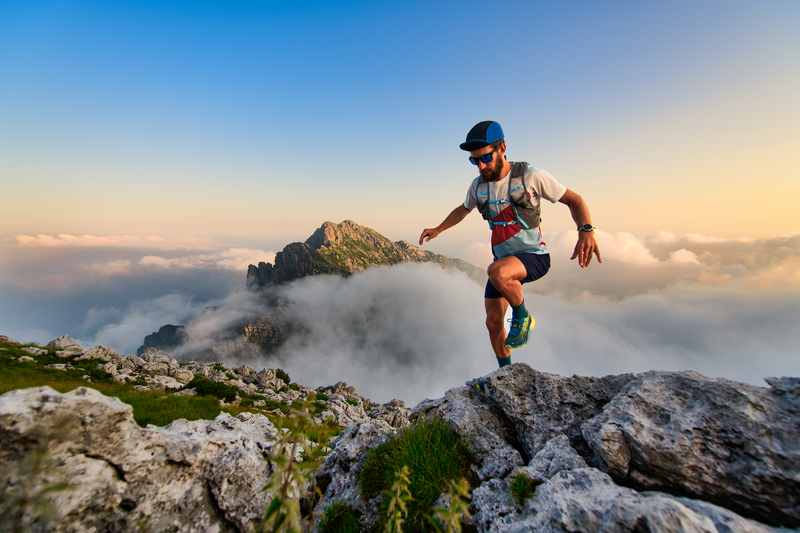
94% of researchers rate our articles as excellent or good
Learn more about the work of our research integrity team to safeguard the quality of each article we publish.
Find out more
ORIGINAL RESEARCH article
Front. Microbiol. , 24 October 2022
Sec. Microbial Physiology and Metabolism
Volume 13 - 2022 | https://doi.org/10.3389/fmicb.2022.1006116
1,3-xylan, an important organic carbon in the ocean, is peculiar to marine algae. 1,3-xylanase-secreting bacteria and their extracellular 1,3-xylanases play pivotal roles in the degradation and biomass conversion of 1,3-xylan. However, only a few 1,3-xylanase-secreting bacteria and 1,3-xylanases have been reported. Here, we identified a novel marine bacterium capable of secreting 1,3-xylanases, designated as strain HB14T. Phylogenetic analysis revealed that strain HB14T clustered tightly with known species of the genus Gilvimarinus, showing the highest 16S rRNA gene sequence similarity (97.7%) with the type strain of Gilvimarinus chinensis. Based on phylogenetic, genomic, chemotaxonomic and phenotypic studies, strain HB14T was classified as a representative of a novel species in the genus Gilvimarinus, for which the name Gilvimarinus xylanilyticus sp. nov. was proposed. The type strain is HB14T (=CCTCC AB 2022109T = KCTC 92379T). Four 1,3-xylanases secreted by strain HB14T were identified based on genome and secretome analyses, and the two (Xyn65 and Xyn80) with relatively higher abundance in secretome were successfully expressed in Escherichia coli and biochemically characterized. They showed the highest activity at pH 6.0–7.0 and 40°C and released mainly 1,3-xylobiose and 1,3-xylotriose from 1,3-xylan. These data suggest that strain HB14T acts as a player in marine 1,3-xylan degradation and recycling and that its extracellular 1,3-xylanases may have a good potential in 1,3-xylooligosaccharides preparation.
The genus Gilvimarinus, belonging to the family Cellvibrionaceae in the class Gammaproteobacteria, was proposed by Du et al. (2009). Currently, four members have been reported in this genus, including G. chinensis (Du et al., 2009), ‘G. agarilyticus’ (Kim et al., 2011), G. polysaccharolyticus (Cheng et al., 2015) and G. japonicus (Kouzui et al., 2016). Gilvimarinus species are Gram-stain-negative, motile, and rod-shaped bacteria having diphosphatidylglycerol, phosphatidylglycerol and phosphatidylethanolamine as the major polar lipids and ubiquinone-8 as the major quinone (Cheng et al., 2015). All species in the genus Gilvimarinus have been isolated from coastal environments, including seawater, sea sand, seaweed and debris (Du et al., 2009; Kim et al., 2011; Cheng et al., 2015; Kouzui et al., 2016), where phytoplankton blooms frequently occur. Furthermore, type strains of all Gilvimarinus species were reported to possess the capability to degrade algal polysaccharides, especially agar and cellulose (Du et al., 2009; Kim et al., 2011; Cheng et al., 2015; Kouzui et al., 2016; Kouzuma and Fujii, 2018; Lee et al., 2018, 2021). Thus, they likely play important ecological roles in seaweed decomposition in coastal marine environments.
1,3-xylan is a homopolymer composed of β-1,3-linked D-xylopyranose units, which is peculiar to marine algae and is the main component in the cell walls of some red and green algae (Iriki et al., 1960; Veluraja and Atkins, 1987; Hsieh and Harris, 2019; Qeshmi et al., 2020). 1,3-xylan is considered the main xylan structure and an important organic carbon in the ocean (Qeshmi et al., 2020; Sun et al., 2021). In addition, 1,3-xylan-containing algal biomass serves as a potential feedstock for producing useful chemical commodities and functional 1,3-xylooligosaccharides (Goddard-Borger et al., 2012; Maeda et al., 2012; Umemoto et al., 2012). 1,3-xylanase-secreting bacteria and their extracellular 1,3-xylanases (EC 3.2.1.32) play pivotal roles in the degradation and biomass conversion of 1,3-xylan in the ocean. So far, some 1,3-xylanase-secreting bacteria and the 1,3-xylanases they secrete have been reported, including Vibrio (Araki et al., 2000; Kiyohara et al., 2005), Pseudomonas (Aoki and Kamei, 2006; Liang et al., 2015), Alcaligenes (Okazaki et al., 2002), Thermotoga (Okazaki et al., 2013) and Flammeovirga (Cai et al., 2018; Yi et al., 2020) species. 1,3-xylanases catalyze the hydrolysis of β-1,3-xylosidic linkages in 1,3-xylan. In the Carbohydrate Active Enzymes (CAZy) database1 (Lombard et al., 2014), all the reported 1,3-xylanases are classified in glycoside hydrolase (GH) family 26. Despite these, more 1,3-xylan-secreting marine bacteria and 1,3-xylanases are required to be identified and studied for a comprehensive understanding of the degradation and recycling of marine 1,3-xylan and for the exploration of 1,3-xylanases with good biotechnological/industrial potentials.
During an investigation of 1,3-xylan-secreting bacteria from green algal samples collected from a Caulerpa lentillifera aquaculture base in Wenchang, Hainan, China, a strain, designated HB14T, was isolated from a green alga (Chaetomorpha sp.; Sun et al., 2021). Strain HB14T was prominent by its low 16S rRNA gene sequence similarity (97.7%) to known species in the genus Gilvimarinus and its ability to secrete 1,3-xylanases on the plate containing 1,3-xylan (Sun et al., 2021). In this study, the exact taxonomic position of strain HB14T was determined by using a polyphasic approach. Moreover, four extracellular 1,3-xylanases secreted by strain HB14T were identified by genome and secretome analyses, and two of these 1,3-xylanases were successfully expressed in E. coli and biochemically characterized. The results indicated that they may have a potential in the preparation of 1,3-xylooligosaccharides from 1,3-xylan.
Strain HB14T was isolated from a green alga (Chaetomorpha sp.) collected from a Caulerpa lentillifera aquaculture base in Wenchang, Hainan, China (Sun et al., 2021). Strain G. chinensis CGMCC 1.7008T was purchased from CGMCC and characterized alongside for comparative purposes. Unless otherwise stated, the strains were routinely cultivated in MBC [marine broth 2216 (MB; Difco) supplemented with 0.05% (w/v) cellobiose] or on MAC [marine broth 2216, 1.5% (w/v) agar and 0.05% (w/v) cellobiose] at 30°C.
