrofloxacin, pradofloxacin, orbifloxacin, marbofloxacin) in urine against enterococci is controversial, and the International Society for Companion Animal Infectious Diseases (ISCAID) recommended to avoid these drugs in the management of enterococcal urinary infections in dogs (de Lastours et al., 2017; Weese et al., 2019). Acquired resistance can also occur in enterococci through sporadic mutations or the acquisition of mobile genetic elements, complicating treatment of enterococcal infections (Oliveira et al., 2020).
Overall, alarming proportions of canine clinical enterococcal isolates were MDR. Compared to our findings, data from South Africa showed higher resistance rates to ampicillin (41.2%), penicillin (45.5%), and chloramphenicol (26.3%), but lower resistance rates against enrofloxacin among enterococci (58%; Oguttu et al., 2021). A Spanish study also revealed a lower percentage of resistance to enrofloxacin (~30%) and higher rates of resistance to chloramphenicol (~13%; Li et al., 2021). However, a recent Polish study described higher resistance rates to all the tested antimicrobials, with 92.2% to enrofloxacin, 90.2% to erythromycin, 88.2% to tetracycline, and 56.9% to chloramphenicol (Stępień-Pyśniak et al., 2021). Resistance rates among enterococci are directly related to the distribution of species (Hollenbeck and Rice, 2012; Mercuro et al., 2018; Zhou et al., 2020). Enterococcus faecium is recognized to have a higher prevalence of resistance to multiple antimicrobials of both clinical and veterinary significance, particularly beta-lactams, tetracycline, fluoroquinolones, and nitrofurantoin, while E. faecalis isolates are more likely to express virulence genes but retain a relatively lower prevalence of resistance to antimicrobials (Johnston and Jaykus, 2004; Zaheer et al., 2020); thus, the resistance rates among enterococci are typically higher in studies in which E. faecium has a relatively high prevalence rate. Interestingly, compared to previous studies performed in the United States (Simjee et al., 2002; Jackson et al., 2009; KuKanich and Lubbers, 2015), this study showed that E. faecalis isolates have higher resistance rates to enrofloxacin, erythromycin, and tetracycline. Regarding E. faecium, isolates from the previous studies showed similar percentage of resistance to penicillins, but higher resistance rates to enrofloxacin, erythromycin, tetracycline, and nitrofurantoin (Simjee et al., 2002; Jackson et al., 2009; KuKanich and Lubbers, 2015). The widespread resistance of enterococci to antimicrobials has without a doubt a substantial impact on the empirical and definitive antimicrobial use and spread of MDR bacteria in the United States.
Vancomycin-resistant enterococci (VRE) have become among the priority pathogens reported by the World Health Organization (Cassini et al., 2019). The CDC categorized VRE as serious threats to current healthcare practices, suggesting the need for increased monitoring and prevention activities (Weiner et al., 2016). Unlike recent data from the United States that described a shocking prevalence of vancomycin-resistant E. faecium nosocomial isolates in human medicine, ranging between 75 and 80% (Zhou et al., 2020), our findings showed a low percentage of vancomycin resistance among E. faecium isolates (0.8%), as well as E. faecalis isolates (0.3%). Higher proportion of vancomycin resistance (54%) was observed among other species, which can be explained by the predominance of E. gallinarum group isolates accounting for 67 out of 68 VRE other than E. faecalis and E. faecium (Table 1). The gallinarum group consisting of the species E. gallinarum, E. casseliflavus, and E. flavescens, possesses intrinsic low-level resistance to vancomycin by synthesis of modified peptidoglycan precursors ending in D-alanine-D-serine (via the vanC gene), but they are responsible only for a minor percentage of enterococcal infections (Monticelli et al., 2018). Overall, five enterococcal isolates with acquired vancomycin resistance were found, belonging to E. faecalis (N = 2), E. faecium (N = 2), and E. canintestini (N = 1) and mainly occurred in skin and soft tissues infections (Supplementary Table S2). All the vancomycin-resistant isolates had MDR patterns, and one was XDR. VRE infections, especially MDR and XDR strains, have become a global public health challenge in human and veterinary medicine involving both drug kinetics and bacterial resistance factors; these infections are often difficult-to-treat and may sometimes be life threatening because there are fewer antimicrobials that can fight these resistant bacteria (Patel and Gallagher, 2015; Zhou et al., 2020). Since vancomycin was not tested until 2017 in the Cornell University AHDC, the presence of VRE in the canine population is probably underestimated. However, interestingly, four out the five vancomycin-resistant isolates were isolated in 2020. To date, VRE remain rare in animals; thus, the recent detection of four resistant isolates in the same year represents an early warning sign on the dissemination of this serious threat between dogs and their environment (Jackson et al., 2009; KuKanich and Lubbers, 2015; Amachawadi et al., 2018; Dungan and Bjorneberg, 2021; Jeamsripong et al., 2021).
