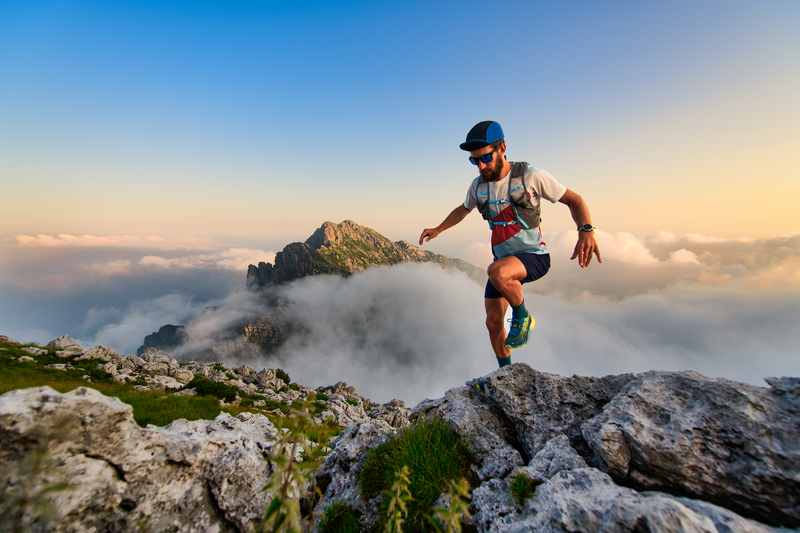
95% of researchers rate our articles as excellent or good
Learn more about the work of our research integrity team to safeguard the quality of each article we publish.
Find out more
ORIGINAL RESEARCH article
Front. Microbiol. , 04 October 2022
Sec. Microorganisms in Vertebrate Digestive Systems
Volume 13 - 2022 | https://doi.org/10.3389/fmicb.2022.1017804
This article is part of the Research Topic The Potential Role of Gut Microbiome in Animal Gut-Linked Diseases View all 16 articles
Slow transit constipation (STC) is the most common type of functional constipation. Drugs with good effects and few side effects are urgently needed form the treatment of STC. Cymbopogon citratus (DC.) Stapf (CC) is an important medicinal and edible spice plant. The wide range of biological activities suggested that CC may have laxative effects, but thus far, it has not been reported. In this study, the loperamide-induced STC mouse model was used to evaluate the laxative effect of the aqueous extract of CC (CCAE), and the laxative mechanism was systematically explored from the perspectives of the enteric nervous system (ENS), neurotransmitter secretion, gastrointestinal motility factors, intestinal inflammation, gut barrier and gut microbiota. The results showed that CCAE not only decreased the serum vasoactive intestinal polypeptide (VIP), induced nitric oxide synthases (iNOS), and acetylcholinesterase (AchE) in STC mice but also increased the expression of gastrointestinal motility factors in colonic interstitial cells of Cajal (ICCs) and smooth muscle cells (SMCs), thereby significantly shortening the defecation time and improving the gastrointestinal transit rate. The significantly affected gastrointestinal motility factors included stem cell factor receptor (c-Kit), stem cell factor (SCF), anoctamin 1 (Ano1), ryanodine receptor 3 (RyR3), smooth muscle myosin light chain kinase (smMLCK) and Connexin 43 (Cx43). Meanwhile, CCAE could repair loperamide-induced intestinal inflammation and intestinal barrier damage by reducing the expression of the pro-inflammatory factor IL-1β and increasing the expression of the anti-inflammatory factor IL-10, chemical barrier (Muc-2) and mechanical barrier (Cldn4, Cldn12, Occludin, ZO-1, and ZO-2). Interestingly, CCAE could also partially restore loperamide-induced gut microbial dysbiosis in various aspects, such as microbial diversity, community structure and species composition. Importantly, we established a complex but clear network between gut microbiota and host parameters. Muribaculaceae, Lachnospiraceae and UCG-010 showed the most interesting associations with the laxative phenotypes; several other specific taxa showed significant associations with serum neurotransmitters, gastrointestinal motility factors, intestinal inflammation, and the gut barrier. These findings suggested that CCAE might promote intestinal motility by modulating the ENS-ICCs-SMCs network, intestinal inflammation, intestinal barrier and gut microbiota. CC may be an effective and safe therapeutic choice for STC.
Graphical Abstract. CCAE might promote intestinal motility by modulating the ENS-ICCs-SMCs network, intestinal inflammation, intestinal barrier, and gut microbiota.
In recent years, due to changes in the human diet and lifestyle, constipation has become increasingly common and has gradually reduced people’s quality of life (Bharucha and Lacy, 2020). Constipation can be roughly divided into secondary constipation and functional constipation. Slow transit constipation (STC) is the most common type of functional constipation. STC is characterized by prolonged colonic transit time and reduced colonic high amplitude propulsion and contraction after eating, and the main symptoms are dry stool, difficulty in defecation, and a decrease in stool weight and frequency. At present, most scholars believe that the pathophysiological mechanism of STC may be related to nervous system diseases, abnormal hormone levels in vivo, smooth muscle dysfunction, abnormal interstitial cells of Cajal (ICCs), and gut microbiota imbalance.
Medications are still the main method for the treatment of STC, and they can be divided into Western medicine, traditional Chinese medicine and microbial drugs. Mainstream Western medicine therapies represented by various laxatives, prokinetic drugs and secretagogues often fail or have only short-term efficacy and induce side effects (Vriesman et al., 2020). For the treatment of STC, drugs with good effects, few side effects and clearly defined functional components are urgently needed. An innovative and efficacious drug for the treatment of STC may be found in food and natural drug resources.
Cymbopogon citratus (DC.) Stapf (CC) is a spice commonly used in soups and grills in Asian countries such as China, India, Thailand, Singapore, Sri Lanka and Vietnam. CC is also a traditional Chinese medicinal plant. Ancient books in China record its effects of dredging wind and collaterals, reducing swelling and pain, and gastric ventilation (Li et al., 2020). Recent scientific studies have shown that CC has antibacterial (Iram et al., 2019), antioxidant (Tiwari et al., 2010), anti-inflammatory (Figueirinha et al., 2010), anti-anxiety (Mendes Hacke et al., 2020) and antidepressant (Umukoro et al., 2017) activities, and it is beneficial in the treatment of diabetes (Borges et al., 2021), liver damage (Uchida et al., 2017), and even cancers (Rojas-Armas et al., 2020; Gomes et al., 2021; Pan et al., 2022).
Although CC has a variety of biological activities, its protective effect on the gastrointestinal tract is the most noteworthy. Volatile CC oil can effectively alleviate gastric ulcers in mice induced by absolute ethanol and aspirin (Fernandes et al., 2012; Venzon et al., 2018) and shows relatively effective inhibition of acetylcholinesterase activity (Madi et al., 2021). CC is also popular as a lemongrass tea in North and South America, West Africa and other countries and can be used to aid digestion (Kieling and Prudencio, 2019), which indicates that the water-soluble part of CC also has good gastrointestinal regulatory function. The research progress of CC in gastrointestinal regulatory activities suggests that it may be effective in relieving constipation. However, whether CC can alleviate constipation and how CC alleviates constipation is still unclear. To this end, the loperamide-induced STC mouse model was used to evaluate the laxative effect of the aqueous extract of CC, and the mechanism was systematically explored from the perspectives of the enteric nervous system (ENS), neurotransmitter secretion, gastrointestinal motility factors, gut barrier, intestinal inflammation and gut microbiota in this study.
Ultramicro-powder of CC leaves was obtained from a local company in Nujiang City, Yunnan Province (China). Then, 200 g ultramicro-powder was boiled for 3 min in 2 L ultrapure water (pH = 6.8). After cooling to room temperature, the extraction solution was immediately centrifuged at 5,000 rpm for 5 min. The precipitates were collected twice under the same conditions and then discarded. All the supernatants were combined and dried in vacuum freeze-drying equipment for 2–3 days. The final product was greenish brown in color, with a yield of 17.6%. The dried extract was weighed and dissolved in distilled water just before administration to experimental animals. The main nutritional components and phytochemical composition of CC aqueous extract (CCAE) were determined by various methods. The methods are described briefly in Supplementary Table 1.
Six-week-old male KM mice (20–25 g) were purchased from Chengdu Dossy Experimental Animals Co., Ltd., China. All mice were housed in specific pathogen-free barrier conditions (24 ± 1°C, 30–50% humidity, 12-h daylight cycle, lights off at 20:00) with a normal chow diet (10.8% fat, 68.7% carbohydrates, and 20.5% protein, according to caloric intake) and water ad libitum. After 7 days of acclimation, the mice were divided into six groups of 12 mice according to their body weights. Three mice were housed together in each cage, and the weight, food intake, and water consumption of the mice were recorded every day. Importantly, 6 mg/kg⋅bw loperamide (LOP, mg/kg⋅body weight, Sigma) was used to generate the STC mouse model.
