- 1AgResearch Limited, Grasslands Research Centre, Palmerston North, New Zealand
- 2IFEVA, CONICET, Facultad de Agronomía, Universidad de Buenos Aires, Buenos Aires, Argentina
- 3Laboratorio de Ecología Integrativa, Instituto de Ciencias Biológicas, Universidad de Talca, Talca, Chile
- 4Centro de Estudios Avanzados en Zonas Áridas (CEAZA), Universidad Católica del Norte, Coquimbo, Chile
- 5Centro de Investigación de Estudios Avanzados del Maule, Universidad Católica del Maule, Talca, Chile
Seeds commonly harbour diverse bacterial communities that can enhance the fitness of future plants. The bacterial microbiota associated with mother plant’s foliar tissues is one of the main sources of bacteria for seeds. Therefore, any ecological factor influencing the mother plant’s microbiota may also affect the diversity of the seed’s bacterial community. Grasses form associations with beneficial vertically transmitted fungal endophytes of genus Epichloë. The interaction of plants with Epichloë endophytes and insect herbivores can influence the plant foliar microbiota. However, it is unknown whether these interactions (alone or in concert) can affect the assembly of bacterial communities in the produced seed. We subjected Lolium multiflorum plants with and without its common endophyte Epichloë occultans (E+, E-, respectively) to an herbivory treatment with Rhopalosiphum padi aphids and assessed the diversity and composition of the bacterial communities in the produced seed. The presence of Epichloë endophytes influenced the seed bacterial microbiota by increasing the diversity and affecting the composition of the communities. The relative abundances of the bacterial taxa were more similarly distributed in communities associated with E+ than E- seeds with the latter being dominated by just a few bacterial groups. Contrary to our expectations, seed bacterial communities were not affected by the aphid herbivory experienced by mother plants. We speculate that the enhanced seed/seedling performance documented for Epichloë-host associations may be explained, at least in part, by the Epichloë-mediated increment in the seed-bacterial diversity, and that this phenomenon may be applicable to other plant-endophyte associations.
Introduction
Plant seeds commonly harbour a diverse community of microbes including bacteria and fungi (Nelson, 2018). The seed-associated microbes are generally critical for plant fitness since they can confer protection against herbivores and pathogens, increase germination, enhance seedlings growth, and increase plant tolerance to stresses (Truyens et al., 2015; Bastias et al., 2017; Rochefort et al., 2021). Members of the seed microbiota can be acquired from different sources namely certain mother plant tissues, plant reproductive structures and the environment (Shade et al., 2017; Fort et al., 2021). Those that are inherited from mother plants are termed as ‘vertically transmitted microbes’, whereas those recruited from the external environment are termed as ‘horizontally transmitted microbes’ (Shade et al., 2017). Since the mother plant’s microbiota is one of the main sources of microbes for seeds, any ecological factor influencing this source could eventually affect the diversity and composition of the seed’s microbial community (Chesneau et al., 2020; Fort et al., 2021). In fact, it is well documented that plant leaf microbial communities can be influenced by the interaction of plants with beneficial persistent microorganisms and herbivores (Nissinen et al., 2019; Seabloom et al., 2019; Humphrey and Whiteman, 2020). However, it is not fully understood whether the effects of these ecological factors on the mother plant microbiota can persist and affect the assemblies of microbes associated with the produced seeds (Wei and Jousset, 2017). A remarkable symbiotic system to evaluate the effects of ecological interactions experienced by mother plants on seed-associated microbial communities is the association between cool-season grasses and asexual fungal endophytes of the genus Epichloë. These endophytes are persistent symbionts of grasses (they grow in foliar host tissues during the entire plant life) and are vertically transmitted through the seeds (Schardl et al., 2004). Because Epichloë endophytes confer antiherbivore protection and can modulate the leaf microbiota of mother plants (e.g., Bastias et al., 2017; Nissinen et al., 2019), we hypothesise that these symbionts may also influence the diversity and composition of the seed microbiota.
The potential effects of Epichloë endophytes on the diversity and composition of seed microbial communities have been barely explored (e.g., Tannenbaum et al., 2021). Epichloë may affect the seed microbiota though its effects on the microbial assemblies of mother plants. For example, the presence of Epichloë coenophiala endophytes modified the leaf-associated fungal communities in Festuca arundinacea plants (Syn. Schedonorus phoenix; Nissinen et al., 2019). In addition, Epichloë festucae var. lolii endophytes re-organised the bacterial communities associated with seedlings of Lolium perenne (Tannenbaum et al., 2020). Epichloë symbionts may also affect the seed microbiota via the transport of certain endophytic microbes into the developing seed. At the host reproductive stage, Epichloë extend hyphae into fertilised ovaries and later, into embryos and other seed structures as part of the vertical transmission process (Gundel et al., 2011b; Liu et al., 2017). Therefore, microbes located on fungal hyphae of Epichloë spp. could eventually be transmitted to seeds. In fact, bacteria inhabiting the external surface of Epichloë mycelia have been recently isolated (Bastías et al., 2020). The potential regulation of the seed microbiota by Epichloë endophytes could also arise due to Epichloë-mediated changes on seed chemical composition. Epichloë endophytes can modify the concentrations of sugars (i.e., mannitol, ribitol and thehalose) and antioxidants (i.e., tocochromanols and glutathione) in seeds (Gundel et al., 2018; Zhang et al., 2019; Hettiarachchige et al., 2021). In addition, the accumulation of Epichloë-derived alkaloids has been shown to modulate bacterial communities associated with host plant leaves (Roberts and Lindow, 2014) and as such may also influence the seed microbiota (Hewitt et al., 2020). Epichloë could also modify the seed microbial composition via competition for plant resources. The successful competition for plant resources by Epichloë occultans endophytes seemed to exclude the pathogen Claviceps purpurea from Lolium multiflorum plant flowers (Pérez et al., 2013).
The interaction of mother plants with herbivores can also affect their foliar-associated microbial communities and, eventually, the microbiota of their produced seed. For example, the bacterial diversity in the leaves of Cardamine cordifolia plants was modified by the attack of the foliar chewing insect Scaptomyza nigrita (Humphrey and Whiteman, 2020). The herbivore-mediated changes in leaf plant microbiota could be explained by the activation of plants defences (acting negatively on microbes), the release of plant nutrients from damaged tissues, and the inoculation of microbes from the herbivore into plant tissues (e.g., microbes inhabiting its oral secretions; Smets and Koskella, 2020). In the case of the interaction between C. cordifolia plants and S. nigrita insects, the plant activation of defences responses due to herbivore attack (i.e., activation of jasmonic acid-dependent responses), explained the changes in bacterial diversity (Humphrey and Whiteman, 2020). Interestingly, since Epichloë spp. can reduce herbivore pressure on plants, due to the production of anti-herbivore alkaloids (Bastias et al., 2017), the effects of herbivores on the mother plant’s microbiota (and eventually on their produced seeds) might be reduced in Epichloë infected associations. In regard to plant defences, it is important to underscore that Epichloë can also modulate host plant immune responses (i.e., salicylic acid and jasmonic acid signalling pathways; Bastias et al., 2017). This endophyte-modulation of host plant immunity could affect directly the mother plant’s microbiota via excluding microbial groups (Shi et al., 2020; Kou et al., 2021), and/or indirectly though changing the levels of plant resistance to herbivores (e.g., Bastías et al., 2018).
Here, we studied the effects of the interaction between Epichloë endophytes and insect herbivory experienced by mother plants on the diversity and composition of seed-associated bacterial communities. We subjected mother plants of L. multiflorum (common name: Italian ryegrass) with and without its common endophyte E. occultans to a challenge with Rhopalosiphum padi aphids to further characterise the bacterial communities associated with seed produced by these plants. This aphid species commonly interacts with the grass L. multiflorum in natural grasslands and cultivated pastures (Omacini et al., 2001; Weibull, 1993). Epichloë occultans is a vertically transmitted fungus that produces loline alkaloids, compounds with known bioactivity against R. padi aphids (Bastías et al., 2017). Due to the documented effects of Epichloë endophytes on the mother plant-associated microbiota and seed biochemical composition, we hypothesise that seed-associated bacterial microbiota will be influenced by the Epichloë presence. We also hypothesise that assemblies of seed-associated bacterial communities will vary due to the herbivory history of mother plants. However, since Epichloë endophytes also modulate plant-herbivore interactions, the potential effect of the herbivory experienced by mother plants on seed-associated bacterial communities may be attenuated.
Materials and Methods
Biological Material
We used the symbiotic interaction between L. multiflorum and the fungal endophyte E. occultans as our study system. Lolium multiflorum is an annual grass native to Mediterranean region (currently worldwide distributed in ecosystems with temperate humid climates), and naturally associated with the fungal endophyte E. occultans (Moon et al., 2000). The vertical transmission efficiency of this endophyte species is usually high (close to 100%; Gundel et al., 2011a).