Although our MKT, Sen’s slope, MLR, and/or survival analysis models suggested that resistance to multiple antimicrobials such as penicillins, enrofloxacin, and rifampin in enterococci is increasing, resistance to tetracyclines is on a downward trajectory compared to AMR data from the last decade. The decrease in the frequency of use of tetracycline may be associated with the decrease in resistance to this antimicrobial class. Unlike penicillins and fluoroquinolones, which are the most commonly prescribed antimicrobial drug classes, tetracyclines are rarely prescribed at Cornell University Hospital for Animals emergency (6%) and critical care (0.8%) services (Robbins et al., 2020). These findings are similar to those recently reported in primary care and specialty practice across three academic veterinary hospitals (Cornell University, North Carolina State University, and Texas A&M University) in the United States (Goggs et al., 2021). Tetracyclines are not excreted in urine at high levels in the canine population and are therefore not recommended to treat urinary infections (Weese et al., 2019). Of note, current guidelines recommend the use of tetracyclines for the treatment of mild to moderate respiratory infections and fluoroquinolones for severe cases (Lappin et al., 2017).
Genetic co-resistance could play a crucial role in selecting resistant bacteria and promoting AMR. For example, our MLR model revealed that tetracycline is significantly associated with resistance to ampicillin, erythromycin, and chloramphenicol (Table 5). Tetracycline resistance is commonly associated with the presence of plasmid-borne tet genes, which confer ribosomal protection or efflux pumps. Moreover, erythromycin resistance is commonly mediated by the acquisition of erm (B) gene located mostly on plasmids, which encodes the ribosomal RNA methylase. All these genetic determinants can be located on the same mobile genetic element (Morroni et al., 2018; Cho et al., 2020), allowing the dissemination of resistance between bacteria in ecosystems (Hollenbeck and Rice, 2012). Dogs are in close contact with their environment; thus, the transmission of drug-resistant enterococci and AMR determinants can easily occur in either direction through direct or indirect contact (Rees et al., 2021). Taken together, we suggest that establishing better hygiene in communities and enhancing the prudent use of antimicrobials, particularly ampicillin, erythromycin and tetracycline, are essential to conserve their therapeutic effects and prevent the co-selection of resistance to other antimicrobials, and consequently tackle the burden of AMR in both human and veterinary settings (Devi, 2020; Charani et al., 2021).
