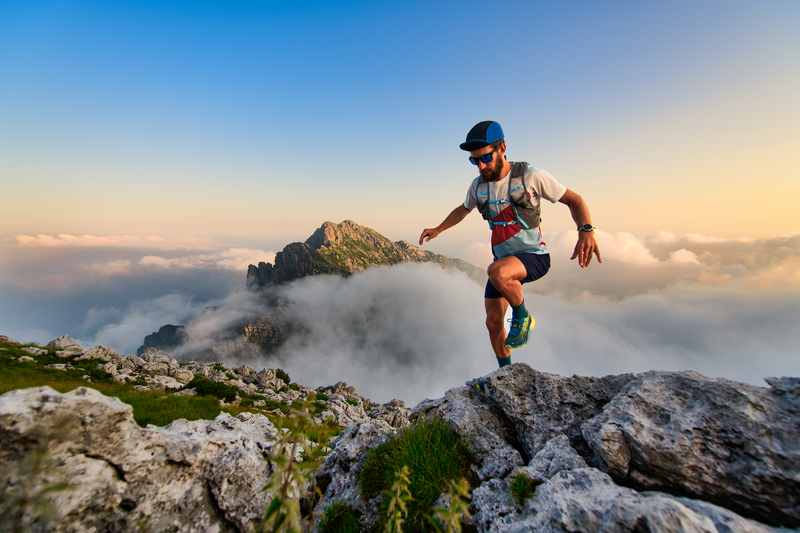
95% of researchers rate our articles as excellent or good
Learn more about the work of our research integrity team to safeguard the quality of each article we publish.
Find out more
ORIGINAL RESEARCH article
Front. Microbiol. , 22 October 2021
Sec. Virology
Volume 12 - 2021 | https://doi.org/10.3389/fmicb.2021.754664
This article is part of the Research Topic Influence of Protein-Protein Interactions (PPIs) on the outcome of viral infections View all 10 articles
Hepatitis C virus (HCV) is a single-stranded RNA virus of positive polarity [ssRNA(+)] that replicates its genome through the activity of one of its proteins, called NS5B. This viral protein is responsible for copying the positive-polarity RNA genome into a negative-polarity RNA strand, which will be the template for new positive-polarity RNA genomes. The NS5B protein is phosphorylated by cellular kinases, including Akt. In this work, we have identified several amino acids of NS5B that are phosphorylated by Akt, with positions S27, T53, T267, and S282 giving the most robust results. Site-directed mutagenesis of these residues to mimic (Glu mutants) or prevent (Ala mutants) their phosphorylation resulted in a reduced NS5B in vitro RNA polymerase activity, except for the T267E mutant, the only non-conserved position of all those that are phosphorylated. In addition, in vitro transcribed RNAs derived from HCV complete infectious clones carrying mutations T53E/A and S282E/A were transfected in Huh-7.5 permissive cells, and supernatant viral titers were measured at 6 and 15 days post-transfection. No virus was rescued from the mutants except for T53A at 15 days post-transfection whose viral titer was statistically lower as compared to the wild type. Therefore, phosphorylation of NS5B by cellular kinases is a mechanism of viral polymerase inactivation. Whether this inactivation is a consequence of interaction with cellular kinases or a way to generate inactive NS5B that may have other functions are questions that need further experimental work.
Hepatitis C virus (HCV) is a positive single-stranded RNA virus [ssRNA(+)] belonging to Flaviviridae family. HCV genome replication takes place in replication complexes where the non-structural NS5B protein produces positive single-stranded genome copies [RNA(+)] through an intermediary of negative polarity [RNA(−)] (Neufeldt et al., 2018; Tabata et al., 2020). The HCV protein NS5B is an RNA-dependent RNA polymerase (RDRP) which, like other polymerases in its class, shows a structure that has been compared to the shape of a right hand with three subdomains called fingers, palm, and thumb (Sesmero and Thorpe, 2015). The subdomain of the palm comprises three well-preserved motifs A (D220-X(4)-D225), B (S282-X(8)-N291), and C (G317D318D319), which define the catalytic center. The aspartic acid residues D220 in motif A and D318 and D319 in motif C are involved in the coordination of the divalent cations (Mg2+ and/or Mn2+) essential for the formation of the phosphodiester bond. Residues D225 of motif A, and S282 and N291 residues of motif B are involved in selection of ribonucleoside triphosphates over dNTPs and, thus, determine whether RNA rather than DNA is synthesized (Sesmero and Thorpe, 2015).
Viruses are obligate intracellular parasites that usurp the cellular machinery to complete their replicative cycle. This means that viral components need to interact with cellular components to direct cellular activity according to viral interests. HCV is no exception, and an enormous amount of interactions between viral components and cellular proteins have already been described (de Chassey et al., 2008; Dolan et al., 2013; Hagen et al., 2014). HCV proteins NS3, NS5A, and core have shown the most extensive network of interactions with host factors (de Chassey et al., 2008). NS5B also interacts with cellular proteins (Hamamoto et al., 2005; Munakata et al., 2005; Watashi et al., 2005; Kusakawa et al., 2007; Inoue et al., 2011; Hillung et al., 2012), and some of its interactions require partial denaturation of NS5B and subsequent loss of RDRP activity. For example, the retinoblastoma tumor suppressor is down regulated by interacting with NS5B via amino acids located in the catalytic center (C motif) (Munakata et al., 2005). For this interaction to occur, the NS5B protein must expose amino acids located in the active center. These data indicate that an inactive NS5B may be modulating an important surveillance pathway.
