- 1Department of Oceanography, Texas A&M University, College Station, TX, United States
- 2Department of Atmospheric Sciences, Texas A&M University, College Station, TX, United States
Ocean aerobiology is defined here as the study of biological particles of marine origin, including living organisms, present in the atmosphere and their role in ecological, biogeochemical, and climate processes. Hundreds of trillions of microorganisms are exchanged between ocean and atmosphere daily. Within a few days, tropospheric transport potentially disperses microorganisms over continents and between oceans. There is a need to better identify and quantify marine aerobiota, characterize the time spans and distances of marine microorganisms’ atmospheric transport, and determine whether microorganisms acclimate to atmospheric conditions and remain viable, or even grow. Exploring the atmosphere as a microbial habitat is fundamental for understanding the consequences of dispersal and will expand our knowledge of biodiversity, biogeography, and ecosystem connectivity across different marine environments. Marine organic matter is chemically transformed in the atmosphere, including remineralization back to CO2. The magnitude of these transformations is insignificant in the context of the annual marine carbon cycle, but may be a significant sink for marine recalcitrant organic matter over long (∼104 years) timescales. In addition, organic matter in sea spray aerosol plays a significant role in the Earth’s radiative budget by scattering solar radiation, and indirectly by affecting cloud properties. Marine organic matter is generally a poor source of cloud condensation nuclei (CCN), but a significant source of ice nucleating particles (INPs), affecting the formation of mixed-phase and ice clouds. This review will show that marine biogenic aerosol plays an impactful, but poorly constrained, role in marine ecosystems, biogeochemical processes, and the Earth’s climate system. Further work is needed to characterize the connectivity and feedbacks between the atmosphere and ocean ecosystems in order to integrate this complexity into Earth System models, facilitating future climate and biogeochemical predictions.
Introduction
The origins of ocean aerobiology can be traced to Darwin (1846), who reported that ships in the Atlantic Ocean often became coated in a layer of fine dust, thought to have originated in Africa. Darwin collaborated with Christian Gottfried Ehrenberg (a pioneer of microscopy) to study the content of dust he himself collected on the HMS Beagle in 1833, and samples collected by sailors on other ships. Ehrenberg identified 67 taxa of “infusoria” (protists), including two marine taxa (Darwin, 1846). Despite early scientific interest in aerobiology, recent estimates of the abundance and biomass of organisms on Earth have ignored the atmosphere (Whitman et al., 1998; Bar-On et al., 2018) or considered the atmosphere to be a minor component (Flemming and Wuertz, 2019). Certainly, the biomass and abundance of microorganisms in the atmosphere (5 × 1022 prokaryotes; Flemming and Wuertz, 2019) is low compared with other environments (1 × 1029 prokaryotes in the ocean; Flemming and Wuertz, 2019), but low abundance does not preclude significance.
Airborne marine microorganisms affect the distribution of specific taxa in the ocean (Womack et al., 2010), ecosystem structure, and genetic exchange between ecosystems. Marine microorganisms are extremely diverse, as exemplified by their range of shapes and sizes (Figure 1). Size plays a major role in determining the deposition velocity of airborne microorganisms (Figure 1). Deposition velocity varies over five orders of magnitude for typical marine microorganisms, with lows of 9 × 10–7 m s–1 for viruses and higher values of 5 × 10–2 m s–1 for large eukaryotes (Figure 1). Therefore, a marine microorganism’s size strongly influences potential distance transported and residence time in the atmosphere (Figure 2). Atmospheric transport potentially carries viable marine microorganisms between locations that are inaccessible via surface currents due to geographical barriers and relatively long timescales (Figures 2, 3). For example, a parcel of water in the Atlantic can take more than 9 years to travel to the Pacific by surface currents (Jönsson and Watson, 2016), whereas atmospheric dispersal between Atlantic and Pacific occurs over a few days (Figure 3). The significance of aerial transport of marine microorganisms depends on three factors: (1) the fluxes of organisms between ocean and atmosphere; (2) how far marine microorganisms are transported in the atmosphere; and, (3) what proportion of marine microorganisms are viable after deposition in the ocean.
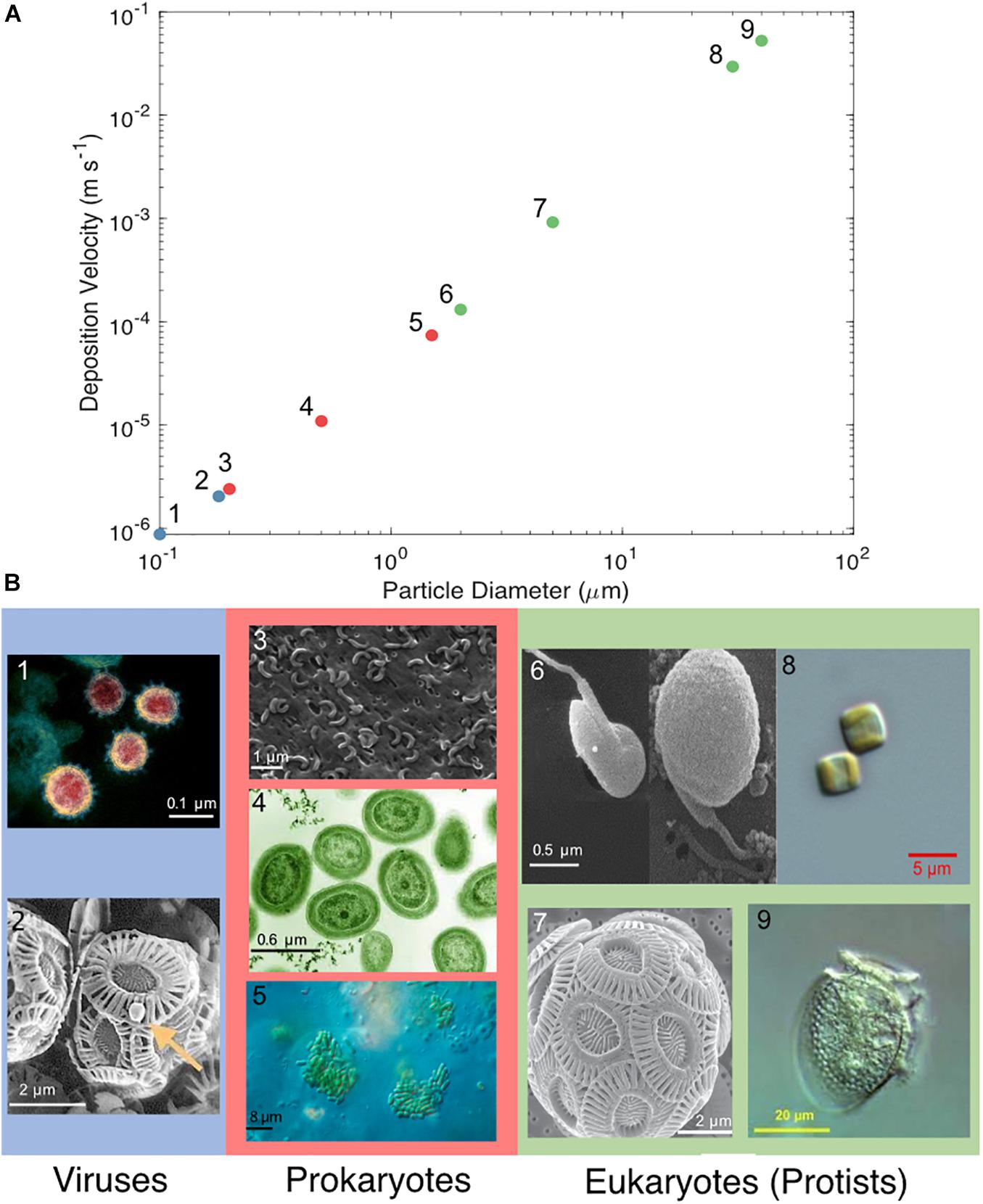
Figure 1. (A) Deposition velocity of varying cell diameters of viruses (blue), prokaryotes (red), and eukaryotes (green). (B) Microscopy images of microorganisms representative of the particle diameter chosen: (1) SARS-CoV-2, (2) Emiliania huxleyi virus, (3) SAR11, (4) Prochlorococcus, (5) Synechococcus, (6) Micromonas pusilla, (7) Emiliania huxleyi, (8) Thalassiosira sp., and (9) Dinophysis acuminata. (1–7) Approximate scale bars were added. The microscopy images in (B) were reproduced under the Creative Commons Attribution International licenses and were captured by (1) NIAID’s Rocky Mountain Laboratories in Hamilton, Montana (2) Wikimedia commons (3) Steindler et al. (2011) (4) Luke Thompson at the Sallie Chisholm Lab and Nikki Watson at Whitehead, MIT (5) Proyecto Agua at Biodiversidad virtual, Cantabria, Spain (6) Manton and Parke (1960) and courtesy of Nordic Microalgae and Aquatic Protozoa (Karlson et al., 2020) (7) Alison R. Taylor, University of North Carolina Wilmington Microscopy Facility (8) Moore et al. (2017) and (9) Plankton Net, Alfred Wegener Institute, Helmholtz Centre for Polar and Marine Research.
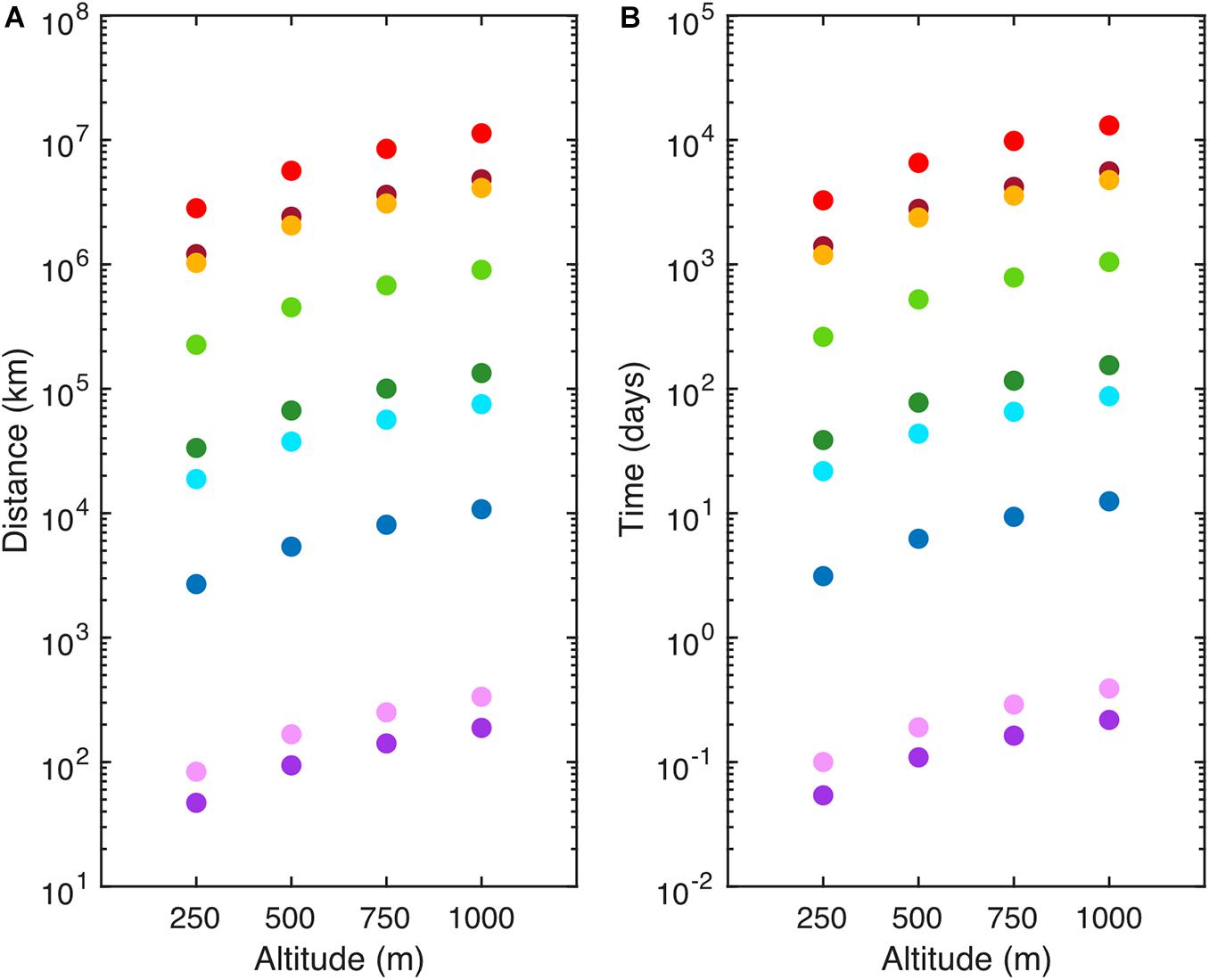
Figure 2. Atmospheric transportation of representative marine microorganisms and Coronavirus (SARS-CoV-2) (A) Potential distance traveled (km) and (B) residence time (days) of SARS-CoV-2 (red), Emiliania huxleyi virus (maroon), SAR11 (orange), Prochlorococcus (light green), Synechococcus (dark green), Micromonas pusilla (light blue), Emiliania huxleyi (dark blue), Thalassiosira weissflogii (light purple), and Dinophysis acuminata (dark purple). Values were determined using Stoke’s Law for aerosol particles released at specific altitudes (250–1,000 m) at the average tropospheric temperature (15°C) in a homogeneous atmosphere. Residence time was calculated using a representative average wind speed of 10 m s–1. Distance traveled and residence time should be considered the upper limit as these estimates do not account for loss processes such as wet deposition.
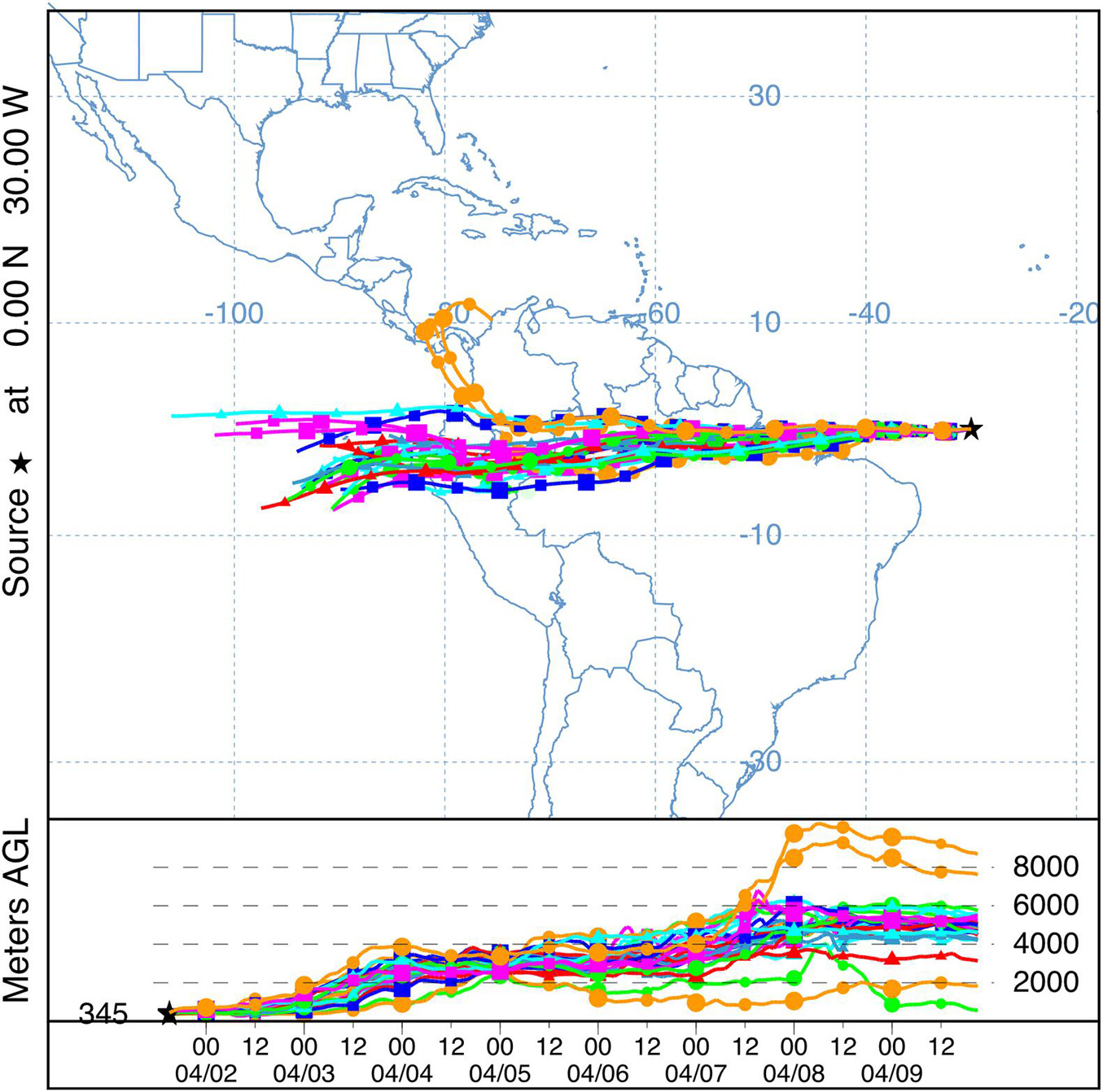
Figure 3. An airmass traveling from the Atlantic to the Pacific was tracked using NOAA Air Resources Laboratory HYSPLIT (Stein et al., 2015; Rolph et al., 2017) forward trajectory ensemble model with a starting point at 0°N, 30°W (black star) over 7 days (04/02/2020 –04/09/2020) with global forecast system (GFS) meteorology data. Each possible trajectory is represented with each point along the trajectory corresponding to a 12-h increment. The estimated mid-boundary layer height was added (345 m) at the starting point (black star) and the height (m) above ground level (AGL) is shown for each trajectory. Multiple colors enables individual trajectories to be visualized.
Air-sea exchange of microbial communities is dynamic, with hundreds of trillions of microorganisms emitted to and deposited from the atmosphere daily (Mayol et al., 2014). Mayol et al. (2017) estimated total emission fluxes of prokaryotes (1 × 103 to 2 × 106 cells m–2 day–1) and eukaryotes (1–2000 cells m–2 day–1) over the tropical and subtropical ocean. The estimated deposition fluxes were up to 6 × 106 prokaryotes m–2 day–1 and 2 × 107 eukaryotes m–2 day–1 over tropical and sub-tropical regions with nearby land masses, indicating terrestrial sources dominate the aerobiota over the ocean in many locations (Mayol et al., 2017). However, the fraction of terrestrial microbial species decreases with distance from land and between 33 and 68% of the microorganisms over the ocean are likely to be of marine origin (Mayol et al., 2017). A lack of spatial and temporal sampling density limits our understanding of atmospheric abundance and biodiversity. There is currently only one study of global airborne microbial communities, and it does not include pristine marine sampling sites (Tignat-Perrier et al., 2019).
This review will explore the composition and formation of biogenic marine aerosol. Secondly, we will review the significance of marine aerosol to ecosystem processes and structure, considering the transport and viability of marine microorganisms in the atmosphere, their biogeographical relevance, and potential to influence marine ecosystem processes and biogeochemistry. Finally, we will review the role of marine biogenic aerosols in weather and climate (DeMott et al., 2016; Brooks and Thornton, 2018; Croft et al., 2021), particularly the role of marine primary aerosol in the natural seeding of clouds (Wilbourn et al., 2020; Hendrickson et al., 2021; Zheng et al., 2021). We will synthesize existing knowledge and suggest future research directions to gain a better understanding of the sources, fate, and significance of marine organic aerosol.