In our group, we maintain two plant biotypes for experimentation, endophyte-symbiotic (E+) and endophyte-free (E-) plants. Endophyte free plants were initially obtained by removing the endophyte from naturally E+ plants with a systemic fungicide (Gundel et al., 2012). Since then, we have been cultivating every year E+ and E- plants as to produce fresh seeds for experiments. We grow and maintain 25 E+ and 25 E- plants during the whole growing cycle to produce seeds. The plants are randomly sowed on a square plot (distance between plants: 0.50 m). The plot is maintained clean from weeds, and plants are watered on demand. Biotypes are maintained as true to type since E. occultans is only vertically transmitted through the seed (this endophyte species cannot be horizontally transmitted between plant biotypes; Gundel et al., 2011b). Furthermore, L. multiflorum is a self-incompatible and wind-pollinated species, thus the exchange of pollen between plants at flowering avoids the genetic differentiation between the E+ and E- biotypes (Gundel et al., 2012). Ripened seeds from each individual plant are harvested every year and the endophytic status assessed (using the ‘seed squash’ technique) prior to generate E+ and E- pools of seed for experimentation (Card et al., 2011).
We used the generalist phytophagous herbivore aphid R. padi L. (Hemiptera; Aphididae) for experimentation. This aphid species is common pest in cereals and grasses (Dixon, 1971; van Emden and Harrington, 2017). Rhopalosiphum padi aphids were collected from volunteer plants, transferred to Petri plates containing leaves of Avena sativa L., and maintained for 5 days to eliminate parasitised insects. Apterous adults free of parasites were placed on young A. sativa plants to initiate the insect population for experimentation. Plants were periodically watered and constantly replaced by healthy ones. The aphid populations were grown under optimal controlled conditions [temperature: 23°C (±1), photoperiod: L16-D8 h, radiation: 150 μmol m−2 s−1].
Experimental Design
We sowed six E+ and six E- plants. Each L. multiflorum plant was individually grown in 3 L pots filled with a commercial potting mix substrate. The experiment was carried out outdoors (at spring season), placing plants on 1-m-high benches, and maintaining 0.2 m of distance between pots. One month after sowing, three plants of each symbiotic biotype were randomly assigned to any of the following treatments: herbivore-free (H-) or herbivory-challenged (H+) with R. padi aphids. Plants were at tillering stage with 6–10 tillers each. In the herbivory treatment, 10 apterous adult aphids were placed on each plant, and resulting populations were allowed to grow for 21 days. Each plant was individually enclosed with a tubular plastic net (0.05 mm mesh) to maintain the aphids under confinement, and to avoid the entrance of any external insect. At day 21, aphids and tubular nets were removed from the experimental plants, and these plants continued growing in the same conditions (on benches, outdoors) until the end of the growing cycle (they were periodically inspected to avoid water deficit and pest attacks). At flowering stage, plants were allowed to exchange pollen freely (with experimental and surrounding plants). At the end of the growing cycle, plants were characterised measuring dry weight (DW) shoot biomass, seed production, and the Epichloë presence within the produced seed. Shoot plant biomass was determined after drying the foliar tissues in an oven (60–70°C) for 48 h. Mature seeds were harvested from each mother plant and placed in paper bags. All the seed bags (n = 12) were stored at 4°C in plastic boxes containing silica gels until DNA extraction (see below). Endophyte presence was determined by inspecting 10 seeds per mother plant using the ‘seed squash’ technique (Card et al., 2011).
Characterisation of Seed-Associated Bacterial Communities
DNA Extraction, Library Preparation and Sequencing
Bacterial communities were characterised by sequencing the bacterial 16S ribosomal RNA (rRNA) gene though Illumina MiSeq sequencer (paired end, 2x300bp; Illumina, United States). For the DNA extraction, 25 seeds were randomly selected from each seed lot. Seeds were surface sterilised with 70% ethanol solution (Ma et al., 2015), and homogenised with sterile ceramic beads using a TissueLyser II (Qiagen, United States). DNA was extracted on 50 mg of homogenised seed material using the GenElute Plant Genomic DNA Miniprep kit (Sigma-Aldrich, United States) following manufacturer’s instructions. Samples were processed under laminar flow chambers to avoid environmental contamination. DNA integrity was assessed by electrophoresis on 1% agarose gels while DNA purity and concentration were checked with a Nanodrop spectrophotometer (Nanodrop Technologies, United States). Libraries for sequencing were prepared by Macrogen Inc. (Seoul, South Korea) using universal primers targeting the V3/V4 region within the 16S rRNA gene sequence (primers 341F: CCTACGGGNGGCWGCAG; 805R: GACTACHVGGGTATCTAATCC). These primers have been frequently used in bacterial metabarcoding studies (Herlemann et al., 2011).
Processing of Sequencing Data
The sequencing instrument produced ~1.22 million read pairs with an average of 102 K read pairs per sample. The amplicon reads were processed using a modified pipeline from Camarinha-Silva et al. (2012). The sequence reads were paired using the program FLASH2 (Magoč and Salzberg, 2011), and the paired reads were quality trimmed using Trimmomatic (Bolger et al., 2014). The trimmed reads were reformatted into FASTA, and read headers were modified to include sample names. All reads were compiled in a single file. The Mothur program suit was used to remove reads with homopolymers longer than 10 nucleotides, and to collapse the reads into unique representatives (Schloss et al., 2009). The Swarm program was used to cluster the collapsed reads (Mahé et al., 2014). The clustered reads were filtered based on their abundances, maintaining the reads that were (a) present in one sample with a relative abundance >0.1%, (b) present in >2% of the samples with a relative abundance >0.01% or (c) present in 5% of the samples at any abundance level. The selected reads were annotated using the QIIME program (version 1.9.1) with the Silva database (version 138; Caporaso et al., 2010; Quast et al., 2013). A Ruby program that implements the above-described abundance filter is available in the AgResearch Gitea website: https://gitea.agresearch.co.nz/JAUREGUIR/Microbiomics. The taxonomic affiliation of each operational taxonomic units (OTU) was verified using the Ribosomal Database Project (RDP) classifier and the 16S rRNA training set 18 as reference database (Wang et al., 2007). OTUs from chloroplast or mitochondrial plant sequences were removed from the database prior to analyses.
The final table included 99 bacterial OTUs representing 282,784 high-quality sequence reads (see Supplementary Table S1). All rarefaction curves associated with seed samples reached asymptotes (number of OTUs vs. number of sequencing reads) (Supplementary Figure S1), indicating that the sequencing depth described most of the bacterial assemblages (Wooley et al., 2010).
A phylogenetic tree was constructed for dominant seed-associated bacterial taxonomic groups using MEGA X software (Kumar et al., 2018). The sequence alignments were performed with MAFFT in Geneious software using default parameters (Kearse et al., 2012). Phylogenetic reconstruction was carried out using the Unweighted Pair Group Method with Arithmetic mean (UPGMA) algorithm with a bootstrap test (9,999 replicates) and default parameters (Hall, 2013).
Statistical Analyses
The effect of the plant symbiosis status and the herbivory history on the response variables ‘plant shoot biomass’, ‘seed production’, ‘bacterial abundance (number of reads)’, ‘species richness (number of OTUs)’, ‘species evenness’ and ‘Shannon diversity’ of the OTUs were analysed using linear effect models with the gls function from the nlme R package (Pinheiro et al., 2009). We assumed independent, identically distributed normal random errors. The models included the plant’s symbiotic status (E+, E-) and herbivory history (H+, H-) as categorical factors. VarIdent variance structures were used on the interaction between symbiotic status and herbivory history to accommodate deviations in variance homogeneity in the bacterial abundance response variable. Assumptions of each analysis of variance were met before to perform the analyses.
The effect of the plant symbiosis status and the herbivory history on the composition of bacterial assemblages of plant seed were analysed using permutational multivariate analysis of variance (PERMANOVA; 9,999 permutations), based on Bray-Curtis distances, with the function adonis from the vegan R package (Anderson, 2001; Oksanen et al., 2020). PERMANOVA allows testing for differences between groups when multivariate responses are measured. Similarly, the model included the plant’s symbiotic status (E+, E-) and herbivory history (H+, H-) as categorical factors. To characterise the composition differences between bacterial assemblages associated with seed, non-metric multidimensional scaling (NMDS) based on Bray-Curtis dissimilarities were performed using the vegan R package (Anderson, 2001; Oksanen et al., 2020). A Shepard diagram correlating NMDS ordination distances and Bray-Curtis community dissimilarities was used to validate NMDS ordination analysis. A high correlation indicates that the calculated NMDS ordination is a good representation of community structures (Leeuw and Mair, 2015). All the presented values in the result section are means ± standard errors (S.E.M). All the analyses were performed on R software (version 3.1.1; R Core Team, 2014).
Results
The plant shoot biomass was not affected by either the plant symbiosis status (F(1,8) = 2.78, p = 0.134) or the aphid herbivory (F(1,8) = 0.00, p = 0.978). E+ plants marginally produced more seed than E- plants (F(1,8) = 4.96, p = 0.05). The aphid herbivory did not affect the plant seed production (F(1,8) = 0.04, p = 0.835). All seed produced by E+ plants contained Epichloë endophytes, whereas seed from E- plants were free of these endophytes. The aphid herbivory treatment did not affect the Epichloë seed transmission (Table 1).
