- 1Department of Medical Sciences, University of Ferrara, Ferrara, Italy
- 2Center for Studies on Gender Medicine, Department of Medical Sciences, University of Ferrara, Ferrara, Italy
- 3Laboratory for Technologies of Advanced Therapies (LTTA), University of Ferrara, Ferrara, Italy
Merkel cell polyomavirus (MCPyV) is a small DNA tumor virus ubiquitous in humans. MCPyV establishes a clinically asymptomatic lifelong infection in healthy immunocompetent individuals. Viral infections are considered to be risk factors for spontaneous abortion (SA), which is the most common adverse complication of pregnancy. The role of MCPyV in SA remains undetermined. Herein, the impact of MCPyV infection in females affected by SA was investigated. Specifically, an indirect enzyme-linked immunosorbent assay (ELISA) method with two linear synthetic peptides/mimotopes mimicking MCPyV antigens was used to investigate immunoglobulin G (IgG) antibodies against MCPyV in sera from 94 females affected by SA [mean ± standard deviation (SD) age 35 ± (6) years] and from 96 healthy females undergoing voluntary pregnancy interruption [VI, mean (±SD) age 32 ± (7) years]. MCPyV seroprevalence and serological profiles were analyzed. The overall prevalence of serum IgG antibodies against MCPyV was 35.1% (33/94) and 37.5% (36/96) in SA and VI females, respectively (p > 0.05). Notably, serological profile analyses indicated lower optical densities (ODs) in females with SA compared to those undergoing VI (p < 0.05), thus indicating a reduced IgG antibody response in SA females. Circulating IgGs were identified in sera from SA and VI females. Our immunological findings indicate that a relatively reduced fraction of pregnant females carry serum anti-MCPyV IgG antibodies, while SA females presented a more pronounced decrease in IgG antibody response to MCPyV. Although yet to be determined, this immunological decrease might prompt an increase in MCPyV multiplication events in females experiencing abortive events. The role of MCPyV in SA, if present, remains to be determined.
Introduction
Merkel cell polyomavirus (MCPyV) is a small DNA tumor virus (Witkin et al., 2020). It is the main causative agent of Merkel cell carcinoma (MCC), which is a rare, but highly aggressive, non-melanoma skin cancer (Rotondo et al., 2017; Jin et al., 2019). MCPyV oncogenic activity is mediated by viral DNA integration into the host genome (Rotondo et al., 2017; Del Marmol and Lebbé, 2019; Pietropaolo et al., 2020), alongside the expression of two viral oncoproteins large T (LT) and small T (sT) antigens (Csoboz et al., 2020; Liu and You, 2020). Two additional MCPyV proteins are major capsid protein 1 (VP1) and minor capsid protein 2 (VP2), which present structural functions (Pietropaolo et al., 2020). VPs have previously been exploited as immunoantigens in studies focusing on the detection of immunoglobulin G (IgG) antibodies against MCPyV in humans (Touzé et al., 2010, 2011). Serological studies have indicated that MCPyV is ubiquitous in the healthy population, with varying rates reported as ranging from 60 to 80%, approximately (Carter et al., 2009; Kean et al., 2009; Pastrana et al., 2009; Faust et al., 2011; Tolstov et al., 2011; Touzé et al., 2011; Viscidi et al., 2011; Coursaget et al., 2013; Van Der Meijden et al., 2013; Šroller et al., 2014; Zhang et al., 2014; Vahabpour et al., 2016; Kamminga et al., 2018; Csoboz et al., 2020; Zhou et al., 2020; Mazziotta et al., 2021a,b). Initial exposure to MCPyV occurs early in life. Then, MCPyV establishes a lifelong, asymptomatic infection in healthy immunocompetent individuals (Hashida et al., 2016; Prezioso et al., 2019). However, in certain circumstances, such as during host immune system impairment, increased MCPyV replication levels/activity can occur, thereby leading to an increase in MCC occurrence (Rotondo et al., 2017; Tabachnick-Cherny et al., 2020; Decaprio, 2021).
Spontaneous abortion (SA) is the natural loss of pregnancy before the 20th week of gestation and represents the most common adverse complication in pregnancy. Approximately 10–20% of clinically recognized pregnancies end in a spontaneous loss of the embryo/fetus (Hertz-Picciotto and Samuels, 1988; American College of Obstetricians and Gynecologists Committee on Practice Bulletins—Gynecology, 2018).
Spontaneous abortion causes comprise genetic abnormalities in either partners, which may lead to abnormal chromosomal numbers or alterations (Eiben et al., 1990; Suzumori and Sugiura-Ogasawara, 2012; Dean et al., 2018). Additional SA causes include negative lifestyle factors, such as unhealthy diet/weight other than smoking, alcohol and drug abuse, and other factors, such as ethnicity, stress, and occupational/chemical exposures (De La Rochebrochard and Thonneau, 2002; Sopori, 2002; Lashen et al., 2004). Hormonal, anatomical, and autoimmune abnormalities, as well as male genetic/epigenetic factors, have also been identified (Toth et al., 2010; Rotondo et al., 2012, 2013, 2021a). Notably, although a variety of SA factors have been reported, other causes are yet to be determined (Miyaji et al., 2019; Fukuta et al., 2020). Indeed, nearly one-half of SA cases, defined as idiopathic, presents an undefined etiology (Jeve and Davies, 2014).
A growing number of studies have identified infectious agents as SA risk factors (Giakoumelou et al., 2016; Contini et al., 2018). Nearly 40% of SA cases are estimated to be linked to infectious agents, including viruses (Donders et al., 2000; Srinivas et al., 2006; Giakoumelou et al., 2016), while over 60% of females experience at least one infection during pregnancy (Collier et al., 2009). Viruses can therefore cause severe complications during pregnancy and are reported as associated with cases of stillbirth and preterm delivery, in addition to SA (Giakoumelou et al., 2016; Racicot and Mor, 2017).
Different human viruses, such as dengue, Zika, adeno- and adeno-associated viruses, human cytomegalovirus (HCMV), herpes simplex viruses 1 and 2 (HSV-1 and HSV-2), and the recently discovered severe acute respiratory syndrome coronavirus 2 (SARS-CoV-2), can potentially impact pregnancy outcome, ending, in the worst cases, in an SA event (Burton and Watson, 1997; Fisher et al., 2000; Giakoumelou et al., 2016; Azevedo et al., 2018; Carabali et al., 2018; de Freitas et al., 2018; Sayyadi-Dehno et al., 2019; Auriti et al., 2021; Rotondo et al., 2021b).
The limited amount of data available preclude robust conclusions on the clinical significance of polyomavirus (PyV) infections, including MCPyV, in SA from being made (Tagliapietra et al., 2019, 2020; Mazzoni et al., 2020). Footprints of viral DNAs from PyVs, including Simian virus 40 (SV40), JCPyV/BKPyV, and MCPyV, have been detected at low prevalence in umbilical cords, placenta, peripheral blood mononuclear cells (PBMCs), and/or chorionic villi from both pregnant and SA females (Sadeghi et al., 2010; Tagliapietra et al., 2019, 2020; Mazzoni et al., 2020). Immunological data have also indicated the presence of circulating IgGs against SV40, JCPyV, and BKPyV in the same study groups (Tagliapietra et al., 2019, 2020). A role in SA has been excluded for other PyVs, i.e., KIPyV and WUPyV (Sadeghi et al., 2010). Notably, until now, only one study has reported on the presence of circulating antibodies against MCPyV in pregnant females (Sadeghi et al., 2010), while MCPyV serology in females with SA is unknown.
The lack of immunological data in this field has raised the question of whether MCPyV infection might be linked to SA. To this purpose, we aimed to assess the MCPyV seroprevalence and serological profiles in two sets of sera belonging to females affected by SA, the case, and healthy females (HF) undergoing voluntary interruption of pregnancy (VI), as control.