5. Limitations of the study
Our dataset did not provide individual animal identifications, only sample submission identification. Although we included only one isolate per sample submission, there could be more than one isolate per patient in the analyzed data. It is important to note that interpretative criteria, specific to dogs, for resistance in enterococci are not available. Only human interpretive criteria are available from CLSI (CLSI, 2020). There is a dog-specific breakpoint for enrofloxacin and streptococci (both the veterinary antibiogram committee of the French Society for Microbiology (CA-SFM, 2021) and CLSI VAST provide the same breakpoint (CLSI, 2020)). We applied this streptococci breakpoint to the enterococci isolates. The antimicrobials interpreted with human breakpoints may not reflect the true prevalence of clinical resistance in dogs because of differences in human and canine antimicrobial pharmacokinetics. Enrofloxacin resistance may be underestimated or overestimated if the enterococci and streptococci have significantly different enrofloxacin pharmacodynamics. However, we expect trends within each antimicrobial to be reliable. Due to the retrospective design of this investigation, we were unable to assess the susceptibility of antimicrobials in all body sites, test other antimicrobials (particularly teicoplanin, daptomycin, linezolid, tedizolid, quinupristin/dalfopristin, fosfomycin, tigecycline, and eravacyline), or to collect more sociodemographic, behavioral, and clinical data that could be associated with resistance patterns. We have lower confidence in the trends of the less-prevalent Enterococcus species (i.e., not Enterococcus faecalis and not Enterococcus faecium) due to the smaller number of isolates. Vancomycin resistance was underestimated since this antibiotic was not tested until 2017 and was not tested in all isolates after that period. Furthermore, we were unable to perform additional phenotypic (e.g., nitrocefin test) and molecular (e.g., whole genome sequencing) analysis to confirm the initial species identification, determine the AMR determinants, and identify the Enterococcus clones circulating in the northeastern United States. Molecular typing is critical to better understand the current epidemiology of Enterococcus in humans and animals from a One Health approach and, therefore, to preserve the effectiveness of existing antimicrobials and reinforce antimicrobial stewardship interventions.
6. Conclusion
We provided a relevant update and an epidemiological evidence base for enterococci AMR patterns for veterinarians in the northeastern United States. Antimicrobial resistant canine enterococci, particularly vancomycin-resistant isolates, are a major public health threat to both human and veterinary medicine. Hence, the critical need to make rational prescribing decisions on the prudent use of antimicrobials and improve the quality of care for patients, especially when empirical antimicrobial treatment for enterococcal infection is common. To better understand the local epidemiology of drug-resistant enterococci and ensure effective treatment, further studies including a large number of human, animal, and environmental samples and aiming to assess other antimicrobials of clinical and veterinary interest, investigate the genetic determinants of AMR, identify the circulating clones, and suggest antimicrobial stewardship interventions are required.
Data availability statement
The datasets presented in this study can be found in online repositories. The names of the repository/repositories and accession number(s) can be found at: 10.5281/zenodo.7126369.
Author contributions
MO: conceptualization, methodology, software, formal analysis, validation, data curation, visualization, writing—original draft, and writing—review and editing. CA: investigation, resources, data curation, and writing—review and editing. CC: conceptualization, methodology, software, validation, resources, data curation, supervision, administration, and writing—review and editing. All authors contributed to the article and approved the submitted version.
Funding
MO is supported by the Atkinson Postdoctoral Fellowship (Cornell University).
Conflict of interest
The authors declare that the research was conducted in the absence of any commercial or financial relationships that could be construed as a potential conflict of interest.
Publisher’s note
All claims expressed in this article are solely those of the authors and do not necessarily represent those of their affiliated organizations, or those of the publisher, the editors and the reviewers. Any product that may be evaluated in this article, or claim that may be made by its manufacturer, is not guaranteed or endorsed by the publisher.
Supplementary material
The Supplementary material for this article can be found online at: https://www.frontiersin.org/articles/10.3389/fmicb.2022.1025242/full#supplementary-material
References
Ahmed, M. O., and Baptiste, K. E. (2018). Vancomycin-resistant enterococci: a review of antimicrobial resistance mechanisms and perspectives of human and animal health. Microb. Drug Resist. 24, 590–606. doi: 10.1089/mdr.2017.0147
Amachawadi, R. G., Giok, F., Shi, X., Soto, J., Narayanan, S. K., Tokach, M. D., et al. (2018). Antimicrobial resistance of Enterococcus faecium strains isolated from commercial probiotic products used in cattle and swine. J. Anim. Sci. 96, 912–920. doi: 10.1093/jas/sky056
Barlow, R. S., Mcmillan, K. E., Duffy, L. L., Fegan, N., Jordan, D., and Mellor, G. E. (2017). Antimicrobial resistance status of Enterococcus from Australian cattle populations at slaughter. PLoS One 12:e0177728. doi: 10.1371/journal.pone.0177728
Benjamini, Y., and Hochberg, Y. (1995). Controlling the false discovery rate: a practical and powerful approach to multiple testing. J. R. Stat. Soc. 57, 289–300. doi: 10.1111/j.2517-6161.1995.tb02031.x
Bourély, C., Cazeau, G., Jouy, E., Haenni, M., Madec, J.-Y., Jarrige, N., et al. (2019). Antimicrobial resistance of Pasteurella multocida isolated from diseased food-producing animals and pets. Vet. Microbiol. 235, 280–284. doi: 10.1016/j.vetmic.2019.07.017
CA-SFM. (2021). Recommandations 2021 du comité de l'antibiogramme de la Société Française de Microbiologie, Groupe de Travail Antibiogramme Vétérinaire. Available at: https://www.sfm-microbiologie.org/boutique/casfm-vet-2021/ (Accessed December 14, 2022).