The 16S rRNA gene of strain HB14T was amplified by PCR with the universal primers 27F (5’-AGAGTTTGATCCTGGCTCAG-3′) and 1492R (5’-GGTTACCTTGTTACGACTT-3′; Weisburg et al., 1991). The amplification product was cloned into the pClone007 Versatile Simple Vector (Tsingke, China), transferred into E. coli DH5α (Vazyme, China) and further sequenced at Tsingke (Qingdao, China). The obtained 16S rRNA gene sequence was compared to those of species validly published through the EzBioCloud server2 (Yoon et al., 2017a). Phylogenetic trees were constructed with MEGA 11 (Tamura et al., 2021) using maximum-likelihood (Felsenstein, 1981), neighbor-joining (Saitou and Nei, 1987) and maximum-parsimony (Fitch, 1971) methods. The topologies of the phylogenetic trees were evaluated by bootstrap analyses (1,000 replications).
Genomic DNA of strain HB14T was extracted and sequenced at Biozeron (Shanghai, China) using a bacterial DNA Kit (Omega, United States) and the Illumina NovaSeq 6,000, respectively. The DNA G + C % content was calculated directly from the draft genome sequence. The obtained draft genome was annotated using the NCBI Prokaryotic Genome Annotation Pipeline (PGAP; Tatusova et al., 2016) and RAST annotation server (Brettin et al., 2015). The digital DNA–DNA hybridization (dDDH) value and the average nucleotide identity (ANI) were calculated using the genome-to-genome distance calculator (GGDC 2.1) online service3 (Meier-Kolthoff et al., 2013) and the ChunLab’s online ANI calculator4 (Yoon et al., 2017b), respectively. The average amino acid identity (AAI) was calculated using an AAI calculator server5 (Rodríguez-R and Konstantinidis, 2014). The percentage of conserved proteins (POCP) was estimated using an approach described by Qin et al. (Qin et al., 2014).
For the analysis of cellular fatty acids, cells were cultivated in MBC at 30°C and collected at the exponential phase. Fatty acid methyl esters were analyzed by gas chromatograph (Agilent 6,850 N) and identified using the Sherlock Microbial Identification System (MIDI, version 6.1) at Yellow Sea Fisheries Research Institute, Chinese Academy of Fishery Sciences (Qingdao, China). For the analysis of polar lipids and quinone, bacterial cells were cultivated in MBC at 30°C and collected at the early stationary phase. Polar lipids were extracted according to the method described by Komagata and Suzuki (Komagata and Suzuki, 1988) and analyzed using the two-dimensional thin layer chromatography (TLC) on silica gel 60 F254 plates (Merck, Germany) with appropriate spraying reagents including ethanolic molybdophosphoric acid (total lipids), ninhydrin (aminolipids) and molybdenum blue (phospholipids). The respiratory quinone of strain HB14T was extracted, separated and analyzed with the method described by Li et al. (Li et al., 2021a).
Cellular morphology was observed by transmission electron microscope (TEM, FEI Tecnai G2 F20) with strain HB14T cells from exponentially growing cultures. Colony morphology was observed after incubation on MAC at 30°C for 4 days. Gram staining and agar corrosion were carried out according to the method described by Tindall et al. (Tindall et al., 2007). Growth at different temperatures (4, 10, 15, 20, 25, 30, 37, 45 and 50°C) was determined in MBC. Growth at different NaCl concentrations [0, 1.0–15.0% (at an interval of 2.0% units), 16 and 17%; w/v] was determined in NP broth (Zhang et al., 2017) supplemented with 0.05% (w/v) cellobiose at 30°C. Growth tests for pH range [pH 5.0–10.0, at an interval of 0.5 pH units, buffered with MES (pH 5.0–6.0, 50 mM), MOPS (pH 6.5–7.0, 50 mM), Tris (pH 7.5–9.0, 50 mM) and CHES (pH 9.5–10.0, 50 mM)] were performed in NP broth supplemented with 0.05% (w/v) cellobiose and 3.0% (w/v) NaCl. Oxidase activity was tested by using commercial oxidase test strips (Tianhe Microorganism Reagent, China) according to the manufacturer’s instructions. Catalase activity was determined by bubble production in 3.0% (v/v) H2O2. Hydrolysis of casein (1.0%, w/v), starch (0.2%, w/v) and Tween 80 (1.0%, v/v) was examined by using MAC as the basal medium and incubation at 30°C. Cellulase activity was tested according to the method described by Kasana et al. (Kasana et al., 2008). Growth under the anaerobic condition was determined in MBC supplemented with potassium nitrate (0.1%, w/v), cysteine hydrochloride (0.05%, w/v) and sodium sulfide (0.05%, w/v) in Hungate tubes filled with oxygen-free N2 at 30°C for 2 weeks. Susceptibility to antibiotics was examined by the disk-diffusion method on MAC. More biochemical characteristics were determined using API ZYM and API 20NE strips (BioMérieux, France) following the manufacturers’ instructions with sea salts (3.0%, w/v, Sigma, United States) for cell suspension. Carbon utilization was determined using Biolog GEN III (Biolog, United States) according to the manufacturer’s instructions with inoculation fluid B for cell suspension.
1,3-xylan was prepared as previously described (Sun et al., 2021). Growth of strain HB14T on 1,3-xylan was determined at 30°C and pH 7.5 with 0.2% (w/v) 1,3-xylan as the sole carbon source by using 0.2% (w/v) xylose and glucose as the control substrates. The compositions of the medium used were as previously described (Sun et al., 2021). During cultivation, the optical density (OD) at 600 nm of the cultures was measured to generate growth curves.
During the cultivation of strain HB14T on 1,3-xylan, the cultures (1 ml) taken at an interval of 12 h were centrifuged (12,857 g at 4°C for 5 min), and the supernatants were collected for determining the extracellular 1,3-xylanase activity. To compare the extracellular 1,3-xylanase activities induced by 1,3-xylan, xylose and glucose, culture supernatants on each substrate at 84 h were collected and the extracellular 1,3-xylanase activities were determined separately. The extracellular 1,3-xylanase activity was measured by the dinitrosalicylic acid (DNS) method (Miller et al., 1960). The reaction system contained 10 μl culture supernatant and 90 μl 1,3-xylan (1.0%, w/v) in the phosphate-buffered saline (PBS, 20 mM, pH 7.0) and incubated at 30°C for 3.5 h. The reaction was terminated by the addition of 200 μl DNS. Then the reaction mixture was boiled at 100°C for 5 min and the OD550 nm was measured to quantify the released xylose. One unit of enzyme activity (1 U) is defined as the amount of enzyme required to release 1 μmol xylose per min.
The putative 1,3-xylanases of strain HB14T were predicted according to genomic annotations and dbCAN analyses (Yin et al., 2012). The signal peptides of 1,3-xylanases were predicted by using SignalP 5.0 (Armenteros et al., 2019). Domain analysis was performed in the NCBI conserved domain database (CDD; Marchler-Bauer et al., 2017) and the Pfam database (Mistry et al., 2021). The molecular weights of 1,3-xylanases were calculated by ExPASy.6 Multiple amino acid sequence alignment was carried out with CLC Sequence Viewer 6 and ESPript 3.0 (Robert and Gouet, 2014).
Strain HB14T was cultivated on 1,3-xylan at 30°C for 60 h, and then 20 ml of the culture was collected and centrifuged (12,857 g for 10 min at 4°C). The supernatant was moved into an ultrafiltration tube (molecular weight cut-off of 3 kDa) and centrifuged (4,476 g at 4°C) for concentration. Then, the concentrated sample (300 μl) was sent to Applied Protein Technology (Shanghai, China) for secretome analysis. In brief, the sample was reduced by dithiothreitol, alkylated by iodoacetamide and digested by trypsin. Then, peptides in the sample were desalted for liquid chromatography-mass spectroscopy/mass spectroscopy (LC–MS/MS) analysis. Finally, the raw data was analyzed by Thermo Scientific Proteome Discoverer™ 1.4 with the genomic data of strain HB14T as the database. The secretome data has been uploaded to the ProteomeXchange Consortium via the PRIDE under the accession number PXD035564.