We have previously described the interaction of NS5B with the cellular kinase Akt and the changes in subcellular localization related with this protein:protein interaction (Valero et al., 2016). Furthermore, we documented that Akt phosphorylates NS5B and that inhibitors of Akt affect HCV replication in cell culture (Valero et al., 2016). Viral RNA polymerase phosphorylation has also been described in other viral systems previously (Barik et al., 1995; Jakubiec et al., 2006; Schmid et al., 2007), and norovirus RNA polymerase is phosphorylated by Akt as well (Eden et al., 2011). The PI3K-Akt pathway, transiently activated during HCV entry (Liu et al., 2012; Qian et al., 2020), has been linked to HCV infection and related metabolic disorders (Qadri et al., 2012; Zhang et al., 2018). Therefore, modulation of Akt and Akt-related proteins could be of great importance for HCV replication.
In this study, we describe the positions of the HCV NS5B protein that are phosphorylated by the cellular kinase Akt. Furthermore, we show that mutants mimicking phosphorylation at these positions lead to proteins with very low RDRP activities. In addition, viruses carrying mutations mimicking Akt phosphorylation of T53 or S282 residues were unable to replicate in cultured cells. Akt:NS5B interaction produces an inactive viral polymerase whose role needs to be elucidated.
Plasmid pET_NS5BΔ21 encoding HCV NS5B from strain HC-J4 with a 21 amino acid deletion at the C-terminal end has been described previously (Lopez-Jimenez et al., 2014). Recombinant Akt/PKB was purchased from Biaffin (PK-PKBA-020, Biaffin GmbH&Co), and lipofectamine 2000 from Invitrogen. Huh7.5 cells were kindly provided by Dr. R. Bartenschlager (University of Heidelberg, Germany).
Point mutants in NS5B were generated by site-directed mutagenesis following the manufacturer’s instructions (QuikChange Site-Directed Mutagenesis, Agilent Technologies). Synthetic oligonucleotides used for point mutant generation are described in Table 1. NS5B wild type and mutants were over-expressed and purified as described previously (Lopez-Jimenez et al., 2014; Valero et al., 2016).
Kinase assays were performed as previously described (Albentosa-Gonzalez et al., 2021). Briefly, HCV NS5B (3 μg) was incubated in 20 mM Hepes pH 7.4, 10 mM MgCl2, 10 mM MnCl2, 1 μCi of γ[32P]-ATP, 1 mM DTT, in the presence of 0.5 μg of recombinant Akt/PKB (PK-PKBA-A020, Biaffin GmbH&Co). Following SDS-PAGE electrophoresis, the gel was dried and exposed to phosphorimager screens, and scanned with Typhoon 9600 (Molecular Dynamics) to detect radiolabeled products.
The identification of phosphorylated NS5B residues was performed as previously described (Albentosa-Gonzalez et al., 2021). Briefly, phosphorylated protein was digested in situ with sequencing grade trypsin (Promega, Madison, WI, United States) and analyzed by RP-LC-MS/MS in an Easy-nLC II system coupled to an ion trap LTQ-Orbitrap-Velos-Pro mass spectrometer (Thermo Scientific). The MS/MS spectra from the peptides were analyzed by assigning the fragment ions to the candidate sequence after calculating the series of theoretical fragmentations.
RNA polymerase assays were performed using the symmetric substrate LE-19, which is capable of de novo initiation (DN) and primer-extension (PE), as previously described (Clemente-Casares et al., 2011; Lopez-Jimenez et al., 2014). 200 nM NS5B was pre-incubated for 30 min in a reaction mixture containing 20 mM MOPS, pH 7.3, and 5 mM MnCl2. Reactions were started by adding 500 μM GTP, 100 μM ATP, and UTP, and 1 μCi α[32P]CTP (3000 Ci mmol, PerkinElmer Life Sciences). Reactions were stopped by adding EDTA/formamide loading buffer at different time points as indicated. Products were separated using denaturing polyacrylamide (23% PAA, 7 M urea) gel electrophoresis. Gels were exposed to phosphorimager screens and scanned with Typhoon (Molecular Dynamics). Quantification was achieved by running samples on parallel gels and determining band volumes using ImageQuant software (GE Healthcare).
The origin of the Huh-7.5 cell line, procedures for cell growth in Dulbecco’s modified Eagle’s medium (DMEM), the virus used in the experiments rescued from plasmid Jc1FLAG2(p7-nsGluc2A) (a chimera of J6 and JFH-1 from genotype 2a), and the procedures used to prepare the initial virus stock HCVp0, to titrate viral infectious particles, and to quantify viral RNA have been described previously (Perales et al., 2013; Sheldon et al., 2014). To perform infections for immunofluorescence and RNA quantification assays, 1 × 105 Huh-7.5 cells were infected with HCVp0 at a multiplicity of infection (MOI) of 0.5 Tissue Culture Infectious Dose (50%) TCID50/cell. The infected cells were further incubated at 37°C for 6 and 15 days. Absence of contamination was checked by maintaining and titrating mock-infected cells and their supernatants in parallel with the infected cultures. No infectivity in the mock-infected cultures was detected in any of the experiments.
Plasmid Jc1FLAG2(p7-nsGluc2A) was used as a template for constructing T53A, T53E, S282A, and S282E mutants by site-directed mutagenesis using the oligonucleotides described in Table 1. Plasmids carrying the selected mutations were transcribed in vitro and the genomic RNA from WT or mutant virus was used to transfect cells as described above to produce HCVp0 virus stock. The supernatant from these transfections was used to titer the virus obtained. Supernatant from infections with mutant virus in which viral titer was obtained was used to purify viral RNA that was retrotranscribed, PCR amplified, and sequenced to test for mutation reversal.