Cassini, A., Högberg, L. D., Plachouras, D., Quattrocchi, A., Hoxha, A., Simonsen, G. S., et al. (2019). Attributable deaths and disability-adjusted life-years caused by infections with antibiotic-resistant bacteria in the EU and the European economic area in 2015: a population-level modelling analysis. Lancet Infect. Dis. 19, 56–66. doi: 10.1016/S1473-3099(18)30605-4
Charani, E., Mckee, M., Ahmad, R., Balasegaram, M., Bonaconsa, C., Merrett, G. B., et al. (2021). Optimising antimicrobial use in humans – review of current evidence and an interdisciplinary consensus on key priorities for research. Lancet Reg. Health Eur. 7:100161. doi: 10.1016/j.lanepe.2021.100161
Cho, S., Barrett, J. B., Frye, J. G., and Jackson, C. R. (2020). Antimicrobial resistance gene detection and plasmid typing among multidrug resistant enterococci isolated from freshwater environment. Microorganisms 8:1338. doi: 10.3390/microorganisms8091338
Chow, J. W. (2000). Aminoglycoside resistance in enterococci. Clin. Infect. Dis. 31, 586–589. doi: 10.1086/313949
CLSI. (2020). CLSI standard VET01—performance standards for antimicrobial disk and dilution susceptibility tests for bacteria isolated from animals. 5th CLSI, Wayne, PA.
Combescure, C., Perneger, T. V., Weber, D. C., Daurès, J.-P., and Foucher, Y. (2014). Prognostic ROC curves: a method for representing the overall discriminative capacity of binary markers with right-censored time-to-event endpoints. Epidemiology 25, 103–109. doi: 10.1097/EDE.0000000000000004
Cummings, K. J., Aprea, V. A., and Altier, C. (2015). Antimicrobial resistance trends among canine Escherichia coli isolates obtained from clinical samples in the northeastern USA, 2004-2011. Can. Vet. J. 56, 393–398.
Damborg, P., Broens, E. M., Chomel, B. B., Guenther, S., Pasmans, F., Wagenaar, J. A., et al. (2016). Bacterial zoonoses transmitted by household pets: state-of-the-art and future perspectives for targeted research and policy actions. J. Comp. Pathol. 155, S27–S40. doi: 10.1016/j.jcpa.2015.03.004
De Lastours, V., Maugy, E., Mathy, V., Chau, F., Rossi, B., Guérin, F., et al. (2017). Ecological impact of ciprofloxacin on commensal enterococci in healthy volunteers. J. Antimicrob. Chemother. 72, 1574–1580. doi: 10.1093/jac/dkx043
De Niederhäusern, S., Sabia, C., Messi, P., Guerrieri, E., Manicardi, G., and Bondi, M. (2004). Glycopeptide-resistance transferability from vancomycin-resistant enterococci of human and animal source to Listeria spp. Lett. Appl. Microbiol. 39, 483–489. doi: 10.1111/j.1472-765X.2004.01598.x
Devi, S. (2020). No time to lower the guard on AMR. Lancet Microbe 1:e198. doi: 10.1016/S2666-5247(20)30129-4
Dungan, R. S., and Bjorneberg, D. L. (2021). Antimicrobial resistance in Escherichia coli and enterococcal isolates from irrigation return flows in a high-desert watershed. Front. Microbiol. 12:660697. doi: 10.3389/fmicb.2021.660697
Francois Watkins, L. K., Laughlin, M. E., Joseph, L. A., Chen, J. C., Nichols, M., Basler, C., et al. (2021). Ongoing outbreak of extensively drug-resistant Campylobacter jejuni infections associated with US pet store puppies, 2016–2020. JAMA Netw. Open 4:e2125203. doi: 10.1001/jamanetworkopen.2021.25203