Generation of Marine Aerosol
Primary marine aerosol are directly ejected into the atmosphere from the ocean surface (Quinn et al., 2014, 2015; Brooks and Thornton, 2018). Wave breaking causes entrainment of air bubbles in the underlying water, which scavenge organic matter as they rise to the surface and burst (Blanchard and Woodcock, 1957; Blanchard, 1963; Gantt and Meskhidze, 2013; Figure 4). Bubble bursting produces two types of primary sea spray aerosol (SSA), film and jet drops, both containing a mixture of sea salt and marine organics (Quinn et al., 2015; Wang et al., 2017). Film drops form due to the fragmentation of the film cap surrounding the bubble, and jet drops are produced when the film cap disintegrates, causing the collapse of the bubble cavity. Jet drop formation typically results in SSA that is supermicrometer in size and contains mainly sea salt and water-soluble organic matter (Wang et al., 2017), as well as larger microorganisms. Film drops are submicrometer (Veron, 2015), comprising hydrophobic organic matter (Wang et al., 2017) and likely small cellular microorganisms (e.g., bacteria) and cell fragments, as well as viral particles (Blanchard and Syzdek, 1982; Rastelli et al., 2017; Michaud et al., 2018). Organic matter is enriched in SSA compared to underlying water in biologically active areas of the ocean (O’Dowd et al., 2004; Gantt et al., 2011). Although significant seasonal variations have been observed (Sanchez et al., 2018; Quinn et al., 2019; Saliba et al., 2020), concentrations of marine aerosol are relatively low (generally less than 500 cm–3), compared with 1,000–2,000 cm–3 over continental landmasses (Wallace and Hobbs, 2006). Nevertheless, given the vast area for air-sea exchange (362 million km2, or 71%, of the Earth’s surface; Charette and Smith, 2010), marine primary aerosols play a significant role in Earth system processes.
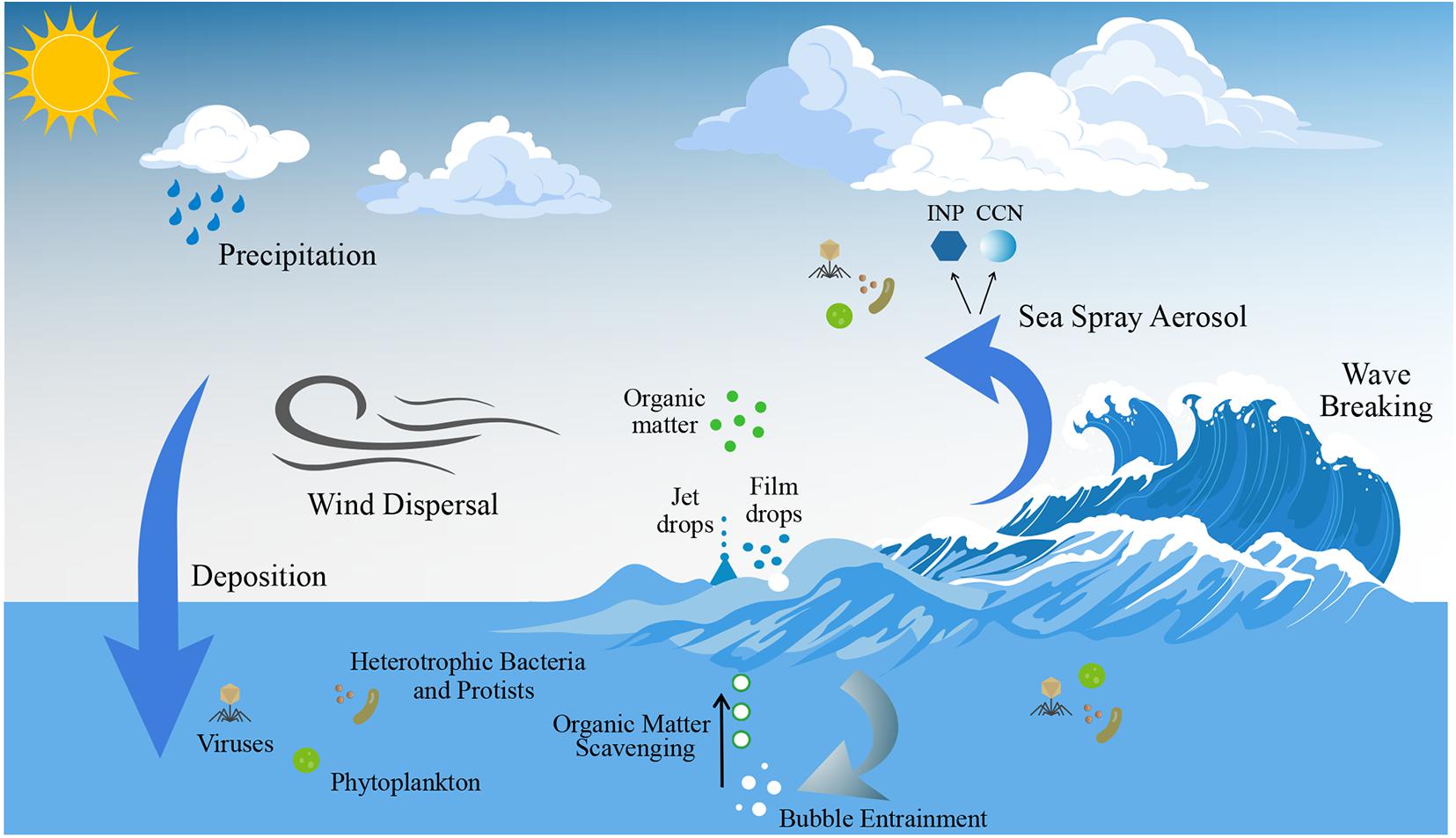
Figure 4. Physical processes associated with the generation of sea spray aerosol (SSA). Breaking waves entrain air, which results in bubbles bursting at the air-sea interface, lofting jet and film drops into the atmosphere, which form SSA. A subset of SSA catalyzes cloud formation by acting as cloud condensation nuclei (CCN) or ice nucleating particles (INPs). Primary marine aerosol formation via sea spray results in the transfer of marine microorganisms and organic matter to the atmosphere, which are transported over the ocean and deposited in a new location via processes such as wet deposition. The vector art used in this figure was downloaded from vecteezy.com.
At wind speeds up to 10–13 m s–1, the ocean is covered in gelatinous “skin” known as the sea surface microlayer (SML) (Salter et al., 2011; Wurl et al., 2011; Sabbaghzadeh et al., 2017). The SML is operationally defined as the top 1–1,000 μm of the ocean’s surface, consisting of a unique environment at the air-sea interface with distinct biological, chemical, and physical properties (Liss and Duce, 1997). Organic matter can become up to 1,000 times enriched in the SML compared to the underlying water (Liss and Duce, 1997) and include surface active proteins, lipids, and carbohydrates, as well as protists, bacteria, and viruses (Leck and Bigg, 2005; Orellana et al., 2011; Cunliffe et al., 2013; Engel et al., 2017). The SML contains a distinctive microbial community composition compared with the underlying water (Franklin et al., 2005; Joux et al., 2006; Cunliffe et al., 2009, 2011; Stolle et al., 2011). Although the exact controls determining the microbial community within the SML are not known, these microorganisms have been shown to withstand meteorological influences, such as increased turbulence and breaking waves (Stolle et al., 2011), and ultraviolet light (Ortega-Retuerta et al., 2009; Santos et al., 2011). Viruses have been overlooked, but viral lysis of cells may be a significant determinant of organic matter enrichment and microbial composition in the SML, and subsequently the composition of organic matter in primary marine aerosol (Rahlff, 2019). Microorganisms become embedded and enriched within a gel matrix of polymeric surfactants in the SML (Aller et al., 2005; Cunliffe and Murrell, 2009; Thornton et al., 2016), such as transparent exopolymer particles (TEP) (Alldredge et al., 1993) and Coomassie staining particles (CSP) (Long and Azam, 1996). This is consistent with the identification of polysaccharides in marine aerosol from the North Atlantic Ocean (Aller et al., 2017; Lawler et al., 2020). It has been presumed that the SML plays a dominant role in determining the composition of the organic matter is SSA. However, recent observations suggest that the direct contribution to SSA from the SML is minor compared with bubble plumes from the underlying water (Chingin et al., 2018; Frossard et al., 2019). Bubble plumes cause the SML to become displaced and therefore remove SML organics from the pathway of bubble bursting at the ocean’s surface (Long et al., 2014). Consequently, the concentration of organic matter in rising bubbles from the subsurface is proportional to the concentration of organic matter observed in the atmosphere via bubble bursting (Tseng et al., 1992).
While primary aerosol are the focus of this review, the significance of secondary aerosol that form in the atmosphere from gaseous precursors must be mentioned for a complete overview of marine biogenic aerosol. Secondary aerosols are produced via the oxidation of volatile organic compounds (VOCs) emitted by phytoplankton and bacteria (Halsey et al., 2017; Davie-Martin et al., 2020; Fox et al., 2020; Moore et al., 2020; Croft et al., 2021; Zheng et al., 2021), as well as photochemical reactions in the SML (Bernard et al., 2016; Brüggemann et al., 2018). Marine VOCs include acetaldehyde, acetone, acetonitrile, dimethyl sulfide (DMS), isoprene, methanethiol, methanol isoprene and halocarbons (Shaw et al., 2003; Dixon et al., 2013; Liu et al., 2013a, b; Halsey et al., 2017; Davie-Martin et al., 2020). DMS is a source of secondary aerosol in the form of non-sea salt sulfates, which is proposed as a significant source of cloud condensation nuclei (CCN) (Charlson et al., 1987; Gantt and Meskhidze, 2013; Quinn et al., 2019). New particle formation from the oxidation of VOCs in the upper marine boundary (Croft et al., 2021; Zheng et al., 2021) is significant, though poorly constrained.
Our understanding of links between seawater properties and overlying aerosol are tenuous (Saliba et al., 2019) due to the range of timescales and complexity of processes occurring in both the ocean and atmosphere. In the ocean, properties such as sea surface temperature, salinity, and surfactant concentration affect the rise time of bubbles and the bubble-burst processes (Fuentes et al., 2010; Forestieri et al., 2018; Saliba et al., 2019). Biological processes affect the composition and concentration of the organic matter available for aerosolization (O’Dowd et al., 2004, 2015; Fuentes et al., 2010; Rinaldi et al., 2013). Once in atmosphere, transport, mixing, and photochemical processing affect the composition of the aerosol (Saliba et al., 2019). The influence of wind speed on the formation and processing of aerosols remains one of the major challenges (Saliba et al., 2019, 2020). Supplementary Tables 1, 2 summarizes global emission estimates and measured concentrations of both organic carbon and sea salt emitted as SSA.
Organic Matter Contributions to Primary Marine Aerosol
Nascent SSA has been linked to short-lived, labile, forms of DOM using sea surface chlorophyll-a concentrations as an indicator of phytoplankton primary production and ecosystem activity (O’Dowd et al., 2004; Fuentes et al., 2010; Rinaldi et al., 2013). Other studies attribute DOM in SSA to older, recalcitrant sources, indicating that the enrichment of SSA with DOM is uncoupled from phytoplankton growth on seasonal and shorter timescales (Bates et al., 2012, 2020; Quinn et al., 2014; Kieber et al., 2016; Beaupré et al., 2019). Because the lifetime of recalcitrant DOM is much longer than the average mixing time of the ocean (Hansell et al., 2012), recalcitrant DOM is well mixed, forming a relatively uniform aerosol source. Understanding the chemical composition and origin of organic matter in SSA is key for incorporating SSA into climate models. SSA dominated by recalcitrant organic matter could be represented by a series of constant and global parameters, whereas a dynamic pool of organic matter in SSA will require complex modeling accounting for ecosystem processes and continuous environmental change, such as the seasons.
While the DOM inventory of the ocean (662 Pg C; Hansell et al., 2009) is dominated by recalcitrant organic matter (>612 Pg C; Hansell, 2013), the major source of labile organic matter is photosynthetic productivity, which is generally associated with sunlit surface waters. Therefore, it is likely that nascent SSA generally contains a complex mixture of DOM from labile to ultra-recalcitrant. Chemical characterization of samples from the North Atlantic by Lawler et al. (2020) supports this hypothesis. Lawler et al. (2020) concluded that there was a seasonal signal associated with labile polysaccharides and a relatively constant pool of recalcitrant organic matter associated with alcohol groups. Strictly speaking, the thermally stable (i.e., refractory) DOM identified by Lawler et al. (2020) is not necessarily equivalent to the recalcitrant pool defined by Hansell (2013). Current hypotheses explaining the stability of recalcitrant DOM are based on unavailability to microorganisms rather than thermal stability (Hansell, 2013).
The major classes of organic compounds in SSA reflects the composition of living organisms and non-living organic matter processed through the microbial food web (Table 1). Processes such as bubble bursting are selective and fractionate DOM across the air-sea interface according to chemical properties, such as surface tension, solubility, and interactions with ions (Cochran et al., 2017). Consequently, the organic composition of SSA is not identical to the available pool of organic matter in the underlying water (Brooks and Thornton, 2018; Table 1). Many compounds are significantly enriched in SSA concentration compared to the underlying seawater (Table 1). Chemical components of SSA should be explored for biomarkers to link the composition of SSA to specific organisms or biogeochemical processes in the originating waters. Chromophoric and fluorescent DOM (Yamashita and Tanoue, 2008), and some proteins (Aluwihare et al., 2005; Table 2), have the potential to be used as biomarkers for recalcitrant DOM. Monosaccharides are present in primary marine aerosol (Russell et al., 2010; Frossard et al., 2014; Zeppenfeld et al., 2021) and could serve as a biomarker for recent biological activity as they are labile (Table 2). Labile organic matter also includes mono- and dicarboxylic acids, fatty acids, lipids, and glycerols (Mochida et al., 2002; Wang et al., 2015; Cochran et al., 2016, 2017; Moran et al., 2016; Rastelli et al., 2017). Rastelli et al. (2017) provided the first indication of extracellular DNA enrichment in marine aerosol samples and hypothesized processes leading to cell lysis, such as viral infection, led to the presence of nucleic acid found in SSA. Amino acids and proteins are enriched in primary aerosol (Kuznetsova et al., 2005; Hawkins and Russell, 2010; Rastelli et al., 2017; Schiffer et al., 2018). Proteins in the form of microbial enzymes (e.g., lipase, protease, and alkaline phosphatase) are a component of marine aerosol (Wang et al., 2017; Schiffer et al., 2018; Malfatti et al., 2019). Some enzymes have a higher activity in SSA when compared to subsurface water and may modify aerosol droplet chemistry and physical properties (Malfatti et al., 2019). Enzymes in the atmosphere potentially impact radiative budgets and aerosol-cloud interactions by changing the composition and surface properties of aerosol.
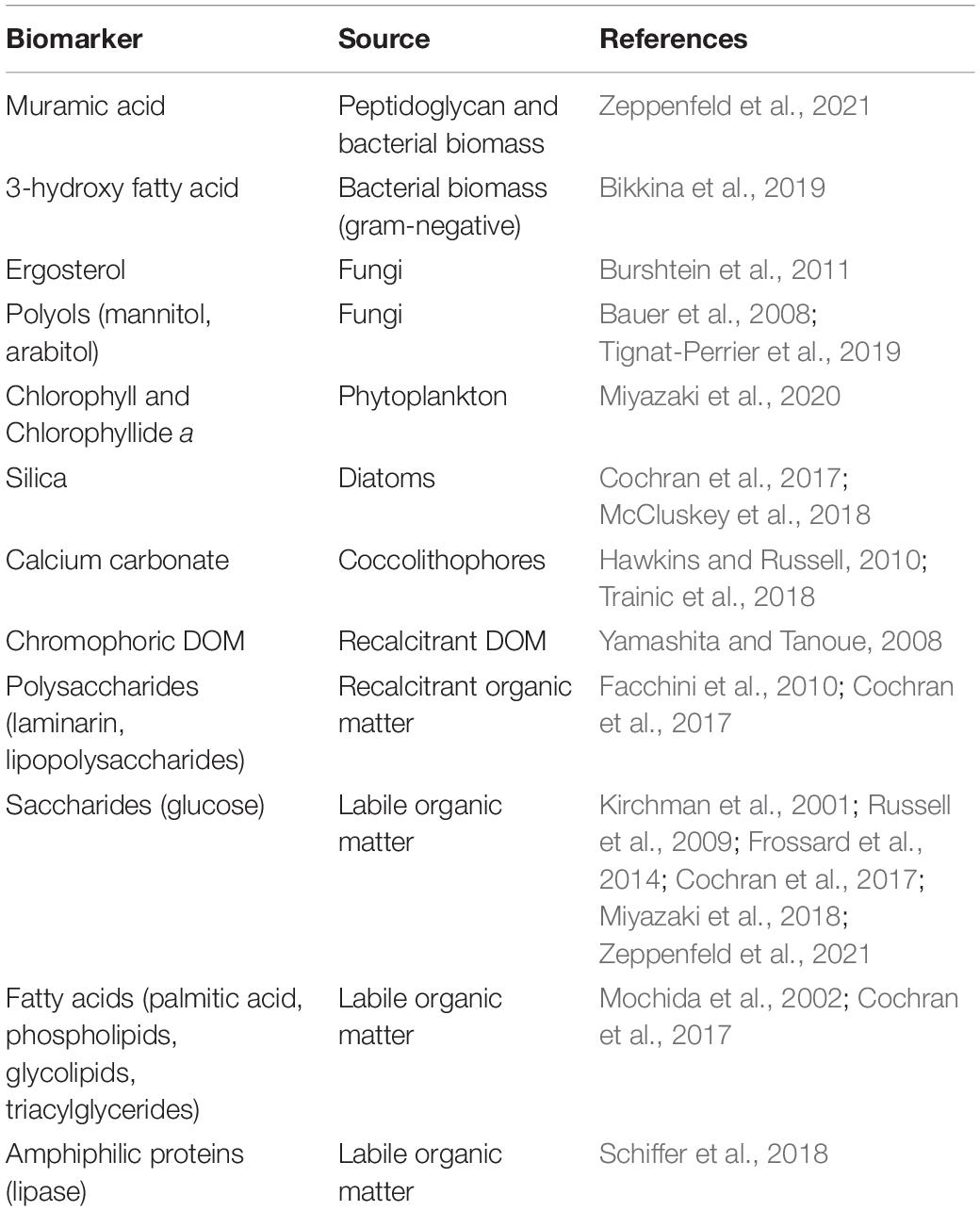
Table 2. Potential biomarkers of different sources of organic matter and microorganisms in the atmosphere.
Humic-like substances (HULIS) are a loosely defined class of large acidic or polyacidic, chromophoric and fluorescent organic molecules, which are often present in the atmosphere (Brooks et al., 2004; Dinar et al., 2007). The presence of HULIS modifies the water uptake (Brooks et al., 2004) and optical properties of aerosol (Hoffer et al., 2006) and may influence droplet formation and ice nucleation in the atmosphere (Chen et al., 2021). HULIS has been identified in marine aerosol (Cavalli et al., 2004; Krivácsy et al., 2008; Deng et al., 2014). Humic and humic-like substances in the ocean are a component of chromophoric dissolved organic matter (CDOM), which is often analyzed using optical techniques (Coble, 1996, 2007). Excitation-emission matrix spectroscopy has shown several common peaks indicating the humic components of CDOM in seawater (Coble, 1996, 2007; Stedmon and Markager, 2005). HULIS are produced by marine ecosystems (Stedmon and Markager, 2005; Murphy et al., 2008) or are transported into the ocean from terrestrial sources by rivers (Hedges et al., 1992; Huguet et al., 2009; Krachler and Krachler, 2021). The relative contributions of HULIS in marine aerosol from marine ecosystem processes, terrestrial ecosystems, and anthropogenic sources (e.g., combustion of coal and biomass; Huo et al., 2021) is uncertain. Much remains to be learned about the molecular diversity of primary marine aerosol and how it relates to processes in the underlying water. There is a need for analytical approaches that directly compare organic matter collected in both ocean and atmosphere to determine which components of marine DOM and particulate organic matter (POM) are transferred into the atmosphere and in what quantities (Brooks and Thornton, 2018).
Emissions of Marine Microorganisms to the Atmosphere
Virus Emissions
Viruses are the most genetically diverse and abundant (109–1012 L–1; Suttle, 2005) biological entities in the ocean and play an important role in both regional and global biogeochemical cycling (Suttle, 2007; Mojica and Brussaard, 2014). Due to their high abundance and small size (20–200 nm diameter; Fuhrman, 1999), viruses are probably an important component of primary marine aerosol. Limited research shows that viruses occur in marine aerosol and are enriched in SSA up to 250-fold compared with the underlying seawater (Baylor et al., 1977; Aller et al., 2005; Leck and Bigg, 2005; Sharoni et al., 2015; Rastelli et al., 2017; Michaud et al., 2018). Viruses attach to exopolymer particles and concentrate in the SML (Aller et al., 2005). Genomic analysis of viral DNA indicates that lipid-enveloped viruses are enriched in SSA, possibly due to their hydrophobic surface properties (Michaud et al., 2018). Enveloped viruses can tolerate desiccation and withstand relative humidity (RH) as low as 15%, which is important for remaining infective during atmospheric transport (Tang, 2009).