The groups were designed as follows: CON group (control group, received saline solution as vehicle), LOP group (model group, received loperamide), POS group (positive control group, received 900 mg/kg⋅bw Maren pill from Beijing Tongrentang Pharmaceutical Co., Ltd, Beijing, China), LCC group (received loperamide and 300 mg/kg⋅bw CCAE, low dosage), MCC group (received loperamide and 600 mg/kg⋅bw CCAE, medium dosage) and HCC (received loperamide and 900 mg/kg⋅bw CCAE, high dosage). STC model mice were induced by administration of LOP for 8 days, and at the same time, the mice were gavaged with daily oral doses of 300 μL of solutions designated by their experimental group assignment. Required doses of the Maren pill and CCAE were dissolved in 300 μL of saline solution (Figure 1A). All mice were deprived of food but not water overnight before the defecation test and gastrointestinal transit test. All procedures were previously approved by Animal Ethics Committee of Yunnan Agriculture University.
Figure 1. Influences of CCAE on loperamide-induced constipation symptoms in mice. (A) Grouping and basic workflow of animal experiment. (B) The defecation time of the first black stool, FBS. (C) Number of feces excreted in 6 h, FN. (D) Wet weight of feces excreted in 6 h, FW. (E) Fecal water content. (F) Representative fecal morphology of each group. (G) Gastrointestinal transit rate, GTR. (H) Liver index. (I) Kidney index. (J) Ink advancing distance and intestinal length. The data are expressed as the means ± SEMs (n = 10-12). *, Compared with the CON group; #, compared with the LOP group. **P < 0.01, ***P < 0.001. #P < 0.05, ##P < 0.01, ###P < 0.001.
At 08:00 on the seventh day of the animal experiment, mice were given the normal dose of the drugs by gavage, the homemade ink (300 μL) was given by gavage 30 min later, and the defecation experiment of mice was officially started. The defecation time of the first black stool (FBS) of each mouse was recorded carefully, and the fecal wet weight (FW), fecal number (FN) and water content of stools were also analyzed for each mouse for 6 h from the start of the defecation test to evaluate the laxative effect.
At 08:00 on the eighth day of the animal experiment, mice were administered the normal dose of the drugs. Thirty minutes later, the ink (300 μL) was given to each mouse. After 20 min, the mice were sacrificed quickly in a chamber saturated with CO2, the abdominal cavity was opened, and the blood was collected from the abdominal aorta. The mesentery of each gastrointestinal tract was carefully stripped, and then the length of the whole small intestine and the length marked by the ink were measured to calculate the gastrointestinal transit rate (GTR).
Meanwhile, the distal ileum, cecum, and proximal colon were accurately dissected from each mouse. The contents of the ileum and colon segments were thoroughly flushed with cold PBS to remove feces. Ceca contents were washed from the cecum in a 2-mL Eppendorf tube containing 1.0 mL cold Milli-Q water. The liver, kidney, and cecum tissues were weighed. Cleaned tissues were subsequently placed in individual cryogenic tubes. Tissues and ceca contents were all flash-frozen in liquid nitrogen and stored at −80°C until analysis.
After the mice were sacrificed, blood was collected immediately and incubated at 37°C for 30 min and centrifuged at 4°C at 3,500 rpm for 10 min, and serum was collected. The contents of neurotransmitters, including vasoactive intestinal polypeptide (VIP), induced nitric oxide synthases (iNOS), acetylcholinesterase (AchE), and serotonin (5-HT), in the serum of mice were determined by using an ultrasensitive enzyme-linked immunosorbent assay (ELISA) kit (Cusabio, China).
FastPure Cell/Tissue Total RNA Isolation Kits (RC101-01, Vazyme, China) were used for the total RNA extraction from mouse tissues. HiScript III RT SuperMix for qPCR (plus gDNA wiper, R323-01, Vazyme, China) was used for RNA reverse transcription, and ChamQ Universal SYBR qPCR Master Mix (Q711-02, Vazyme, China) was used for the quantitative PCR analysis of gene expression. The relative amount of the target mRNA was normalized to the RPL-19 level, and the results were calculated by the 2–ΔΔCt method. The detailed method has been described previously (Gao et al., 2017). The primer sequences are presented in Supplementary Table 2.
After the mice of each group were sacrificed, the proximal colon was collected immediately, fixed with 10% formalin, embedded in paraffin, sectioned to a thickness of 5 mm, deparaffinized and submitted to hematoxylin and eosin (H&E, Sigma-Aldrich, Shanghai, China) staining. Immunohistochemistry was performed using a previously described method (Huang et al., 2019). Briefly, 3 μm sections were deparaffinized in xylene and rehydrated in graded alcohol. After quenching endogenous peroxidase activity and blocking non-specific binding, the sections were incubated with a rabbit monoclonal antibody [EPR22566-344] against c-Kit (Abcam, ab256345) overnight at 4°C and then incubated with the secondary antibody goat anti-rabbit IgG (H + L) HRP (ab0101, Abways) at room temperature for 30 min. Finally, the slides were incubated with reagents from the Avidin-Biotin Complex Kit (Vector Laboratories, Inc., Burlingame, USA) and a 3,3′-diaminobenzidine kit (Tiangen, China) according to the manufacturer’s instructions. Images were captured with a Nikon Ci-S microscope and Nikon DS-U3 imaging system. The proportion of c-Kit-positive cells in the colonic muscle layer was determined using an image analyzer (Image-Pro Plus 6.0, Media Cybernetics, Inc., Rockville, USA).
After the mice of each group were sacrificed, the contents of the cecum were collected immediately, and the total microbial genomic DNA was extracted by the E.Z.N.A.® soil DNA Kit (Omega Bio-Tek, Norcross, GA, USA) according to the manufacturer’s instructions. Agarose gel electrophoresis (1.0%) and a NanoDrop® ND-2000 spectrophotometer (Thermo Scientific Inc., Shanghai, United States) were used to check the quality and concentration of DNA. Qualified DNA was kept at −80°C until further use. The hypervariable region V3–V4 of the bacterial 16S rRNA gene was amplified with the primer pairs 338F (5′-ACTCCTACGGGAGGCAGCAG-3′) and 806R (5′-GGACTACHVGGGTWTCTAAT-3′) by an ABI GeneAmp® 9700 PCR thermocycler (ABI, Arlington, USA). The PCR conditions and the purification of the PCR products were performed using a previously described method (Gao et al., 2020).
According to the standard protocols by Majorbio Bio-Pharm Technology Co. Ltd. (Shanghai, China), purified amplicons were pooled in equimolar amounts and paired-end sequenced on an Illumina MiSeq PE300 platform (Illumina, San Diego, CA, United States). The raw sequencing reads were deposited into the NCBI Sequence Read Archive database.
After demultiplexing, the resulting sequences were quality filtered with Fastp and merged with FLASH. Then, the high-quality sequences were de-noised using DADA2 (plugin in the QIIME2 pipeline with recommended parameters), which obtains single nucleotide resolution based on error profiles within samples. DADA2 de-noised sequences are usually called amplicon sequence variants (ASVs). To minimize the effects of sequencing depth on alpha and beta diversity measurements, the number of sequences from each sample was rarefied to 20,000, which still yielded an average Good’s coverage of 97.90%. Taxonomic assignment of ASVs was performed using the Naive Bayes consensus taxonomy classifier implemented in QIIME2 and the SILVA 16S rRNA database.
The Majorbio Cloud platform1 was used to analyze the gut microbiota. Based on the ASV information, rarefaction curves and alpha diversity indices, including observed ASVs, were calculated with Mothur v1.30.1. The similarity among the microbial communities in different samples was determined by principal coordinate analysis (PCoA) based on Bray-Curtis dissimilarity using the Vegan v2.5-3 package. Linear discriminant analysis (LDA) effect size (LEfSe)2 was performed to identify the significantly abundant taxa (phylum to genera) of bacteria among the different groups (LDA score > 2.0, P < 0.05).
The data are expressed as the means ± standard errors of the means (SEMs). The unpaired two-tailed Student’s t-test was performed to analyze two independent groups. Bivariate correlations were calculated using Spearman’s r coefficients. Heatmaps were constructed using HemI 1.0 software3. Unless otherwise specified in the figure legends, the results were considered statistically significant at a P-value of < 0.05.
The contents of the main nutritional components are shown in Supplementary Table 1. The macronutrients included protein (8.54%), carbohydrate (60.10%), fat (1.99%), water (4.06%), dietary fiber (11.79%), and crude polysaccharide (0.65%). The ash, total acid, and sodium contents were 13.52, 0.26, and 0.11%, respectively.
The phytochemical compositions of CCAE were also examined by widely targeted metabolomics (HPLC-QQQ-MS/MS). As shown in Supplementary Table 3, among the top ten chemical compound categories found in CCAE, flavonoids showed the highest relative abundance, accounting for 24.30%. Other compounds that accounted for more than 10% of the total were nucleotides (19.66%), amino acids (15.81%), organ oxygen compounds and carboxylic acids (14.85%), and alkaloids (13.51%).
There were 23 compounds with a relative abundance of more than 1% (Supplementary Table 4). Unexpectedly, the compound with the highest abundance belonged to the alkaloid betaine (13.59%). The compounds that accounted for more than 5% of the total are pyrrolidonecarboxylic acid (9.91%), vidarabine (7.45%), proline (6.50%), and homoorientin (5.74%).
During the experiments, all mice appeared healthy and showed no abnormal behaviors. Loperamide and CCAE treatment did not show an obvious influence on body weight, food intake, or water consumption (Supplementary Figure 1). Compared with the CON group, FBS in the LOP group was significantly increased, and CCAE treatment shortened the time (Figure 1B). In addition, FW and FN in the LOP group were significantly reduced, and CCAE treatment significantly reversed these characteristics of feces (Figures 1C,D). However, CCAE did not increase the water content of the feces significantly (Figure 1E).