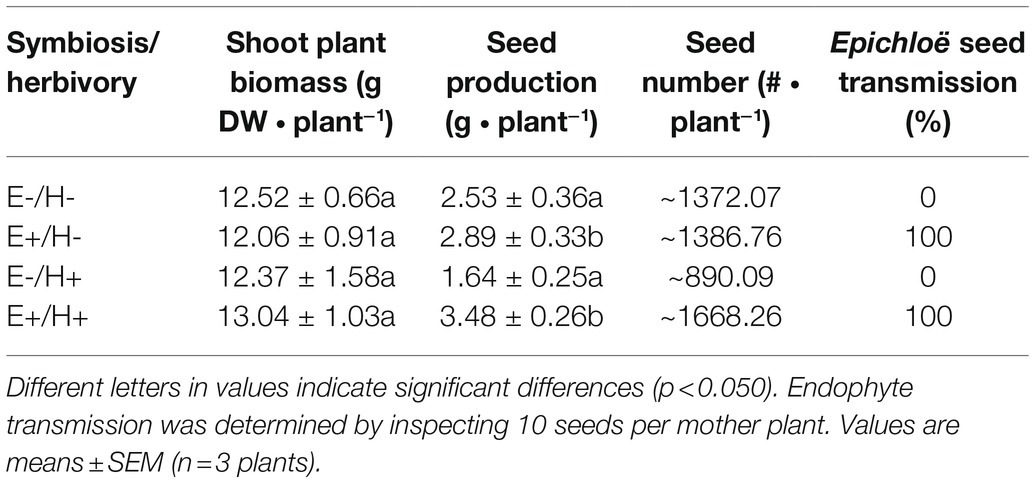
Table 1. Shoot biomass, seed production, seed number and endophyte seed transmission in Lolium multiflorum plants symbiotic (E+) and non-symbiotic (E-) with the fungal endophyte Epichloë occultans, that were challenged (H+) or not (H-) with Rhopalosiphum padi aphids.
We identified 99 bacterial OTUs in seed produced by L. multiflorum plants, with an average of 56.58 ± 2.11 OTUs per seed sample (i.e., from a mother plant). These bacterial OTUs were identified in phyla Proteobacteria (74% of OTUs), Actinobacteria (13% of OTUs), and Firmicutes (4% of OTUs; the remaining 9% of OTUs could not be classified at the phylum level; Supplementary Table S1). Considering the experimental conditions (i.e., endophyte presence within seed and plant herbivory history), bacterial assemblages were dominated by 6–13 OTUs (relative abundances > 1%; number of OTUs: E-/H- = 6, E-/H+ = 6, E+/H- = 11, E+/H+ = 13; Figure 1). Mostly these OTUs were identified in phylum Proteobacteria (i.e., Pantoea, Pseudomonas, Erwinia and Kosakonia) with only one belonging to Actinobacteria (i.e., Curtobacterium; Figure 1).
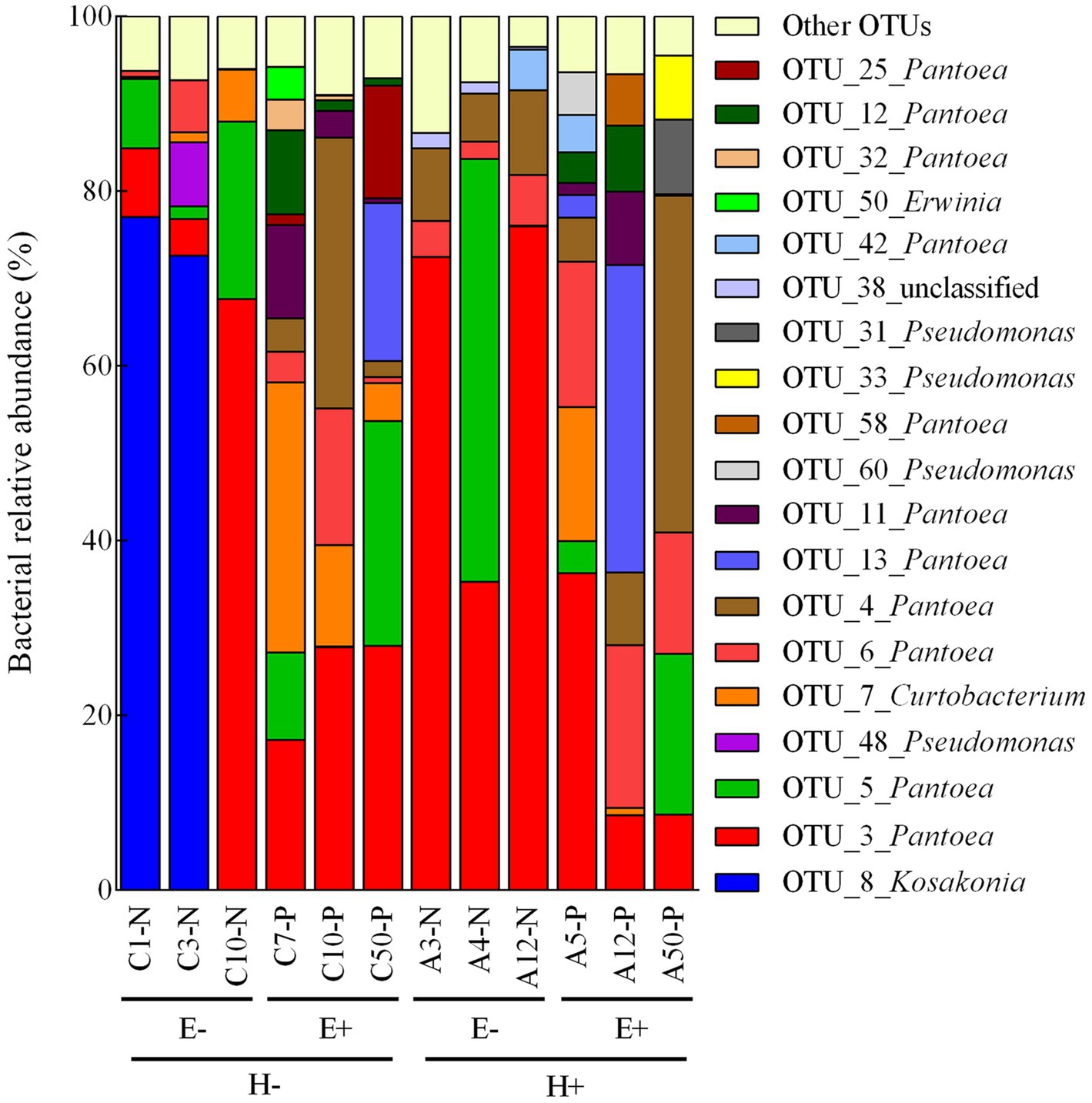
Figure 1. Relative abundance of dominant bacterial OTUs (operational taxonomic units) associated with individual seed samples of Lolium multiflorum plants symbiotic (E+) or not (E-) with the fungal endophyte Epichloë occultans, and challenged (H+) or not (H-) with the aphid herbivore Rhopalosiphum padi. Dominant bacterial OTUs were those with relative abundances above 1%, on average, in at least one group (i.e., E-/H-, E+/H-, E+/H- and E+/H+). Dominant bacterial OTUs represented 86-95% of the abundances of sequence reads associated with each seed sample. OTUs with relative abundances below 1% were compiled in the ‘other OTUs’ group.
The composition of the seed bacterial assemblages differed between endophyte-symbiotic and endophyte-free L. multiflorum plants, but it was independent on the plant herbivory history (Table 2). This was visualised with a NMDS ordination that showed that bacterial communities were mainly grouped by the symbiotic status of seeds rather than by the maternal herbivory history (Figure 2). The Shannon diversity index increased ca. 42% by the endophyte presence (E+ = 2.03 ± 0.06, E- = 1.17 ± 0.07; Table 2; Figure 3A). Whereas the bacterial richness associated with plant seed was not affected by the treatments, the evenness of bacterial assemblages increased ca. 42% by the endophyte presence (E+ = 0.50 ± 0.01, E- = 0.29 ± 0.01; Table 2; Figures 3B,C). In agreement with the endophyte-mediated effect on the evenness of bacterial communities, the relative abundances of the dominant taxonomic groups (relative abundances >1%) were more similarly distributed in endophyte-symbiotic seed compared with their non-symbiotic counterparts (Figure 4).
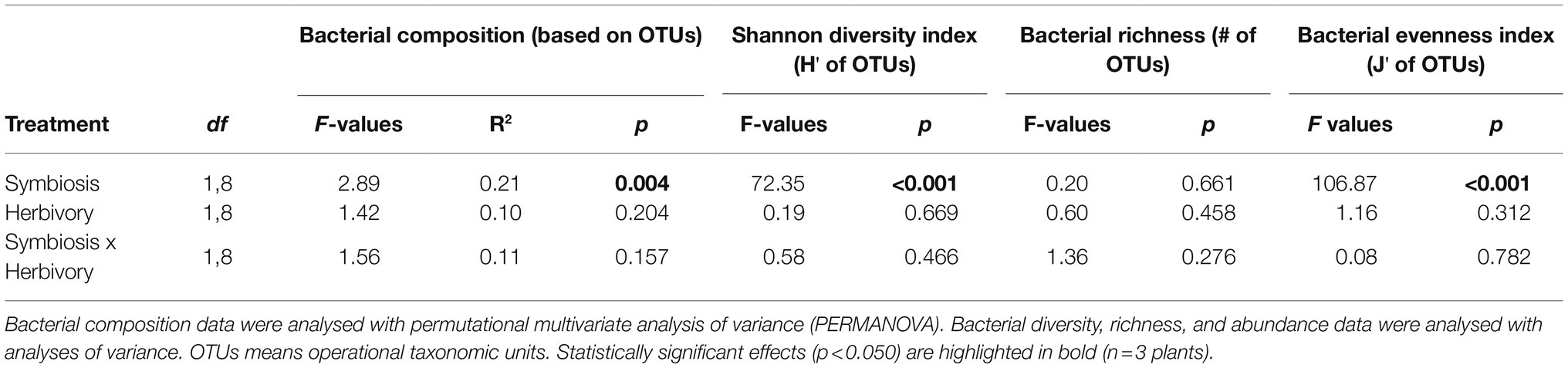
Table 2. Effect of the endophyte symbiotic status and maternal herbivory history on the bacterial composition, bacterial diversity (Shannon index, H'), bacterial richness and bacterial abundance (evenness index, J') of seed of Lolium multiflorum plants symbiotic or not with the fungal endophyte Epichloë occultans, and challenged or not with the aphid herbivore Rhopalosiphum padi.