The genes xyn65 and xyn80 without the signal peptide were cloned from the genomic DNA of strain HB14T by PCR amplification and inserted into the pET22b vector (Novagen, Germany). The primers used are shown in Supplementary Table S1. The constructed plasmids were transferred into E. coli BL21(DE3; Vazyme, China). The cells were cultivated in the Luria-Bertani (LB) medium containing 0.1 mg/ml ampicillin at 37°C and 180 rpm to OD600 ≈ 1.0 and then induced at 18°C and 120 rpm for 18 h with 0.5 mM isopropyl-β-D-thiogalactopyranoside (IPTG). The cells were harvested by centrifugation (11,500 g for 5 min at 4°C) and lysed in a lysis buffer [50 mM Tris–HCl, 100 mM NaCl, 0.5% (v/v) glycerol, pH 8.0] by a pressure crusher (JNBIO JN-02C). The recombinant proteins were purified by the nickel-nitrilotriacetic acid resin (GE Healthcare, United States) and then desalted by PD-10 columns (GE Healthcare, United States). Protein purity and apparent molecular weight were determined by SDS-PAGE. Protein concentration was determined by the bicinchoninic acid (BCA) method using a BCA protein assay kit (Thermo, United States).
The enzyme assays of Xyn65 and Xyn80 on xylans were determined by using the DNS method (vide supra). The standard reaction mixture contained 10 μl enzyme solution (36.8 μM for Xyn65 or 31.4 μM for Xyn80) and 90 μl xylan (1.0%, w/v) in PBS (20 mM, pH 7.0) and was incubated at 40°C for 10 min.
The substrate specificities of Xyn65 and Xyn80 were determined at 40°C in PBS (20 mM, pH 7.0) with 1,3-xylan, wheat arabinoxylan (Megazyme, Ireland), beechwood xylan (Megazyme, Ireland) and 1,3/1,4-xylan (Elicityl, France) as substrates at a concentration of 1.0% (w/v). The effect of temperature on enzyme activities was determined with 1,3-xylan as substrate in PBS (20 mM, pH 7.0) from 10°C to 60°C at an interval of 10°C. The effect of pH on enzyme activities was determined with 1,3-xylan as substrate at 40°C in the Britton-Robinson buffer from pH 3.0 to 10.0 at an interval of 1.0 pH units.
The reaction mixture containing 20 μl enzyme solution (36.8 μM for Xyn65 or 31.4 μM for Xyn80) and 180 μl 1,3-xylan (1.0%, w/v) in PBS (20 mM, pH 7.0) was incubated at 40°C. At 0 h, 1 h and 24 h of the reaction, aliquots (200 μl) were taken out, boiled for 5 min and analyzed by gel filtration on a Superdex 30 Increase 10/300 GL column (GE Healthcare, United States) using high-performance liquid chromatography (Shimadzu LC-20AT) equipped with an evaporative light scattering detector (Shimadzu ELSD LT II; HPLC-ELSD). The injected volume was 20 μl. The products were eluted with deionized water for 65 min with a flow rate of 0.3 ml/min. A mixture of xylose, 1,3-xylobiose and 1,3-xylotriose (5 mM each) was used as the external standard. 1,4-xylohexaose (5 mM) was used as the internal standard. 1,3-xylobiose and 1,3-xylotriose were prepared from 1,3-xylan hydrolyzed by 1,3-xylanase Xyl4 as described previously (Sun et al., 2021).
Strain HB14T was isolated from the surface of a marine green alga (Chaetomorpha sp.; Sun et al., 2021). The nearly full-length 16S rRNA gene sequence (1,496 bp, ON521705) of strain HB14T was obtained by PCR, which was identical to that extracted from its genomic sequence. HB14T showed the highest 16S rRNA gene sequence similarity to the type strain of G. chinensis (97.7%), followed by those of G. polysaccharolyticus (94.8%) and G. japonicus (93.7%), and less than 93.0% to the type strains of other species in the family Cellvibrionaceae, which were all below the threshold (98.7%) for bacterial species delineation (Kim et al., 2014). In the maximum-likelihood tree based on the 16S rRNA gene sequences, strain HB14T fell within the clade comprising known members of the genus Gilvimarinus (Figure 1) with high bootstrap support (91%), where it formed a well-supported (bootstrap value of 100%) peripheral branch together with G. chinensis. This stable topology was also supported by the neighbor-joining (Supplementary Figure S1) and maximum-parsimony trees (Supplementary Figure S2). These results suggested that strain HB14T belongs to the genus Gilvimarinus and represents a novel species within the genus.
Figure 1. Maximum-likelihood phylogenetic tree based on 16S rRNA gene sequences of strain HB14T (in bold) and the type strains of its closely related species. Bootstrap values (>70%) based on 1,000 replicates are shown at the branching points. Bar, 0.02 substitutions per nucleotide position. Limnobacter litoralis NBRC 105857T was used as the outgroup.
The assembled draft genome of strain HB14T (JAMFTH000000000) was 3,930,865 bp in length, with 13 contigs and an N50 value of 2,053,438 bp. According to the annotation by PGAP, a total of 3,427 genes were predicted, including 3,361 protein-coding genes, 49 RNA genes and 17 pseudogenes. The calculated genomic DNA G + C content of strain HB14T was 54.0 mol%, a value higher than that of G. chinensis CGMCC 1.7008T (51.2 mol%), the closest relative (Du et al., 2009). Further, the ANI and dDDH values between the genomes of strain HB14T and G. chinensis CGMCC 1.7008T were 77.1 and 21.8%, respectively, which were all significantly lower than the suggested cut-off values for ANI (95.0–96.0%) and dDDH (70.0%) used to discriminate bacterial species (Goris et al., 2007; Richter and Rosselló-Móra, 2009; Meier-Kolthoff et al., 2013). The AAI and POCP values between the genomes of strain HB14T and G. chinensis CGMCC 1.7008T were 78.9 and 77.9%, respectively, which were higher than the suggested cut-off values for AAI (76.0%) and POCP (50%) to delimit bacterial genera (Qin et al., 2014; Nicholson et al., 2020; Li et al., 2021b). Altogether, the genomic analyses indicated that strain HB14T represents a novel species of the genus Gilvimarinus, supporting our conclusion derived from the phylogenetic analysis based on 16S rRNA gene sequences.
The predominant cellular fatty acids (>10.0%) of strain HB14T were summed feature 3 (contains C16:1 ω6c and/or C16:1 ω7c), summed feature 8 (contains C18:1 ω7c and/or C18:1 ω6c) and C16:0, similar to those of G. chinensis CGMCC 1.7008T (Supplementary Table S2). The main polar lipids of strain HB14T were diphosphatidylglycerol, phosphatidylglycerol and phosphatidylethanolamine (Supplementary Figure S3). The predominant respiratory quinone of strain HB14T was ubiquinone-8, common for members of the family Cellvibrionaceae.
Cells of strain HB14T were Gram-stain-negative, rod-shaped and motile with a single polar flagellum (Figure 2A). Strain HB14T was resistant to cephalexin (30 μg), but sensitive to ampicillin (10 μg), carbenicillin (100 μg), chloramphenicol (30 μg), colistin sulphate (10 μg), erythromycin (15 μg), gentamicin (10 μg), neomycin (10 μg), novobiocin (5 μg), oleandomycin (15 μg), penicillin G (10 units), polymyxin B (300 units), streptomycin (10 μg), tetracycline (30 μg) and vancomycin (30 μg). The differential characteristics between strain HB14T and G. chinensis CGMCC 1.7008T were listed in Table 1. More detailed morphological, physiological and biochemical characteristics of strain HB14T were presented in the species description.