Materials and Methods
Human Sera
Human sera were obtained from females who had experienced SA (n = 94), i.e., the case, and females undergoing VI (n = 96), i.e., the control. SA and VI sera were from our archive (Tognon et al., 2020). Samples were collected within 12 h from the abortion. Samples were stored at −80°C until testing, as reported previously (Mazziotta et al., 2021a,b). The mean ages [± standard deviation (SD)] of SA and VI groups were 35 ± 6 and 32 ± 7 years (p > 0.05), respectively. SA and VI inclusion criteria were (i) patients aged 18–42 years; (ii) gestational age within the first 12 weeks; and (iii) for the VI group, females selected according to Italian Law 194, article 6, comma B. Exclusion criteria were (i) severe hormonal or uterine dysregulations; (ii) immunosuppressive therapies known to cause SA events; (iii) genetic disorders; (iv) presence of infections, such as hepatitis B virus (HBV), human immunodeficiency virus (HIV), hepatitis C virus (HCV), and syphilis; (v) use of teratogenic drugs; and (vi) acquired/congenital immunodeficiency syndromes/diseases. Written informed consent was obtained from all subjects/patients according to the Declaration of Helsinki. The Ethical Committee of Ferrara, Italy, authorized the study (ID: 151078). In addition, immunological data from a set of sera belonging to a cohort of age-matched HF [n = 95, mean age ± SD, 34 ± (9) years] (p > 0.05), from our previous study (Mazziotta et al., 2021b), were included herein for comparison.
MCPyV Linear Synthetic Peptides
The indirect enzyme-linked immunosorbent assay (ELISA) employed in this study to detect IgGs to MCPyV in sera from SA and VI was recently developed and validated (Mazziotta et al., 2021a,b). The immunoassay uses two linear synthetic peptides/mimotopes, known as MCPyV VP1 S and VP2 F (or S and F peptides) for detecting circulating IgGs against MCPyV in healthy adult and elderly individuals, as described (Mazziotta et al., 2021a,b). The peptides were synthesized using standard procedures and purchased from UFPeptides s.r.l., Ferrara, Italy. Amino acid (a.a.) sequences of VP1 S (24 a.a. residues) and VP2 F (25 a.a. residues) peptides are as follows:
VP1 S: NH2-NSPDLPTTSNWYTYTYDLQPKGSS-COOH and
VP2 F: NH2-SLSPTSRLQIQSNLVNLILNSRWVF-COOH.
Indirect Enzyme-Linked Immunosorbent Assay
Indirect ELISA was performed as reported (Mazziotta et al., 2021a,b). S and F peptides, 5 μg each, were diluted in 100 μl of coating buffer 1X, pH 9.6 (Candor Bioscience, Wangen, Germany), which was used to coat each well of the immunological plates (Nunc-Immuno PolySorp, Thermo Fisher Scientific, Milan, Italy). The peptide-coated plates were incubated at 4°C for 16 h. Successively, immunological plates were rinsed three times with a washing buffer (Candor Bioscience, Wangen, Germany) to remove unbound peptides. For the blocking phase, 200 μl per well of blocking solution containing the casein and Tween detergent (Candor Bioscience, Wangen, Germany) was added to each well and incubated at 37°C for 90 min. Plates were washed three times with the washing buffer before serum samples were added. Each well was covered with 100 μl of serum samples diluted 1:20 in a low cross-buffer (Candor Bioscience, Wangen, Germany). Sera in each plate included (i) positive controls, that is, immune human sera derived from patients with MCPyV-positive MCC (Mazziotta et al., 2021b); (ii) negative controls, that is, three human MCPyV-negative sera (Mazziotta et al., 2021b); and (iii) sera from SA and VI under analysis. Immunological plates with sera were incubated at 37°C for 90 min. Each sample was analyzed in triplicate. Wells were washed three times, and then the secondary antibody was added to each sample. This solution consists of a goat anti-human IgG heavy (H)- and light (L)-chain-specific peroxidase conjugate (Calbiochem-Merck, Darmstadt, Germany) diluted 1:10,000 in a low cross-buffer. The solution was added to each well, whereas plates were incubated at room temperature (RT) for 90 min. After 90-min incubation, the plates were washed three times; then 100 μl of 2,2′-azino-bis-3-ethylbenzothiazoline-6-sulfonic acid (ABTS) solution (Sigma-Aldrich, Milan, Italy) was added to each well. Plates were incubated at RT for 45 min. ABTS reacted with the peroxidase enzyme to yield the color reaction. Finally, the plate was read with a spectrophotometer (Thermo Electron Corp., model Multiskan EX, Vantaa, Finland) at a wavelength (λ) of 405 nm. Color intensity in wells was determined by an optical density (OD) reading. The OD readings correspond to the amount of immune complexes formed by the specific antibodies binding to S and F synthetic peptides/mimotopes.
The cutoff for each S and F peptide was set in each indirect ELISA run, as the mean of the OD readings of negative control sera (n = 3) plus three SDs of mean (mean + 3 SDs) (Classen et al., 1987; Saraswati et al., 2019; Mazziotta et al., 2021a,b), as described previously for other ELISA methods (Meyer, 2001; Lardeux et al., 2016).
Immune serum samples were considered MCPyV positive when reacting to both S and F synthetic peptides, in three replica ELISA experiments carried out by three independent operators, without data variability.
Statistical Analysis
Merkel cell polyomavirus seroprevalence rates were statistically analyzed applying a two-sided chi-square test (Tognon et al., 2020; Oton-Gonzalez et al., 2021). Values were analyzed using the D’Agostino Pearson normality test, and parametric and non-parametric tests were applied according to normal and non-normal variables, respectively, as reported (Nakagawa and Schielzeth, 2010; Rotondo et al., 2020a). In detail, ODs were analyzed using a one-way analysis of variance (ANOVA) and Kruskal–Wallis multiple comparison tests, according to normal and non-normal variables, respectively [OD medians, 95% confidence interval (CI)]. The Spearman correlation coefficient r was used to evaluate the OD concordance between S and F peptides. Statistical analyses were carried out using GraphPad Prism version 8.0 for Windows (GraphPad, La Jolla, CA, United States) (Rotondo et al., 2020b). A p-value < 0.05 was considered statistically significant (Mazzoni et al., 2020).
Results
Indirect Enzyme-Linked Immunosorbent Assay Reliability Assessment
In the first phase of this investigation, the reliability of our immunoassay was assessed in sera from female patients with SA and those who had undergone VI, by determining the OD concordance between MCPyV VP1 S and VP2 F peptides. OD concordance between S and F peptides was evaluated using the Spearman correlation analysis in SA (n = 94) and VI (n = 96) sera. In addition, immunological data from a group of age-matched HF (n = 95), which had previously been investigated in our laboratory for MCPyV serology (Mazziotta et al., 2021b), were included herein for statistical comparisons. OD concordance between S and F peptides was therefore analyzed in SA, VI, and HF sera considered alone, as well as in combination (n = 285). Results indicate a good degree of correlation between ODs for S and F peptides for the SA group, with a Spearman coefficient r of 0.7727 (p < 0.0001) (Figure 1A), and for the VI group, with an r of 0.6221 (p < 0.0001) (Figure 1B). A good correlation between S and F peptide ODs was also found in HF sera with an r of 0.7686 (p < 0.0001) (Figure 1C). In addition, when evaluating the combined SA, VI, and HF sera, a good concordance between S and F was determined with an r of 0.7854 (p < 0.0001) (Figure 1D). These data indicate that both peptides can be used simultaneously, therefore underlining that our assay is reliable in detecting anti-MCPyV IgGs in sera from SA and VI females.
