- Department of Analytical Development, Center of Excellence, BioGaia AB, Eslöv, Sweden
The interest in probiotics has increased rapidly the latest years together with the global market for probiotic products. Consequently, establishing reliable microbiological methods for assuring the presence of a certain number of viable microorganisms in probiotic products has become increasingly important. To assure adequate numbers of viable cells, authorities are enquiring for information on viability rates within a certain shelf-life in colony forming units (CFU). This information is obtained from plate count enumeration, a method that enables detection of bacterial cells based on their ability to replicate. Although performing plate count enumeration is one manner of assessing viability, cells can still be viable without possessing the ability to replicate. Thus, to properly assess probiotic viability, further analysis of a broader group of characteristics using several types of methods is proposed. In addition to viability, it is crucial to identify how well the cells in a probiotic product can survive in the gastrointestinal tract (GIT) and thus be able to mediate the desired health benefit while passing through the human body. A broad spectrum of different assay designs for assessing probiotic gastric tolerance have been used in research and quality control. However, the absence of any consensus on how to assess these qualities makes it difficult to compare between laboratories and to translate the results into in vivo tolerance. This review presents and discusses the complexity of assuring that a probiotic is suitable for beneficial consumption. It summarizes the information that can be subtracted from the currently available methods for assessment of viability and stress tolerance of a probiotic, hereby altogether defined as “activity.” Strengths and limitations of the different methods are presented together with favorable method combinations. Finally, the importance of choosing a set of analyses that reveals the necessary aspects of probiotic activity for a certain product or application is emphasized.
Introduction
The awareness of the health benefits of probiotic microorganisms, most often lactic acid bacteria (LAB), has increased rapidly the latest years and so has the global market for probiotic products (Rosenstiel and Stange, 2010; Hill et al., 2014; Hansen et al., 2018; Jackson et al., 2019; Fallico et al., 2020; Kiefer et al., 2020). Consequently, the market supply of probiotic products has become increasingly diverse, with a large number of both fermented food products and supplements, formulations, species, and strains (Kolaček et al., 2017).
The broad variety of products on the market makes it crucial that reliable methods are available for assuring probiotic viability (Kolaček et al., 2017; Hansen et al., 2018). Probiotics are a unique class of products since they consist of live organisms (Jackson et al., 2019). The term probiotic is defined by FAO/WHO as “live microorganisms that, when administered in adequate amounts, confer a health benefit on the host” (Rosenstiel and Stange, 2010; Hill et al., 2014). To assure that a probiotic product contains an adequate number of live organisms, authorities are asking for information on viability rates within a certain shelf-life in colony forming units (CFU) obtained from plate count enumeration (Hill et al., 2014; Fenster et al., 2019; Fiore et al., 2020; Hansen et al., 2020). The health effects of probiotics are reliant on dose and the minimum recommended amount to be consumed is often defined as 109 CFU per day, labeled as CFU/ml or CFU/gram (Minelli and Benini, 2009). Measuring CFU by plate count enumeration is one manner of detecting live cells, but viability is more complex than solely defined by the ability to form colonies.
To properly assess viability, further analysis of a broader group of characteristics is required to obtain a better overview of the state of the probiotic. The fact that the products often consist of several strains, in some cases combined with additional active ingredients, and that the probiotic effect is specific for this specific combination, further complicates the viability analysis (Hill et al., 2014; Hansen et al., 2018).
As previously mentioned, the definition of probiotics is based on live microorganisms (Rosenstiel and Stange, 2010; Hill et al., 2014). This definition assumes that a large enough number of microorganisms also remain viable and survive the transit through the gastrointestinal tract (GIT), while facing stressors such as bile and gastric acid (Fredua-Agyeman and Gaisford, 2015). The numbers of viable microorganisms required to obtain a clinical effect is generally considered to be 106 CFU/ml in the small bowel and 108 CFU/g in the colon (Minelli and Benini, 2009). However, it is currently not required by authorities to account for analysis of tolerance against these stressors (Fiore et al., 2020). In addition, if tolerance assays are performed, it is often during strain selection and evaluation in the discovery and research phase, instead of after freeze-drying and formulation of the final probiotic product that will be offered to the consumer. The survival after exposure to bile and gastric acid can differ significantly, sometimes by several log units depending on formulation, freeze-drying and storage conditions (Saarela et al., 2009; Charnchai et al., 2016; Kim et al., 2018).
This review summarizes the information that can be subtracted from the currently available methods for determination of viability and stress tolerance of probiotics, here altogether defined as “activity”. It presents strengths and limitations of the different methods and discusses the importance of choosing a study design that reveals the whole picture of the activity of a probiotic.
Viable but Not Culturable and the Great Plate Anomaly
The definition of bacterial viability has been debated in microbiology for a large period of time. Already in 1982, Xu et al. (1982) discovered that the waterborne pathogens that were subjects to their research were detected by direct viable counting but were not able to grow on plates. This additional population of cells had passed over to a viable but not culturable (VBNC) state, which lead to the questioning of the viability definition as the ability to form colonies. Three years later, Staley and Konopka (1985) coined the term “the great plate anomaly,” that has further on been used for describing the problem that a large portion of microorganisms in fact cannot be cultured under presently known conditions. The same authors concluded that these non-culturable bacteria indeed can be metabolically active.
So, what defines microbial viability? The question can without difficulty result in almost philosophical discussions. Although most researchers and scientists for long have agreed on that the definition is not limited to in vitro culturability, the answers to what defines microbial viability are not unambiguous and remains controversial. Through the years, several propositions on how to divide the non-culturable microbial population have been published (Kell et al., 1998; Breeuwer and Abee, 2000; Davey, 2011). One of the most recent definitions was published by Davis in 2014, who divided non-culturable microorganisms into the following states: (1) the non-replicating state (active physiology and membrane integrity), (2) the starving state (dramatic decrease in metabolism), (3) the dormant state (low metabolic activity and inability to divide without additional recovery attempts, VBNC), and (4) the irreparably damaged state (progressively declining metabolism that terminates in death).
There are a number of stresses in the manufacturing process and during storage, such as temperature shift-down, a lack of nutrients, and exposure to toxic agents such as hydrogen peroxide from autoclaved media, that can trigger the transfer of culturable populations into non-culturable states (Bogosian et al., 2000; Keer and Birch, 2003; Rittershaus et al., 2013). Lahtinen et al. (2006) showed in 2006 by flow cytometric (FC) analysis and plate count enumeration that three different probiotic strains lost their culturability during storage but maintained esterase activity, membrane integrity, and pH gradient across the cell membrane. Two years later, the same author showed that several Bifidobacterium strains, included in fermented food products, lost their culturability during storage but maintained high levels of rRNA and reductase activity (Lahtinen et al., 2008). Kramer et al. (2009) obtained similar results in 2009 when analyzing a lyophilized product containing both Lactobacillus acidophilus and Bifidobacterium animalis with polymerase chain reaction (PCR) and FC. The strains lost their culturability during storage but maintained their membrane integrity.
Besides the debate on the definition of viability, there has been several publications suggesting that non-viable probiotic microorganisms, sometimes referred to as parabiotics, also can provide benefits to the consumer (Taverniti and Guglielmetti, 2011; Fujiki et al., 2012; Lahtinen, 2012; de Almada et al., 2016; Piqué et al., 2019). However, parabiotics are outside the scope of this review.
Stressors During the Lifetime of a Probiotic Product
A probiotic product must be able to tolerate exposure to a number of different stressors during its lifetime (Figure 1). Firstly, during manufacturing and secondly, during storage and transportation and finally, during its passage through the GIT.
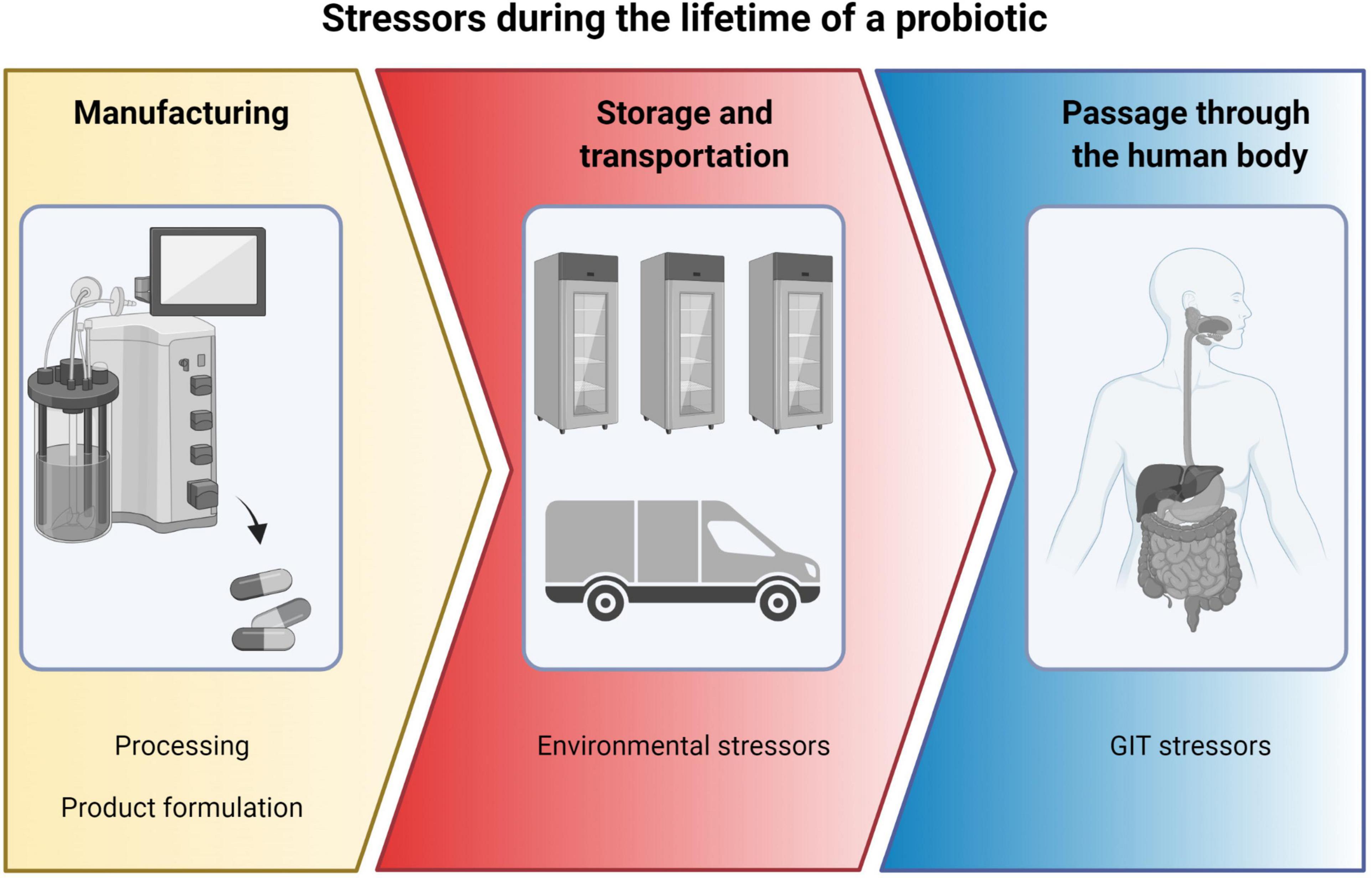
Figure 1. Overview of stressors during the lifetime of a probiotic, from manufacturing to storage and transportation and finally to passage through the human body. Created with BioRender.com.
Manufacturing
After discovery of a beneficial probiotic strain, manufacturing is required before a probiotic product can be brought onto the market. The different operations included in manufacturing can affect many aspects such as survival, viability, and growth (Kolaček et al., 2017).