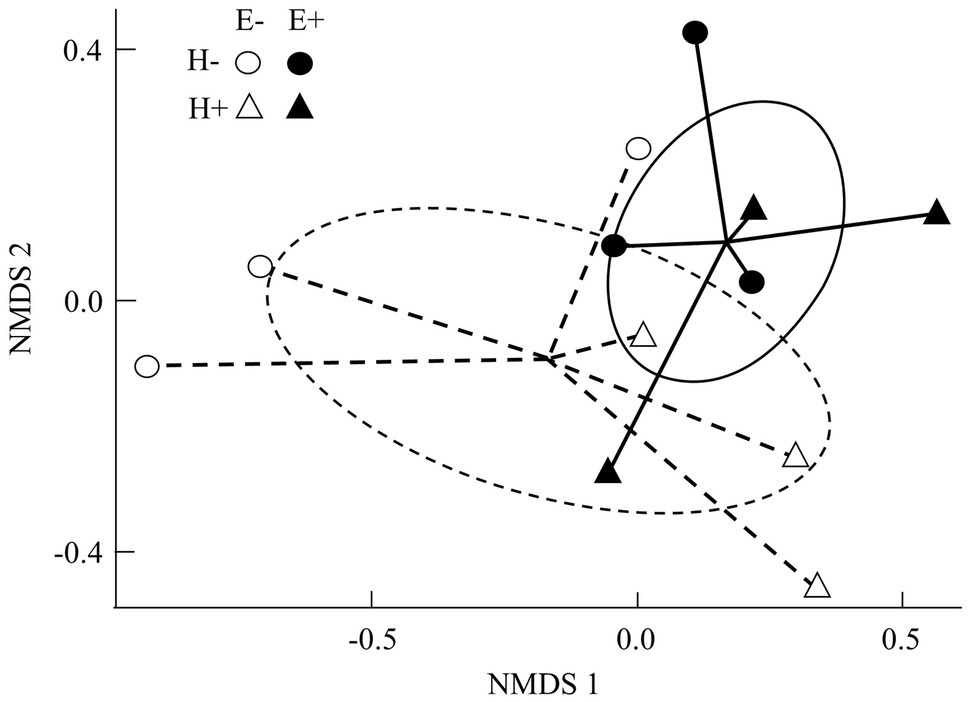
Figure 2. Non-metric multidimensional scaling ordinations (NMDS; stress = 0.09) for bacterial assemblages associated with seed of Lolium multiflorum plants symbiotic (E+, filled symbols) or not (E-, open symbols) with the fungal endophyte Epichloë occultans, and challenged (H+, triangles) or not (H-, circles) with the aphid herbivore Rhopalosiphum padi. Lines indicate compositional distances between centroids and E+ and E- seed-associated bacterial communities (continuous and discontinuous lines, respectively). Ellipses represent 95% confidence intervals around centroids and show clustering of bacterial compositions in seed based on the presence/absence of Epichloë endophytes (continuous and discontinuous lines, respectively). The Shepard diagram (Supplementary Figure S2) showed a good linear fit between ordination distances and Bray-Curtis dissimilarities (n = 3 plants).
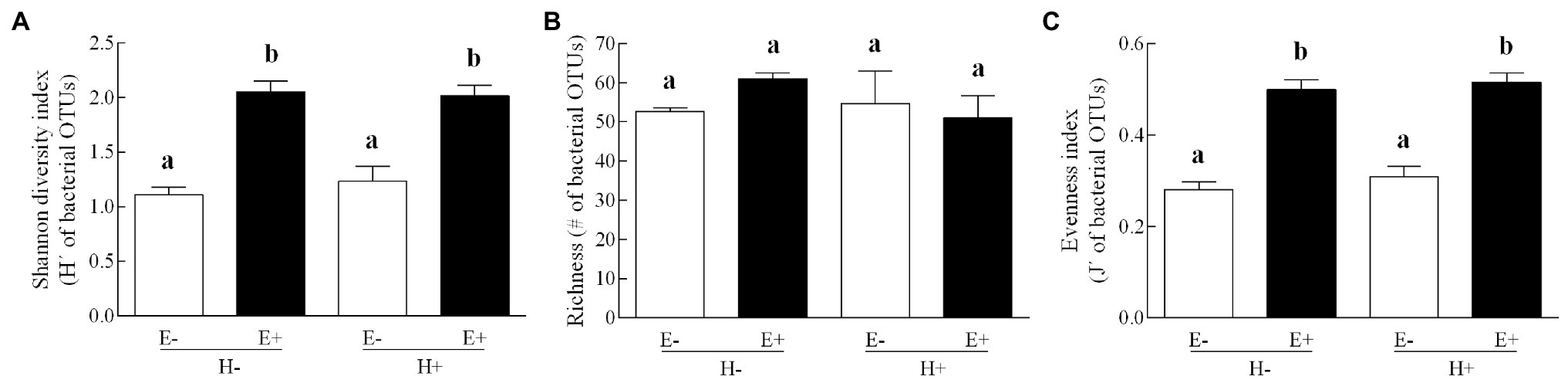
Figure 3. Bacterial diversity indexes (panel A: Shannon index; panel B: richness; panel C: evenness index) associated with seed of Lolium multiflorum plants symbiotic (E+, filled bars) or not (E-, open bars) with the fungal endophyte Epichloë occultans, and challenged (H+) or not (H-) with the aphid herbivore Rhopalosiphum padi. OTUs means operational taxonomic units. Different letters indicate significant differences at p < 0.050. Bars represent means values ± SEM (n = 3 plants).
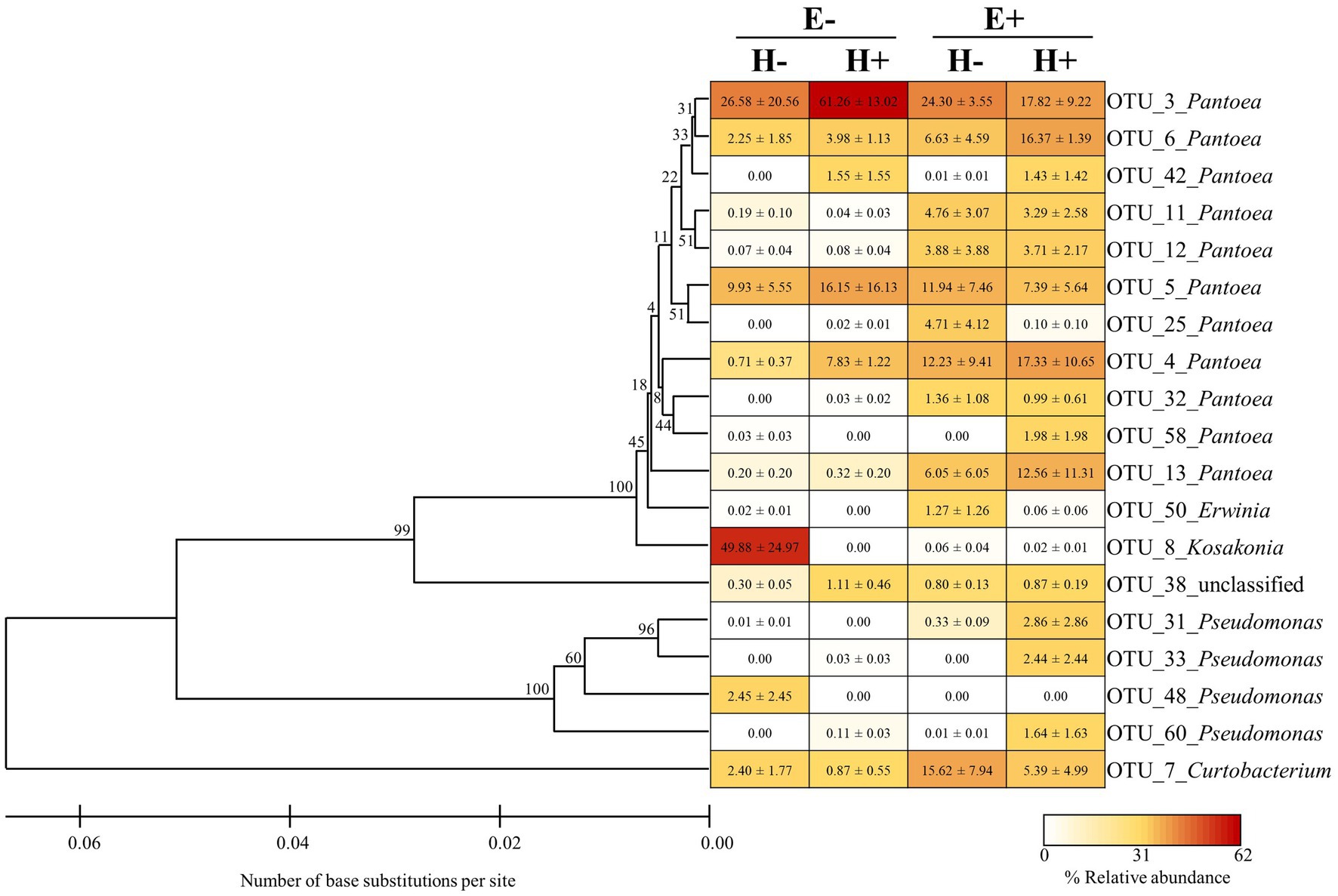
Figure 4. Phylogenetic tree and relative abundance of bacterial OTUs (operational taxonomic units) associated with seed of Lolium multiflorum plants symbiotic (E+) or not (E-) the fungal endophyte Epichloë occultans, and challenged (H+) or not (H-) with the aphid herbivore Rhopalosiphum padi. The tree was inferred using the Unweighted Pair Group Method with Arithmetic mean (UPGMA) algorithm with a bootstrap test (9,999 replicates). Bootstrap values for ingroup clades are shown next to branches. Evolutionary distances were calculated using the Maximum Composite Likelihood method. The heat map represents the relative abundances of dominant bacterial OTU associated with each seed group. Dominant bacterial OTUs (>1% relative abundance) represented 91-94% of the abundances of sequence reads associated with each seed group. Values in cells indicate means ± SEM (n = 3 plants).