Figure 2. Morphological and genomic analysis of strain HB14T possessing strong polysaccharide degradation capabilities. (A) Cell morphological observation of strain HB14T by transmission electron micrograph. Strain HB14T cells for observation were incubated on MAC at 30°C for 3 days. (B) Quantification of the carbohydrate-active enzymes (CAZymes) and their family classifications encoded by the genome of strain HB14T. GH, glycoside hydrolase; PL, polysaccharide lyase; CE, carbohydrate esterase; CBM, carbohydrate-binding module.
Some Gilvimarinus strains have been reported to exhibit the capability to degrade marine algal polysaccharides, such as agar, cellulose, carrageenan and laminarin (Du et al., 2009; Kim et al., 2011; Cheng et al., 2015; Kouzui et al., 2016; Kouzuma and Fujii, 2018; Lee et al., 2018, 2021). Based on the genomic annotation and dbCAN analysis, the genome of strain HB14T encodes 120 GHs, 23 polysaccharide lyases (PL), 38 carbohydrate esterases (CE) and 57 carbohydrate-binding modules (CBM), distributed in 40, 14, 12 and 24 families, respectively (Figure 2B). These data suggested the potential capability of strain HB14T to degrade polysaccharides. Strain HB14T has been shown to form an apparent hydrolytic zone on the plate containing 1,3-xylan (Sun et al., 2021), suggesting that it can secrete 1,3-xylanase. To further investigate its 1,3-xylan-utilizing ability and extracellular 1,3-xylanase activity, the growth of strain HB14T was determined in a liquid medium with 1,3-xylan as the sole carbon source, with xylose and glucose as the control carbon sources. Strain HB14T was able to grow on 1,3-xylan, as well as xylose and glucose (Figure 3A), indicating that it could utilize 1,3-xylan for growth. In the culture supernatant of strain HB14T grown on 1,3-xylan, the 1,3-xylanase activity reached the highest level (0.14 ± 0.003 U/ml) at the late-log phase (Figure 3B). In contrast, no extracellular 1,3-xylanase activity was detected when strain HB14T was grown on xylose or glucose (Figure 3C), suggesting that the extracellular 1,3-xylanases were 1,3-xylan inducible.
Figure 3. Growth of strain HB14T on 1,3-xylan and the induction of extracellular 1,3-xylanase activity. (A) Growth curves of strain HB14T on 1,3-xylan, xylose and glucose. Strain HB14T was cultivated at 30°C and pH 7.5 with 0.2% (w/v) 1,3-xylan, xylose or glucose as the sole carbon source. The culture without carbon source was treated as the control. (B) The extracellular 1,3-xylanase activities during the cultivation of strain HB14T on 1,3-xylan. The 1,3-xylanase activities were determined at 30°C in PBS (pH 7.0). The extracellular 1,3-xylanase activity of strain HB14T cultivated without carbon source was treated as the control. (C), The extracellular 1,3-xylanase activity of strain HB14T grown on 1,3-xylan, xylose or glucose at the late-log phase. Cultures of strain HB14T at 84 h on each substrate of 1,3-xylan, xylose and glucose were collected separately. Then, the extracellular 1,3-xylanase activities of the culture were determined at 30°C in PBS (pH 7.0). The data shown in the graph are from triplicate experiments (mean ± S.D.).
The genome of strain HB14T encodes four putative 1,3-xylanases, which were named Xyn49, Xyn65, Xyn80 and Xyn89 (Table 2). All the four 1,3-xylanases have a signal peptide predicted by SignalP 5.0 (Figure 4), implying that they are likely secretory enzymes. Based on secretome analysis, the four putative 1,3-xylanases were all identified in the culture of strain HB14T cultivated with 1,3-xylan as the sole carbon source. Of these, Xyn65 was the most abundant (82.3%), followed by Xyn80 (12.0%), Xyn49 (4.5%) and Xyn89 (1.2%; Table 2). The results suggested that these 1,3-xylanases were all likely involved in the extracellular degradation of 1,3-xylan of strain HB14T, and that Xyn65 might be the most important one.
Table 2. Information of the extracellular 1,3-xylanases secreted by strain HB14T identified by secretome analysis.
Figure 4. The domain architectures of the four 1,3-xylanases secreted by strain HB14T. Signal peptides were predicted by SignalP 5.0. Domain analysis was performed in the NCBI CDD database and the Pfam database. Domains were illustrated in different colors based on their functional annotations. Big, bacterial immunoglobulin (Ig)-like domain; DHOR, di-haem oxidoreductase superfamily.
Several 1,3-xylanases have been reported, which are derived from marine Vibrio (Araki et al., 2000; Kiyohara et al., 2005), Alcaligenes (Okazaki et al., 2002), Pseudomonas (Aoki and Kamei, 2006; Liang et al., 2015), Thermotoga (Okazaki et al., 2013) and Flammeovirga (Cai et al., 2018; Yi et al., 2020). They are generally modular proteins containing one GH26 catalytic domain and one or more CBMs, mainly belonging to CBM6 and CBM31 (Table 3). The function of the CBMs is to bind insoluble 1,3-xylan and facilitate the hydrolysis of 1,3-xylan (Okazaki et al., 2002). In addition to modular members, Xyn26A and Xyl512 are two single-domain 1,3-xylanases, consisting of only one GH26 catalytic domain (Okazaki et al., 2013; Cai et al., 2018). Among the extracellular 1,3-xylanases of strain HB14T, according to domain architecture prediction, Xyn49 and Xyn89 are single-domain enzymes, and Xyn65 and Xyn80 are modular enzymes (Figure 4). They all have a GH26 catalytic domain (Figure 4), which exhibits top amino acid sequence identities (30.0–56.0%) with the previously reported 1,3-xylanase Xyl4 (Kiyohara et al., 2005; Table 2). The catalytic domain of Xyl4 have been structurally investigated with two glutamate residues as the catalytic residues (Goddard-Borger et al., 2012). Multiple sequence alignment indicated that the two residues are conserved in these four 1,3-xylanases secreted by strain HB14T (Supplementary Figure S4), suggesting a similar catalysis mechanism. In addition to the GH26 domain, Xyn65 and Xyn80 also possess a CBM31 and a CBM6 (Figure 4). Moreover, Xyn65 also contains a bacterial immunoglobulin (Ig)-like domain (Big) and a domain belonging to the di-haem oxidoreductase (DHOR) superfamily. To our knowledge, the domain architecture of Xyn65 is distinct from any previously reported 1,3-xylanase (Figure 4). Big domains are commonly distributed in GHs, and may have different functions, such as binding carbohydrates (Xing et al., 2022), stabilizing the catalytic domain (Liu et al., 2010) and serving as a thermostabilizing module (da Silva et al., 2020). DHOR domains appear to act as di-heme oxidoreductases with probable peroxidase activity (Goonesekere et al., 2010), which, however, have not been found in GHs. We hypothesize that in Xyn65 (1) the DHOR domain may enable Xyn65 a novel activity (e.g., oxidoreductase activity) on 1,3-xylan; (2) although DHOR shows sequence similarities to di-heme oxidoreductases, it may evolve new functions (e.g., binding 1,3-xylan or stabilizing Xyn65 structure). Thus, Xyn65 is likely a new GH26 member with a novel structure, which awaits further investigation.
