- 1Sichuan Nursing Vocational College, Chengdu, China
- 2School of the 1st Clinical Medical Sciences, Wenzhou Medical University, Wenzhou, China
- 3School of Nursing, Chengdu University of Traditional Chinese Medicine, Chengdu, China
- 4Animal Breeding and Genetics Key Laboratory of Sichuan Province, Sichuan Academy of Animal Science, Chengdu, China
Ellagic acid (EA), a plant polyphenol mainly found in nuts and fruits, exhibits various biological effects. However, the effects of EA on intestinal health remain poorly understood. Hence, the present study aimed to assess the effects of EA supplementation on jejunal morphology, digestive enzyme activities, antioxidant capacity, and microbiota in C57BL/6J mice. A total of 144 mice were randomly assigned to three treatments groups: the control (CON) group received a standard pellet diet, the 0.1% EA group received a standard pellet diet plus 0.1% EA, and the 0.3% EA group received a standard pellet diet plus 0.3% EA. The mice were killed at the end of the experimental period, and jejunal samples were collected. The results revealed that the mice in the 0.3% EA group had higher (P < 0.05) average daily gain and greater (P < 0.05) jejunal villus height than those in the CON group. In addition, the jejunal lactase and sucrase activities were higher (P < 0.05) in the 0.1% EA and 0.3% EA groups, and the alkaline phosphatase activity was higher (P < 0.05) in the 0.3% EA group than in the CON group. Compared with the CON group, the administration of EA increased (P < 0.05) the superoxide dismutase and catalase activities but decreased (P < 0.05) the malonaldehyde content in the jejunum. Moreover, the jejunal messenger RNA expression levels of nuclear factor-E2-related factor 2 (Nrf2) and haem oxygenase-1 (HO-1) were higher (P < 0.05) in the 0.3% EA group than in the CON group. Furthermore, compared with the CON group, the count of Escherichia coli decreased (P < 0.05), and that of Lactobacillus species increased (P < 0.05) in the 0.3% EA group. In general, our findings indicate that the administration of EA can enhance the growth of mice, promote intestinal development, increase the antioxidant capacity, and regulate the intestinal microbiota.
Introduction
The small intestine serves as the crucial site for nutrient digestion and absorption while simultaneously acting as an important line of defense against the invasion of antigens and pathogens (Nagler-Anderson, 2001; Hirata et al., 2007; Barszcz and Skomiał, 2011). However, the health status of the intestine in young animals could be easily deteriorated by many factors, including pathogen infection, inflammation, and oxidative stress (Liu, 2015; Wan et al., 2021); these factors lead to intestinal mucosal damage and dysfunction and, in turn, negatively affect the growth performance and health of animals (Liu et al., 2008). Interestingly, there is clear evidence that adequate nutrition can help maintain intestinal integrity in animals (He et al., 2017; Wan et al., 2018). Thus, it is extremely important to maintain the structural and functional integrity of the small intestine and to thereby ensure that its absorptive and protective functions are not compromised (Yu et al., 2010).
In recent years, there has been increasing interest in understanding the role of polyphenolic compounds and their possible mechanisms of action in maintaining intestinal health. Ellagic acid (EA), a natural phenolic phytochemical antioxidant, mainly occurs in vegetables and fruits, such as persimmon, raspberries, blackberries, and strawberries, in addition to nuts (Derosa et al., 2016). It has important biological activities, such as antioxidative (Yüce et al., 2007), anti-inflammatory (Marín et al., 2013), anticancer (Umesalma and Sudhandiran, 2011), and antidiabetic (Fatima et al., 2017) activities. Previous studies have shown that EA can scavenge free radicals (Zheng et al., 2020) and inhibit lipid peroxidation (Kilic et al., 2014). More recently, EA supplementation has been reported to improve the activity of antioxidant enzymes and ameliorate intestinal inflammation in vivo and in vitro (Han et al., 2006; Mishra and Vinayak, 2014). However, little information is available about the effects of different EA doses and their protective effects on intestinal health in mice. In light of the earlier information, we assessed the effects of EA supplementation on jejunal development and antioxidant capacity in mice.
Materials and Methods
Animals and Treatment
The Ethics Review Committee for Animal Experimentation of Sichuan Academy of Animal Science (Chengdu, China) approved the animal experimental protocols. The animal experimental protocols were conducted in accordance with the practical animal protection law and the Guide for the Care and Use of Laboratory Animals formulated by the National Research Council (China). A total of 144 C57BL/6J mice weighing 26–30 g were obtained from Dossy Experimental Animals Co., Ltd. (Chengdu, China) and randomly divided into three groups (each group has had 48 mice): the control (CON) group received a standard pellet diet, the 0.1% EA group received a standard pellet diet plus 0.1% EA, and the 0.3% EA group received a standard pellet diet plus 0.3% EA. All the mice were maintained at 25°C with a 12-h light/dark cycle and given food and water ad libitum for 21 consecutive days. All the mice were weighed on the morning of days 1 and 22 to calculate the average daily gain (ADG).
Sample Collection
At the end of the experimental regimen and after 16 h of fasting, the mice were killed by ether anesthesia. The jejunum was removed, and the jejunal contents were collected and frozen at −80°C for microbial DNA analysis. Jejunal tissue samples were collected, washed in ice-cold physiological saline (0.9%), and blotted dry. Then, the jejunal section was divided into two pieces: one was fixed in 4% paraformaldehyde solution for histological studies, and the other was stored at −80°C for further analysis.