Firstly, the new strain must be able to retain its quality through scaled-up fermentation. In the scaled-up fermentation several factors can differ substantially from lab-scale, such as medium composition, cultivation time, pH, and gas atmosphere, which all can affect how well the cells tolerate later processing steps (Saarela et al., 2009; Kolaček et al., 2017; Stage et al., 2020). Other challenges in large-scale fermentations compared to initial lab-scale fermentations are keeping the same tight control, holding times and homogeneity (Fenster et al., 2019).
Secondly, most often a drying step is preferred for convenient further handling, such as freeze-drying (lyophilization) or spray-drying (Dolly et al., 2011). Drying is a manner of putting the cells into a resting, non-metabolic state for long-term preservation until consumption (Santivarangkna et al., 2008; Pegg et al., 2015; Broeckx et al., 2016; Hansen et al., 2018). Dehydration in general implicates shear stress and severe mechanical stress to the cell membrane which might lead to cell death (Santivarangkna et al., 2008; Iaconelli et al., 2015; Huang et al., 2018). The removal of water rapidly increases the ratio between cell surface area and cell volume, leading to membrane deformation. Increased contact of the cell membrane with the surrounding air imposes an increased risk for damage by reactive oxygen species.
Depending on the chosen method for drying, additional stresses are introduced. In freeze-drying, the initial formation of extracellular ice crystals leads to an increasing concentration of medium solutes, resulting in osmotic stress (Broeckx et al., 2016; Obruca et al., 2016). Ice crystals are also formed intracellularly, possibly leading to membrane destruction and organelle disruption (Fuller, 2004). In addition, ice crystal formation can lead to increased concentrations of reactive oxygen species (Baek and Skinner, 2012). In spray-drying, heat is the main stressor, affecting a large part of the cellular components (Santivarangkna et al., 2008; Broeckx et al., 2016). Although the exact inactivation mechanisms in spray drying are not fully understood, exposure to thermal stress can lead to denaturation of proteins and destabilization of membranes. Ribosomal damage might be the critical component in heat inactivation.
Although drying provides efficient protection of the cells, the following product formulation exposes the bacteria to different stresses, weather it is inclusion into a food product or into a supplement.
Transportation and Storage
After manufacturing, the probiotic products must be transported from the manufacturing site and survive storage in given conditions in periods of time often exceeding 12 months (Fiore et al., 2020). During storage, the bacterial cells can be exposed to environmental stressors such as temperature, water, oxidation, pH, and light (Santivarangkna et al., 2008; Pegg et al., 2015; Broeckx et al., 2016; Kolaček et al., 2017; Fenster et al., 2019; Fiore et al., 2020). One of the undesired effects during storage is membrane lipid oxidation (Santivarangkna et al., 2008). For dried cells, the relative humidity is of high importance to be able to remain in the protecting dried state. In general, low temperatures and low humidity contributes to higher survival rates during storage.
Passage Through the Human Body
In addition to coping with the different stresses of manufacturing, storage, and transportation, the final probiotic product should be able to survive and maintain its activity while passing through the human body. Either before or during consumption, depending on the product type, the dried cells must undergo rehydration. Rehydration is challenging for the cell membrane, since it includes moving from a gel-like state into a liquid crystalline (Broeckx et al., 2016).
After rehydration, the bacterial cells must survive through the challenging upper GIT (Derrien and van Hylckama Vlieg, 2015). The upper GIT offers a number of trials to the ingested probiotic. The first challenge is passage through the stomach, where the gastric juice exposes bacteria to low pH (< 3) and high concentrations of pepsin (Derrien and van Hylckama Vlieg, 2015). Both these two stressors can lead to cell inactivation and death. However, the pH, composition, buffering capacity and volume of the gastric juice, and the transit time are largely affected by the recent food consumption history. In addition, survival through the GIT is highly strain dependent and depends on the product matrix, e.g., powder or capsule (Fredua-Agyeman and Gaisford, 2015).
The second challenge is the passage through the duodenal loop of the small intestine where the main bile salt exposure occurs (Taranto et al., 2003). Bile is more harmful to bacteria than low pH since it acts as a detergent and thus disrupts the membrane (Ilango et al., 2016). Exposure to bile changes the lipids in the cell membrane, possibly affecting both cell permeability and the interactions between the membrane and its environment (Taranto et al., 2003). As highlighted by Bustos et al. (2012) differences in bile tolerance between strains are also reliant on the ability to express bile salt hydrolase enzyme. LAB strains that are bile salt hydrolase positive are more tolerant to the consequences of bile exposure (Bustos et al., 2012). The ability to hydrolyze bile salts is considered as one of the key features of probiotic bacteria according to the World Health Organization, although far from all probiotics have this ability (WHO and FAO, 2006). In addition to bile salt hydrolysis, LAB strains can possess other abilities to cope with bile exposure such as active efflux of bile salts and changes in the cell wall and cell membrane composition (Ruiz et al., 2013). Apart from bile salts, the small intestine also contains pancreatin and lipase, that as well are potentially harmful to bacteria (Derrien and van Hylckama Vlieg, 2015).
In addition to surviving the passage through the human body, the probiotic bacteria must be in a sufficient state for being able to affiliate beneficial health effects. Syntrophic interactions with the existing microbiota, enhancement of the epithelial barrier, immunomodulation, secretion of antimicrobial substances, prolonged persistence in the gut by colonization, and the ability to form biofilms, are factors that are associated with probiotic function (Bermudez-Brito et al., 2012; Frese et al., 2013; Segers and Lebeer, 2014; Li et al., 2021). Mucus-binding pili, that enables both host interactions, adherence, and biofilm formation, and that might be involved in immunomodulatory interactions, can be expressed to a lesser extent by a challenging host environment or be damaged by exposure to for example shear stress (Segers and Lebeer, 2014).
Manners of Assessing the Activity of Probiotics
The manners of assessing the activity of probiotics have here been divided into viability assessment (Figure 2A) and stress tolerance assessment (Figure 2B). The described methods for viability assessment have been further divided into two subcategories: (1) culture-dependent methods, that are based on the ability of the cells to replicate, and (2) culture-independent methods, that are based on other cell characteristics than the ability to replicate.
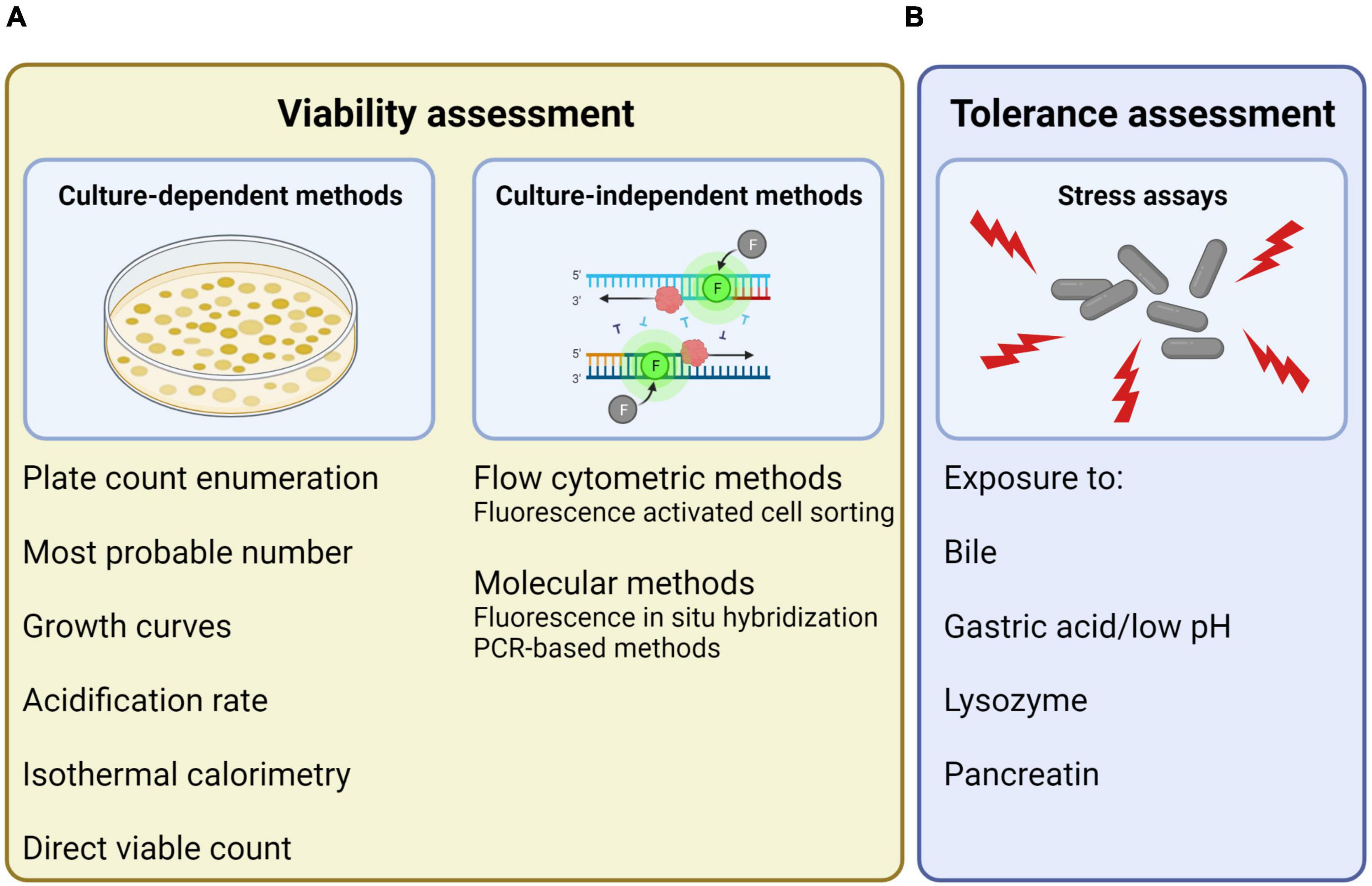
Figure 2. Overview of different manners of assessing probiotic activity, here divided into (A) viability assessment, which can be performed using both culture-dependent and culture-independent methods, and (B) tolerance assessment, which can be performed by exposure to different stressors. Created with BioRender.com.
Culture-Dependent Methods for Viability Assessment
Culture-dependent methods, where plate count enumeration is the most prevalent, have for long been the standard for viability measurement of probiotic cultures and products (Lahtinen et al., 2006; Davis, 2014; Hansen et al., 2020; Kiefer et al., 2020). They are uncomplicated to perform but laborious and require media and conditions that are well adapted to the strain of interest (Davis, 2014). Culture media are always, to a certain extent, selective for a certain type of bacteria and might be unequally optimal between bacteria from different genera or even between different strains (Coeuret et al., 2003). Factors such as oxygen tolerance, antibiotic susceptibility, and nutritional preferences must be considered when choosing and designing suitable media and cultivation conditions. Thus, although sometimes possible with antibiotics, it can be difficult to separate a mixture of two or several strains with highly similar growth requirements (Jackson et al., 2002; Hansen et al., 2018; Vinderola et al., 2019; Kiefer et al., 2020).
Plate Count Enumeration
There are multiple ways of performing plate count enumeration. In brief, the probiotic sample is dissolved/suspended in a buffer/medium and diluted to an appropriate concentration. The resulting solution is then distributed either onto a petri dish filled with solid agar medium or mixed with melted agar medium and let to solidify in a petri dish. After incubation under suitable conditions, the colonies that have been formed can be counted and approximated to represent the number of viable cells present in the original sample.
There are several advantages of using plate count techniques for assessing the viability of a probiotics. For example, low costs are required, they enable detection of proven viability and ability to replicate, are easy to set up in a laboratory, and give highly visual results, allowing observation of colony morphologies and potential contamination. In addition, since these methods have traditionally been the established way of performing microbiological viability assessments, the available published historic data on their various applications are extensive. Specifications of the viability of probiotic products are also inquired for in CFU from authorities (Hill et al., 2014; Fenster et al., 2019; Fiore et al., 2020; Hansen et al., 2020). The International Organization for Standardization (ISO) offers standardized plate count enumeration protocols for probiotics, both for mesophilic lactic acid bacteria in general, ISO 15214 (ISO, 1998), for Lactobacillus acidophilus, ISO 20128 (ISO, 2006) and for presumptive bifidobacteria, ISO 29981 (ISO, 2010).
