- Departamento de Microbiología Molecular, Instituto de Biotecnología, Universidad Nacional Autónoma de México, Cuernavaca, Mexico
Bacillus thuringiensis (Bt) are soil ubiquitous bacteria. They produce a great variability of insecticidal proteins, where certain of these toxins are used worldwide for pest control. Through their adaptation to diverse ecosystems, certain Bt strains have acquired genetic mobile elements by horizontal transfer, harboring genes that encode for different virulent factors and pesticidal proteins (PP). Genomic characterization of Bt strains provides a valuable source of PP with potential biotechnological applications for pest control. In this work, we have sequenced the complete genome of the bacterium Bt GR007 strain that is toxic to Spodoptera frugiperda and Manduca sexta larvae. Four replicons (one circular chromosome and three megaplasmids) were identified. The two largest megaplasmids (pGR340 and pGR157) contain multiple genes that codify for pesticidal proteins: 10 cry genes (cry1Ab, cry1Bb, cry1Da, cry1Fb, cry1Hb, cry1Id, cry1Ja, cry1Ka, cry1Nb, and cry2Ad), two vip genes (vip3Af and vip3Ag), two binary toxin genes (vpa2Ac and vpb1Ca), five genes that codify for insecticidal toxin components (Tc’s), and a truncated cry1Bd-like gene. In addition, genes that codify for several virulent factors were also found in this strain. Proteomic analysis of the parasporal crystals of GR007 revealed that they are composed of eight Cry proteins. Further cloning of these genes for their individual expression in Bt acrystalliferous strain, by means of their own intrinsic promoter showed expression of seven Cry proteins. These proteins display differential toxicity against M. sexta and S. frugiperda larvae, where Cry1Bb showed to be the most active protein against S. frugiperda larvae and Cry1Ka the most active protein against M. sexta larvae.
Introduction
The Bacillus cereus sensu lato group encompasses several ubiquitous, endospore-forming Gram-positive bacteria including B. cereus, B. anthracis, and Bacillus thuringiensis (Bt). Despite their close phylogenetic relationship, their pathogenic properties are contrasting, since B. anthracis causes anthrax disease, B. cereus produces food poisoning in mammals, and Bt is a well-known pathogen of invertebrates (Helgason et al., 2000). Phylogenetic analysis showed that among the species of B. cereus sensu lato group, B. cereus and Bt are the closest bacteria. The main differences between them are found in their mobile genetic elements (MGE), such as insertion sequences, prophages, and plasmids. It was proposed that the acquisition of such MGE in B. cereus and Bt was facilitated by the absence of a functional CRISPR-Cas systems in many of the B. cereus sensu lato strains, allowing incorporation of novel genetic elements that may help for their better adaptation to diverse ecological niches (Zheng et al., 2020).
Due to their insecticidal properties, Bt have been exploited worldwide as biological pesticides for the control of multiple insect pests (Sanahuja et al., 2011). These bacteria are characterized by the production of pesticidal proteins (PPs) during their sporulation phase of growth. Most of these PPs accumulate as parasporal inclusions within the mother cell compartment and have been recently renamed in the Bacterial Pesticidal Protein Resource Center (BPPRC1) as follows: PPs belonging to the three domain crystal proteins retained the mnemonic name of Cry, while proteins related to Cyt family retained the mnemonic name of Cyt. PPs with homology to Etx-Mtx2 family are now named Mpp. The PPs with homology to aegerolysin are Gpp. Those with homology to the Toxin10/Bin family received the name of Tpp and the PPs with a predominantly alpha helical structure are named App (Crickmore et al., 2020). In addition, some Bt bacteria also produce and secrete additional PPs during their vegetative growth, such as proteins related to the vegetative insecticidal protein Vip3 that retained the mnemonic name of Vip, while the proteins related to the catalytic component of Vip2 are now named Vpa, and the PPs related to the Vip1 binding partner from the binary toxin are now recognized as Vpb (Crickmore et al., 2020). All these proteins are encoded in large megaplasmids as individual genes or grouped in pathogenic islands (PAI), accompanied by repeat sequences, insertion elements, and transposases, which may allow a higher recombination rate among diverse Bt strains (Mahillon and Chandler, 1998; Fiedoruk et al., 2017).
In searching novel PPs produced by Bt, that could be useful for pest control in agriculture or farming activities, a large number of Bt strains have been isolated from insect corpses, soil, and phylloplane. Characterization of such strains is not an easy task. Probably, the faster and low-cost approach to screen insecticidal toxins from a Bt strain collection is the traditional PCR strategy, either using a set of universal primers or designed specific primers (Cerón et al., 1994; Cerón et al., 1995; Porcar and Juárez-Pérez, 2003). In addition, also DNA-hybridization and DNA-microarray techniques were shown to be useful for the identification of specific cry genes (Beard et al., 2001; Letowski et al., 2005). However, these DNA-based techniques are limited to detect genes that were previously identified and lack information regarding to their expression. Proteomic analysis of parasporal crystal is more accurate to determine the presence and abundance of PPs expressed in specific Bt crystal inclusions, and such studies have been performed to identify the PPs produced by Bt subsp galleriae VKPMB-1757 and wuhanensis VKPMB-1226 strains (Chestukhina et al., 1994). Recently the advances in sequence strategies have shown that compete genome sequencing is the most efficient strategy to characterize interesting Bt strains and to discover novel PPs. Multiple Bt strains have already been characterized by using Illumina or PacBio strategies in order to obtain their complete genome sequence (Doggett et al., 2013; Liu et al., 2013; Palma et al., 2014; Gao et al., 2015; Cao et al., 2018).
Spodoptera frugiperda is a maize and rice insect pest that has become important worldwide, since this pest has recently migrated from America to Africa and Asia (Sun et al., 2021). This insect shows low susceptibility to Cry1Ac toxin from Bt but it is efficiently controlled with Cry1Fa toxin (Blanco et al., 2010). However, some populations of S. frugiperda have already evolved resistance to Cry1Fa-maize in different countries (Storer et al., 2012; Banerjee et al., 2017; Boaventura et al., 2020). Thus, the identification of additional toxins with high toxicity and no cross-resistance to Cry1Fa is needed to provide effective alternative tools for efficient control of this insect pest. Bt strain GR007 is a strain from our Bt strain collection toxic for lepidopteran insects including S. frugiperda. However, the identity of the PP in GR007 remains unknown. In this work, we have combined third-generation sequencing to obtain the complete genome of GR007 strain and used MS/MS proteomic analysis of its parasporal crystal characterization, in order to identify the expressed proteins. The Bt GR007 genome consists of four replicons: a circular chromosome and three extrachromosomal megaplasmids. Our analysis showed that the two large plasmids harbor multiple PPs arranged in PAIs. We have identified 20 genes encoding for PPs: 10 Cry proteins, two Vip proteins, two proteins that act as binary toxins (Vpa-Vpb), one Mpp protein and a cluster of five genes for insecticidal toxin components (Tc’s) that were originally described in Photorhabdus luminescens (Waterfield et al., 2001).
According to our proteomic analysis, the crystal inclusion of this strain is confirmed by eight Cry proteins. The genes for these Cry proteins were cloned to analyze their individual expression in Bt cells. Only seven Cry proteins were able to form parasporal crystals when expressed in Bt and all of them displayed insecticidal activity against to at least one lepidopteran species. Our work shows that combining these two approaches, whole genome sequence and LC-MS proteomic analysis of crystal inclusions, allows a complete characterization of novel Bt strains containing PPs with potential biotechnological applications.
Materials and Methods
Genomic DNA Purification
Bt GR007 strain was obtained from a recent screening of soil samples from the state of Morelos, Mexico, and selected for its toxicity against S. frugiperda larvae. Bt GR007 was cultured in LB liquid medium for 12 h at 30°C and bacteria were harvested by centrifugation. Genomic DNA was purified using AxyPrep Bacterial genomic DNA miniprep kit from Axygen (Corning Life Sciences, Glendale AZ) following the manufacturer’s instructions. DNA was analyzed by electrophoresis in agarose gel and quantified in NanoDrop 2000 spectrophotometer (Thermo Scientific). The 20 kb templates used for sequencing were prepared by using Blue Pippin Size-Selection System (Sage Science, Beverly, MA) that allows resolving and collecting high molecular weight DNA, according to the manufacturer’s instructions.