Reche et al. (2018) found that atmospheric viruses are associated with particles, such as soil grains and marine organic aggregates. However, virus deposition was correlated with small aerosol particles (<0.7 μm diameter) compared with the larger particles (>0.7 μm diameter) associated with bacterial deposition. Based on this observation, Reche et al. (2018) hypothesized that viruses are transported further in the atmosphere than bacteria. Reche et al. (2018) measured deposition rates as two sites in the Sierra Nevada Mountains (Spain), which were located above the atmospheric boundary layer (1.7 ± 0.5 km above sea level at this location) at 2.9 and 3.0 km above sea level. Deposition rates of viruses were 9–461 times greater than those of bacteria and ranged from 0.26 × 109 to >7 × 109 m–2 per day. Back trajectories indicated higher virus deposition rates were associated with air masses originating from the Atlantic Ocean rather than terrestrial sources (Reche et al., 2018).
Viruses are a major source of microbial mortality, influencing phytoplankton bloom termination and the abundance and composition of Bacterial and Archaeal assemblages. Lytic viral infection results in the release of small POM particles and DOM composed of the major classes of organic matter (carbohydrates, proteins, lipids, and nucleic acids) (Wilhelm and Suttle, 1999; Suttle, 2005), altering the composition and distribution of organic matter, and influencing organic particle size distributions (Fuhrman, 1999). Consequently, in addition to the aerosolization of viruses themselves, interactions between viruses and their hosts have significant impact on the composition of the pool of organic matter available for aerosolization from the ocean.
Archaea Emissions
Widely used cell counting methods, including staining cells with fluorescent probes, such as 4′,6-diamidino-2-phenylindole (DAPI) (Porter and Feig, 1980), are designed to give total counts rather than discriminate between different taxa. Consequently, many counts of microorganisms in aerosol samples do not distinguish between Bacteria and Archaea and “bacterial” counts potentially include cells from both domains of life (e.g., Aller et al., 2005; Amato et al., 2007). Archaea in the atmosphere are poorly characterized as a separate group, with a limited number of studies from urban (Radosevich et al., 2002; Brodie et al., 2007; Bowers et al., 2013; Robertson et al., 2013; Smith et al., 2013), terrestrial and coastal (Yooseph et al., 2013; Fröhlich-Nowoisky et al., 2014), and remote ocean regions (Cho and Hwang, 2011; Xia et al., 2015). The emission flux of marine Archaea into the atmosphere has not been estimated, though it is likely to be significantly less than that of Bacteria. While Archaea are numerically abundant in the ocean, they make up a small proportion (<20%) of the total prokaryote population (Karner et al., 2001; Church et al., 2003; Kirchman et al., 2007) at the ocean surface. Limited sequencing studies show that Bacteria are more prevalent than Archaea in the atmosphere (Cho and Hwang, 2011; Cáliz et al., 2018).
Bacteria Emissions
Sea spray aerosol is enriched in bacteria compared with the underlying seawater (10–2,500-fold) (Blanchard and Syzdek, 1982; Marks et al., 2001; Aller et al., 2005; Rastelli et al., 2017). Marine air masses contain primarily Gram-negative bacteria, whereas continental air masses are dominated by Gram-positive (Fahlgren et al., 2010; Cho and Hwang, 2011; Urbano et al., 2011). This analysis suggests bacteria of marine origin are significant in air masses over the ocean as Gram-negative bacteria comprise up to 80–95% of the total bacteria counts in seawater (Watson et al., 1977). Molecular techniques, including 16S rRNA gene sequencing, quantitative PCR (qPCR) (Cho and Hwang, 2011; Urbano et al., 2011; DeLeon-Rodriguez et al., 2013; Fahlgren et al., 2015; Mayol et al., 2017; Romano et al., 2019; Uetake et al., 2020), and metagenomics (Michaud et al., 2018; Amato et al., 2019) are used to determine composition and indicate atmospheric abundances. Many non-culturable bacterial clades are emitted to the atmosphere, and therefore the community is more diverse and abundant than previously thought based on traditional culturing methods (Cho and Hwang, 2011; Cáliz et al., 2018; Uetake et al., 2020). Different regions of the ocean emit distinct bacteria to the atmosphere, resulting in significant differences in airborne microbial communities (Seifried et al., 2015; Michaud et al., 2018; Amato et al., 2019). Processes at the air-sea interface are selective and some bacteria are preferentially lofted into the atmosphere, such as mycolic acid-coated taxa with hydrophobic surface properties (Michaud et al., 2018).
It is not known whether ubiquitous marine clades, such as SAR11 (Morris et al., 2002; Giovannoni, 2017), are prevalent components of SSA. SAR11 has the potential to travel long distances in the atmosphere due to its small size (Figures 1, 2). Aerial dispersal could play a role in the population ecology and biogeography of SAR11 and other abundant clades of bacteria. Of particular interest are the cyanobacteria due to their numerical abundance in surface waters and key ecosystem role as photosynthetic primary producers. Prochlorococcus is the most numerically abundant photosynthetic organism on Earth (Schattenhofer et al., 2009; Flombaum et al., 2013; Biller et al., 2015), and Synechococcus is numerically abundant with a wider geographical distribution (Partensky et al., 1999; Flombaum et al., 2013). Sequencing of the 16S rRNA gene showed relatively low abundances of cyanobacteria in aerosol over coastal regions, though this was likely due to dilution by terrestrial heterotrophic bacteria (Cho and Hwang, 2011; Seifried et al., 2015; Xia et al., 2015; Hu et al., 2017). It is important to know if bacterial populations, particularly those separated by geographical barriers such as continents, are connected via the atmosphere sufficiently to affect their population ecology. This is a significant challenge, requiring a better understanding the population structure of bacteria in the ocean, which should be integrated into studies of bacterial population structure and viability in SSA. This approach should be applied to numerically abundant, widely distributed, and biogeochemically significant clades from the Earth’s largest biome, the open ocean.
Eukaryote Emissions
In contrast to Bacteria and Archaea, eukaryotes are characterized by structurally complex cells that contain membrane bound structures, including a nucleus. Two groups of eukaryotes are commonly found in the marine atmosphere; protists and fungi. Protists are a diverse, and predominantly unicellular, paraphyletic group of organisms (O’Malley et al., 2013; Adl et al., 2019; Burki et al., 2020). Paraphyly refers to a grouping of organisms that are all descended from a common ancestor, but not all the descendants of the common ancestor are included in the group. For example, animals and plants share a common ancestor with all protists, but these multicellular organisms are not protists. Examples of marine protists include eukaryote phytoplankton (e.g., dinoflagellates, diatoms, coccolithophores, and chlorophytes) and heterotrophic organisms such as ciliates, foraminifera, and radiolaria (Adl et al., 2019).
The transfer of eukaryote phytoplankton to the atmosphere from the ocean occurs in the form of intact cells and recognizable cell fragments. Laboratory studies have found fragments of coccoliths from Emiliania huxleyi cell walls (Hawkins and Russell, 2010; Trainic et al., 2018). Diatom cells and cell fragments (including siliceous material from cell walls) were observed in several field and laboratory studies (Bigg and Leck, 2001; Leck and Bigg, 2005; Alpert et al., 2015; Lee et al., 2015; Cochran et al., 2017; Marks et al., 2019). The North Atlantic spring bloom, which is dominated by diatoms, produces organic-rich SSA (O’Dowd et al., 2004, 2015; Yoon et al., 2007; Sciare et al., 2009; Rinaldi et al., 2013). Diatoms are hypothesized to have a greater atmospheric significance than other eukaryotes due to their observed enrichment in SSA (Fuentes et al., 2010). Cell wall components could be used as biomarkers of major phytoplankton groups in marine aerosol (Table 2).
Laboratory experiments showed that rising bubbles scavenge small diatoms and eject them into the atmosphere when the bubbles burst at the air-water interface, with an enrichment factor of up to 307 in the emitted jet drops compared with the concentration in the bulk water (Marks et al., 2019). Aerial dispersal of eukaryote phytoplankton on the order of 102 to 103 km is possible in the troposphere (Figure 2). Airborne eukaryotes from terrestrial, coastal, and freshwater sources colonize new habitats (Schlichting, 1969; Genitsaris et al., 2011; Tesson and Santl-Temkiv, 2018) and therefore it is likely that marine eukaryote phytoplankton are also dispersed to new habitats, affecting their distribution and geographical range. The atmosphere is a relatively harsh environment for eukaryote phytoplankton (Figure 5) and little is known about how atmospheric conditions in the troposphere reduce phytoplankton viability over time. Estimates of eukaryote phytoplankton emitted to the atmosphere from remote marine regions are not known, but total eukaryote abundances in the boundary layer from the North Atlantic Ocean range from 102 to 104 cells m–3 (Mayol et al., 2014). Estimates from subtropical and tropical regions may be higher (102 to 105 eukaryotes m–3), but likely contain mostly terrestrial sources and low phytoplankton abundances (Mayol et al., 2017).
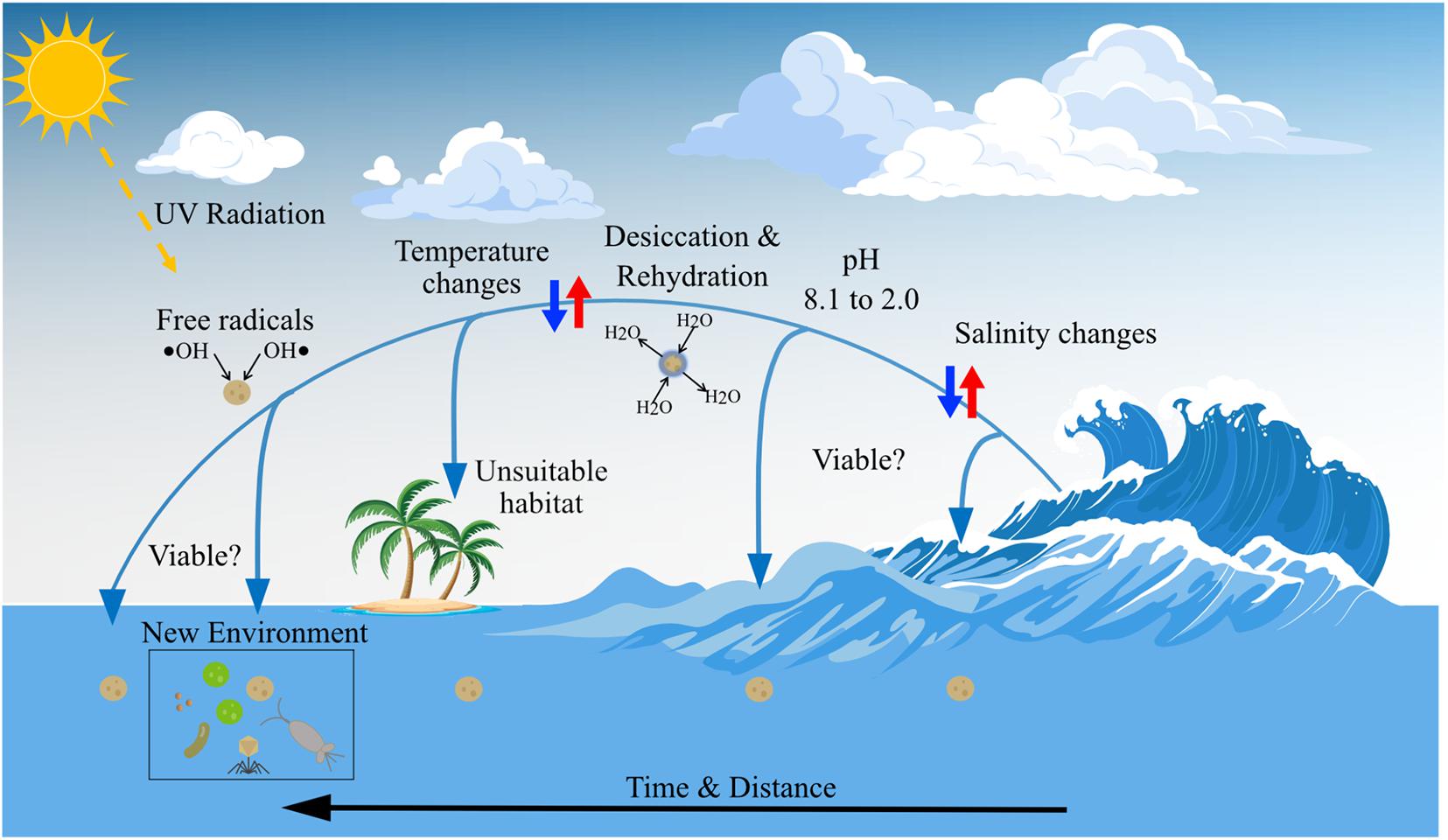
Figure 5. Marine microorganisms are exposed to stressors in the atmosphere that potentially reduce viability: changes in water salinity and pH, temperature change, desiccation, and rehydration, exposure to free radicals and other oxidants, exposure to solar radiation (including UV). Other stressors include the rapid rates of environmental change that can occur on transport across the air-sea interface, and deposition in an unsuitable environment. The vector art used in this figure was downloaded from vecteezy.com.
One approach to assessing biogeographic significant of eukaryotes would be to focus on widely distributed bloom forming taxa, such as diatoms and coccolithophores. The coccolithophore Emiliania huxleyi is globally distributed (Paasche, 2001; Taylor et al., 2017) and coccoliths from E. huxleyi are enriched in SSA (Trainic et al., 2018). The optical properties of E. huxleyi mean that regional blooms, with length scales of 103 kilometers (Balch, 2018) are quantifiable from satellite observations. It would be possible to track the composition and properties of air-masses over a significant period of time as they pass over a bloom. Given the long length and timescales of E. huxleyi blooms, it may be possible to link composition of atmosphere and ocean during these events. In the laboratory, controlled experiments using a marine aerosol reference tank (MART) (Fuentes et al., 2010; Stokes et al., 2013) could be used to investigate the potential aerosolization of specific phytoplankton taxa with ecological and biogeochemical significance. For example, ubiquitous picoeukaryotes (<2 μm diameter) (de Vargas et al., 2015), such as Micromonas sp. and Ostreococcus sp., have the potential to be transported great distances in the atmosphere (Figure 2).
Fungi and heterotrophic protists have been observed in a limited number of studies over the open ocean (Mayol et al., 2017) and in coastal regions (Marks et al., 2001; Prospero et al., 2005; Fu et al., 2013; Genitsaris et al., 2014; Cáliz et al., 2018). The majority of fungal biomass in the ocean consists of single-celled Ascomycota species (Shearer et al., 2007). Their smaller size (less than 3 μm) indicates they may be transported longer distances than the primarily terrestrial fungal group, the Basidiomycota (greater than 3 μm) (Fröhlich-Nowoisky et al., 2009, 2012). On a global scale, marine fungal spore emissions are several orders of magnitude smaller than terrestrial emissions (Elbert et al., 2007; Heald and Spracklen, 2009; Fröhlich-Nowoisky et al., 2012). Using 18S rRNA gene sequencing, Cáliz et al. (2018) determined >75% of total eukaryotes from the atmosphere belonged to Ascomycota and Basidiomycota at a coastal site in the Mediterranean, with air masses originating from both open ocean and continental sources. Fungal spores can survive harsh environmental conditions during atmospheric dispersal (Fröhlich-Nowoisky et al., 2016) and may have relatively long viable residence times. It is not clear how far fungi and other eukaryotes travel in the atmosphere. Potentially, a cell 3 μm in diameter could be dispersed over 30,000 km, starting at an altitude of 1,000 m with a wind speed of 10 m s–1 (see Figure 2 for an explanation). This journey would take 35 days; it is unlikely that viable eukaryotes travel such distances as they would be returned to the ocean through wet deposition en route, or lose viability in the atmosphere.
Ecological Significance of Marine Microorganisms in the Atmosphere
Biogeographical patterns of microorganisms in the ocean are mainly determined by local selection and dispersal mechanisms (Hanson et al., 2012), such as relatively slow-moving oceanic currents and fast-moving winds (Wilkins et al., 2013). Community similarity decreases with increasing geographical distance due to limitations of dispersal and adaptation (Morlon et al., 2008; Nemergut et al., 2013). Relatively few studies have focused on the ecological implications of airborne microbial communities (Kellogg and Griffin, 2006; Smith et al., 2013). Potentially, the dispersal of viable marine microorganisms through the atmosphere would enable them to overcome environmental barriers (Figure 5) between suitable habitats (Hamilton and Lenton, 1998; Thornton, 1999). Such aerial connectivity may contribute to the genetic composition (Sharma and Singh, 2010; Womack et al., 2010) and emergent phenotypic traits of populations. For example, Prochlorococcus is known to have distinct ecotypes found in different environments, with differences in both physiology and geographical distribution (Rocap et al., 2003; Biller et al., 2015). Tracking the potential aerial emission and dispersal of different Prochlorococcus genotypes using molecular techniques could provide insight into genetic dispersal, variability between distant marine regions, and the evolution of the different ecotypes observed today.
After emission, marine microorganisms may travel for several days, with an estimated 10% remaining in the atmosphere after 4 days (Mayol et al., 2014), covering distances more than sufficient to traverse the Atlantic or Indian Oceans. The deposition of marine microorganisms was estimated to be 9.85 eukaryotes m–2 s–1 and 49.00 prokaryotes m–2 s–1 over the North Atlantic Ocean, indicating it would take up to 0.7 days to deposit 50% of aerosolized microorganisms (Mayol et al., 2014). It is difficult to extrapolate these estimates to the global ocean because so few studies have been conducted to determine fluxes and deposition rates (Burrows et al., 2009a). Larger eukaryotic phytoplankton have residence times of less than 1 day and potential to travel a few hundred kilometers, whereas smaller microorganisms (<5 μm), such as cyanobacteria, SAR11, and viruses, have potential residence times of years and travel much longer distances (Figure 2). Calculations of atmospheric residence time and distance transported based on organism size are likely to be overestimates as most atmospheric microorganisms are associated with larger particles (Reche et al., 2018). Atmospheric residence time is based on the gravitational forces associated with mass, as well as the drag forces associated with size, density, and shape (Tesson et al., 2016). Additionally, atmospheric properties such as wind speed, direction, and precipitation affect distance traveled. Atmospheric structures, such as clouds and fog, may limit dispersal to higher altitudes (Carson and Brown, 1976). Microorganisms are deposited by dry or wet deposition (Tesson et al., 2016). Dry deposition includes settling and impaction of particles under the influence of meteorological conditions such as wind, RH, and temperature (Tesson et al., 2016). Wet deposition is a major removal mechanism of microorganisms from the atmosphere (Burrows et al., 2009b), which limits exposure to environmental stressors by significantly reducing atmospheric residence time (Tesson et al., 2016).
It is estimated that the minority (1–25%) of microorganisms emitted to the atmosphere are viable upon deposition (Womack et al., 2010; Polymenakou, 2012). If aerosolization and processing in the atmosphere results in cell death, then aerial transport cannot play a significant role in determining ecosystem structure, population genetics, or colonization of distant locations. During atmospheric dispersal marine microorganisms face harsh environmental conditions (Figure 5), including increased UV radiation exposure, desiccation and rehydration, temperature changes, exposure to free radicals and other oxidants, rapid salinity changes, relatively low pH, and limited nutrients for growth (Marthi et al., 1990; Walter et al., 1990; Womack et al., 2010; Tesson et al., 2016; Tesson and Santl-Temkiv, 2018; Wilbourn et al., 2020; Angle et al., 2021). Studies of aerosolization have indicated viability is dependent on temperature, RH, salt concentration, and droplet size (Marthi et al., 1990; Walter et al., 1990). Alsved et al. (2018) concluded, from experiments with the terrestrial plant bacterial pathogen Pseudomonas syringae, that the conditions under which cells dry in the atmosphere are key to determining viability. Marine microorganisms in SSA are likely to be exposed to significant and rapid changes in salinity during atmospheric transport and therefore significant osmotic challenges. Freshly emitted SSA has the salinity of local seawater, which is significantly reduced during cloud formation processes to due to rapid uptake of water by aerosol particles (Wilbourn et al., 2020). Conversely, salt within SSA may increase to concentrations several times that of seawater as water evaporates from an aerosol particle. The pH of the ocean is 8.1; airborne microorganisms are exposed to acidic conditions (pH ∼2) in fresh SSA (Angle et al., 2021) and precipitation (pH ∼5.6) (Charlson and Rodhe, 1982). Exposure to UV radiation reduces viability, but many microorganisms produce pigments (e.g., carotenoids) or contain high DNA guanine-cytosine (GC) content (Matallana-Surget et al., 2008) to avoid photodamage.
