- 1Division of Discovery Biology, Bacteriology and Microbiome, Elanco Animal Health, Greenfield, IN, United States
- 2Division of Global Computational Sciences, Elanco Animal Health, Greenfield, IN, United States
- 3Division of Nutritional Health, Elanco Animal Health, Greenfield, IN, United States
Microbial feed ingredients or probiotics have been used widely in the poultry industry to improve production efficiency. Spore-forming Bacillus spp. offer advantages over traditional probiotic strains as Bacillus spores are resilient to high temperature, acidic pH, and desiccation. This results in increased strain viability during manufacturing and feed-pelleting processes, extended product shelf-life, and increased stability within the animal’s gastrointestinal tract. Despite numerous reports on the use of Bacillus spores as feed additives, detailed characterizations of Bacillus probiotic strains are typically not published. Insufficient characterizations can lead to misidentification of probiotic strains in product labels, and the potential application of strains carrying virulence factors, toxins, antibiotic resistance, or toxic metabolites. Hence, it is critical to characterize in detail the genomic and phenotypic properties of these strains to screen out undesirable properties and to tie individual traits to clinical outcomes and possible mechanisms. Here, we report a screening workflow and comprehensive multi-omics characterization of Bacillus spp. for use in broiler chickens. Host-derived Bacillus strains were isolated and screened for desirable probiotic properties. The phenotypic, genomic and metabolomic analyses of three probiotic candidates, two Bacillus amyloliquefaciens (Ba ATCC PTA126784 and ATCC PTA126785), and a Bacillus subtilis (Bs ATCC PTA126786), showed that all three strains had promising probiotic traits and safety profiles. Inclusion of Ba ATCC PTA12684 (Ba-PTA84) in the feed of broiler chickens resulted in improved growth performance, as shown by a significantly improved feed conversion ratio (3.3%), increased of European Broiler Index (6.2%), and increased average daily gain (ADG) (3.5%). Comparison of the cecal microbiomes from Ba PTA84-treated and control animals suggested minimal differences in microbiome structure, indicating that the observed growth promotion presumably was not mediated by modulation of cecal microbiome.
Introduction
An increasing demand for poultry meat and egg has put significant pressure on the poultry industry to improve production efficiency. The Food and Agriculture Organization of the United Nations (FAO) projected that global meat and egg consumption will increase by 52 and 39%, respectively, in 2050 compared to 2012 (FAO-UN, 2018). The challenge is further heightened by restrictions from European Union and United States regulatory bodies on the use of antibiotics as growth promoters (AGPs) and prophylactic care; this change is due to public health concerns related to the development and spread of antibiotic resistant bacteria (US-FDA, 2013; Koutsoumanis et al., 2020). Antibiotics have been used as AGPs for over half a century, aiding growth performance and controlling disease outbreaks (Moore et al., 1946).
For the above reasons, microbial feed ingredients, also called direct-fed microorganisms (DFMs) or probiotics, have attracted tremendous interest as an alternative to AGPs to support improved production efficiency. Probiotics are defined as “live microorganisms that, when administered in adequate amounts, confer a health benefit on the host” (Hill et al., 2014). Probiotics are believed to exert their benefits through the following proposed mechanisms: assisting with nutrition and digestion, competitive exclusion of pathogens, modulating the immune system and gut microbiota, improving epithelial integrity, and/or producing small molecule metabolites that are beneficial to the host (Grant et al., 2018; Jha et al., 2020). In addition to the above probiotic effects, microorganisms used as probiotics must survive environmental and processing challenges prior to reaching their target site. This includes low acidity of the upper gastrointestinal tract (GIT), bile acid toxicity, and heat exposure during feed pelleting application, which could pose challenges to the use of traditional probiotic strains such as those belonging to the genera Lactobacillus and Bifidobacterium, as they are often sensitive to some of these extreme conditions.
Endospore-forming Bacillus spp. offer advantages over traditional probiotic strains due to the ability of Bacillus spores to withstand hostile environments such as high temperature, desiccation, and acidic pH, resulting in increased viability during the manufacturing and feed-pelleting process, increased stability inside animals’ GIT and extended product shelf-life. Thus, Bacillus strains have been widely used to support improved production parameters (Barbosa et al., 2005; Guo et al., 2006; Setlow, 2006; Shivaramaiah et al., 2011). Bacillus spp., commonly found in soil, enter the animal GIT through feed or ingestion of fecal material. Once inside the GIT, the spores germinate into metabolically active vegetative cells, eliciting their probiotic effect (Hoa et al., 2001; Casula and Cutting, 2002; Latorre et al., 2014; Bernardeau et al., 2017). Within the Bacillus genus, species commonly used as probiotics are Bacillus subtilis, Bacillus coagulans, Bacillus clausii, Bacillus amyloliquefaciens, and Bacillus licheniformis (Mingmongkolchai and Panbangred, 2018). Bacillus strains are known to produce commercial enzymes, antimicrobial peptides, and small metabolites that may confer health benefits to the host by supporting improved feed digestion, suppressing undesirable organisms, and by maintaining a healthy gut microbiota and immune system [reviewed in Grant et al. (2018)].