Discussion
We hypothesised that vertically transmitted Epichloë endophytes influence the composition and diversity of the seed-associated bacterial microbiota of their host plants. Furthermore, we posited that the herbivory experienced by mother plants also affect the composition and diversity of seed bacterial communities, but this effect of herbivores would interact with the presence of Epichloë endophytes in mother plants. Our results showed that Epichloë indeed influenced the composition of the bacterial communities in host seeds. The seed-associated bacterial communities were more diverse in endophyte-symbiotic than endophyte-free seeds. Instead of an increase in species richness, this higher diversity was attributed to the relative abundances among bacterial taxa being more similar in communities associated with E+ than E- seed (bacterial communities in E- seed were dominated by few taxa). Contrary to our expectation, seed bacterial communities were not affected by the herbivory experienced by mother plants.
The seed-associated bacterial communities are generally simpler in terms of composition and diversity than communities from soils, seedlings and adult plant tissues (Chesneau et al., 2020; Rochefort et al., 2021; Walsh et al., 2021). Bacteria within phylum Proteobacteria, including members from Pantoea spp. and Pseudomonas spp., normally dominate seed bacterial communities (Truyens et al., 2015; Kim and Lee, 2021). In agreement with this, bacterial communities in L. multiflorum seeds were dominated by members from the Proteobacteria, including the above-mentioned genera and a few other groups (e.g., Erwinia spp.). This pattern of bacterial diversity in L. multiflorum seeds is similar to those documented in seeds from other grass species such as Lolium perenne (Tannenbaum et al., 2020, 2021), Elymus nutans (Guo et al., 2021), Oryza sativa (Eyre et al., 2019; Wang et al., 2020a) and Triticum aestivum (Hone et al., 2021; Walsh et al., 2021). Several beneficial bacteria within the Pantoea and Pseudomonas genera have been isolated from plant seeds (Feng et al., 2006; Díaz Herrera et al., 2016; Verma et al., 2018). Experimental evidence has shown that beneficial seed-inhabiting Pantoea and Pseudomonas can efficiently colonise seedlings and that the colonisation of these bacteria can provide advantages to seedlings during establishment (Ferreira et al., 2008; Pavlova et al., 2017; Verma et al., 2017; Morella et al., 2019; Soluch et al., 2021).
Epichloë fungal endophytes can enhance the performance of host seed and seedlings (Clay, 1987; Novas et al., 2003; Stefanoni-Rubio et al., 2021). This Epichloë-mediated enhancement in host performance can be even more significant when symbiotic seed and seedlings experience stressful conditions (e.g., pathogens, salinity, drought, soil contamination and nutrient limitation; Gwinn and Gavin, 1992; Gundel et al., 2006; Zhang et al., 2010; Vázquez de Aldana et al., 2014; Ding et al., 2015; Pérez et al., 2016; Wang et al., 2020b). The increased performance of symbiotic seed/seedlings has been normally attributed to the biochemical changes exerted by Epichloë on host plants (e.g., alkaloids, antioxidants and sugars; Gundel et al., 2018; Zhang et al., 2019; Hewitt et al., 2020; Ueno et al., 2020). However, enhanced performance could also be explained, at least in part, by the beneficial activities derived from other microbial symbionts co-inhabiting Epichloë-symbiotic seed and seedlings. In the present study, Epichloë endophyte presence was associated with increased diversity and changes on composition of seed-associated bacterial communities, particularly in members from Pantoea and Pseudomonas. Interestingly, both genera contain species/strains described to provide a suite of benefits to plants such as growth promotion, nitrogen fixation, phosphate solubilisation and protection against pathogens/plant competitors (Elmore et al., 2019; Guo et al., 2021). For instance, several plant-growth promoting Pseudomonas isolates increased the seed germination and seedling growth of Solanum lycopersicum plants (through production of auxins, phosphate-solubilising compounds and another growth-promoting compounds; Qessaoui et al., 2019). The stress-protective Pantoea alhagi strain NX-11 bacterium increased the salt stress resistance of Oryza sativa seedlings (via exopolysaccharide production, compounds that inhibit the plant absorption of salt; Sun et al., 2020). The antimicrobial-producing Pseudomonas sp. strain SY1 bacterium protected seedlings of Oryza sativa, Cynodon dactylon and Poa annua from the fungal pathogen Fusarium oxysporum (Verma et al., 2018). Therefore, it is likely that the performance of symbiotic seed/seedlings can be also increased by the action of those beneficial bacteria (e.g., Pantoea spp. and Pseudomonas spp.) promoted by the association of host plants with Epichloë endophytes.
We did not detect an effect of the mother plant herbivory (or via interaction with the endophyte presence) on the seed-associated bacterial communities. In the present experiment, plants were interacting with aphids for 21 days (plants were at tillering stage), and the rest of the growing cycle were maintained free of herbivores (i.e., ca. 45 days). It is possible that any impact from the aphid treatment was transitory such that changes in the plant associated microbiota did not persist until the plant flowering stage when microbes are transmitted into seeds. This seems likely since herbivory triggers changes in plant defence and plant nutrient release, both of which likely impact the plant microbiota indirectly, in addition to microbes being added directly through the herbivore itself (Smets and Koskella, 2020). It is also possible that we observed minimal herbivory-associated changes on the plant’s bacterial communities because these are driven more by the colonisation of microbes from external sources such as the soil, air and biotic vectors (Tombolini et al., 1999). In fact, experimental evidence has shown that plant microbial communities can recover their pre-perturbation structures by colonisation of microbes from environmental sources (Haas et al., 2018; Stone and Jackson, 2021).
In conclusion, this study highlights the influence of Epichloë endophytes in the diversity of bacterial communities associated with seed. Our results indicate that these endophytes affect the seed bacterial microbiota by modifying the composition and increasing the diversity of these bacterial communities. Epichloë fungal endophytes may increase diversity by reducing the overall bacterial load and by preventing establishment of dominance of certain groups. It is likely that this Epichloë-mediated increment in bacterial diversity contributes to the documented enhanced performance of endophyte-associated seed/seedlings (e.g., Clay, 1987; Gwinn and Gavin, 1992; Pérez et al., 2016). Furthermore, due to the lack of effect of our herbivory treatment, we speculate that stresses occurring close to the plant flowering stage, when microbes are transmitted, may have larger impacts on seed-associated microbial communities than stresses acting at earlier stages (Shade et al., 2017). In fact, it has been documented that certain seed attributes in L. multiflorum plants can be affected by stresses occurring at, or close to, the reproductive stage (e.g., seed production and longevity; Ueno et al., 2020, 2021). Considering the relative abundances of bacterial taxa across treatments, Kosakonia was remarkable because this taxon exhibited high abundance and was virtually only present within E-/H- seed. Perhaps Kosakonia spp. were particularly susceptible to potential chemical changes exhibited by seed due to the experimental treatments. In agreement with this hypothesis, Hou et al. (2020) found that the abundance of Kosakonia spp. effectively depended on the plant chemical composition (in this study, Kosakonia spp. were also associated with only E- plants). Further experiments that isolate bacteria from Epichloë-associated seed will be essential to characterise the microbiota and also to identify those beneficial bacteria for plants. Sequencing of whole bacterial genomes, transcriptomes and/or inoculation of bacterial isolates into plants can be useful tools for characterising the isolated bacteria (Bastías et al., 2020; Ju et al., 2021; Li et al., 2021).
Data Availability Statement
The data presented in the study are deposited in the NCBI Short Read Archive (SRA), accession number PRJNA782330.