Goggs, R., Menard, J. M., Altier, C., Cummings, K. J., Jacob, M. E., Lalonde-Paul, D. F., et al. (2021). Patterns of antimicrobial drug use in veterinary primary care and specialty practice: a 6-year multi-institution study. J. Vet. Intern. Med. 35, 1496–1508. doi: 10.1111/jvim.16136
González-Zorn, B., and Courvalin, P. (2003). vanA-mediated high level glycopeptide resistance in MRSA. Lancet Infect. Dis. 3, 67–68. doi: 10.1016/S1473-3099(03)00510-3
Harada, K., Okada, E., Shimizu, T., Kataoka, Y., Sawada, T., and Takahashi, T. (2012). Antimicrobial resistance, virulence profiles, and phylogenetic groups of fecal Escherichia coli isolates: a comparative analysis between dogs and their owners in Japan. Comp. Immunol. Microbiol. Infect. Dis. 35, 139–144. doi: 10.1016/j.cimid.2011.12.005
Hata, A., Fujitani, N., Ono, F., and Yoshikawa, Y. (2022). Surveillance of antimicrobial-resistant Escherichia coli in sheltered dogs in the Kanto region of Japan. Sci. Rep. 12:773. doi: 10.1038/s41598-021-04435-w
Hollenbeck, B. L., and Rice, L. B. (2012). Intrinsic and acquired resistance mechanisms in Enterococcus. Virulence 3, 421–569. doi: 10.4161/viru.21282
Holmes, A. H., Moore, L. S. P., Sundsfjord, A., Steinbakk, M., Regmi, S., Karkey, A., et al. (2016). Understanding the mechanisms and drivers of antimicrobial resistance. Lancet 387, 176–187. doi: 10.1016/S0140-6736(15)00473-0
Jackson, C. R., Fedorka-Cray, P. J., Davis, J. A., Barrett, J. B., and Frye, J. G. (2009). Prevalence, species distribution and antimicrobial resistance of enterococci isolated from dogs and cats in the United States. J. Appl. Microbiol. 107, 1269–1278. doi: 10.1111/j.1365-2672.2009.04310.x
Jeamsripong, S., Li, X., Aly, S. S., Su, Z., Pereira, R. V., and Atwill, E. R. (2021). Antibiotic resistance genes and associated phenotypes in Escherichia coli and Enterococcus from cattle at different production stages on a dairy farm in Central California. Antibiotics (Basel) 10:1042. doi: 10.3390/antibiotics10091042
Johnson, A. P., and Woodford, N. (2002). Glycopeptide-resistant Staphylococcus aureus. J. Antimicrob. Chemother. 50, 621–623. doi: 10.1093/jac/dkf244
Johnston, L. M., and Jaykus, L.-A. (2004). Antimicrobial resistance of Enterococcus species isolated from produce. Appl. Environ. Microbiol. 70, 3133–3137. doi: 10.1128/AEM.70.5.3133-3137.2004
Joosten, P., Ceccarelli, D., Odent, E., Sarrazin, S., Graveland, H., Van Gompel, L., et al. (2020). Antimicrobial usage and resistance in companion animals: a cross-sectional study in three European countries. Antibiotics (Basel) 9:87. doi: 10.3390/antibiotics9020087
Komiyama, E. Y., Lepesqueur, L. S. S., Yassuda, C. G., Samaranayake, L. P., Parahitiyawa, N. B., Balducci, I., et al. (2016). Enterococcus species in the oral cavity: prevalence, virulence factors and antimicrobial susceptibility. PLoS One 11:e0163001. doi: 10.1371/journal.pone.0163001
Kubašová, I., Strompfová, V., and Lauková, A. (2017). Safety assessment of commensal enterococci from dogs. Folia Microbiol. (Praha) 62, 491–498. doi: 10.1007/s12223-017-0521-z
Kukanich, K. S., and Lubbers, B. V. (2015). Review of enterococci isolated from canine and feline urine specimens from 2006 to 2011. J. Am. Anim. Hosp. Assoc. 51, 148–154. doi: 10.5326/JAAHA-MS-6070