Morphological Examination
The 4% paraformaldehyde solution-fixed jejunal samples were dehydrated in ethanol and embedded in paraffin. Then, 5-μm thick transverse sections were cut and stained with hematoxylin and eosin. The villus height and crypt depth of 10 well-oriented villi were measured using a BH2 Olympus microscope (Olympus, Tokyo, Japan).
Jejunal Enzyme Activity Measurements
The jejunal mucosa was homogenized after ensuring that the weight of the intestinal mucosa (g)/volume of physiological saline pre-cooled on ice (ml) was 1:9 and was centrifuged at 2,500 × g for 10 min to obtain the supernatant. Then, the enzyme activities in the jejunal mucosal supernatant were measured strictly in accordance with the instructions provided in the respective kits (Nanjing Jiancheng Bioengineering Institute, Nanjing, China).
Jejunal Antioxidant Capacity Measurements
The superoxide dismutase (SOD) activity, catalase (CAT) activity, malonaldehyde (MDA) content, and total antioxidant capacity (T-AOC) in the jejunal homogenates were measured using commercially available kits (Nanjing Jiancheng Bioengineering Institute, Nanjing, China).
Messenger RNA Abundance Analysis
Total RNA was extracted from the jejunal mucosa using TRIzol reagent (Invitrogen, Carlsbad, CA, United States), according to the manufacturer’s instructions. The sequences of primers used in the present study are provided in Table 1. The RNA concentration was measured using NanoDrop 1000 (Thermo Fisher Scientific), and the RNA integrity was assessed by electrophoresis on 1% agarose gel. The reaction mixture consisted of 5-μl fresh SYBR ® Premix Ex Taq II (Tli RNase H Plus), 0.5-μl forward primer, 0.5-μl reverse primer, 1-μl complementary DNA, and 3-μl diethylpyrocarbonate-treated water. The polymerase chain reaction (PCR) protocol was as follows: 1 cycle at 95°C for 30 s, 40 cycles at 95°C for 5 s and 60°C for 34 s and 1 cycle at 95°C for 15 s, 60°C for 1 min and 95°C for 15 s. The relative expression levels of the target genes to the housekeeping gene (β-actin) were assessed using the 2–ΔΔCt method (Che et al., 2017).
Bacterial DNA Extraction and Real-Time Quantitative Polymerase Chain Reaction