Author Contributions
DB, LB, and PG conceived the experiment. LB and AB performed the experiments. DB, RJ, IA-R, and PG analysed data. IA-R, RJ, MM-M, and PG wrote the manuscript. All authors contributed to the article and approved the submitted version.
Funding
The research was funded by agencies FONCyT-Argentina (project PICT-2018-01593) and FONDECYT-Chile (project 1210908).
Conflict of Interest
The authors declare that the research was conducted in the absence of any commercial or financial relationships that could be construed as a potential conflict of interest.
Publisher’s Note
All claims expressed in this article are solely those of the authors and do not necessarily represent those of their affiliated organizations, or those of the publisher, the editors and the reviewers. Any product that may be evaluated in this article, or claim that may be made by its manufacturer, is not guaranteed or endorsed by the publisher.
Acknowledgments
We would like to thank Richard D. Johnson and Christina D. Moon for critical revision of the manuscript and Gabriel I. Ballesteros for technical advice. We also thank the reviewers for their valuable comments on the manuscript.
Supplementary Material
The Supplementary Material for this article can be found online at: https://www.frontiersin.org/articles/10.3389/fmicb.2021.795354/full#supplementary-material
Supplementary Figure 1 | Rarefaction curves of bacterial OTUs (operational taxonomic units) associated with each seed sample used in the present study.
Supplementary Figure 2 | Shepard diagram of the non-metric multidimensional scaling (NMDS) ordination related to Figure 2.
Supplementary Table 1 | Matrix of bacterial OTUs (operational taxonomic units) vs. read counts associated with seed samples.
References
Anderson, M. J. (2001). A new method for non-parametric multivariate analysis of variance. Austral Ecol. 26, 32–46. doi: 10.1111/j.1442-9993.2001.01070.pp.x
Bastias, D. A., Martínez-Ghersa, M. A., Ballaré, C. L., and Gundel, P. E. (2017). Epichloë fungal endophytes and plant defenses: not just alkaloids. Trends Plant Sci. 22, 939–248. doi: 10.1016/j.tplants.2017.08.005
Bastías, D. A., Martínez-Ghersa, M. A., Newman, J. A., Card, S. D., Mace, W. J., and Gundel, P. E. (2018). The plant hormone salicylic acid interacts with the mechanism of anti-herbivory conferred by fungal endophytes in grasses. Plant Cell Environ. 41, 395–405. doi: 10.1111/pce.13102
Bastías, D. A., Ruy, J., Applegate, E. R., Altermann, E., Card, S. D., and Johnson, L. J. (2020). Complete genome sequence of Paenibacillus sp. strain E222, a bacterial symbiont of an Epichloë fungal endophyte of ryegrass. Microbiol. Resour. Announc. 9, e00786–e00720. doi: 10.1128/MRA.00786-20
Bastías, D. A., Ueno, A. C., Machado Assefh, C. R., Alvarez, A. E., Young, C. A., and Gundel, P. E. (2017). Metabolism or behavior: explaining the performance of aphids on alkaloid-producing fungal endophytes in annual ryegrass (Lolium multiflorum). Oecologia 185, 245–256. doi: 10.1007/s00442-017-3940-2
Bolger, A. M., Lohse, M., and Usadel, B. (2014). Trimmomatic: a flexible trimmer for Illumina sequence data. Bioinformatics 30, 2114–2120. doi: 10.1093/bioinformatics/btu170
Camarinha-Silva, A., Jáuregui, R., Pieper, D. H., and Wos-Oxley, M. L. (2012). The temporal dynamics of bacterial communities across human anterior nares. Environ. Microbiol. Rep. 4, 126–132. doi: 10.1111/j.1758-2229.2011.00313.x
Caporaso, J. G., Kuczynski, J., Stombaugh, J., Bittinger, K., Bushman, F. D., Costello, E. K., et al. (2010). QIIME allows analysis of high-throughput community sequencing data. Nat. Methods 7, 335–336. doi: 10.1038/nmeth.f.303
Card, S. D., Rolston, M. P., Park, Z., Cox, N., and Hume, D. E. (2011). Fungal endophyte detection in pasture grass seed utilising the infection layer and comparison to other detection techniques. Seed Sci. Technol. 39, 581–592. doi: 10.15258/sst.2011.39.3.05
Chesneau, G., Torres-Cortes, G., Briand, M., Darrasse, A., Preveaux, A., Marais, C., et al. (2020). Temporal dynamics of bacterial communities during seed development and maturation. FEMS Microbiol. Ecol. 96:fiaa190. doi: 10.1093/femsec/fiaa190
Clay, K. (1987). Effects of fungal endophytes on the seed and seedling biology of Lolium perenne and Festuca arundinacea. Oecologia 73, 358–362. doi: 10.1007/BF00385251
Díaz Herrera, S., Grossi, C., Zawoznik, M., and Groppa, M. D. (2016). Wheat seeds harbour bacterial endophytes with potential as plant growth promoters and biocontrol agents of Fusarium graminearum. Microbiol. Res. 186–187, 37–43. doi: 10.1016/j.micres.2016.03.002
Ding, N., Kupper, J. V., and McNear, D. H. Jr. (2015). Phosphate source interacts with endophyte strain to influence biomass and root system architecture in tall fescue. Agron. J. 107, 662–670. doi: 10.2134/agronj14.0135
Dixon, A. F. G. (1971). The life-cycle and host preferences of the bird cherry-oat aphid, Rhopalosiphum padi L., and their bearing on the theories of host alternation in aphids. Ann. Appl. Biol. 68, 135–147. doi: 10.1111/j.1744-7348.1971.tb06450.x
Elmore, M. T., White, J. F., Kingsley, K. L., Diehl, K. H., and Verma, S. K. (2019). Pantoea spp. associated with smooth crabgrass (Digitaria ischaemum) seed inhibit competitor plant species. Microorganisms 7:143. doi: 10.3390/microorganisms7050143
Eyre, A. W., Wang, M., Oh, Y., and Dean, R. A. (2019). Identification and characterization of the core rice seed microbiome. Phytobiomes J. 3, 148–157. doi: 10.1094/PBIOMES-01-19-0009-R
Feng, Y., Shen, D., and Song, W. (2006). Rice endophyte Pantoea agglomerans YS19 promotes host plant growth and affects allocations of host photosynthates. J. Appl. Microbiol. 100, 938–945. doi: 10.1111/j.1365-2672.2006.02843.x
Ferreira, A., Quecine, M. C., Lacava, P. T., Oda, S., Azevedo, J. L., and Araújo, W. L. (2008). Diversity of endophytic bacteria from eucalyptus species seeds and colonization of seedlings by Pantoea agglomerans. FEMS Microbiol. Lett. 287, 8–14. doi: 10.1111/j.1574-6968.2008.01258.x
Fort, T., Pauvert, C., Zanne, A. E., Ovaskainen, O., Caignard, T., Barret, M., et al. (2021). Maternal effects shape the seed mycobiome in Quercus petraea. New Phytol. 230, 1594–1608. doi: 10.1111/nph.17153
Gundel, P. E., Garibaldi, L. A., Martínez-Ghersa, M. A., and Ghersa, C. M. (2011a). Neotyphodium endophyte transmission to Lolium multiflorum seeds depends on the host plant fitness. Environ. Exp. Bot. 71, 359–366. doi: 10.1016/j.envexpbot.2011.02.002
Gundel, P. E., Martínez-Ghersa, M. A., Omacini, M., Cuyeu, R., Pagano, E., Ríos, R., et al. (2012). Mutualism effectiveness and vertical transmission of symbiotic fungal endophytes in response to host genetic background. Evol. Appl. 5, 838–849. doi: 10.1111/j.1752-4571.2012.00261.x
Gundel, P. E., Maseda, P. H., Vila-Aiub, M. M., Ghersa, C. M., and Benech-Arnold, R. (2006). Effects of Neotyphodium fungi on Lolium multiflorum seed germination in relation to water availability. Ann. Bot. 97, 571–577. doi: 10.1093/aob/mcl004
Gundel, P. E., Rudgers, J. A., and Ghersa, C. M. (2011b). Incorporating the process of vertical transmission into understanding of host–symbiont dynamics. Oikos 120, 1121–1128. doi: 10.1111/j.1600-0706.2011.19299.x
Gundel, P. E., Seal, C. E., Biganzoli, F., Molina-Montenegro, M. A., Vázquez-de-Aldana, B. R., Zabalgogeazcoa, I., et al. (2018). Occurrence of alkaloids in grass seeds symbiotic with vertically-transmitted Epichloë fungal endophytes and its relationship with antioxidants. Front. Ecol. Evol. 6:211. doi: 10.3389/fevo.2018.00211
Guo, J., Bowatte, S., and Hou, F. (2021). Diversity of endophytic bacteria and fungi in seeds of Elymus nutans growing in four locations of Qinghai Tibet plateau, China. Plant Soil 459, 49–63. doi: 10.1007/s11104-020-04608-y