DNA Sequencing and Assembly
The genomic sequence of Bt GR007 was obtained by Beijing Sinobiocore Biological Technology Co., using PacBio RSII platform. A total of 138,274 reads were obtained which represent 928,053,411 bp and total 151X fold coverage of the genome. All reads were assembled by using the Single Molecule, Real-Time (SMRT) sequencing data SMRTPipe v 2.3.0 from PacBio.2 Annotation of coding sequences (CDS), ribosomal RNA (rRNA), transfer RNA (tRNA), and miscellaneous RNA (miscRNA) were performed using Prokka v1.11 software (Seemann, 2014). The identity of CDS was searched using SwissProt and BLAST (nr/nt) databases. Classification of protein in Clusters of Orthologous Groups (COG) was performed using eggNOG-mapper (Huerta-Cepas et al., 2017). Insertion sequences were identified using ISFinder database (Siguier et al., 2006). Identification of ProPhages regions was performed using Phage_Finder (Fouts, 2006). Identification of CRISPR arrays were predicted using PILERCR v1.06 software (Edgar, 2007). Circular genome maps and plasmid comparison were generated using GView server (Petkau et al., 2010).
Construction of Single Nucleotide Polymorphism Phylogenomic Tree
In this work we selected to work with the reported assembled genomes from different Bt strains that were found in the NCBI database. In this database we found genomic information of 670 Bt strains. However, only 63 genomes and 21 chromosomes are completely assembled. After taking out sequences that were duplicated, we downloaded 73 completely assembled genomes from this database. We used the Parsnp tool from the Harvest Suite software for fast multiple alignment of genomic sequences based on single nucleotide polymorphism (SNP) (Treangen et al., 2014). We ran Parsnp version 1.2 with the -c parameter. The phylogenomic tree was visualized and stylized in iTOL server (Letunic and Bork, 2019).
Cloning of Cry Toxins
With the aim to clone the regulatory and terminator regions of each cry gene, PCR oligonucleotides were designed to amplify a fragment containing the cry gene flanked by ∼450 bp at 5′ and 3′ ends (Table 1). All PCR were performed with Phusion HD DNA Polymerase (Thermo Scientific Fisher) using as template the genomic DNA from Bt GR007 strain. The cry genes were cloned into pHT315 shuttle vector (Arantes and Lereclus, 1991) using In-Phusion HD Cloning Kit (Takara, Shiga, Japan) following the manufacturer’s instruction. Briefly, pHT315 linearized with SmaI endonuclease was fused with the PCR fragments using In-Phusion Kit enzyme premix during 15 min at 50°C, and the cloning reaction was electrotransformed into E. coli DH5α cells. Transformant cells were analyzed by colony PCR and plasmids of positive clones were purified and further sequenced.
Parasporal Crystal Production
Plasmids harboring cry genes were electrotransformed into the acrystalliferous Bt strain 407, as previously described (Macaluso and Mettus, 1991). Bt strains were grown during 72 h at 30°C in HCT medium (Lecadet et al., 1980) supplemented with 10 μg/ml erythromycin. After sporulation, spore/crystal mixture was recovered and washed three times with wash solution (300 mM NaCl, 10 mM EDTA) and three times with 1 mM PMSF. After these washing steps the spore/crystal mixture was then suspended in ddH20. These samples were directly suspended in SDS-PAGE Laemmli buffer, heated 3 min at 100°C, and analyzed by 10% SDS-PAGE. Protein concentration of the final spore/crystal mixtures suspended in ddH20 was estimated by Bradford method using a BSA standard curve as reference.
Liquid Chromatography-Mass Spectrometry Analysis
Spore/crystal inclusion suspensions of Bt GR007 were mixed in Laemmli loading buffer, boiled for three min, and proteins were separated on a 10% SDS-PAGE. Protein bands were excised, digested “in gel” with trypsin, and desalted. Cleaved peptides were subsequently analyzed by Liquid Chromatography-Mass Spectrometry (LC-MS) system composed of a nanoflux pump EASY-nLC II and mass spectrophotometer LTQ-Orbitrap Velos (Thermo Fisher) at the proteomic facility of the Institute of Biotechnology from National Autonomous University of Mexico. For protein identification, data were screened against the Cry and Vip protein sequences obtained from genome sequencing of this work using the Proteome Discoverer software. A minimum False Discovery Rate (FDR) of 0.01 y maximum FDR of 0.05 were used for peptide identification.
Bioassays
Manduca sexta and Spodoptera frugiperda insect colonies were reared on artificial diets (Bell and Joachim, 1976; Villegas-Mendoza and Rosas-García, 2013), under controlled conditions of relative humidity (70–80%), temperature (25 ± 2°C) and photoperiod (10 h/14 h, light/dark). Bioassays were performed with neonate larvae using six different doses of the spore/crystal mixture that were poured on the surface of the diet. For bioassays against M. sexta larvae we used the following concentrations: 70, 35, 17.5, 8.7, 4.3, and 2.1 ng/cm2. For bioassays against S. frugiperda larvae we used the following concentrations: 2000, 1000, 500, 250, 125, and 62 ng/cm2. The Cry1Ka y Cry1Hb proteins were tested up to 5000 ng/cm2. The negative control was water added to the surface of the diet. We used 24 well polystyrene plates and one plate was used per each concentration of spore/crystal dose in triplicate (a total of 72 larvae were used per toxin concentration in each repetition, thus in each bioassay 432 larvae were analyzed for the 6 different concentrations of spore/crystal mixtures and 72 larvae for the negative control). Mortality was analyzed after 7 days and the 50% lethal concentration (LC50) was calculated with Probit LeOra software. Mortality of the control was lower than 5%. Complete bioassays with three technical replicates were performed in duplicate (864 larvae in total per bioassay performed with each toxin).
Results
Genome and Phylogenetic Analysis of Bt GR007
Bt GR007 strain was selected due to its toxicity against S. frugiperda during preliminary screening assays compared to other native strains. In order to gain access to the gene sequences of potential PP in Bt GR007 strain, the whole genome of this strain was obtained and assembled (BioProject: PRJNA736034). The genome of this strain consists of four replicons, the chromosome and three plasmids (Figure 1A). The circular chromosome contains 5,659,016 bp with 36.2% GC content (Gene Bank Accession number CP076539). A total number of 5,714 CDS were predicted, from which 4,746 proteins were identified using the database of SwissProt and BLAST analysis. The predicted proteins were further classified with COG functional assignation using eggNOG database. The chromosome also contains 30 rRNAs (10 23S, 10 16S, and 10 5S), 104 tRNAs, 145 miscRNAs, and one tmRNA. DNA regions related with genetic mobility were found along the chromosome, among these we found seven large prophage regions and 89 insertion sequences for transposases (Tnp). In agreement with most of the Bt strains that have been sequenced previously, Bt GR007 lacks a functional CRISPR system (Zheng et al., 2020; Table 2).
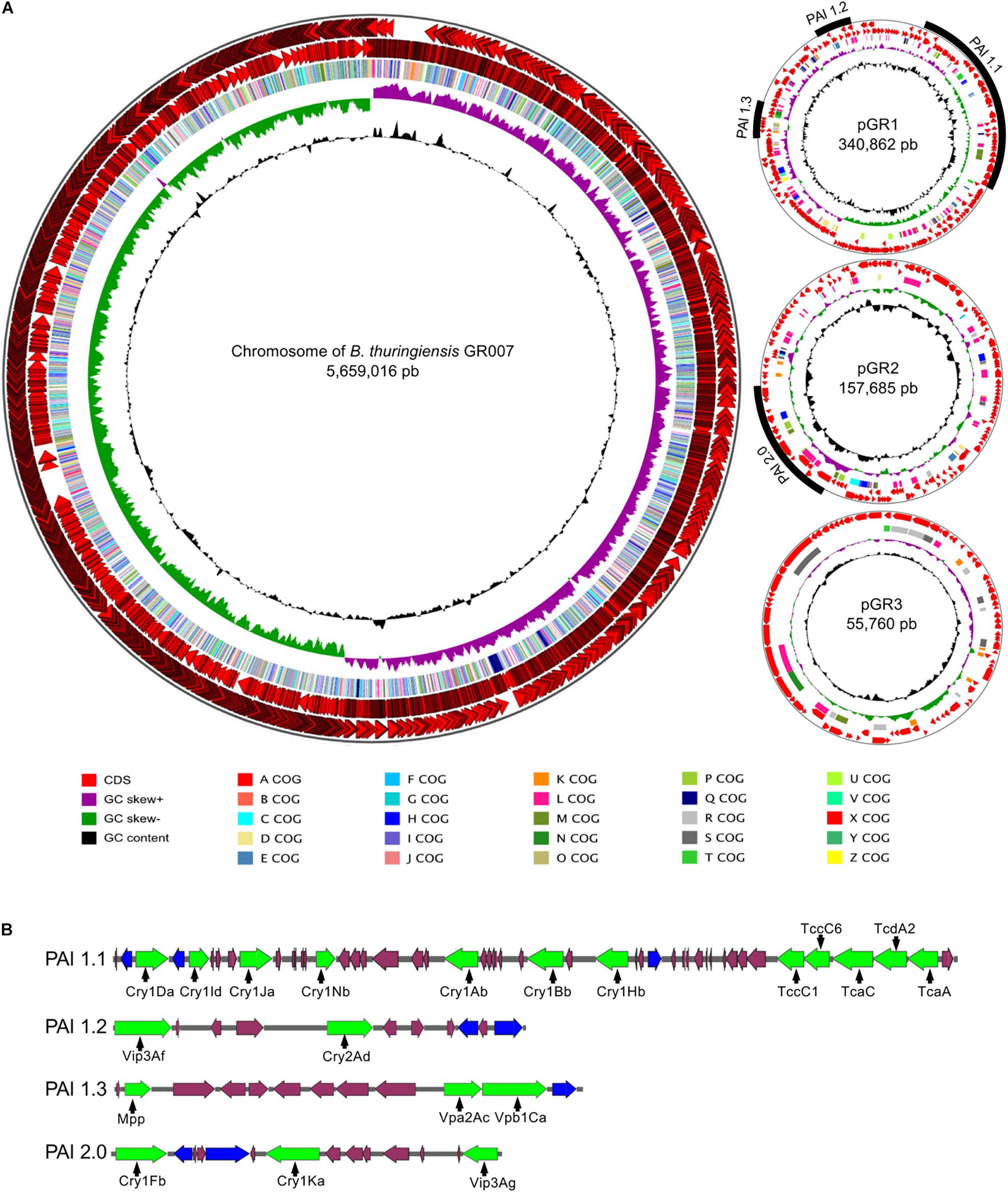
Figure 1. Genome of B. thuringiensis strain GR007. (A) Circular maps of chromosome and plasmids. The distribution of circles from outside to inside indicate CDS forward, CDS reverse, COG functional assignation, GC skew, GC content. The pathogenic islands (PAI) identified are indicated in the plasmids pGR340 and pGR157. (B) Representation of PAIs. Pesticidal proteins are represented in green arrows, transposases in blue arrows and additional ORF into the PAIs with purple arrows.