Previous results from our laboratory have shown that the Akt protein phosphorylates the NS5B polymerase of HCV (Valero et al., 2016). To analyze the effect that this phosphorylation causes in the biology of the virus, we first wanted to identify the residues phosphorylated by this Ser/Thr kinase. To this end, we performed an in vitro Akt phosphorylation assay using recombinant protein NS5B (66 kDa) fused to the fluorescent protein EGFP (NS5B-FP) and γ[32P]-ATP as substrates. The fused protein shows a molecular mass of approximately 95 kDa that can easily be distinguished from Akt (68 kDa). The products of the phosphorylation reaction were resolved by SDS-PAGE and two bands were detected, one at about 100 kDa corresponding to NS5B-FP, and the other at about 65 kDa corresponding to the autophosphorylated Akt (Figure 1A). The phosphorylation experiment was repeated with cold ATP for proteomic purposes. The NS5B-FP band was extracted and digested with trypsin, yielding a sequence coverage of 70% in the proteomic analysis (Figure 1B).
Figure 1. (A) SDS-PAGE gel showing products obtained after Akt phosphorylation of NS5B-FP (lane 1). Unphosphorylated NS5B-FP is shown in lane 2. Molecular weight marker (MWM) is on the left of the gel and sizes for NS5B-FP and Akt are indicated on the right. (B) Amino acid sequence of HCV NS5B from strain HC-J4. Phosphorylated sites identified in phosphoproteomics are in red (with increased font size). Sequence uncovered by the phosphoproteomic analysis is in gray color.
Proteomic analysis of the trypsin digestion products allowed the identification of the phosphorylated residues S29, T53, T267, and S282 (Figure 2). Ser29 is located in the subdomain called fingertips, and is involved in the interaction with the thumb (Figure 3A). Residues T53 and T267 are located in the fingers subdomain, and are involved in the helix-helix interactions that stabilize this subdomain (Figure 3A). Finally, the S282 residue is part of the motif B site and interacts with the D225 residue of motif A for the correct positioning of the NTP during the formation of the phosphodiester bond. Two other residues (S27 and S269) could be phosphorylated and were not well identified because of their proximity to the most likely ones (S29 and T267). In any case, it is much less likely that they are phosphorylated and this was the reason they were not included in some experiments.
Figure 2. Phosphoproteomic details from the phosphorylation experiment described in Figure 1. MS/MS profiles for NS5B-FP tryptic peptides encompassing residues T53 (A,B), S282 (C), S29 (D), and T267 (E). Peptide sequences corresponding to each spectrum are indicated with the identified phosphorylated residue marked in red.
Figure 3. (A) Localization of the residues phosphorylated by Akt. The positions of the amino acids identified by proteomics are shown in magenta on the structure of the NS5B HC-J4 (PDB: 1NB4) protein. The amino acids of the catalytic triad are shown in red. The most important structural elements (template channel, nucleotide entry channel and loops Δ1 and Δ2) are also indicated. (B) SDS-PAGE gel showing purified WT and Glu (upper panel) or Ala (lower panel) mutant proteins for each position. The molecular weight marker is shown on the left. The identity of the protein in each lane is indicated above the protein bands.
Next, we sought to determine the effect of the phosphorylation of the residues described above on the RDRP activity of the NS5B protein. To this end, mutations that mimic phosphorylated Ser or Thr residues at these positions were introduced by site-directed mutagenesis, yielding proteins carrying the mutations S27E, S29E, T53E, T267E, S269E, and S282E. We also generated mutants carrying Ala in these positions. All these proteins were overexpressed in E. coli and purified to homogeneity by affinity chromatography, as judged by SDS-PAGE (Figure 3B).
RDRP activity assays were carried out using oligonucleotide LE19, which allows de novo initiation and primer extension to be analyzed at the same time. Results with Ala mutants showed activity levels below 50% compared to WT in all cases and for both types of activity. Of all the Ala mutants tested, T267A showed the highest activity levels (Figure 4). Assays with the Glu mutants showed imperceptible activity levels for all mutants except T267E, which showed RNA polymerase activity similar to or even higher than the WT protein (Figure 5). Therefore, changes that mimic or abrogate phosphorylation at the NS5B protein positions identified to be phosphorylated by Akt yield proteins with much lower levels of RDRP activity than the WT protein for both de novo and primer-dependent synthesis, with the sole exception of the T267E mutant.
Figure 4. Elongation of LE19 oligonucleotide by de novo initiation and primer extension RDRP activity of NS5B Ala mutants. NS5B WT and Ala mutants of residues S27, S29, T53, T267, S269, and S282 were assayed as described in “Materials and Methods” section. Aliquots were stopped at 15, 30, and 60 min, and resolved in denaturing polyacrylamide gels (PAA 23%). (A) Representative experiment showing products obtained by primer extension (PE) and de novo initiation (DN) using LE19 RNA as a template. Recombinant proteins used are indicated at the top. (B) RNA-dependent RNA polymerase de novo activity products at 60 min were quantified and represented in arbitrary units. (C) RNA-dependent RNA polymerase primer extension activity products at 60 min were quantified and represented in arbitrary units. The mutated residue (Ala mutant) corresponding to each bar is indicated at the bottom. Values in (B,C) are the averages (normalized to WT) and corresponding Standard Errors of the Mean (SEM) from at least four independent experiments. Statistically significant differences (Student’s t-test) are represented as follows: *p < 0.05; **p < 0.005; ***p < 0.0005. Asterisks over the bar indicate the p-value comparing to WT.
Figure 5. Elongation of LE19 oligonucleotide by de novo initiation and primer extension of NS5B Glu mutants. (A) Representative experiment showing products obtained by primer extension (PE) and de novo initiation (DN) by NS5B WT and mutants imitating phosphorylation (Glu) of residues S27, S29, T53, T267, S269, and S282, using LE19 RNA as a template. Recombinant proteins used are indicated at the top. Quantitative analysis of RNA-dependent RNA polymerase de novo (B) and primer extension (C) activity products at 60 min quantified as described in Figure 4. The mutated residue (Glu mutant) corresponding to each bar is shown at the bottom. Values in (B,C) are the averages (normalized to WT) and corresponding Standard Errors of the Mean (SEM) from at least four independent experiments. Statistically significant differences (Student’s t-test) are represented as follows: **p < 0.005; ***p < 0.0005. Asterisks over the bar indicate the p-value comparing to WT.