Culturing organisms isolated from aerosol samples is a proven method for determining viability (Amato et al., 2005; 2007; Ahern et al., 2007; Renard et al., 2016), though only a minority (<1%) of prokaryotes in the ocean and atmosphere are amenable to culture using current methods (Amato et al., 2005, 2007; Kirchman et al., 2009; Fröhlich-Nowoisky et al., 2016). The vast majority of taxa (>99%) are simply ignored in abundance and community composition studies dependent on culturing methods. Studies combining culture-independent (e.g., 16S and 18S RNA gene sequencing) and culture-dependent approaches illustrate the limitations of culturing, with sequencing methods showing higher abundances and a more diverse population (Cho and Hwang, 2011; Ravva et al., 2012; Smith et al., 2012; DeLeon-Rodriguez et al., 2013). Culture-independent methods indicate the presence of a particular operational taxonomic unit (OTU), but not whether it was alive in the aerosol. Culture-dependent methods directly show viability, but only for the limited number of taxa that are selected for by the culture conditions. A lack of standard sampling methods makes interpretation and comparison between studies challenging (Burrows et al., 2009a).
Methods are needed that combine the power and resolution of culture-independent methods with measurements of viability. Viability is commonly determined using live/dead staining techniques based on fluorescent probes (Hu et al., 2017, 2020; Alsved et al., 2018). These methods provide information on the relative proportion of viable cells in a sample, but not which taxa are viable. New molecular based methods, such as viability PCR (vPCR), could be used to not only characterize the atmospheric microbial community but also differentiate viable from non-viable cells (Cangelosi and Meschke, 2014; Baymiev et al., 2020). Live/dead staining and vPCR are not direct measures of viability; it is assumed that cell permeability indicates compromised cell membranes and therefore non-viable cells. Active gene expression provides an alternative approach to assay for viability in airborne microbial communities, using methods such as serial analysis of gene expression (SAGE) (Velculescu et al., 1995; Hu and Polyak, 2006). Even defining living and dead microorganisms is complex and debated (Emerson et al., 2017), which raises the question of whether using a single method is conclusive. The current list of available culture-dependent and independent studies of airborne microbial communities is summarized in Supplementary Table 3.
A major unknown is what proportion of the marine aerobiota is metabolically active and whether this has a significant effect on aerosol-cloud interactions and the processing of marine DOM in the atmosphere. Cloud water contains DOM, which could support microbial metabolism (Khaled et al., 2020), including the remineralization of DOM back to carbon dioxide. Ervens and Amato (2020) estimated that the global loss of DOM in clouds is 0.008–0.011 Pg C yr–1, which is insignificant when compared with the 662 Pg C as DOM in the ocean (Hansell et al., 2009), or estimates of annual oceanic photosynthetic production of 45–55 Pg C yr–1 (Longhurst et al., 1995; Field et al., 1998; Carr et al., 2006; Westberry et al., 2008). It seems highly unlikely that overlooking the microbial remineralization of organic matter in the atmosphere has resulted in significant error in global carbon cycling models. Untargeted metatranscriptomics of samples collected in clouds at a terrestrial site (Puy de Dôme mountain, France) indicated a diverse range of metabolic processes within active cells; including energy metabolism, stress responses, transcription and translation, transport, and biosynthesis (Lallement et al., 2018; Amato et al., 2019). To date, metatranscriptomic approaches have not been used to determine microorganism activity in SSA collected over the ocean. Cell division within aerosol and rainwater has been shown (Dimmick et al., 1979; Herlihy et al., 1987; Sattler et al., 2001). Ervens and Amato (2020) estimated that bacterial growth and cell division generates 3.7 Tg yr–1 of secondary biological aerosol globally. This estimate is poorly constrained due to limited data. Research on living microorganisms in the atmosphere is in its infancy, and the connections between marine microorganisms and microbial activity in the atmosphere are unknown. At present, it is not known whether metabolically active bacteria are rare exceptions or whether there are microbial communities forming the network of interactions associated with ecosystems (Smets et al., 2016).
The preceding discussion has focused on cellular organisms, but the aerial dispersal of viable viruses has implications for their host populations, in addition to the biogeography and population genetics of the viruses themselves. Emiliania huxleyi viruses (EhVs) affect regional-scale biogeochemical processes due to their role in terminating Emiliania huxleyi blooms (Bratbak et al., 1993; Lehahn et al., 2014; Sharoni et al., 2015). Emiliania huxleyi viruses (EhVs) are emitted as primary aerosol, with evidence that they are transported and remain infective over hundreds of kilometers (Sharoni et al., 2015).
In conclusion, aerial dispersal is an overlooked, but potentially important, mechanism that may lead to a better understanding of marine microbial biogeography. Atmospheric dispersal has important implications for microbial assemblage composition and genetic diversity through ecological processes such as horizontal gene transfer and competition (Womack et al., 2010; Nemergut et al., 2013). However, more data are needed on biogenic aerosol emission, transport, and particularly viability, before we can integrate atmospheric processes into our models of microbial oceanography, and biogeochemistry.
Effect of Aerosolized Marine Organic Matter on Atmospheric Processes
Aerosol plays a significant role in climate by directly scattering or absorbing solar radiation and indirectly by affecting cloud properties by acting as INPs or CCN (Boucher et al., 2013; Figure 4). SSA is the dominant driver of light scattering in the marine boundary layer (Lu et al., 2015; Quinn et al., 2015) and global modeling suggests SSA radiative forcing could be greater than natural continental sources, such as mineral dust or sulfate aerosol particles (Jacobson, 2001; Takemura et al., 2002). A fraction of the organic matter in SSA will be chemically altered in the atmosphere due to solar radiation exposure, acidic conditions, and oxidation (Mopper et al., 1991; Keene et al., 2007; Frossard et al., 2014; Kieber et al., 2016; Beaupré et al., 2019; Figure 5). Chemical degradation forms inorganic volatile carbon species (e.g., CO2 and CO; Kieber et al., 2016; Beaupré et al., 2019) or low-molecular-weight organic compounds such as aldehydes, ketones, and organic acids (Frossard et al., 2014; Kieber et al., 2016), and inorganic species containing nutrients (N and P) (Beaupré et al., 2019). The photochemical oxidation of marine recalcitrant organic matter in the atmosphere affects the formation of inorganic carbon, or labile molecules that are remineralized to inorganic carbon by heterotrophic bacteria on deposition in the ocean. Thus, atmospheric photochemical oxidation is a poorly constrained sink for marine recalcitrant organic matter (Keene et al., 2015; Kieber et al., 2016). Processing of organic matter in SSA that alters fundamental properties, such as particle size and chemical composition, modify the ability of aerosol to act as CCN and INPs.
Some studies have shown that primary marine aerosol enriched in organic matter has increased CCN activity (Meskhidze et al., 2011; Ovadnevaite et al., 2011), while other work showed that organic matter reduces CCN activity (Fuentes et al., 2011) or has little to no effect on CCN activation (Deng et al., 2014; Schwier et al., 2015; Collins et al., 2016; Bates et al., 2020; Hendrickson et al., 2021). Sea salt is a major source of CCN in remote marine regions (O’Dowd et al., 1997; Clarke et al., 2006). Due to the large emission of sea salt to the atmosphere (2,000–10,000 Tg yr–1) and small contribution of organic matter (10 ± 5 Tg yr–1) (Gantt and Meskhidze, 2013), organics likely have a small or negligible effect on CCN activity, especially in SSA particles that are a mixture of sea salt and organic matter, as there is simply not enough organic matter to affect CCN properties (Hendrickson et al., 2021) (see Supplementary Table 1). In addition, it is challenging to establish links between ecosystems and CCN due to the complexity and different timescales of processes in both ocean and atmosphere. For example, coccolithophore blooms are proposed as a major source of DMS (Malin et al., 1993; Matrai and Keller, 1993), which is oxidized in the atmosphere to form aerosol that activate as CCN (Charlson et al., 1987; Quinn and Bates, 2011). Laboratory experiments showed that viral infection induces the coccolithophore, Emiliania huxleyi, to shed coccoliths, which became enriched in SSA (Trainic et al., 2018). Coccolith enrichment in SSA potentially increases cloud droplet alkalinity, leading to reactions between sulfur dioxide and ozone, and reducing CCN activity from DMS-derived sulfur (Sievering et al., 2004; Trainic et al., 2018).
Clouds containing ice are present at all latitudes (Shupe et al., 2008; Korolev et al., 2017), affecting Earth’s radiative budget and precipitation patterns (Boucher et al., 2013; Vali et al., 2015). Homogeneous freezing of pure water droplets in the atmosphere occurs below −38°C due to the stearic challenge of freezing in tiny droplets (Hoose and Möhler, 2012; Kanji et al., 2017). Heterogeneous freezing catalyzed by INPs occurs at warmer temperatures, although still below 0°C (Vali, 1971; Hoose and Möhler, 2012). Heterogeneous freezing drives the formation and properties of mixed-phase and ice clouds in the troposphere. Although the composition of an effective INP remains poorly understood, there are certain characteristics that promote ice nucleation. These include a crystalline structure, large particle surface area, an amorphous semi-solid or viscous liquid phase (Hoose and Möhler, 2012; Collier and Brooks, 2016; Kanji et al., 2017; Knopf et al., 2018). Efficient INPs may contain hydroxyl and amino groups that initiate ice formation via hydrogen bonding to water molecules (Kanji et al., 2017). Sea salt suppresses the freezing temperature of INPs and therefore inhibits the ability of organic-rich aerosol to efficiently act as an INP (Wilbourn et al., 2020). However, the activation of SSA as CCN dilutes the salt enabling marine organic matter to act as efficient immersion INPs (Wilbourn et al., 2020). Global modeling indicates marine organics may be an important source of INPs, particularly in remote marine regions (Burrows et al., 2013; McCluskey et al., 2019).
Both laboratory and field studies indicate SSA generated from biologically productive marine waters nucleate ice moderately efficiently (i.e., 5–15°C warmer than homogeneous nucleation) (Schnell and Vali, 1976; DeMott et al., 2016; Wilbourn et al., 2020). Ice nucleation of biogenic aerosol may change depending on phytoplankton physiological status (Prather et al., 2013; McCluskey et al., 2017), and phytoplankton senescence is proposed as a major source of INPs due to the release of organic matter (McCluskey et al., 2018). INPs are associated with a diverse range of phytoplankton groups, including diatoms (Knopf et al., 2010; Alpert et al., 2011a; Wilson et al., 2015), coccolithophores (Alpert et al., 2011b), cyanobacteria (Wolf et al., 2019; Wilbourn et al., 2020), and chlorophytes (Alpert et al., 2011b). Wilbourn et al. (2020) used flow cytometry to sort phytoplankton from the North Atlantic by size and determined picoeukaryotes (1–3 μm), nano-eukaryotes (3–50 μm), and the cyanobacterium, Synechococcus (0.5–1 μm), were all moderately efficient at ice nucleation in the immersion mode. There is a significant amount of data showing that marine biogenic INPs consistently freeze ∼10°C warmer than homogeneous freezing in the immersion mode, which suggests a common, though unknown, property affecting ice nucleation.
Several studies have shown INPs are present in seawater samples that have been filtered and contain particles <0.3 μm in diameter (Rosinski et al., 1987; Wilson et al., 2015; DeMott et al., 2016; Irish et al., 2017). These results indicate that organic INPs are not whole cells, which are generally >0.5 μm in length (Ducklow, 2001). Size and chemical composition suggests that viruses may be important in the formation of ice in clouds. Marine viruses are within the size class (<0.3 μm in diameter) of known INPs (Rosinski et al., 1987; Wilson et al., 2015; DeMott et al., 2016; Irish et al., 2017) and viral capsids are composed of protein (Jover et al., 2014) and therefore contain hydroxyl and amino groups. However, the only known study of marine viruses concluded that they are not efficient INPs (Junge and Swanson, 2008). In contrast, recent measurements determined that in some locations, supermicron aerosol particles may contain a significant proportion of the total INP population, including those freezing at the warmest temperatures (Mason et al., 2016; Creamean et al., 2018; Si et al., 2018; Gong et al., 2020; Mitts et al., 2021). Collectively, these results show that marine INPs are a range of sizes and that both cell fragments and whole cells are potential sources of biogenic INPs.
Conclusion and Future Research
Ocean aerobiology offers an interdisciplinary framework that integrates microbial oceanography, biogeochemistry, atmospheric sciences, climate science, and biogeography to understand the significance of marine organic matter in the atmosphere both today and in response to future climate change. Ocean aerobiology has been overlooked compared with research on microorganisms in ocean water, despite the daily exchange of hundreds of trillions of microorganisms between ocean and atmosphere (Mayol et al., 2014, 2017). Biogeochemical processes in the ocean and overlying atmosphere are often regarded as separate, which downplays the significance of processes occurring across the air-sea interface, and the connection between physical and chemical processes in the atmosphere with biology in the ocean (Brooks and Thornton, 2018).
The potential for marine microorganisms to be dispersed thousands of kilometers in the troposphere within days indicates atmospheric transport could provide a mechanism for dispersal between ocean basins and population connectivity. Under sampling means we know relatively little about which taxa of marine microorganism are found in the atmosphere, their distribution, variability, and seasonality. Characterization of marine aerobiota in space and time is insufficient to address fundamental ecological questions; characterization of community composition must be coupled with an understanding of where organisms originated and where they are deposited, emphasizing a need for aerosol transport modeling applied to ocean aerobiology. Viability is key to determining whether intact marine microorganisms functionally and genetically link distant marine ecosystems via the atmosphere, or are merely a source of organic matter in SSA. Gene expression and viability assays should be employed to determine the potential for airborne marine microorganisms to colonize and grow in new environments, including in the atmosphere itself. A list of stressors that impact marine microorganisms in the atmosphere have been identified (Figure 5), but the physiology of acclimation to those stressors is poorly understood, as well as how different stressors interact. It is likely that the most rapid and greatest magnitude changes in environmental conditions occur during the initial (i.e., aerosolization) and final (i.e., deposition) steps of the atmospheric journey, which may be key in determining controls on viability. The physical and chemical microhabitat of SSA has not been characterized and may play a role moderating microorganisms’ exposure to atmospheric stressors (e.g., UV radiation).
The organic composition of SSA is poorly characterized and there is still much to be learned about how processes, such as bubble bursting, determine which microorganisms and components of DOM are enriched in sea spray. Linking organic matter composition in the atmosphere and ocean requires sampling procedures and analytical techniques conducive for direct and quantitative comparison of organic matter in both (Brooks and Thornton, 2018). This chemical complexity presents analytical and conceptual challenges. Targeted analyses and biomarkers (Table 2) for specific taxa or chemical pathways provide a route to simplify complexity and test specific hypotheses. Biomarkers are limited in that their application requires prior knowledge of the system. While untargeted analyses using ultra-high- resolution mass spectrometry and multivariate statistics (Kujawinski et al., 2009; Tolić et al., 2017) overcomes this limitation, interpreting the large volume of complex chemical data generated by these approaches is challenging.
Marine organic matter is an important source of INPs and may significantly contribute to the formation and properties of mixed-phase and ice clouds on a global scale (DeMott et al., 2016; Brooks and Thornton, 2018). Further work is needed to understand which components of the organic matter in SSA act as INPs. Once structures or compounds that are effective INPs have been identified, then it may be possible to address the fundamental microphysical question of what makes an effective organic INP. Furthermore, identifying the source of INPs will make it possible to design experiments to determine the physiological or ecosystem processes in the water column that produce INPs. A long-term goal should be to construct predictive models that connect marine ecosystems to SSA and their effects on cloud microphysics and climate. As much of the organic matter in marine primary aerosol is derived from phytoplankton, models based on phytoplankton growth and distribution offer the most potential for linking ecosystem processes to the chemical composition of SSA and its atmospheric properties. For example, phytoplankton resource allocation models (Droop, 1983; Geider and La Roche, 2002; Klausmeier et al., 2004) describe phytoplankton growth and chemical composition based on resource availability and physiology. Such models could be used to predict the composition of organic matter produced by phytoplankton based on environmental conditions. The global distribution of different functional groups of phytoplankton in the ocean can be predicted using trait-based models (Follows et al., 2007; Litchman and Klausmeier, 2008; Follows and Dutkiewicz, 2011).
Bioaerosols are ubiquitous in the atmosphere, but are one of the least understood components of the Earth’s biosphere (Fröhlich-Nowoisky et al., 2016; Amato et al., 2017, 2019). Bioaerosols have direct societal implications due to their role in human health, agriculture, and climate (Fröhlich-Nowoisky et al., 2016). The constant exchange of marine microorganisms with the atmosphere shows that the ocean surface is not a hard boundary to marine ecosystems. There is a need to mechanistically understand and quantify the impact of marine organisms and organic matter in the atmosphere. Ocean aerobiology has implications for ecosystem structure and function, biogeochemical cycles, weather, climate, and human wellbeing.
Author Contributions
DT and AA conceived and planned the review. AA wrote the first draft of the manuscript, compiled the tables, and made the figures. DT and SB, in consultation with AA, revised and edited the manuscript prior to submission. All authors contributed to the article and approved the submitted version.
Funding
SB and DT were supported by the National Science Foundation (United States) under Grant No. AGS-1539881. Any opinions, findings, and conclusions or recommendations expressed in this material are those of the authors and do not necessarily reflect the views of the National Science Foundation.
Conflict of Interest
The authors declare that the research was conducted in the absence of any commercial or financial relationships that could be construed as a potential conflict of interest.
Publisher’s Note
All claims expressed in this article are solely those of the authors and do not necessarily represent those of their affiliated organizations, or those of the publisher, the editors and the reviewers. Any product that may be evaluated in this article, or claim that may be made by its manufacturer, is not guaranteed or endorsed by the publisher.
Acknowledgments
The authors gratefully acknowledge the NOAA Air Resources Laboratory (ARL) for the provision of the HYSPLIT transport and dispersion model and/or READY website (https://www.ready.noaa.gov) used in this publication.
Supplementary Material
The Supplementary Material for this article can be found online at: https://www.frontiersin.org/articles/10.3389/fmicb.2021.764178/full#supplementary-material
Abbreviations
CCN, cloud condensation nuclei; CSP, Coomassie staining particles; DMS, dimethyl sulfide; DOM, dissolved organic matter; INPs, ice nucleating particles; MART, marine aerosol reference tank; OTU, operational taxonomic unit; POM, particulate organic matter; RH, relative humidity; SAGE, serial analysis of gene expression; SML, sea surface microlayer; SSA, sea spray aerosol; TEP, transparent exopolymer particles; VOCs, volatile organic compounds; vPCR, viability polymerase chain reaction.