In the gastrointestinal transit test, the medium and high doses of CCAE (MCC and HCC groups) significantly reduced the symptoms of lower GTR induced by loperamide in mice (Figure 1G). Notably, CCAE reversed the decrease in liver and kidney indices induced by loperamide (Figures 1H,I). In general, CCAE not only alleviated a series of STC symptoms but also reversed the possible negative effects on mouse organs caused by loperamide. CCAE showed a mild and good laxative effect, which was comparable to the effect of the traditional Chinese drug Maren pill.
We investigated whether loperamide-induced defecation delay were accompanied by alterations in the molecular regulators for STC, including iNOS, AchE, VIP, and 5-HT, because they are associated with the proliferation of interstitial cells of Cajal and gastrointestinal mobility. Similar expression patterns were observed for VIP, iNOS, and AchE. These levels were significantly increased only in the LOP, whereas the HCC mice showed reduced levels of these molecules (Figures 2A–C). However, the neurotransmitter 5-HT did not have a significant reversal trend in the CCAE treatment groups. In general, LOP increased the serum level of inhibitory neurotransmitters and AchE, and the high dosage of CCAE (HCC group) reduced them to the control level.
Figure 2. (A) Vasoactive intestinal polypeptide (VIP), (B) induced nitric oxide synthases (iNOS), (C) acetylcholinesterase (AchE), and (D) serotonin (5-HT) in serum. The data are expressed as the means ± SEMs (n = 8). *, compared with the CON group; #, compared with the LOP group. *P < 0.05; **P < 0.01; ***P < 0.001; ##P < 0.01; ###P < 0.001.
Interstitial cells of Cajal (ICCs) and smooth muscle cells (SMCs) play a very important role in regulating gastrointestinal motility. The contraction and relaxation of smooth muscle is controlled by the slow wave of smooth muscle. As the pacemaker cells of smooth muscle and mediators of neurotransmitters, ICCs exist in the muscle layer. Stem cell factor receptor (c-Kit) is a biomarker of ICC. The stem cell factor (SCF) and c-Kit signaling pathways play vital roles in maintaining the development, differentiation and phenotype of ICCs. In mice in the LOP group, loperamide significantly reduced the mRNA expression of c-Kit and SCF in the colon; interestingly, CCAE treatment significantly increased their expression (Figures 3A,B). The immunohistochemistry results showed that c-Kit expression in colon muscle was decreased with the onset of STC induced by loperamide and increased with CCAE treatment (Figures 3C,D).
Figure 3. Aqueous extract of Cymbopogon citratus (DC.) Stapf (CCAE) increased the expression of gastrointestinal motility factors in the colon of STC mice. (A,B) The mRNA expression of stem cell factor (SCF) and stem cell factor receptor (c-Kit) in the colon. (C) Percentage of c-Kit-positive area in colon muscle. (D) Representative immunostaining images of colon sections stained for c-Kit. (E–H) The mRNA expression of Anoctamin 1 (Ano1), Ryanodine receptor 3 (RyR3), smooth muscle myosin light chain kinase (smMLCK), and Connexin 43 (Cx43) in the colon. The data are expressed as the means ± SEMs (n = 8). *, Compared with the CON group; #, compared with the LOP group. *P < 0.05, **P < 0.01, ***P < 0.001. #P < 0.05, ##P < 0.01, ###P < 0.001.
In ICCs, calcium channels and calcium-activated chloride channels are mainly used to generate Ca2+ transients and then generate slow-wave currents, which are transmitted to smooth muscle cells through the network structure. The opening up of ryanodine receptor 3 (RyR3) in calcium channels can increase the release of Ca2+, activate myosin light chain kinase (MLCK), and finally cause smooth muscle contraction. Anoctamin 1 (Ano1), a calcium-activated chloride channel, also exists in ICCs. Connexin 43 (Cx43) is the most important connexin constituting gap junctions, which widely exist between ICCs and SMCs and play an important role in slow wave transmission. Mutation and reduction of Cx43 affect the number of gap junction channels on the cell membrane, thereby hindering the transmission of intercellular signals and resulting in gastrointestinal motility dysfunction. In this study, we found that loperamide significantly or nearly significantly reduced the mRNA expression levels of Ano1, RyR3, Cx43 and smooth muscle myosin light chain kinase (smMLCK), while CCAE significantly restored the expression levels of these genes to varying degrees, even exceeding the expression levels of the CON group (Figures 3E–H). In general, CCAE might promote defecation in STC mice by increasing the expression of key gastrointestinal motility factors present in colonic ICCs and SMCs.
Intestinal barrier function is essential for maintaining intestinal homeostasis. Dysfunction of the intestinal barrier may trigger an excessive immune response and prolong the inflammatory state, resulting in a variety of gastrointestinal diseases. The mRNA expression of IL-1β in the LOP group was higher than that in the CON group, while CCAE treatment significantly reduced it (Figure 4A). However, loperamide and CCAE treatment did not have an obvious influence on the mRNA expression of MCP-1, TNF-α or IL-6 (Supplementary Figures 2A–C). Interestingly, the gene expression of the anti-inflammatory factor IL-10 was significantly inhibited by loperamide, and CCAE effectively restored the expression level of IL-10 in the colon of the STC model mice (Figure 4B). The regulatory effect on iNOS also suggests that CCAE has a certain anti-inflammatory ability (Figure 2B).
Figure 4. Effects of CCAE administration on the mRNA expression of inflammation- and intestinal barrier-related factors in the colons of mice. (A–H) IL-1β, IL-10, Muc-2, Cldn4, Cldn12, Occludin, ZO-1, and ZO-2. The data are expressed as the means ± SEMs (n = 8). *, Compared with the NCD group; #, compared with the LOP group. *P < 0.05, **P < 0.01, ***P < 0.001. #P < 0.05, ##P < 0.01, ###P < 0.001.
The mucus layer is mainly lined with the muco-protein (Muc) skeleton and complex O-linked oligosaccharides, which can separate the bacteria in the intestinal lumen from the intestinal epithelial cells and allow the absorption of nutrients. We found that the mRNA expression of Muc-2 in the LOP group was inhibited, and CCAE treatment could prevent this inhibition and restore the expression of Muc-2 to the control level (Figure 4C).
Claudin, Occludin, and ZO family proteins play an important role in maintaining the normal physiological functions of epithelial cells. The mRNA expression of tight junction proteins, including Claudin4, Claudin12, Occludin, ZO-1, and ZO-2, showed similar trends among the groups, all of which were significantly lower in the LOP group than in the CON group, and the CCAE treatment groups had higher levels than the LOP group (Figures 4D–H). In general, CCAE showed a good ability to prevent loperamide-induced intestinal inflammation and impaired barrier function.
The gut microbiota plays an important role in the progression of STC. The V3–V4 regions of the 16S rRNA genes were sequenced to determine the effect of CCAE (HCC group) on the STC model mice. We obtained 532,597 sequences in a total of 18 samples from the CON, LOP, and HCC groups, each with more than 21,571 valid sequences for subsequent taxonomic analysis. Through systematic bioinformatics analysis, we identified 591 ASVs, 84 genera, 40 families, and 9 phyla. The rarefaction curve of the Sobs index of each sample plateau with the current sequencing indicated that the sequencing result was credible (Figure 5A).
Figure 5. HCC restores the LOP-induced gut microbial community structural and compositional shift. (A) The rarefaction curve of the Sobs index of each sample plateau at the ASV level. (B) Alpha diversity estimated by the Shannon index. (C) PCoA (principal coordinate analysis) plot based on weighted UniFrac distance. (D) Linear discriminant analysis effect size (LEfSe) analyses (LDA score of > 2.0). (E–N) Relative abundances of gut microbiota at the phylum, family, and genus levels, which were significantly affected by LOP or HCC, especially those reversed by HCC treatment. The data are expressed as the means ± SEMs (n = 6). *, Compared with the CON group; #, compared with the LOP group. *P < 0.05, **P < 0.01. #P < 0.05, ##P < 0.01.
The community diversity (Shannon, Sobs, Chao, and Ace) and community evenness (Shannoneven, Simpsoneven) indices were all reduced in the LOP group compared with the CON group (Figure 5B and Supplementary Figure 3). CCAE administration reversed these loperamide-induced diversity index changes to varying degrees. Notably, CCAE administration significantly improved the loperamide-induced decrease in the Shannon, Shannoneven, and Simpsoneven indices (P < 0.05, Figure 5B and Supplementary Figures 3A,B). These results indicated that CCAE could increase the α diversity of the gut microbiota in STC mice. CCAE also altered β diversity in STC mice (Figure 5C). Although the administration of high-dose CCAE could not completely reverse the significant changes induced by loperamide, CCAE still appeared to regulate the abnormal gut microbiota in STC mice. Moreover, CCAE changed the cecal microbial composition of STC mice (Supplementary Figures 4A–C), and the microbial composition of CCAE-treated mice was clustered with that of the CON group (Supplementary Figures 4D–F).