To characterize the 1,3-xylanases secreted by strain HB14T, we attempted to express Xyn49, Xyn65, Xyn80 and Xyn89 in E. coli, but this was only successful in Xyn65 and Xyn80. SDS-PAGE analysis showed that the purified recombinant Xyn65 and Xyn80 exhibited apparent molecular weights of approximately 180 kDa and 80 kDa, respectively (Figure 5A), consistent with their theoretical molecular weights (Table 2). Substrate specificity analysis showed that Xyn65 and Xyn80 hydrolyzed only 1,3-xylan, with specific activities of 76.9 ± 4.7 U/μmol and 91.9 ± 0.6 U/μmol, respectively (Table 4), indicating that they are strict 1,3-xylanases. Both 1,3-xylanases displayed the highest activity at 40°C and pH 6.0–7.0 (Figures 5B–E). During the hydrolysis of 1,3-xylan by Xyn65 and Xyn80, 1,3-xylooligosaccharides with degrees of polymerization (DP) from one to four were detected, with 1,3-xylobiose and 1,3-xylotriose as the major final products (Figure 6), suggesting the endo-action pattern of both enzymes on 1,3-xylan. Together, Xyn65 and Xyn80 are two strict endo-1,3-xylanases with similar enzymatic characteristics.
Figure 5. SDS-PAGE analysis and biochemical characterization of recombinant 1,3-xylanases Xyn65 and Xyn80. (A) SDS-PAGE analysis of Xyn65 and Xyn80. The enzyme bands are indicated by red arrows. M, protein molecular weight marker. (B) Effect of temperature on Xyn65 activity. (C) Effect of pH on Xyn65 activity. (D) Effect of temperature on Xyn80 activity. (E), Effect of pH on Xyn80 activity. In B and D, the 1,3-xylanase activities were determined in PBS (pH 7.0) from 10°C to 60°C. In C and E, the 1,3-xylanase activities were determined in the Britton-Robinson buffer with different pH values (pH 3.0–10.0). The data shown in the graph are from triplicate experiments (mean ± S.D.).
Figure 6. Time-course analysis of the 1,3-xylan degradation by recombinant 1,3-xylanases Xyn65 (A) and Xyn80 (B). Xyn65 and Xyn80 were separately incubated with 1,3-xylan at 40°C in PBS (pH 7.0). At 0 h, 1 h and 24 h, the reaction was terminated by boiling for 5 min and the products were analyzed by HPLC-ELSD. A mixture of xylose, 1,3-xylobiose (1,3X2) and 1,3-xylotriose (1,3X3) was used as the external standard. 1,4-xylohexaose (1,4X6) was used as the internal standard. The data are representatives of the results of triplicate experiments.
All previously reported 1,3-xylanases are neutral enzymes, displaying the highest activity at pH 6.5–8.0 (Araki et al., 2000; Okazaki et al., 2002; Kiyohara et al., 2005; Aoki and Kamei, 2006; Okazaki et al., 2013; Liang et al., 2015; Cai et al., 2018; Yi et al., 2020). Except the cold-adapted Xyl512 (Cai et al., 2018) and the thermostable Xyn26A (Okazaki et al., 2013), the other 1,3-xylanases are all mesophilic enzymes. Their major products released from 1,3-xylan are generally xylose, 1,3-xylobiose, 1,3-xylotriose and 1,3-xylotetraose (Table 3). Similarly, Xyn65 and Xyn80 function at neutral pH and mesophilic conditions, releasing mainly 1,3-xylobiose and 1,3-xylotriose from 1,3-xylan. 1,3-xylanase is an effective tool in the conversion of 1,3-xylan and the produced 1,3-xylooligosaccharides could be the important ingredients in some functional foods (Cai et al., 2018). Although the specific activities of Xyn65 (76.9 ± 4.7 U/μmol) and Xyn80 (91.9 ± 0.6 U/μmol) are not very high compared with those of the reported 1,3-xylanases (86.7 ~ 4,933.2 U/μmol; Table 3), both enzymes released mainly 1,3-xylooligosaccharides from 1,3-xylan with a little xylose (Figure 6). They are two newly discovered candidates for the conversion of 1,3-xylan into 1,3-xylooligosaccharides, the potentials of which need further exploration.
In this study, a taxonomic study was carried out on a 1,3-xylanase-secreting bacterium, strain HB14T, isolated from a marine green alga. Phylogenetic and genomic analyses indicated that strain HB14T should be assigned to the genus Gilvimarinus. Strain HB14T exhibits the typical characteristics of the genus Gilvimarinus, such as Gram-stain-negative, rod-shaped, oxidase- and catalase-positive, motile with a single polar flagellum, having ubiquinone-8 as the sole respiratory quinone, and having diphosphatidylglycerol, phosphatidylglycerol and phosphatidylethanolamine as the major polar lipids. However, strain HB14T can be distinguished from its most closely related species G. chinensis by several phenotypic characteristics, such as its abilities to grow with 15.0% NaCl, hydrolyze urea and reduce nitrate, and its inabilities to grow at 4°C or corrode agar. Thus, based on the phylogenetic, genomic, chemotaxonomic and phenotypic analyses, strain HB14T represents a new species of the genus Gilvimarinus, for which the name Gilvimarinus xylanilyticus sp. nov. is proposed. Furthermore, the 1,3-xylanases secreted by strain HB14T were found to be 1,3-xylan inducible, and four 1,3-xylanases were identified in the secretome. Among them, Xyn65 is the most abundant and has a novel domain architecture distinct from other 1,3-xylanases. Xyn65 and Xyn80 were biochemically characterized to be two endo-1,3-xylanases. They exhibited the highest activity at neutral pH and mesophilic conditions and released mainly 1,3-xylobiose and 1,3-xylotriose from 1,3-xylan, suggesting that they may have a potential in 1,3-xylooligosaccharides preparation. This is the first report on the identification and characterization of 1,3-xylanases derived from Gilvimarinus, which indicates that Gilvimarinus may play a role in 1,3-xylan degradation in the ocean.
Gilvimarinus xylanilyticus (xy.la.ni.ly’ti.cus. N.L. neut. adj. xylanum, xylan; N.L. masc. adj. lyticus, dissolving; from Gr. masc. adj. lytikos, dissolving; N.L. masc. adj. xylanilyticus, hydrolyzing xylan).
Cells are Gram-stain-negative, aerobic, rod-shaped (approximately 0.3–0.7 μm wide and 1.1–2.7 μm long) and motile with a single polar flagellum. Colonies are circular with regular edges, slightly convex, opaque and approximately 0.5–1.8 mm in diameter on MAC 4 days-incubation at 30°C. Growth occurs at 10–40°C (optimum, 30°C) and pH 6.0–9.0 (optimum, 7.5) with 1.0–15.0% NaCl (optimum, 3.0–5.0%). Cells are positive for oxidase, catalase, 1,3-xylanase, amylase and cellulase, but could not hydrolyze casein and Tween 80. In the API ZYM strips, cells are positive for alkaline phosphatase, esterase (C4), esterase lipase (C8), leucine arylamidase, valine arylamidase, cystine arylamidase, acid phosphatase, naphthol-AS-B1-phosphophydrolase, α-galactosidase, β-galactosidase, α-glucosidase, N-acetyl-β-glucosaminidase, but negative for lipase (C14), trypsin, α-chymotrypsin, β-glucuronidase, β-glucosidase, α-mannosidase and α-fucosidase. In API 20 NE tests, cells are positive for nitrate reduction, urease and aesculin hydrolysis, but negative for indole production, acid production from glucose, arginine dihydrolase, β-galactosidase, gelatine hydrolysis, or assimilation of glucose, arabinose, mannose, mannitol, N-acetyl-glucosamine, maltose, gluconate, caprate, adipate, malate, citrate and phenyl-acetate. On Biolog GEN III microplates (7 days), dextrin, maltose, trehalose, cellobiose, gentiobiose, α-D-lactose, melibiose, methyl-β-D-glucoside, D-salicin, α-D-glucose, glucuronamide and L-malic acid are oxidized; turanose, raffinose, D-mannose, D-fructose, D-galactose, D-fucose, L-fucose, L-rhamnose, D-glucose-6-phosphate, D-fructose-6-phosphate, L-glutamic acid, L-histidine, D-galacturonic acid, L-galactonic acid lactone, D-gluconic acid, D-glucuronic acid, α-ketoglutaric acid, acetoacetic acid and acetic acid are weakly oxidized; sucrose, stachyose, N-acetyl-D-glucosamine, N-acetyl-β-D-mannosamine, N-acetyl-D-galactosamine, N-acetyl neuraminic acid, 3-methyl glucose, inosine, D-sorbitol, D-mannitol, D-arabitol, myo-inositol, glycerol, D-aspartic acid, D-serine, gelatin, glycyl-L-proline, L-alanine, L-arginine, L-aspartic acid, L-pyroglutamic acid, L-serine, pectin, mucic acid, quinic acid, D-saccharic acid, p-hydroxyphenylacetic acid, methyl pyruvate, D-lactic acid methyl ester, L-lactic acid, citric acid, D-malic acid, bromosuccinic acid, Tween 40, γ-aminobutryric acid, α-hydroxybutyric acid, β-hydroxy-D,L-butyric acid, α-ketobutyric acid, propionic acid and formic acid are not oxidized. The predominant cellular fatty acids (>10.0%) are summed feature 3 (contains C16:1 ω6c and/or C16:1 ω7c), summed feature 8 (contains C18:1 ω7c and/or C18:1 ω6c) and C16:0. The predominant respiratory quinone is ubiquinone-8 and the main polar lipids are diphosphatidylglycerol, phosphatidylglycerol and phosphatidylethanolamine.