References
Adl, S. M., Bass, D., Lane, C. E., Lukes, J., Schoch, C. L., Smirnov, A., et al. (2019). Revisions to the classification, nomenclature, and diversity of Eukaryotes. J. Eukaryot. Microbiol. 66, 4–119. doi: 10.1111/jeu.12691
Ahern, H. E., Walsh, K. A., Hill, T. C. J., and Moffett, B. F. (2007). Ice-nucleation negative fluorescent pseudomonads isolated from Hebridean cloud and rain water produce biosurfactants. Biogeosciences 4, 115–124. doi: 10.5194/bg-4-115-2007
Alldredge, A. L., Passow, U., and Logan, B. E. (1993). The abundance and significance of a class of large, transparent organic particles in the ocean. Deep Sea Res. I 40, 1131–1140. doi: 10.1016/0967-0637(93)90129-Q
Aller, J. Y., Kuznetsova, M. R., Jahns, C. J., and Kemp, P. F. (2005). The sea surface microlayer as a source of viral and bacterial enrichment in marine aerosols. J. Aerosol Sci. 36, 801–812. doi: 10.1016/j.jaerosci.2004.10.012
Aller, J. Y., Radway, J. C., Kilthau, W. P., Bothe, D. W., Wilson, T. W., Vaillancourt, R. D., et al. (2017). Size-resolved characterization of the polysaccharidic and proteinaceous components of sea spray aerosol. Atmos. Environ. 154, 331–347. doi: 10.1016/j.atmosenv.2017.01.053
Alpert, P. A., Aller, J. Y., and Knopf, D. A. (2011a). Ice nucleation from aqueous NaCl droplets with and without marine diatoms. Atmos. Chem. Phys. 11, 5539–5555. doi: 10.5194/acp-11-5539-2011
Alpert, P. A., Aller, J. Y., and Knopf, D. A. (2011b). Initiation of the ice phase by marine biogenic surfaces in supersaturated gas and supercooled aqueous phases. Phys. Chem. Chem. Phys. 13, 19882–19894. doi: 10.1039/c1cp21844a
Alpert, P. A., Kilthau, W. P., Bothe, D. W., Radway, J. C., Aller, J. Y., and Knopf, D. A. (2015). The influence of marine microbial activities on aerosol production: a laboratory mesocosm study. J. Geophys. Res. Atmos. 120, 8841–8860. doi: 10.1002/2015JD023469
Alsved, M., Holm, S., Christiansen, S., Smidt, M., Rosati, B., Ling, M., et al. (2018). Effect of aerosolization and drying on the viability of Pseudomonas syringae cells. Front. Microbiol. 9:3086. doi: 10.3389/fmicb.2018.03086
Aluwihare, L. I., Repeta, D. J., Pantoja, S., and Johnson, C. G. (2005). Two chemically distinct pools of organic nitrogen accumulate in the ocean. Science 308, 1007–1010. doi: 10.1126/science.1108925
Amato, P., Menager, M., Sancelme, M., Laj, P., Mailhot, G., and Delort, A. M. (2005). Microbial population in cloud water at the Puy de Dome: implications for the chemistry of clouds. Atmos. Environ. 39, 4143–4153. doi: 10.1016/j.atmosenv.2005.04.002
Amato, P., Besaury, L., Joly, M., Penaud, B., Deguillaume, L., and Delort, A.-M. (2019). Metatranscriptomic exploration of microbial functioning in clouds. Sci. Rep. 9:4383. doi: 10.1038/s41598-019-41032-4
Amato, P., Joly, M., Besaury, L., Oudart, A., Taib, N., Mone, A. I., et al. (2017). Active microorganisms thrive among extremely diverse communities in cloud water. PLoS One 12:e0182869. doi: 10.1371/journal.pone.0182869
Amato, P., Parazols, M., Sancelme, M., Laj, P., Mailhot, G., and Delort, A. M. (2007). Microorganisms isolated from the water phase of tropospheric clouds at the Puy de Dôme: major groups and growth abilities at low temperatures. FEMS Microbiol. Ecol. 59, 242–254. doi: 10.1111/j.1574-6941.2006.00199.x
Angle, K. J., Crocker, D. R., Simpson, R. M. C., Mayer, K. J., Garofalo, L. A., Moore, A. N., et al. (2021). Acidity across the interface from the ocean surface to sea spray aerosol. Proc. Natl. Acad. Sci. U. S. A. 118:e2018397118. doi: 10.1073/pnas.2018397118
Balch, W. M. (2018). The ecology, biogeochemistry, and optical properties of coccolithophores. Ann. Rev. Mar. Sci. 10, 71–98. doi: 10.1146/annurev-marine-121916-063319
Bar-On, Y. M., Phillips, R., and Milo, R. (2018). The biomass distribution on Earth. Proc. Natl. Acad. Sci. U. S. A. 115, 6506–6511. doi: 10.1073/pnas.1711842115
Bates, T. S., Quinn, P. K., Coffman, D. J., Johnson, J. E., Upchurch, L., Saliba, G., et al. (2020). Variability in marine plankton ecosystems are not observed in freshly emitted sea spray aerosol over the North Atlantic Ocean. Geophys. Res. Lett. 47:e2019GL085938. doi: 10.1029/2019GL085938
Bates, T. S., Quinn, P. K., Frossard, A. A., Russell, L. M., Hakala, J., Petäjä, T., et al. (2012). Measurements of ocean derived aerosol off the coast of California. J. Geophys. Res. Atmos. 117, 1–13. doi: 10.1029/2012JD017588
Bauer, H., Schueller, E., Weinke, G., Berger, A., Hitzenberger, R., Marr, I. L., et al. (2008). Significant contributions of fungal spores to the organic carbon and to the aerosol mass balance of the urban atmospheric aerosol. Atmos. Environ. 42, 5542–5549. doi: 10.1016/j.atmosenv.2008.03.019
Baylor, E. R., Baylor, M. B., Blanchard, D. C., Syzdek, L. D., and Appel, C. (1977). Virus transfer from surf to wind. Science 198, 575–580. doi: 10.1126/science.918656
Baymiev, A. K., Baymiev, A. K., Kuluev, B. R., Shvets, K. Y., Yamidanov, R. S., Matniyazov, R. T., et al. (2020). Modern approaches to differentiation of live and dead bacteria using selective amplification of nucleic acids. Microbiology 89, 13–27. doi: 10.1134/S0026261720010038
Beaupré, S. R., Kieber, D. J., Keene, W. C., Long, M. S., Maben, J. R., Lu, X., et al. (2019). Oceanic efflux of ancient marine dissolved organic carbon in primary marine aerosol. Sci. Adv. 5:eaax6535. doi: 10.1126/sciadv.aax6535
Bernard, F., Ciuraru, R., Boréave, A., and George, C. (2016). Photosensitized formation of secondary organic aerosols above the air/water interface. Environ. Sci. Technol. 50, 8678–8686. doi: 10.1021/acs.est.6b03520
Bigg, E. K., and Leck, C. (2001). Cloud-active particles over the central Arctic Ocean. J. Geophys. Res. Atmos. 106, 32155–32166. doi: 10.1029/1999JD901152
Bikkina, P., Kawamura, K., Bikkina, S., Kunwar, B., Tanaka, K., and Suzuki, K. (2019). Hydroxy fatty acids in remote marine aerosols over the Pacific Ocean: impact of biological activity and wind speed. ACS Earth Space Chem. 3, 366–379. doi: 10.1021/acsearthspacechem.8b00161
Biller, S. J., Berube, P. M., Lindell, D., and Chisholm, S. W. (2015). Prochlorococcus: the structure and function of collective diversity. Nat. Rev. Microbiol. 13, 13–27. doi: 10.1038/nrmicro3378
Blanchard, D. C. (1963). The electrification of the atmosphere by particles from bubbles in the sea. Prog. Oceanogr. 1, 71–202. doi: 10.1016/0079-6611(63)90004-1
Blanchard, D. C., and Syzdek, L. D. (1982). Water-to-air transfer and enrichment of bacteria in drops from bursting bubbles. Appl. Environ. Microbiol. 43, 1001–1005. doi: 10.1128/aem.43.5.1001-1005.1982
Blanchard, D. C., and Woodcock, A. H. (1957). Bubble formation and modification in the sea and its meteorological significance. Tellus 9, 145–158. doi: 10.1111/j.2153-3490.1957.tb01867.x
Boucher, O., Randall, D., Artaxo, P., Bretherton, C., Feingold, G., Forster, P., et al. (2013). “Clouds and aerosols,” in Climate Change 2013: the Physical Science Basis. Contribution of Working Group I to the Fifth Assessment Report of the Intergovernmental Panel on Climate Change, eds T. F. Stocker, D. Qin, G.-K. Plattner, M. Tignor, S. K. Allen, J. Boschung, et al. (Cambridge, NY: Camgridge University Press), 571–658. doi: 10.1017/CBO9781107415324.016
Bowers, R. M., Clements, N., Emerson, J. B., Wiedinmyer, C., Hannigan, M. P., and Fierer, N. (2013). Seasonal variability in bacterial and fungal diversity of the near-surface atmosphere. Environ. Sci. Technol. 47, 12097–12106. doi: 10.1021/es402970s
Bratbak, G., Egge, J. K., and Heldal, M. (1993). Viral mortality of the marine alga Emiliania huxleyi (Haptophyceae) and termination of algal blooms. Mar. Ecol. Prog. Ser. 93, 39–48.
Brodie, E. L., DeSantis, T. Z., Moberg Parker, J. P., Zubietta, I. X., Piceno, Y. M., and Andersen, G. L. (2007). Urban aerosols harbor diverse and dynamic bacterial populations. Proc. Natl. Acad. Sci. U. S. A. 104, 299–304. doi: 10.1073/pnas.0608255104
Brooks, S. D., DeMott, P. J., and Kreidenweis, S. M. (2004). Water uptake by particles containing humic materials and mixtures of humic materials with ammonium sulfate. Atmos. Environ. 38, 1859–1868. doi: 10.1016/j.atmosenv.2004.01.009
Brooks, S. D., and Thornton, D. C. O. (2018). Marine aerosols and clouds. Ann. Rev. Mar. Sci 10, 289–313.
Brüggemann, M., Hayeck, N., and George, C. (2018). Interfacial photochemistry at the ocean surface is a global source of organic vapors and aerosols. Nat. Commun. 9:2101. doi: 10.1038/s41467-018-04528-7
Burki, F., Roger, A. J., Brown, M. W., and Simpson, A. G. B. (2020). The new tree of Eukaryotes. Trends Ecol. Evol 35, 43–55. doi: 10.1016/j.tree.2019.08.008
Burrows, S. M., Elbert, W., Lawrence, M. G., and Pöschl, U. (2009a). Bacteria in the global atmosphere - Part 1: review and synthesis of literature data for different ecosystems. Atmos. Chem. Phys. 9, 9263–9280. doi: 10.5194/acp-9-9263-2009
Burrows, S. M., Elbert, W., Lawrence, M. G., and Pöschl, U. (2009b). Bacteria in the global atmosphere - Part 2: modeling of emissions and transport between different ecosystems. Atmos. Chem. Phys. 9, 9263–9280. doi: 10.5194/acp-9-9263-2009
Burrows, S. M., Hoose, C., Pöschl, U., and Lawrence, M. G. (2013). Ice nuclei in marine air: biogenic particles or dust? Atmos. Chem. Phys. 13, 245–267. doi: 10.5194/acp-13-245-2013
Burshtein, N., Lang-Yona, N., and Rudich, Y. (2011). Ergosterol, arabitol and mannitol as tracers for biogenic aerosols in the eastern Mediterranean. Atmos. Chem. Phys. 11, 829–839. doi: 10.5194/acp-11-829-2011
Cáliz, J., Triadó-Margarit, X., Camarero, L., and Casamayor, E. O. (2018). A long-term survey unveils strong seasonal patterns in the airborne microbiome coupled to general and regional atmospheric circulations. Proc. Natl. Acad. Sci. U. S. A. 115, 12229–12234. doi: 10.1073/pnas.1812826115
Cangelosi, G. A., and Meschke, J. S. (2014). Dead or alive: molecular assessment of microbial viability. Appl. Environ. Microbiol. 80, 5884–5891. doi: 10.1128/AEM.01763-14
Carr, M. E., Friedrichs, M. A. M., Schmeltz, M., Noguchi Aita, M., Antoine, D., Arrigo, K. R., et al. (2006). A comparison of global estimates of marine primary production from ocean color. Deep Res. II 53, 741–770. doi: 10.1016/j.dsr2.2006.01.028
Carson, J., and Brown, R. (1976). The correlation of soil algae, airborne algae, and fern spores with meteorological conditions on the Island of Hawaii. Pac. Sci. 30, 197–205.
Cavalli, F., Facchini, M. C., Decesari, S., Mircea, M., Emblico, L., Fuzzi, S., et al. (2004). Advances in characterization of size-resolved organic matter in marine aerosol over the North Atlantic. J. Geophys. Res. Atmos. 109, 1–14. doi: 10.1029/2004JD005137
Charette, M. A., and Smith, W. H. F. (2010). The volume of Earth’ s ocean. Oceanography 23, 112–114.
Charlson, R. J., Lovelock, J. E., Andreae, M. O., and Warren, S. G. (1987). Oceanic phytoplankton, atmospheric sulphur, cloud albedo and climate. Nature 326, 655–661. doi: 10.1038/326655a0
Charlson, R. J., and Rodhe, H. (1982). Factors controlling the acidity of natural rainwater. Nature 295, 683–685. doi: 10.1038/295683a0
Chen, J., Wu, Z. J., Zhao, X., Wang, Y. J., Chen, J. C., Qiu, Y. T., et al. (2021). Atmospheric humic-like substances (HULIS) act as ice active entities. Geophys. Res. Lett. 48:e2021GL092443. doi: 10.1029/2021GL092443
Chingin, K., Yan, R., Zhong, D., and Chen, H. (2018). Enrichment of surface-active compounds in bursting bubble aerosols. ACS Omega 3, 8709–8717. doi: 10.1021/acsomega.8b01157
Cho, B. C., and Hwang, C. Y. (2011). Prokaryotic abundance and 16S rRNA gene sequences detected in marine aerosols on the East Sea (Korea). FEMS Microbiol. Ecol. 76, 327–341. doi: 10.1111/j.1574-6941.2011.01053.x
Church, M. J., DeLong, E. F., Ducklow, H. W., Karner, M. B., Preston, C. M., and Karl, D. M. (2003). Abundance and distribution of planktonic Archaea and Bacteria in the waters west of the Antarctic Peninsula. Limnol. Oceanogr. 48, 1893–1902. doi: 10.4319/lo.2003.48.5.1893
Clarke, A. D., Owens, S. R., and Zhou, J. (2006). An ultrafine sea-salt flux from breaking waves: implications for cloud condensation nuclei in the remote marine atmosphere. J. Geophys. Res. Atmos. 111, 1–14. doi: 10.1029/2005JD006565
Coble, P. G. (1996). Characterization of marine and terrestrial DOM in seawater using excitation emission matrix spectroscopy. Mar. Chem. 51, 325–346. doi: 10.1016/0304-4203(95)00062-3
Coble, P. G. (2007). Marine optical biogeochemistry: the chemistry of ocean color. Chem. Rev. 107, 402–418. doi: 10.1021/cr050350+
Cochran, R. E., Laskina, O., Jayarathne, T., Laskin, A., Laskin, J., Lin, P., et al. (2016). Analysis of organic anionic surfactants in fine and coarse fractions of freshly emitted sea spray aerosol. Environ. Sci. Technol. 50, 2477–2486. doi: 10.1021/acs.est.5b04053
Cochran, R. E., Laskina, O., Trueblood, J. V., Estillore, A. D., Morris, H. S., Jayarathne, T., et al. (2017). Molecular diversity of sea spray aerosol particles: impact of ocean biology on particle composition and hygroscopicity. Chem 2, 655–667. doi: 10.1016/j.chempr.2017.03.007
Collier, K. N., and Brooks, S. D. (2016). Role of organic hydrocarbons in atmospheric ice formation via contact freezing. J. Phys. Chem. A 120, 10169–10180. doi: 10.1021/acs.jpca.6b11890
Collins, D. B., Bertram, T. H., Sultana, C. M., Lee, C., Axson, J. L., and Prather, K. A. (2016). Phytoplankton blooms weakly influence the cloud forming ability of sea spray aerosol. Geophys. Res. Lett. 43, 9975–9983. doi: 10.1002/2016GL069922
Creamean, J. M., Kirpes, R. M., Pratt, K. A., Spada, N. J., Maahn, M., de Boer, G., et al. (2018). Marine and terrestrial influences on ice nucleating particles during continuous springtime measurements in an Arctic oilfield location. Atmos. Chem. Phys. 18, 18023–18042. doi: 10.5194/acp-18-18023-2018
Croft, B., Martin, R. V., Moore, R. H., Ziemba, L. D., Crosbie, E. C., Liu, H., et al. (2021). Factors controlling marine aerosol size distributions and their climate effects over the northwest Atlantic Ocean region. Atmos. Chem. Phys. 21, 1889–1916. doi: 10.5194/acp-21-1889-2021
Cunliffe, M., Engel, A., Frka, S., Gašparović, B., Guitart, C., Murrell, J. C., et al. (2013). Sea surface microlayers: a unified physicochemical and biological perspective of the air–ocean interface. Prog. Oceanogr. 109, 104–116. doi: 10.1016/j.pocean.2012.08.004
Cunliffe, M., and Murrell, J. C. (2009). The sea-surface microlayer is a gelatinous biofilm. ISME J. 3, 1001–1003. doi: 10.1038/ismej.2009.69
Cunliffe, M., Salter, M., Mann, P. J., Whiteley, A. S., Upstill-Goddard, R. C., and Murrell, J. C. (2009). Dissolved organic carbon and bacterial populations in the gelatinous surface microlayer of a Norwegian fjord mesocosm. FEMS Microbiol. Lett. 299, 248–254. doi: 10.1111/j.1574-6968.2009.01751.x
Cunliffe, M., Upstill-Goddard, R. C., and Murrell, J. C. (2011). Microbiology of aquatic surface microlayers. FEMS Microbiol. Rev. 35, 233–246. doi: 10.1111/j.1574-6976.2010.00246.x
Darwin, C. (1846). Geological observations on South America: being the third part of the geology of the voyage of the Beagle, under the command of Capt. Fitzroy, RN during the years 1832 to 1836. Cornhill: Smith, Elder and Company.