Although widely used, plate count enumeration methods for viability assessments comes with several limitations. Firstly, culturing bacteria on agar plates is highly time-consuming, mostly due to preparation of media and long incubation times (Fredua-Agyeman and Gaisford, 2015; Vinderola et al., 2019). Secondly, plate count enumeration is based on the ability of the cells to form colonies by replication. However, as previously mentioned, the definition of viability includes more abilities than replication (Xu et al., 1982). Plate count enumeration is also based on the assumption that only one cell will form one colony. However, during manufacturing of a culture powder, there is a risk for clumping (Davis, 2014; Vinderola et al., 2019). The clumping can give rise to uneven spreading of the cells during plating, which might result in one colony being formed by multiple cells. Plate count enumeration of bacterial cells exposed to stress conditions e.g., due to freeze-drying and manufacturing, can also affect culturability (Olszewska et al., 2019). In general, plate count methods are associated with underestimations and large variations, sometimes reaching up to 35% (Kogure et al., 1979; Olszewska et al., 2019). This can partly be explained by media quality, fluctuations in growth conditions, and inherent errors in serial dilutions in the sample preparation (Kogure et al., 1979; Corry et al., 2007; Hansen et al., 2020).
Most Probable Number
The most probable number method is based on that a liquid medium turns turbid during bacterial growth (Bibiloni et al., 2001; Sutton, 2010; Angelov, 2014). By making a 10-fold serial dilution of a bacterial cell solution in replicates, the point will be reached where the solution is so diluted that only a few of the replicates of a certain dilution give rise to turbidity. The number of tubes with confirmed turbidity, thus growth, at the different dilutions can be used to estimate the number of viable cells in the original sample by utilizing premade tables based on statistics using 95% confidence intervals. Although not traditionally used for viability measurements in probiotics, there are a few examples (Bibiloni et al., 2001; Angelov, 2014).
The most probable number methodology is based on several assumptions (Blodgett, 2020). These assumptions are listed in the FDA Bacteriological Analytical Manual (BAM), namely: random bacterial distribution within the sample, that the bacteria exist as separate units without clustering and repelling, that growth from as few as one single cell will be detected, and that individual tubes are treated as independent samples.
Although often used when handling very diluted samples, which is often not the case with probiotics, this method presents an alternative to plate count enumeration when facing problems with colony formation e.g., from a disturbing matrix (Sutton, 2010; Blodgett, 2020). However, it is important to remember that results obtained from this method are only the statistical probability of the number of replicating bacteria in a sample, compared to for example plate count enumeration that results in an actual number.
Growth Curves
Growth curves are created by culturing bacterial cells in a liquid medium and continuously measuring the increase in optical density (OD) as the cells replicate and then plotting the measured OD values against time. The resulting curves can reveal a number of different characteristics. Factors especially interesting for assessing replication potential are the maximum growth rate, μmax, and the duration of the lag phase, tlag (Bircher et al., 2018). It has been observed that tlag negatively correlates with viable cell counts, in that particular case obtained with the most probable number method, and that previous manufacturing and storage conditions, such as freezing, freeze-drying, and spray-drying, have an impact on both generation times and growth initiation (Squires and Hartsell, 1955; Iaconelli et al., 2015; Lipson, 2015; Bircher et al., 2018). Indications of low viability, as interpreted from growth curves, may have a direct link to metabolic activity in the GIT (Bircher et al., 2018). However, further research is called for to fully elucidate the relationship between μmax, tlag and viability.
One issue in growth curve viability assessment is how to decide the starting viable cell count when comparing different cell samples. Whether you choose to start your growth curve with a sample concentration based on OD, membrane integrity measured by FC, or on CFU from plate count enumeration, can seriously affect your result. Which method that is the most suitable for deciding the start concentration of a cell sample for a growth curve, can be debated. Starting at the same OD might introduce uncertainty from differences in the amount of non-viable cells and debris between samples (Beal et al., 2020). CFU from plate count enumeration is based on replication, as is growth curve assessment. However, plate count enumeration results in a cell number based on the ability to replicate on an agar plate at given conditions, which is not the same conditions as in the growth curve assessment. Starting at a concentration based on live/dead assessment with FC (described later) can reveal a live population that might be able to replicate during growth curve assessment. However, FC results also reveal a damaged cell population, of which the part that is able to replicate in the given conditions is unknown.
Acidification Rate
Acidification rate analysis, performed by simply measuring the change in pH in a liquid medium culture with time, is most commonly used for assessing the quality of milk starter cultures containing LAB (Fonseca et al., 2000). For this application, an ISO method is available, ISO 26323 (ISO, 2009). However, the amount of produced lactic acid might also be used as a complement or substitute to other viability measurements in a variety of samples containing probiotic LAB (Saez-Lara et al., 2015). Fonseca et al. (2000) used acidification rate to compare the resistance to freezing between a number of different LAB strains and could see that the acidification rate was proportional to cell concentration under well-defined experimental conditions. Poor reliability in plate count enumeration due to chain building can be circumvented by instead performing acidification rate measurements.
Isothermal Microcalorimetry
Isothermal microcalorimetry is a technology that is based on that heat is produced by a replicating, metabolizing bacterial population (Mihhalevski et al., 2011; Garcia et al., 2017; Fredua-Agyeman and Gaisford, 2019; Nykyri et al., 2019). The heat production is approximately 2 pW per cell, enabling measurements in bacterial samples with cell concentrations from approximately 106 CFU/ml (Fredua-Agyeman and Gaisford, 2019). The heat production is proportional to the number of bacterial cells in a sample and can be plotted as μW against time. For the most simple isothermal microcalorimetry measurements, a bacterial sample is placed in a glass ampoule. The heat production is then recorded for as long as it occurs (Braissant et al., 2010). By following the heat production of the bacterial population, it is possible to obtain real-time, continuous viability data, in contrast to for example plate count enumeration that only gives the endpoint of the viability measurement (Braissant et al., 2010; Fredua-Agyeman and Gaisford, 2019). From the heat measurement, several different growth specific data can be estimated, such as biomass yield and specific growth rate (Mihhalevski et al., 2011). Since the heat recordings are passive, the bacterial sample is available for further analysis (Braissant et al., 2010).
Apart from the above-mentioned advantages of isothermal microcalorimetry, the technology is appreciated for not requiring laborious sample preparation steps, for giving results within a few hours, and for requiring very low running costs (von Ah et al., 2008; Entenza et al., 2014; Garcia et al., 2017). The method enables high sensitivity measurements due to the low detection levels of the produced heat and the limited scattering of the data, thus, providing viability data with high accuracy and low standard errors (Braissant et al., 2010; Garcia et al., 2017). Exposure to stressors such as drying followed by rehydration does not seem to affect isothermal microcalorimetry results.
Despite the many advantages of using isothermal microcalorimetry for viability measurement of probiotic cultures, this method also has a number of drawbacks. For example, it requires careful calibration with a reference sample at the exact temperature of measurement in order to obtain high accuracy and sensitivity (Braissant et al., 2010). In addition, since measurement of heat is non-specific for bacterial growth, it cannot be excluded that other cellular processes are contributing to the resulting signal. Finally, isothermal microcalorimetry is still a technology that is not as established for enumeration of probiotic cultures, as for example Plate count enumeration or PCR techniques. Consequently, the availability of large studies and comparative material is limited.
Direct Viable Count
Direct viable count is a direct counting technique that enables differentiation between viable and non-viable cells by their ability to replicate. By incubating the bacterial sample with nutrients and a gyrase inhibitor, cell division is inhibited, resulting in viable cells to elongate and become considerably larger than non-viable cells (Kogure et al., 1979; García-Hernández et al., 2012). Viable cells can then be distinguished from non-viable cells merely on size during microscopic observation.
Direct viable count is a method that requires a relatively small amount of time for sample preparations and that enables simple counting using a microscope. On the other hand, examination by the eye is often necessary, which puts requirements on the skills of the technician performing the analysis and compromises efficacy.
Culture-Independent Methods for Viability Assessment
The methods included in this section are divided into flow cytometric methods and molecular methods. The described methods all have in common that they do not require that the microorganisms have the ability to replicate in order to be detected.
Flow Cytometric Methods
Although first developed for the hematology field, FC has become an increasingly used tool within microbiology (Davis, 2014). FC is a technology that enables cell-by-cell observation of up to millions of cells and division of these into subpopulations based on different characteristics (Maciorowski et al., 2017). Briefly, the cell sample is passed through a so-called sheath fluid stream, centering single cells (Shapiro, 2003; Tracy et al., 2010; Adan et al., 2016). The centered cells are hit by a light source on their passage, most often a laser beam, that will result in light scattering or in excitation of any applied fluorescent probes. Optical detection of light scattering reveals morphological and structural cell characteristics while detection of the emission from excited fluorescent probes can give information of specific cell properties or cell components. The optical signal is transmitted to an electronic network that converts it to digital data that enables computational recordings of the light intensity.
In microbial viability FC measurements (often called live/dead assessment), dead cells are most commonly distinguished from live cells by determining membrane integrity using the DNA stains propidium iodide (PI) and SYTO (Figure 3). SYTO will enter all cells, while PI only will enter cells with compromised and permeable membranes (Díaz et al., 2010; Tracy et al., 2010). The resulting information from these two stains is used for dividing the total cell population into one live (impermeable) and one dead (permeable) subpopulation (Breeuwer and Abee, 2000). Apart from these two subpopulations, it is possible to detect cells in intermediate states that are not completely permeable to PI but will be stained to a smaller extent (Olszewska et al., 2019). This subpopulation is often interpreted as damaged cells. Apart from PI and SYTO staining, there are a wide variety of different fluorescent probes, or fluorochromes, that can be used for detecting different cell properties and components (Adan et al., 2016). The available ISO method for viability measurement of LAB in probiotics, ISO 19344, contains protocols for determination of enzymatic activity, membrane integrity, and metabolic activity (ISO, 2015).