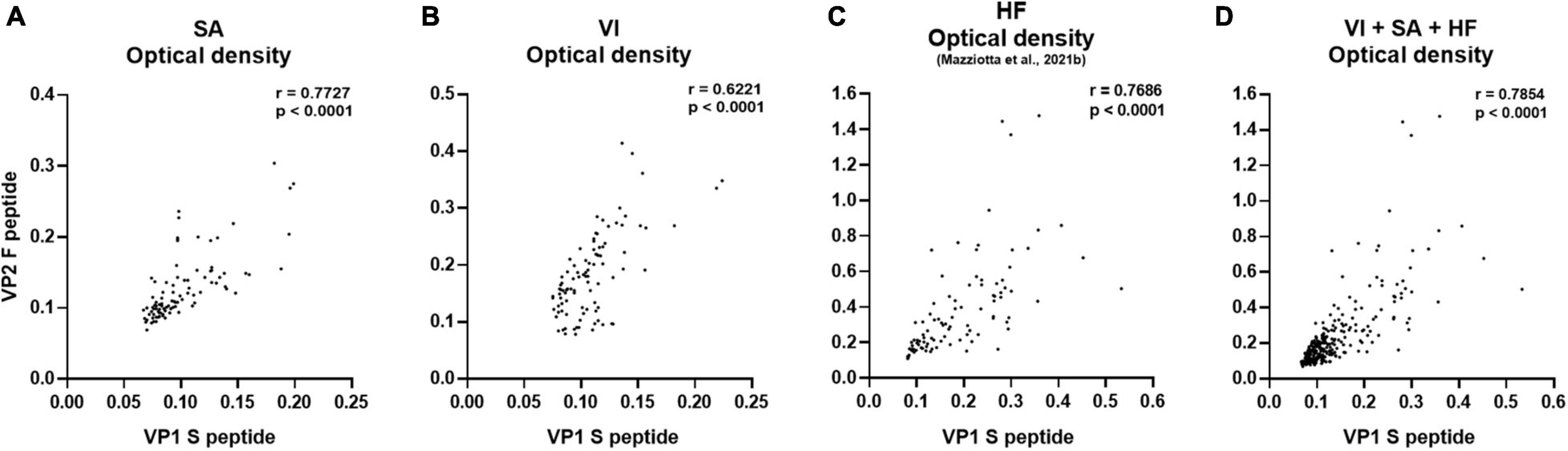
Figure 1. Correlation of OD values obtained using MCPyV VP1 S and VP2 F mimotopes. The concordance in ODs between VP1 S and VP2 F peptides was evaluated in sera from females who had SA (n = 94), the case; females undergoing VI (n = 96), the control; age-matched HF (n = 95); and the entire set of combined SA, VI, and HF sera (n = 285), using Spearman correlation analysis. A good correlation between VP1 S and VP2 F peptide was found in SA, VI, and HF sera considered alone, as well as in combined SA, VI, and HF sera with an r of 0.7727 and p < 0.0001 (A), 0.6221 and p < 0.0001 (B), 0.7686 and p < 0.0001 (C), and 0.7854 and p < 0.0001 (D), respectively.
Detection of Serum IgG Antibodies Against MCPyV by Indirect Enzyme-Linked Immunosorbent Assay
Human sera, from SA (n = 94) and VI (n = 96) females, were analyzed by indirect ELISA for IgG Ab reactivity to MCPyV VP1 S and VP2 F peptides/mimotopes. The prevalence of anti-MCPyV IgGs was therefore determined in SA and VI groups. A statistically similar overall prevalence of 36.2% (34/94) and 43.6% (41/94) was obtained in SA sera, when tested with S and F peptides, respectively (p > 0.05) (Table 1). Comparable prevalence rates of 39.6% (38/96) and 47.9% (46/96) were obtained in VI sera reacting to S and F peptides, respectively (p > 0.05). With only a few exceptions, sera testing negative for S peptide did not react to F peptide and vice versa. In detail, 8.5% (8/94) of SA sera resulting negative for S peptide were positive for F peptide, while 1.1% (1/94) of sera tested F peptide negative, while being positive for S peptide. In VI, 10.4% (10/96) of sera tested F peptide positive were negative for S peptide, whereas 2.1% (2/96) of sera negative for F peptide were positive for S peptide.

Table 1. Seroprevalence of IgG antibodies reacting with Merkel cell polyomavirus VP1 S and VP2 F peptides in sera from females who experienced spontaneous abortion (SA) and females undergoing voluntary interruption (VI).
In this study, sera were considered MCPyV positive when reacting with both mimotopes S and F, as previously reported (Mazziotta et al., 2021a,b).
The combined overall prevalence of IgGs against MCPyV, for both S and F peptides, was 35.1% (33/94) and 37.5% (36/96) in serum samples from SA and VI groups, respectively (p > 0.05).
Serological Profiles of Serum IgG Antibodies Reacting to MCPyV
Serological profiles for IgG reactivity to MCPyV VP1 S and VP2 F peptides/mimotopes were analyzed, both for the single peptide and in combination. Immunological data were taken from sera belonging to SA (n = 94) and VI (n = 96) females. Results are reported as OD readings at λ 405 nm. The median [interquartile range (IQR)] ODs for S peptide and F peptide, alone and in combination, were then determined in SA and VI sera; then values were compared.
Serologic profile analysis indicated that the median (IQR) OD values for S peptide resulted as 0.09 (0.08–0.11) and 0.11 (0.09–0.12) in SA and VI groups, respectively (Figure 2A). The median (IQR) OD values for F peptide resulted as 0.11 (0.1–0.14) and 0.18 (0.13–0.23) in SA and VI groups, respectively (Figure 2B). Lastly, the median (IQR) OD values for combined S and F peptides resulted as 0.1 (0.09–0.13) in SA and 0.12 (0.1–0.18) in VI groups (Figure 2C). The difference in OD levels between SA and VI groups was statistically significant for both S and F peptides considered alone, as well as upon combining ODs of the two peptides (p < 0.05, for S peptide; p < 0.0001, for both F peptide alone and combined S and F peptides) (Figure 2).
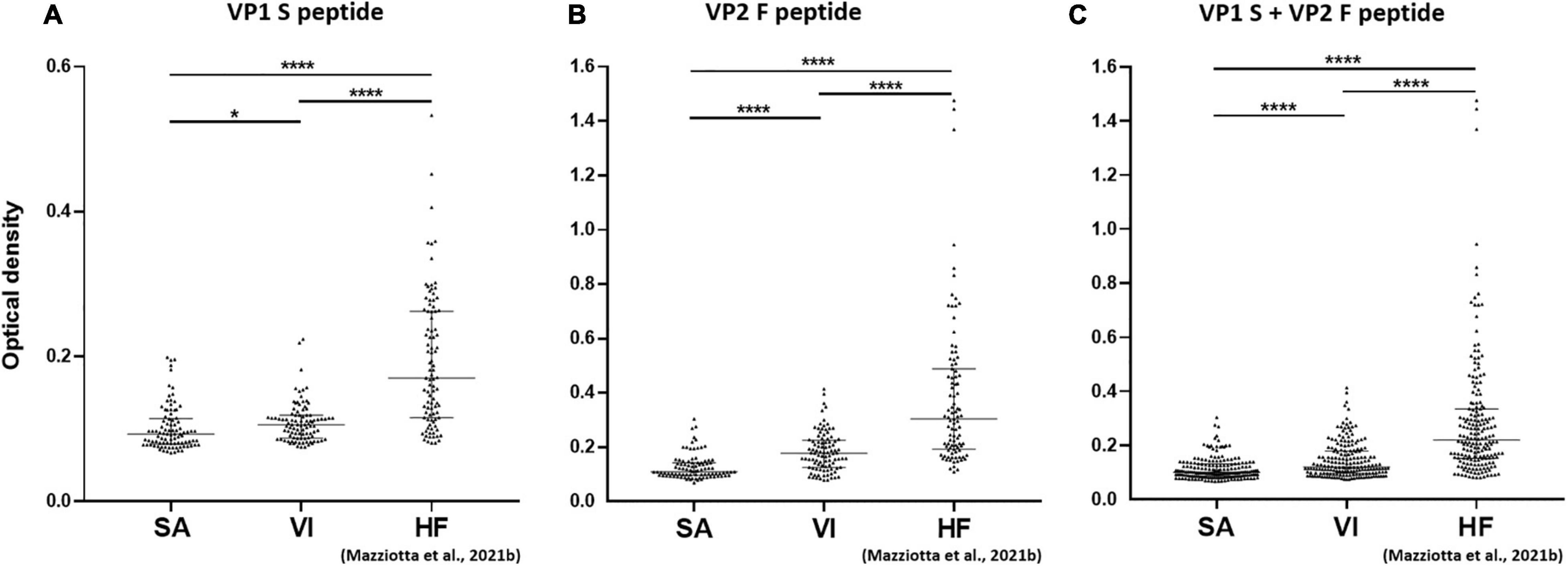
Figure 2. Serological profiles of serum antibody reactivity to MCPyV VP1 S (A), VP2 F (B) peptides, and combined S and F peptides (C) in females who had SA, females who had undergone VI, and age-matched HF. Immunologic data are from SA (n = 94), VI (n = 96), and HF (n = 95) females, whereas data are reported as OD value readings at λ 405 nm for sera assayed in indirect ELISA. In the scatter dot plot, each dot represents the dispersion of ODs for each sample. The median is indicated by the line inside the scatter plot with the [interquartile range (IQR)] in SA and VI cohorts. (A) *p < 0.05, ****p < 0.0001; (B) ****p < 0.0001; (C) ****p < 0.0001.