Phosphorylation of Ser and Thr residues leads to the introduction of negative net charges in these positions. Ser282, one of the NS5B residues that becomes phosphorylated by Akt, is part of the active center of the polymerase. We therefore wanted to analyze whether the mutant that mimics phosphorylation in this position maintains the ability to bind RNA. For this purpose, we carried out electromobility shift assay (EMSA) experiments comparing WT and S282E proteins. First, we determined the concentration of WT protein that was able to bind and delay a fixed RNA concentration (corresponding to 10,000 cpm) and at a 100 mM NaCl concentration. The results show that at 100 nM protein all RNA is forming RNA:protein complexes (Figure 6A). Then, with those conditions (10,000 cpm RNA and 100 nM protein) as starting point, we performed electromobility shift assays varying the NaCl concentration to compare WT and S282E proteins. Since RNA:protein interactions are predominantly electrostatic, an increase in ionic strength will result in the loss of interaction. When the experiment was carried out at the lowest NaCl concentration (30 mM), all of the RNA with WT protein is forming RNA:protein complexes (Figure 6B). However, with the protein carrying the S282E mutation under these conditions, a free-form RNA band is observed, indicating less interaction between RNA and mutant protein compared to the WT protein (Figure 6B). In addition, whereas the WT protein even shows an intense band corresponding to RNA: protein complexes at the highest NaCl concentration tested (530 mM), the mutant protein shows very faint complex bands at concentrations higher than 330 mM NaCl (Figure 6B). These results indicate that, under the same conditions, the interaction of RNA with the S282E mutant protein is weaker than with the WT protein.
Figure 6. Electromobility shift assays. (A) Gel showing retardation of a labeled RNA (10,000 cpm each lane) by increasing concentrations of WT NS5B. Concentration of WT NS5B is indicated at the top (nM). The protein concentration chosen for the following experiments is indicated by a blue box. (B) Gel showing retardation of a labeled RNA by WT and S282E NS5B proteins at increasing concentrations (30, 130, 230, 330, 430, and 530 mM) of NaCl. Free labeled probe (RNA) and retarded products (NS5B:RNA) are indicated.
Next, the effect of NS5B phosphorylation by Akt or, alternatively, its inability to completely phosphorylate the polymerase, on HCV’s replicative capacity was analyzed. To do this, we constructed T53A, T53E, S282A, and S282E mutants in the Jc1FLAG2(p7-nsGluc2A) plasmid, the resulting plasmids were transcribed in vitro, and the RNA product of the transcription was used to transfect Huh-7.5 cells. The supernatant from these transfected cells was used to titrate for the presence of HCV following a described procedure (Perales et al., 2013; Sheldon et al., 2014). WT virus could be recovered from the supernatant of all three replicates of transfected cells with the corresponding RNA (Figure 7A). The average viral titer was 4.40 × 103 TCID50/ml at 6 days post-transfection, and 1.96 × 103 TCID50/ml at 15 days post-transfection. On the contrary, only two of the mutant virus replicates, both corresponding to the T53A mutant 15 days post-transfection, gave a viral titer value above the cut-off value (Figure 7A). T53A TCID50/ml values were 4.64 × 101 and 2.43 × 101.
Figure 7. Infectivity of T53 and S282 mutants. (A) TCID50/ml values obtained 6 and 15 days post-transfection (blue and red bars, respectively) after transfecting Huh7.5 cells with RNA corresponding to WT virus and the T53A, T53E, S282A, and S282E mutants. The threshold value was established at 10 TCID50/ml. Statistically significant differences (Student’s t-test) are represented as follows: *p < 0.05. Asterisk over the bar indicate the p-value comparing to WT. (B) Chromatogram showing the sequence obtained from the RNA of the T53A mutant rescued 15 days post-transfection. The GCT codon corresponding to the amino acid Ala is identified.
To rule out that the virus collected from the supernatant of the cells transfected with the mutant T53A was a product of mutation reversal, we purified the viral RNA, subjected it to an RT-PCR reaction and sequenced the DNA product. The result allowed us to confirm that the T53A change was present in the rescued virus (Figure 7B).
Previous work in our laboratory has shown that the cellular kinase Akt interacts with and phosphorylates the HCV polymerase NS5B (Valero et al., 2016). Now, we have identified phosphorylated positions, and analyzed the effect these modifications have on RDRP activity and virus replication. We identified positions S29 (or much less likely S27), T53, S269 (or much less probable T267) and S282 as substrates of the cellular Akt kinase (Figure 1). Coverage of the NS5B proteomic analysis was 70% of the sequence, leaving the possibility that some other phosphorylable positions have not been identified. Previous work has shown that Akt is important for virus replication in HCV-infected cells in culture (13 and references therein), suggesting that the HCV-Akt relationship is important in vivo and supporting the in vitro results described in the present work. Residue T267 is not conserved among genotypes (Figure 8A), and mutations at this position rendered the highest RDRP activity values among all mutants analyzed in this study, and even better than WT in the case of the T267E mutant. Residue T53 has not been described so far in relation to NS5B activity nor HCV replication. S29 and S282 residues have been previously described as important for both RDRP activity and viral replication. S29 has already been described previously as substrate of other important kinases in the HCV replicative cycle (Han et al., 2014; Hernandez et al., 2015). Furthermore, S29 is the homologous position of T33 of norovirus, which is also phosphorylated by Akt (Eden et al., 2011). These antecedents indicate us that phosphorylation of S29 seems to be very important for the replicative cycle of HCV (Han et al., 2014). Finally, S282 is a residue involved in substrate recognition by interacting with other amino acids (D225) and ribonucleotide substrates (Figure 8B; Appleby et al., 2015). Residue S282 has also been related to sofosbuvir and ribavirin resistance (Aloia et al., 2012; Ji et al., 2015; Kulkarni et al., 2016; Fourati et al., 2019). Therefore, with the exception of position T267, the positions identified in this work that are modified by Akt are critical for the RDRP activity of the NS5B protein as any change greatly affects RDRP activity.