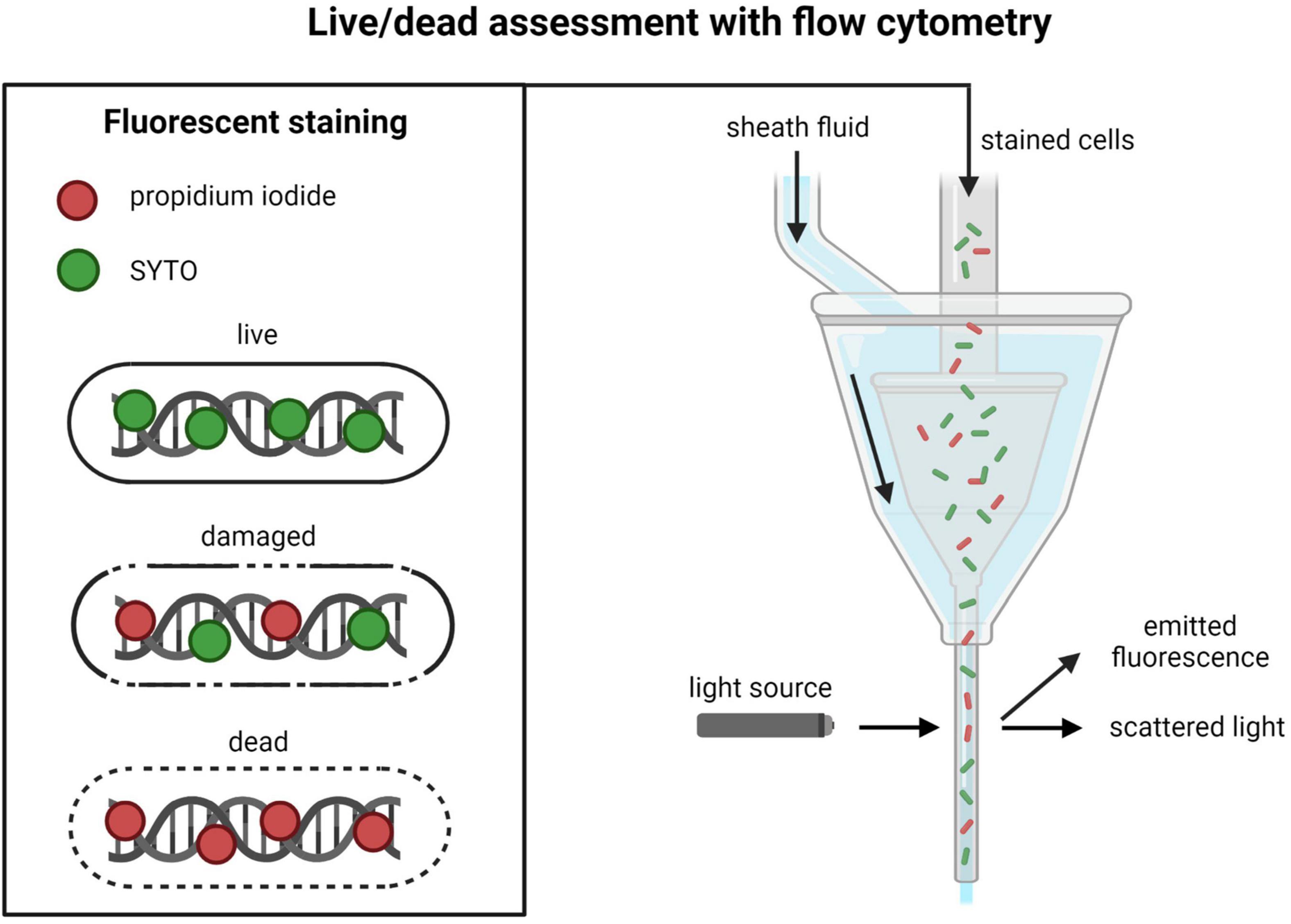
Figure 3. Overview of live/dead assessment with propidium iodide/SYTO staining and flow cytometry. Created with BioRender.com.
There are many advantages of using FC and fluorescent probes for assessing probiotic activity. The use of fluorescent probes offers a high sensitivity and the ability to observe several parameters simultaneously (Shapiro, 2003; Lahtinen et al., 2006; Díaz et al., 2010; ISO, 2015). The instrument also allows high-throughput analysis in the regions of several thousands of cells per second (Davis, 2014). In addition, FC indicates the heterogeneity of a population, which can be valuable information for bacterial cultures intended for probiotic products (Tracy et al., 2010). Compared to for example plate count enumeration, the time required from sample preparation to result is considerably shorter (Breeuwer and Abee, 2000).
Despite providing very detailed information about all cells in sample, FC results are presented on a population basis and not as single cells (Olszewska et al., 2016). This requires certain knowledge of the cell population to be able to achieve an experimental design that will yield the maximal amount of information. If using several fluorescent probes at the same time, optimization of any potential overlaps between their emission spectra must be performed to avoid misinterpretations (Adan et al., 2016). In addition, if using fluorescent labeling of the cells, bacterial autofluorescence may disturb the results (Moter and Göbel, 2000). As in plate count enumeration, FC results can be affected by clumping, although it is often possible to identify an inhomogeneous population (Kramer et al., 2009). FC detects phenotypic differences, which can be a limitation when differentiating between strains with only small genetic differences. This presents a problem when analyzing probiotic products containing multiple strains.
Fluorescence Activated Cell Sorting
Fluorescence activated cell sorting (FACS) is a technology that enables separation of the sample analyzed by FC into the detected subpopulations and collection of these for further analysis (Tracy et al., 2010). This additional feature allows observation of for example culturability in connection to the different characteristics detected in the FC analysis (Olszewska et al., 2019).
Although taking the FC analysis one step further, one limitation of FACS is the risk of damaging the sorted cells during measurement by e.g., laser exposure, shear stress and mechanical sorting (Olszewska et al., 2019). Thus, there is a risk that for instance the culturability of a FACS subpopulation might be compromised.
Molecular Methods
Usage of molecular methods, which are based on nucleic acid detection, enables detection and in combination with identification of bacteria on a strain-level with high specificity and sensitivity in a high-throughput manner (Keer and Birch, 2003). Therefore, molecular methods are of great use in samples or products containing multiple probiotic strains (Keer and Birch, 2003; Postollec et al., 2011; Hansen et al., 2018; Kiefer et al., 2020).
However, nucleic acid levels alone are not considered to be a reliable viability marker (Keer and Birch, 2003; Amor et al., 2007; Kiefer et al., 2020). Nucleic acid content was previously considered to distinguish live cells from dead cells because of post-death degradation (Keer and Birch, 2003; Nogva et al., 2003). However, the DNA molecule is very stable and can persist in cells even after death has occurred. The RNA molecule on the other hand, is very unstable and is therefore not optimal to be the base of high accuracy live/dead measurements. In addition, in live cells, both growth rate and placement in the cell cycle can give large variations in nucleic acid levels (Moter and Göbel, 2000; Bouvier and Del Giorgio, 2003). However, the strength of molecular methods to identify specific probiotic species and strains, makes them optimal to use on combination with other methods detecting viability markers, or in combination with viability dyes for strain-selective detection of viable cells.
Fluorescent in situ Hybridization
Fluorescent in situ hybridization is a technique where hybridization of bacterial cells with complementary fluorescence-labeled oligonucleotide probes is used, enabling distinction between groups of bacteria in a mixed population (Amann et al., 1990, 1995; Coeuret et al., 2003). Depending on the choice of probe, the groups of bacteria can be separated on species or even on strain level (Coeuret et al., 2003). Fluorescent probes with different emission wavelengths can be used on the same sample, enabling simultaneous detection of several sequences (Moter and Göbel, 2000). In addition, fluorescent in situ hybridization does not require elaborate sample preparation steps and is relatively fast to perform (Moter and Göbel, 2000; Blasco et al., 2003). This method also offers a very high sensitivity if using a carefully designed probe. Fluorescent in situ hybridization does not itself provide information on viability but can be successfully combined with e.g., microscopy, FC, and direct viable count for further information on strain-specific viability (Amann et al., 1990; Moter and Göbel, 2000; Blasco et al., 2003; Ercolini et al., 2006; Tracy et al., 2010; García-Hernández et al., 2012; Olszewska et al., 2016).
Problems may arise due to insufficient optimization of permeabilization treatment prior to hybridization (Moter and Göbel, 2000). As any other fluorescence-based technology, autofluorescence from the analyzed bacteria can also compromise the reliability of the results and decrease the signal-to-noise ratio. Matrix-dependent differences have been observed when using this method, emphasizing the importance of careful evaluation of the influence of the matrix when setting up a fluorescent in situ hybridization method (Davis, 2014).
Polymerase Chain Reaction-Based Methods
There are several molecular methods used for detection of probiotic bacteria that are based on PCR. Alone, usage of these methods does not enable selective quantification of genetic material only from viable cells. However, it is possible in combination with the nucleic acid-intercalating dyes ethidium monoazide (EMA) and propidium monoazide (PMA).
Real-Time Quantitative Polymerase Chain Reaction
Real-time quantitative PCR (qPCR) enables identification and quantification of microorganisms on strain level (Kramer et al., 2009; Postollec et al., 2011; Villarreal et al., 2013). In brief, a specific DNA sequence is enzymatically amplified by a thermostable DNA polymerase that attaches to pre-designed oligonucleotide primers that have hybridized to the template DNA (Postollec et al., 2011). Variation of the reaction temperature enables denaturation of the two DNA strands, hybridization of the primer, attachment of the polymerase and replication of the DNA sequence, followed by polymerase detachment. Repetition of this chain of events in cycles results in an exponential amplification of the targeted DNA sequence. In qPCR, a fluorescent reporter probe is used for monitoring the increasing nucleic acid levels. There are two different reporter probes that are typically used—the DNA-binding SYBR ® Green and TaqMan ® (Applied Biosystems), that is used for the hydrolysis probe method (5′ nuclease assay) (Gibson et al., 1996; Wittwer et al., 1997; Postollec et al., 2011). SYBR ® Green is not specific for a certain DNA sequence and can therefore offer the flexibility of being used in many different setups of genetic detection. TaqMan ® on the other hand is used for detection of particular amplicons and therefore results in a higher specificity. By plotting the fluorescence of the probes against the number of cycles, quantification can be achieved.
If performed with thorough knowledge and optimized protocols, qPCR technology is rapid, offers high specificity and sensitivity, and can distinguish between probiotic strains with large genetic similarities (Bustin et al., 2005; Postollec et al., 2011). In addition, both the sample preparation steps, and the analysis are possible to automate (Postollec et al., 2011).
Although qPCR can identify a certain organism on the molecular level, quantification requires a previously optimized standard curve, based on purified DNA (Hansen et al., 2018). There are many commercially available kits for DNA purification, but usage of these does not always result in complete extraction. In addition, the PCR amplification reaction is sensitive to inhibitors (Gobert et al., 2018). Amplification must result in DNA concentrations above the background level of the standard curve, which limits the possibilities of quantification of genetic material present in very low concentrations (Hindson et al., 2011). For a reliable qPCR protocol, proper positive and negative controls must be analyzed to exclude contamination (Bustin et al., 2005).
Digital Polymerase Chain Reaction
Digital PCR (dPCR) is a PCR method where the sample is extensively diluted and then divided into separate minireactors, as droplets or in a chip-based manner (Hindson et al., 2011; Huggett et al., 2013; Hansen et al., 2018; Kiefer et al., 2020). Consequently, a number of the minireactors will not contain the target sequence due to the high dilution grade. The PCR amplification reactions take place within the minireactors and the number of positive (generated from TaqMan ® chemistry) and negative signals from the separate reactions are calculated and translated to gene copies per μl using Poisson statistics.
Similar to qPCR, dPCR can quantitatively distinguish between highly similar probiotic strains, with high precision within a short amount of time (Hansen et al., 2018). However, in opposite to traditional qPCR technologies, dPCR enables absolute quantification of DNA sequence copy numbers, which are not based on a standard curve using purified DNA (Hindson et al., 2011; Hansen et al., 2018). Thus, the dPCR approach is less sensitive to inhibitors compared to qPCR and can detect lower concentrations of genetic material (Huggett et al., 2013; Gobert et al., 2018).
However, similar to qPCR, dPCR also requires careful design and optimized controls to be able to deliver robust results (Huggett et al., 2013). In addition, although dPCR presents many advantages compared to qPCR, it is still more expensive, and requires more advanced technology and complicated workflows (Hindson et al., 2011; Gobert et al., 2018). Although it could be argued that dPCR results are built on estimations, limiting their credibility compared to absolute values, the very large number of minireactors enables statistical calculations with very high precision (Hindson et al., 2011).
Polymerase Chain Reaction-Based Methods in Combination With Ethidium Monoazide and Propidium Monoazide Staining
The inability of molecular methods to selectively quantify genetic material only from viable cells was circumvented by Nogva et al. (2003) in 2003 by utilizing staining with EMA and later by Nocker et al. (2007) in 2007 with PMA, both in combination with PCR technology. These dyes are nucleic acid-intercalating and binds irreversibly upon photoactivation, thus strongly inhibiting further amplification attempts by PCR (Nogva et al., 2003; Nocker et al., 2007; Fittipaldi et al., 2012). EMA is membrane-permeable, and PMA is membrane-impermeable. Hence, these stains enable selective amplification of genetic material from cells with intact membranes. PCR methods utilizing PMA and EMA staining, are often referred to as viability PCR, or v-PCR (Figure 4). Both qPCR and dPCR have been used for separation of viable and non-viable bacteria on a strain level with PMA/EMA chemistry (Rudi et al., 2005; Kramer et al., 2009; Gobert et al., 2018; Kiefer et al., 2020).