Approximately 1-g jejunal content was used for bacterial DNA extraction using the QIAamp DNA Stool Kit (Qiagen, Hilden, Germany) according to the manufacturer’s instructions. Bacterial DNA extracted from the jejunal contents was used for gene sequence amplification by quantitative PCR using the primers specified in Table 2. Primer specificity was assessed on the basis of the 16S rRNA gene sequence. The reaction conditions for quantitative PCR were as follows: 50°C for 2 min, 95°C for 5 min and 40 cycles of denaturation at 94°C for 20 s, primer annealing at a species-specific temperature for 30 s, and primer extension at 60°C for 1 min (Su et al., 2018).
Statistical Analysis
All results are expressed as the means ± standard errors. Data were analyzed by one-way analysis of variance using the GLM procedure of SAS software (Version 9; SAS Institute, Inc., Cary, NC, United States). All statements of statistical significance are based on a probability of P < 0.05.
Results
Growth Performance
As shown in Figure 1, the mice in the 0.3% EA group had significantly higher (P < 0.05) ADG than those in the CON group.
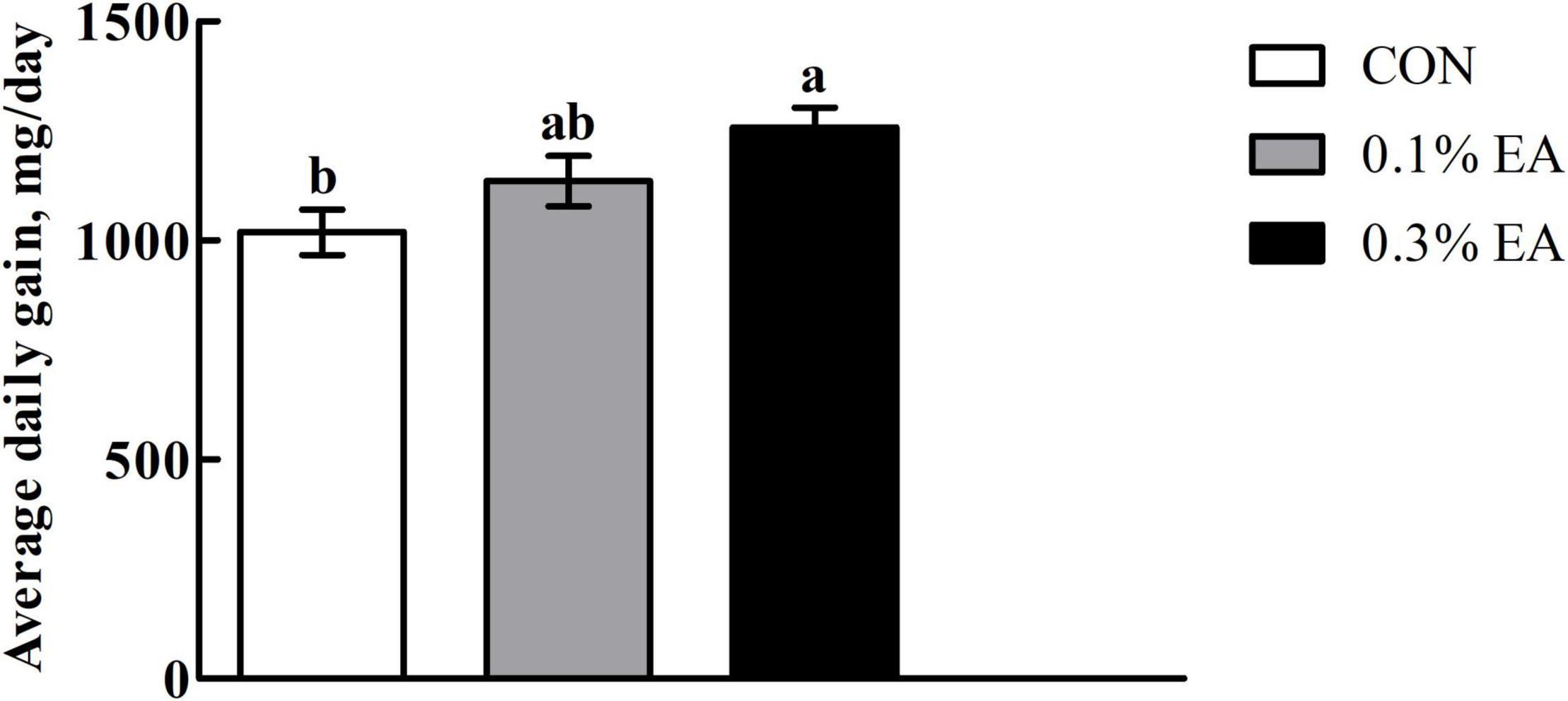
Figure 1. Effects of ellagic acid (EA) supplementation on growth performance of mice. Results are presented as means ± standard errors. a,bMean values followed by different letters within a row indicate significant differences (P < 0.05). CON, control group, mice received a standard pellet diet; 0.1% EA, mice received a standard pellet diet plus 0.1% EA; 0.3% EA, mice received a standard pellet diet plus 0.3% EA.
Jejunal Morphology
The jejunal villus height (Figure 2A) was greater in the mice fed with the EA diet than those fed with the basal diet. The villus height was greater (P < 0.05) in the 0.3% EA group than in the CON group (Figure 2B). However, the crypt depth (Figure 2C) and villus height/crypt depth ratio (Figure 2D) did not differ among the three groups (P > 0.05).
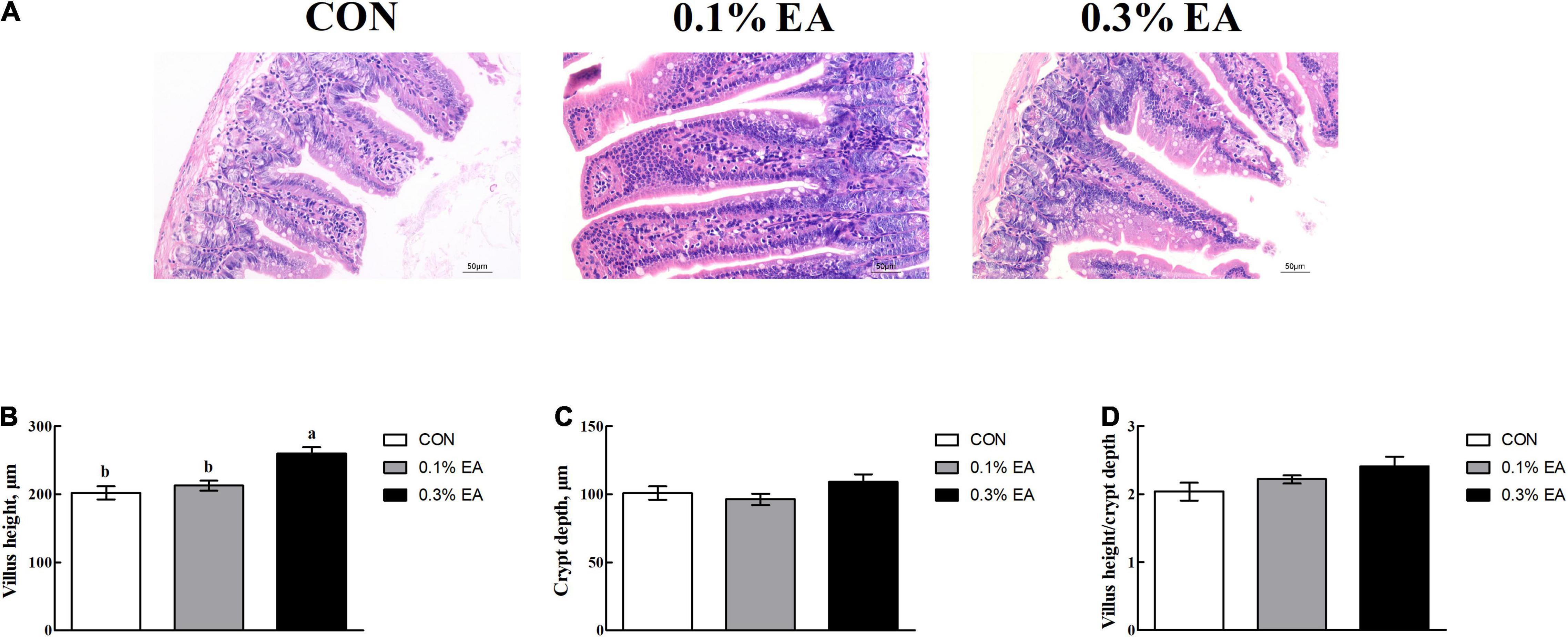
Figure 2. Effects of EA supplementation on jejunal morphology in mice. (A) Image showing jejunal morphology (200×); (B) villus height; (C) crypt depth; (D) villus height/crypt depth ratio. Results are presented as means ± standard errors. a,bMean values followed by different letters within a row indicate significant differences (P < 0.05). CON, control group, mice received a standard pellet diet; 0.1% EA, mice received a standard pellet diet plus 0.1% EA; 0.3% EA, mice received a standard pellet diet plus 0.3% EA.