Figure 8. Sequence and structure of the NS5B protein. (A) Partial amino acid sequence alignment of the consensus sequences of the major HCV genotypes (G1, G2, G3, G4, G5, and G6) showing the positions described in this work (S27, S29, T53, S269, and S282 in red and T267 in blue) as well as the surrounding residues. The consensus sequence is shown at the top (C). Conserved residues are represented as a dot. (B) Zoom of the fingertip region of the NS5B protein showing residues S29 (magenta, fingertip domain) and R503 (blue, thumb domain) and the hydrogen bond connecting them (red dashed line). (C) Zoom of the catalytic center of NS5B obtained by aligning the protein structures in the presence of ADP (blue backbone) and sofosbuvir (orange backbone). The catalytic triad coordinated with beta and gamma phosphates of the incoming nucleotide (ADP in light green and sofosbuvir in dark green), and positions D225 and S282 are shown. The most likely rotamers for the S282E mutant are shown in red. (D) Zoom showing the Δ1 loop in dark gray. Fingertips with the amino acid S29 and its partner R503 are shown on the left side of the image. On the right are residues S1 (gray), W6 (green) and T53 (orange), as well as the most likely rotamer for the T53E mutant (in red). Hydrogen-bridging bonds between T53 and W6 are shown with dotted blue lines.
S29 is located in the fingertips subdomain and is part of the residues involved in fingers-thumb interaction (Cai et al., 2005; Figures 3, 8B). Previous in vitro work focused on this NS5B region showed that fingers:thumb interactions were key for RDRP activity, binding of RNA and for the transition from de novo initiation to primer extension (Chinnaswamy et al., 2008, 2010). In addition, viruses carrying the S29A mutation have serious replication defects in cell culture (Han et al., 2014), which is consistent with the low levels of RDRP activity we have found (Figures 4, 5). The S29E change decreases the distance with R503, a residue that is at binding distance and is also part of the fingers:thumb interaction region (Figure 8B). The distance between S29 and R503 is between 3.3 and 3.8 Å, while the distance between the most stable rotamer of mutant S29E and R503 is between 3.4 and 2.6 Å. In addition, the phosphorylation of S29 introduces a negative charge in that location, and, considering the proximity of the R503 residue (≈3 Å), the interactions of this area could be distorted. This NS5B region must undergo large conformational changes for allowing the transition from de novo synthesis to primer extension (Chinnaswamy et al., 2008, 2010; Appleby et al., 2015). Mutations in these positions (e.g., phosphorylation of S29) could prevent these conformational changes or even impair a proper folding of the NS5B protein to carry out the replication of the genome.
Position S282 is part of the NS5B catalytic pocket (Figure 8C). This residue is part of the motif B next to D225 and is essential for the accurate positioning of NTP and the subsequent formation of the phosphodiester bond. The crystalline structure of NS5B in the presence of ADP shows that S282 has a hydrogen bond with the ribose of the nucleotide (Figure 8C; Appleby et al., 2015). By introducing the mutation S282E to mimic the phosphorylation in that position, all the rotamers including the more stable ones lost that hydrogen bond (Figure 8A). Previously, it has been described that changes S282T/G/C/R are associated with resistance to sofosbuvir (Lam et al., 2012; Perales et al., 2015; Chen et al., 2016; Gane et al., 2017). Changes at this position, even the most subtle (S282T) that could be also phosphorylated by Akt, give rise to viruses with very low replication efficiency (Suda et al., 2019; Figure 7). The crystalline structure of the NS5B protein with sofosbuvir located in its active center has been determined (Appleby et al., 2015). This structure showed that S282 is displaced to avoid steric hindrance (compare the protein structures with ADP in cyan and with sofosbuvir in orange in Figure 8C). In addition, the S282E mutation does not allow the hydrogen bond with the ribose ring of the incoming nucleotide in any of the structures, neither in the presence of ADP nor sofosbuvir, as the WT protein does. The lack of an interaction network together with the displacement of protein motifs could be related to the results shown in Figures 4–7.
Position T53 is the least studied of the above-mentioned phosphorylable residues. It is fully conserved in all HCV genotypes. The amino acid T53 is probably involved in maintaining the structure of the Δ1 loop and fingertips through interactions with other amino acids such as W6, with which it establishes hydrogen bonds (Figure 8D). Other hydrogen bonds involved would be those formed by residues S1:R56, M2:R56, S3:V52, S3:F54, and E17:S42. Notably, the Δ1 loop S42 residue is a substrate of the serine kinase PRK2, and mutations at this position also affect RDRP activity and HCV replication (Han et al., 2014). Therefore, phosphorylation of S42 and T53, together with S29, could be destabilizing the interactions that allow the maintenance of an active NS5B structure. The large number of interactions between positions 1–6 and 52–56 could be responsible for maintaining the structure of this region of the NS5B protein and thus for the replication of the T53A mutant (Figure 7), albeit at much lower levels than the parental virus.