Davie-Martin, C. L., Giovannoni, S. J., Behrenfeld, M. J., Penta, W. B., and Halsey, K. H. (2020). seasonal and spatial variability in the biogenic production and consumption of volatile organic compounds (VOCs) by marine plankton in the North Atlantic Ocean. Front. Mar. Sci. 7:611870. doi: 10.3389/fmars.2020.611870
de Vargas, C., Audic, S., Henry, N., Decelle, J., Mahé, F., Logares, R., et al. (2015). Eukaryotic plankton diversity in the sunlit ocean. Science 348:1261605. doi: 10.1126/science.1261605
DeLeon-Rodriguez, N., Lathem, T. L., Rodriguez, L. M., Barazesh, J. M., Anderson, B. E., Beyersdorf, A. J., et al. (2013). Microbiome of the upper troposphere: species composition and prevalence, effects of tropical storms, and atmospheric implications. Proc. Natl. Acad. Sci. U. S. A. 110, 2575–2580. doi: 10.1073/pnas.1212089110
DeMott, P. J., Hill, T. C. J., McCluskey, C. S., Prather, K. A., Collins, D. B., Sullivan, R. C., et al. (2016). Sea spray aerosol as a unique source of ice nucleating particles. Proc. Natl. Acad. Sci. U. S. A. 113, 5797–5803. doi: 10.1073/pnas.1514034112
Deng, C., Brooks, S. D., Vidaurre, G., and Thornton, D. C. O. (2014). Using Raman microspectroscopy to determine chemical composition and mixing state of airborne marine aerosols over the Pacific Ocean. Aerosol Sci. Technol. 48, 193–206. doi: 10.1080/02786826.2013.867297
Dimmick, R. L., Wolochow, H., and Chatigny, M. A. (1979). Evidence for more than one division of bacteria within airborne particles. Appl. Environ. Microbiol. 38, 642–643. doi: 10.1128/aem.38.4.642-643.1979
Dinar, E., Taraniuk, I., Graber, E. R., Anttila, T., Mentel, T. F., and Rudich, Y. (2007). Hygroscopic growth of atmospheric and model humic-like substances. J. Geophys. Res. Atmos. 112, 1–13. doi: 10.1029/2006JD007442
Dixon, J. L., Beale, R., and Nightingale, P. D. (2013). Production of methanol, acetaldehyde, and acetone in the Atlantic Ocean. Geophys. Res. Lett. 40, 4700–4705. doi: 10.1002/grl.50922
Droop, M. R. (1983). 25 years of algal growth kinetics – A personal view. Botanica Marina 26, 99–112. doi: 10.1515/botm.1983.26.3.99
Ducklow, H. W. (2001). “Bacterioplankton,” in Encyclopedia of Ocean Sciences, eds J. Steele, S. Thorpe, and K. Turekian (Cambridge, MA: Academic Press), 217–224. doi: 10.1006/rwos.2001.0493
Elbert, W., Taylor, P. E., Andreae, M. O., and Pöschl, U. (2007). Contribution of fungi to primary biogenic aerosols in the atmosphere: wet and dry discharged spores, carbohydrates, and inorganic ions. Atmos. Chem. Phys. 7, 4569–4588. doi: 10.5194/acp-7-4569-2007
Emerson, J. B., Adams, R. I., Román, C. M. B., Brooks, B., Coil, D. A., Dahlhausen, K., et al. (2017). Schrödinger’s microbes: tools for distinguishing the living from the dead in microbial ecosystems. Microbiome 5:86. doi: 10.1186/s40168-017-0285-3
Engel, A., Bange, H. W., Cunliffe, M., Burrows, S. M., Friedrichs, G., Galgani, L., et al. (2017). The ocean’s vital skin: toward an integrated understanding of the sea surface microlayer. Front. Mar. Sci. 4:165. doi: 10.3389/fmars.2017.00165
Ervens, B., and Amato, P. (2020). The global impact of bacterial processes on carbon mass. Atmos. Chem. Phys. 20, 1777–1794. doi: 10.5194/acp-20-1777-2020
Facchini, M. C., Rinaldi, M., Decesari, S., and Fuzzi, S. (2010). Marine organic aerosol and biological oceanic activity. Chem. Eng. Trans. 22, 107–112. doi: 10.3303/CET1022017
Fahlgren, C., Gómez-Consarnau, L., Zábori, J., Lindh, M. V., Krejci, R., Mårtensson, E. M., et al. (2015). Seawater mesocosm experiments in the Arctic uncover differential transfer of marine bacteria to aerosols. Environ. Microbiol. Rep. 7, 460–470. doi: 10.1111/1758-2229.12273
Fahlgren, C., Hagström, Å, Nilsson, D., and Zweifel, U. L. (2010). Annual variations in the diversity, viability, and origin of airborne bacteria. Appl. Environ. Microbiol. 76, 3015–3025. doi: 10.1128/AEM.02092-09
Field, C. B., Behrenfeld, M. J., Randerson, J. T., and Falkowski, P. (1998). Primary production of the biosphere: integrating terrestrial and oceanic components. Science 281, 237–240. doi: 10.1126/science.281.5374.237
Flemming, H. C., and Wuertz, S. (2019). Bacteria and archaea on Earth and their abundance in biofilms. Nat. Rev. Microbiol. 17, 247–260. doi: 10.1038/s41579-019-0158-9
Flombaum, P., Gallegos, J. L., Gordillo, R. A., Rincon, J., Zabala, L. L., Jiao, N., et al. (2013). Present and future global distributions of the marine cyanobacteria Prochlorococcus and Synechococcus. Proc. Natl. Acad. Sci. U. S. A. 110, 9824–9829. doi: 10.1073/pnas.1307701110
Follows, M. J., and Dutkiewicz, S. (2011). Modeling diverse communities of marine microbes. Ann. Rev. Mar. Sci. 3, 427–451. doi: 10.1146/annurev-marine-120709-142848
Follows, M. J., Dutkiewicz, S., Grant, S., and Chisholm, S. W. (2007). Emergent biogeography of microbial communities in a model ocean. Science 315, 1843–1846. doi: 10.1126/science.1138544
Forestieri, S. D., Staudt, S. M., Kuborn, T. M., Faber, K., Ruehl, C. R., Bertram, T. H., et al. (2018). Establishing the impact of model surfactants on cloud condensation nuclei activity of sea spray aerosol mimics. Atmos. Chem. Phys. 18, 10985–11005. doi: 10.5194/acp-18-10985-2018
Fox, J., Behrenfeld, M. J., Haëntjens, N., Chase, A., Kramer, S. J., Boss, E., et al. (2020). Phytoplankton growth and productivity in the western North Atlantic: observations of regional variability from the NAAMES field campaigns. Front. Mar. Sci. 7:24. doi: 10.3389/fmars.2020.00024
Franklin, M. P., McDonald, I. R., Bourne, D. G., Owens, N. J. P., Upstill-Goddard, R. C., and Murrell, J. C. (2005). Bacterial diversity in the bacterioneuston (sea surface microlayer): the bacterioneuston through the looking glass. Environ. Microbiol. 7, 723–736. doi: 10.1111/j.1462-2920.2004.00736.x
Fröhlich-Nowoisky, J., Burrows, S. M., Xie, Z., Engling, G., Solomon, P. A., Fraser, M. P., et al. (2012). Biogeography in the air: fungal diversity over land and oceans. Biogeosciences 9, 1125–1136. doi: 10.5194/bg-9-1125-2012
Fröhlich-Nowoisky, J., Kampf, C. J., Weber, B., Huffman, J. A., Pöhlker, C., Andreae, M. O., et al. (2016). Bioaerosols in the Earth system: climate, health, and ecosystem interactions. Atmos. Res. 182, 346–376. doi: 10.1016/j.atmosres.2016.07.018
Fröhlich-Nowoisky, J., Pickersgill, D. A., Després, V. R., and Pöschl, U. (2009). High diversity of fungi in air particulate matter. Proc. Natl. Acad. Sci. U. S. A. 106, 12814–12819. doi: 10.1073/pnas.0811003106
Fröhlich-Nowoisky, J., Ruzene Nespoli, C., Pickersgil, D. A., Galand, P. E., Müller-Germann, I., Nunes, T., et al. (2014). Diversity and seasonal dynamics of airborne archaea. Biogeosciences 11, 6067–6079. doi: 10.5194/bg-11-6067-2014
Frossard, A. A., Gérard, V., Duplessis, P., Kinsey, J. D., Lu, X., Zhu, Y., et al. (2019). Properties of seawater surfactants associated with primary marine aerosol particles produced by bursting bubbles at a model air-sea interface. Environ. Sci. Technol. 53, 9407–9417. doi: 10.1021/acs.est.9b02637
Frossard, A. A., Russell, L. M., Burrows, S. M., Elliott, S. M., Bates, T. S., and Quinn, P. K. (2014). Sources and composition of submicron organic mass in marine aerosol particles. J. Geophys. Res. Atmos. 119, 12977–13003.
Fu, P. Q., Kawamura, K., Chen, J., Charrière, B., and Sempéré, R. (2013). Organic molecular composition of marine aerosols over the Arctic Ocean in summer: contributions of primary emission and secondary aerosol formation. Biogeosciences 10, 653–667. doi: 10.5194/bg-10-653-2013
Fuentes, E., Coe, H., Green, D., de Leeuw, G., and McFiggans, G. (2010). Laboratory-generated primary marine aerosol via bubble-bursting and atomization. Atmos. Meas. Tech. 3, 141–162. doi: 10.5194/amt-3-141-2010
Fuentes, E., Coe, H., Green, D., and McFiggans, G. (2011). On the impacts of phytoplankton-derived organic matter on the properties of the primary marine aerosol - Part 2: composition, hygroscopicity and cloud condensation activity. Atmos. Chem. Phys. 11, 2585–2602. doi: 10.5194/acp-11-2585-2011
Fuhrman, J. A. (1999). Marine viruses and their biogeochemical and ecological effects. Nature 399, 541–548. doi: 10.1038/21119
Gantt, B., and Meskhidze, N. (2013). The physical and chemical characteristics of marine primary organic aerosol: a review. Atmos. Chem. Phys. 13, 3979–3996. doi: 10.5194/acp-13-3979-2013
Gantt, B., Meskhidze, N., Facchini, M. C., Rinaldi, M., Ceburnis, D., and O’Dowd, C. D. (2011). Wind speed dependent size-resolved parameterization for the organic mass fraction of sea spray aerosol. Atmos. Chem. Phys. 11, 8777–8790. doi: 10.5194/acp-11-8777-2011
Gao, Q., Leck, C., Rauschenberg, C., and Matrai, P. A. (2012). On the chemical dynamics of extracellular polysaccharides in the high Arctic surface microlayer. Ocean Sci. 8, 401–418. doi: 10.5194/os-8-401-2012
Geider, R. J., and La Roche, J. (2002). Redfield revisited: variability of C:N:P in marine microalgae and its biochemical basis. Eur. J. Phycol. 37, 1–17. doi: 10.1017/S0967026201003456
Genitsaris, S., Kormas, K. A., Christaki, U., Monchy, S., and Moustaka-Gouni, M. (2014). Molecular diversity reveals previously undetected air-dispersed protist colonists in a Mediterranean area. Sci. Total Environ. 478, 70–79. doi: 10.1016/j.scitotenv.2014.01.071
Genitsaris, S., Moustaka-Gouni, M., and Kormas, K. A. (2011). Airborne microeukaryote colonists in experimental water containers: diversity, succession, life histories and established food webs. Aquat. Microb. Ecol. 62, 139–152. doi: 10.3354/ame01463
Giovannoni, S. J. (2017). SAR11 bacteria: the most bbundant plankton in the oceans. Ann. Rev. Mar. Sci. 9, 231–255. doi: 10.1146/annurev-marine-010814-015934
Gong, X., Wex, H., Voigtländer, J., Wadinga Fomba, K., Weinhold, K., Van Pinxteren, M., et al. (2020). Characterization of aerosol particles at Cabo Verde close to sea level and at the cloud level-Part 1: particle number size distribution, cloud condensation nuclei and their origins. Atmos. Chem. Phys. 20, 1431–1449. doi: 10.5194/acp-20-1431-2020
Halsey, K. H., Giovannoni, S. J., Graus, M., Zhao, Y., Landry, Z., Thrash, J. C., et al. (2017). Biological cycling of volatile organic carbon by phytoplankton and bacterioplankton. Limnol. Oceanogr. 62, 2650–2661. doi: 10.1002/lno.10596
Hamilton, W. D., and Lenton, T. M. (1998). Spora and gaia: how microbes fly with their clouds. Ethol. Ecol. Evol. 10, 1–16. doi: 10.1080/08927014.1998.9522867
Hansell, D. A. (2013). Recalcitrant dissolved organic carbon fractions. Ann. Rev. Mar. Sci. 5, 421–445. doi: 10.1146/annurev-marine-120710-100757
Hansell, D. A., Carlson, C. A., Repeta, D. J., and Schlitzer, R. (2009). Dissolved organic matter in the ocean: a controversy stimulates new insights. Oceanography 22, 202–211. doi: 10.5670/oceanog.2009.109
Hansell, D. A., Carlson, C. A., and Schlitzer, R. (2012). Net removal of major marine dissolved organic carbon fractions in the subsurface ocean. Global Biogeochem. Cycles 26, 1–9. doi: 10.1029/2011GB004069
Hanson, C. A., Fuhrman, J. A., Horner-Devine, M. C., and Martiny, J. B. H. (2012). Beyond biogeographic patterns: processes shaping the microbial landscape. Nat. Rev. Microbiol. 10, 497–506. doi: 10.1038/nrmicro2795
Hawkins, L. N., and Russell, L. M. (2010). Polysaccharides, proteins, and phytoplankton fragments: four chemically distinct types of marine primary organic aerosol classified by single particle spectromicroscopy. Adv. Meteorol. 2010:612132. doi: 10.1155/2010/612132
Heald, C. L., and Spracklen, D. V. (2009). Atmospheric budget of primary biological aerosol particles from fungal spores. Geophys. Res. Lett. 36, 1–5. doi: 10.1029/2009GL037493
Hedges, J. I., Hatcher, P. G., Ertel, J. R., and Meyersschulte, K. J. (1992). A comparison of dissolved humic substances from seawater with amazon river counterparts by 13C-NMR spectrometry. Geochim. Cosmochim. Acta 56, 1753–1757. doi: 10.1016/0016-7037(92)90241-a
Hendrickson, B. N., Brooks, S. D., Thornton, D. C. O., Moore, R. H., Crosbie, E., Ziemba, L. D., et al. (2021). Role of sea surface microlayer properties in cloud formation. Front. Mar. Sci. 7:596225. doi: 10.3389/fmars.2020.596225
Herlihy, L. J., Galloway, J. N., and Mills, A. L. (1987). Bacterial utilization of formic and acetic acid in rainwater. Atmos. Environ. 21, 2397–2402. doi: 10.1016/0004-6981(87)90374-X
Hoffer, A., Gelencser, A., Guyon, P., Kiss, G., Schmid, O., Frank, G. P., et al. (2006). Optical properties of humic-like substances (HULIS) in biomass-burning aerosols. Atmos. Chem. Phys. 6, 3563–3570. doi: 10.5194/acp-6-3563-2006
Hoose, C., and Möhler, O. (2012). Heterogeneous ice nucleation on atmospheric aerosols: a review of results from laboratory experiments. Atmos. Chem. Phys. 12, 9817–9854. doi: 10.5194/acp-12-9817-2012
Hu, M., and Polyak, K. (2006). Serial analysis of gene expression. Nat. Protoc. 1, 1743–1760. doi: 10.1038/nprot.2006.269
Hu, W., Murata, K., Fan, C. L., Huang, S., Matsusaki, H., Fu, P. Q., et al. (2020). Abundance and viability of particle-attached and free-floating bacteria in dusty and nondusty air. Biogeosciences 17, 4477–4487. doi: 10.5194/bg-17-4477-2020
Hu, W., Murata, K., Fukuyama, S., Kawai, Y., Oka, E., Uematsu, M., et al. (2017). Concentration and viability of airborne bacteria over the Kuroshio extension region in the northwestern Pacific Ocean: data from three cruises. J. Geophys. Res. Atmos. 122, 12892–12905. doi: 10.1002/2017JD027287
Huguet, A., Vacher, L., Relexans, S., Saubusse, S., Froidefond, J. M., and Parlanti, E. (2009). Properties of fluorescent dissolved organic matter in the Gironde Estuary. Org. Geochem. 40, 706–719. doi: 10.1016/j.orggeochem.2009.03.002
Huo, Y. Q., Guo, Z. H., Li, Q., Wu, D., Ding, X., Liu, A. L., et al. (2021). Chemical fingerprinting of HULIS in particulate matters emitted from residential coal and biomass combustion. Environ. Sci. Technol. 55, 3593–3603. doi: 10.1021/acs.est.0c08518
Irish, V. E., Elizondo, P., Chen, J., Chou, C., Charette, J., Lizotte, M., et al. (2017). Ice-nucleating particles in Canadian Arctic sea-surface microlayer and bulk seawater. Atmos. Chem. Phys. 17, 10583–10595. doi: 10.5194/acp-17-10583-2017
Jacobson, M. Z. (2001). Global direct radiative forcing due to multicomponent anthropogenic and natural aerosols. J. Geophys. Res. Atmos. 106, 1551–1568. doi: 10.1029/2000jd900514
Jayarathne, T., Sultana, C. M., Lee, C., Malfatti, F., Cox, J. L., Pendergraft, M. A., et al. (2016). Enrichment of saccharides and divalent cations in sea spray aerosol during two phytoplankton blooms. Environ. Sci. Technol. 50, 11511–11520. doi: 10.1021/acs.est.6b02988
Jönsson, B. F., and Watson, J. R. (2016). The timescales of global surface-ocean connectivity. Nat. Commun. 7:11239. doi: 10.1038/ncomms11239
Joux, F., Obernosterer, I., Dupuy, C., Reinthaler, T., Herndl, G. J., and Lebaron, P. (2006). Microbial community structure in the sea surface microlayer at two contrasting coastal sites in the northwestern Mediterranean Sea. Aquat. Microb. Ecol. 42, 91–104. doi: 10.3354/ame042091
Jover, L. F., Effler, T. C., Buchan, A., Wilhelm, S. W., and Weitz, J. S. (2014). The elemental composition of virus particles: implications for marine biogeochemical cycles. Nat. Rev. Microbiol. 12, 519–528. doi: 10.1038/nrmicro3289
Junge, K., and Swanson, B. D. (2008). High-resolution ice nucleation spectra of sea-ice bacteria: implications for cloud formation and life in frozen environments. Biogeosciences 5, 865–873. doi: 10.5194/bg-5-865-2008
Kanji, Z. A., Ladino, L. A., Wex, H., Boose, Y., Burkert-Kohn, M., Cziczo, D. J., et al. (2017). Overview of ice nucleating particles. Meteorol. Monogr. 58, 1.1–1.33. doi: 10.1175/AMSMONOGRAPHS-D-16-0006.1
Karlson, B., Andreasson, A., Johansen, M., Karlberg, M., Loo, A., and Skjevik, A.-T. (2020). Nordic Microalgae. Micromonas pusilla. Available online at: http://nordicmicroalgae.org (accessed October 4, 2021).
Karner, M. B., Delong, E. F., and Karl, D. M. (2001). Archaeal dominance in the mesopelagic zone of the Pacific Ocean. Nature 409, 507–510. doi: 10.1038/35054051
Keene, W. C., Galloway, J. N., Likens, G. E., Deviney, F. A., Mikkelsen, K. N., Moody, J. L., et al. (2015). atmospheric wet deposition in remote regions: benchmarks for environmental change. J. Atmos. Sci. 72, 2947–2978. doi: 10.1175/JAS-D-14-0378.1
Keene, W. C., Maring, H., Maben, J. R., Kieber, D. J., Pszenny, A. A. P., Dahl, E. E., et al. (2007). Chemical and physical characteristics of nascent aerosols produced by bursting bubbles at a model air-sea interface. J. Geophys. Res. Atmos. 112, 1–16. doi: 10.1029/2007JD008464
Kellogg, C. A., and Griffin, D. W. (2006). Aerobiology and the global transport of desert dust. Trends Ecol. Evol. 21, 638–644. doi: 10.1016/j.tree.2006.07.004
Khaled, A., Zhang, M., Amato, P., Delort, A.-M., and Ervens, B. (2020). Biodegradation by bacteria in clouds: an underestimated sink for some organics in the atmospheric multiphase system. Atmos. Chem. Phys. Discuss. 21, 3123–3141. doi: 10.5194/acp-2020-778
Kieber, D. J., Keene, W. C., Frossard, A. A., Long, M. S., Maben, J. R., Russell, L. M., et al. (2016). Coupled ocean-atmosphere loss of marine refractory dissolved organic carbon. Geophys. Res. Lett. 43, 2765–2772.
Kirchman, D. L., Elifantz, H., Dittel, A. I., Malmstrom, R. R., and Cottrell, M. T. (2007). Standing stocks and activity of Archaea and Bacteria in the western Arctic Ocean. Limnol. Oceanogr. 52, 495–507. doi: 10.4319/lo.2007.52.2.0495
Kirchman, D. L., Meon, B., Ducklow, H. W., Carlson, C. A., Hansell, D. A., and Steward, G. F. (2001). Glucose fluxes and concentrations of dissolved combined neutral sugars (polysaccharides) in the Ross Sea and Polar Front Zone, Antarctica. Deep Res. Part II Top. Stud. Oceanogr. 48, 4179–4197. doi: 10.1016/S0967-0645(01)00085-6
Kirchman, D. L., Morán, X. A. G., and Ducklow, H. (2009). Microbial growth in the polar oceans - Role of temperature and potential impact of climate change. Nat. Rev. Microbiol. 7, 451–459. doi: 10.1038/nrmicro2115
Klausmeier, C. A., Litchman, E., Daufresne, T., and Levin, S. (2004). Optimal nitrogen-to-phosphorus stoichiometry of phytoplankton. Nature 429, 171–174. doi: 10.1038/nature02508
Knopf, D. A., Alpert, P. A., and Wang, B. (2018). The role of organic aerosol in atmospheric ice nucleation: a review. ACS Earth Space Chem. 2, 168–202. doi: 10.1021/acsearthspacechem.7b00120
Knopf, D. A., Alpert, P. A., Wang, B., and Aller, J. Y. (2010). Stimulation of ice nucleation by marine diatoms. Nat. Geosci. 4, 88–90. doi: 10.1038/NGEO1037
Korolev, A., McFarquhar, G., Field, P. R., Franklin, C., Lawson, P., and Wang, Z. (2017). “Mixed-Phase Clouds: progress and Challenges,” in Ice Formation and Evolution in Clouds and Precipitation: measurement and Modeling Challenges, et al Edn, eds D. Baumgardner, G. M. McFarquhar, and A. J. Heymsfield (Boston, MA: American MeteorologicalSociety), 5.1–5.50. doi: 10.1175/amsmonographs-d-17-0001.1
Krachler, R., and Krachler, R. F. (2021). Northern high-latitude organic soils as a vital source of river-borne dissolved iron to the ocean. Environ. Sci. Technol. 55, 9672–9690. doi: 10.1021/acs.est.1c01439
Krivácsy, Z., Kiss, G., Ceburnis, D., Jennings, G., Maenhaut, W., Salma, I., et al. (2008). Study of water-soluble atmospheric humic matter in urban and marine environments. Atmos. Res. 87, 1–12. doi: 10.1016/j.atmosres.2007.04.005
Kujawinski, E. B., Longnecker, K., Blough, N. V., Del Vecchio, R., Finlay, L., Kitner, J. B., et al. (2009). Identification of possible source markers in marine dissolved organic matter using ultrahigh resolution mass spectrometry. Geochim. Cosmochim. Acta 73, 4384–4399. doi: 10.1016/j.gca.2009.04.033
Kuznetsova, M., Lee, C., and Aller, J. (2005). Characterization of the proteinaceous matter in marine aerosols. Mar. Chem. 96, 359–377. doi: 10.1016/j.marchem.2005.03.007
Lallement, A., Besaury, L., Tixier, E., Sancelme, M., Amato, P., Vinatier, V., et al. (2018). Potential for phenol biodegradation in cloud waters. Biogeosciences 15, 5733–5744. doi: 10.5194/bg-15-5733-2018
Lawler, M. J., Lewis, S. L., Russell, L. M., Quinn, P. K., Bates, T. S., Coffman, D. J., et al. (2020). North Atlantic marine organic aerosol characterized by novel offline thermal desorption mass spectrometry: polysaccharides, recalcitrant material, and secondary organics. Atmos. Chem. Phys. 20, 16007–16022. doi: 10.5194/acp-20-16007-2020
Leck, C., and Bigg, E. K. (2005). Biogenic particles in the surface microlayer and overlaying atmosphere in the central Arctic Ocean during summer. Tellus B Chem. Phys. Meteorol. 57, 305–316. doi: 10.3402/tellusb.v57i4.16546
Lee, C., Sultana, C. M., Collins, D. B., Santander, M. V., Axson, J. L., Malfatti, F., et al. (2015). Advancing model systems for fundamental laboratory studies of sea spray aerosol using the microbial loop. J. Phys. Chem. A 119, 8860–8870. doi: 10.1021/acs.jpca.5b03488
Lehahn, Y., Koren, I., Schatz, D., Frada, M., Sheyn, U., Boss, E., et al. (2014). Decoupling physical from biological processes to assess the impact of viruses on a mesoscale algal bloom. Curr. Biol. 24, 2041–2046. doi: 10.1016/j.cub.2014.07.046
Liss, P. S., and Duce, R. A. (1997). The Sea Surface and Global Change. Cambridge: Cambridge University Press. doi: 10.1017/CBO9780511525025
Litchman, E., and Klausmeier, C. A. (2008). Trait-based community ecology of phytoplankton. Annu. Rev. Ecol. Evol. Syst. 39, 615–639. doi: 10.1146/annurev.ecolsys.39.110707.173549
Liu, Y., Yvon-Lewis, S. A., Thornton, D. C. O., Butler, J. H., Bianchi, T. S., Campbell, L., et al. (2013a). Spatial and temporal distributions of bromoform and dibromomethane in the Atlantic Ocean and their relationship with photosynthetic biomass. J. Geophys. Res. Oceans 118, 3950–3965. doi: 10.1002/jgrc.20299
Liu, Y., Yvon-Lewis, S. A., Thornton, D. C. O., Campbell, L., and Bianchi, T. S. (2013b). Spatial distribution of brominated very short-lived substances in the eastern Pacific. J. Geophys. Res. Oceans 118, 2318–2328. doi: 10.1002/jgrc.20183
Long, M. S., Keene, W. C., Kieber, D. J., Frossard, A. A., Russell, L. M., Maben, J. R., et al. (2014). Light-enhanced primary marine aerosol production from biologically productive seawater. Geophys. Res. Lett. 41, 2661–2670.