Despite several reports on the benefits of Bacillus spores as probiotics and DFMs on human and animal health, respectively, detailed characterizations of Bacillus spp. strains to not only help explain their potential probiotic properties but also to evaluate their safety are typically unavailable in the public domain. Insufficient characterization of probiotic strains could result in the following undesirable outcomes: (1) misleading identification at the genus and species levels of probiotic strains in commercial products; and (2) the occurrence of antimicrobial resistance traits and toxins in probiotic strains that could negatively impact the host and raise public health concerns. As an example of the first instance, commercial probiotics labeled as B. coagulans were later shown to be Lactobacillus sporogenes, and B. clausii-containing probiotics were mislabeled as comprised of B. subtilis (Green et al., 1999; Weese, 2002; Weese and Martin, 2011).
To fill the knowledge gap in the genomic and phenotypic characterization of Bacillus spp. DFMs, we take advantage of DNA sequencing and omics technologies for the comprehensive identification, screening, and characterization of Bacillus spp. to assess their safety and efficacy as probiotic candidates. Detailed strain characterizations employing multi-omics approaches could uncover correlations between strain properties and the effects of probiotic administration on the host, underpin possible mechanisms of action of probiotic strains, identify biomolecules that could be used in place of live bacteria (i.e., peptides, enzymes, and metabolites), and help to rationally design strain consortia to maximize positive effects on the host.
Here, we present a comprehensive screening and multi-omics characterization for Bacillus spp. as probiotics for use in poultry. Our analysis provides insights into the genotypic, phenotypic, and metabolomic properties of three Bacillus spp. strains that show desirable probiotic traits and safety profiles. Furthermore, results from a clinical study of the administration of one of the strains -B. amyloliquefaciens Ba PTA84- showed a significant improvement of broiler growth performance. Such in-depth characterization and the data made available will guide future efforts to develop next generation probiotics, microbial-derived nutritional health products, and inform decisions to design microbial consortia for the potential improvement of poultry production efficiency.
Materials and Methods
Microbial Strains and Growth Conditions
The Bacillus spp. strains were routinely grown in Lysogeny Broth (LB) and incubated at 37°C overnight while shaking at 200 rpm. Avian pathogenic Escherichia coli (APEC) serotypes O2, O18, O78, and Clostridium perfringens NAH 1314-JP1011 were obtained from the Elanco pathogen library. Salmonella enterica serovar Typhimurium ATCC 14028 was purchased from the American Type Culture Collection (ATCC, Manassas, VA, United States). E. coli strains and S. Typhimurium, were routinely grown in LB, and C. perfringens was grown in anaerobic Brain Heart Infusion (BHI) broth supplemented with yeast extract (5.0 g/L) and L-cysteine (0.5 g/L). For growth in liquid culture, a colony from the respective agar plate was inoculated into a 10 mL tube containing liquid media and the tube was incubated in a shaker incubator at 37°C and 200 rpm for E. coli and S. Typhimurium, and statically at 39°C for C. perfringens inside a Bactron anaerobic chamber (Sheldon Manufacturing, Inc., Cornelius, OR, United States). The anaerobic chamber contained a mixture of N2:CO2:H2 (87.5:10:2.5, v/v/v).
Vero Cells Growth Condition
Vero cells were obtained from Elanco cell culture collection and were maintained in Opti-MEM® I reduced serum media containing 5% Fetal Bovine Serum (FBS) (Cytiva, Marlborough, MA, United States) and Gentamicin (Opti-5-Gent) (Life Technologies, Carlsbad, CA, United States). The serum-free cell culture medium was similarly prepared with Minimal Essential Medium with Earle’s Balanced Salt Solution (MEM/EBSS), 10% fetal bovine serum (FBS), 1% non-essential amino acids and 1% L-glutamine in place of FBS. To generate wells containing 100% confluent cells for the cytotoxicity assay, Vero cells grown for 2–3 days were divided into a 96-well flat bottom tissue culture plate (Fisher Scientific, Waltham, MA, United States) where each well contained 1 × 104 cells. The cells were then incubated on the plate for 48–72 h inside a CO2 incubator (37oC; %CO2 was maintained at 5 ± 1%).