In summary, the positions described in this work are phosphorylated in vitro by Akt, a Ser/Thr kinase involved in many cellular processes. The positions identified are critical for NS5B RDRP activity, and Ser/Thr to Glu changes at these positions (imitating phosphorylation) as well as changes to Ala result in loss of HCV polymerase activity. Furthermore, mutants that mimic phosphorylation are unable to replicate in cell culture. The reason why HCV polymerase is a substrate for cellular kinases is currently unclear. The inactive NS5B protein product of phosphorylation might be able to interact with other viral or cellular proteins, thus modulating the viral and cellular replicative cycle. These lines of research are currently being explored in our laboratory.
The raw data supporting the conclusions of this article will be made available by the authors, without undue reservation.
RS and AM: conceptualization, formal analysis, and writing—original draft preparation. RS, LA-G, BP, PC-C, ER, CG-C, IG, AÁ, and AM: investigation. RS, CP, ED, and AM: methodology. RS, LA-G, PC-C, and AM: writing—review and editing. CP, ED, and AM: funding acquisition. All authors contributed to the article and approved the submitted version.
The proteomic analysis: Protein Identification by LC-MS/MS was carried out in the “CBMSO PROTEIN CHEMISTRY FACILITY” that belongs to ProteoRed, PRB3-ISCIII, supported by Grant PT17/0019. The work at UCLM was funded by MINISTERIO DE CIENCIA E INNOVACIÓN, Grant Nos. SAF2016-80451-P and PID2019-106068GB-I00 and UNIVERSIDAD DE CASTILLA-LA MANCHA Grant Nos. GI20163501, GI20174100, and 2019-GRIN-27080. BP is supported by predoctoral contract 2020-PREDUCLM-16195 from UCLM. The work at CBMSO and IIS-FJD was supported by grants SAF2017-87846-R and BFU2017-91384-EXP from Ministerio de Ciencia, Innovación y Universidades (MCIU), PI18/00210 from Instituto de Salud Carlos III, and S2018/BAA-4370 (PLATESA2 from Comunidad de Madrid/FEDER). CP was supported by the Miguel Servet program of the Instituto de Salud Carlos III (CPII19/00001), cofinanced by the European Regional Development Fund (ERDF). CG-C was supported by the predoctoral contract PRE2018-083422 from MCIU. CIBERehd (Centro de Investigación en Red de Enfermedades Hepáticas y Digestivas) was funded by the Instituto de Salud Carlos III. Institutional grants from the Fundación Ramón Areces and Banco Santander to the CBMSO are also acknowledged. The team at CBMSO belongs to the Global Virus Network (GVN).
The authors declare that the research was conducted in the absence of any commercial or financial relationships that could be construed as a potential conflict of interest.
All claims expressed in this article are solely those of the authors and do not necessarily represent those of their affiliated organizations, or those of the publisher, the editors and the reviewers. Any product that may be evaluated in this article, or claim that may be made by its manufacturer, is not guaranteed or endorsed by the publisher.
The Huh7.5 cell line was kindly supplied by Bartenschlager (University of Heidelberg). Dr. Sandra Franco is acknowledged for valuable discussions about generation of HCVp0 wt and mutant viruses. Dr. Piet de Groot is acknowledged for critical reading the manuscript. José Joaquín Lorenzo was acknowledged for technical assistance.
Albentosa-Gonzalez, L., Sabariegos, R., Arias, A., Clemente-Casares, P., and Mas, A. (2021). Akt interacts with usutu virus polymerase, and its activity modulates viral replication. Pathogens 10:244. doi: 10.3390/pathogens10020244
Aloia, A. L., Locarnini, S., and Beard, M. R. (2012). Antiviral resistance and direct-acting antiviral agents for HCV. Antivir Ther. 17(6 Pt B), 1147–1162. doi: 10.3851/imp2426
Appleby, T. C., Perry, J. K., Murakami, E., Barauskas, O., Feng, J., Cho, A., et al. (2015). Viral replication. Structural basis for RNA replication by the hepatitis C virus polymerase. Science 347, 771–775.
Barik, S., McLean, T., and Dupuy, L. C. (1995). Phosphorylation of Ser232 directly regulates the transcriptional activity of the P protein of human respiratory syncytial virus: phosphorylation of Ser237 may play an accessory role. Virology 213, 405–412. doi: 10.1006/viro.1995.0013
Cai, Z., Yi, M., Zhang, C., and Luo, G. (2005). Mutagenesis analysis of the rGTP-specific binding site of hepatitis C virus RNA-dependent RNA polymerase. J. Virol. 79, 11607–11617. doi: 10.1128/jvi.79.18.11607-11617.2005
Chen, Z. W., Li, H., Ren, H., and Hu, P. (2016). Global prevalence of pre-existing HCV variants resistant to direct-acting antiviral agents (DAAs): mining the GenBank HCV genome data. Sci. Rep. 6:20310.
Chinnaswamy, S., Murali, A., Li, P., Fujisaki, K., and Kao, C. C. (2010). Regulation of de novo-initiated RNA synthesis in hepatitis C virus RNA-dependent RNA polymerase by intermolecular interactions. J. Virol. 84, 5923–5935. doi: 10.1128/jvi.02446-09
Chinnaswamy, S., Yarbrough, I., Palaninathan, S., Kumar, C. T., Vijayaraghavan, V., Demeler, B., et al. (2008). A locking mechanism regulates RNA synthesis and host protein interaction by the hepatitis C virus polymerase. J. Biol. Chem. 283, 20535–20546. doi: 10.1074/jbc.m801490200
Clemente-Casares, P., Lopez-Jimenez, A. J., Bellon-Echeverria, I., Encinar, J. A., Martinez-Alfaro, E., Perez-Flores, R., et al. (2011). De novo polymerase activity and oligomerization of hepatitis C virus RNA-dependent RNA-polymerases from genotypes 1 to 5. PLoS One 6:e18515. doi: 10.1371/journal.pone.0018515
de Chassey, B., Navratil, V., Tafforeau, L., Hiet, M. S., Aublin-Gex, A., Agaugue, S., et al. (2008). Hepatitis C virus infection protein network. Mol. Syst. Biol. 4:230.