Linear discriminant analysis (LDA) effect size (LEfSe) analyses were used to obtain the dominant microbiota at different levels for each group (Figure 5D and Supplementary Figures 5A–D). Here, a total of 43 different taxa from the 3 groups are displayed, including 5 phyla, 5 classes, 6 orders, 10 families, and 17 genera (Supplementary Figures 5A–C). We focused on the taxa that were significantly affected by LOP or HCC, especially those significantly changed by HCC treatment (Supplementary Figure 5D).
At the phylum level (Figure 5E), the relative abundances of six phyla were all significantly altered by loperamide treatment, including Firmicutes, Bacteroidota, Proteobacteria, Actinobacteriota, Desulfobacterota, and Patescibacteria. This finding suggests a comprehensive effect of loperamide on the gut microbiota in mice. CCAE treatment had different degrees of reversal effects on five phyla, except for Patescibacteria, but these reversal effects did not reach statistical significance (Figure 5E).
At the family level, the dominant families of gut bacteria, Muribaculaceae and Lachnospiraceae, belonging to Firmicutes and Bacteroidota, respectively, were significantly upregulated and downregulated in relative abundance under loperamide induction, respectively; importantly, CCAE could significantly reverse the loperamide-induced changes (Figure 5F). Similarly, CCAE significantly reduced the relative abundance of Flavobacteriaceae and UCG-010 compared to the LOP group, although loperamide was not able to increase their abundances significantly (Figure 5G). There were also some other families that were significantly upregulated or downregulated under the induction by loperamide, and CCAE treatment had a certain reversal effect on them, but there was no statistical significance (Figures 5G,H). Among them, Eubacterium coprostanoligenes group and Sutterellaceae were significantly upregulated (Figure 5G); Desulfovibrionaceae, Lactobacillaceae, Ruminococcaceae, Saccharimonadaceae, Anaerovoracaceae, and Eggerthellaceae were significantly downregulated (Figure 5H).
At the genus level, the most interesting taxa still belonged to Firmicutes and Bacteroidota. Unclassified_f__Lachnospiraceae, Lachnospiraceae_UCG-006 and GCA-900066575 belong to Lachnospiraceae, and their relative abundances were all significantly reduced in mice of the LOP group but reversed significantly in the HCC group (Figures 5I,J). Notably, norank_f__Muribaculaceae (belonging to Bacteroidota) and Anaeroplasma (belonging to Firmicutes) showed the opposite variation (Figures 5I,N). Some other genera in Firmicutes also showed nearly the same patterns as Lachnospiraceae, including Family_XIII_UCG-001 and UCG-005 (Figure 5J). CCAE treatment significantly increased the relative abundance of Paludicola, UCG-009, Eubacterium xylanophilum group, and norank_f__Lachnospiraceae, although loperamide did not reduce their abundances significantly. Interestingly, Prevotellaceae_UCG-001 and Rikenellaceae_RC9_gut_group (belonging to Bacteroidota) showed a similar pattern with Lachnospiraceae (Figure 5K). Anaerotruncus, unclassified_f__Oscillospiraceae, Lactobacillus, and Lachnospiraceae_NK4A136_group were also significantly reduced by loperamide, while the reversal effects of CCAE did not reach statistical significance (Figure 5L). The same pattern was exhibited in some genera belonging to Actinobacteriota, Desulfobacterota and Patescibacteria, including unclassified_f__Eggerthellaceae, Enterorhabdus, Bilophila, Desulfovibrio, and Candidatus_Saccharimonas (Figure 5M). In addition, loperamide treatment enhanced the relative abundance of norank_f__Flavobacteriaceae, norank_f__UCG-010 and Parasutterella to varying extents; CCAE treatment significantly reduced the abundance of the first two of them (Figure 5N). These results fully demonstrated that CCAE could partially restore loperamide-induced gut microbial dysbiosis in many aspects, such as microbial diversity, community structure, and species composition.
To further clarify the possible role of gut microbiota in the amelioration of loperamide-induced STC progression by CCAE, we systematically analyzed the correlations between taxa-specific gut bacteria and core host parameters, such as laxative phenotypic indicators, serum neurotransmitters, gastrointestinal motility factors, intestinal inflammation, and intestinal barrier function, at the phylum, family, and genus levels.
Correlations between the specific gut bacteria and the laxative phenotypic indicators are shown in Figures 6A–C and Supplementary Figure 6. FBS is the core host parameter that could directly reflect the laxative effect of CCAEs on mice. At the phylum level (Figure 6A), correlations between Firmicutes, Bacteroidota and FBS showed opposite trends. Firmicutes and Desulfobacterota were significantly negatively correlated with FBS, and Bacteroidota and Proteobacteria were significantly positively correlated with FBS. Actinobacteriota and Patescibacteria were significantly positively correlated with FN.
Figure 6. Network and heatmaps showing correlations between specific gut bacteria and core host parameters in STC mice. (A–C) Two-factor correlation network analysis (P < 0.05; Spearman, n = 6 in each group). Red lines represent r values ≥ 0.4, and green lines represent r values ≤ 0.4. Correlations between gut bacteria and the laxative phenotypic indicators, including (A) at the phylum level, (B) at the family level, and (C) at the genus level. FBS, the defecation time of the first black stool; the wet weight of the feces (FW) and the number of feces excreted in 6 h (FN); the gastrointestinal transit rate (GTR). (D,E) Bivariate correlations (P < 0.05, n = 6 in each group), including correlations between gut bacteria and intestinal inflammation, gut barrier function in the colon of mice (D), correlations between gut bacteria and serum neurotransmitters, gastrointestinal motility factors (E). IL-10, ZO-1, ZO-2, Cldn4, Cldn12, Occludin, c-Kit, SCF, Ano1, and Cx43 indicate their mRNA expression levels in the colons of mice. 5-HT, iNOS, and VIP indicate their expression levels in serum. The color at each intersection indicates the value of the r coefficient; P -alues were adjusted for multiple testing according to the Bonferroni and Hochberg procedures. * Indicates a significant correlation between these two parameters.
At the family level, Anaerovoracaceae and Lachnospiraceae were significantly negatively correlated with FBS and positively correlated with FN and FW. Muribaculaceae, Flavobacteriaceae, and UCG-010 show completely opposite correlations. Ruminococcaceae and Desulfovibrionaceae were also significantly negatively correlated with FBS, while Sutterellaceae and Peptococcaceae also showed a positive correlation with FBS. Notably, there was also a significant negative correlation between UCG-010 and GTR.
At the genus level, correlations between the microbial groups and defecation phenotype are relatively complex, but the correlation laws are still clear. The genera with a strong correlation mainly belonged to Firmicutes and Bacteroidota. The important genera with a significant negative correlation with FBS mainly included unclassified_f__Lachnospiraceae, Lachnospiraceae_UCG-006, norank_f__Ruminococcaceae, UCG-005, Roseburia, unclassified_f__Eggerthellaceae, and GCA-900066575; meanwhile, they showed a significant positive correlation with FN or FW. The important genera with a significant positive correlation with FBS mainly included norank_f__Muribaculaceae, norank_f__Flavobacteriaceae, Parasutterella, and norank_f__UCG-010; meanwhile, they showed a significant negative correlation with FN or FW. It is worth mentioning that unclassified_f__Eggerthellaceae (belonging to Actinobacteriota) also exhibited a strong negative correlation with FBS and a positive correlation with GTR and FN. Interestingly, GTR showed significant correlations with a few genera, only negatively correlated with norank_f__UCG-010, Muribaculum, and norank_f__Peptococcaceae and positively correlated with unclassified_f__Eggerthellaceae and unclassified_f__Lachnospiraceae. Of course, some other genera of gut bacteria also showed strong associations with mouse laxative phenotypes, such as FN and FW. All these numerous correlations suggest that gut microbiota might play an important role in regulating the laxative phenotype.
Correlations between the specific gut bacteria and intestinal inflammation and gut barrier function are shown in Figure 6D. Interestingly and importantly, the anti-inflammatory factor IL-10 was only significantly positively correlated with the highly abundant genus unclassified_f__Lachnospiraceae; moreover, both unclassified_f__Lachnospiraceae and IL-10 were significantly reversed by CCAE in STC mice. Among the gut barrier function factors, ZO-1 showed the most correlations with the microbial taxa. Several taxa of Actinobacteria had a strong negative correlation with ZO-1, and the Eubacterium_coprostanoligenes_group of Firmicutes had a strong positive correlation with ZO-1. Ruminococcaceae and UCG-009 also showed a strong positive correlation with Cldn4. This suggests that some specific groups of Firmicutes and Actinobacteria might play important and positive roles in CCAE against loperamide-induced colonic inflammation and impaired barrier function.
The heatmap in Figure 6E clearly shows the correlations between the specific gut bacteria and serum neurotransmitters and gastrointestinal motility factors. c-kit, RyR3, and iNOS showed more correlations with gut microbes. At the family and genus levels, Eubacterium_coprostanoligenes_group, Parabacteroides, Parasutterella, and norank_f__Muribaculaceae were negatively correlated with c-kit and RyR3, while Candidatus_Saccharimonas, Enterorhabdus, Eggerthellaceae, Lactobacillus, unclassified_f__Oscillospiraceae, and Anaerotruncus exhibited the opposite correlation. Except for Desulfovibrionaceae, the taxa with significant negative correlations with iNOS all belong to Firmicutes, mainly including norank_f__Lachnospiraceae, GCA-900066575, Lachnospiraceae, Eubacterium_xylanophilum_group, Paludicola, UCG-005, and Family_XIII_UCG-001, in which norank_f__Lachnospiraceae and GCA-900066575 belong to Lachnospiraceae. Notably, the genus Lachnospiraceae_NK4A136_group and family UCG-010 were negatively correlated with gap junction Cx43, which also belongs to Firmicutes. Interestingly, the genus Prevotellaceae_UCG-001 showed a significantly positive correlation. These results implied that some specific groups of Firmicutes, Bacteroidota, and Actinobacteriota might play different and important roles in CCAE regulating gastrointestinal peristalsis in STC mice.