Jejunal Enzyme Activities
The jejunal enzyme activities are presented in Table 3. Compared with the CON group, the jejunal lactase and sucrase activities were enhanced (P < 0.05) in the 0.1% EA and 0.3% EA groups. Moreover, 0.3% EA supplementation was found to increase (P < 0.05) the jejunal alkaline phosphatase activity.
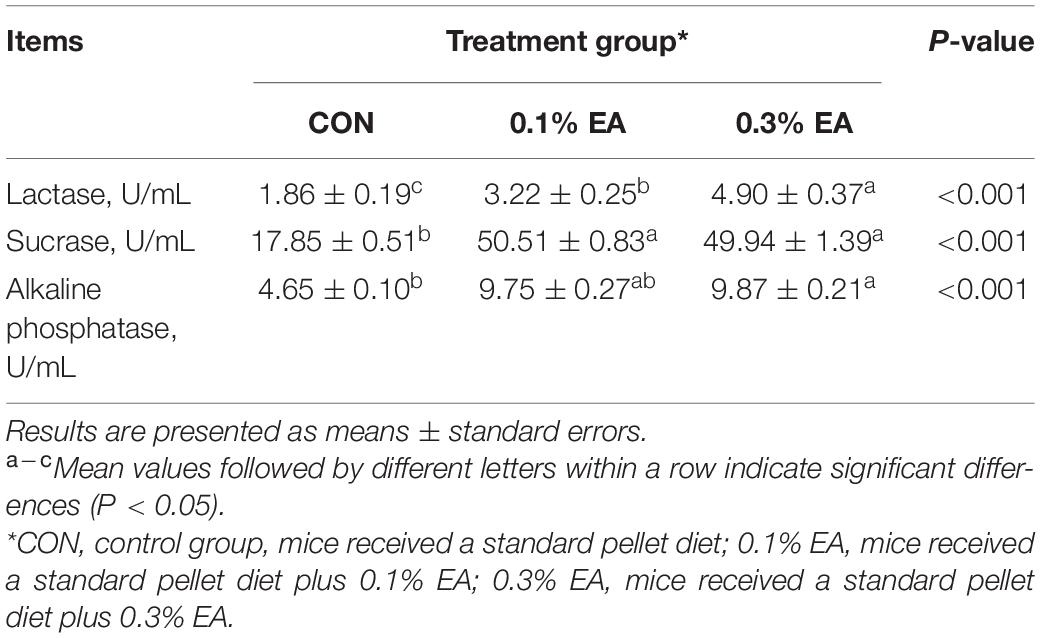
Table 3. Effects of ellagic acid (EA) supplementation on jejunal digestive enzyme activities in mice.
Jejunal Microbiota
The counts of Bacteroidetes and Firmicutes species in the jejunal contents did not change significantly (P > 0.05) after EA supplementation (Table 4). Compared with the CON group, the count of Escherichia coli decreased (P < 0.05), and that of Lactobacillus species increased (P < 0.05) in the 0.3% EA group.
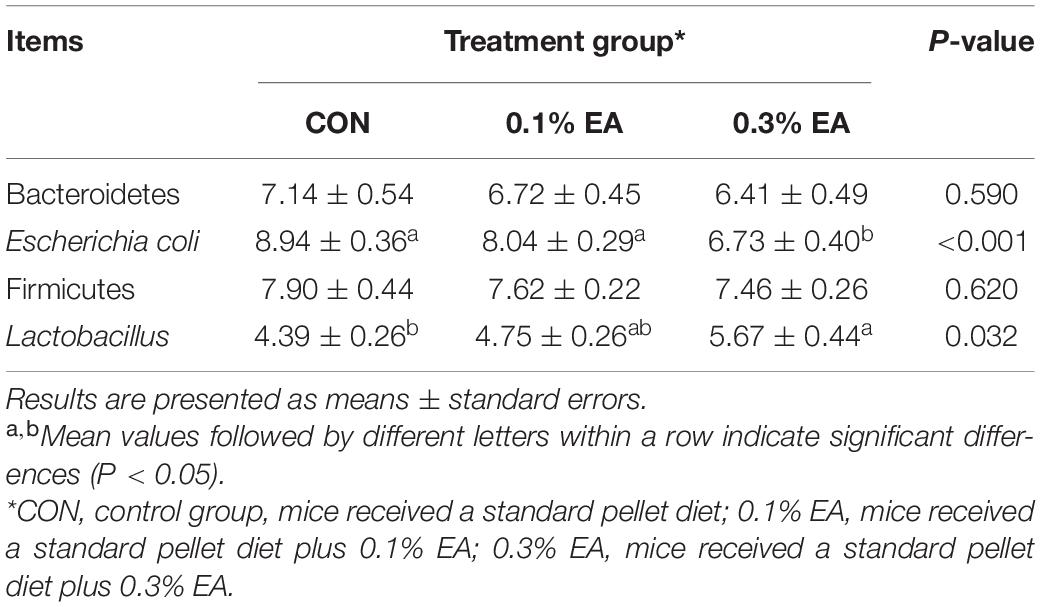
Table 4. Effects of EA supplementation on bacteria in jejunal digesta in mice [log 10 (copies per gram)].
Jejunal Antioxidant Capacity
As shown in Table 5, EA supplementation increased (P < 0.05) the SOD and CAT activities and decreased (P < 0.05) the MDA content in the jejunum of mice.