Gwinn, K. D., and Gavin, A. M. (1992). Relationship between endophyte infestation level of tall fescue seed lots and Rhizoctonia zeae seedling disease. Plant Dis. 76, 911–914. doi: 10.1094/PD-76-0911
Haas, J. C., Street, N. R., Sjödin, A., Lee, N. M., Högberg, M. N., Näsholm, T., et al. (2018). Microbial community response to growing season and plant nutrient optimisation in a boreal Norway spruce forest. Soil Biol. Biochem. 125, 197–209. doi: 10.1016/j.soilbio.2018.07.005
Hall, B. G. (2013). Building phylogenetic trees from molecular data with MEGA. Mol. Biol. Evol. 30, 1229–1235. doi: 10.1093/molbev/mst012
Herlemann, D. P., Labrenz, M., Jürgens, K., Bertilsson, S., Waniek, J. J., and Andersson, A. F. (2011). Transitions in bacterial communities along the 2000 km salinity gradient of the Baltic Sea. ISME J. 5, 1571–1579. doi: 10.1038/ismej.2011.41
Hettiarachchige, I. K., Vander Jagt, C. J., Mann, R. C., Sawbridge, T. I., Spangenberg, G. C., and Guthridge, K. M. (2021). Global changes in asexual Epichloë transcriptomes during the early stages, from seed to seedling, of symbiotum establishment. Microorganisms 9:991. doi: 10.3390/microorganisms9050991
Hewitt, K. G., Mace, W. J., McKenzie, C. M., Matthew, C., and Popay, A. J. (2020). Fungal alkaloid occurrence in endophyte-infected perennial ryegrass during seedling establishment. J. Chem. Ecol. 46, 410–421. doi: 10.1007/s10886-020-01162-w
Hone, H., Mann, R., Yang, G., Kaur, J., Tannenbaum, I., Li, T., et al. (2021). Profiling, isolation and characterisation of beneficial microbes from the seed microbiomes of drought tolerant wheat. Sci. Rep. 11:11916. doi: 10.1038/s41598-021-91351-8
Hou, W., Xia, C., Christensen, M. J., Wang, J., Li, X., Chen, T., et al. (2020). Effect of Epichloë gansuensis endophyte on rhizosphere bacterial communities and nutrient concentrations and ratios in the perennial grass species Achnatherum inebrians during three growth seasons. Crop Pasture Sci. 71, 1050–1066. doi: 10.1071/CP20145
Humphrey, P. T., and Whiteman, N. K. (2020). Insect herbivory reshapes a native leaf microbiome. Nat. Ecol. Evol. 4, 221–229. doi: 10.1038/s41559-019-1085-x
Ju, Y., Kou, M., Zhong, R., Christensen, M. J., and Zhang, X. (2021). Alleviating salt stress on seedings using plant growth promoting rhizobacteria isolated from the rhizosphere soil of Achnatherum inebrians infected with Epichloë gansuensis endophyte. Plant Soil 465, 349–366. doi: 10.1007/s11104-021-05002-y
Kearse, M., Moir, R., Wilson, A., Stones-Havas, S., Cheung, M., Sturrock, S., et al. (2012). Geneious basic: an integrated and extendable desktop software platform for the organization and analysis of sequence data. Bioinformatics 28, 1647–1649. doi: 10.1093/bioinformatics/bts199
Kim, H., and Lee, Y.-H. (2021). Spatiotemporal assembly of bacterial and fungal communities of seed-seedling-adult in rice. Front. Microbiol. 12:708475. doi: 10.3389/fmicb.2021.708475
Kou, M.-Z., Bastías, D. A., Christensen, M. J., Zhong, R., Nan, Z.-B., and Zhang, X.-X. (2021). The plant salicylic acid signalling pathway regulates the infection of a biotrophic pathogen in grasses associated with an Epichloë endophyte. J. Fungi 7:633. doi: 10.3390/jof7080633
Kumar, S., Stecher, G., Li, M., Knyaz, C., and Tamura, K. (2018). MEGA X: molecular evolutionary genetics analysis across computing platforms. Mol. Biol. Evol. 35, 1547–1549. doi: 10.1093/molbev/msy096
Leeuw, J. D., and Mair, P. (2015). “Shepard diagram,” in Wiley StatsRef: Statistics Reference Online, eds. N. Balakrishnan, T. Colton, B. Everitt, W. Piegorsch, and F. Ruggeri, and J. L. Teugels (American Cancer Society), 1–3.
Li, T., Mann, R., Kaur, J., Spangenberg, G., and Sawbridge, T. (2021). Transcriptomics differentiate two novel bioactive strains of Paenibacillus sp. isolated from the perennial ryegrass seed microbiome. Sci. Rep. 11:15545. doi: 10.1038/s41598-021-94820-2
Liu, J., Nagabhyru, P., and Schardl, C. L. (2017). Epichloë festucae endophytic growth in florets, seeds, and seedlings of perennial ryegrass (Lolium perenne). Mycologia 109, 691–700. doi: 10.1080/00275514.2017.1400305
Ma, M., Christensen, M. J., and Nan, Z. (2015). Effects of the endophyte Epichloë festucae var. lolii of perennial ryegrass (Lolium perenne) on indicators of oxidative stress from pathogenic fungi during seed germination and seedling growth. Eur. J. Plant Pathol. 141, 571–583. doi: 10.1007/s10658-014-0563-x
Magoč, T., and Salzberg, S. L. (2011). FLASH: fast length adjustment of short reads to improve genome assemblies. Bioinformatics 27, 2957–2963. doi: 10.1093/bioinformatics/btr507
Mahé, F., Rognes, T., Quince, C., de Vargas, C., and Dunthorn, M. (2014). Swarm: robust and fast clustering method for amplicon-based studies. PeerJ 2:e593. doi: 10.7717/peerj.593
Moon, C. D., Scott, B., Schardl, C. L., and Christensen, M. J. (2000). The evolutionary origins of Epichloë endophytes from annual ryegrasses. Mycologia 92, 1103–1118. doi: 10.2307/3761478
Morella, N. M., Zhang, X., and Koskella, B. (2019). Tomato seed-associated bacteria confer protection of seedlings against foliar disease caused by pseudomonas syringae. Phytobiomes J. 3, 177–190. doi: 10.1094/PBIOMES-01-19-0007-R
Nelson, E. B. (2018). The seed microbiome: origins, interactions, and impacts. Plant Soil 422, 7–34. doi: 10.1007/s11104-017-3289-7
Nissinen, R., Helander, M., Kumar, M., and Saikkonen, K. (2019). Heritable Epichloë symbiosis shapes fungal but not bacterial communities of plant leaves. Sci. Rep. 9:5253. doi: 10.1038/s41598-019-41603-5
Novas, M. V., Gentile, A., and Cabral, D. (2003). Comparative study of growth parameters on diaspores and seedlings between populations of Bromus setifolius from Patagonia, differing in Neotyphodium endophyte infection. Flora 198, 421–426. doi: 10.1078/0367-2530-00115
Oksanen, J., Blanchet, F. G., Friendly, M., Kindt, R., Legendre, P., McGlinn, D., et al. (2020). vegan: community ecology package. R package, Version 2.5-7.
Omacini, M., Chaneton, E. J., Ghersa, C. M., and Muller, C. B. (2001). Symbiotic fungal endophytes control insect host-parasite interaction webs. Nature 409, 78–81. doi: 10.1038/35051070
Pavlova, A. S., Leontieva, M. R., Smirnova, T. A., Kolomeitseva, G. L., Netrusov, A. I., and Tsavkelova, E. A. (2017). Colonization strategy of the endophytic plant growth-promoting strains of Pseudomonas fluorescens and Klebsiella oxytoca on the seeds, seedlings and roots of the epiphytic orchid, Dendrobium nobile Lindl. J. Appl. Microbiol. 123, 217–232. doi: 10.1111/jam.13481
Pérez, L. I., Gundel, P. E., Ghersa, C. M., and Omacini, M. (2013). Family issues: fungal endophyte protects host grass from the closely related pathogen Claviceps purpurea. Fungal Ecol. 6, 379–386. doi: 10.1016/j.funeco.2013.06.006
Pérez, L. I., Gundel, P. E., and Omacini, M. (2016). Can the defensive mutualism between grasses and fungal endophytes protect non-symbiotic neighbours from soil pathogens? Plant Soil 405, 289–298. doi: 10.1007/s11104-015-2568-4
Pinheiro, J., Bates, D., DebRoy, S., Sarkar, D., and Core Team, R. (2009). nlme: linear and nonlinear mixed effects models. R package. Vienna, Austria: R Foundation for Statistical Computing.
Qessaoui, R., Bouharroud, R., Furze, J. N., El Aalaoui, M., Akroud, H., Amarraque, A., et al. (2019). Applications of new rhizobacteria pseudomonas isolates in agroecology via fundamental processes complementing plant growth. Sci. Rep. 9:12832. doi: 10.1038/s41598-019-49216-8
Quast, C., Pruesse, E., Yilmaz, P., Gerken, J., Schweer, T., Yarza, P., et al. (2013). The SILVA ribosomal RNA gene database project: improved data processing and web-based tools. Nucleic Acids Res. 41, D590–D596. doi: 10.1093/nar/gks1219
R Core Team (2014). R: a language and environment for statistical computing. Vienna, Austria.: R Foundation for Statistical Computing Available at: http://www.R-project.org/ (Accessed: September, 2021).