The three extrachromosomal plasmids pGR340, pGR157, and pGR55 consist of 340,862, 157,685, and 55,760 bp, respectively (Figure 1A) (Gene Bank accession numbers CP076541, CP076540, and CP076542). The two largest plasmids, pGR340 and pGR157, harbor the genes that encode for potential PPs, which are grouped in PAIs, and their annotation was done according to the new database of PPs (see text footnote 1). The plasmid pGR340 contains three PAIs designed as PAI-1.1, PAI-1.2, and PAI-1.3 (Figure 1B). The length of PAI-1.1 is 88.2 kbp and contains seven cry genes encoding for lepidopteran specific Cry proteins (Cry1Da, Cry1Id, Cry1Ja, Cry1Nb, Cry1Ab, Cry1Bb, and Cry1Hb) and a cluster of five genes codifying for insecticidal toxin components (Tcs) (TccC1, TccC6, TcaC, TcdA2, and TcaA). The PAI-1.2 of 17.3 kbp contains two genes encoding for PPs, named Vip3Af and Cry2Ad. The PAI-1.3 with a length of 16.5 kbp has an homologous gene that codifies for a protein related to the Etx/Mtx2 toxin family (recently designed as Mpp). This PAI-1.3 island also contains genes that codify for the binary toxins Vpa2Ac and Vpb1Ca (old names were Vip2Ac and Vip1Ca, respectively) arranged in an operon. The plasmid pGR157 contains a single PAI named PAI2.0 of 26.5 kbp that contains two genes encoding for Cry proteins (Cry1Fb and Cry1Ka) and one gene encoding for a Vip protein (Vip3Ag). In addition, pGR157 contains a gene for a non-functional truncated Cry1Bd-like protein since it lacks the N-terminal region that is present in the Cry1Bd protein (NCBI Acc. No AAD10292). All PAIs were associated with Tnp’s (displayed as blue arrows in Figure 1B). Supplementary File 1 contains the detailed information of Bt GR007 genome annotation.
Bt encodes a large number of virulence-associated genes. We searched for these genes and found that most of them were found in the chromosome of GR007 strain (Table 3), while some virulence factors were found in PAI 1.1 such as cytolysin operon (hblL2, hblL1, and hblB), the phospholipase C (plc-6), subtilisin (sub-8), the cell wall binding/hydrolysis protein (scwl-9), and internalin (inlA-5) (Table 3). The PAI2.0 island also codifies for a cell wall hydrolase (cwlJ-3) (Table 3).
To investigate the phylogenetic relationship of Bt GR007 strain with other previously sequenced Bt strains, we constructed a phylogenomic tree based on SNP of their circular chromosomes (Figure 2). Figure 2 shows that Bt GR007 strain was grouped in a clade of Bacillus strains that kill insects and nematodes, such as Bt subsp. israelensis (Dipteran-specific), Bt subsp. morrisoni BGSC 4AA1 (Colepteran-specific), and Bt YBT-1518 (Nematoda-specific). However, the overall structure of this phylogenomic tree showed a low phylogenetic correlation among the linage of Bt GR007 with other well characterized Lepidopteran-specific Bt strains, such as the HD1, HD73, and YBT1520 strains that were grouped in a different branch. Based on the data of the phylogenomic tree, the closest Bt strain to GR007 was Bt HD12 strain. The Cry proteins content of Bt GR007 is similar to Bt HD12 (BioProject: PRJNA302106), with the exception that the latter strain lacks cry1Fb and vip3Ag genes. In addition, the number of plasmids is different between these two strains, since Bt HD12 contains six plasmids (named as pHD120017, pHD120038, pHD120039, pHD120112, pHD120161, and pHD120345) (Gene Bank Accession Numbers CP014848.1 to CP014853.1). A detailed analysis of the plasmids present in these two strains showed that pGR340 from GR007 strain was essentially identical to pHD120345 from HD12 strain (AN CP014853.1) and contains the same number of the PAIs, while pGR157 and pGR55 from GR007 strain matched with pHD120161 from HD12 strain (AN CP014852.1), suggesting a recombination of these two plasmids in Bt GR007 to form a single plasmid lacking cry1Fb and vip3Ag genes (pHD120161) in Bt HD12 strain (Supplementary Figure 1).
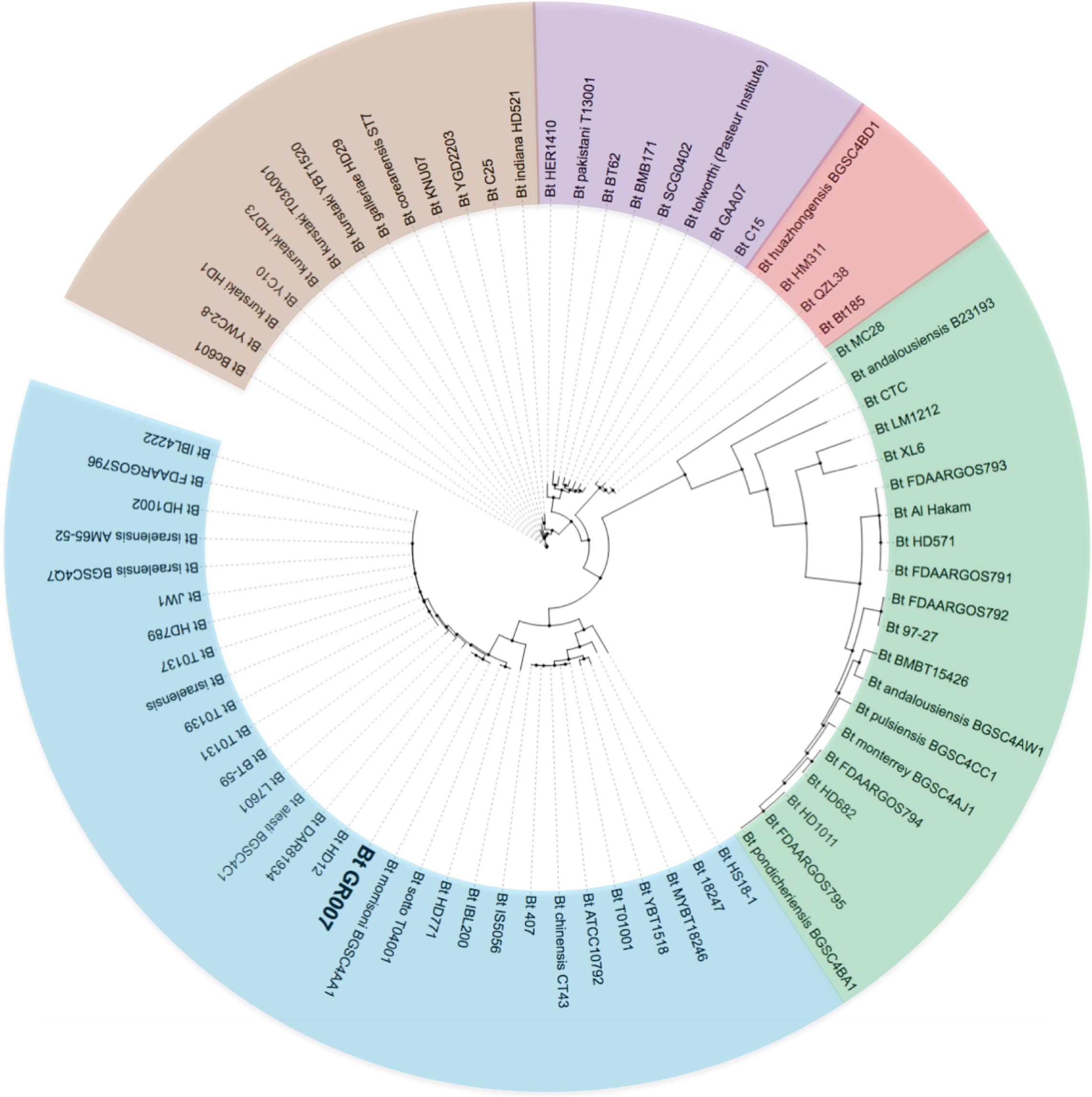
Figure 2. Unrooted phylogenomic tree based on SNP of core genomes of GR007 and other 73 B. thuringiensis strains from NCBI that were reported as assembled genomes. Clades are represented with blue, green, red, purple, and brown colors.