Lappin, M. R., Blondeau, J., Boothe, D., Breitschwerdt, E. B., Guardabassi, L., Lloyd, D. H., et al. (2017). Antimicrobial use guidelines for treatment of respiratory tract disease in dogs and cats: antimicrobial guidelines working group of the International Society for Companion Animal Infectious Diseases. J. Vet. Intern. Med. 31, 279–294. doi: 10.1111/jvim.14627
Leclercq, R., Derlot, E., Weber, M., Duval, J., and Courvalin, P. (1989). Transferable vancomycin and teicoplanin resistance in Enterococcus faecium. Antimicrob. Agents Chemother. 33, 10–15. doi: 10.1128/AAC.33.1.10
Leonard, E. K., Pearl, D. L., Finley, R. L., Janecko, N., Reid-Smith, R. J., Peregrine, A. S., et al. (2011). Comparison of antimicrobial resistance patterns of Salmonella spp. and Escherichia coli recovered from pet dogs from volunteer households in Ontario (2005–06). J. Antimicrob. Chemother. 67, 174–181. doi: 10.1093/jac/dkr430
Li, Y., Fernández, R., Durán, I., Molina-López, R. A., and Darwich, L. (2021). Antimicrobial resistance in bacteria isolated from cats and dogs from the Iberian peninsula. Front. Microbiol. 11:621597. doi: 10.3389/fmicb.2020.621597
Llor, C., and Bjerrum, L. (2014). Antimicrobial resistance: risk associated with antibiotic overuse and initiatives to reduce the problem. Ther. Adv. Drug Saf. 5, 229–241. doi: 10.1177/2042098614554919
Magiorakos, A. P., Srinivasan, A., Carey, R. B., Carmeli, Y., Falagas, M. E., Giske, C. G., et al. (2012). Multidrug-resistant, extensively drug-resistant and pandrug-resistant bacteria: an international expert proposal for interim standard definitions for acquired resistance. Clin. Microbiol. Infect. 18, 268–281. doi: 10.1111/j.1469-0691.2011.03570.x
Mercuro, N. J., Davis, S. L., Zervos, M. J., and Herc, E. S. (2018). Combatting resistant enterococcal infections: a pharmacotherapy review. Expert. Opin. Pharmacother. 19, 979–992. doi: 10.1080/14656566.2018.1479397
Monticelli, J., Knezevich, A., Luzzati, R., and Di Bella, S. (2018). Clinical management of non-faecium non-faecalis vancomycin-resistant enterococci infection. Focus on Enterococcus gallinarum and Enterococcus casseliflavus/flavescens. J. Infect. Chemother. 24, 237–246. doi: 10.1016/j.jiac.2018.01.001
Morroni, G., Brenciani, A., Antonelli, A., Maria D’andrea, M., Di Pilato, V., Fioriti,, et al. (2018). Characterization of a multiresistance plasmid carrying the optrA and cfr resistance genes from an Enterococcus faecium clinical isolate. Front. Microbiol. 9:2189. doi: 10.3389/fmicb.2018.02189
Murray, C. J. L., Ikuta, K. S., Sharara, F., Swetschinski, L., Robles Aguilar, G., Gray, A., et al. (2022). Global burden of bacterial antimicrobial resistance in 2019: a systematic analysis. Lancet 399, 629–655. doi: 10.1016/S0140-6736(21)02724-0
Oguttu, J. W., Qekwana, D. N., and Odoi, A. (2021). Prevalence and predictors of antimicrobial resistance among Enterococcus spp. from dogs presented at a veterinary teaching hospital, South Africa. Front. Vet. Sci. 7:589439. doi: 10.3389/fvets.2020.589439
Oliveira, D. M. P. D., Forde, B. M., Kidd, T. J., Harris, P. N. A., Schembri, M. A., Beatson, S. A., et al. (2020). Antimicrobial resistance in ESKAPE pathogens. Clin. Microbiol. Rev. 33:e00181-19. doi: 10.1128/CMR.00181-19