Bacillus spp. Isolation and Identification
Bacillus Isolation
Bacillus spp. were isolated from cecal contents of healthy 30–42 day old chickens raised at poultry research farms in Arkansas, Georgia, and Indiana, United States employing a combination of a high-throughput isolation platform employing Prospector® (GALT, Inc., San Carlos, CA, United States) following the manufacture’s protocol, and a classical isolation method as described previously (Koransky et al., 1978). For both approaches, isolation protocols were preceded by selection of Bacillus spores from the starting cecal contents by applying heat at 95°C for 5 min or treatment with ethanol. For the latter, frozen cecal samples from the Elanco library preserved in BHI containing 20% glycerol were thawed and equal amounts of Tryptic Soy Broth (TSB) medium were added and mixed. An equal amount of absolute ethanol was added to the sample to a final concentration of 50% and the mixture was incubated at 30°C for an hour. The ethanol-treated samples were then used for isolation. For Bacillus spp. isolation employing conventional methods, 10-fold serial-dilution was applied to the treated cecal samples to ensure separate colonies recovered on agar plates. Each colony was purified by three sequential passages onto agar plates.
Strain Identification
For an initial strain identification, Bacillus cell lysates were sent to the TACGen genomic sequencing facility (Richmond, CA, United States) for strain identification. The strain identities were determined by Sanger sequencing of amplified regions of a partial length of 16S ribosomal RNA (rRNA) gene employing primers 27F (5′ AGA GTT TGA TCM TGG CTC AG 3′) and 1492R (5′ CGG TTA CCT TGT TAC GAC TT3′). The resulting 16S rRNA sequences were then searched against the NCBI 16S rRNA database using BLAST searches with an e-value cutoff of <10–20 and a percent sequence identity value of >95%. Strain identification of select isolates were further confirmed by ortholog analyses as described in the following section “Genome-Based Strain Identification and Comparative Genomic Analyses”.
In vitro Microorganism Inhibition Assay
Eight Bacillus spp. strains were screened for their antimicrobial activity against five microorganisms, namely APEC serotypes O2, O18, O78, Salmonella Typhimurium ATCC 14028, and C. perfringens NAH 1314-JP1011. The assays were modified from a protocol described in Latorre et al. (2016) and performed in duplicate. The detailed protocol is presented in Supplementary Materials.
Enzyme Activities
The β-mannanase assay was adapted from a protocol as described by McCleary (1978). Assays for amylase and protease activities were done following protocols in Latorre et al. (2016). These protocols are presented in Supplementary Materials.
Cytotoxicity Assay
Cytotoxicity assays of eight Bacillus culture supernatants were performed following the protocol described in EFSA guidelines (EFSA Panel on Additives and Products or Substances used in Animal Feed [FEEDAP] et al., 2018). A detailed protocol is described in Supplementary Materials. Culture supernatant of B. cereus ATCC 14579 and B. licheniformis ATCC14580 were used as positive and negative controls, respectively.
Antimicrobial Susceptibility Assessment
Antibiotic susceptibility assays of Bacillus spp. for tetracycline, chloramphenicol, streptomycin, kanamycin, erythromycin, vancomycin, gentamycin, ampicillin, and clindamycin were performed and assessed according to an EFSA guideline for Antimicrobial resistance of the Bacillus spp. as direct fed microbials (DFM) (EFSA Panel on Additives and Products or Substances used in Animal Feed [FEEDAP] et al., 2018). Bacillus spp. strains on LB agar plates were sent to Microbial Research, Inc. (Fort Collins, CO, United States) for analysis following protocols in compliance with Clinical Laboratory Standard Institute (CLSI) document VET01 (World, 2021). Briefly, minimum inhibitory concentration (MIC) plates were prepared using cation-adjusted Mueller Hinton Broth (MHB) and the antimicrobials were twofold serially diluted to obtain a final concentration range of 0.06–32 μg/mL. Growth of Bacillus spp. in the presence of each of nine antimicrobials with different dilutions was monitored. Susceptibility was interpreted as the lack of Bacillus spp. growth in the presence of antimicrobial at a concentration that was lower that the cut-off values of the respective antimicrobials described in the EFSA guideline. For quality control, the following organisms were used as controls, E. coli ATCC 25922, Enterococcus faecalis ATCC 29212, Pseudomonas aeruginosa ATCC 27853 and Staphylococcus aureus ATCC 29213.