Proteomic Analysis of the Proteins Expressed in the Crystal Inclusion of GR007
A proteomic analysis was performed to analyze the PP’s composition of the parasporal crystal produced by Bt GR007 strain. The strain was grown until the sporulation phase and parasporal crystals were separated by SDS-PAGE. Proteins with apparent molecular weight of 130, 95, 72, and 32 kDa, which correspond to the molecular weight of PPs identified in the megaplasmids, were excised directly from the gel after Coomassie blue staining (Figure 3, lane Bt GR007) and analyzed by LC-MS analysis. Peptides identified by LC-MS matched to eight Cry proteins (Cry1Ab, Cry1Bb, Cry1Da, Cry1Fb, Cry1Hb, Cry1Ja, Cry1Ka, and Cry1Nb) showing high sequence coverage in the 130 and 72 kDa bands, that correspond to protoxin and activated toxin, respectively (Table 4).
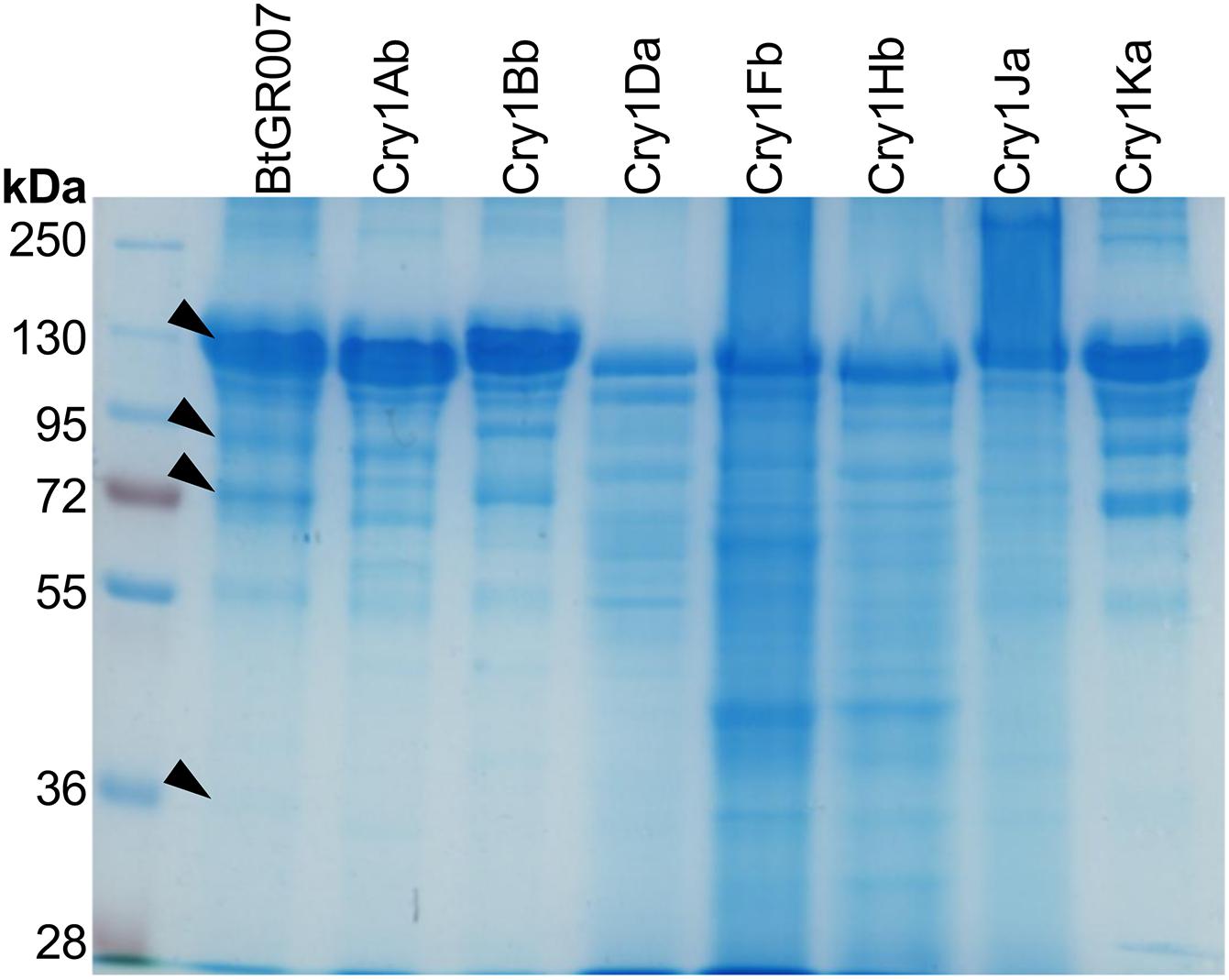
Figure 3. SDS-PAGE of parasporal crystal proteins. Proteins of Bt GR007 analyzed by LC-MS are labeled with black arrows. The cry genes codifying for Cry1Ab, Cry1Bb, Cry1Da, Cry1Fb, Cry1Hb, Cry1Ja, and Cry1Ka proteins were cloned in pHT315 shutter vector and electrotransformed into acrystalliferous Bt 407 strain. All transformant Bt strains were grown until sporulation and the spore/crystal mixture of these strains were analyzed by SDS-PAGE. Cry1Ab, Cry1Bb, Cry1Da, Cry1Fb, Cry1Hb, Cry1Ja, and Cry1Ka correspond to the protein profiles observed in each one of these Bt transformant strains.
Characterization of Pesticidal Cry Proteins
To further analyze the Cry proteins expressed in Bt GR007, we cloned all cry genes identified in this strain to evaluate their toxicity when expressed in the acrystalliferous Bt strain 407. The cry genes codifying for Cry1Ab, Cry1Bb, Cry1Da, Cry1Fb, Cry1Hb, Cry1Ja, Cry1Ka, and Cry1Nb proteins were identified in the pGR340 and pGR157 megaplasmids. Oligonucleotides were designed to amplify via PCR the complete gene including a DNA fragment approximately 450 bp upstream of the start codon of the gene, and 450 bp after the stop codon. We expected that these DNA fragments should contain the intrinsic promotor and terminator regions from each cry gene for their individual expression. These DNA fragments were cloned in pHT315 shutter vector and electrotransformed into acrystalliferous Bt 407 strain. All transformant Bt strains were grown until sporulation and the spore/crystal mixtures were analyzed by SDS-PAGE. Cry1Ab, Cry1Bb, Cry1Da, Cry1Fb, Cry1Hb, Cry1Ja, and Cry1Ka proteins were successfully produced (Figure 3). The Cry1Id and Cry2Ad that were not identified in the parasporal crystal were also cloned, but these proteins were not found as inclusion bodies when transformed into Bt 407 (Supplementary Figure 2).
We analyzed the promotor region of these genes, the upstream nucleotide sequence was aligned with the cry1Ab promoter region that is depend in SigK or SigE factors for its expression. The promotor regions of cry1Da, cry1Ja, cry1Bb, cry1Hb, cry1Fb, and cry1Ka are similar to cry1Ab, while promotor regions from cry1Nb, cry1Id, and cry2Ad showed different sequences, suggesting that their regulation is not dependent on SigE and SigK factors (Supplementary Figure 3).