Nuclear Factor-E2-Related Factor 2 Pathway-Related Gene Expressions Levels
The differences in jejunal nuclear factor-E2-related factor 2 (Nrf2) pathway-related gene expression levels among the three groups are presented in Figure 3. The jejunal messenger RNA (mRNA) expression levels of Nrf2 and haem oxygenase-1 (HO-1) were higher in the 0.3% EA group (P < 0.05) than in the CON group. However, no significant differences (P > 0.05) were noted in the mRNA expression levels of Keap1 and NQO-1 among the three groups.
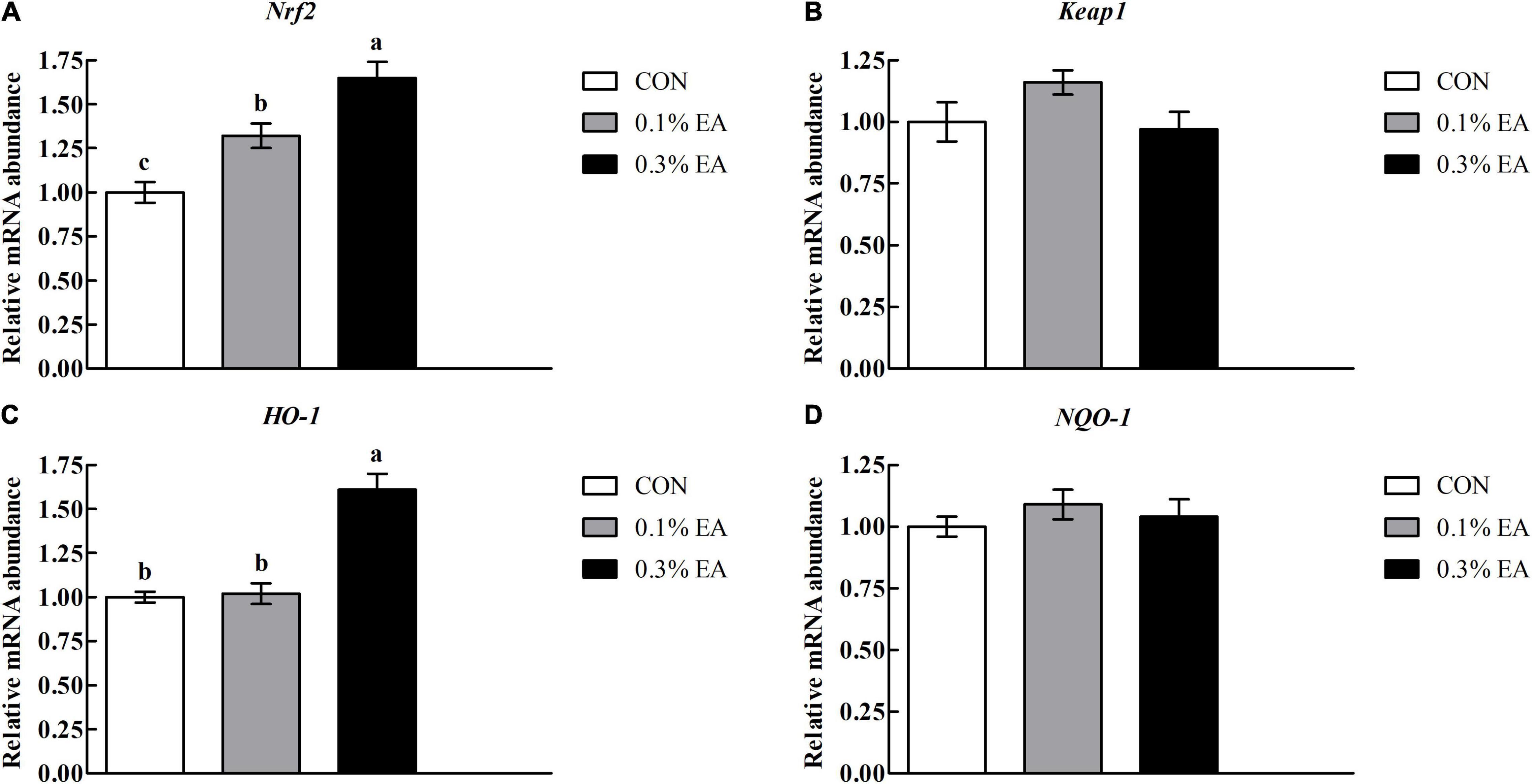
Figure 3. Effects of EA supplementation on nuclear factor-E2-related factor 2 (Nrf2) pathway-related mRNA expression levels in mouse jejunum. (A) Nrf2; (B) Keap1; (C) HO-1; (D) NQO-1. Results are presented as means ± standard errors. a–cMean values followed by different letters within a row indicate significant differences (P < 0.05). CON, control group, mice received a standard pellet diet; 0.1% EA, mice received a standard pellet diet plus 0.1% EA; 0.3% EA, mice received a standard pellet diet plus 0.3% EA.