Roberts, E., and Lindow, S. (2014). Loline alkaloid production by fungal endophytes of fescue species select for particular epiphytic bacterial microflora. ISME J. 8, 359–368. doi: 10.1038/ismej.2013.170
Rochefort, A., Simonin, M., Marais, C., Guillerm-Erckelboudt, A.-Y., Barret, M., and Sarniguet, A. (2021). Transmission of seed and soil microbiota to seedling. mSystems 6, e00446–e00421. doi: 10.1128/mSystems.00446-21
Schardl, C. L., Leuchtmann, A., and Spiering, M. J. (2004). Symbioses of grasses with seedborne fungal endophytes. Annu. Rev. Plant Biol. 55, 315–340. doi: 10.1146/annurev.arplant.55.031903.141735
Schloss, P. D., Westcott, S. L., Ryabin, T., Hall, J. R., Hartmann, M., Hollister, E. B., et al. (2009). Introducing mothur: open-source, platform-independent, community-supported software for describing and comparing microbial communities. Appl. Environ. Microbiol. 75, 7537–7541. doi: 10.1128/AEM.01541-09
Seabloom, E. W., Condon, B., Kinkel, L., Komatsu, K. J., Lumibao, C. Y., May, G., et al. (2019). Effects of nutrient supply, herbivory, and host community on fungal endophyte diversity. Ecology 100:e02758. doi: 10.1002/ecy.2758
Shade, A., Jacques, M.-A., and Barret, M. (2017). Ecological patterns of seed microbiome diversity, transmission, and assembly. Curr. Opin. Microbiol. 37, 15–22. doi: 10.1016/j.mib.2017.03.010
Shi, X., Qin, T., Liu, H., Wu, M., Li, J., Shi, Y., et al. (2020). Endophytic fungi activated similar defense strategies of Achnatherum sibiricum host to different trophic types of pathogens. Front. Microbiol. 11:1607. doi: 10.3389/fmicb.2020.01607
Smets, W., and Koskella, B. (2020). Microbiome: insect herbivory drives plant phyllosphere dysbiosis. Curr. Biol. 30, R412–R414. doi: 10.1016/j.cub.2020.03.039
Soluch, R., Hülter, N. F., Romero Picazo, D., Özkurt, E., Stukenbrock, E. H., and Dagan, T. (2021). Colonization dynamics of Pantoea agglomerans in the wheat root habitat. Environ. Microbiol. 23, 2260–2273. doi: 10.1111/1462-2920.15430
Stefanoni-Rubio, P. J., Gundel, P. E., Novas, M. V., and Iannone, L. J. (2021). Ecotype-specific effects of fungal endophytes on germination responses of seeds of the south American wild forage grass Bromus auleticus. Ann. Appl. Biol. 1–12. doi: 10.1111/aab.12732
Stone, B. W. G., and Jackson, C. R. (2021). Seasonal patterns contribute more towards phyllosphere bacterial community structure than short-term perturbations. Microb. Ecol. 81, 146–156. doi: 10.1007/s00248-020-01564-z
Sun, L., Lei, P., Wang, Q., Ma, J., Zhan, Y., Jiang, K., et al. (2020). The endophyte Pantoea alhagi NX-11 alleviates salt stress damage to rice seedlings by secreting exopolysaccharides. Front. Microbiol. 10:3112. doi: 10.3389/fmicb.2019.03112
Tannenbaum, I., Kaur, J., Mann, R., Sawbridge, T., Rodoni, B., and Spangenberg, G. (2020). Profiling the Lolium perenne microbiome: from seed to seed. Phytobiomes J. 4, 281–289. doi: 10.1094/PBIOMES-03-20-0026-R
Tannenbaum, I., Rodoni, B., Spangenberg, G., Mann, R., and Sawbridge, T. (2021). An assessment of the Lolium perenne (perennial ryegrass) seedborne microbiome across cultivars, time, and biogeography: implications for microbiome breeding. Microorganisms 9:1205. doi: 10.3390/microorganisms9061205
Tombolini, R., van der Gaag, D. J., Gerhardson, B., and Jansson, J. K. (1999). Colonization pattern of the biocontrol strain pseudomonas chlororaphis MA 342 on barley seeds visualized by using green fluorescent protein. Appl. Environ. Microbiol. 65, 3674–3680. doi: 10.1128/AEM.65.8.3674-3680.1999
Truyens, S., Weyens, N., Cuypers, A., and Vangronsveld, J. (2015). Bacterial seed endophytes: genera, vertical transmission and interaction with plants. Environ. Microbiol. Rep. 7, 40–50. doi: 10.1111/1758-2229.12181
Ueno, A. C., Gundel, P. E., Ghersa, C. M., Agathokleous, E., and Martínez-Ghersa, M. A. (2021). Seed-borne fungal endophytes constrain reproductive success of host plants under ozone pollution. Environ. Res. 202:111773. doi: 10.1016/j.envres.2021.111773
Ueno, A. C., Gundel, P. E., Ghersa, C. M., Demkura, P. V., Card, S. D., Mace, W. J., et al. (2020). Ontogenetic and trans-generational dynamics of a vertically transmitted fungal symbiont in an annual host plant in ozone-polluted settings. Plant Cell Environ. 43, 2540–2550. doi: 10.1111/pce.13859
Vázquez de Aldana, B. R., Gundel, P. E., García Criado, B., García Ciudad, A., García Sánchez, A., and Zabalgogeazcoa, I. (2014). Germination response of endophytic Festuca rubra seeds in the presence of arsenic. Grass Forage Sci. 69, 462–469. doi: 10.1111/gfs.12049
Verma, S. K., Kingsley, K. L., Bergen, M. S., Kowalski, K. P., and White, J. F. (2018). Fungal disease prevention in seedlings of rice (Oryza sativa) and other grasses by growth-promoting seed-associated endophytic bacteria from invasive Phragmites australis. Microorganisms 6:21. doi: 10.3390/microorganisms6010021
Verma, S. K., Kingsley, K., Irizarry, I., Bergen, M., Kharwar, R. N., and White, J. F. Jr. (2017). Seed-vectored endophytic bacteria modulate development of rice seedlings. J. Appl. Microbiol. 122, 1680–1691. doi: 10.1111/jam.13463
Walsh, C. M., Becker-Uncapher, I., Carlson, M., and Fierer, N. (2021). Variable influences of soil and seed-associated bacterial communities on the assembly of seedling microbiomes. ISME J. 15, 2748–2762. doi: 10.1038/s41396-021-00967-1
Wang, M., Eyre, A. W., Thon, M. R., Oh, Y., and Dean, R. A. (2020a). Dynamic changes in the microbiome of rice during shoot and root growth derived from seeds. Front. Microbiol. 11:559728. doi: 10.3389/fmicb.2020.559728
Wang, Q., Garrity, G. M., Tiedje, J. M., and Cole, J. R. (2007). Naive bayesian classifier for rapid assignment of rRNA sequences into the new bacterial taxonomy. Appl. Environ. Microbiol. 73, 5261–5267. doi: 10.1128/AEM.00062-07
Wang, Z., Li, C., and White, J. (2020b). Effects of Epichloë endophyte infection on growth, physiological properties and seed germination of wild barley under saline conditions. J. Agron. Crop Sci. 206, 43–51. doi: 10.1111/jac.12366
Wei, Z., and Jousset, A. (2017). Plant breeding goes microbial. Trends Plant Sci. 22, 555–558. doi: 10.1016/j.tplants.2017.05.009
Weibull, J. H. W. (1993). Bird cherry-oat aphid (Homoptera: Aphididae) performance on annual and perennial temperate-region grasses. Environ. Entomol. 22, 149–153. doi: 10.1093/ee/22.1.149
Wooley, J. C., Godzik, A., and Friedberg, I. (2010). A primer on metagenomics. PLoS Comput. Biol. 6:e1000667. doi: 10.1371/journal.pcbi.1000667
Zhang, X., Fan, X., Li, C., and Nan, Z. (2010). Effects of cadmium stress on seed germination, seedling growth and antioxidative enzymes in Achnatherum inebrians plants infected with a Neotyphodium endophyte. Plant Growth Regul. 60, 91–97. doi: 10.1007/s10725-009-9422-8
Keywords: seed microbiota, plant-associated bacterial communities, Epichloë endophytes, plant-microbe interactions, herbivory
Citation: Bastías DA, Bustos LB, Jáuregui R, Barrera A, Acuña-Rodríguez IS, Molina-Montenegro MA and Gundel PE (2022) Epichloë Fungal Endophytes Influence Seed-Associated Bacterial Communities. Front. Microbiol. 12:795354. doi: 10.3389/fmicb.2021.795354
Edited by:
Angel Valverde, Spanish National Research Council (CSIC), SpainReviewed by:
Eric Pereira, Institute of Natural Resources and Agrobiology of Salamanca, Spanish National Research Council (CSIC), SpainMatthew Agler, Friedrich Schiller University Jena, Germany
Copyright © 2022 Bastías, Bustos, Jáuregui, Barrera, Acuña-Rodríguez, Molina-Montenegro and Gundel. This is an open-access article distributed under the terms of the Creative Commons Attribution License (CC BY). The use, distribution or reproduction in other forums is permitted, provided the original author(s) and the copyright owner(s) are credited and that the original publication in this journal is cited, in accordance with accepted academic practice. No use, distribution or reproduction is permitted which does not comply with these terms.
*Correspondence: Daniel A. Bastías, ZGFuaWVsLmJhc3RpYXNAYWdyZXNlYXJjaC5jby5ueg==