Serum IgG Reactivity to MCPyV in Females Experiencing Spontaneous Abortion and Healthy Females Who Had Undergone Voluntary Interruption vs. Healthy Non-pregnant Females
In a second phase, MCPyV seroprevalence and serologic profiles in SA (n = 94) and VI (n = 96) females were compared to those obtained in sera from a group of age-matched HF (n = 95) females (p > 0.05), which had previously been investigated in our laboratory for MCPyV serology (Mazziotta et al., 2021b).
Merkel cell polyomavirus seroprevalence was found to be lower in both SA (35.1%, 33/94) and VI groups (37.5%, 36/96) than in the HF group (62.1%, 59/95) (p < 0.001). Serologic profile analysis indicated that S-peptide ODs were lower in both SA [n = 94, median (IQR), 0.09, 0.08–0.11] and VI groups (n = 96, 0.11, 0.09–0.12) than in HF (n = 95, 0.17, 0.12–0.26, p < 0.0001) (Figure 2A). Similarly, F-peptide ODs were lower in both SA (0.11, 0.1–0.14) and VI (0.18, 0.13–0.23) compared to the HF group (0.3, 0.19–0.49, p < 0.0001) (Figure 2B). Furthermore, lower ODs for combined S- and F-peptides were detected in both SA (0.1, 0.09–0.13) and VI sera (0.12, 0.1–0.18) compared to HF (0.22, 0.15–0.34, p < 0.0001) (Figure 2C).
Discussion
The role of MCPyV in SA remains undetermined. Herein, MCPyV seroprevalence and serological profiles were investigated, for the first time, in sera from SA females using an indirect ELISA with two linear synthetic peptides/mimotopes, which mimic MCPyV VP antigens. The lack of immunological studies into the involvement of MCPyV in SA prompted us to apply our newly developed immunoassay (Mazziotta et al., 2021a,b). Sera were considered MCPyV positive when IgGs reacted to both S and F peptides (Mazziotta et al., 2021a,b).
Overall MCPyV-positive prevalence, resulted as 35.1% in SA, was comparable to the 37.5% determined in VI (p > 0.05). Our data indicate that both SA and VI female groups present circulating IgGs to MCPyV, while, at least in terms of seroprevalence, a relationship between MCPyV and SA might be unlikely.
The involvement of MCPyV infection in SA has been poorly investigated (Giakoumelou et al., 2016). Early findings indicate the presence of MCPyV DNA in uterine cervical cancer tissues (Imajoh et al., 2012), suggesting that, after infecting the uterus, this PyV could potentially participate as a SA risk factor. Nevertheless, few copies of MCPyV DNA and/or RNA molecules have been previously identified at low and similar prevalence in chorionic villi and PBMCs from SA and VI females (Tagliapietra et al., 2020), suggesting that MCPyV may not be involved in SA.
It is important to point out that SA females showed lower ODs compared to females who had undergone VI (p < 0.05), thus indicating a reduced anti-MCPyV IgG antibody response in females experiencing SA. In addition, both SA and VI females exhibited lower rates and ODs compared to those determined in age-matched, non-pregnant HF (62.1%), which had been investigated in our previous study conducted on healthy individuals (Mazziotta et al., 2021b). These findings indicate not only that serum IgG antibody response to MCPyV may decline in pregnancy but also that this decline is de facto more pronounced in females experiencing SA.
Notably, to the best of our knowledge, this is the first study reporting on MCPyV serology in SA. Only one previous study has reported on MCPyV serology in pregnancy, describing a rate of nearly 46% in a cohort of pregnant females (Sadeghi et al., 2010), a proportion similar to that obtained herein in our study/control groups. Regarding the healthy population, previous works have reported that a considerable fraction of adults, both males and females, is exposed to an asymptomatic MCPyV infection, with seroprevalence rates ranging from 60 to 80%, according to the study under consideration (Carter et al., 2009; Kean et al., 2009; Pastrana et al., 2009; Faust et al., 2011; Tolstov et al., 2011; Touzé et al., 2011; Viscidi et al., 2011; Coursaget et al., 2013; Van Der Meijden et al., 2013; Šroller et al., 2014; Zhang et al., 2014; Vahabpour et al., 2016; Kamminga et al., 2018; Csoboz et al., 2020; Zhou et al., 2020; Mazziotta et al., 2021a,b). Our immunological findings, alongside those previously reported, cumulatively indicate that a relatively reduced fraction of pregnant females carries anti-MCPyV IgGs compared to the healthy population, while SA females have a lower IgG antibody response to MCPyV.
The similar rate of immunological decrease determined in SA and VI females compared to non-pregnant females might be accounted for by the well-known immune adaptation/modulation process occurring in pregnancy (Longman and Johnson, 2007). Pregnant females present a unique state of immunity, as they develop a tolerance for the semi-allogeneic embryo/fetus, compared to non-pregnant females (Giakoumelou et al., 2016). In other words, despite recognizing the paternal antigens expressed by the embryo/fetus, the maternal immune system undergoes an adaptation to hamper her/his rejection (Mor and Cardenas, 2010). This adaptation is mainly, but not only, due to a decrease in the production and/or function of (i) lymphocyte T and natural killer cells, involved in the adaptive immune response (Longman and Johnson, 2007); and (ii) B cells, deputies in the production of antibodies (Medina et al., 1993). A transient immune modulation during pregnancy can therefore affect how SA and VI females respond to viral agents, making them more susceptible to viral infections (Giakoumelou et al., 2016), probably including MCPyV infection. The diminished serum IgG antibody response to MCPyV in both SA and VI females compared to non-pregnant females might be a reflection of the transient modulation in the immune function being experienced during pregnancy. The lower IgG antibody response to MCPyV determined in SA females might be linked to their more increased susceptibility to MCPyV infection. However, the relationship between this possible immunologic dysregulation and SA is unknown.
Conditions of physiological immune impairment in the host can favor a minor response to MCPyV as a result of antiviral surveillance decline (Ma and Brewer, 2014). This phenomenon can lead to an increase in MCPyV replication levels/activity or reinfection, ultimately leading to MCC (Ma and Brewer, 2014). A higher MCC rate has been determined in patients with immunocompromising conditions such as oncologic patients (Yu and Dasanu, 2016; Zheng et al., 2019) as well as in patients/individuals being pharmacologically treated for organ transplantation and/or autoimmune diseases (Lanoy and Engels, 2010; Boldorini et al., 2014; Clarke et al., 2015; Rotondo et al., 2017; Pietropaolo et al., 2020). Notably, MCC cases have also been identified in pregnant females (Chao et al., 1990; Paterson et al., 2003; Kukko et al., 2008; Tyler, 2020), thus supporting the view that a dysregulation in transient immune modulation during pregnancy can, at least in certain circumstances, favor an increase in MCPyV activity. It should be recalled that, despite being oncogenic, MCPyV is ubiquitous in humans, while establishing a lifelong and asymptomatic infection in healthy immunocompetent individuals. The decreased IgG antibody response to MCPyV might account for greater tolerance to viral infections experienced during pregnancy, which in turn might potentially lead to an increase in MCPyV replication levels/activity in SA females, as reported for other viral agents (Giakoumelou et al., 2016). Indeed, previous findings indicate that maternal immune tolerance impairment may result in SA or preeclampsia (Wang and Li, 2020).