Discussion
The intestine has an enormous surface area that is optimized to efficiently absorb nutrients, water, and electrolytes from food (König et al., 2016). Maintaining the normal morphology of the intestinal mucosa is essential to ensure efficient digestion and absorption in animals (Mou et al., 2019). The villus height, crypt depth, and villus height/crypt depth ratio are the most direct indices that can reflect the morphology of the small intestine (Qin et al., 2018). In the present study, the jejunal villus height was found to be greater in the 0.3% EA group than in the CON group. This finding was consistent with that of Sun et al. (2017), suggesting that EA administration can change the morphological structure of the mouse jejunum and can promote the development of the small intestine in mice. It is well known that digestive brush border enzymes mainly attach to the apical parts of villi (Asp et al., 1975). Moreover, the proliferation of villi helps more digestive enzymes to enter the intestine, thereby facilitating the digestion and absorption of more nutrients (Cao et al., 2015). However, changes in intestinal morphology are associated with alterations in enzyme activities (Wan et al., 2017). In the current study, we found that 0.1 or 0.3% EA supplementation enhanced the jejunal lactase, sucrase, and alkaline phosphatase activities in mice. BW changes are a comprehensive reflection of health. In our study, mice fed the 0.3% EA diet had higher ADG than those fed the basal diet. This is a comprehensive reflection of EA-induced small intestinal development and improved intestinal nutrient digestion and absorption capacity.
The intestinal antioxidant capacity is also crucial for maintaining intestinal health (Kim et al., 2021). The body has a series of defense mechanisms for controlling oxidative stress; one of these is the enzymatic antioxidant system (Pari and Sivasankari, 2008). SOD and CAT are important enzymatic antioxidants that can provide major antioxidant defenses against ROS (Bhattacharyya et al., 2014; Shen et al., 2014). In the present study, the jejunal SOD and CAT activities significantly increased, whereas the jejunal MDA content decreased in mice fed EA. These findings suggest that EA can improve the jejunal antioxidant capacity. Evidence suggests that the antioxidant effect of EA may be mediated by the stimulation of Nrf2 and the activation of antioxidant response elements, such as HO-1 (Ding et al., 2014). Nrf2 is an important transcription factor that can control the induction of antioxidant genes (Baluchnejadmojarad et al., 2017). In this study, we found that EA supplementation increased Nrf2 and HO-1 mRNA expression levels in the mouse jejunum, suggesting that EA can protect against oxidative stress via the Nrf2/HO-1 pathway.
The intestinal microflora plays an important role in maintaining intestinal microenvironment homeostasis (Liu et al., 2019). Previous studies have revealed an increase in the abundance of Firmicutes and Lactobacillus species and a decrease in the abundance of Verrucomicrobia and Bacteroidetes species in mice after polyphenols supplementation (Kim et al., 2021; Lu et al., 2021). Our results demonstrated that EA supplementation decreased the count of E. coli but increased the count of Lactobacillus species. These results may be attributed to the bacteriostatic or bactericidal action of polyphenols or their ability to inhibit the adhesion of pathogenic bacteria to the intestinal tract cells (Abu Hafsa and Ibrahim, 2018). Furthermore, the intestinal microflora has been reported to affect intestinal morphology (Forder et al., 2007). For instance, dietary Lactobacillus casei supplementation was found to increase the count of Enterobacteriaceae species and improve the intestinal villus length in mice (Allori et al., 2000). In the present study, the increased villus height noted in the 0.3% EA group was consistent with the increased Lactobacillus count in the jejunum, similar to previous findings.
Conclusion
In conclusion, the present study demonstrated that EA administration could enhance the growth of mice, promote jejunal development, improve the jejunal antioxidant capacity, and regulate the intestinal microbiota. These findings provide guidance on EA supplementation.
Data Availability Statement
The original contributions presented in the study are included in the article/supplementary material, further inquiries can be directed to the corresponding author.
Ethics Statement
The animal study was reviewed and approved by the animal experimental protocols were approved by the Ethics Review Committee for Animal Experimentation of Sichuan Academy of Animal Science (Chengdu, China). The animal experimental protocols were conducted in accordance with the practical animal protection law and the Guide for the Care and Use of Laboratory Animals formulated by the National Research Council (China).
Author Contributions
QX and HD conceived the present study and wrote the manuscript. MS and YH conducted the experiments and performed the data analysis. All authors contributed to the article and approved the submitted version.
Funding
This study was financially supported by the school-level project of Sichuan Nursing Vocational College (2021ZRZ02).
Conflict of Interest
The authors declare that the research was conducted in the absence of any commercial or financial relationships that could be construed as a potential conflict of interest.
Publisher’s Note
All claims expressed in this article are solely those of the authors and do not necessarily represent those of their affiliated organizations, or those of the publisher, the editors and the reviewers. Any product that may be evaluated in this article, or claim that may be made by its manufacturer, is not guaranteed or endorsed by the publisher.
References
Abu Hafsa, S. H., and Ibrahim, S. A. (2018). Effect of dietary polyphenol-rich grape seed on growth performance, antioxidant capacity and ileal microflora in broiler chicks. J. Anim. Physiol. Anim. Nutr. 102, 268–275. doi: 10.1111/jpn.12688
Allori, C., Agüero, G., de Ruiz Holgado, A. P., De Nader, O. M., and Perdigon, G. (2000). Gut mucosa morphology and microflora changes in malnourished mice after renutrition with milk and administration of Lactobacillus casei. J. Food Prot. 63, 83–90. doi: 10.4315/0362-028x-63.1.83
Asp, N.-G., Gudmand-Höyer, E., Andersen, B., Berg, N.-O., and Dahlqvist, A. (1975). Distribution of disaccharidases, alkaline phosphatase, and some intracellular enzymes along the human small intestine. Scand. J. Gastroent. 10, 647–651. doi: 10.1080/00365521.1975.12097025
Baluchnejadmojarad, T., Rabiee, N., Zabihnejad, S., and Roghani, M. (2017). Ellagic acid exerts protective effect in intrastriatal 6-hydroxydopamine rat model of Parkinson’s disease: possible involvement of ERβ/Nrf2/HO-1 signaling. Brain Res. 1662, 23–30. doi: 10.1016/j.brainres.2017.02.021
Barszcz, M., and Skomiał, J. (2011). The development of the small intestine of piglets-chosen aspects. J. Anim. Feed Sci. 20, 3–15.