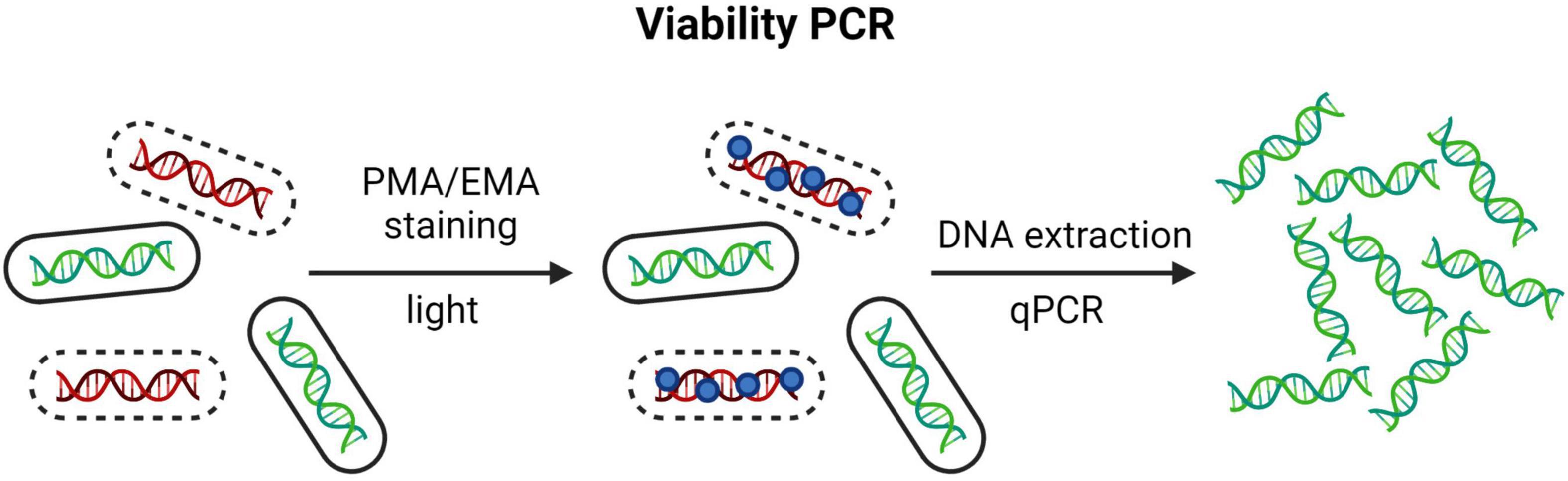
Figure 4. The principle of qPCR combined with PMA/EMA chemistry, so called viability PCR. Created with BioRender.com.
Despite revolutionizing PCR technology by extending molecular detection with membrane permeability markers, EMA and PMA chemistry does have its limitations. Several publications have addressed differences in permeability to these dyes depending on a collection of factors (Fittipaldi et al., 2012; Gobert et al., 2018; Kiefer et al., 2020). Influencing factors are dye concentration, physicochemical properties of the matrix, interplay between permeability and active efflux systems, differences in the compositions of cell membrane and cell walls, cytotoxicity of the dyes, cell concentration, and sample ratio between live and dead cells. In other words, careful optimization and use of controls are crucial when performing viability detection with PMA/EMA.
Stress Tolerance Assessment
Apart from remaining viable in the probiotic culture or product, the included microorganisms must also have the ability to survive the passage through the GIT. To assess this ability, many different setups of both culture-dependent and culture-independent assays have been presented that are designed to reveal probiotic survival in the presence of different stress factors. The most prevalent stress factors used in tolerance assays are bile and gastric acid/low pH (with or without pepsin, a proteolytic enzyme secreted in stomach), representing the passage through the stomach and the duodenum, respectively (Saarela et al., 2005). Exposure to lysozyme (found in saliva) and pancreatin (present in the small intestine) are also included in a few published studies.
Although widely used, there is no consensus or standardized manner on how to perform stress assays to estimate the survival of probiotics during their passage through the human body. A variety of stressors and different compositions of these are used, and the resulting tolerance is analyzed with different methods. In some cases, the survival after an exposure to a stress factor is assessed and in other cases the growth in the presence of a stress factor is the target of observation. Tables 1–4 summarize the conditions in a selection of published stress assays assessing probiotic tolerance against gastric acid/pH/pepsin, bile, pancreatin, and lysozyme. The designs of these assays are highly varying, both in terms of tested material (fresh cells/freeze-dried culture, spray-dried culture), type of stressor (artificial, biological), physiological or non-physiological concentrations, exposure times, and chosen methods for assessing the effects of the stress exposure on the bacteria. The assay design of course has a large impact on the results, thus making different assay setups difficult to compare. Saarela et al. (2005) highlighted in 2005 the consequences of using different types of bile in a tolerance assay on Bifidobacterium animalis ssp. lactis cells. Bile extract was severely more harmful to the cells than bile acid. There is also a batch-to-batch variation to consider when using biological bile and differences depending on species of origin. Fredua-Agyeman and Gaisford also pointed out in a publication from 2015 that simulated gastric conditions in stress assays often present a very poor similarity to the complex environment that probiotic bacteria are exposed to in the GIT. For example, the bile salt concentration in the gut is varying over time and in the different parts of the intestine (Marteau et al., 1997). In addition, in vivo, the combined stresses of exposure to e.g., bile and gastric acid, can be much more damaging than in an in vitro assay only exposing the cells to one stressor. Continuous exposure to bile, as seen in vivo, is more detrimental compared to a shock exposure for a short period of time, as often seen in vitro (Taranto et al., 2003). All of these factors have to be considered when designing a stress assay.
Except from the studies presented in Tables 1–4, a number of publications aim to assess the tolerance of passing through a simulated version of the complete gastrointestinal system to create a further realistic situation of the environment. Charnchai et al. (2016) tested freeze-dried Bifidobacterium animalis for its tolerance against simulated in vivo saliva conditions, the esophagus-stomach environment, and passage through the duodenum and ileum. Fredua-Agyeman and Gaisford exposed eight commercially available probiotic products to one kind of porcine gastric fluid and two kinds of simulated gastric fluids.
Discussion
The growing interest in probiotics requires reliable methods for assuring the activity of the numerous products on the market (Kolaček et al., 2017; Hansen et al., 2018). Currently, the only information asked for by authorities are viability rates within a certain shelf-life in CFU, obtained from plate count enumeration (Hill et al., 2014; Fenster et al., 2019; Fiore et al., 2020; Hansen et al., 2020). Measuring CFU by plate count enumeration is one manner of assessing viability, but the concept is more complex than the ability to replicate. In this review, both viability and the ability to survive the passage through the human body and therefore be able to give a beneficial health effect are altogether defined as probiotic activity. The different methods, that are reviewed in this paper, each offers a piece of information contributing to a better understanding of probiotic activity.
One fundamental problem with methods based solely on culturability is their inability to detect all subpopulations that are included in the viable cell population (Iaconelli et al., 2015; Jackson et al., 2019). As mentioned before, several different divisions of a cell population based on viability markers have been published. Davis defined in 2014 four different non-culturable populations as the following: (1) non-replicating (active physiology and membrane integrity), (2) starving (dramatic decrease in metabolism), (3) dormant (low metabolic activity and inability to divide without additional recovery attempts, VBNC), and (4) irreparably damaged (progressively declining metabolism that finally terminates in death). Different methods have to be used for detection of these four populations. Therefore, to limit the definition of viability to culturability alone can be highly misleading and result in large underestimations of the number of active cells (Davey and Kell, 1996; Vinderola et al., 2019; Fiore et al., 2020).
Since label specifications on probiotic products describe viability numbers based on plate count enumeration, the question arises if the present non-culturable population not accounted for contributes to the overall health effect after consumption. Fiore et al. (2020) discussed the possible effects of both the presence of a VBNC population and the presence of dead cells in probiotic products, defining these two populations as “hidden microbial fractions.” Since microbial cells that are not able to grow on agar plates still can possess active metabolisms, there is a possibility that they can promote health benefits (de Almada et al., 2016). There is also a chance that dormant cells may reacquire the ability to reproduce once arriving in a favorable environment such as the gut (Senoh et al., 2012). In addition, non-viable cells, or parabiotics, have been indicated to promote health effects in a number of studies (Taverniti and Guglielmetti, 2011; Fujiki et al., 2012; Lahtinen, 2012; de Almada et al., 2016; Piqué et al., 2019). The ratio between the numbers of culturable cells and non-culturable cells will also change over time when stressed cells transfer to different non-culturable states, possibly moving the measured CFU further and further away from the actual viable cell count. As a result, there is a risk that the presence of this “hidden population” may affect the results of both stability studies and clinical trials on probiotics and consequently dose specifications. Today, a large collection of methods that have the abilities to reveal different viability characteristics are available. Culture-dependent methods reveal the ability to replicate but are reliant on optimized medium for the specific strain to be analyzed, thus often making strain separation impossible. In addition, culturability can be affected to a large extent by the suitability of the medium and previous stress exposure (García-Hernández et al., 2012; Olszewska et al., 2019; Vinderola et al., 2019). Among culture-independent methods, FC and other methods based on labeling with fluorescent probes, can reveal multiple important cell characteristics at the same time, such as membrane integrity, enzymatic activity, and metabolic activity (ISO, 2015). However, flow cytometric methods are as well often limited when it comes to strain separation. Other culture-independent methods based on molecular detection have the strength to be able to identify and separate probiotics on a strain level but are limited in the detection of viability markers. The use of PMA/EMA chemistry enables molecular technologies to combine strain-specific detection with assessment of membrane integrity (Rudi et al., 2005; García-Cayuela et al., 2009; Kramer et al., 2009; Gobert et al., 2018; Kiefer et al., 2020).
Since the herein described methods assess different viability markers, the number of detected viable cells can vary to a large extent between methods (Iaconelli et al., 2015; Vinderola et al., 2019). This aspect needs to be taken into consideration when comparing results from different analyses in stability studies and quality assurance. For example, a more rapid decrease in the number of active cells during storage has been seen with culturability analysis by plate count enumeration compared to membrane integrity analysis by FC (Foglia et al., 2020). In many cases, it is fruitless to strive for conformity between different methods, but instead see them as complementary to each other. Fiore et al. (2020) suggested that a total cell count by FC measurement should be added on probiotic product specifications to account for the populations not detected by plate count enumeration.
Although a probiotic culture has been determined to be viable after manufacturing, storage, and transportation, survival during its passage through the human body is desirable to enable positive health effects. The ability to tolerate exposure to the different stressors of the GIT have been assessed using many different assays designs. However, there is no established standard for assessing GIT stress tolerance and the link to in vivo survival is lacking.
It would be advantageous for the probiotic industry to rethink and extend the viability concept to an overall definition of activity that includes additional characteristics to culturability. Preferably, study designs can consist of a combination of several methods revealing a broader picture of probiotic activity—both characteristics indicating viability and tolerance against the stressors of the GIT.
It is crucial to carefully consider which kind of information that is desired to retrieve from a study design. Which activity markers are relevant for this particular sample and what are the limitations of the different methods available for their measurement? Are the results to be used in research or in quality assurance? In the case of stress tolerance assays, is the purpose to mimic the in vivo GIT environment with as high similarity as possible or is it to find a specific condition that will separate suitable from non-suitable probiotic cultures before product formulation?
To be able to compare results, it would be advantageous to define further standardized manners of assessing activity. However, a certain dynamic for strain dependence and type of sample has to be accounted for Vinderola et al. (2019). Assay results are often very strain dependent and affected by both sample type and formulation (Derrien and van Hylckama Vlieg, 2015; Iaconelli et al., 2015).