How a diminished antibody response to MCPyV in SA females might negatively impact embryo development remains to be determined. Following an increase in MCPyV multiplication, this PyV might reach the embryo/fetus from the mother via vertical transmission, through the mother’s blood, as demonstrated for other viruses (Xu et al., 2015). As immune cells can allow viral crossing through placental barriers (Robbins and Bakardjiev, 2012; Cordeiro et al., 2015), a similar mechanism for MCPyV cannot be excluded. Notably, MCPyV DNA has been detected in white cells from pregnant females (Tagliapietra et al., 2020). An ascending transmission from the maternal reproductive system might be an alternative mechanism, as the uterine cervix may be prone to MCPyV infection (Imajoh et al., 2012). Likely, pregnancy may be affected following a maternal response to an MCPyV activity increase, even in the absence of a viral transmission, as found for other viruses (Mor and Cardenas, 2010; Racicot and Mor, 2017). Viral infections nearby placental barriers can trigger a mild inflammatory response represented by an increased cytokine production (Koga et al., 2009; Huang et al., 2014), promoting, in turn, an inflammatory response in the embryo/fetus, which can lead to pregnancy complications/abortion. A potential MCPyV activity increase may determine a mother’s inflammatory response, ultimately affecting pregnancy. Excluding chorionic villi and PBMCs (Tagliapietra et al., 2020), an increase in MCPyV activity, if present, due to a reduced anti-MCPyV immunological response in SA females might occur in a tissue type, which is currently unknown. Notably, the reservoir cell type of MCPyV is still unclear (Liu et al., 2019). Evidently, due to the lack of molecular data, further studies should be performed.
It is important to underline that MCPyV activity in terms of DNA replication and gene expression was not investigated herein. Therefore, the hypothesis that MCPyV activity might increase following a reduced antibody response to MCPyV, possibly making SA and VI females more susceptible to MCPyV infection, remains to be verified. Further studies evaluating the presence of viral DNA/RNA/proteins in key embryo development tissues including cord blood, amniotic fluids, cervical tissues, and fetal membranes should be conducted, as performed for other viruses (Giakoumelou et al., 2016). Since the basic immune conditions of SA, VI, and non-pregnant females might be different, determining the total IgG fraction, alongside IgGs to MCPyV, might help to better understand how pregnant females respond to MCPyV infection. Moreover, the prospective monitoring of the immunological status of pregnant females throughout the different gestation phases might be considered as an alternative investigative approach. These molecular and immunological experiments can be part of further investigations.
To avoid confounding variables, we excluded herein females who were positive for (i) congenital/acquired immunodeficiency syndromes or who were receiving immunosuppressive therapies; (ii) known causes of SA, i.e., genetic factors and anatomic/hormonal complications; and (iii) HIV, HBV, HCV, and syphilis infections. SA and VI females were similar in age (p > 0.05); samples were collected within 12 h from the abortion, while females were at a gestational age within the first 12 weeks.
In conclusion, our indirect ELISA proved to be reliable in identifying circulating IgGs reacting to MCPyV VP mimotopes in females experiencing SA and who had undergone VI. For the first time, IgG antibodies against oncogenic MCPyV were found in sera from SA females. Our results indicate that a reduced fraction of SA and VI females carries IgGs to MCPyV compared to healthy, non-pregnant females, while SA females presented a more pronounced decrease in IgG antibody response to MCPyV. Although yet to be determined, females experiencing abortive events might potentially present an increase in MCPyV multiplication events as a result of the immunological decline against MCPyV. Future investigations are needed to elucidate the relationship between MCPyV infection and SA.
Data Availability Statement
The raw data supporting the conclusions of this article will be made available by the authors, without undue reservation.
Ethics Statement
The studies involving human participants were reviewed and approved by the Ethical Committee of Ferrara, Italy, authorized the study (ID: 151078). The patients/participants provided their written informed consent to participate in this study.
Author Contributions
JR: conceptualization. CM and JR: methodology. CM, JR, and GP: software. CM: formal analysis, resources, data curation, statistical analysis, and visualization. CM and GP: investigation. CM, MT, and JR: writing—original draft preparation. MT and FM: writing—review and editing. JR and MT: supervision. JR, MT, and FM: funding acquisition. All authors have read and agreed to the published version of the manuscript.
Funding
This work was supported, in part, by grants MFAG 21956 (to JR), IG 21617 (to MT) from the Associazione Italiana per la Ricerca sul Cancro (AIRC), Milan, Italy, and University of Ferrara, FAR grants 2021 (to FM and MT).
Conflict of Interest
The authors declare that the research was conducted in the absence of any commercial or financial relationships that could be construed as a potential conflict of interest.
Publisher’s Note
All claims expressed in this article are solely those of the authors and do not necessarily represent those of their affiliated organizations, or those of the publisher, the editors and the reviewers. Any product that may be evaluated in this article, or claim that may be made by its manufacturer, is not guaranteed or endorsed by the publisher.
Acknowledgments
We would like to thank Georgia Emma Gili for revising the English text of the manuscript.
References
American College of Obstetricians and Gynecologists Committee on Practice Bulletins—Gynecology (2018). ACOG Practice bulletin no. 200: early pregnancy loss. Obstet. Gynecol. 132, E197–E207. doi: 10.1097/AOG.0000000000002899
Auriti, C., De Rose, D., Santisi, A., Martini, L., Piersigilli, F., Bersani, I., et al. (2021). Pregnancy and viral infections: mechanisms of fetal damage, diagnosis and prevention of neonatal adverse outcomes from cytomegalovirus to SARS-CoV-2 and Zika virus. Biochim. Biophys. Acta Mol. Basis Dis. 1867:166198.
Azevedo, R. S. S., Araujo, M. T., Oliveira, C. S., Filho, A. J. M., Nunes, B. T. D., Henriques, D. F., et al. (2018). Zika virus epidemic in Brazil. II. Post-mortem analyses of neonates with microcephaly, stillbirths, and miscarriage. J. Clin. Med. 7:496. doi: 10.3390/jcm7120496
Boldorini, R., Allegrini, S., Tognon, M., Miglio, U., Rossi, D., Pawlita, M., et al. (2014). Merkel cell carcinoma arising in inguinal lymph node in a patient with von Willebrand disease after multiple blood transfusions. J. Clin. Virol. 60, 73–75. doi: 10.1016/j.jcv.2014.02.007
Burton, G. J., and Watson, A. L. (1997). The structure of the human placenta: implications for initiating and defending against virus infections. Rev. Med. Virol. 7, 219–228.