The type strain is HB14T (=CCTCC AB 2022109T = KCTC 92379T) isolated from a marine green alga (Chaetomorpha sp.) collected from Hainan, China. The genomic G + C content of the type strain (calculated from the draft genome) is 54.0 mol%.
The datasets presented in this study can be found in online repositories. The names of the repository/repositories and accession number(s) can be found at: https://www.ncbi.nlm.nih.gov/genbank/, ON521705 https://www.ncbi.nlm.nih.gov/genome/, JAMFTH000000000, and https://www.ebi.ac.uk/pride, PXD035564.
Y-JZ and FZ designed and directed the research. Y-JZ, H-NS, T-TX, and C-MY performed the experiments. Y-JZ, FZ, Y-QZ, and H-NS wrote the manuscript. D-LZ and YZ helped in data analysis. X-LC and X-YZ revised the manuscript. All authors contributed to the article and approved the submitted version.
The work was supported by the National Science Foundation of China (U2006205, 42076151, and 41906195, awarded to X-LC, X-YZ, and YZ, respectively), the Natural Science Foundation of Shandong Province, China (ZR2021QC024, awarded to FZ), and the China Postdoctoral Science Foundation (2021 M691960, awarded to Y-QZ).
We thank Xiang-Mei Ren (State Key Laboratory of Microbial Technology, Shandong University, China) for her help and guidance in HPLC-ELSD. We thank Xiao-Ju Li (State Key Laboratory of Microbial Technology, Shandong University, China) and Mei-Ling Sun (College of Marine Life Sciences, Ocean University of China) for their help in TEM. We thank Jing-Yao Qu, Jing Zhu and Zhi-Feng Li from the State Key Laboratory of Microbial Technology, Shandong University, China for the help and guidance in LC–MS. We thank Guang-Ya Zhang (Department of Biotechnology and Bioengineering, Huaqiao University, China) for technical help in 1,3-xylan extraction.
The authors declare that the research was conducted in the absence of any commercial or financial relationships that could be construed as a potential conflict of interest.
All claims expressed in this article are solely those of the authors and do not necessarily represent those of their affiliated organizations, or those of the publisher, the editors and the reviewers. Any product that may be evaluated in this article, or claim that may be made by its manufacturer, is not guaranteed or endorsed by the publisher.
The Supplementary material for this article can be found online at: https://www.frontiersin.org/articles/10.3389/fmicb.2022.1006116/full#supplementary-material
2. ^http://www.ezbiocloud.net/
3. ^http://ggdc.dsmz.de/ggdc.php
4. ^https://www.ezbiocloud.net/tools/ani
Aoki, Y., and Kamei, Y. (2006). Preparation of recombinant polysaccharide-degrading enzymes from the marine bacterium, Pseudomonas sp. ND137 for the production of protoplasts of Porphyra yezoensis. Eur. J. Phycol. 41, 321–328. doi: 10.1080/09670260600801682
Araki, T., Hashikawa, S., and Morishita, T. (2000). Cloning, sequencing, and expression in Escherichia coli of the new gene encoding beta-1,3-xylanase from a marine bacterium, Vibrio sp. strain XY-214. Appl. Environ. Microbiol. 66, 1741–1743. doi: 10.1128/AEM.66.4.1741-1743.2000
Araki, T., Tani, S., Maeda, K., Hashikawa, S., Nakagawa, H., and Morishita, T. (1999). Purification and characterization of beta-1,3-xylanase from a marine bacterium, Vibrio sp. XY-214. Biosci. Biotechnol. Biochem. 63, 2017–2019. doi: 10.1271/bbb.63.2017
Armenteros, J. J. A., Tsirigos, K. D., Sonderby, C. K., Petersen, T. N., Winther, O., Brunak, S., et al. (2019). SignalP 5.0 improves signal peptide predictions using deep neural networks. Nat. Biotechnol. 37, 420–423. doi: 10.1038/s41587-019-0036-z
Brettin, T., Davis, J. J., Disz, T., Edwards, R. A., Gerdes, S., Olsen, G. J., et al. (2015). Rasttk: a modular and extensible implementation of the Rast algorithm for building custom annotation pipelines and annotating batches of genomes. Sci. Rep. 5:8365. doi: 10.1038/srep08365
Cai, Z. W., Ge, H. H., Yi, Z. W., Zeng, R. Y., and Zhang, G. Y. (2018). Characterization of a novel psychrophilic and halophilic beta-1,3-xylanase from deep-sea bacterium, Flammeovirga pacifica strain WPAGA1. Int. J. Biol. Macromol. 118, 2176–2184. doi: 10.1016/j.ijbiomac.2018.07.090
Cheng, H., Zhang, S., Huo, Y. Y., Jiang, X. W., Zhang, X. Q., Pan, J., et al. (2015). Gilvimarinus polysaccharolyticus sp. nov., an agar-digesting bacterium isolated from seaweed, and emended description of the genus Gilvimarinus. Int. J. Syst. Evol. Microbiol. 65, 562–569. doi: 10.1099/ijs.0.065078-0
da Silva, V. M., Cabral, A. D., Speranca, M. A., Squina, F. M., Muniz, J. R. C., Martin, L., et al. (2020). High-resolution structure of a modular hyperthermostable endo-beta-1,4-mannanase from Thermotoga petrophila: the ancillary immunoglobulin-like module is a thermostabilizing domain. Biochim. Biophys. Acta, Proteins Proteomics 1868:140437. doi: 10.1016/j.bbapap.2020.140437
Du, Z. J., Zhang, D. C., Liu, S. N., Chen, J. X., Tian, X. L., Zhang, Z. N., et al. (2009). Gilvimarinus chinensis gen. Nov., sp. nov., an agar-digesting marine bacterium within the class Gammaproteobacteria isolated from coastal seawater in Qingdao, China. Int. J. Syst. Evol. Microbiol. 59, 2987–2990. doi: 10.1099/ijs.0.001313-0
Felsenstein, J. (1981). Evolutionary trees from DNA sequences: a maximum likelihood approach. J. Mol. Evol. 17, 368–376. doi: 10.1007/bf01734359
Fitch, W. M. (1971). Toward defining the course of evolution: minimum change for a specific tree topology. Syst. Zool. 20, 406–416. doi: 10.2307/2412116
Goddard-Borger, E. D., Sakaguchi, K., Reitinger, S., Watanabe, N., Ito, M., and Withers, S. G. (2012). Mechanistic insights into the 1,3-xylanases: useful enzymes for manipulation of algal biomass. J. Am. Chem. Soc. 134, 3895–3902. doi: 10.1021/ja211836t
Goonesekere, N. C., Shipely, K., and O'Connor, K. (2010). The challenge of annotating protein sequences: the tale of eight domains of unknown function in Pfam. Comput. Biol. Chem. 34, 210–214. doi: 10.1016/j.compbiolchem.2010.04.001
Goris, J., Konstantinidis, K. T., Klappenbach, J. A., Coenye, T., Vandamme, P., and Tiedje, J. M. (2007). DNA-DNA hybridization values and their relationship to whole-genome sequence similarities. Int. J. Syst. Evol. Microbiol. 57, 81–91. doi: 10.1099/ijs.0.64483-0