The toxicity of GR007 strain was tested against two lepidopteran insects, S. frugiperda an M. sexta. We also assayed insecticidal activity against the dipteran insect, Aedes aegypti. Toxicity of this strain was confirmed only for the two lepidopteran larvae while no toxicity was observed against dipteran insects (data not shown). Finally the toxicity of the individual Cry1Ab, Cry1Bb, Cry1Da, Cry1Fb, Cry1Hb, Cry1Ja, and Cry1Ka proteins was analyzed in bioassays against S. frugiperda an M. sexta larvae. Table 5 shows that all Cry toxins were highly toxic to M. sexta larvae but showed differential toxicity against S. frugiperda. The Cry1Bb protein was the most active toxin against S. frugiperda with an LC50 value of 128.5 ng/cm2 while Cry1Hb and Cry1Ka showed no toxicity even at the highest concentration of protein that was used in the bioassay (5000 ng/cm2) (Table 5).
Discussion
Whole genome sequence screening of Bt strains has been one of the most successful approaches for PP gene discovery in recent years. In addition, it has provided data for the analysis of the evolution of the Bt genome and analysis of horizontal gene transfer events that have contributed to the evolution of Bt strains (Zheng et al., 2017). Bt bacteria are characterized by the presence of insecticidal proteins that accumulate in crystal inclusions during their sporulation phase of growth and such proteins are codified in different plasmids. It was shown that the B. cereus group selectively inactivated the CRISPR Cas system, which correlates with acquisition of mobile elements, such as different plasmids containing genetic information that could help in the adaptation of these bacteria to diverse environments (Zheng et al., 2020). Bt GR007 strain did not contain a functional CRISPR Cas system, which correlated with its high PP gene content, encoded in different plasmids. Phylogenetic analysis of whole genomes showed that Bt GR007 strain (BioProject: PRJNA736034) is closely related with Bt HD12 strain (BioProject: PRJNA302106). Interestingly, the genome sequence comparison between both strains suggested that HD12 might had a genome rearrangement where information contained in plasmids pGR157 and pGR55, that are present in Bt GR007 strain, recombined to form a single large plasmid named pHD12016 in HD12 strain, loosing cry1Fb and vip3Ag genes during this recombination process.
It is now recognized that different Bt strains harbor several PP that in theory may expand their host specificity by improving its toxic activity against different insect targets (Wang et al., 2020). Bt GR007 strain showed insecticidal activity against lepidopteran insects. This insect specificity correlated with the identification of Cry1 proteins expressed in the crystal inclusions that are specific against lepidopteran insects (Crickmore et al., 2020). However, other PP genes found in PAI-1.3 island of this strain, such as vpa2Ac and vpb1Ca genes and the putative novel mpp gene, suggest that it may also display also coleopteran toxicity, but this hypothesis remains to be studied in the future.
The genomic analysis allowed the identification of multiple PAI islands. The PAI-1.1 is the longest PAI region in this strain, containing seven different cry genes. All of them codify for lepidopteran specific Cry proteins (Cry1Da, Cry1Id, Cry1Ja, Cry1Nb, Cry1Ab, Cry1Bb, and Cry1Hb) (Crickmore et al., 2020). This PAI-1.1 also codifies for a cluster of five insecticidal toxin components (Tcs). The Tcs proteins were originally identified in enterobacteria P. luminescens and Xenorhabdus nematophila, which are symbiont of nematodes (Waterfield et al., 2001). These Tcs proteins are pore-forming toxins that kill lepidopteran and dipteran insects. It was previously shown that tcs genes may be found in other bacteria such as Serratia entomophila that is also an insect pathogen or Yersinia pestis, that is mammalian pathogen transmitted by an insect vector (Waterfield et al., 2001). It was also reported that some Bt strains may harbor these genes and that tcaA and tcaB genes were expressed during the infection of gypsy moth larvae by these Bt strains (Blackburn et al., 2011). In the case of GR007 strain, it still remains to be demonstrated if these Tcs proteins participate in toxicity.
The PAI-1.2 contains only two genes encoding for PPs (Vip3Af and Cry2Ad), which are also recognized for their toxicity against lepidopteran insects (Estruch et al., 1996; Liao et al., 2015). In contrast the PAI-1.3 codifies for proteins that have been associated with toxicity against different insect orders, like the PP that shows 34.6% identity with Mpp4Aa from Lysinibacillus sphaericus that belongs to the Mtx2-protein family active against mosquito larvae (Rey et al., 2016). In Bt the Mtx2 like-toxins (now named as Mpp or Gpp) are toxic to other insect orders, such as the Mpp64Ba and Mpp64Ca toxins (previously known as Cry64Ba and Cry64Ca) that are highly active against hemipteran pests (Liu et al., 2018), or Gpp34Aa/Tpp35Aa proteins (previously known as Cry34/Cry35) that showed toxicity against coleopteran pests (Kelker et al., 2014). However, the new PP found in GR007 strain was not classified in the BPPRC web site (see text footnote 1), since we lack information of its pesticidal activity and its identity with other Bt PPs is lower than 35%. This PAI-1.3 island also contains genes that codify for the binary Vpa2Ac and Vpb1Ca toxins, that were previously shown to be toxic to coleopteran larvae (Bi et al., 2015). However, all these PP proteins were not detected in the parasporal crystal inclusion of Bt GR007 so their expression and insect specificity remains to be analyzed. Finally The PAI2.0 island contains three genes encoding for PP that have been shown to be toxic to lepidopteran larvae (Cry1Fb, Cry1Ka, and Vip3Ag) (Koo et al., 1995; Estruch et al., 1996; DaSilva et al., 2016). The Vip3A proteins are expressed in the vegetative phase of growth and are secreted into the medium. The expression of Vip3Ag and Vip3Af in GR007 strain remains to be characterized.
All PAIs from GR007 strain showed multiple Tnp’s sequences (blue arrows in Figure 1B). It has been proposed that Tnp sequences have contributed to the transfer of PP genes and their recombination among differed Bt strains to generate the great variability of these proteins (Mahillon and Chandler, 1998).
Analysis of non-toxin virulence factors produced by Bt GR007 strain showed that most of them are codified in the chromosome of this bacterium (Table 3). It has been proposed that Chitinases, collagenases, cell wall hydrolases, phospholipases, and proteases such as metalloproteases, camelysin (a surface metalloproteinase), bacillolysins (a neutral proteinase), and subtilisin (a serine protease) may be important for efficient larval body utilization during the infection process (Malovichko et al., 2019). Enhancin and collagenase proteins may also participate in the utilization of insect tissues during the last stages of saprophytic colonization (Malovichko et al., 2019). The cytolysins such as hblL2, hblL1, and hblB, a three-component hemolytic complex, was shown to be produced by B. cereus sensu lato strains and has been implicated as a cause of diarrhea associated with food poisoning (Worthy et al., 2021). These cytolysin genes and other cytolysins such as hly II and hly III, xhlA, tlyA were found in the chromosome of GR007 strain. Finally, the internalin proteins were initially described in Listeria monocytogenes, playing an important role in cell invasion (Dramsi et al., 1997). In the case of B. cereus it was proposed that internalins may play a role during pathogenesis and that they are induced in insects after oral ingestion (Fedhila et al., 2006). All these virulence factors may potentially add competitive advantages to the Bt strains, improving their toxicity to the target insect as previously suggested (Malovichko et al., 2019).
Here, we cloned the cry genes found in GR007 strain and analyzed their expression and toxicity against two lepidopteran insects, M. sexta and S. frugiperda. The toxicity of the Bt GR007 strain to these insect pests was relatively low compared to the toxicity of some Cry proteins expressed individually (Table 5). Seven proteins were successfully expressed in Bt cells. The toxicity of Cry1Ab against M. sexta (LC50 8.6 ng/cm2) was relatively low when compared to previous reports that have showed LC50 values two or threefold lower (Gómez et al., 2018; Pacheco et al., 2018). In contrast, the toxicity of this protein against S. frugiperda showed similar values to other reports (Gómez et al., 2018). However, it is important to mention that it was shown that different S. frugiperda populations showed great variability in their susceptibility to Cry1Ab, ranging from not susceptible at all, up to highly susceptible (Graser et al., 2017; Gómez et al., 2018; Figueiredo et al., 2019). The Cry1Bb protein showed to be highly active against M. sexta with LC50 value of 5.4 ng/cm2 and this protein was the most active toxin against S. frugiperda among all tested proteins in this work, with a LC50 value of 128.5 ng/cm2 (Table 5), showing two times higher toxicity to S. frugiperda than previously reported (Luo et al., 1999). The toxicity of Cry1Da against M. sexta and S. frugiperda was similar to previous reports (Höfte and Whiteley, 1989; Wang et al., 2019). In the case of Cry1Fb, Cry1Hb, and Cry1Ka there are no reports previous in the literature about the toxicity of these proteins against these target insects. It was only shown that Cry1Fb was active against Agrotis ípsilon and was not toxic against Heliothis virescens (de Maagd et al., 2003; Karlova et al., 2005); and that Cry1Ka was active against Arfogeia rupae, but not active against Plutella xvlostella, Spodoptera exigua, or Bombyx mori (Koo et al., 1995). Finally the toxicity of Cry1Ja was previously analyzed against S. exigua showing high toxicity (Herrero et al., 2001; Ibargutxi et al., 2006) and the reported toxicity of Cry1Ja against M. sexta was much higher than the LC50 value reported in this work (Herrero et al., 2001).