Carabali, M., Austin, N., King, N. B., and Kaufman, J. S. (2018). The Zika epidemic and abortion in Latin America: a scoping review. Glob. Heal. Res. Policy 3:15. doi: 10.1186/s41256-018-0069-8
Carter, J. J., Paulson, K. G., Wipf, G. C., Miranda, D., Madeleine, M. M., Johnson, L. G., et al. (2009). Association of merkel cell polyomavirus-specific antibodies with merkel cell carcinoma. J. Natl. Cancer Inst. 101, 1510–1522. doi: 10.1093/jnci/djp332
Chao, T., Park, J., Rhee, H., and Greager, J. (1990). Merkel cell tumor of the back detected during pregnancy. Plast. Reconstr. Surg. 86, 347–351. doi: 10.1097/00006534-199008000-00028
Clarke, C. A., Robbins, H. A., Tatalovich, Z., Lynch, C. F., Pawlish, K. S., Finch, J. L., et al. (2015). Risk of Merkel cell carcinoma after solid organ transplantation. J. Natl. Cancer Inst. 107:dju382. doi: 10.1093/jnci/dju382
Classen, D. C., Morningstar, J. M., and Shanley, J. D. (1987). Detection of antibody to murine cytomegalovirus by enzyme-linked immunosorbent and indirect immunofluorescence assays. J. Clin. Microbiol. 25, 600–604. doi: 10.1128/jcm.25.4.600-604.1987
Collier, S. A., Rasmussen, S. A., Feldkamp, M. L., and Honein, M. A. (2009). Prevalence of self-reported infection during pregnancy among control mothers in the National Birth Defects Prevention Study. Birth Defects Res. Part A Clin. Mol. Teratol. 85, 193–201. doi: 10.1002/BDRA.20540
Contini, C., Rotondo, J. C., Magagnoli, F., Maritati, M., Seraceni, S., Graziano, A., et al. (2018). Investigation on silent bacterial infections in specimens from pregnant women affected by spontaneous miscarriage. J. Cell. Physiol. 234, 100–107. doi: 10.1002/jcp.26952
Cordeiro, C. N., Tsimis, M., and Burd, I. (2015). Infections and brain development. Obstet. Gynecol. Surv. 70, 644–655. doi: 10.1097/OGX.0000000000000236
Coursaget, P., Samimi, M., Nicol, J. T. J., Gardair, C., and Touzé, A. (2013). Human Merkel cell polyomavirus: virological background and clinical implications. APMIS 121, 755–769. doi: 10.1111/apm.12122
Csoboz, B., Rasheed, K., Sveinbjørnsson, B., and Moens, U. (2020). Merkel cell polyomavirus and non-Merkel cell carcinomas: guilty or circumstantial evidence? APMIS 128, 104–120. doi: 10.1111/apm.13019
de Freitas, L. B., Pereira, C. C., Merçon-de-Vargas, P. R., and Spano, L. C. (2018). Human papillomavirus in foetal and maternal tissues from miscarriage cases. J. Obstet. Gynaecol. (Lahore) 38, 1083–1087. doi: 10.1080/01443615.2018.1454408
De La Rochebrochard, E., and Thonneau, P. (2002). Paternal age and maternal age are risk factors for miscarriage; results of a multicentre European study. Hum. Reprod. 17, 1649–1656. doi: 10.1093/humrep/17.6.1649
Dean, D. D., Agarwal, S., and Tripathi, P. (2018). Connecting links between genetic factors defining ovarian reserve and recurrent miscarriages. J. Assist. Reprod. Genet. 35, 2121–2128. doi: 10.1007/s10815-018-1305-3
Decaprio, J. A. (2021). Molecular Pathogenesis of Merkel Cell Carcinoma. Annu. Rev. Pathol. Mech. Dis. 16, 69–91. doi: 10.1146/annurev-pathmechdis-012419-032817
Del Marmol, V., and Lebbé, C. (2019). New perspectives in Merkel cell carcinoma. Curr. Opin. Oncol. 31, 72–83. doi: 10.1097/CCO.0000000000000508
Donders, G. G., Van Bulck, B., Caudron, J., Londers, L., Vereecken, A., and Spitz, B. (2000). Relationship of bacterial vaginosis and mycoplasmas to the risk of spontaneous abortion. Am. J. Obstet. Gynecol. 183, 431–437. doi: 10.1067/mob.2000.105738
Eiben, B., Bartels, I., Bahr-Porsch, S., Borgmann, S., Gatz, G., Gellert, G., et al. (1990). Cytogenetic analysis of 750 spontaneous abortions with the direct-preparation method of chorionic villi and its implications for studying genetic causes of pregnancy wastage. Am. J. Hum. Genet. 47, 656–663.
Faust, H., Pastrana, D. V., Buck, C. B., Dillner, J., and Ekström, J. (2011). Antibodies to Merkel cell polyomavirus correlate to presence of viral DNA in the skin. J. Infect. Dis. 203, 1096–1100. doi: 10.1093/infdis/jiq173
Fisher, S., Genbacev, O., Maidji, E., and Pereira, L. (2000). Human cytomegalovirus infection of placental cytotrophoblasts in vitro and in utero: implications for transmission and pathogenesis. J. Virol. 74, 6808–6820. doi: 10.1128/jvi.74.15.6808-6820.2000
Fukuta, K., Yoneda, S., Yoneda, N., Shiozaki, A., Nakashima, A., Minamisaka, T., et al. (2020). Risk factors for spontaneous miscarriage above 12 weeks or premature delivery in patients undergoing cervical polypectomy during pregnancy. BMC Pregnancy Childbirth 20:27. doi: 10.1186/s12884-019-2710-z
Giakoumelou, S., Wheelhouse, N., Cuschieri, K., Entrican, G., Howie, S. E. M., and Horne, A. W. (2016). The role of infection in miscarriage. Hum. Reprod. Update 22, 116–133. doi: 10.1093/humupd/dmv041
Hashida, Y., Kamioka, M., Tanaka, M., Hosokawa, S., Murakami, M., Nakajima, K., et al. (2016). Ecology of merkel cell polyomavirus in healthy skin among individuals in an asian cohort. J. Infect. Dis. 213, 1708–1716. doi: 10.1093/infdis/jiw040
Hertz-Picciotto, I., and Samuels, S. (1988). Incidence of early loss of pregnancy. N. Engl. J. Med. 319, 1483–1484. doi: 10.1056/NEJM198812013192214
Huang, Q. T., Chen, J. H., Zhong, M., Xu, Y. Y., Cai, C. X., Wei, S. S., et al. (2014). The risk of placental abruption and placenta previa in pregnant women with chronic hepatitis B viral infection: a systematic review and meta-analysis. Placenta 35, 539–545. doi: 10.1016/J.PLACENTA.2014.05.007
Imajoh, M., Hashida, Y., Nemoto, Y., Oguri, H., Maeda, N., Furihata, M., et al. (2012). Detection of Merkel cell polyomavirus in cervical squamous cell carcinomas and adenocarcinomas from Japanese patients. Virol. J. 91, 1–9. doi: 10.1186/1743-422X-9-154
Jeve, Y. B., and Davies, W. (2014). Evidence-based management of recurrent miscarriages. J. Hum. Reprod. Sci. 7, 159–169. doi: 10.4103/0974-1208.142475
Jin, H. T., Park, S. J., Choi, E. K., and Kim, Y. S. (2019). The frequency of Merkel cell polyomavirus in whole blood from immunocompetent and immunosuppressed patients with kidney disease and healthy donors. Microb. Pathog. 131, 75–80. doi: 10.1016/j.micpath.2019.03.020
Kamminga, S., Van Der Meijden, E., Feltkamp, M. C. W., and Zaaijer, H. L. (2018). Seroprevalence of fourteen human polyomaviruses determined in blood donors. PLoS One 13:e0206273. doi: 10.1371/journal.pone.0206273
Kean, J. M., Rao, S., Wang, M., and Garcea, R. L. (2009). Seroepidemiology of human polyomaviruses. PLoS Pathog. 5:e1000363. doi: 10.1371/journal.ppat.1000363
Koga, K., Cardenas, I., Aldo, P., Abrahams, V. M., Peng, B., Fill, S., et al. (2009). ORIGINAL ARTICLE: activation of TLR3 in the trophoblast is associated with preterm delivery. Am. J. Reprod. Immunol. 61, 196–212. doi: 10.1111/J.1600-0897.2008.00682.X
Kukko, H., Vuola, J., Suominen, S., and Koljonen, V. (2008). Merkel cell carcinoma in a young pregnant woman. J. Plast. Reconstr. Aesthetic Surg. 61, 1530–1533. doi: 10.1016/J.BJPS.2007.06.016
Lanoy, E., and Engels, E. A. (2010). Skin cancers associated with autoimmune conditions among elderly adults. Br. J. Cancer 103, 112–114. doi: 10.1038/sj.bjc.6605733
Lardeux, F., Torrico, G., and Aliaga, C. (2016). Calculation of the ELISA’s cut-off based on the change-point analysis method for detection of Trypanosoma cruzi infection in Bolivian dogs in the absence of controls. Mem. Inst. Oswaldo Cruz 111, 501–504. doi: 10.1590/0074-02760160119
Lashen, H., Fear, K., and Sturdee, D. W. (2004). Obesity is associated with increased risk of first trimester and recurrent miscarriage: matched case-control study. Hum. Reprod. 19, 1644–1646. doi: 10.1093/humrep/deh277
Liu, W., Krump, N. A., Buck, C. B., and You, J. (2019). Merkel cell polyomavirus infection and detection. J. Vis. Exp. 7:e58950. doi: 10.3791/58950
Liu, W., and You, J. (2020). Molecular mechanisms of Merkel Cell polyomavirus transformation and replication. Annu. Rev. Virol. 7, 289–307. doi: 10.1146/annurev-virology-011720-121757
Longman, R. E., and Johnson, T. R. B. (2007). Viral respiratory disease in pregnancy. Curr. Opin. Obstet. Gynecol. 19, 120–125. doi: 10.1097/GCO.0B013E328028FDC7
Ma, J., and Brewer, J. D. (2014). Merkel cell carcinoma in immunosuppressed patients. Cancers (Basel) 6, 1328–1350. doi: 10.3390/CANCERS6031328
Mazziotta, C., Lanzillotti, C., Govoni, M., Pellielo, G., Mazoni, E., Tognon, M. G., et al. (2021a). Decreased IgG antibody response to viral protein mimotopes of oncogenic Merkel cell polyomavirus in sera from healthy elderly subjects. Front. Immunol. 12:738486.