Dolan, P. T., Zhang, C., Khadka, S., Arumugaswami, V., Vangeloff, A. D., Heaton, N. S., et al. (2013). Identification and comparative analysis of hepatitis C virus-host cell protein interactions. Mol. Biosyst. 9, 3199–3209. doi: 10.1039/c3mb70343f
Eden, J. S., Sharpe, L. J., White, P. A., and Brown, A. J. (2011). Norovirus RNA-dependent RNA polymerase is phosphorylated by an important survival kinase. Akt. J. Virol. 85, 10894–10898. doi: 10.1128/jvi.05562-11
Fourati, S., Rodriguez, C., Hezode, C., Soulier, A., Ruiz, I., Poiteau, L., et al. (2019). Frequent antiviral treatment failures in patients infected with hepatitis C virus genotype 4. Subtype 4r. Hepatology 69, 513–523. doi: 10.1002/hep.30225
Gane, E. J., Metivier, S., Nahass, R., Ryan, M., Stedman, C. A., Svarovskaia, E. S., et al. (2017). The emergence of NS5B resistance associated substitution S282T after sofosbuvir-based treatment. Hepatol. Commun. 1, 538–549. doi: 10.1002/hep4.1060
Hagen, N., Bayer, K., Rosch, K., and Schindler, M. (2014). The intraviral protein interaction network of hepatitis C virus. Mol. Cell Proteomics 13, 1676–1689. doi: 10.1074/mcp.m113.036301
Hamamoto, I., Nishimura, Y., Okamoto, T., Aizaki, H., Liu, M., Mori, Y., et al. (2005). Human VAP-B is involved in hepatitis C virus replication through interaction with NS5A and NS5B. J. Virol. 79, 13473–13482. doi: 10.1128/jvi.79.21.13473-13482.2005
Han, S. H., Kim, S. J., Kim, E. J., Kim, T. E., Moon, J. S., Kim, G. W., et al. (2014). Phosphorylation of hepatitis C virus RNA polymerases ser29 and ser42 by protein kinase C-related kinase 2 regulates viral RNA replication. J. Virol. 88, 11240–11252. doi: 10.1128/jvi.01826-14
Hernandez, S., Figueroa, D., Correa, S., Diaz, A., Aguayo, D., and Villanueva, R. A. (2015). Phosphorylation at the N-terminal finger subdomain of a viral RNA-dependent RNA polymerase. Biochem. Biophys. Res. Commun. 466, 21–27. doi: 10.1016/j.bbrc.2015.08.082
Hillung, J., Ruiz-Lopez, E., Bellon-Echeverria, I., Clemente-Casares, P., and Mas, A. (2012). Characterization of the interaction between hepatitis C virus NS5B and the human oestrogen receptor alpha. J. Gen. Virol. 93(Pt 4), 780–785. doi: 10.1099/vir.0.039396-0
Inoue, Y., Aizaki, H., Hara, H., Matsuda, M., Ando, T., Shimoji, T., et al. (2011). Chaperonin TRiC/CCT participates in replication of hepatitis C virus genome via interaction with the viral NS5B protein. Virology 410, 38–47. doi: 10.1016/j.virol.2010.10.026
Jakubiec, A., Tournier, V., Drugeon, G., Pflieger, S., Camborde, L., Vinh, J., et al. (2006). Phosphorylation of viral RNA-dependent RNA polymerase and its role in replication of a plus-strand RNA virus. J. Biol. Chem. 281, 21236–21249. doi: 10.1074/jbc.m600052200
Ji, H., Kozak, R. A., Biondi, M. J., Pilon, R., Vallee, D., Liang, B. B., et al. (2015). Next generation sequencing of the hepatitis C virus NS5B gene reveals potential novel S282 drug resistance mutations. Virology 477, 1–9. doi: 10.1016/j.virol.2014.12.037
Kulkarni, A. S., Damha, M. J., Schinazi, R. F., Mo, H., Doehle, B., Sagan, S. M., et al. (2016). A complex network of interactions between S282 and G283 of hepatitis C virus nonstructural protein 5b and the template strand affects susceptibility to sofosbuvir and ribavirin. Antimicrob Agents Chemother. 60, 2018–2027. doi: 10.1128/aac.02436-15
Kusakawa, T., Shimakami, T., Kaneko, S., Yoshioka, K., and Murakami, S. (2007). Functional interaction of hepatitis C Virus NS5B with Nucleolin GAR domain. J. Biochem. 141, 917–927. doi: 10.1093/jb/mvm102
Lam, A. M., Espiritu, C., Bansal, S., Micolochick Steuer, H. M., Niu, C., Zennou, V., et al. (2012). Genotype and subtype profiling of PSI-7977 as a nucleotide inhibitor of hepatitis C virus. Antimicrob Agents Chemother. 56, 3359–3368. doi: 10.1128/aac.00054-12
Liu, Z., Tian, Y., Machida, K., Lai, M. M., Luo, G., Foung, S. K., et al. (2012). Transient activation of the PI3K-AKT pathway by hepatitis C virus to enhance viral entry. J. Biol. Chem. 287, 41922–41930. doi: 10.1074/jbc.m112.414789
Lopez-Jimenez, A. J., Clemente-Casares, P., Sabariegos, R., Llanos-Valero, M., Bellon-Echeverria, I., Encinar, J. A., et al. (2014). Hepatitis C virus polymerase-polymerase contact interface: significance for virus replication and antiviral design. Antiviral Res. 108, 14–24. doi: 10.1016/j.antiviral.2014.04.009
Munakata, T., Nakamura, M., Liang, Y., Li, K., and Lemon, S. M. (2005). Down-regulation of the retinoblastoma tumor suppressor by the hepatitis C virus NS5B RNA-dependent RNA polymerase. Proc. Natl. Acad. Sci. U S A. 102, 18159–18164. doi: 10.1073/pnas.0505605102