Apart from being able to conclude if a strain has the ability to maintain active throughout the manufacturing process, storage, and passage through the human body, it is of large importance to assess its ability to achieve beneficial health effects in the host. There are a number of available assays for assessing probiotic characteristics such as antimicrobial effects, the ability to colonize, and immunomodulatory characteristics (Papadimitriou et al., 2015). However, further assay development and standardization have to be performed to be able to mimic the complex intestinal environment in vitro and to be able to obtain reliable results.
As the probiotic field continues to evolve and flourish, the need for reliable methods for assessing probiotic activity has never been greater. With emerging technology, the possibilities of filling this need are extensive. The probiotic industry of tomorrow would greatly gain from an extended viability definition and standardized manners of identifying the complete activity state of a probiotic culture or product.
Author Contributions
The author confirms being the sole contributor of this work and has approved it for publication.
Conflict of Interest
UW was employed by company BioGaia AB.
Publisher’s Note
All claims expressed in this article are solely those of the authors and do not necessarily represent those of their affiliated organizations, or those of the publisher, the editors and the reviewers. Any product that may be evaluated in this article, or claim that may be made by its manufacturer, is not guaranteed or endorsed by the publisher.
Acknowledgments
The author wishes to acknowledge the support and feedback from colleagues at BioGaia AB during the writing of this review manuscript.
References
Adan, A., Alizada, G., Kiraz, Y., Baran, Y., and Nalbant, A. (2016). Flow cytometry: basic principles and applications. Crit Rev Biotechnol 37, 163–176. doi: 10.3109/07388551.2015.1128876
Amann, R. I., Binder, B. J., Olson, R. J., Chisholm, S. W., Devereux, R., and Stahl, D. A. (1990). Combination of 16S rRNA-targeted oligonucleotide probes with flow cytometry for analyzing mixed microbial populations. Appl. Environ. Microbiol. 56, 1919–1925. doi: 10.1128/aem.56.6.1919-1925.1990
Amann, R. I., Ludwig, W., and Schleifer, K.-H. (1995). Phylogenetic identification and in situ detection of individual microbial cells without cultivation. Microbiol. Rev. 59, 143–169. doi: 10.1128/mr.59.1.143-169.1995
Amor, K. B., Vaughan, E. E., and de Vos, W. M. (2007). Advanced molecular tools for the identification of lactic acid bacteria. J. Nutr. 137(3 Suppl. 2) 741S–747S. doi: 10.1093/jn/137.3.741S
Angelov, A. (2014). Assessment of lactic acid bacteria and Enterobacteriaceae counts in bulgarian probiotic products by TEMPO ® system and ISO methods. J. Nutr. Heal. Food Eng. 1, 192–196. doi: 10.15406/jnhfe.2014.01.00029
Baek, K.-H., and Skinner, D. Z. (2012). Production of reactive oxygen species by freezing stress and the protective roles of antioxidant enzymes in plants. J. Agric. Chem. Environ. 2012, 34–40. doi: 10.4236/jacen.2012.11006
Beal, J., Farny, N. G., Haddock-Angelli, T., Selvarajah, V., Baldwin, G. S., Buckley-Taylor, R., et al. (2020). Robust estimation of bacterial cell count from optical density. Commun. Biol. 3:512. doi: 10.1038/s42003-020-01127-5
Bermudez-Brito, M., Plaza-Díaz, J., Muñoz-Quezada, S., Gómez-Llorente, C., and Gil, A. (2012). Probiotic mechanisms of action. Ann. Nutr. Metab. 61, 160–174. doi: 10.1159/000342079
Bibiloni, R., Zavaglia, A. G., and Antoni, G. D. (2001). Enzyme-based most probable number method for the enumeration of Bifidobacterium in dairy products. J. Food Prot. 64, 2001–2006. doi: 10.4315/0362-028x-64.12.2001
Bircher, L., Geirnaert, A., Hammes, F., Lacroix, C., and Schwab, C. (2018). Effect of cryopreservation and lyophilization on viability and growth of strict anaerobic human gut microbes. Microb. Biotechnol. 11, 721–733. doi: 10.1111/1751-7915.13265
Blasco, L., Ferrer, S., and Pardo, I. (2003). Development of specific fluorescent oligonucleotide probes for in situ identification of wine lactic acid bacteria. FEMS Microbiol. Lett. 225, 115–123. doi: 10.1016/S0378-1097(03)00501-9
Blodgett, R. (2020). FDA Bacteriological Analytical Manual (BAM), Appendix 2: Most Probable Number from Serial Dilutions. Available online at: https://www.fda.gov/food/laboratory-methods-food/bam-appendix-2-most-probable-number-serial-dilutions (accessed May 4, 2021).
Bogosian, G., Aardema, N. D., Bourneuf, E. V., Morris, P. J. L., and O’Neil, J. P. (2000). Recovery of hydrogen peroxide-sensitive culturable cells of vibrio vulnificus gives the appearance of resuscitation from a viable but nonculturable state. J. Bacteriol. 182, 5070–5075. doi: 10.1128/jb.182.18.5070-5075.2000
Bouvier, T., and Del Giorgio, P. A. (2003). Factors influencing the detection of bacterial cells using fluorescence in situ hybridization (FISH): a quantitative review of published reports. FEMS Microbiol. Ecol. 44, 3–15. doi: 10.1016/S0168-6496(02)00461-0
Braissant, O., Wirz, D., Göpfert, B., and Daniels, A. U. (2010). Use of isothermal microcalorimetry to monitor microbial activities. FEMS Microbiol. Lett. 303, 1–8. doi: 10.1111/j.1574-6968.2009.01819.x
Breeuwer, P., and Abee, T. (2000). Assessment of viability of microorganisms employing fluorescence techniques. Int. J. Food Microbiol. 55, 193–200. doi: 10.1016/s0168-1605(00)00163-x
Brink, M., Todorov, S. D., Martin, J. H., Senekal, M., and Dicks, L. M. T. (2006). The effect of prebiotics on production of antimicrobial compounds, resistance to growth at low pH and in the presence of bile, and adhesion of probiotic cells to intestinal mucus. J. Appl. Microbiol. 100, 813–820. doi: 10.1111/j.1365-2672.2006.02859.x
Broeckx, G., Vandenheuvel, D., Claes, I. J. J., Lebeer, S., and Kiekens, F. (2016). Drying techniques of probiotic bacteria as an important step towards the development of novel pharmabiotics. Int. J. Pharmaceut. 505, 303–318. doi: 10.1016/j.ijpharm.2016.04.002
Bustin, S. A., Benes, V., Nolan, T., and Pfaffl, M. W. (2005). Quantitative real-time RT-PCR – a perspective. J. Mol. Endocrinol. 34, 597–601. doi: 10.1677/jme.1.01755
Bustos, A. Y., Saavedra, L., de Valdez, G. F., Raya, R. R., and Taranto, M. P. (2012). Relationship between bile salt hydrolase activity, changes in the internal pH and tolerance to bile acids in lactic acid bacteria. Biotechnol. Lett. 34, 1511–1518. doi: 10.1007/s10529-012-0932-5
Charnchai, P., Jantama, S. S., Prasitpuriprecha, C., Kanchanatawee, S., and Jantama, K. (2016). Effects of the food manufacturing chain on the viability and functionality of bifidobacterium animalis through simulated gastrointestinal conditions. PLoS One 11:e0157958. doi: 10.1371/journal.pone.0157958
Coeuret, V., Dubernet, S., Bernardeau, M., Gueguen, M., and Vernoux, J. P. (2003). Isolation, characterisation and identification of lactobacilli focusing mainly on cheeses and other dairy products. Le Lait 83, 269–306. doi: 10.1051/lait:2003019
Corry, J. E. L., Jarvis, B., Passmore, S., and Hedges, A. (2007). A critical review of measurement uncertainty in the enumeration of food micro-organisms. Food Microbiol. 24, 230–253. doi: 10.1016/j.fm.2006.05.003
Davey, H. M. (2011). Life, death, and in-between: meanings and methods in microbiology. Appl. Environ. Microb. 77, 5571–5576. doi: 10.1128/aem.00744-11
Davey, H. M., and Kell, D. B. (1996). Flow cytometry and cell sorting of heterogeneous microbial populations: the importance of single-cell analyses. Microbiol. Rev. 60, 641–696. doi: 10.1128/mr.60.4.641-696.1996
Davis, C. (2014). Enumeration of probiotic strains: review of culture-dependent and alternative techniques to quantify viable bacteria. J. Microbiol. Method 103, 9–17. doi: 10.1016/j.mimet.2014.04.012
de Almada, C. N., Almada, C. N., Martinez, R. C. R., and Sant’Ana, A. S. (2016). Paraprobiotics: evidences on their ability to modify biological responses, inactivation methods and perspectives on their application in foods. Trends Food Sci. Tech. 58, 96–114. doi: 10.1016/j.tifs.2016.09.011
Derrien, M., and van Hylckama Vlieg, J. E. (2015). Fate, activity, and impact of ingested bacteria within the human gut microbiota. Trends Microbiol. 23, 354–366. doi: 10.1016/j.tim.2015.03.002
Díaz, M., Herrero, M., García, L. A., and Quirós, C. (2010). Application of flow cytometry to industrial microbial bioprocesses. Biochem. Eng. J. 48, 385–407. doi: 10.1016/j.bej.2009.07.013
Dolly, P., Anishaparvin, A., Joseph, G. S., and Anandharamakrishnan, C. (2011). Microencapsulation of Lactobacillus plantarum (mtcc 5422) by spray-freeze-drying method and evaluation of survival in simulated gastrointestinal conditions. J. Microencapsul. 28, 568–574. doi: 10.3109/02652048.2011.599435
Entenza, J. M., Bétrisey, B., Manuel, O., Giddey, M., Sakwinska, O., Laurent, F., et al. (2014). Rapid detection of staphylococcus aureus strains with reduced susceptibility to vancomycin by isothermal microcalorimetry. J. Clin. Microbiol. 52, 180–186. doi: 10.1128/jcm.01820-13
Ercolini, D., Villani, F., Aponte, M., and Mauriello, G. (2006). Fluorescence in situ hybridisation detection of Lactobacillus plantarum group on olives to be used in natural fermentations. Int. J. Food Microbiol. 112, 291–296. doi: 10.1016/j.ijfoodmicro.2006.09.003
Fallico, V., Rea, M., Stanton, C., Ilestam, N., and McKinney, J. (2020). Next-generation multiparameter flow cytometry assay improves the assessment of oxidative stress in probiotics. Food Microbiol. 91:103501. doi: 10.1016/j.fm.2020.103501
Fenster, K., Freeburg, B., Hollard, C., Wong, C., Laursen, R. R., and Ouwehand, A. C. (2019). The production and delivery of probiotics: a review of a practical approach. Microorganisms 7:83. doi: 10.3390/microorganisms7030083
Fiore, W., Arioli, S., and Guglielmetti, S. (2020). The neglected microbial components of commercial probiotic formulations. Microorganisms 8:1177. doi: 10.3390/microorganisms8081177
Fittipaldi, M., Nocker, A., and Codony, F. (2012). Progress in understanding preferential detection of live cells using viability dyes in combination with DNA amplification. J. Microbiol. Method 91, 276–289. doi: 10.1016/j.mimet.2012.08.007
Foglia, C., Allesina, S., Amoruso, A., Prisco, A. D., and Pane, M. (2020). New insights in enumeration methodologies of probiotic cells in finished products. J. Microbiol. Methods 175:105993. doi: 10.1016/j.mimet.2020.105993
Fonseca, F., Béal, C., and Corrieu, G. (2000). Method of quantifying the loss of acidification activity of lactic acid starters during freezing and frozen storage. J. Dairy Res. 67, 83–90. doi: 10.1017/s002202999900401x
Fredua-Agyeman, M., and Gaisford, S. (2015). Comparative survival of commercial probiotic formulations: tests in biorelevant gastric fluids and real-time measurements using microcalorimetry. Benef. Microbes 6, 141–151. doi: 10.3920/bm2014.0051
Fredua-Agyeman, M., and Gaisford, S. (2019). Assessing inhibitory activity of probiotic culture supernatants against Pseudomonas aeruginosa: a comparative methodology between agar diffusion, broth culture and microcalorimetry. World J. Microbiol. Biotechnol. 35:49. doi: 10.1007/s11274-019-2621-1
Frese, S. A., MacKenzie, D. A., Peterson, D. A., Schmaltz, R., Fangman, T., Zhou, Y., et al. (2013). Molecular characterization of host-specific biofilm formation in a vertebrate gut symbiont. PLoS Genet. 9:e1004057. doi: 10.1371/journal.pgen.1004057
Fujiki, T., Hirose, Y., Yamamoto, Y., and Murosaki, S. (2012). Enhanced immunomodulatory activity and stability in simulated digestive juices of Lactobacillus plantarum L-137 by heat treatment. Biosci. Biotechnol. Biochem. 76, 918–922. doi: 10.1271/bbb.110919
Fuller, J. B. (2004). Cryoprotectants: the essential antifreezes to protect life in the frozen state. Cryo Lett. 25, 375–388.