Hsieh, Y. S. Y., and Harris, P. J. (2019). Xylans of red and green algae: what is known about their structures and how they are synthesised? Polymers 11:354. doi: 10.3390/polym11020354
Iriki, Y., Suzuki, T., Nisizawa, K., and Miwa, T. (1960). Xylan of siphonaceous green algae. Nature 187, 82–83. doi: 10.1038/187082a0
Kasana, R. C., Salwan, R., Dhar, H., Dutt, S., and Gulati, A. (2008). A rapid and easy method for the detection of microbial cellulases on agar plates using gram's iodine. Curr. Microbiol. 57, 503–507. doi: 10.1007/s00284-008-9276-8
Kim, B. C., Kim, M. N., Lee, K. H., Kim, H. S., Min, S. R., and Shin, K. S. (2011). Gilvimarinus agarilyticus sp. nov., a new agar-degrading bacterium isolated from the seashore of Jeju Island. Antonie Van Leeuwenhoek 100, 67–73. doi: 10.1007/s10482-011-9565-2
Kim, M., Oh, H. S., Park, S. C., and Chun, J. (2014). Towards a taxonomic coherence between average nucleotide identity and 16S rRNA gene sequence similarity for species semarcation of prokaryotes. Int. J. Syst. Evol. Microbiol. 64, 346–351. doi: 10.1099/ijs.0.059774-0
Kiyohara, M., Sakaguchi, K., Yamaguchi, K., Araki, T., Nakamura, T., and Ito, M. (2005). Molecular cloning and characterization of a novel beta-1,3-xylanase possessing two putative carbohydrate-binding modules from a marine bacterium Vibrio sp. strain AX-4. Biochem. J. 388, 949–957. doi: 10.1042/BJ20050190
Komagata, K., and Suzuki, K. I. (1988). Lipid and cell-wall analysis in bacterial systematics. Methods Microbiol. 19, 161–207. doi: 10.1016/s0580-9517(08)70410-0
Kouzui, H., Tokikawa, K., Satomi, M., Negoro, T., Shimabukuro, K., and Fujii, K. (2016). Gilvimarinus japonicus sp. nov., a cellulolytic and agarolytic marine bacterium isolated from coastal debris. Int. J. Syst. Evol. Microbiol. 66, 5417–5423. doi: 10.1099/ijsem.0.001534
Kouzuma, S., and Fujii, K. (2018). Biochemical characteristics of cellulose and a green alga degradation by Gilvimarinus japonicas 12-2T, and its application potential for seaweed saccharification. Biosci. Biotechnol. Biochem. 82, 2198–2204. doi: 10.1080/09168451.2018.1516542
Lee, Y., Jo, E., Lee, Y. J., Eom, T. Y., Gang, Y., Kang, Y. H., et al. (2021). A novel agarase, Gaa16B, isolated from the marine bacterium Gilvimarinus agarilyticus JEA5, and the moisturizing effect of its partial hydrolysis products. Mar. Drugs 20:2. doi: 10.3390/md20010002
Lee, Y., Jo, E., Lee, Y. J., Hettiarachchi, S. A., Park, G. H., Lee, S. J., et al. (2018). A novel glycosyl hydrolase family 16 β-agarase from the agar-utilizing marine bacterium Gilvimarinus agarilyticus JEA5: the first molecular and biochemical characterization of agarase in genus Gilvimarinus. J. Microbiol. Biotechnol. 28, 776–783. doi: 10.4014/jmb.1709.09050
Li, J., Cheng, J. H., Teng, Z. J., Sun, Z. Z., He, X. Y., Wang, P., et al. (2021a). Taxonomic and enzymatic characterization of Flocculibacter collagenilyticus gen. Nov., sp. nov., a novel Gammaproteobacterium with high collagenase production. Front. Microbiol. 12:621161. doi: 10.3389/fmicb.2021.621161
Li, M., Hou, L. Z., Xu, X. H., Wang, X. X., Zheng, M. C., Zhang, Y. J., et al. (2021b). Phylogenomic analyses of a clade within the family Flavobacteriaceae suggest taxonomic reassignments of species of the genera Algibacter, Hyunsoonleella, Jejuia, and Flavivirga, and the proposal of Pseudalgibacter gen. Nov. and Pseudalgibacter alginicilyticus comb. nov. Curr. Microbiol. 78, 3277–3284. doi: 10.1007/s00284-021-02559-w
Liang, W. S., Fang, T. Y., Lin, H. T., Liu, T. C., Lu, W. J., Tzou, W. S., et al. (2015). Cloning, expression, and characterization of Pseudomonas vesicularis MA103 beta-1,3-xylanase in Escherichia coli Clearcoli BL21(DE3). Fish. Sci. 81, 1135–1143. doi: 10.1007/s12562-015-0933-0
Liu, H. B., Pereira, J. H., Adams, P. D., Sapra, R., Simmons, B. A., and Sale, K. L. (2010). Molecular simulations provide new insights into the role of the accessory immunoglobulin-like domain of Cel9A. FEBS Lett. 584, 3431–3435. doi: 10.1016/j.febslet.2010.06.041
Lombard, V., Ramulu, H. G., Drula, E., Coutinho, P. M., and Henrissat, B. (2014). The carbohydrate-active enzymes database (CAZy) in 2013. Nucleic Acids Res. 42, D490–D495. doi: 10.1093/nar/gkt1178
Maeda, R., Ida, T., Ihara, H., and Sakamoto, T. (2012). Induction of apoptosis in MCF-7 cells by beta-1,3-xylooligosaccharides prepared from Caulerpa lentillifera. Biosci. Biotechnol. Biochem. 76, 1032–1034. doi: 10.1271/bbb.120016
Marchler-Bauer, A., Bo, Y., Han, L., He, J., Lanczycki, C. J., Lu, S., et al. (2017). CDD/SPARCLE: functional classification of proteins via subfamily domain architectures. Nucleic Acids Res. 45, D200–D203. doi: 10.1093/nar/gkw1129
Meier-Kolthoff, J. P., Auch, A. F., Klenk, H. P., and Gker, M. (2013). Genome sequence-basedspecies delimitation with confidence intervals and improved distance functions. BMC Bioinformatics 14:60. doi: 10.1186/1471-2105-14-60
Miller, G. L., Blum, R., Glennon, W. E., and Burton, A. L. (1960). Measurement of carboxymethylcellulase activity. Anal. Biochem. 1, 127–132. doi: 10.1016/0003-2697(60)90004-X
Mistry, J., Chuguransky, S., Williams, L., Qureshi, M., Salazar, G. A., Sonnhammer, E. L. L., et al. (2021). Pfam: the protein families database in 2021. Nucleic Acids Res. 49, D412–D419. doi: 10.1093/nar/gkaa913
Nicholson, A. C., Gulvik, C. A., Whitney, A. M., Humrighouse, B. W., Bell, M. E., Holmes, B., et al. (2020). Division of the genus Chryseobacterium: observation of discontinuities in amino acid identity values, a possible consequence of major extinction events, guides transfer of nine species to the genus Epilithonimonas, eleven species to the genus Kaistella, and three species to the genus Halpernia gen. Nov., with description of Kaistella daneshvariae sp. nov. and Epilithonimonas vandammei sp. nov. derived from clinical specimens. Int. J. Syst. Evol. Microbiol. 70, 4432–4450. doi: 10.1099/ijsem.0.003935
Okazaki, F., Nakashima, N., Ogino, C., Tamaru, Y., and Kondo, A. (2013). Biochemical characterization of a thermostable beta-1,3-xylanase from the hyperthermophilic eubacterium, Thermotoga neapolitana strain DSM 4359. Appl. Microbiol. Biotechnol. 97, 6749–6757. doi: 10.1007/s00253-012-4555-5