Spodoptera frugiperda has been shown to be highly susceptible to Cry1Fa and transgenic maize expressing Cry1Fa has been shown to be effective in controlling this insect pest. However, S. frugiperda has evolved resistance to Cry1Fa-maize in Puerto Rico, United States, Brazil, and Argentina (Storer et al., 2012; Farias et al., 2014; Chandrasena et al., 2018). Thus, additional Cry proteins that show no cross-resistance to Cry1Fa are likely to provide tools to counter resistance of this pest to transgenic maize. In the case of Cry1Bb, it was shown that this toxin and Cry1Fa share a binding site in the brush border membranes of S. frugiperda suggesting that Cry1Bb and Cry1Fa should show cross-resistance in this insect species (Luo et al., 1999). However, the Vip3Aa has been shown to be effective against the Cry1Fa resistant populations (Welch et al., 2015). The toxicity of the other PP proteins codified in Bt GR007 strain such as Cry1Id, Cry1Nb, Cry2Ad, the new Mpp, Vpa2Ac, Vpb1Ca Vip3Af, and Vip3Ag remains to be analyzed against different target insects including insect populations that have evolved resistance to other Cry proteins in the field.
Finally, we analyzed the promotor region of GR007 PP genes. We found that cry1Da, cry1Ja, cry1Bb, cry1Hb, cry1Fb, and cry1Ka genes have promotor regions highly similar to cry1Ab gene suggesting that all of them may be regulated by SigE and SigK factors. Previously the regulation of some cry genes from HD12 strain was analyzed showing that the expression of cry1Ae, cry1Bb, cry1Fb, and cry1Ja genes is regulated by both sigma factors, while cry1Da was only regulated by SigE. However, the problem was that the upstream sequence of cry1Da gene was not fully analyzed (Song et al., 2017). They did not included the −35 region of SigK promoter, resulting in the loss of SigK function when fused to LacZ gene (Song et al., 2017). Here we show that the cry1Da gene that is present in GR007 strain has the complete promoter region including −35 and −10 DNA sequence for binding of both sigma factors, suggesting that its expression is determined by both sigma factors. Our analysis also showed that promotor regions from cry1Nb, cry1Id, and cry2Ad showed different sequences, suggesting that they do not share the same regulation as the other cry genes present in this strain (Supplementary Figure 3). Blast analysis showed that the promotor region from cry2Ad is similar to the upstream sequence of cry2Ab gene. The promotor region of cry1Id is similar to the upstream sequence of cry1Ia, while the promotor region of cry1Nb has no similarities to any other sequence including all known upstream sequences from other cry genes.
Overall, our data indicate that in nature certain Bt strains contain and express multiple PP genes codifying for proteins that display activities against different target pest. These strains could be excellent candidates to generate novel formulations. The information provided here regarding the genome of Bt GR007 strain will help with the understanding Bt toxin gene diversity and regulation of its PPs.
Data Availability Statement
The data for this study can be found in BioProject: PRJNA736034 and Gene Bank Accession numbers CP076539, CP076541, CP076540, and CP076542.
Author Contributions
JS collected the sample. SP, IG, and JS conducted the experiments. SP and MC analyzed the data. AB and MS conceived of the idea and designed the study. SP, MS, and AB participated in drafting the manuscript. All authors contributed to the article and approved the submitted version.
Funding
This work was supported by CONACyT Ciencia de Frontera 6693 and DGAPA IN203619 and IN206721.
Conflict of Interest
The authors declare that the research was conducted in the absence of any commercial or financial relationships that could be construed as a potential conflict of interest.
Publisher’s Note
All claims expressed in this article are solely those of the authors and do not necessarily represent those of their affiliated organizations, or those of the publisher, the editors and the reviewers. Any product that may be evaluated in this article, or claim that may be made by its manufacturer, is not guaranteed or endorsed by the publisher.
Acknowledgments
We thank Lizbeth Cabrera for technical assistance.
Supplementary Material
The Supplementary Material for this article can be found online at: https://www.frontiersin.org/articles/10.3389/fmicb.2021.758314/full#supplementary-material
Footnotes
References
Arantes, O., and Lereclus, D. (1991). Construction of cloning vectors for Bacillus thuringiensis. Gene 108, 115–119. doi: 10.1016/0378-1119(91)90495-W
Banerjee, R., Hasler, J., Meagher, R., Nagoshi, R., Hietala, L., Huang, F., et al. (2017). Mechanism and DNA-based detection of field-evolved resistance to transgenic Bt corn in fall armyworm (Spodoptera frugiperda). Sci. Rep. 7:10877. doi: 10.1038/s41598-017-09866-y
Beard, C. E., Ranasinghe, C., and Akhurst, R. J. (2001). Screening for novel cry genes by hybridization. Lett. Appl. Microbiol. 33, 241–245. doi: 10.1046/j.1472-765x.2001.00982.x
Bell, R. A., and Joachim, F. G. (1976). Techniques for rearing laboratory colonies of tobacco hornworms and pink bollworms. Ann. Entomol. Soc. Am. 69, 365–373.
Bi, Y., Zhang, Y., Shu, Ch, Crickmore, N., Wang, Q., Du, L., et al. (2015). Genomic sequencing identifies novel Bacillus thuringiensis Vip1/Vip2 binary and Cry8 toxins that have high toxicity to Scarabaeoidea larvae. Appl. Microbiol. Biotechnol. 99, 753–760. doi: 10.1007/s00253-014-5966-2
Blackburn, M. B., Martin, P. A. W., Kuhar, D., Farrar, R. R. Jr., and Gundersen-Rindal, D. E. (2011). The occurrence of Photorhabdus-Like toxin complexes in Bacillus thuringiensis. PLoS One 6:e18122. doi: 10.1371/journal.pone.0018122
Blanco, C., Portilla, M., Jurat-Fuentes, J. L., Sanchez, J. F., Viteri, D., Vega-Aquino, P., et al. (2010). Susceptibility of isofamilies of Spodoptera frugiperda (Lepidoptera:Noctuidae) to Cry1Ac and Cry1Fa proteins from Bacillus thuringiensis. Southwestern Entomol. 35, 409–415. doi: 10.3958/059.035.0325
Boaventura, D., Ulrich, J., Lueke, B., Bolzan, A., Okuma, D., Gutbrod, O., et al. (2020). Molecular characterization of Cry1F resistance in fall armyworm, Spodoptera frugiperda from Brazil. Insect Biochem. Mol. Biol. 116:103280. doi: 10.1016/j.ibmb.2019.103280
Cao, Z. -l., Tan, T.-T., Jiang, K., Mei, S.-Q., Hou, X.-H., and Cai, J. (2018). Complete genome sequence of Bacillus thuringiensis L-7601, a wild strain with high production of melanin. J. Biotechnol. 275, 40–43. doi: 10.1016/.biotec.2018.03.020
Cerón, J., Covarrubias, L., Quintero, R., Ortíz, A., Ortíz, M., Aranda, E., et al. (1994). PCR analysis of the CryI insecticidal crystal family genes from Bacillus thuringiensis. Appl. Environ. Microbiol. 60, 353–356. doi: 10.1128/aem.60.1.353-356.1994
Cerón, J., Ortíz, A., Quintero, R., Güereca, L., and Bravo, A. (1995). Specific PCR reaction primers directed to identify cryI and cryIII genes within a Bacillus thuringiensis strain collection. Appl. Environ. Microbiol. 61, 3826–3831. doi: 10.1128/aem.61.113826-3831.1995
Chandrasena, D., Signorini, A. M., Abratti, G., Storer, N. P., Olaciregui, M. L., Alves, A. P., et al. (2018). Characterization of field-evolved resistance to Bacillus thuringiensis-derived Cry1F δ-endotoxin in Spodoptera frugiperda populations from Argentina. Pest. Manag. Sci. 74, 746–754. doi: 10.1002/ps.4776
Chestukhina, G. G., Kostina, L. I., Zalunin, I. A., Revina, L. P., Mikhailova, A. L., and Stepanov, V. M. (1994). Production of multiple delta-endotoxins by Bacillus thuringiensis: delta-endotoxins produced by strains of the subspecies galleriae and wuhanensis. Can. J. Microbiol. 40, 1026–1034. doi: 10.1139/m94-163
Crickmore, N., Berry, C., Panneerselvam, S., Mishra, R., Connor, T. R., and Bonning, B. C. (2020). A structure-based nomenclature for Bacillus thuringiensis and other bacteria-derived pesticidal proteins. J. Invertebr. Pathol. 9:107438. doi: 10.1016/j.jip.2020.107438
DaSilva, K., Spencer, T. A., Crespo, A. L. B., and Siegfried, B. D. (2016). Susceptibility of Spodoptera frugiperda (Lepidoptera: Noctuidae) field populations to the Cry1F Bacillus thuringiensis insecticidal protein. Florida Entomol. 99, 629–633. doi: 10.1653/024.099.0407
de Maagd, R. A., Weemen-Hendriks, M., Molthoff, J. W., and Naimov, S. (2003). Activity of wild-type and hybrid Bacillus thuringiensis δ-endotoxins against Agrotis ipsilon. Arch. Microbiol. 179, 363–367.