Whole Genome Sequencing, Assembly, and Annotation
Genomic DNA Isolation
High molecular weight genomic DNA of Bacillus spp. were extracted employing a Phenol:Chloroform:Isoamyl alcohol (PCI) method as described previously (Rao et al., 2018). Bacterial cells were harvested by centrifugation at 7,000 × g for 10 min from an overnight culture of Bacillus spp. grown in 25 mL LB supplemented with 0.005% Tween 80 in 50 mL sterile Falcon tube (Fisher Scientific, Waltham, MA, United States). The resulting cell pellet was resuspended in 0.75 mL of 1X Tris-EDTA (TE) buffer (Life Technologies, Carlsbad, CA, United States), pH 8, containing Tris-HCl and EDTA at final concentrations of 10 and 1 mM, respectively, in a 2 mL Eppendorf tube (Fisher Scientific, Waltham, MA, United States). To lyse the cells, Lysozyme (Sigma Aldrich, St. Louis, MO, United States) was added at a final concentration of 7 mg/mL and the mixture was incubated at 37°C for an hour. Then, SDS and Proteinase K (Sigma-Aldrich, St. Louis, MO, United States) were added to the mixture at final concentrations of 2% and 400 μg/mL, respectively, and the lysate was incubated at 60°C for 1 h. To remove RNA from the cell lysate, 10 μL of RNase (Thermo Fisher Scientific, Waltham, MA, United States) were added and the mixture was incubated at 37°C for 30 min. An equal volume of a mixture of PCI (25:24:1, v/v/v) was added to the supernatant and was mixed by carefully inverting tubes 5–10 times rigorously. The aqueous phase containing DNA was separated from the organic phase by centrifugation at 12,000 × g for 15 min, and the top aqueous layer was collected into a fresh 2 mL Eppendorf tube. An equal volume of a mixture of Chloroform:Isoamyl alcohol (24:1, v/v) was added to this aqueous phase containing DNA, and mixed by carefully inverting the tube. The mixture was centrifuged at 12,000 × g for 10 min. DNA from the aqueous layer was precipitated by an addition of one tenth volume of sodium acetate (3 M, pH 5.2) followed by centrifugation at 16,000 × g for 20 min. The DNA pellet was washed three times with ice-cold 70% ethanol, air-dried, and resuspended in 0.5 mL 1X TE buffer.
PacBio Long Read Genome Sequencing
The bacterial genomic DNA samples were shipped on dry-ice to DNA Link, Inc. (San Diego, CA, United States) for whole genome sequencing using PacBio RSII platform. Briefly, 20 kb DNA fragments were generated by shearing genomic DNA using the covaris G-tube according to the manufacturer’s recommended protocol (Covaris, Woburn, MA, United States). Smaller fragments were purified by the AMpureXP bead purification system (Beckman Coulter, Brea, CA, United States). For library preparation, 5 μg of genomic DNA were used. The SMRTbell library was constructed using SMRTbellTM Template Prep Kit 1.0 (PacBio®, Menlo Park, CA, United States). Small fragments were removed using the BluePippin Size selection system (Sage Science, Beverly, MA, United States). The remaining DNA sample was used for large-insert library preparation. A sequencing primer was annealed to the SMRTbell template and DNA polymerase was bound to the complex using DNA/Polymerase Binding kit P6 (PacBio®, Menlo Park, CA, United States). Following the polymerase binding reaction, the MagBead was bound to the library complex with MagBeads Kit (PacBio®, Menlo Park, CA, United States). This polymerase-SMRTbell-adaptor complex was loaded into zero-mode waveguides. The SMRTbell library was sequenced by 2 PacBio SMRT cells (PacBio®, Menlo Park, CA, United States) using the DNA sequencing kit 4.0 with C4 chemistry (PacBio®, Menlo Park, CA, United States). A 1 × 240-min movie was captured for each SMRT cell using the PacBio RS sequencing platform.
Genome Assembly, Annotation and Features Prediction
The genome was assembled by DNA link, Inc. with HGAP.3. Genome annotation was carried out using a custom annotation pipeline by combining several prediction tools. Coding sequences, transfer RNA and transmembrane RNA were predicted and annotated using Prokka (Laslett and Canback, 2004; Hyatt et al., 2010; Seemann, 2014). Ribosomal binding site (RBS) prediction was carried out using RBSFinder (Suzek et al., 2001). TranstermHP was used to predict Rho-independent transcription terminators (TTS) (Kingsford et al., 2007). Ribosomal RNA and other functional RNAs such as riboswitches and non-coding RNA was annotated with Infernal (Nawrocki et al., 2009). Operons were predicted based on primary genome sequence information with Rockhopper v2.0.3 using default parameters (Tjaden, 2020). Insertion sequence prediction was done using ISEscan v.1.7.2.1 (Xie and Tang, 2017). Prophage prediction was done using PhiSpy v4.2.6 which combines similarity- and composition-based strategies (Akhter et al., 2012).