Garcia, A. H., Herrmann, A. M., and Håkansson, S. (2017). Isothermal microcalorimetry for rapid viability assessment of freeze-dried Lactobacillus reuteri. Process Biochem. 55, 49–54. doi: 10.1016/j.procbio.2017.01.012
García-Cayuela, T., Tabasco, R., Peláez, C., and Requena, T. (2009). Simultaneous detection and enumeration of viable lactic acid bacteria and bifidobacteria in fermented milk by using propidium monoazide and real-time PCR. Int. Dairy J. 19, 405–409. doi: 10.1016/j.idairyj.2009.02.001
García-Hernández, J., Moreno, Y., Amorocho, C. M., and Hernández, M. (2012). A combination of direct viable count and fluorescence in situ hybridization for specific enumeration of viable Lactobacillus delbrueckii subsp. bulgaricus and Streptococcus thermophilus. Lett. Appl. Microbiol. 54, 247–254. doi: 10.1111/j.1472-765x.2011.03201.x
Gibson, U. E. M., Heid, C. A., and Williams, P. M. (1996). A novel method for real time quantitative RT-PCR. Genome Res. 6, 995–1001. doi: 10.1101/gr.6.10.995
Gobert, G., Cotillard, A., Fourmestraux, C., Pruvost, L., Miguet, J., and Boyer, M. (2018). Droplet digital PCR improves absolute quantification of viable lactic acid bacteria in faecal samples. J. Microbiol. Methods 148, 64–73. doi: 10.1016/j.mimet.2018.03.004
Gul, O., and Atalar, I. (2019). Different stress tolerance of spray and freeze dried Lactobacillus casei Shirota microcapsules with different encapsulating agents. Food Sci. Biotechnol. 28, 807–816. doi: 10.1007/s10068-018-0507-x
Hansen, S. J. Z., Morovic, W., DeMeules, M., Stahl, B., and Sindelar, C. W. (2018). Absolute enumeration of probiotic strains Lactobacillus acidophilus NCFM ® and Bifidobacterium animalis subsp. lactis Bl-04 ® via Chip-Based Digital PCR. Front. Microbiol. 9:704. doi: 10.3389/fmicb.2018.00704
Hansen, S. J. Z., Tang, P., Kiefer, A., Galles, K., Wong, C., and Morovic, W. (2020). Droplet digital PCR is an improved alternative method for high-quality enumeration of viable probiotic strains. Front. Microbiol. 10:3025. doi: 10.3389/fmicb.2019.03025
Hernández, A., Larsson, C. U., Sawicki, R., van Niel, E. W. J., Roos, S., and Håkansson, S. (2019). Impact of the fermentation parameters pH and temperature on stress resilience of Lactobacillus reuteri DSM 17938. Amb. Express 9:66. doi: 10.1186/s13568-019-0789-2
Hill, C., Guarner, F., Reid, G., Gibson, G. R., Merenstein, D. J., Pot, B., et al. (2014). The international scientific association for probiotics and prebiotics consensus statement on the scope and appropriate use of the term probiotic. Nat. Rev. Gastroenterol. 11, 506–514. doi: 10.1038/nrgastro.2014.66
Hindson, B. J., Ness, K. D., Masquelier, D. A., Belgrader, P., Heredia, N. J., Makarewicz, A. J., et al. (2011). High-throughput droplet digital PCR system for absolute quantitation of DNA copy number. Anal. Chem. 83, 8604–8610. doi: 10.1021/ac202028g
Huang, S., Gaucher, F., Cauty, C., Jardin, J., Loir, Y. L., Jeantet, R., et al. (2018). Growth in hyper-concentrated sweet whey triggers multi stress tolerance and spray drying survival in Lactobacillus casei BL23: from the molecular basis to new perspectives for sustainable probiotic production. Front. Microbiol. 9:2548. doi: 10.3389/fmicb.2018.02548
Huggett, J. F., Foy, C. A., Benes, V., Emslie, K., Garson, J. A., Haynes, R., et al. (2013). The digital MIQE guidelines: minimum information for publication of quantitative digital PCR experiments. Clin. Chem. 59, 892–902. doi: 10.1373/clinchem.2013.206375
Iaconelli, C., Lemetais, G., Kechaou, N., Chain, F., Bermúdez-Humarán, L. G., Langella, P., et al. (2015). Drying process strongly affects probiotics viability and functionalities. J. Biotechnol. 214, 17–26. doi: 10.1016/j.jbiotec.2015.08.022
Ilango, S., Pandey, R., and Antony, U. (2016). Functional characterization and microencapsulation of probiotic bacteria from koozh. J. Food Sci. Technol. 53, 977–989. doi: 10.1007/s13197-015-2169-5
ISO (1998). ISO 15214:1998 Microbiology of Food and Animal Feeding Stuffs — Horizontal Method for the Enumeration of Mesophilic Lactic Acid Bacteria — Colony-Count Technique at 30 Degrees C. Geneva: International Organization for Standardization.
ISO (2006). ISO 20128:2006 [IDF 192:2006] Milk Products — Enumeration of Presumptive Lactobacillus Acidophilus on a Selective Medium — Colony-Count Technique at 37 degrees C. Geneva: International Organization for Standardization.
ISO (2009). ISO 26323:2009 [IDF 213:2009] Milk Products — Determination of the Acidification Activity of Dairy Cultures by Continuous pH Measurement (CpH). Geneva: International Organization for Standardization.
ISO (2010). ISO 29981:2010 [IDF 220:2010] Milk Products — Enumeration of Presumptive Bifidobacteria — Colony Count Technique at 37 Degrees C. Geneva: International Organization for Standardization.
ISO (2015). ISO 19344:2015 [IDF 232:2015] Milk and Milk Products — Starter Cultures, Probiotics and Fermented Products — Quantification of Lactic Acid Bacteria by Flow Cytometry. Geneva: International Organization for Standardization.
Jackson, M. S., Bird, A. R., and McOrist, A. L. (2002). Comparison of two selective media for the detection and enumeration of Lactobacilli in human faeces. J. Microbiol. Method 51, 313–321. doi: 10.1016/s0167-7012(02)00102-1
Jackson, S. A., Schoeni, J. L., Vegge, C., Pane, M., Stahl, B., Bradley, M., et al. (2019). Improving end-user trust in the quality of commercial probiotic products. Front. Microbiol. 10:739. doi: 10.3389/fmicb.2019.00739
Jacobsen, C. N., Nielsen, V. R., Hayford, A. E., Michaelsen, K. F., Møller, P. L., Pærregaard, A., et al. (1999). Screening of probiotic activities of forty-seven strains of Lactobacillus spp. by in vitro techniques and evaluation of the colonization ability of five selected strains in humans. Appl. Environ. Microbiol. 65, 4949–4956. doi: 10.1128/AEM.65.11.4949-4956.1999
Keer, J. T., and Birch, L. (2003). Molecular methods for the assessment of bacterial viability. J. Microbiol. Methods 53, 175–183. doi: 10.1016/s0167-7012(03)00025-3
Kell, D. B., Kaprelyants, A. S., Weichart, D. H., Harwood, C. R., and Barer, M. R. (1998). Viability and activity in readily culturable bacteria: a review and discussion of the practical issues. Ant. Leeuwenhoek 73, 169–187. doi: 10.1023/a:1000664013047
Kiefer, A., Tang, P., Arndt, S., Fallico, V., and Wong, C. (2020). Optimization of viability treatment essential for accurate droplet digital PCR enumeration of probiotics. Front. Microbiol. 11:1811. doi: 10.3389/fmicb.2020.01811
Kim, M., Nam, D., Kim, S., Im, P., Choe, J., and Choi, A. (2018). Enhancement of viability, acid, and bile tolerance and accelerated stability in lyophilized Weissella cibaria JW15 with protective agents. Food Sci. Nutr. 6, 1904–1913. doi: 10.1002/fsn3.762
Kogure, K., Simidu, U., and Taga, N. (1979). A tentative direct microscopic method for counting living marine bacteria. Can. J. Microbiol. 25, 415–420. doi: 10.1139/m79-063
Kolaček, S., Hojsak, I., Canani, R. B., Guarino, A., Indrio, F., Orel, R., et al. (2017). Commercial probiotic products: a call for improved quality control. a position paper by the ESPGHAN working group for probiotics and prebiotics. J. Pediatr. Gastr. Nutr. 65, 117–124. doi: 10.1097/mpg.0000000000001603
Kramer, M., Obermajer, N., Matijašić, B. B., Rogelj, I., and Kmetec, V. (2009). Quantification of live and dead probiotic bacteria in lyophilised product by real-time PCR and by flow cytometry. Appl. Microbiol. Biol. 84, 1137–1147. doi: 10.1007/s00253-009-2068-7
Lahtinen, S. J. (2012). Probiotic viability – does it matter? Microb. Ecol. Health Dis. 18:23. doi: 10.3402/mehd.v23i0.18567
Lahtinen, S. J., Ahokoski, H., Reinikainen, J. P., Gueimonde, M., Nurmi, J., Ouwehand, A. C., et al. (2008). Degradation of 16S rRNA and attributes of viability of viable but nonculturable probiotic bacteria. Lett. Appl. Microbiol. 46, 693–698. doi: 10.1111/j.1472-765x.2008.02374.x
Lahtinen, S. J., Ouwehand, A. C., Reinikainen, J. P., Korpela, J. M., Sandholm, J., and Salminen, S. J. (2006). Intrinsic properties of so-called dormant probiotic bacteria, determined by flow cytometric viability assays. Appl. Environ. Microb. 72, 5132–5134. doi: 10.1128/aem.02897-05
Li, F., Cheng, C. C., Zheng, J., Liu, J., Quevedo, R. M., Li, J., et al. (2021). Limosilactobacillus balticus sp. nov., Limosilactobacillus agrestis sp. nov., Limosilactobacillus albertensis sp. nov., Limosilactobacillus rudii sp. nov. and Limosilactobacillus fastidiosus sp. nov., five novel Limosilactobacillus species isolated from the vertebrate gastrointestinal tract, and proposal of six subspecies of Limosilactobacillus reuteri adapted to the gastrointestinal tract of specific vertebrate hosts. Int. J. Syst. Evol. Microbiol. 71:004644. doi: 10.1099/ijsem.0.004644
Lipson, D. A. (2015). The complex relationship between microbial growth rate and yield and its implications for ecosystem processes. Front. Microbiol. 6:615. doi: 10.3389/fmicb.2015.00615
Maciorowski, Z., Chattopadhyay, P. K., and Jain, P. (2017). Basic multicolor flow cytometry. Curr. Protoc. Immunol. 117, 5.4.1–5.4.38. doi: 10.1002/cpim.26
Marteau, P., Minekus, M., Havenaar, R., and Veld, J. H. J. H. I. (1997). Survival of lactic acid bacteria in a dynamic model of the stomach and small intestine: validation and the effects of bile. J. Dairy Sci. 80, 1031–1037. doi: 10.3168/jds.s0022-0302(97)76027-2
Mihhalevski, A., Sarand, I., Viiard, E., Salumets, A., and Paalme, T. (2011). Growth characterization of individual rye sourdough bacteria by isothermal microcalorimetry. J. Appl. Microbiol. 110, 529–540. doi: 10.1111/j.1365-2672.2010.04904.x
Minelli, E. B., and Benini, A. (2009). Relationship between number of bacteria and their probiotic effects. Microb. Ecol. Health D 20, 180–183. doi: 10.1080/08910600802408095