Doggett, N. A., Stubben, C. J., Chertkov, O., Bruce, D. C., Detter, J. Ch, Johnson, S. L., et al. (2013). Complete genome sequence of Bacillus thuringiensis serovar israelensis strain HD-789. Genome Announc. 26, e01023–13. doi: 10.1128/genomeA.01023-13
Dramsi, S., Dehoux, P., Lebrun, M., Goossens, P. L., and Cossart, P. (1997). Identification of four new members of the internalin multiple family of Listeria monocytogenes EGD. Inf. Immun. 65, 1615–1625. doi: 10.1128/iai.65.5.1615-1625.1997
Edgar, R. C. (2007). PILER-CR: fast and accurate identification of CRISPR repeats. BMC Bioinform. 8:18. doi: 10.1186/1471-2105-8-18
Estruch, J. J., Warren, G. W., Mullins, M. A., Nye, G. J., Craig, J. A., and Kozil, M. G. (1996). Vip3A, a novel Bacillus thuringiensis vegetative insecticidal protein with a wide spectrum of activities against lepidopteran insects. Proc. Natl. Acad. Sci. U. S. A. 93, 5389–5394. doi: 10.1073/pnas.93.11.5389
Farias, J. R., Andow, D. A., Horikoshi, R. J., Sorgatto, R. J., Fresia, P., dos Santos, A. C., et al. (2014). Field evolved resistance to Cry1F maize by Spodoptera frugiperda (Lepidoptera: Noctuidae) in Brazil. Crop Prot. 64, 150–158. doi: 10.1016/j.cropro.2014.06.019
Fedhila, S., Daou, N., Lereclus, D., and Nielsen-LeRoux, C. (2006). Identification of Bacillus cereus internalin and other candidate virulence genes specifically induced during oral infection in insects. Mol. Microbiol. 62, 339–355. doi: 10.1111/j.1365-2958.2006.05362.x
Fiedoruk, K., Daniluk, T., Mahillon, J., Leszczynska, K., and Swiecicka, I. (2017). Genetic Environment of cry1 genes indicates their common origin. Genome Biol. Evol. 9, 2265–2275. doi: 10.1093/gbe/evx165
Figueiredo, C. S., Lemes, A. R. N., Sebastiao, I., and Desiderio, J. A. (2019). Synergism of the Bacillus thuringiensis Cry1, Cry2, and Vip3 proteins in Spodoptera frugiperda control. Appl. Biochem. Biotechnol. 188, 798–809.
Fouts, D. E. (2006). Phage_Finder: automated identification and classification of prophage regions in complete bacterial genome sequences. Nucleic Acids Res. 34, 5839–5851. doi: 10.1093/nar/gkl732
Gao, Q., Zheng, J., Zhu, L., Ruan, L., Peng, D., and Sun, M. (2015). Complete genome sequence of Bacillus thuringiensis tenebrionis 4AA1, a typical strain with toxicity to Coleopteran insects. J. Biotechnol. 204, 15–16. doi: 10.1016/j.jbiotec.2015.03.009
Gómez, I., Ocelotl, J., Sánchez, J., Garcia-Gomez, B. I, Lima, Ch, Martins, E., et al. (2018). Enhancement of Bacillus thuringiensis Cry1Ab and Cry1Fa toxicity to Spodoptera frugiperda by domain III mutations indicates two distinct limiting steps for toxicity. Appl. Environ. Microbiol. 84, e01393–18. doi: 10.1128/AEM.01393-18
Graser, G., Walters, F. S., Burns, A., Sauve, A., and Raybould, A. (2017). A general approach to test for interaction among mixtures of insecticidal proteins which target different orders of insect pests. J. Insect Sci. 17:39. doi: 10.1093/jisesa/iex003
Helgason, E., Okstad, O. A., Caugant, D. A., Johansen, H. A., Fouet, A., Mock, M., et al. (2000). Bacillus anthracis, Bacillus cereus, and Bacillus thuringiensis-one species on the basis of genetic evidence. Appl. Environ. Microbiol. 66, 2627–2630. doi: 10.1128/aem.66.6.2627-2630.2000
Herrero, S., Gonzaìlez-Cabrera, J., Tabashnik, B. E., and Ferreì, J. (2001). Shared binding sites in lepidoptera for Bacillus thuringiensis Cry1Ja and Cry1A toxins. Appl. Environ. Microbiol. 67, 5729–5734.
Höfte, H., and Whiteley, H. R. (1989). Insecticidal crystal proteins of Bacillus thuringiensis. Microbiol Rev. 53, 242–255.
Huerta-Cepas, J., Forslund, K., Coelho, L. P., Szklarczyk, D., Jensen, L. J., von Mering, C., et al. (2017). Fast genome-wide functional annotation through orthology assignment by eggNOG-Mapper. Mol. Biol. Evol. 34, 2115–2122. doi: 10.1093/molbev/msx148
Ibargutxi, M. A., Estela, A., Ferré, J., and Caballero, P. (2006). Use of Bacillus thuringiensis toxins for control of the cotton pest Earias insulana (Boisd.) (Lepidoptera: Noctuidae) Appl. Environ. Microbiol. 72, 437–442.
Karlova, R., Weemen-Hendriksb, M., Naimova, S., Cerón, J., Dukiandjieva, S., and de Maag, R. A. (2005). Bacillus thuringiensis endotoxin Cry1Ac domain III enhances activity against Heliothis virescens in some, but not all Cry1-Cry1Ac hybrids. J. Invertebr. Pathol. 88, 169–172.