Prolonged constipation often produces a variety of adverse reactions, causing serious distress to the affected people. Loperamide is an opioid receptor agonist that is used for the treatment of acute and chronic diarrhea caused by various etiologies. Therefore, it is widely used to induce constipation in animals (Hu et al., 2021). Loperamide mainly inhibits intestinal motility by blocking calcium channels, inhibiting calmodulin, reducing cell bypass permeability, and reducing the release of acetylcholine from intestinal nerve endings (Baker, 2007). In this study, loperamide also showed an excellent ability to shape the STC model.
Intestinal homeostasis plays an extremely important role in intestine-related diseases. Studies have shown that loperamide-induced STC animals generally have impaired intestinal homeostasis (Lin et al., 2021). Therefore, we systematically evaluated the effect of CCAE on loperamide-induced STC from three aspects, including intestinal movement, intestinal barrier and gut microbiota. We found that CCAE not only significantly decreased the expression of VIP, iNOS, and AchE (Figure 2), but also significantly increased the expression of the gastrointestinal motility factors SCF, c-Kit, Ano1, RyR3, and smMLCK (Figure 3), thereby improving the gastrointestinal transport rate and shortening the defecation time (Figure 1). At the same time, CCAE decreased the mRNA expression of the inflammatory factor IL-1β and increased the mRNA expression of the anti-inflammatory factor IL-10, chemical barrier Muc-2, mechanical barrier Cldn4, Cldn12, Occludin, ZO-1, and ZO-2 to repair the gut barrier and maintain intestinal homeostasis (Figure 4). Interestingly, CCAE also changed the intestinal microbial community structure and composition in loperamide-induced STC mice, and some important taxa of gut microbiota were significantly regulated by CCAE (Figure 5).
In recent years, the above neurotransmitters and gastrointestinal functional factors have received increasing attention in the research of STC, but there are few comprehensive reports that connect the important factors of each part of the ENS-ICCs-SMCs network. The ENS-ICCs-SMCs network is the basic functional unit of gastrointestinal movement, and it is mainly organized through the functions of ICCs. As a pacemaker cell for gastrointestinal activity, ICCs are also promoters of gastrointestinal electrical activity transmission and regulators of neurotransmitter transmission (Zhu et al., 2021). There are a large number of neurotransmitter receptors on the cell membrane of ICCs. When some neurotransmitters bind to the corresponding nerve receptors on ICCs, they can transmit excitatory or inhibitory nerve signals to SMCs through ICCs to regulate the relaxation and contraction of smooth muscle (Kim et al., 2006, 2013; Choi et al., 2010; Cipriani et al., 2011; Liu et al., 2020). Our results indicated that CCAE could reverse the loperamide-induced decrease in the expression of ICC cell marker c-Kit and changes in serum neurotransmitters to a certain extent. The pacing effect of ICCs on SMCs depends on the generation of slow waves, which are generated by Ca2+-induced potential changes in ICCs (Drumm et al., 2019). In this study, the mRNA expression of RyR3 of calcium channels and Ano1 of calcium-activated chloride channels was significantly inhibited in the LOP group, which was significantly reversed by CCAE. After the slow wave is generated, it is transmitted to SMCs via Cx43 to contract smooth muscle (Sancho et al., 2011). smMLCK is a key regulatory enzyme that controls the initiation of smooth muscle contraction and is widely present in smooth muscle (Zhang et al., 2016). We found that loperamide could significantly inhibit the expression of smMLCK and Cx43 in the mouse colon, while CCAE could significantly upregulate them. Therefore, we believe that CCAE can alleviate loperamide-induced STC by regulating the ENS-ICCs-SMCs network.
Intestinal homeostasis is mainly maintained by intestinal barrier function (Salinas et al., 2021). The normal intestinal mucosal barrier is mainly composed of four parts: mechanical barrier, chemical barrier, immune barrier and biological barrier. The occludin family, claudin family and ZO family are important protein molecules that constitute the tight junctions between cells (Zhang J. et al., 2021). The basic structure of the chemical barrier is the mucus layer, which is mainly composed of mucus and other substances secreted by the intestinal epithelium (Lu et al., 2021). When the mechanical barrier and chemical barrier are dysfunctional, bacteria and toxic products enter the immune barrier and induce production of inflammatory factors (Stolfi et al., 2022). The mRNA expression levels of ZO-1, Occludin, Cldn1, and Muc-2 could be significantly downregulated in the colon of constipated animals, and their expression levels were significantly upregulated after probiotic treatment (Eor et al., 2019; Lee et al., 2019; Lu et al., 2021). CCAE can also repair the intestinal barrier and maintain intestinal homeostasis by enhancing the mRNA expression levels of chemical barriers (Muc-2), mechanical barriers (Cldn4, Cldn12, Occludin, ZO-1, and ZO-2) and anti-inflammatory factors (IL-10).
The gut microbiota is an important part of the intestinal barrier and belongs to the biological barrier. The importance of the gut microbiota to health is now well known. Accumulating evidence supports the critical role of gut microbiota in regulating gut motility (Ohkusa et al., 2019; Zhang S. et al., 2021). Studies have confirmed that bacterial colonization in the gut is critical for the development and maturation of the ENS (Joly et al., 2021). Abnormal gastrointestinal microbiota composition may lead to disruption of “microbiota-gut-brain axis” signaling, leading to altered gut motility (Kennedy et al., 2012; Carabotti et al., 2015). Metabolites of gut microbiota could stimulate the ENS and affect gut motility (Barbara et al., 2005). Therefore, altering the gut microbiota may affect defecation behavior by regulating intestinal motility and secretion. In this study, CCAE partially reversed the gut microbiota changes induced by loperamide.
According to the analysis results of gut microbiota, we speculate that some high-abundance families and genera might play vital roles in the process of CCAE alleviating STC. For example, Muribaculaceae, Lachnospiraceae, norank_f__Muribaculaceae, and unclassified_f__Lachnospiraceae (Figures 5F,I) not only showed significant reversal-type changes during CCAE treatment but were also significantly associated with the laxative phenotypes, serum neurotransmitters, gastrointestinal motility factors, intestinal barrier, and intestinal inflammation (Figure 6). A series of previous clinical research results confirmed the rationality of our findings and speculations in mice. For example, a clinical study of irritable bowel syndrome showed that a higher Firmicutes to Bacteroidetes ratio was positively associated with loose stools in patients (Hollister et al., 2020). Parthasarathy et al. (2016) found that genera from Bacteroidetes were more abundant in the colonic mucosal microbiota of patients with constipation, and that the profile of the fecal microbiota was associated with colonic transit; genera from Firmicutes correlated with faster colonic transit; there was a decrease in the proportion of Firmicutes and an increase in Bacteroidetes in subjects with functional constipation, while ID-HWS1000 (composed of probiotics and prebiotics) directly improved the discomfort associated with bowel movements, decreased the proportion of Lachnospiraceae, and increased the proportion of Bacteroidaceae (Kim M. C. et al., 2021).
Previous animal experimental studies also agreed with our findings. Phlorotannins derived from Ecklonia cava could improve the constipation phenotype and restore the abundance of Muribaculaceae in the fecal microbiota of STC rats (Kim J. E. et al., 2021). Goji Berry and soluble fiber dextrin from tapioca promote the growth of butyrate-producing bacteria, including Lachnospiraceae and Ruminococcaceae, while reducing proinflammatory factors in IL-10-deficient mice (Valcheva et al., 2015; Kang et al., 2018). We found that both unclassified_f__Lachnospiraceae and IL-10 were significantly reversed by CCAE in STC mice, and IL-10 was only significantly positively correlated with the highly abundant genus unclassified_f__Lachnospiraceae.
Although the abovementioned high abundance families and genera performed well in the correlation analysis with the phenotypic indicators of constipation, they generally performed well in the correlation analysis with serum neurotransmitters, gastrointestinal motility factors and intestinal barrier. In contrast, the comprehensive performance of some low abundance taxa is remarkable, including Flavobacteriaceae, UCG-010, Anaerovoracaceae, norank_f__Flavobacteriaceae, Lachnospiraceae_UCG-006, GCA-900066575, Family_XIII_UCG-001, UCG-005, Paludicola, UCG-009, and Prevotellaceae_UCG-001, although some of these taxa did not show the most prominent abundance changes in this study. We could hardly find literature reports of these taxa related to constipation. Only Lachnospiraceae_UCG-006 was found to be associated with yellow tea extract interventions for constipation relief (Cao et al., 2021), which is consistent with our findings. In addition, several low abundance taxa of Actinobacteria (unclassified_f__Eggerthellaceae, Eggerthellaceae and Enterorhabdus) had a strong correlation with intestinal barrier and gastrointestinal motility factors in this study. The correlation between the taxa and intestinal barrier has been mentioned in previous studies (Chen et al., 2021; Han et al., 2021), but there is no report on their association with gastrointestinal motility factors. This also suggests that our study may reveal more potential associations between microbes and constipation-related markers that have not yet been addressed.