Oliveira, M., Tavares, M., Gomes, D., Touret, T., São Braz, B., Tavares, L., et al. (2016). Virulence traits and antibiotic resistance among enterococci isolated from dogs with periodontal disease. Comp. Immunol. Microbiol. Infect. Dis. 46, 27–31. doi: 10.1016/j.cimid.2016.04.002
Osman, M., Albarracin, B., Altier, C., Gröhn, Y. T., and Cazer, C. (2022). Antimicrobial resistance trends among canine Escherichia coli isolated at a New York veterinary diagnostic laboratory between 2007 and 2020. Prev. Vet. Med. 208:105767. doi: 10.1016/j.prevetmed.2022.105767
Overgaauw, P. A. M., Vinke, C. M., Hagen, M., and Lipman, L. J. A. (2020). A one health perspective on the human-companion animal relationship with emphasis on zoonotic aspects. Int. J. Environ. Res. Public Health 17:3789. doi: 10.3390/ijerph17113789
Papich, M. G. (2021). Antimicrobial agent use in small animals what are the prescribing practices, use of PK-PD principles, and extralabel use in the United States? J. Vet. Pharmacol. Ther. 44, 238–249. doi: 10.1111/jvp.12921
Patel, R., and Gallagher, J. C. (2015). Vancomycin-resistant enterococcal bacteremia pharmacotherapy. Ann. Pharmacother. 49, 69–85. doi: 10.1177/1060028014556879
Ramos, S., Silva, V., Dapkevicius, M. D. L. E., Igrejas, G., and Poeta, P. (2020). Enterococci, from harmless bacteria to a pathogen. Microorganisms 8:1118. doi: 10.3390/microorganisms8081118
Rees, E. M., Minter, A., Edmunds, W. J., Lau, C. L., Kucharski, A. J., and Lowe, R. (2021). Transmission modelling of environmentally persistent zoonotic diseases: a systematic review. Lancet Planet. Health 5, e466–e478. doi: 10.1016/S2542-5196(21)00137-6
Robbins, S. N., Goggs, R., Lhermie, G., Lalonde-Paul, D. F., and Menard, J. (2020). Antimicrobial prescribing practices in small animal emergency and critical care. Front. Vet. Sci. 7:110. doi: 10.3389/fvets.2020.00110
Simjee, S., White, D. G., Mcdermott, P. F., Wagner, D. D., Zervos, M. J., Donabedian,, et al. (2002). Characterization of Tn1546 in vancomycin-resistant Enterococcus faecium isolated from canine urinary tract infections: evidence of gene exchange between human and animal enterococci. J. Clin. Microbiol. 40, 4659–4665. doi: 10.1128/JCM.40.12.4659-4665.2002
Spruance, S. L., Reid, J. E., Grace, M., and Samore, M. (2004). Hazard ratio in clinical trials. Antimicrob. Agents Chemother. 48, 2787–2792. doi: 10.1128/AAC.48.8.2787-2792.2004
Stępień-Pyśniak, D., Bertelloni, F., Dec, M., Cagnoli, G., Pietras-Ożga, D., Urban-Chmiel, R., et al. (2021). Characterization and comparison of Enterococcus spp. isolates from feces of healthy dogs and urine of dogs with UTIs. Animals (Basel) 11:2845. doi: 10.3390/ani11102845
Švec, P., and Devriese, L. A. (2015). “Enterococcus” in Bergey’s Manual of Systematics of Archaea and Bacteria. eds. W. B. Whitman, F. Rainey, P. Kämpfer, M. Trujillo, J. Chun, P. DeVos, et al. (England: John Wiley & Sons, Ltd, Chichester), 1–25.
Sykes, J. E. (2014). “Chapter 34—streptococcal and enterococcal infections” in Canine and Feline Infectious Diseases. ed. J. E. Sykes (Saint Louis, MO: W.B. Saunders), 334–346.