Bhattacharyya, A., Chattopadhyay, R., Mitra, S., and Crowe, S. E. (2014). Oxidative stress: an essential factor in the pathogenesis of gastrointestinal mucosal diseases. Physiol. Rev. 94, 329–354. doi: 10.1152/physrev.00040.2012
Cao, W., Liu, G. M., Fang, T. T., Wu, X. J., Jia, G., Zhao, H., et al. (2015). Effects of spermine on the morphology, digestive enzyme activities, and antioxidant status of jejunum in suckling rats. Rsc. Adv. 5, 76607–76614. doi: 10.1039/c5ra15793e
Che, L. Q., Xu, Q., Wu, C., Luo, Y. H., Huang, X. B., Zhang, B., et al. (2017). Effects of dietary live yeast supplementation on growth performance, diarrhoea severity, intestinal permeability and immunological parameters of weaned piglets challenged with enterotoxigenic Escherichia coli K88. Br. J. Nutr. 118, 949–958. doi: 10.1017/S0007114517003051
Derosa, G., Maffioli, P., and Sahebkar, A. (2016). Ellagic acid and its role in chronic diseases. Adv. Exp. Med. Biol. 928, 473–479.
Ding, Y., Zhang, B., Zhou, K. Y., Chen, M. C., Wang, M. M., Jia, Y. Y., et al. (2014). Dietary ellagic acid improves oxidant-induced endothelial dysfunction and atherosclerosis: role of Nrf2 activation. Int. J. Cardiol. 175, 508–514. doi: 10.1016/j.ijcard.2014.06.045
Fatima, N., Hafizur, R. M., Hameed, A., Ahmed, S., Nisar, M., and Kabir, N. (2017). Ellagic acid in Emblica officinalis exerts anti-diabetic activity through the action on β-cells of pancreas. Eur. J. Nutr. 56, 591–601. doi: 10.1007/s00394-015-1103-y
Forder, R. E. A., Howarth, G. S., Tivey, D. R., and Hughes, R. J. (2007). Bacterial modulation of small intestinal goblet cells and mucin composition during early posthatch development of poultry. Poultry Sci. 86, 2396–2403. doi: 10.3382/ps.2007-00222
Han, D. H., Lee, M. J., and Kim, J. H. (2006). Antioxidant and apoptosis-inducing activities of ellagic acid. Anticancer Res. 26, 3601–3606.
He, L. Q., Zhou, X. H., Huang, N., Li, H., Cui, Z. J., Tian, J. Q., et al. (2017). Administration of alpha-ketoglutarate improves epithelial restitution under stress injury in early-weaning piglets. Oncotarget 8, 91965–91978. doi: 10.18632/oncotarget.20555
Hirata, Y., Broquet, A. H., Menchén, L., and Kagnoff, M. F. (2007). Activation of innate immune defense mechanisms by signaling through RIG-I/IPS-1 in intestinal epithelial cells. J. Immunol. 179, 5425–5432. doi: 10.4049/jimmunol.179.8.5425
Kilic, I., Yeşiloğlu, Y., and Bayrak, Y. (2014). Spectroscopic studies on the antioxidant activity of ellagic acid. Spectroc. Acta Pt. A Molec. Biomolec. Spectr. 130, 447–452. doi: 10.1016/j.saa.2014.04.052
Kim, D.-H., Sim, Y., Hwang, J.-H., Kwun, I.-S., Lim, J.-H., Kim, J., et al. (2021). Ellagic acid prevents binge alcohol-induced leaky gut and liver injury through inhibiting gut dysbiosis and oxidative stress. Antioxidants 10:1386. doi: 10.3390/antiox10091386
König, J., Wells, J., Cani, P. D., García-Ródenas, C. L., MacDonald, T., Mercenier, A., et al. (2016). Human intestinal barrier function in health and disease. Clin. Transl. Gastroenterol. 7:e196.