Overall, we believe that gut microbiota play a very important role in regulating the laxative phenotype; some specific taxa of Firmicutes and Actinobacteria might play positive roles in CCAE against loperamide-induced colonic inflammation and impaired barrier function; some specific taxa of Firmicutes, Bacteroidota and Actinobacteriota might play different and important roles in CCAE regulating gastrointestinal peristalsis.
CCAE is a nutrient-rich, phytochemically diverse complex (Supplementary Tables 1, 3,4). Although we have systematically evaluated the laxative effect of CCAE, the laxative active components and in vivo pharmacodynamic substance of CCAE are still unclear. However, some main components of CCAE exhibit laxative-related biological activity. For example, dietary fiber intake can obviously increase stool frequency in patients with constipation (Yang et al., 2012); as a metabolite, the betaine content decreased in constipated rats and increased significantly after the symptoms of constipation were relieved (Kim et al., 2019); betaine can also ameliorate intestinal injury in heat-challenged broilers by suppressing inflammatory responses and enhancing mucosal barrier function (Alhotan et al., 2021); vitexin exerted neural protective effects via antioxidant, anti-inflammatory and gut microbiota modulating properties (Li et al., 2021); and crotonoside, cordycepin, and cynaroside have significant anti-inflammatory effects (Lin et al., 2020; Pei et al., 2021; Tan et al., 2021). These results on major bioactive components of CCAE not only provide evidence for our research results of CCAE relieving constipation but also provide references for us to further clarify the molecular basis how CCAE relieves constipation.
In summary, we systematically studied the effect of CCAE on host parameters and the gut microbiota in loperamide-induced STC mice. We found that CCAE could significantly improve loperamide-induced constipation symptoms. We believe that CCAE might promote intestinal motility by modulating the ENS-ICCs-SMCs network, intestinal inflammation, intestinal barrier and gut microbiota, thereby relieving constipation. Meanwhile, the established correlation networks between the gut microbiota and the laxative phenotypic indicators in STC mice provided a foundation for further clarifying the relationship between the gut microbiota and host metabolism in STC mice.
The data presented in this study are deposited in the SRA repository, accession number PRJNA840843.
The animal study was reviewed and approved by Animal Ethics Committee of Yunnan Agriculture University.
XG: conceptualization, data curation, formal analysis, methodology, and writing – original draft, review and editing. YH: data curation, formal analysis, methodology, visualization, and writing – original draft. YTa, SL, HC, and JL: methodology. YZ: visualization. JS: conceptualization and funding acquisition. YTi: conceptualization and supervision. YF: resources, supervision, and funding acquisition. All authors read and approved the final manuscript.
This research was funded by the Major Project of Science and Technology Department of Yunnan Province (202102AE090027-2), YEFICRC project of Yunnan provincial key programs (2019ZG009), and Yunnan Fundamental Research Projects (202001AT070123).
The authors declare that the research was conducted in the absence of any commercial or financial relationships that could be construed as a potential conflict of interest.
All claims expressed in this article are solely those of the authors and do not necessarily represent those of their affiliated organizations, or those of the publisher, the editors and the reviewers. Any product that may be evaluated in this article, or claim that may be made by its manufacturer, is not guaranteed or endorsed by the publisher.
The Supplementary Material for this article can be found online at: https://www.frontiersin.org/articles/10.3389/fmicb.2022.1017804/full#supplementary-material
Supplementary Figure 1 | Effects of CCAE on loperamide-induced constipation symptoms in mice. (A) The body weight. (B) Food intake. (C) The water consumption. (D) Spleen index. (E) The small intestine length. (F) Cecum index.
Supplementary Figure 2 | Gene expression of intestinal inflammatory factors in colon of STC mice. (A–C) Inflammatory factor MCP-1, TNF-α, and IL-6.
Supplementary Figure 3 | (A–F) Shannoneven, simpsoneven, ace, chao, sobs, simpson index of ASV level. *compared with the NCD group, using the Kruskal-Wallis H test. **P < 0.01.
Supplementary Figure 4 | Effect of CCAE on the cecum microbial composition in STC mice. (A) Phylum level. (B) Family level. (C) Genus level. (D–F) Cluster heatmaps of gut microbiota in different groups.
Supplementary Figure 5 | Linear discriminant analysis effect size (LEfSe) analyses (LDA score > 2.0). (A–C) LEfSe analyses based on the CON, LOP and HCC groups. (A) At the phylum, class and order level. (B) At the family level. (C) At the genus level. (D) LEfSe analyses based on the LOP and HCC groups, from the phylum level to the genus level.
Supplementary Figure 6 | Heat maps showing correlations between specific gut bacteria and the core laxative phenotypic indicators in STC mice. Bivariate correlations (P < 0.05, n = 6 in each group), including correlations between gut bacteria and the core laxative phenotypic indicators. FBS, the defecation time of the first black stool; the fecal wet weight (FW) and the fecal number (FN) in 6 h; the gastrointestinal transit rate (GTR). The color at each intersection indicates the value of the r coefficient; P-values were adjusted for multiple testing according to the Bonferroni and Hochberg procedures. * indicates a significant correlation between these two parameters (P < 0.05).
STC, slow transit constipation; CC, Cymbopogon citratus (DC.) Stapf; CCAE, aqueous extract of Cymbopogon citratus (DC.) Stapf; ENS, enteric nervous system; VIP, vasoactive intestinal polypeptide; iNOS, induced nitric oxide synthases; AchE, acetylcholinesterase; 5-HT, serotonin; ICCs, interstitial cells of Cajal; SMCs, smooth muscle cells; c-Kit, stem cell factor receptor; SCF, stem cell factor; Ano1, anoctamin 1; RyR3, ryanodine receptor 3; smMLCK, smooth muscle myosin light chain kinase; Cx43, connexin 43; FBS, the defecation time of the first black stool; FW, fecal wet weight; FN, fecal number; GTR, gastrointestinal transit rate; TNF- α, tumor necrosis factor-alpha; IL-1 β, interleukin-1 β; IL-6, interleukin-6; IL-10, interleukin-10; MCP-1, monocyte chemotactic protein-1; Muc2, mucin 2; Occludin, occluding; ZO-1, zonula occludens protein 1; ZO-2, zonula occludens protein 2; Cldn4, claudin 4; Cldn12, claudin 12; LEfSe, linear discriminant analysis effect size; PCoA, principal coordinate analysis; CON, control group; POS, positive control group; LOP, model group, received loperamide; LCC, LCC group (received loperamide and low dosage of CCAE); MCC, MCC group (received loperamide and medium dosage of CCAE); HCC, HCC (received loperamide and high dosage of CCAE).
Alhotan, R. A., Al Sulaiman, A. R., Alharthi, A. S., and Abudabos, A. M. (2021). Protective influence of betaine on intestinal health by regulating inflammation and improving barrier function in broilers under heat stress. Poult. Sci. 100:101337. doi: 10.1016/j.psj.2021.101337
Baker, D. E. (2007). Loperamide: A pharmacological review. Rev. Gastroenterol. Disord. 7(Suppl. 3) S11–S18.
Barbara, G., Stanghellini, V., Brandi, G., Cremon, C., Di Nardo, G., De Giorgio, R., et al. (2005). Interactions between commensal bacteria and gut sensorimotor function in health and disease. Am. J. Gastroenterol. 100, 2560–2568. doi: 10.1111/j.1572-0241.2005.00230.x
Bharucha, A. E., and Lacy, B. E. (2020). Mechanisms, evaluation, and management of chronic constipation. Gastroenterology 158, 1232–1249. doi: 10.1053/j.gastro.2019.12.034
Borges, P., Pedreiro, S., Baptista, S. J., Geraldes, C., Batista, M. T., Silva, M., et al. (2021). Inhibition of α-glucosidase by flavonoids of cymbopogon citratus (DC) Stapf. J. Ethnopharmacol. 280:114470. doi: 10.1016/j.jep.2021.114470
Cao, P. Q., Li, X. P., Ou-Yang, J., Jiang, R. G., Huang, F. F., Wen, B. B., et al. (2021). The protective effects of yellow tea extract against loperamide-induced constipation in mice. Food Funct. 12, 5621–5636. doi: 10.1039/d0fo02969f
Carabotti, M., Scirocco, A., Maselli, M. A., and Severi, C. (2015). The gut-brain axis: Interactions between enteric microbiota, central and enteric nervous systems. Ann. Gastroenterol. 28, 203–209.