Long, R. A., and Azam, F. (1996). Abundant protein-containing particles in the sea. Aquat. Microb. Ecol. 10, 213–221. doi: 10.3354/ame010213
Longhurst, A., Sathyendranath, S., Platt, T., and Caverhill, C. (1995). An estimate of global primary production in the ocean from satellite radiometer data. J. Plankton Res. 17, 1245–1271. doi: 10.1093/plankt/17.6.1245
Lu, Z., Streets, D. G., Winijkul, E., Yan, F., Chen, Y., Bond, T. C., et al. (2015). Light absorption properties and radiative effects of primary organic aerosol emissions. Environ. Sci. Technol. 49, 4868–4877. doi: 10.1021/acs.est.5b00211
Malfatti, F., Lee, C., Tinta, T., Pendergraft, M. A., Celussi, M., Zhou, Y., et al. (2019). Detection of active microbial enzymes in nascent sea spray aerosol: implications for atmospheric chemistry and climate. Environ. Sci. Technol. Lett. 6, 171–177. doi: 10.1021/acs.estlett.8b00699
Malin, G., Turner, S., Liss, P., Holligan, P., and Harbour, D. (1993). Dimethylsulfide and dimethylsulphoniopropionate in the northeast atlantic during the summer coccolithophore bloom. Deep Sea Res. I 40, 1487–1508. doi: 10.1016/0967-0637(93)90125-m
Manton, I., and Parke, M. (1960). Further observations on small green flagellates with special reference to possible relatives of Chromulina pusilla Butcher. J. Mar. Biol. Assoc. U. K. 39, 275–298.
Marks, R., Górecka, E., McCartney, K., and Borkowski, W. (2019). Rising bubbles as mechanism for scavenging and aerosolization of diatoms. J. Aerosol Sci. 128, 79–88. doi: 10.1016/j.jaerosci.2018.12.003
Marks, R., Kruczalak, K., Jankowska, K., and Michalska, M. (2001). Bacteria and fungi in air over the Gulf of Gdansk and Baltic Sea. J. Aerosol Sci. 32, 237–250. doi: 10.1016/S0021-8502(00)00064-
Marthi, B., Fieland, V. P., Walter, M., and Seidler, R. J. (1990). Survival of bacteria during aerosolization. Appl. Environ. Microbiol. 56, 3463–3467. doi: 10.1128/aem.56.11.3463-3467.1990
Mason, R. H., Si, M., Chou, C., Irish, V. E., Dickie, R., Elizondo, P., et al. (2016). Size-resolved measurements of ice-nucleating particles at six locations in North America and one in Europe. Atmos. Chem. Phys. 16, 1637–1651. doi: 10.5194/acp-16-1637-2016
Matallana-Surget, S., Meador, J. A., Joux, F., and Douki, T. (2008). Effect of the GC content of DNA on the distribution of UVB-induced bipyrimidine photoproducts. Photochem. Photobiol. Sci. 7, 794–801. doi: 10.1039/b719929e
Matrai, P. A., and Keller, M. D. (1993). Dimethylsulfide in a large-scale coccolithophore bloom in the Gulf of Maine. Cont. Shelf Res. 13, 831–843. doi: 10.1016/0278-4343(93)90012-M
Mayol, E., Arrieta, J. M., Jimenez, M. A., Martinez-Asensio, A., Garcias-Bonet, N., Dachs, J., et al. (2017). Long-range transport of airborne microbes over the global tropical and subtropical ocean. Nat. Commun. 8:201. doi: 10.1038/s41467-017-00110-9
Mayol, E., Jimenez, M. A., Herndl, G. J., Duarte, C. M., and Arrieta, J. M. (2014). Resolving the abundance and air-sea fluxes of airborne microorganisms in the North Atlantic Ocean. Front. Microbiol. 5:557. doi: 10.3389/fmicb.2014.00557
McCluskey, C. S., DeMott, P. J., Ma, P. L., and Burrows, S. M. (2019). Numerical representations of marine ice-nucleating particles in remote marine environments evaluated against observations. Geophys. Res. Lett. 46, 7838–7847. doi: 10.1029/2018GL081861
McCluskey, C. S., Hill, T. C. J., Malfatti, F., Sultana, C. M., Lee, C., Santander, M. V., et al. (2017). A dynamic link between ice nucleating particles released in nascent sea spray aerosol and oceanic biological activity during two mesocosm experiments. J. Atmos. Sci. 74, 151–166. doi: 10.1175/JAS-D-16-0087.1
McCluskey, C. S., Hill, T. C. J., Sultana, C. M., Laskina, O., Trueblood, J., Santander, M. V., et al. (2018). A mesocosm double feature: insights into the chemical makeup of marine ice nucleating particles. J. Atmos. Sci. 75, 2405–2423. doi: 10.1175/JAS-D-17-0155.1
Meskhidze, N., Xu, J., Gantt, B., Zhang, Y., Nenes, A., Ghan, S. J., et al. (2011). Global distribution and climate forcing of marine organic aerosol: 1. Model improvements and evaluation. Atmos. Chem. Phys. 11, 11689–11705. doi: 10.5194/acp-11-11689-2011
Michaud, J. M., Thompson, L. R., Kaul, D., Espinoza, J. L., Richter, R. A., Xu, Z. Z., et al. (2018). Taxon-specific aerosolization of bacteria and viruses in an experimental ocean-atmosphere mesocosm. Nat. Commun. 9:2017. doi: 10.1038/s41467-018-04409-z
Mitts, B. A., Wang, X., Lucero, D. D., Beall, C. M., Deane, G. B., DeMott, P. J., et al. (2021). Importance of supermicron ice nucleating particles in nascent sea spray. Geophys. Res. Lett. 48, 1–10. doi: 10.1029/2020GL089633
Miyazaki, Y., Suzuki, K., Tachibana, E., Yamashita, Y., Müller, A., Kawana, K., et al. (2020). New index of organic mass enrichment in sea spray aerosols linked with senescent status in marine phytoplankton. Sci. Rep. 10:17042. doi: 10.1038/s41598-020-73718-5
Miyazaki, Y., Yamashita, Y., Kawana, K., Tachibana, E., Kagami, S., Mochida, M., et al. (2018). Chemical transfer of dissolved organic matter from surface seawater to sea spray water-soluble organic aerosol in the marine atmosphere. Sci. Rep. 8:14861. doi: 10.1038/s41598-018-32864-7
Mochida, M., Kitamori, Y., Kawamura, K., Nojiri, Y., and Suzuki, K. (2002). Fatty acids in the marine atmosphere: factors governing their concentrations and evaluation of organic films on sea-salt particles. J. Geophys. Res. Atmos. 107, AAC1–1–AAC1–10. doi: 10.1029/2001JD001278
Mojica, K. D. A., and Brussaard, C. P. D. (2014). Factors affecting virus dynamics and microbial host-virus interactions in marine environments. FEMS Microbiol. Ecol. 89, 495–515. doi: 10.1111/1574-6941.12343
Moore, E. R., Bullington, B. S., Weisberg, A. J., Jiang, Y., Chang, J., and Halsey, K. H. (2017). Morphological and transcriptomic evidence for ammonium induction of sexual reproduction in Thalassiosira pseudonana and other centric diatoms. PLoS One 12:e0181098. doi: 10.1371/journal.pone.0181098
Moore, E. R., Davie-Martin, C. L., Giovannoni, S. J., and Halsey, K. H. (2020). Pelagibacter metabolism of diatom-derived volatile organic compounds imposes an energetic tax on photosynthetic carbon fixation. Environ. Microbiol. 22, 1720–1733. doi: 10.1111/1462-2920.14861
Mopper, K., Zhou, X., Kieber, R. J., Kieber, D. J., Sikorski, R. J., and Jones, R. D. (1991). Photochemical degradation of dissolved organic carbon and its impact on the oceanic carbon cycle. Nature 353, 60–62. doi: 10.1038/353060a0
Moran, M. A., Kujawinski, E. B., Stubbins, A., Fatland, R., Aluwihare, L. I., Buchan, A., et al. (2016). Deciphering ocean carbon in a changing world. Proc. Natl. Acad. Sci. U. S. A. 113, 3143–3151. doi: 10.1073/pnas.1514645113
Morlon, H., Chuyong, G., Condit, R., Hubbell, S., Kenfack, D., Thomas, D., et al. (2008). A general framework for the distance-decay of similarity in ecological communities. Ecol. Lett. 11, 904–917. doi: 10.1111/j.1461-0248.2008.01202.x
Morris, R. M., Rappé, M. S., Connon, S. A., Vergin, K. L., Siebold, W. A., Carlson, C. A., et al. (2002). SAR11 clade dominates ocean surface bacterioplankton communities. Nature 420, 806–810. doi: 10.1038/nature01240.1
Murphy, K. R., Stedmon, C. A., Waite, T. D., and Ruiz, G. M. (2008). Distinguishing between terrestrial and autochthonous organic matter sources in marine environments using fluorescence spectroscopy. Mar. Chem. 108, 40–58. doi: 10.1016/j.marchem.2007.10.003
Nemergut, D. R., Schmidt, S. K., Fukami, T., O’Neill, S. P., Bilinski, T. M., Stanish, L. F., et al. (2013). Patterns and processes of microbial community assembly. Microbiol. Mol. Biol. Rev. 77, 342–356. doi: 10.1128/mmbr.00051-12
O’Dowd, C., Ceburnis, D., Ovadnevaite, J., Bialek, J., Stengel, D. B., Zacharias, M., et al. (2015). Connecting marine productivity to sea-spray via nanoscale biological processes: phytoplankton dance or death disco? Sci. Rep. 5:14883. doi: 10.1038/srep14883
O’Dowd, C. D., Facchini, M. C., Cavalli, F., Ceburnis, D., Mircea, M., Decesari, S., et al. (2004). Biogenically driven organic contribution to marine aerosol. Nature 431, 676–680. doi: 10.1038/nature02970
O’Dowd, C. D., Langmann, B., Varghese, S., Scannell, C., Ceburnis, D., and Facchini, M. C. (2008). A combined organic-inorganic sea-spray source function. Geophys. Res. Lett. 35, 1–5. doi: 10.1029/2007GL030331
O’Dowd, C. D., Smith, M. H., Consterdine, I. E., and Lowe, J. A. (1997). Marine aerosol, sea-salt, and the marine sulphur cycle: a short review. Atmos. Environ. 31, 73–80. doi: 10.1016/S1352-2310(96)00106-9
O’Malley, M. A., Simpson, A. G. B., and Roger, A. J. (2013). The other eukaryotes in light of evolutionary protistology. Biol. Philos. 28, 299–330. doi: 10.1007/s10539-012-9354-y
Orellana, M. V., Matrai, P. A., Leck, C., Rauschenberg, C. D., Lee, A. M., and Coz, E. (2011). Marine microgels as a source of cloud condensation nuclei in the high arctic. Proc. Natl. Acad. Sci. U. S. A. 108, 13612–13617. doi: 10.1073/pnas.1102457108
Ortega-Retuerta, E., Passow, U., Duarte, C. M., and Reche, I. (2009). Effects of ultraviolet B radiation on (not so) transparent exopolymer particles. Biogeosciences 6, 3071–3080. doi: 10.5194/bg-6-3071-2009
Ovadnevaite, J., Ceburnis, D., Martucci, G., Bialek, J., Monahan, C., Rinaldi, M., et al. (2011). Primary marine organic aerosol: a dichotomy of low hygroscopicity and high CCN activity. Geophys. Res. Lett. 38, 1–5. doi: 10.1029/2011GL048869
Paasche, E. (2001). A review of the coccolithophorid Emiliania huxleyi (Prymnesiophyceae), with particular reference to growth, coccolith formation, and calcification-photosynthesis interactions. Phycologia 40, 503–529. doi: 10.2216/i0031-8884-40-6-503.1
Partensky, F., Blanchot, J., and Vaulot, D. (1999). Differential distribution and ecology of Prochlorococcus and Synechococcus in oceanic waters: a review. Mar. Cyanobacteria 19, 419–434.
Polymenakou, P. N. (2012). Atmosphere: a source of pathogenic or beneficial microbes? Atmosphere 3, 87–102. doi: 10.3390/atmos3010087
Porter, K. G., and Feig, Y. S. (1980). The use of DAPI for identifying and counting aquatic microflora1. Limnol. Oceanogr. 25, 943–948. doi: 10.4319/lo.1980.25.5.0943
Prather, K. A., Bertram, T. H., Grassian, V. H., Deane, G. B., Stokes, M. D., Demott, P. J., et al. (2013). Bringing the ocean into the laboratory to probe the chemical complexity of sea spray aerosol. Proc. Natl. Acad. Sci. U. S. A. 110, 7550–7555. doi: 10.1073/pnas.1300262110
Prospero, J. M., Blades, E., Mathison, G., and Naidu, R. (2005). Interhemispheric transport of viable fungi and bacteria from Africa to the Caribbean with soil dust. Aerobiologia 21, 1–19. doi: 10.1007/s10453-004-5872-7
Quinn, P. K., and Bates, T. S. (2011). The case against climate regulation via oceanic phytoplankton sulphur emissions. Nature 480, 51–56. doi: 10.1038/nature10580
Quinn, P. K., Bates, T. S., Coffman, D. J., Upchurch, L., Johnson, J. E., Moore, R., et al. (2019). Seasonal variations in western North Atlantic remote marine aerosol properties. J. Geophys. Res. Atmos. 124, 14240–14261. doi: 10.1029/2019JD031740
Quinn, P. K., Bates, T. S., Schulz, K. S., Coffman, D. J., Frossard, A. A., Russell, L. M., et al. (2014). Contribution of sea surface carbon pool to organic matter enrichment in sea spray aerosol. Nat. Geosci. 7, 228–232. doi: 10.1038/NGEO2092
Quinn, P. K., Collins, D. B., Grassian, V. H., Prather, K. A., and Bates, T. S. (2015). Chemistry and related properties of freshly emitted sea spray aerosol. Chem. Rev. 115, 4383–4399. doi: 10.1021/cr500713g
Radosevich, J. L., Wilson, W. J., Shinn, J. H., DeSantis, T. Z., and Andersen, G. L. (2002). Development of a high-volume aerosol collection system for the identification of air-borne micro-organisms. Lett. Appl. Microbiol. 34, 162–167. doi: 10.1046/j.1472-765x.2002.01048.x
Rahlff, J. (2019). The Virioneuston: a review on viral-bacterial associations at air-water interfaces. Viruses 11:191. doi: 10.3390/v11020191
Rastelli, E., Corinaldesi, C., Dell’Anno, A., Lo Martire, M., Greco, S., Cristina Facchini, M., et al. (2017). Transfer of labile organic matter and microbes from the ocean surface to the marine aerosol: an experimental approach. Sci. Rep. 7:11475. doi: 10.1038/s41598-017-10563-z
Ravva, S. V., Hernlem, B. J., Sarreal, C. Z., and Mandrell, R. E. (2012). Bacterial communities in urban aerosols collected with wetted-wall cyclonic samplers and seasonal fluctuations of live and culturable airborne bacteria. J. Environ. Monit. 14, 473–481. doi: 10.1039/c1em10753d
Reche, I., D’Orta, G., Mladenov, N., Winget, D. M., and Suttle, C. A. (2018). Deposition rates of viruses and bacteria above the atmospheric boundary layer. ISME J. 12, 1154–1162. doi: 10.1038/s41396-017-0042-4
Renard, P., Canet, I., Sancelme, M., Wirgot, N., Deguillaume, L., and Delort, A. M. (2016). Screening of cloud microorganisms isolated at the Puy de Dôme (France) station for the production of biosurfactants. Atmos. Chem. Phys. 16, 12347–12358. doi: 10.5194/acp-16-12347-2016
Rinaldi, M., Fuzzi, S., Decesari, S., Marullo, S., Santoleri, R., Provenzale, A., et al. (2013). Is chlorophyll-a the best surrogate for organic matter enrichment in submicron primary marine aerosol? J. Geophys. Res. Atmos. 118, 4964–4973. doi: 10.1002/jgrd.50417
Robertson, C. E., Baumgartner, L. K., Harris, J. K., Peterson, K. L., Stevens, M. J., Frank, D. N., et al. (2013). Culture-independent analysis of aerosol microbiology in a metropolitan subway system. Appl. Environ. Microbiol. 79, 3485–3493. doi: 10.1128/AEM.00331-13
Rocap, G., Larimer, F. W., Lamerdin, J., Malfatti, S., Chain, P., and Ahlgren, N. A. (2003). Genome divergence in two Prochlorococcus ecotypes reflects oceanic niche differentiation. Nature 424, 1042–1047. doi: 10.1038/nature01947
Rolph, G., Stein, A., and Stunder, B. (2017). Real-time environmental applications and display system: READY. Environ. Model. Softw. 95, 210–228. doi: 10.1016/j.envsoft.2017.06.025
Romano, S., Di Salvo, M., Rispoli, G., Alifano, P., Perrone, M. R., and Talà, A. (2019). Airborne bacteria in the Central Mediterranean: structure and role of meteorology and air mass transport. Sci. Total Environ. 697:134020. doi: 10.1016/j.scitotenv.2019.134020
Rosinski, J., Haagenson, P. L., Nagamoto, C. T., and Parungo, F. (1987). Nature of ice-forming nuclei in marine air masses. J. Aerosol Sci. 18, 291–309. doi: 10.1016/0021-8502(87)90024-3
Russell, L. M., Bahadur, R., Hawkins, L. N., Allan, J., Baumgardner, D., Quinn, P. K., et al. (2009). Organic aerosol characterization by complementary measurements of chemical bonds and molecular fragments. Atmos. Environ. 43, 6100–6105. doi: 10.1016/j.atmosenv.2009.09.036
Russell, L. M., Hawkins, L. N., Frossard, A. A., Quinn, P. K., and Bates, T. S. (2010). Carbohydrate-like composition of submicron atmospheric particles and their production from ocean bubble bursting. Proc. Natl. Acad. Sci. U. S. A. 107, 6652–6657. doi: 10.1073/pnas.0908905107
Sabbaghzadeh, B., Upstill-Goddard, R. C., Beale, R., Pereira, R., and Nightingale, P. D. (2017). The Atlantic Ocean surface microlayer from 50°N to 50°S is ubiquitously enriched in surfactants at wind speeds up to 13 m s–1. Geophys. Res. Lett. 44, 2852–2858. doi: 10.1002/2017GL072988
Saliba, G., Chen, C. L., Lewis, S., Russell, L. M., Quinn, P. K., Bates, T. S., et al. (2020). Seasonal differences and variability of concentrations, chemical composition, and cloud condensation nuclei of marine aerosol over the North Atlantic. J. Geophys. Res. Atmos. 125, 1–24. doi: 10.1029/2020JD033145
Saliba, G., Chen, C. L., Lewis, S., Russell, L. M., Rivellini, L. H., Lee, A. K. Y., et al. (2019). Factors driving the seasonal and hourly variability of sea-spray aerosol number in the North Atlantic. Proc. Natl. Acad. Sci. U. S. A. 116, 20309–20314. doi: 10.1073/pnas.1907574116
Salter, M. E., Upstill-Goddard, R. C., Nightingale, P. D., Archer, S. D., Blomquist, B., Ho, D. T., et al. (2011). Impact of an artificial surfactant release on air-sea gas fluxes during Deep Ocean Gas Exchange Experiment II. J. Geophys. Res. Oceans 116, 1–9. doi: 10.1029/2011JC007023
Sanchez, K. J., Chen, C. L., Russell, L. M., Betha, R., Liu, J., Price, D. J., et al. (2018). Substantial seasonal contribution of observed biogenic sulfate particles to cloud condensation nuclei. Sci. Rep. 8:3235. doi: 10.1038/s41598-018-21590-9
Santos, A. L., Henriques, I., Gomes, N. C. M., Almeida, A., Correia, A., and Cunha, A. (2011). Effects of ultraviolet radiation on the abundance, diversity and activity of bacterioneuston and bacterioplankton: insights from microcosm studies. Aquat. Sci. 73, 63–77. doi: 10.1007/s00027-010-0160-9
Sattler, B., Puxbaum, H., and Psenner, R. (2001). Bacterial growth in supercooled cloud droplets. Geophys. Res. Lett. 28, 239–242. doi: 10.1029/2000GL011684
Schattenhofer, M., Fuchs, B. M., Amann, R., Zubkov, M. V., Tarran, G. A., and Pernthaler, J. (2009). Latitudinal distribution of prokaryotic picoplankton populations in the Atlantic Ocean. Environ. Microbiol. 11, 2078–2093. doi: 10.1111/j.1462-2920.2009.01929.x
Schiffer, J. M., Luo, M., Dommer, A. C., Thoron, G., Pendergraft, M., Santander, M. V., et al. (2018). Impacts of lipase enzyme on the surface properties of marine aerosols. J. Phys. Chem. Lett. 9, 3839–3849. doi: 10.1021/acs.jpclett.8b01363
Schlichting, H. E. (1969). The importance of airborne algae and protozoa. J. Air Pollut. Control Assoc. 19, 946–951. doi: 10.1080/00022470.1969.10469362
Schmitt-Kopplin, P., Liger-Belair, G., Koch, B. P., Flerus, R., Kattner, G., Harir, M., et al. (2012). Dissolved organic matter in sea spray: a transfer study from marine surface water to aerosols. Biogeosciences 9, 1571–1582. doi: 10.5194/bg-9-1571-2012
Schnell, R. C., and Vali, G. (1976). Biogenic ice nuclei: part I. Terrestrial and marine sources. J. Atmos. Sci. 33, 1554–1564.