Mazziotta, C., Lanzillotti, C., Torreggiani, E., Oton-Gonzalez, L., Iaquinta, M. R., Mazzoni, E., et al. (2021b). Serum antibodies against the oncogenic Merkel Cell Polyomavirus detected by an innovative immunological assay with mimotopes in healthy subjects. Front. Immunol. 12:676627. doi: 10.3389/fimmu.2021.676627
Mazzoni, E., Pellegrinelli, E., Mazziotta, C., Lanzillotti, C., Rotondo, J. C., Bononi, I., et al. (2020). Mother-to-child transmission of oncogenic polyomaviruses BKPyV, JCPyV and SV40. J Infect 163, 91–98. doi: 10.1016/j.jinf.2020.02.006
Medina, K. L., Smithson, G., and Kincade, P. W. (1993). Suppression of B lymphopoiesis during normal pregnancy. J. Exp. Med. 178, 1507–1515. doi: 10.1084/JEM.178.5.1507
Meyer, P. (2001). Crowther J.R., The ELISA Guidebook, Methods in Molecular Biology, Vol. 149. Totowa, NJ: Humana Press, 421. doi: 10.1016/s0923-2508(00)01178-5
Miyaji, M., Deguchi, M., Tanimura, K., Sasagawa, Y., Morizane, M., Ebina, Y., et al. (2019). Clinical factors associated with pregnancy outcome in women with recurrent pregnancy loss. Gynecol. Endocrinol. 35, 913–918. doi: 10.1080/09513590.2019.1604657
Mor, G., and Cardenas, I. (2010). Review article: the immune system in pregnancy: a unique complexity. Am. J. Reprod. Immunol. 63, 425–433. doi: 10.1111/J.1600-0897.2010.00836.X
Nakagawa, S., and Schielzeth, H. (2010). Repeatability for Gaussian and non-Gaussian data: a practical guide for biologists. Biol. Rev. 85, 935–956. doi: 10.1111/j.1469-185X.2010.00141.x
Oton-Gonzalez, L., Rotondo, J. C., Cerritelli, L., Malagutti, N., Lanzillotti, C., Bononi, I., et al. (2021). Association between oncogenic human papillomavirus type 16 and Killian polyp. Infect. Agent. Cancer 16:3. doi: 10.1186/s13027-020-00342-3
Pastrana, D. V., Tolstov, Y. L., Becker, J. C., Moore, P. S., Chang, Y., and Buck, C. B. (2009). Quantitation of human seroresponsiveness to Merkel cell polyomavirus. PLoS Pathog. 5:e1000578. doi: 10.1371/journal.ppat.1000578
Paterson, C., Musselman, L., Chorneyko, K. S. R., and Rawlinson, J. (2003). Merkel cell (neuroendocrine) carcinoma of the anal canal: report of a case. Dis. Colon Rectum 46, 676–678. doi: 10.1007/S10350-004-6630-1
Pietropaolo, V., Prezioso, C., and Moens, U. (2020). merkel cell polyomavirus and merkel cell carcinoma. Cancers (Basel) 12:1774. doi: 10.3390/cancers12071774
Prezioso, C., Di Lella, F. M., Rodio, D. M., Bitossi, C., Trancassini, M., Mele, A., et al. (2019). Merkel cell polyomavirus dna detection in respiratory samples: study of a cohort of patients affected by cystic fibrosis. Viruses 11:571. doi: 10.3390/v11060571
Racicot, K., and Mor, G. (2017). Risks associated with viral infections during pregnancy. J. Clin. Invest. 127, 1591–1599. doi: 10.1172/JCI87490
Robbins, J. R., and Bakardjiev, A. I. (2012). Pathogens and the placental fortress. Curr. Opin. Microbiol. 15, 36–43. doi: 10.1016/J.MIB.2011.11.006
Rotondo, J. C., Bononi, I., Puozzo, A., Govoni, M., Foschi, V., Lanza, G., et al. (2017). Merkel cell carcinomas arising in autoimmune disease affected patients treated with biologic drugs including anti-TNF. Clin. Cancer Res. 23, 3929–3934. doi: 10.1158/1078-0432.CCR-16-2899
Rotondo, J. C., Bosi, S., Bazzan, E., Di Domenico, M., De Mattei, M., Selvatici, R., et al. (2012). Methylenetetrahydrofolate reductase gene promoter hypermethylation in semen samples of infertile couples correlates with recurrent spontaneous abortion. Hum. Reprod. 27, 3632–3638. doi: 10.1093/humrep/des319
Rotondo, J. C., Lanzillotti, C., Mazziotta, C., Tognon, M., and Martini, F. (2021a). Epigenetics of male infertility: the role of DNA methylation. Front. Cell Dev. Biol. 9:689624.
Rotondo, J. C., Martini, F., Maritati, M., Mazziotta, C., Di Mauro, G., Lanzillotti, C., et al. (2021b). SARS-CoV-2 infection: new molecular, phylogenetic, and pathogenetic insights. Efficacy of current vaccines and the potential risk of variants. Viruses 13:1687.