Chen, K. J., Chen, Y. L., Ueng, S. H., Hwang, T. L., Kuo, L. M., and Hsieh, P. W. (2021). Neutrophil elastase inhibitor (MPH-966) improves intestinal mucosal damage and gut microbiota in a mouse model of 5-fluorouracil-induced intestinal mucositis. Biomed. Pharmacother. 134:111152. doi: 10.1016/j.biopha.2020.111152
Choi, S., Sun, J. M., Shahi, P. K., Zuo, D. C., Kim, H. I., and Jun, J. Y. (2010). Capsaicin inhibits the spontaneous pacemaker activity in interstitial cells of cajal from the small intestine of mouse. J. Neurogastroenterol. Motil. 16, 265–273. doi: 10.5056/jnm.2010.16.3.265
Cipriani, G., Serboiu, C. S., Gherghiceanu, M., Faussone-Pellegrini, M. S., and Vannucchi, M. G. (2011). NK receptors, substance P, Ano1 expression and ultrastructural features of the muscle coat in Cav-1(-/-) mouse ileum. J. Cell. Mol. Med. 15, 2411–2420. doi: 10.1111/j.1582-4934.2011.01333.x
Drumm, B. T., Hwang, S. J., Baker, S. A., Ward, S. M., and Sanders, K. M. (2019). Ca2+ signaling behaviors of intramuscular interstitial cells of Cajal in the murine colon. J. Physiol. 597, 3587–3617. doi: 10.1113/JP278036
Eor, J. Y., Tan, P. L., Lim, S. M., Choi, D. H., Yoon, S. M., Yang, S. Y., et al. (2019). Laxative effect of probiotic chocolate on loperamide-induced constipation in rats. Food Res. Int. 116, 1173–1182. doi: 10.1016/j.foodres.2018.09.062
Fernandes, C., De Souza, H., De Oliveria, G., Costa, J., Kerntopf, M., and Campos, A. (2012). Investigation of the mechanisms underlying the gastroprotective effect of Cymbopogon citratus essential oil. J. Young Pharm. JYP 4, 28–32. doi: 10.4103/0975-1483.93578
Figueirinha, A., Cruz, M. T., Francisco, V., Lopes, M. C., and Batista, M. T. (2010). Anti-inflammatory activity of Cymbopogon citratus leaf infusion in lipopolysaccharide-stimulated dendritic cells: Contribution of the polyphenols. J. Med. Food 13, 681–690. doi: 10.1089/jmf.2009.0115
Gao, X., Chang, S., Liu, S., Peng, L., Xie, J., Dong, W., et al. (2020). Correlations between α-Linolenic acid-improved multitissue homeostasis and gut microbiota in mice fed a high-fat diet. mSystems 5, e00391–20. doi: 10.1128/mSystems.00391-20
Gao, X., Xie, Q., Liu, L., Kong, P., Sheng, J., and Xiang, H. (2017). Metabolic adaptation to the aqueous leaf extract of Moringa oleifera Lam.-supplemented diet is related to the modulation of gut microbiota in mice. Appl. Microbiol. Biotechnol. 101, 5115–5130. doi: 10.1007/s00253-017-8233-5
Gomes, L. F., Longhi, P., Machado, L., da Cruz, I., Montano, M., Martins, M., et al. (2021). Lemongrass (Cymbopogon citratus (D.C.) Stapf) presents antitumoral effect and improves chemotherapy activity in prostate cancer cells. Anti-Cancer Agents Med. Chem. 21, 2337–2350. doi: 10.2174/1871520621666210112111711
Han, R., Ma, Y., Xiao, J., You, L., Pedisiæ, S., and Liao, L. (2021). The possible mechanism of the protective effect of a sulfated polysaccharide from Gracilaria Lemaneiformis against colitis induced by dextran sulfate sodium in mice. Food Chem. Toxicol. 149:112001. doi: 10.1016/j.fct.2021.112001
Hollister, E. B., Cain, K. C., Shulman, R. J., Jarrett, M. E., Burr, R. L., Ko, C., et al. (2020). Relationships of microbiome markers with extra-intestinal, psychological distress and gastrointestinal symptoms, and quality of life in women with irritable bowel syndrome. J. Clin. Gastroenterol. 54, 175–183. doi: 10.1097/MCG.0000000000001107
Hu, M., Fang, C., Liu, Y., Gao, M., Zhang, D., Shi, G., et al. (2021). Comparative study of the laxative effects of Konjac oligosaccharides and Konjac glucomannan on loperamide-induced constipation in rats. Food Funct. 12, 7709–7717. doi: 10.1039/d1fo01237a
Huang, Y. W., Zhu, Q. Q., Yang, X. Y., Xu, H. H., Sun, B., Wang, X. J., et al. (2019). Wound healing can be improved by (-)-epigallocatechin gallate through targeting Notch in streptozotocin-induced diabetic mice. FASEB J. 33, 953–964. doi: 10.1096/fj.201800337R
Iram, F., Tariq, M., and Sobia Kanwal. (2019). Microbiostatic, antioxidative and cytotoxic potentiation of some grasses of Bahawalpur, Pakistan. J. Tradit. Chine. Med. 39, 482–491.
Joly, A., Leulier, F., and De Vadder, F. (2021). Microbial modulation of the development and physiology of the enteric nervous system. Trends Microbiol. 29, 686–699. doi: 10.1016/j.tim.2020.11.007
Kang, Y., Yang, G., Zhang, S., Ross, C. F., and Zhu, M. J. (2018). Goji Berry modulates gut microbiota and alleviates colitis in IL-10-Deficient Mice. Mol. Nutr. Food Res. 62:e1800535. doi: 10.1002/mnfr.201800535
Kennedy, P. J., Clarke, G., Quigley, E. M., Groeger, J. A., Dinan, T. G., and Cryan, J. F. (2012). Gut memories: Towards a cognitive neurobiology of irritable bowel syndrome. Neurosci. Biobehav. Rev. 36, 310–340. doi: 10.1016/j.neubiorev.2011.07.001
Kieling, D. D., and Prudencio, S. H. (2019). Blends of lemongrass derivatives and lime for the preparation of mixed beverages: Antioxidant, physicochemical, and sensory properties. J. Sci. Food Agric. 99, 1302–1310. doi: 10.1002/jsfa.9305
Kim, B. J., Kim, H. W., Lee, G. S., Choi, S., Jun, J. Y., So, I., et al. (2013). Poncirus trifoliate fruit modulates pacemaker activity in interstitial cells of Cajal from the murine small intestine. J. Ethnopharmacol. 149, 668–675. doi: 10.1016/j.jep.2013.07.017
Kim, B. J., Lee, J. H., Jun, J. Y., Chang, I. Y., So, I., and Kim, K. W. (2006). Vasoactive intestinal polypeptide inhibits pacemaker activity via the nitric oxide-cGMP-protein kinase G pathway in the interstitial cells of Cajal of the murine small intestine. Mol. Cells 21, 337–342.
Kim, J. E., Choi, Y. J., Lee, S. J., Gong, J. E., Jin, Y. J., Park, S. H., et al. (2021). Laxative effects of phlorotannins derived from Ecklonia cava on loperamide-induced constipation in SD Rats. Molecules 26:7209. doi: 10.3390/molecules26237209
Kim, J. E., Lee, Y. J., Ryu, S. H., Park, J. W., Kang, M. J., Choi, H. J., et al. (2019). Metabolomics approach to serum biomarker for laxative effects of red Liriope platyphylla in loperamide-induced constipation of SD rats. Lab. Anim. Res. 35:9. doi: 10.1186/s42826-019-0009-x
Kim, M. C., Lee, S., Park, J. K., Park, J., Lee, D., Park, J., et al. (2021). Effects of ID-HWS1000 on the perception of bowel activity and microbiome in subjects with functional constipation: A randomized, double-blind placebo-controlled study. J. Med. Food 24, 883–893. doi: 10.1089/jmf.2020.4746
Lee, C. S., Tan, P. L., Eor, J. Y., Choi, D. H., Park, M., Seo, S. K., et al. (2019). Prophylactic use of probiotic chocolate modulates intestinal physiological functions in constipated rats. J. Sci. Food Agric. 99, 3045–3056. doi: 10.1002/jsfa.9518
Li, M., Liu, B., Bernigaud, C., Fischer, K., Guillot, J., and Fang, F. (2020). Lemongrass (Cymbopogon citratus) oil: A promising miticidal and ovicidal agent against Sarcoptes scabiei. PLoS Negl. Trop. Dis. 14:e0008225. doi: 10.1371/journal.pntd.0008225
Li, S., Liang, T., Zhang, Y., Huang, K., Yang, S., Lv, H., et al. (2021). Vitexin alleviates high-fat diet induced brain oxidative stress and inflammation via anti-oxidant, anti-inflammatory and gut microbiota modulating properties. Free Rad. Biol. Med. 171, 332–344. doi: 10.1016/j.freeradbiomed.2021.05.028
Lin, S. C., Lin, C. C., Li, S., Lin, W. Y., Lehman, C. W., Bracci, N. R., et al. (2020). Alleviation of collagen-induced arthritis by crotonoside through modulation of dendritic cell differentiation and activation. Plants 9:1535. doi: 10.3390/plants9111535
Lin, X., Liu, Y., Ma, L., Ma, X., Shen, L., Ma, X., et al. (2021). Constipation induced gut microbiota dysbiosis exacerbates experimental autoimmune encephalomyelitis in C57BL/6 mice. J. Trans. Med. 19:317. doi: 10.1186/s12967-021-02995-z
Liu, J., Du, P., and Rudd, J. A. (2020). Acetylcholine exerts inhibitory and excitatory actions on mouse ileal pacemaker activity: Role of muscarinic versus nicotinic receptors. Am. J. Physiol. Gastroint. Liver Physiol. 319, G97–G107. doi: 10.1152/ajpgi.00003.2020