Liu, J., Wang, H.-W., Lin, L., Miao, C.-Y., Zhang, Y., and Zhou, B.-H. (2019). Intestinal barrier damage involved in intestinal microflora changes in fluoride-induced mice. Chemosphere 234, 409–418. doi: 10.1016/j.chemosphere.2019.06.080
Liu, Y. L. (2015). Fatty acids, inflammation and intestinal health in pigs. J. Anim. Sci. Biotechnol. 6, 1–9. doi: 10.1016/j.plefa.2018.10.006
Liu, Y. L., Huang, J. J., Hou, Y. Q., Zhu, H. L., Zhao, S. J., Ding, B. Y., et al. (2008). Dietary arginine supplementation alleviates intestinal mucosal disruption induced by Escherichia coli lipopolysaccharide in weaned pigs. Br. J. Nutr. 100, 552–560. doi: 10.1017/S0007114508911612
Lu, F., Li, Y. Y., Wang, X., Hu, X. S., Liao, X. J., and Zhang, Y. (2021). Early-life polyphenol intake promotes Akkermansia growth and increase of host goblet cells in association with the potential synergistic effect of Lactobacillus. Food Res. Int. 149:110648. doi: 10.1016/j.foodres.2021.110648
Marín, M., Giner, R. M., Ríos, J.-L., and Recio, M. C. (2013). Intestinal anti-inflammatory activity of ellagic acid in the acute and chronic dextrane sulfate sodium models of mice colitis. J. Ethnopharmacol. 150, 925–934. doi: 10.1016/j.jep.2013.09.030
Mishra, S., and Vinayak, M. (2014). Ellagic acid inhibits PKC signaling by improving antioxidant defense system in murine T cell lymphoma. Mol. Biol. Rep. 41, 4187–4197. doi: 10.1007/s11033-014-3289-0
Mou, Q., Yang, H.-S., Yin, Y.-L., and Huang, P.-F. (2019). Amino acids influencing intestinal development and health of the piglets. Animals 9:302. doi: 10.3390/ani9060302
Nagler-Anderson, C. (2001). Man the barrier! Strategic defences in the intestinal mucosa. Nat. Rev. Immunol. 1, 59–67. doi: 10.1038/35095573
Pari, L., and Sivasankari, R. (2008). Effect of ellagic acid on cyclosporine A-induced oxidative damage in the liver of rats. Fundam. Clin. Pharmacol. 22, 395–401. doi: 10.1111/j.1472-8206.2008.00609.x
Qin, Q., Xu, X., Wang, X. Y., Wu, H. T., Zhu, H. L., Hou, Y. Q., et al. (2018). Glutamate alleviates intestinal injury, maintains mTOR and suppresses TLR4 and NOD signaling pathways in weanling pigs challenged with lipopolysaccharide. Sci. Rep. 8:15124. doi: 10.1038/s41598-018-33345-7
Shen, X. J., Yi, D., Ni, X. Q., Zeng, D., Jing, B., Lei, M. X., et al. (2014). Effects of Lactobacillus plantarum on production performance, immune characteristics, antioxidant status, and intestinal microflora of bursin-immunized broilers. Can. J. Microbiol. 60, 193–202. doi: 10.1139/cjm-2013-0680
Su, J. Y., Zhu, Q., Zhao, Y., Han, L., Yin, Y. L., Blachier, F., et al. (2018). Dietary supplementation with chinese herbal residues or their fermented products modifies the colonic microbiota, bacterial metabolites, and expression of genes related to colon barrier function in weaned piglets. Front. Microbiol. 9:3181. doi: 10.3389/fmicb.2018.03181
Sun, Y.-Q., Tao, X., Men, X.-M., Xu, Z.-W., and Wang, T. (2017). In vitro and in vivo antioxidant activities of three major polyphenolic compounds in pomegranate peel: ellagic acid, punicalin, and punicalagin. J. Integr. Agric. 16, 1808–1818.
Umesalma, S., and Sudhandiran, G. (2011). Ellagic acid prevents rat colon carcinogenesis induced by 1, 2 dimethyl hydrazine through inhibition of AKT-phosphoinositide-3 kinase pathway. Eur. J. Pharmacol. 660, 249–258. doi: 10.1016/j.ejphar.2011.03.036
Wan, J., Jiang, F., Xu, Q. S., Chen, D. W., Yu, B., Huang, Z. Q., et al. (2017). New insights into the role of chitosan oligosaccharide in enhancing growth performance, antioxidant capacity, immunity and intestinal development of weaned pigs. RSC. Adv. 7, 9669–9679. doi: 10.1039/c7ra00142h
Wan, J., Zhang, J., Chen, D. W., Yu, B., Huang, Z. Q., Mao, X. B., et al. (2018). Alginate oligosaccharide enhances intestinal integrity of weaned pigs through altering intestinal inflammatory responses and antioxidant status. RSC. Adv. 8, 13482–13492.
Wan, J., Zhang, J., Xu, Q. S., Yin, H., Chen, D. W., Yu, B., et al. (2021). Alginate oligosaccharide protects against enterotoxigenic Escherichia coli-induced porcine intestinal barrier injury. Carbohydr. Polym. 270:118316. doi: 10.1016/j.carbpol.2021.118316
Yu, J., Yin, P., Liu, F. H., Cheng, G. L., Guo, K. J., Lu, A., et al. (2010). Effect of heat stress on the porcine small intestine: a morphological and gene expression study. Comp. Biochem. Physiol. A Mol. Integr. Physiol. 156, 119–128. doi: 10.1016/j.cbpa.2010.01.008
Yüce, A., Ateşşahin, A., ÇeribaşSi, A. O., and Aksakal, M. (2007). Ellagic acid prevents cisplatin-induced oxidative stress in liver and heart tissue of rats. Basic Clin. Pharmacol. Toxicol. 101, 345–349. doi: 10.1111/j.1742-7843.2007.00129.x
Keywords: ellagic acid, morphology, digestive enzyme activities, antioxidant, microbiota, Nrf2, HO-1
Citation: Xu Q, Shen M, Han Y and Diao H (2021) Effects of Ellagic Acid Supplementation on Jejunal Morphology, Digestive Enzyme Activities, Antioxidant Capacity, and Microbiota in Mice. Front. Microbiol. 12:793576. doi: 10.3389/fmicb.2021.793576
Received: 12 October 2021; Accepted: 01 November 2021;
Published: 08 December 2021.
Edited by:
Zheng Ruan, Nanchang University, ChinaReviewed by:
Junqiu Luo, Sichuan Agricultural University, ChinaLuoyi Zhu, Zhejiang University, China
Copyright © 2021 Xu, Shen, Han and Diao. This is an open-access article distributed under the terms of the Creative Commons Attribution License (CC BY). The use, distribution or reproduction in other forums is permitted, provided the original author(s) and the copyright owner(s) are credited and that the original publication in this journal is cited, in accordance with accepted academic practice. No use, distribution or reproduction is permitted which does not comply with these terms.
*Correspondence: Qiuying Xu, eHVxaXV5aW5nMDgwOEAxNjMuY29t