Moter, A., and Göbel, U. B. (2000). Fluorescence in situ hybridization (FISH) for direct visualization of microorganisms. J. Microbiol. Methods 41, 85–112. doi: 10.1016/s0167-7012(00)00152-4
Nocker, A., Sossa-Fernandez, P., Burr, M. D., and Camper, A. K. (2007). Use of propidium monoazide for live/dead distinction in microbial ecology. Appl. Environ. Microb. 73, 5111–5117. doi: 10.1128/aem.02987-06
Nogva, H. K., Drmtorp, S. M., Nissen, H., and Rudi, K. (2003). Ethidium monoazide for DNA-based differentiation of viable and dead bacteria by 5-nuclease PCR. Biotechniques 34, 804–813. doi: 10.2144/03344rr02
Nykyri, J., Herrmann, A. M., and Håkansson, S. (2019). Isothermal microcalorimetry for thermal viable count of microorganisms in pure cultures and stabilized formulations. BMC Microbiol. 19:65. doi: 10.1186/s12866-019-1432-8
Obruca, S., Sedlacek, P., Krzyzanek, V., Mravec, F., Hrubanova, K., Samek, O., et al. (2016). Accumulation of Poly(3-hydroxybutyrate) helps bacterial cells to survive freezing. PLoS One 11:e0157778. doi: 10.1371/journal.pone.0157778
Olszewska, M. A., Kocot, A. M., Nynca, A., and Łaniewska-Trokenheim, Ł (2016). Utilization of physiological and taxonomic fluorescent probes to study Lactobacilli cells and response to pH challenge. Microbiol. Res. 192, 239–246. doi: 10.1016/j.micres.2016.07.011
Olszewska, M. A., Nynca, A., Białobrzewski, I., Kocot, A. M., and Łaguna, J. (2019). Assessment of the bacterial viability of chlorine- and quaternary ammonium compounds-treated Lactobacillus cells via a multi-method approach. J. Appl. Microbiol. 126, 1070–1080. doi: 10.1111/jam.14208
Papadimitriou, K., Zoumpopoulou, G., Foligné, B., Alexandraki, V., Kazou, M., Pot, B., et al. (2015). Discovering probiotic microorganisms: in vitro, in vivo, genetic and omics approaches. Front. Microbiol. 6:58. doi: 10.3389/fmicb.2015.00058
Pegg, D. E., Fahy, G. M., Wowk, B., Benson, J. D., Adams, G. D. J., Cook, S., et al. (2015). “Cryopresevation and freeze-drying protocols,” in Methods in Molecular Biology, eds W. F. Wolkers and H. Oldenhof (New York, NY: Humana Press), doi: 10.1007/978-1-4939-2193-5
Piqué, N., Berlanga, M., and Miñana-Galbis, D. (2019). Health benefits of heat-killed (Tyndallized) probiotics: an overview. Int. J. Mol. Sci. 20:2534. doi: 10.3390/ijms20102534
Postollec, F., Falentin, H., Pavan, S., Combrisson, J., and Sohier, D. (2011). Recent advances in quantitative PCR (qPCR) applications in food microbiology. Food Microbiol. 28, 848–861. doi: 10.1016/j.fm.2011.02.008
Rittershaus, E. S. C., Baek, S., and Sassetti, C. M. (2013). The normalcy of dormancy. Cell Host Microbe 13, 643–651.
Rosander, A., Connolly, E., and Roos, S. (2008). Removal of antibiotic resistance gene-carrying plasmids from Lactobacillus reuteri ATCC 55730 and characterization of the resulting daughter strain, L. reuteri DSM 17938. Appl. Environ. Microb. 74, 6032–6040. doi: 10.1128/aem.00991-08
Rosenstiel, P., and Stange, E. F. (2010). Probiotics and intestinal diseases. Ann. Nutr. Metab. 57, 27–28. doi: 10.1159/000309094
Rudi, K., Moen, B., Drømtorp, S. M., and Holck, A. L. (2005). Use of ethidium monoazide and pcr in combination for quantification of viable and dead cells in complex samples. Appl. Environ. Microb. 71, 1018–1024. doi: 10.1128/aem.71.2.1018-1024.2005
Ruiz, L., Margolles, A., and Sánchez, B. (2013). Bile resistance mechanisms in Lactobacillus and Bifidobacterium. Front. Microbiol. 4:396. doi: 10.3389/fmicb.2013.00396
Saarela, M., Virkajärvi, I., Alakomi, H. L., Mattila-Sandholm, T., Vaari, A., Suomalainen, T., et al. (2005). Influence of fermentation time, cryoprotectant and neutralization of cell concentrate on freeze-drying survival, storage stability, and acid and bile exposure of Bifidobacterium animalis ssp. lactis cells produced without milk-based ingredients. J. Appl. Microbiol. 99, 1330–1339. doi: 10.1111/j.1365-2672.2005.02742.x
Saarela, M. H., Alakomi, H. L., Puhakka, A., and Mättö, J. (2009). Effect of the fermentation pH on the storage stability of Lactobacillus rhamnosus preparations and suitability of in vitro analyses of cell physiological functions to predict it. J. Appl. Microbiol. 106, 1204–1212. doi: 10.1111/j.1365-2672.2008.04089.x
Saez-Lara, M. J., Gomez-Llorente, C., Plaza-Diaz, J., and Gil, A. (2015). The role of probiotic lactic acid bacteria and bifidobacteria in the prevention and treatment of inflammatory bowel disease and other related diseases: a systematic review of randomized human clinical trials. Biomed. Res. Int. 2015:505878. doi: 10.1155/2015/505878
Santivarangkna, C., Kulozik, U., and Foerst, P. (2008). Inactivation mechanisms of lactic acid starter cultures preserved by drying processes. J. Appl. Microbiol. 105, 1–13. doi: 10.1111/j.1365-2672.2008.03744.x
Segers, M. E., and Lebeer, S. (2014). Towards a better understanding of Lactobacillus rhamnosus GG - host interactions. Microb Cell Fact. 13, S7–S7. doi: 10.1186/1475-2859-13-s1-s7
Senoh, M., Ghosh-Banerjee, J., Ramamurthy, T., Colwell, R. R., Miyoshi, S., Nair, G. B., et al. (2012). Conversion of viable but nonculturable enteric bacteriato culturable by co-culture with eukaryotic cells. Microbiol. Immunol. 56, 342–345. doi: 10.1111/j.1348-0421.2012.00440.x
Shapiro, H. (2003). Practical Flow Cytometry, 4th Edn. Hoboken, NJ: John Wiley & Sons, Inc, doi: 10.3182/20131111-3-kr-2043.90001
Squires, R. W., and Hartsell, S. E. (1955). Survival and growth initiation of defrosted Escherichia coli as affected by frozen storage menstrua. Appl. Microbiol. 3, 40–45. doi: 10.1128/am.3.1.40-45.1955
Stage, M., Wichmann, A., Jørgensen, M., Vera-Jimenéz, N. I., Wielje, M., Nielsen, D. S., et al. (2020). Lactobacillus rhamnosus GG genomic and phenotypic stability in an industrial production process. Appl. Environ. Microbiol. 86, e2780–e2719. doi: 10.1128/aem.02780-19
Staley, J., and Konopka, A. (1985). Measurement of in situ activities of nonphotosynthetic microorganisms in aquatic and terrestrial habitats. Annu. Rev. Microbiol. 39, 321–346. doi: 10.1146/annurev.micro.39.1.321
Sutton, S. (2010). The most probable number method and its uses in enumeration, qualification, and validation. Microbiol. Top. 16:36.
Taranto, M. P., Murga, M. L. F., Lorca, G., and Valdez, G. F. (2003). Bile salts and cholesterol induce changes in the lipid cell membrane of Lactobacillus reuteri. J. Appl. Microbiol. 95, 86–91. doi: 10.1046/j.1365-2672.2003.01962.x
Taverniti, V., and Guglielmetti, S. (2011). The immunomodulatory properties of probiotic microorganisms beyond their viability (ghost probiotics: proposal of paraprobiotic concept). Genes Nutr. 6, 261–274. doi: 10.1007/s12263-011-0218-x
Tee, W., Nazaruddin, R., Tan, Y., and Ayob, M. (2013). Effects of encapsulation on the viability of potential probiotic Lactobacillus plantarum exposed to high acidity condition and presence of bile salts. Food Sci. Technol. Int. 20, 399–404. doi: 10.1177/1082013213488775
Tracy, B. P., Gaida, S. M., and Papoutsakis, E. T. (2010). Flow cytometry for bacteria: enabling metabolic engineering, synthetic biology and the elucidation of complex phenotypes. Curr. Opin. Biotech. 21, 85–99. doi: 10.1016/j.copbio.2010.02.006
Turchi, B., Mancini, S., Fratini, F., Pedonese, F., Nuvoloni, R., Bertelloni, F., et al. (2013). Preliminary evaluation of probiotic potential of Lactobacillus plantarum strains isolated from Italian food products. World J. Microbiol. Biotechnol. 29, 1913–1922. doi: 10.1007/s11274-013-1356-7
Villarreal, M. L. M., Padilha, M., Vieira, A. D. S., Franco, B. D. G., de, M., Martinez, R. C. R., et al. (2013). Advantageous direct quantification of viable closely related probiotics Inpetit-Suisse cheeses under in vitro gastrointestinal conditions by propidium monoazide - qPCR. PLoS One 8:e82102. doi: 10.1371/journal.pone.0082102
Vinderola, G., Reinheimer, J., and Salminen, S. (2019). The enumeration of probiotic issues: from unavailable standardised culture media to a recommended procedure? Int. Dairy J. 96, 58–65. doi: 10.1016/j.idairyj.2019.04.010
von Ah, U., Wirz, D., and Daniels, A. U. (2008). Rapid MSSA-MRSA differentiation and MIC determinations by isothermal microcalorimetry. J. Clin. Microbiol. 46, 2083–2087. doi: 10.1128/JCM.00611-08
WHO and FAO (2006). Probiotics in Food: Health and Nutritional Properties and Guidelines for Evaluation. Rome: WHO.
Wittwer, C. T., Herrmann, M. G., Moss, A. A., and Rasmussen, R. P. (1997). Continuous fluorescence monitoring of rapid cycle DNA amplification. Biotechniques 22, 130–138. doi: 10.2144/97221bi01
Keywords: probiotic, lactic acid bacteria, enumeration, viability, viable but not culturable, activity, microbiological methods, stress tolerance
Citation: Wendel U (2022) Assessing Viability and Stress Tolerance of Probiotics—A Review. Front. Microbiol. 12:818468. doi: 10.3389/fmicb.2021.818468
Received: 19 November 2021; Accepted: 27 December 2021;
Published: 27 January 2022.
Edited by:
Giorgio Giraffa, Council for Agricultural and Economics Research (CREA), ItalyReviewed by:
Paola Mattarelli, University of Bologna, ItalyValentina Taverniti, University of Milan, Italy
Copyright © 2022 Wendel. This is an open-access article distributed under the terms of the Creative Commons Attribution License (CC BY). The use, distribution or reproduction in other forums is permitted, provided the original author(s) and the copyright owner(s) are credited and that the original publication in this journal is cited, in accordance with accepted academic practice. No use, distribution or reproduction is permitted which does not comply with these terms.
*Correspondence: Ulrika Wendel, dXdAYmlvZ2FpYS5zZQ==