Lu, Y., Yu, Z., Zhang, Z., Liang, X., Gong, P., Yi, H., et al. (2021). Bifidobacterium animalis F1-7 in combination with Konjac glucomannan improves constipation in mice via humoral transport. Food Funct. 12, 791–801. doi: 10.1039/d0fo02227f
Madi, Y. F., Choucry, M. A., Meselhy, M. R., and El-Kashoury, E. A. (2021). Essential oil of Cymbopogon citratus cultivated in Egypt: Seasonal variation in chemical composition and anticholinesterase activity. Nat. Prod. Res. 35, 4063–4067. doi: 10.1080/14786419.2020.1713125
Mendes Hacke, A. C., Miyoshi, E., Marques, J. A., and Pereira, R. P. (2020). Anxiolytic properties of Cymbopogon citratus (DC.) stapf extract, essential oil and its constituents in Zebrafish (Danio rerio). J. Ethnopharmacol. 260:113036. doi: 10.1016/j.jep.2020.113036
Ohkusa, T., Koido, S., Nishikawa, Y., and Sato, N. (2019). Gut microbiota and chronic constipation: A review and update. Front. Med. 6:19. doi: 10.3389/fmed.2019.00019
Pan, D., Machado, L., Bica, C. G., Machado, A. K., Steffani, J. A., and Cadoná, F. C. (2022). In vitro evaluation of antioxidant and anticancer activity of lemongrass (Cymbopogon citratus (D.C.) Stapf). Nutr. Cancer 74, 1474–1488. doi: 10.1080/01635581.2021.1952456
Parthasarathy, G., Chen, J., Chen, X., Chia, N., O’Connor, H. M., Wolf, P. G., et al. (2016). Relationship between microbiota of the colonic mucosa vs feces and symptoms, colonic transit, and methane production in female patients with chronic Constipation. Gastroenterology 150, 367–379.e1. doi: 10.1053/j.gastro.2015.10.005
Pei, L., Le, Y., Chen, H., Feng, J., Liu, Z., Zhu, J., et al. (2021). Cynaroside prevents macrophage polarization into pro-inflammatory phenotype and alleviates cecal ligation and puncture-induced liver injury by targeting PKM2/HIF-1α axis. Fitoterapia 152:104922. doi: 10.1016/j.fitote.2021.104922
Rojas-Armas, J. P., Arroyo-Acevedo, J. L., Palomino-Pacheco, M., Herrera-Calderón, O., Ortiz-Sánchez, J. M., Rojas-Armas, A., et al. (2020). The essential oil of Cymbopogon citratus Stapt and Carvacrol: an approach of the antitumor effect on 7,12-Dimethylbenz-[α]-anthracene (DMBA)-induced breast cancer in female rats. Molecules 25:3284. doi: 10.3390/molecules25143284
Salinas, E., Reyes-Pavón, D., Cortes-Perez, N. G., Torres-Maravilla, E., Bitzer-Quintero, O. K., Langella, P., et al. (2021). Bioactive compounds in food as a current therapeutic approach to maintain a healthy intestinal epithelium. Microorganisms 9:1634. doi: 10.3390/microorganisms9081634
Sancho, M., Triguero, D., and Garcia-Pascual, A. (2011). Direct coupling through gap junctions is not involved in urethral neurotransmission. Am. J. Physiol. Renal Physiol. 300, F864–F872. doi: 10.1152/ajprenal.00641.2010
Stolfi, C., Maresca, C., Monteleone, G., and Laudisi, F. (2022). Implication of intestinal barrier fysfunction in gut fysbiosis and diseases. Biomedicines 10:289. doi: 10.3390/biomedicines10020289
Tan, L., Song, X., Ren, Y., Wang, M., Guo, C., Guo, D., et al. (2021). Anti-inflammatory effects of cordycepin: A review. Phytother. Res. 35, 1284–1297. doi: 10.1002/ptr.6890
Tiwari, M., Dwivedi, U. N., and Kakkar, P. (2010). Suppression of oxidative stress and pro-inflammatory mediators by Cymbopogon citratus D. Stapf extract in lipopolysaccharide stimulated murine alveolar macrophages. Food Chem. Toxicol. 48, 2913–2919. doi: 10.1016/j.fct.2010.07.027
Uchida, N. S., Silva-Filho, S. E., Aguiar, R. P., Wiirzler, L., Cardia, G., Cavalcante, H., et al. (2017). Protective effect of Cymbopogon citratus essential oil in experimental model of acetaminophen-induced liver injury. Am. J. Chine. Med. 45, 515–532. doi: 10.1142/S0192415X17500318
Umukoro, S., Ogboh, S. I., Omorogbe, O., Adekeye, A. A., and Olatunde, M. O. (2017). Evidence for the involvement of monoaminergic pathways in the antidepressant-like activity of Cymbopogon citratus in mice. Drug Res. 67, 419–424. doi: 10.1055/s-0043-106586
Valcheva, R., Hotte, N., Gillevet, P., Sikaroodi, M., Thiessen, A., and Madsen, K. L. (2015). Soluble dextrin fibers alter the intestinal microbiota and reduce proinflammatory cytokine secretion in male IL-10-deficient mice. J. Nutr. 145, 2060–2066. doi: 10.3945/jn.114.207738
Venzon, L., Mariano, L., Somensi, L. B., Boeing, T., de Souza, P., Wagner, T. M., et al. (2018). Essential oil of Cymbopogon citratus (lemongrass) and geraniol, but not citral, promote gastric healing activity in mice. Biomed. Pharmacother. 98, 118–124. doi: 10.1016/j.biopha.2017.12.020
Vriesman, M. H., Koppen, I., Camilleri, M., Di Lorenzo, C., and Benninga, M. A. (2020). Management of functional constipation in children and adults. Nat. Rev. Gastroenterol. Hepatol. 17, 21–39. doi: 10.1038/s41575-019-0222-y
Yang, J., Wang, H. P., Zhou, L., and Xu, C. F. (2012). Effect of dietary fiber on constipation: A meta-analysis. World J. Gastroenterol. 18, 7378–7383. doi: 10.3748/wjg.v18.i48.7378
Zhang, C. H., Wang, P., Liu, D. H., Chen, C. P., Zhao, W., Chen, X., et al. (2016). The molecular basis of the genesis of basal tone in internal anal sphincter. Nat. Commun. 7:11358. doi: 10.1038/ncomms11358
Zhang, J., Cao, L., Sun, Y., Qing, D. G., Xu, X. Q., Wang, J. C., et al. (2021). The regulatory effects of Licochalcone A on the intestinal epithelium and gut microbiota in murine colitis. Molecules 26:4149. doi: 10.3390/molecules26144149
Zhang, S., Wang, R., Li, D., Zhao, L., and Zhu, L. (2021). Role of gut microbiota in functional constipation. Gastroenterol. Rep. 9, 392–401. doi: 10.1093/gastro/goab035
Zhu, G. Y., Jia, D. D., Yang, Y., Miao, Y., Wang, C., and Wang, C. M. (2021). The Effect of Shaoyao Gancao decoction on sphincter of oddi dysfunction in hypercholesterolemic rabbits via protecting the enteric nervous system-interstitial cells of Cajal-smooth muscle cells network. J. Inflamm. Res. 14, 4615–4628. doi: 10.2147/JIR.S326416
Keywords: gut microbiota, lemon grass, constipation, gastrointestinal motility, enteric nervous system, intestinal inflammation, gut barrier, Muribaculaceae
Citation: Gao X, Hu Y, Tao Y, Liu S, Chen H, Li J, Zhao Y, Sheng J, Tian Y and Fan Y (2022) Cymbopogon citratus (DC.) Stapf aqueous extract ameliorates loperamide-induced constipation in mice by promoting gastrointestinal motility and regulating the gut microbiota. Front. Microbiol. 13:1017804. doi: 10.3389/fmicb.2022.1017804
Received: 12 August 2022; Accepted: 09 September 2022;
Published: 04 October 2022.
Edited by:
Hui Zhang, South China Agricultural University, ChinaReviewed by:
Teng-Gen Hu, Guangdong Academy of Agricultural Sciences (GDAAS), ChinaCopyright © 2022 Gao, Hu, Tao, Liu, Chen, Li, Zhao, Sheng, Tian and Fan. This is an open-access article distributed under the terms of the Creative Commons Attribution License (CC BY). The use, distribution or reproduction in other forums is permitted, provided the original author(s) and the copyright owner(s) are credited and that the original publication in this journal is cited, in accordance with accepted academic practice. No use, distribution or reproduction is permitted which does not comply with these terms.
*Correspondence: Jun Sheng, c2hlbmdqdW5feW5hdUAxNjMuY29t; Yang Tian, dGlhbnlhbmcxMjA4QDE2My5jb20=; Yuanhong Fan, MjI0Nzg4ODEzNkBxcS5jb20=
†These authors have contributed equally to this work
Disclaimer: All claims expressed in this article are solely those of the authors and do not necessarily represent those of their affiliated organizations, or those of the publisher, the editors and the reviewers. Any product that may be evaluated in this article or claim that may be made by its manufacturer is not guaranteed or endorsed by the publisher.
Research integrity at Frontiers
Learn more about the work of our research integrity team to safeguard the quality of each article we publish.