Rotondo, J. C., Oton-Gonzalez, L., Mazziotta, C., Lanzillotti, C., Iaquinta, M. R., Tognon, M., et al. (2020a). Simultaneous detection and viral DNA load quantification of different human papillomavirus types in clinical specimens by the high analytical droplet digital PCR method. Front. Microbiol. 11:591452. doi: 10.3389/fmicb.2020.591452
Rotondo, J. C., Oton-Gonzalez, L., Selvatici, R., Rizzo, P., Pavasini, R., Campo, G. C., et al. (2020b). SERPINA1 gene promoter is differentially methylated in peripheral blood mononuclear cells of pregnant women. Front. Cell Dev. Biol. 8:5505. doi: 10.3389/fcell.2020.550543
Rotondo, J. C., Selvatici, R., Di Domenico, M., Marci, R., Vesce, F., Tognon, M., et al. (2013). Methylation loss at H19 imprinted gene correlates with methylenetetrahydrofolate reductase gene promoter hypermethylation in semen samples from infertile males. Epigenetics 8, 990–997. doi: 10.4161/epi.25798
Sadeghi, M., Riipinen, A., Väisänen, E., Chen, T., Kantola, K., Surcel, H.-M., et al. (2010). Newly discovered KI, WU, and Merkel cell polyomaviruses: no evidence of mother-to-fetus transmission. Virol. J. 71, 1–5. doi: 10.1186/1743-422X-7-251
Saraswati, K., Phanichkrivalkosil, M., Day, N. P. J., and Blacksell, S. D. (2019). The validity of diagnostic cut-offs for commercial and in-house scrub typhus IgM and IgG ELISAs: a review of the evidence. PLoS Negl. Trop. Dis. 13:e0007158. doi: 10.1371/journal.pntd.0007158
Sayyadi-Dehno, Z., Seyed Khorrami, S. M., Ghavami, N., Ghotbi-Zadeh, F., Khushideh, M., Hosseini, M., et al. (2019). Molecular detection of adeno-associated virus DNA in cases of spontaneous and therapeutic abortion. Fetal Pediatr. Pathol. 38, 206–214. doi: 10.1080/15513815.2019.1576817
Sopori, M. (2002). Effects of cigarette smoke on the immune system. Nat. Rev. Immunol. 2, 372–377. doi: 10.1038/nri803
Srinivas, S. K., Ma, Y., Sammel, M. D., Chou, D., McGrath, C., Parry, S., et al. (2006). Placental inflammation and viral infection are implicated in second trimester pregnancy loss. Am. J. Obstet. Gynecol. 195, 797–802. doi: 10.1016/j.ajog.2006.05.049
Šroller, V., Hamšíková, E., Ludvíková, V., Vochozková, P., Kojzarová, M., Fraiberk, M., et al. (2014). Seroprevalence rates of BKV, JCV, and MCPyV polyomaviruses in the general czech republic population. J. Med. Virol. 86, 1560–1568. doi: 10.1002/jmv.23841
Suzumori, N., and Sugiura-Ogasawara, M. (2012). Genetic factors as a cause of miscarriage. Curr. Med. Chem. 17, 3431–3437. doi: 10.2174/092986710793176302
Tabachnick-Cherny, S., Pulliam, T., Church, C., Koelle, D. M., and Nghiem, P. (2020). Polyomavirus-driven Merkel cell carcinoma: prospects for therapeutic vaccine development. Mol. Carcinog. 59, 807–821. doi: 10.1002/mc.23190
Tagliapietra, A., Rotondo, J. C. C., Bononi, I., Mazzoni, E., Magagnoli, F., Maritati, M., et al. (2019). Footprints of BK and JC polyomaviruses in specimens from females affected by spontaneous abortion. Hum. Reprod. 34, 433–440. doi: 10.1093/humrep/dey375
Tagliapietra, A., Rotondo, J. C. J. C., Bononi, I., Mazzoni, E., Magagnoli, F., Gonzalez, L. O., et al. (2020). Droplet-digital PCR assay to detect Merkel cell polyomavirus sequences in chorionic villi from spontaneous abortion affected females. J. Cell. Physiol. 34, 1888–1894. doi: 10.1002/jcp.29213
Tognon, M., Tagliapietra, A., Magagnoli, F., Mazziotta, C., Oton-Gonzalez, L., Lanzillotti, C., et al. (2020). Investigation on spontaneous abortion and human papillomavirus infection. Vaccines 8:473. doi: 10.3390/vaccines8030473
Tolstov, Y. L., Knauer, A., Chen, J. G., Kensler, T. W., Kingsley, L. A., Moore, P. S., et al. (2011). Asymptomatic primary merkel cell polyomavirus infection among adults. Emerg. Infect. Dis. 17, 1371–1380. doi: 10.3201/eid1708.110079
Toth, B., Jeschke, U., Rogenhofer, N., Scholz, C., Würfel, W., Thaler, C. J., et al. (2010). Recurrent miscarriage: current concepts in diagnosis and treatment. J. Reprod. Immunol. 85, 25–32. doi: 10.1016/j.jri.2009.12.006
Touzé, A., Gaitan, J., Arnold, F., Cazal, R., Fleury, M. J., Combelas, N., et al. (2010). Generation of Merkel cell polyomavirus (MCV)-like particles and their application to detection of MCV antibodies. J. Clin. Microbiol. 48, 1767–1770. doi: 10.1128/JCM.01691-09
Touzé, A., Le Bidre, E., Laude, H., Fleury, M. J. J., Cazal, R., Arnold, F., et al. (2011). High levels of antibodies against merkel cell polyomavirus identify a subset of patients with merkel cell carcinoma with better clinical outcome. J. Clin. Oncol. 29, 1612–1619. doi: 10.1200/JCO.2010.31.1704
Vahabpour, R., Aghasadeghi, M. R., Salehi-Vaziri, M., Mohajel, N., Keyvani, H., Nasimi, M., et al. (2016). Prevalence of Merkel cell polyomavirus in Tehran: an age-specific serological study. Iran. Red Crescent Med. J. 18, e26097. doi: 10.5812/ircmj.26097
Van Der Meijden, E., Bialasiewicz, S., Rockett, R. J., Tozer, S. J., Sloots, T. P., and Feltkamp, M. C. W. (2013). Different serologic behavior of MCPyV, TSPyV, HPyV6, HPyV7 and HPyV9 polyomaviruses found on the skin. PLoS One 8:e81078. doi: 10.1371/journal.pone.0081078
Viscidi, R. P., Rollison, D. E., Sondak, V. K., Silver, B., Messina, J. L., Giuliano, A. R., et al. (2011). Age-specific seroprevalence of merkel cell polyomavirus, BK virus, and JC virus. Clin. Vaccine Immunol. 18, 1737–1743. doi: 10.1128/CVI.05175-11
Wang, X., and Li, J. (2020). The mechanisms by which trophoblast-derived molecules induce maternal-fetal immune tolerance. Cell Mol. Immunol. 17, 1204–1207.
Witkin, A. E., Banerji, J., and Bullock, P. A. (2020). A model for the formation of the duplicated enhancers found in polyomavirus regulatory regions. Virology 543, 27–33. doi: 10.1016/j.virol.2020.01.013
Xu, Y. Y., Liu, H. H., Zhong, Y. W., Liu, C., Wang, Y., Jia, L. L., et al. (2015). Peripheral blood mononuclear cell traffic plays a crucial role in mother-to-infant transmission of hepatitis B virus. Int. J. Biol. Sci. 11, 266–273. doi: 10.7150/ijbs.10813
Yu, K. K., and Dasanu, C. A. (2016). Rapidly fatal dissemination of Merkel cell carcinoma in a patient treated with alemtuzumab for chronic lymphocytic leukemia. Conn. Med. 80, 353–358.
Zhang, C., Liu, F., He, Z., Deng, Q., Pan, Y., Liu, Y., et al. (2014). Seroprevalence of Merkel cell polyomavirus in the general rural population of Anyang. China. PLoS One 9:e106430. doi: 10.1371/journal.pone.0106430
Zheng, G., Chattopadhyay, S., Sud, A., Sundquist, K., Sundquist, J., Försti, A., et al. (2019). Second primary cancers in patients with acute lymphoblastic, chronic lymphocytic and hairy cell leukaemia. Br. J. Haematol. 185, 232–239. doi: 10.1111/bjh.15777
Keywords: spontaneous abortion, miscarriage, pregnancy, viral infection, Merkel cell polyomavirus, antibodies, IgG, immune system
Citation: Mazziotta C, Pellielo G, Tognon M, Martini F and Rotondo JC (2021) Significantly Low Levels of IgG Antibodies Against Oncogenic Merkel Cell Polyomavirus in Sera From Females Affected by Spontaneous Abortion. Front. Microbiol. 12:789991. doi: 10.3389/fmicb.2021.789991
Received: 05 October 2021; Accepted: 27 October 2021;
Published: 14 December 2021.
Edited by:
Chunfu Zheng, University of Calgary, CanadaReviewed by:
Yi-Quan Wu, National Cancer Institute (NCI), United StatesYan Yan, Wuxi No. 5 People’s Hospital, China
Copyright © 2021 Mazziotta, Pellielo, Tognon, Martini and Rotondo. This is an open-access article distributed under the terms of the Creative Commons Attribution License (CC BY). The use, distribution or reproduction in other forums is permitted, provided the original author(s) and the copyright owner(s) are credited and that the original publication in this journal is cited, in accordance with accepted academic practice. No use, distribution or reproduction is permitted which does not comply with these terms.
*Correspondence: Fernanda Martini, bXJmQHVuaWZlLml0; orcid.org/0000-0001-9137-0805; John Charles Rotondo, cnRuam5jQHVuaWZlLml0; orcid.org/0000-0001-5179-1525