Kelker, M. S., Berry, C., Evans, S. L., Pai, R., McCaskill, D. G., Wang, N. X., et al. (2014). Structural and biophysical characterization of Bacillus thuringiensis insecticidal proteins Cry34Ab1 and Cry35Ab1. PLoS One 9:e112555. doi: 10.1371/journal.pone.0112555
Koo, B. T., Park, S. H., Choi, S. K., Sjin, B. S., Kim, J. I., and Yu, J. H. (1995). Cloning of a novel crystal protein gene cry1K from Bacillus thuringiensis subsp. morrisoni. FEMS Microbiol. Lett. 134, 159–164. doi: 10.1111/j.1574-6968.1995.tb07931.x
Lecadet, M.-M., Blondel, M. O., and Ribier, J. (1980). Generalized transduction in Bacillus thuringiensis var. berliner 1715, using bacteriophage CP54 Ber. J. Gen. Microbiol. 121, 203–212. doi: 10.1099/00221287-121-1-203
Letowski, J., Bravo, A., Brousseau, R., and Masson, L. (2005). Assessment of cry1 gene contents of Bacillus thuringiensis strains by use of DNA microarrays. Appl. Environ. Microbiol. 71, 5391–5398. doi: 10.1128/aem.71.9.5391-5398.2005
Letunic, I., and Bork, P. (2019). Interactive Tree Of Life (iTOL) v4: recent updates and new developments. Nucleic Acids Res. 47, W256–W259. doi: 10.1093/nar/gkz239
Liao, J. Y., Gao, Y. Q., Wu, Q. Y., Zhu, Y. C., and Yasin, N. (2015). Purification of the insecticidal Cry2Ad protein from a Bt-isolated BRC-HZP10 strain and toxin assay to the diamondback moth. Plutella xylostella (L.). Gen. Mol. Res. 14, 7661–7670. doi: 10.4238/2015.July.13.11
Liu, G., Song, L., Shu, Ch, Wang, P., Deng, Ch, and Peng, Q. (2013). Complete genome sequence of Bacillus thuringiensis subsp. kurstaki strain HD73. Genome Announc. 1, e00080–13. doi: 10.1128/genomeA.00080-13
Liu, Y., Wang, Y., Shu, Ch, Lin, K., Song, F., Bravo, A., et al. (2018). Cry64Ba and Cry64Ca, two ETX/MTX2 Bacillus thuringiensis insecticidal proteins against hemipteran pests. Appl. Environ. Microbiol. 84, e01996–17. doi: 10.1128/AEM.01996-17
Luo, K., Bank, D., and Adang, M. J. (1999). Toxicity, binding, and permeability analyses of four Bacillus thuringiensis Cry1 d-endotoxins using brush border membrane vesicles of Spodoptera exigua and Spodoptera frugiperda. Appl. Environ. Microbiol. 65, 457–454. doi: 10.1128/aem.65.2.457-464.1999
Macaluso, A., and Mettus, A. M. (1991). Efficient transformation of Bacillus thuringiensis requires nonmethylated plasmid DNA. J. Bacteriol. 173, 1353–1356. doi: 10.1128/jb.173.3.1353-1356.1991
Mahillon, J., and Chandler, M. (1998). Insertion sequences. Microbiol. Mol. Biol. Rev. 62, 725–774. doi: 10.1128/MMBR.62.3.725-774.1998
Malovichko, Y. V., Nizhnikov, A. A., and Antonets, K. S. (2019). Repertoire of the Bacillus thuringiensis virulence factors unrelated to major classes of protein toxins and its role in specificity of host-pathogen interactions. Toxins 11:347. doi: 10.3390/toxins11060347
Pacheco, S., Gómez, I., Sánchez, J., García-Gómez, B. I., Czajkowsky, D., Zhang, J., et al. (2018). Helix α-3 inter-molecular salt bridges and conformational changes are essential for toxicity of Bacillus thuringiensis 3D-Cry toxin family. Sci. Rep. 8:10331. doi: 10.1038/s41598-018-28753-8
Palma, L., Muñoz, D., Berry, C., Murillo, J., and Caballero, P. (2014). Draft Genome sequences of two Bacillus thuringiensis strains and characterization of a putative 41.9-kDa insecticidal toxin. Toxins 6, 1490–1504. doi: 10.3390/toxins6051490
Petkau, A., Stuart-Edwards, M., Stothard, P., and Van Domselaar, G. (2010). Interactive microbial genome visualization with GView. Bioinformatics 26, 3125–3126. doi: 10.1093/bioinformatics/tbq588
Porcar, M., and Juárez-Pérez, V. (2003). PCR-based identification of Bacillus thuringiensis pesticidal crystal genes. FEMS Microbiol. Rev. 26, 419–432. doi: 10.1111/j.1574-6976.2003.tb00624.x
Rey, A., Silva-Quintero, L., and Dussan, J. (2016). Complete genome sequencing and comparative genomic analysis of functionally diverse Lysinibacillus sphaericus III(3)7. Genome Data 9, 78–86. doi: 10.1016/j.gdata.2016.06.002
Sanahuja, G., Banakar, R., Twyman, R. M., Capell, T., and Christou, P. (2011). Bacillus thuringiensis: a century of research, development and commercial applications. Plant Biotechnol. J. 9, 283–300. doi: 10.1111/j.1467-7652.2011.00595.x
Seemann, T. (2014). Prokka: rapid prokaryotic genome annotation. Bioinformatics 30, 2068–2069. doi: 10.1093/bioinformatics/btu153
Siguier, P., Perochon, J., Lestrade, L., Mahillon, J., and Chandler, M. (2006). ISfinder: the reference center for bacterial insertion sequences. Nucleic Acids Res. 34, D32–D36. doi: 10.1093/nar/gkj014
Song, Z.-R., Peng, Q., Shu, Ch, Zhang, J., Sun, D., and Song, F. (2017). Identification of similar transcriptional regulatory mechanisms in multiple cry genes in Bacillus thuringiensis HD12. J. Integrative Agricul. 16, 135–143. doi: 10.1016/S2095-3119(16)61398-9
Storer, N. P., Kubiszak, M. E., King, J., Thompson, G. D., and Santos, A. C. (2012). Status of resistance to Bt maize in Spodoptera frugiperda: lessons from Puerto Rico. J. Invertebr. Pathol. 110, 294–300. doi: 10.1016/j.jip.2012.04.007
Sun, X., Hu, Ch, Jia, H., Wu, Q., Shen, X., and Zhao, Sh, et al. (2021). Case study on the immigration of fall armyworm, Spodoptera frugiperda invading into China. J. Integrative Agricul. 20, 664–672. doi: 10.1016/S2095-3119(19)62839-x
Treangen, T. J., Ondov, B. D., Koren, S., and Phillippy, A. M. (2014). The Harvest suite for rapid core-genome alignment and visualization of thousands of intraspecific microbial genomes. Genome Biol. 15:524. doi: 10.1186/s13059-014-0524-x
Villegas-Mendoza, J. M., and Rosas-García, N. M. (2013). Visual and gustatory responses of Spodoptera frugiperda (Lepidoptera: Noctuidae) larvae to artificial food dyes. Florida Entomol. 96, 1102–1106.
Wang, Y., Wang, J., Fu, X., Nageotte, J. R., Silverman, J., Bretsnyder, E. C., et al. (2019). Bacillus thuringiensis Cry1Da-7 and Cry1B.868 protein interactions with novel receptors allow control of resistant fall armyworms, Spodoptera frugiperda (J.E. Smith). Invertebr. Microbiol. 85, e00579–19. doi: 10.1128/AEM.00579-19
Wang, Z., Wang, K., Bravo, A., Soberón, M., Cai, J., Shu, C., et al. (2020). Coexistence of Cry9 and Vip3 in an identical plasmid of Bacillus thuringiensis indicates their synergistic insecticidal toxicity. J. Agricul. Food Chem. 68, 14081–14090. doi: 10.1021/acs.jafc.0c05304
Waterfield, N. R., Bowen, D. J., Fetherstone, J. D., Perry, R. D., and ffrench-Constant, R. H. (2001). The tc genes of Photorhabdus: a growing family. Trends Microbiol. 9, 185–91. doi: 10.1016/s0966-842x(01)01978-3
Welch, K. L., Unnithan, G. C., Degain, B. A., Wei, J., Zhang, J., Li, X., et al. (2015). Cross-resistance to toxins used in pyramided Bt crops and resistance to Bt sprays in Helicoverpa zea. J. Invertebr. Pathol. 132, 149–156. doi: 10.1016/j.jip.2015.10.003
Worthy, H. L., Williamson, L. J., Auhim, H. S., Leppla, S. H., Sastalla, I., Jones, D. D., et al. (2021). The crystal structure of Bacillus cereus HbiL1. Toxins 13:253. doi: 10.3390/toxins13040253
Zheng, J. S., Gao, Q. L., Liu, L. L., Liu, H. L., Wang, Y. Y., Peng, D. H., et al. (2017). Comparative genomics of Bacillus thuringiensis reveals a path to specialized exploitation of multiple invertebrate hosts. Mbio 8, e00822–17. doi: 10.1128/mBio.00822-17
Keywords: genome sequencing, Bacillus thuringiensis, Cry toxin, MS/MS, biocontrol
Citation: Pacheco S, Gómez I, Chiñas M, Sánchez J, Soberón M and Bravo A (2021) Whole Genome Sequencing Analysis of Bacillus thuringiensis GR007 Reveals Multiple Pesticidal Protein Genes. Front. Microbiol. 12:758314. doi: 10.3389/fmicb.2021.758314
Received: 13 August 2021; Accepted: 05 October 2021;
Published: 02 November 2021.
Edited by:
Nikolai Ravin, Institute of Bioengineering, Research Center of Biotechnology, Russian Academy of Sciences (RAS), RussiaReviewed by:
Jakub Baranek, Adam Mickiewicz University, PolandFengliang Jin, South China Agricultural University, China
Copyright © 2021 Pacheco, Gómez, Chiñas, Sánchez, Soberón and Bravo. This is an open-access article distributed under the terms of the Creative Commons Attribution License (CC BY). The use, distribution or reproduction in other forums is permitted, provided the original author(s) and the copyright owner(s) are credited and that the original publication in this journal is cited, in accordance with accepted academic practice. No use, distribution or reproduction is permitted which does not comply with these terms.
*Correspondence: Alejandra Bravo, YWxlamFuZHJhLmJyYXZvQGlidC51bmFtLm14