Schwier, A. N., Rose, C., Asmi, E., Ebling, A. M., Landing, W. M., Marro, S., et al. (2015). Primary marine aerosol emissions from the Mediterranean Sea during pre-bloom and oligotrophic conditions: correlations to seawater chlorophyll a from a mesocosm study. Atmos. Chem. Phys. 15, 7961–7976. doi: 10.5194/acp-15-7961-2015
Sciare, J., Favez, O., Sarda-Estève, R., Oikonomou, K., Cachier, H., and Kazan, V. (2009). Long-term observations of carbonaceous aerosols in the Austral Ocean atmosphere: evidence of a biogenic marine organic source. J. Geophys. Res. Atmos. 114, 1–10. doi: 10.1029/2009JD011998
Seifried, J. S., Wichels, A., and Gerdts, G. (2015). Spatial distribution of marine airborne bacterial communities. Microbiologyopen 4, 475–490. doi: 10.1002/mbo3.253
Sharma, N. K., and Singh, S. (2010). Differential aerosolization of algal and cyanobacterial particles in the atmosphere. Indian J. Microbiol. 50, 468–473. doi: 10.1007/s12088-011-0146-x
Sharoni, S., Trainic, M., Schatz, D., Lehahn, Y., Flores, M. J., Bidle, K. D., et al. (2015). Infection of phytoplankton by aerosolized marine viruses. Proc. Natl. Acad. Sci. U. S. A. 112, 6643–6647. doi: 10.1073/pnas.1423667112
Shaw, S. L., Chisholm, S. W., and Prinn, R. G. (2003). Isoprene production by Prochlorococcus, a marine cyanobacterium, and other phytoplankton. Mar. Chem. 80, 227–245. doi: 10.1016/S0304-4203(02)00101-9
Shearer, C. A., Descals, E., Kohlmeyer, B., Kohlmeyer, J., Marvanová, L., Padgett, D., et al. (2007). Fungal biodiversity in aquatic habitats. Biodivers. Conserv. 16, 49–67. doi: 10.1007/s10531-006-9120-z
Shupe, M. D., Daniel, J. S., De Boer, G., Eloranta, E. W., Kollias, P., Long, C. N., et al. (2008). A focus on mixed-phase clouds: the status of ground-based observational methods. Bull. Am. Meteorol. Soc. 89, 1549–1562. doi: 10.1175/2008BAMS2378.1
Si, M., Irish, V. E., Mason, R. H., Vergara-Temprado, J., Hanna, S. J., Ladino, L. A., et al. (2018). Ice-nucleating ability of aerosol particles and possible sources at three coastal marine sites. Atmos. Chem. Phys. 18, 15669–15685. doi: 10.5194/acp-18-15669-2018
Sievering, H., Cainey, J., Harvey, M., McGregor, J., Nichol, S., and Quinn, P. (2004). Aerosol non-sea-salt sulfate in the remote marine boundary layer under clear-sky and normal cloudiness conditions: ocean-derived biogenic alkalinity enhances sea-salt sulfate production by ozone oxidation. J. Geophys. Res. Atmos. 109, 1–12. doi: 10.1029/2003JD004315
Smets, W., Moretti, S., Denys, S., and Lebeer, S. (2016). Airborne bacteria in the atmosphere: presence, purpose, and potential. Atmos. Environ. 139, 214–221. doi: 10.1016/j.atmosenv.2016.05.038
Smith, D. J., Jaffe, D. A., Birmele, M. N., Griffin, D. W., Schuerger, A. C., Hee, J., et al. (2012). Free tropospheric transport of microorganisms from Asia to North America. Microb. Ecol. 64, 973–985. doi: 10.1007/s00248-012-0088-9
Smith, D. J., Timonen, H. J., Jaffe, D. A., Griffin, D. W., Birmele, M. N., Perry, K. D., et al. (2013). Intercontinental dispersal of acteria and Archaea by transpacific Winds. Appl. Environ. Microbiol. 79, 1134–1139. doi: 10.1128/AEM.03029-12
Stedmon, C. A., and Markager, S. (2005). Tracing the production and degradation of autochthonous fractions of dissolved organic matter by fluorescence analysis. Limnol. Oceanogr. 50, 1415–1426. doi: 10.4319/lo.2005.50.5.1415
Stein, A. F., Draxler, R. R., Rolph, G. D., Stunder, B. J. B., Cohen, M. D., and Ngan, F. (2015). NOAA’s HYSPLIT atmospheric transport and dispersion modeling system. Bull. Am. Meteorol. Soc. 96, 2059–2077. doi: 10.1175/BAMS-D-14-00110.1
Steindler, L., Schwalbach, M. S., Smith, D. P., Chan, F., and Giovannoni, S. J. (2011). Energy starved Candidatus Pelagibacter Ubique substitutes light-mediated ATP production for endogenous carbon respiration. PLoS One 6:e19725. doi: 10.1371/journal.pone.0019725
Stokes, M. D., Deane, G. B., Prather, K., Bertram, T. H., Ruppel, M. J., Ryder, O. S., et al. (2013). A Marine Aerosol Reference Tank system as a breaking wave analogue for the production of foam and sea-spray aerosols. Atmos. Meas. Tech. 6, 1085–1094. doi: 10.5194/amt-6-1085-2013
Stolle, C., Labrenz, M., Meeske, C., and Jürgens, K. (2011). Bacterioneuston Community Structure in the Southern Baltic Sea and Its Dependence on Meteorological Conditions. Appl. Environ. Microbiol. 77, 3726–3733. doi: 10.1128/AEM.00042-11
Suttle, C. A. (2007). Marine viruses-major players in the global ecosystem. Nat. Rev. Microbiol. 5, 801–812. doi: 10.1038/nrmicro1750
Takemura, T., Nakajima, T., Dubovik, O., Holben, B. N., and Kinne, S. (2002). Single-scattering albedo and radiative forcing of various aerosol species with a global three-dimensional model. J. Clim. 15, 333–352. doi: 10.1175/1520-0442
Tang, J. W. (2009). The effect of environmental parameters on the survival of airborne infectious agents. J. R. Soc. Interface 6, S737–S746. doi: 10.1098/rsif.2009.0227
Taylor, A. R., Brownlee, C., and Wheeler, G. (2017). Coccolithophore cell biology: chalking up progress. Ann. Rev. Mar. Sci. 9, 283–310. doi: 10.1146/annurev-marine-122414-034032
Tesson, S. V. M., and Santl-Temkiv, T. (2018). Ice nucleation activity and aeolian dispersal success in airborne and aquatic microalgae. Front. Microbiol. 9:2681. doi: 10.3389/fmicb.2018.02681
Tesson, S. V. M., Skjøth, C. A., Šantl-Temkiv, T., and Löndahl, J. (2016). Airborne microalgae: insights, opportunities, and challenges. Appl. Environ. Microbiol. 82, 1978–1991. doi: 10.1128/AEM.03333-15
Thornton, D. C. O. (1999). Phytoplankton mucilage production in coastal waters: a dispersal mechanism in a front dominated system? Ethol. Ecol. Evol. 11, 179–185. doi: 10.1080/08927014.1999.9522835
Thornton, D. C. O., Brooks, S. D., and Chen, J. (2016). Protein and carbohydrate exopolymer particles in the sea surface microlayer (SML). Front. Mar. Sci. 3:135. doi: 10.3389/fmars.2016.00135
Tignat-Perrier, R., Dommergue, A., Thollot, A., Keuschnig, C., Magand, O., Vogel, T. M., et al. (2019). Global airborne microbial communities controlled by surrounding landscapes and wind conditions. Sci. Rep. 9:14441. doi: 10.1038/s41598-019-51073-4
Tolić, N., Liu, Y., Liyu, A., Shen, Y., Tfaily, M. M., Kujawinski, E. B., et al. (2017). Formularity: software for automated formula assignment of natural and other organic matter from ultrahigh-resolution mass spectra. Anal. Chem. 89, 12659–12665. doi: 10.1021/acs.analchem.7b03318
Trainic, M., Koren, I., Sharoni, S., Frada, M., Segev, L., Rudich, Y., et al. (2018). Infection dynamics of a bloom-forming alga and its virus determine airborne coccolith emission from seawater. iScience 6, 327–335.
Tseng, R.-S., Viechnicki, J. T., Skop, R. A., and Brown, J. W. (1992). Sea-to-air transfer of surface-active organic compounds by bursting bubbles. J. Geophys. Res. 97, 5201–5206. doi: 10.1029/91jc00954
Uetake, J., Hill, T. C. J., Moore, K. A., DeMott, P. J., Protat, A., and Kreidenweis, S. M. (2020). Airborne bacteria confirm the pristine nature of the Southern Ocean boundary layer. Proc. Natl. Acad. Sci. U. S. A. 117, 13275–13282. doi: 10.1073/pnas.2000134117
Urbano, R., Palenik, B., Gaston, C. J., and Prather, K. A. (2011). Detection and phylogenetic analysis of coastal bioaerosols using culture dependent and independent techniques. Biogeosciences 8, 301–309. doi: 10.5194/bg-8-301-2011
Vali, G. (1971). Quantitative evaluation of experimental results and the heterogeneous freezing nucleation of supercooled liquids. J. Atmos. Sci. 28, 402–409.
Vali, G., DeMott, P. J., Möhler, O., and Whale, T. F. (2015). Technical Note: a proposal for ice nucleation terminology. Atmos. Chem. Phys. 15, 10263–10270. doi: 10.5194/acp-15-10263-2015
Velculescu, V. E., Zhang, L., Vogelstein, B., and Kinzler, K. W. (1995). Serial analysis of gene expression. Science 270, 484–487. doi: 10.1126/science.270.5235.484
Veron, F. (2015). Ocean Spray. Annu. Rev. Fluid Mech. 47, 507–538. doi: 10.1146/annurev-fluid-010814-014651
Vignati, E., Facchini, M. C., Rinaldi, M., Scannell, C., Ceburnis, D., Sciare, J., et al. (2010). Global scale emission and distribution of sea-spray aerosol: sea-salt and organic enrichment. Atmos. Environ. 44, 670–677. doi: 10.1016/j.atmosenv.2009.11.013
Wallace, J. M., and Hobbs, P. V. (2006). Atmospheric Science: an Introductory Survey. Second Edition. Burlington, MA: Academic Press.
Walter, M. V., Marthi, B., Fieland, V. P., and Ganio, L. M. (1990). Effect of aerosolization on subsequent bacterial survival. Appl. Environ. Microbiol. 56, 3468–3472. doi: 10.1128/aem.56.11.3468-3472.1990
Wang, X., Deane, G. B., Moore, K. A., Ryder, O. S., Stokes, M. D., Beall, C. M., et al. (2017). The role of jet and film drops in controlling the mixing state of submicron sea spray aerosol particles. Proc. Natl. Acad. Sci. U. S. A. 114, 6978–6983. doi: 10.1073/pnas.1702420114
Wang, X., Sultana, C. M., Trueblood, J., Hill, T. C. J., Malfatti, F., Lee, C., et al. (2015). Microbial control of sea spray aerosol composition: a tale of two blooms. ACS Cent. Sci. 1, 124–131. doi: 10.1021/acscentsci.5b00148
Watson, S. W., Novitsky, T. J., Quinby, H. L., and Valois, F. W. (1977). Determination of bacterial number and biomass in the marine environment. Appl. Environ. Microbiol. 33, 940–946. doi: 10.1128/aem.33.4.940-946.1977
Westberry, T., Behrenfeld, M. J., Siegel, D. A., and Boss, E. (2008). Carbon-based primary productivity modeling with vertically resolved photoacclimation. Global Biogeochem. Cycles 22, 1–18. doi: 10.1029/2007GB003078
Whitman, W. B., Coleman, D. C., and Wiebe, W. J. (1998). Prokaryotes: the unseen majority. Proc. Natl. Acad. Sci. U. S. A. 95, 6578–6583. doi: 10.1073/pnas.95.12.6578
Wilbourn, E. K., Thornton, D. C. O., Ott, C., Graff, J., Quinn, P. K., Bates, T. S., et al. (2020). Ice nucleation by marine aerosols over the North Atlantic Ocean in late spring. J. Geophys. Res. Atmos. 125, 1–17. doi: 10.1029/2019JD030913
Wilhelm, S. W., and Suttle, C. A. (1999). Viruses and nutrient cycles in the sea. Bioscience 49, 781–788. doi: 10.2307/1313569
Wilkins, D., Van Sebille, E., Rintoul, S. R., Lauro, F. M., and Cavicchioli, R. (2013). Advection shapes Southern Ocean microbial assemblages independent of distance and environment effects. Nat. Commun. 4:2457. doi: 10.1038/ncomms3457
Wilson, T. W., Ladino, L. A., Alpert, P. A., Breckels, M. N., Brooks, I. M., Browse, J., et al. (2015). A marine biogenic source of atmospheric ice-nucleating particles. Nature 525, 234–238. doi: 10.1038/nature14986
Wolf, M. J., Coe, A., Dove, L. A., Zawadowicz, M. A., Dooley, K., Biller, S. J., et al. (2019). Investigating the heterogeneous ice nucleation of sea spray aerosols using Prochlorococcus as a model source of marine organic matter. Environ. Sci. Technol. 53, 1139–1149. doi: 10.1021/acs.est.8b05150
Womack, A. M., Bohannan, B. J. M., and Green, J. L. (2010). Biodiversity and biogeography of the atmosphere. Philos. Trans. R. Soc. B Biol. Sci. 365, 3645–3653. doi: 10.1098/rstb.2010.0283
Wurl, O., Wurl, E., Miller, L., Johnson, K., and Vagle, S. (2011). Formation and global distribution of sea-surface microlayers. Biogeosciences 8, 121–135. doi: 10.5194/bg-8-121-2011
Xia, X., Wang, J., Ji, J., Zhang, J., Chen, L., and Zhang, R. (2015). Bacterial communities in marine aerosols revealed by 454 pryrosequencing of the 16S rRNA gene. J. Atmos. Sci. 72, 2997–3008. doi: 10.1175/JAS-D-15-0008.1
Yamashita, Y., and Tanoue, E. (2008). Production of bio-refractory fluorescent dissolved organic matter in the ocean interior. Nat. Geosci. 1, 579–582. doi: 10.1038/ngeo279
Yoon, Y. J., Ceburnis, D., Cavalli, F., Jourdan, O., Putaud, J. P., Facchini, M. C., et al. (2007). Seasonal characteristics of the physicochemical properties of North Atlantic marine atmospheric aerosols. J. Geophys. Res. Atmos. 112:D04206. doi: 10.1029/2005JD007044
Yooseph, S., Andrews-Pfannkoch, C., Tenney, A., McQuaid, J., Williamson, S., Thiagarajan, M., et al. (2013). A metagenomic framework for the study of airborne microbial communities. PLoS One 8:e81862. doi: 10.1371/journal.pone.0081862
Zeppenfeld, S., Van Pinxteren, M., Van Pinxteren, D., Wex, H., Berdalet, E., Vaqué, D., et al. (2021). Aerosol marine primary carbohydrates and atmospheric transformation in the Western Antarctic Peninsula. ACS Earth Space Chem. 5, 1032–1047. doi: 10.1021/acsearthspacechem.0c00351
Keywords: aerobiota, biogenic aerosol, microbial oceanography, cloud condensation nuclei (CCN), ice nucleating particles (INPs), sea spray aerosol (SSA), atmospheric dispersal, air-sea interaction
Citation: Alsante AN, Thornton DCO and Brooks SD (2021) Ocean Aerobiology. Front. Microbiol. 12:764178. doi: 10.3389/fmicb.2021.764178
Received: 25 August 2021; Accepted: 27 September 2021;
Published: 29 October 2021.
Edited by:
Angelica Bianco, UMR 6016, Laboratoire de Météorologie Physique (LAMP), FranceReviewed by:
Francesca Malfatti, University of Trieste, ItalyMarco J. L. Coolen, Curtin University, Australia
Copyright © 2021 Alsante, Thornton and Brooks. This is an open-access article distributed under the terms of the Creative Commons Attribution License (CC BY). The use, distribution or reproduction in other forums is permitted, provided the original author(s) and the copyright owner(s) are credited and that the original publication in this journal is cited, in accordance with accepted academic practice. No use, distribution or reproduction is permitted which does not comply with these terms.
*Correspondence: Daniel C. O. Thornton, ZHRob3JudG9uQHRhbXUuZWR1