Tóth, A.G., Tóth, I., Rózsa, B., Kovács, E.G., Dubecz, A., Patai, Á., et al. (2022). Canine saliva is a source of interspecies antimicrobial resistance gene transfer bioRxiv [Preprint].
Tyne, D. V., Manson, A. L., Huycke, M. M., Karanicolas, J., Earl, A. M., and Gilmore, M. S. (2019). Impact of antibiotic treatment and host innate immune pressure on enterococcal adaptation in the human bloodstream. Sci. Transl. Med. 11:eaat8418. doi: 10.1126/scitranslmed.aat8418
Wada, Y., Irekeola, A. A., Engku, A. R. E. N. S., Yusof, W., Lih Huey, L., Ladan Muhammad, S., et al. (2021). Prevalence of vancomycin-resistant enterococcus (VRE) in companion animals: the first meta-analysis and systematic review. Antibiotics (Basel) 10:138. doi: 10.3390/antibiotics10020138
Weese, J. S., Blondeau, J., Boothe, D., Guardabassi, L. G., Gumley, N., Papich, M., et al. (2019). International Society for Companion Animal Infectious Diseases (ISCAID) guidelines for the diagnosis and management of bacterial urinary tract infections in dogs and cats. Vet. J. 247, 8–25. doi: 10.1016/j.tvjl.2019.02.008
Weiner, L. M., Fridkin, S. K., Aponte-Torres, Z., Avery, L., Coffin, N., Dudeck, M. A., et al. (2016). Vital signs: preventing antibiotic-resistant infections in hospitals - United States, 2014. MMWR Morb. Mortal. Wkly Rep. 65, 235–241. doi: 10.15585/mmwr.mm6509e1
Weinstein, M. P., and Lewis, J. S. 2nd (2020). The clinical and laboratory standards institute subcommittee on antimicrobial susceptibility testing: background, organization, functions, and processes. J. Clin. Microbiol. 58, e01864–e01819. doi: 10.1128/JCM.01864-19
Wisell, K. T., Kahlmeter, G., and Giske, C. G. (2008). Trimethoprim and enterococci in urinary tract infections: new perspectives on an old issue. J. Antimicrob. Chemother. 62, 35–40. doi: 10.1093/jac/dkn147
Zaheer, R., Cook, S. R., Barbieri, R., Goji, N., Cameron, A., Petkau, A., et al. (2020). Surveillance of enterococcus spp. reveals distinct species and antimicrobial resistance diversity across a one-health continuum. Sci. Rep. 10:3937. doi: 10.1038/s41598-020-61002-5
Zhang, X.-F., Doi, Y., Huang, X., Li, H.-Y., Zhong, L.-L., Zeng, K.-J., et al. (2016). Possible transmission of mcr-1-harboring Escherichia coli between companion animals and human. Emerg. Infect. Dis. 22, 1679–1681. doi: 10.3201/eid2209.160464
Keywords: Enterococcus spp., antimicrobial resistance, epidemiology, canine, surveillance, temporal trends
Citation: Osman M, Altier C and Cazer C (2023) Antimicrobial resistance among canine enterococci in the northeastern United States, 2007–2020. Front. Microbiol. 13:1025242. doi: 10.3389/fmicb.2022.1025242
Edited by:
Santi M. Mandal, Indian Institute of Technology Kharagpur, IndiaReviewed by:
Ahmad Al Atrouni, Lebanese University, LebanonBabafela Awosile, Texas Tech University, United States
Brian V. Lubbers, Kansas State University, United States
Copyright © 2023 Osman, Altier and Cazer. This is an open-access article distributed under the terms of the Creative Commons Attribution License (CC BY). The use, distribution or reproduction in other forums is permitted, provided the original author(s) and the copyright owner(s) are credited and that the original publication in this journal is cited, in accordance with accepted academic practice. No use, distribution or reproduction is permitted which does not comply with these terms.
*Correspondence: Marwan Osman, ✉ bW8zNjhAY29ybmVsbC5lZHU=