Neufeldt, C. J., Cortese, M., Acosta, E. G., and Bartenschlager, R. (2018). Rewiring cellular networks by members of the flaviviridae family. Nat. Rev. Microbiol. 16, 125–142. doi: 10.1038/nrmicro.2017.170
Perales, C., Beach, N. M., Gallego, I., Soria, M. E., Quer, J., Esteban, J. I., et al. (2013). Response of hepatitis C virus to long-term passage in the presence of alpha interferon: multiple mutations and a common phenotype. J. Virol. 87, 7593–7607. doi: 10.1128/jvi.02824-12
Perales, C., Quer, J., Gregori, J., Esteban, J. I., and Domingo, E. (2015). Resistance of hepatitis c virus to inhibitors: complexity and clinical implications. Viruses 7, 5746–5766. doi: 10.3390/v7112902
Qadri, I., Choudhury, M., Rahman, S. M., Knotts, T. A., Janssen, R. C., Schaack, J., et al. (2012). Increased phosphoenolpyruvate carboxykinase gene expression and steatosis during hepatitis C virus subgenome replication: role of nonstructural component 5A and CCAAT/enhancer-binding protein beta. J. Biol. Chem. 287, 37340–37351. doi: 10.1074/jbc.m112.384743
Qian, X., Xu, C., Wu, B., Tang, H., Zhao, P., and Qi, Z. (2020). SNORD126 promotes hepatitis C virus infection by upregulating Claudin-1 via activation of PI3K-AKT signaling pathway. Front. Microbiol. 11:565590. doi: 10.3389/fmicb.2020.565590
Schmid, S., Mayer, D., Schneider, U., and Schwemmle, M. (2007). Functional characterization of the major and minor phosphorylation sites of the P protein of Borna disease virus. J. Virol. 81, 5497–5507. doi: 10.1128/jvi.02233-06
Sesmero, E., and Thorpe, I. F. (2015). Using the hepatitis C virus RNA-Dependent RNA polymerase as a model to understand viral polymerase structure. Funct. Dynam. Viruses 7, 3974–3994. doi: 10.3390/v7072808
Sheldon, J., Beach, N. M., Moreno, E., Gallego, I., Pineiro, D., Martinez-Salas, E., et al. (2014). Increased replicative fitness can lead to decreased drug sensitivity of hepatitis C virus. J. Virol. 88, 12098–12111. doi: 10.1128/jvi.01860-14
Suda, G., Kimura, M., Shigesawa, T., Suzuki, K., Nakamura, A., Ohara, M., et al. (2019). Effects of resistance-associated variants in genotype 2 hepatitis C virus on viral replication and susceptibility to antihepatitis C virus drugs. Hepatol. Res. 49, 1275–1285. doi: 10.1111/hepr.13401
Tabata, K., Neufeldt, C. J., and Bartenschlager, R. (2020). Hepatitis C virus replication. Cold Spring Harb. Perspect. Med. 10:a037093.
Valero, M. L., Sabariegos, R., Cimas, F. J., Perales, C., Domingo, E., Sanchez-Prieto, R., et al. (2016). Hepatitis C virus RNA-Dependent RNA polymerase interacts with the Akt/PKB kinase and induces its subcellular relocalization. Antimicrob Agents Chemother 60, 3540–3550. doi: 10.1128/aac.03019-15
Watashi, K., Ishii, N., Hijikata, M., Inoue, D., Murata, T., Miyanari, Y., et al. (2005). Cyclophilin B is a functional regulator of hepatitis C virus RNA polymerase. Mol. Cell 19, 111–122. doi: 10.1016/j.molcel.2005.05.014
Keywords: HCV (hepatitis C), NS5B (non-structural protein) polymerase, Akt, virus replication, phosphorylation
Citation: Sabariegos R, Albentosa-González L, Palmero B, Clemente-Casares P, Ramírez E, García-Crespo C, Gallego I, de Ávila AI, Perales C, Domingo E and Mas A (2021) Akt Phosphorylation of Hepatitis C Virus NS5B Regulates Polymerase Activity and Hepatitis C Virus Infection. Front. Microbiol. 12:754664. doi: 10.3389/fmicb.2021.754664
Received: 06 August 2021; Accepted: 20 September 2021;
Published: 22 October 2021.
Edited by:
Eva Mittler, Albert Einstein College of Medicine, United StatesReviewed by:
Leiliang Zhang, Shandong First Medical University, ChinaCopyright © 2021 Sabariegos, Albentosa-González, Palmero, Clemente-Casares, Ramírez, García-Crespo, Gallego, de Ávila, Perales, Domingo and Mas. This is an open-access article distributed under the terms of the Creative Commons Attribution License (CC BY). The use, distribution or reproduction in other forums is permitted, provided the original author(s) and the copyright owner(s) are credited and that the original publication in this journal is cited, in accordance with accepted academic practice. No use, distribution or reproduction is permitted which does not comply with these terms.
*Correspondence: Antonio Mas, QW50b25pby5NYXNAdWNsbS5lcw==
Disclaimer: All claims expressed in this article are solely those of the authors and do not necessarily represent those of their affiliated organizations, or those of the publisher, the editors and the reviewers. Any product that may be evaluated in this article or claim that may be made by its manufacturer is not guaranteed or endorsed by the publisher.
Research integrity at Frontiers
Learn more about the work of our research integrity team to safeguard the quality of each article we publish.