Okazaki, F., Tamaru, Y., Hashikawa, S., Li, Y. T., and Araki, T. (2002). Novel carbohydrate-binding module of beta-1,3-xylanase from a marine bacterium, Alcaligenes sp. strain XY-234. J. Bacteriol. 184, 2399–2403. doi: 10.1128/JB.184.9.2399-2403.2002
Qeshmi, F. I., Homaei, A., Fernandes, P., Hemmati, R., Dijkstra, B. W., and Khajeh, K. (2020). Xylanases from marine microorganisms: a brief overview on scope, sources, features and potential applications. Biochim. Biophys. Acta, Proteins Proteomics 1868:140312. doi: 10.1016/j.bbapap.2019.140312
Qin, Q. L., Xie, B. B., Zhang, X. Y., Chen, X. L., Zhou, B. C., Zhou, J., et al. (2014). A proposed genus boundary for the prokaryotes based on genomic insights. J. Bacteriol. 196, 2210–2215. doi: 10.1128/jb.01688-14
Richter, M., and Rosselló-Móra, R. (2009). Shifting the genomic gold standard for the prokaryotic species definition. Proc. Natl. Acad. Sci. U. S. A. 106, 19126–19131. doi: 10.1073/pnas.0906412106
Robert, X., and Gouet, P. (2014). Deciphering key features in protein structures with the new ENDscript server. Nucleic Acids Res. 42, W320–W324. doi: 10.1093/nar/gku316
Rodríguez-R, L. M., and Konstantinidis, K. T. (2014). Bypassing cultivation to identify bacterial species: culture-independent genomic approaches identify credibly distinct clusters, avoid cultivation bias, and provide true insights into microbial species. Microbe Magazine 9, 111–118. doi: 10.1128/microbe.9.111.1
Saitou, N., and Nei, M. (1987). The neighbor-joining method: a new method for reconstructing phylogenetic trees. Mol. Biol. Evol. 4, 406–425. doi: 10.1093/oxfordjournals.molbev.a040454
Sun, H. N., Yu, C. M., Fu, H. H., Wang, P., Fang, Z. G., Zhang, Y. Z., et al. (2021). Diversity of marine 1,3-xylan-utilizing bacteria and characters of their extracellular 1,3-xylanases. Front. Microbiol. 12:721422. doi: 10.3389/fmicb.2021.721422
Tamura, K., Stecher, G., and Kumar, S. (2021). MEGA 11: molecular evolutionary genetics analysis version 11. Mol. Biol. Evol. 38, 3022–3027. doi: 10.1093/molbev/msab120
Tatusova, T., DiCuccio, M., Badretdin, A., Chetvernin, V., Nawrocki, E. P., Zaslavsky, L., et al. (2016). NCBI prokaryotic genome annotation pipeline. Nucleic Acids Res. 44, 6614–6624. doi: 10.1093/nar/gkw569
Tindall, B. J., Sikorski, J., Smibert, R. A., and Krieg, N. R. (2007). “Phenotypic characterization and the principles of comparative systematics” in In Methods for General and Molecular Microbiology. eds. C. A. Reddy, T. J. Beveridge, J. A. Breznak, G. A. Marzluf, T. M. Schmidt, and L. R. Snyder (Washington, FL: ASM Press), 330–393.
Umemoto, Y., Shibata, T., and Araki, T. (2012). D-xylose isomerase from a marine bacterium, Vibrio sp. strain XY-214, and D-xylulose production from beta-1,3-xylan. Mar. Biotechnol. 14, 10–20. doi: 10.1007/s10126-011-9380-9
Veluraja, K., and Atkins, E. D. T. (1987). Helical structure of beta(1-3) xylan and the water mediated hydrogen-bonding schemes. Carbohydr. Polym. 7, 133–141. doi: 10.1016/0144-8617(87)90055-5
Weisburg, W. G., Barns, S. M., Pelletier, D. A., and Lane, D. J. (1991). 16S ribosomal DNA amplification for phylogenetic study. J. Bacteriol. 173, 697–703. doi: 10.1128/jb.173.2.697-703.1991
Xing, M., Wang, Y., Zhao, Y., Chi, Z., Chi, Z., and Liu, G. (2022). C-terminal bacterial immunoglobulin-like domain of kappa-carrageenase derves as a multifunctional module to promote kappa-carrageenan hydrolysis. J. Agric. Food Chem. 70, 1212–1222. doi: 10.1021/acs.jafc.1c07233
Yi, Z., Cai, Z., Zeng, B., Zeng, R., and Zhang, G. (2020). Identification and characterization of a novel thermostable and salt-tolerant beta-1,3-xylanase from Flammeovirga pacifica strain WPAGA1. Biomol. Ther. 10:1287. doi: 10.3390/biom10091287
Yin, Y., Mao, X., Yang, J., Chen, X., Mao, F., and Xu, Y. (2012). dbCAN: a web resource for automated carbohydrate-active enzyme annotation. Nucleic Acids Res. 40, W445–W451. doi: 10.1093/nar/gks479
Yoon, S. H., Ha, S. M., Kwon, S., Lim, J., Kim, Y., Seo, H., et al. (2017a). Introducing EzBioCloud: a taxonomically united database of 16S rRNA gene sequences and whole-genome assemblies. Int. J. Syst. Evol. Microbiol. 67, 1613–1617. doi: 10.1099/ijsem.0.001755
Yoon, S. H., Ha, S. M., Lim, J., Kwon, S., and Chun, J. (2017b). A large-scale evaluation of algorithms to calculate average nucleotide identity. Antonie Van Leeuwenhoek 110, 1281–1286. doi: 10.1007/s10482-017-0844-4
Keywords: Gilvimarinus, polyphasic taxonomy, sp. nov., 1,3-xylanase-secreting bacteria, 1,3-xylanases
Citation: Zhang Y-J, Sun H-N, Xu T-T, Zhao D-L, Yu C-M, Zhang Y, Zhang X-Y, Chen X-L, Zhang Y-Q and Zhao F (2022) Gilvimarinus xylanilyticus sp. nov., a novel 1,3-xylanase-secreting bacterium isolated from a marine green alga. Front. Microbiol. 13:1006116. doi: 10.3389/fmicb.2022.1006116
Received: 20 August 2022; Accepted: 07 October 2022;
Published: 24 October 2022.
Edited by:
Xiaozhen Jen Mou, Kent State University, United StatesReviewed by:
Jialing Li, Sun Yat-sen University, ChinaCopyright © 2022 Zhang, Sun, Xu, Zhao, Yu, Zhang, Zhang, Chen, Zhang and Zhao. This is an open-access article distributed under the terms of the Creative Commons Attribution License (CC BY). The use, distribution or reproduction in other forums is permitted, provided the original author(s) and the copyright owner(s) are credited and that the original publication in this journal is cited, in accordance with accepted academic practice. No use, distribution or reproduction is permitted which does not comply with these terms.
*Correspondence: Fang ZhaoemZhbmc5OTJAMTYzLmNvbQ==">,emZhbmc5OTJAMTYzLmNvbQ==; Yu-Qiang Zhang, eXF6aDE5ODlAc2R1LmVkdS5jbg==
†These authors have contributed equally to this work and share first authorship
Disclaimer: All claims expressed in this article are solely those of the authors and do not necessarily represent those of their affiliated organizations, or those of the publisher, the editors and the reviewers. Any product that may be evaluated in this article or claim that may be made by its manufacturer is not guaranteed or endorsed by the publisher.
Research integrity at Frontiers
Learn more about the work of our research integrity team to safeguard the quality of each article we publish.