Genome-Based Strain Identification and Comparative Genomic Analyses
Taxonomic labeling of the assembled microbial genomes was carried out using CAMITAX (Bremges et al., 2020). CAMITAX is a scalable workflow that combines genome distance–, 16S ribosomal RNA gene–, and gene homology–based taxonomic assignments with phylogenetic placement. OrthoFinder v2.3.1 (Emms and Kelly, 2019) was used to determine orthologous relationships (Sayers et al., 2021).
Phylogenetic Analysis
Phylogenetic relationships of the genomes were explored with UBCG v3.0 using default settings (Na et al., 2018). This software tool employs a set of 92 single-copy core genes commonly present in all bacterial genomes. These genes then were aligned and concatenated within UBCG using default parameters. The estimation of robustness of the nodes is done through the gene support index (GSI), defined as the number of individual gene trees, out of the total genes used, that present the same node. A maximum-likelihood phylogenetic tree was inferred using FastTree v.2.1.10 with the GTR + CAT model (Price et al., 2010).
Patent Depository of Bacillus amyloliquefaciens ATCC PTA-126784 and PTA-126785, and B. subtilis ATCC PTA-126786
Bacillus amyloliquefaciens ATCC PTA-126784 and PTA-126785, and B. subtilis ATCC PTA-126786 strains were deposited in the ATCC culture collection (Manassas, VA, United States). For simplicity, Bacillus amyloliquefaciens ATCC PTA-126784 and PTA-126785, and B. subtilis ATCC PTA-126786 strains are referred to as Ba PTA84 and Ba PTA85, and Bs PTA86, respectively, throughout the manuscript.
Global Untargeted Metabolomic Analysis
Bacillus strains Bs PTA86, Ba PTA84, and Ba PTA85 were grown as three single strain cultures, and then were analyzed as a two-strain (Ba-PTA84 and PTA85) or three-strain (Bs-PTA86, Ba-PTA84, and Ba-PTA85) consortia in 5 mL of minimal or rich liquid media. For growth in minimal media, medium containing 1X M9 salts, and glucose at a final concentration of 0.5% (w/v) was used. Rich medium contained the following entities (g/L): peptone 30; sucrose 30; yeast extract 8; KH2PO4 4; MgSO4 1; and MnSO4 0.025. The culture was grown at 37°C overnight. Bacillus cells were pelleted by centrifugation at 10,000 × g for 10 min, cell pellets were washed three times with ice-cold PBS. The resulting cell pellets and cell-free supernatants were stored at −80°C and sent to metabolon Inc. (Durham, NC, United States) for global untargeted metabolomic profiling. The experiment was performed in one biological replicate. Detailed description of metabolomic analysis is presented in Supplementary Materials.
In vivo Assessment of Bacillus DFM for Improvement of Growth Performance in Broiler Chickens
Spore Generation
Bacillus spores were generated employing a modified protocol as described in Setlow (1990). Bacillus spp. was grown in a liquid Difco sporulation medium containing Nutrient Broth (BD Difco, Franklin Lakes, NJ, United States), 8.0 g/L; KCl, 1g/L, and MgSO4⋅7H2O, 0.12 g/L. The mixture was adjusted to pH 7.6 with additions of NaOH. After adjusting the pH and sterilizing the media by the use of an autoclave at 121°C, 1 mL of each of the following mineral sterile stock solutions were added to broth media, 1.0 M CaCl2, 0.01 M MnSO4, 1.0 mM FeSO4. A sterile glucose solution was also added to the medium mixture to a final concentration of 5.0 g/L. A single colony was taken from an agar plate and was inoculated into 100 mL of the sporulation medium. The culture was incubated overnight at 37°C with shaking at 200 rpm. This culture served as a seeding culture for 1 L of liquid culture. All growth were done employing vented baffled flasks. This culture was incubated at 37°C while shaking at 200 rpm for at least 72 h. The presence of spores was monitored with a brightfield microscope. The spores were harvested at 17,000 rpm and washed three times with pre-chilled sterile distilled water. The spores were then resuspended in 30 mL of pre-chilled sterile distilled water and the spore suspension was mixed with irradiated ground rice hulls (Rice Hull Specialty Products, Stuttgart, AR, United States), dried at 60°C for 3–4 h to eliminate vegetative cells. To determine spore inclusion in the rice hulls, 0.25 g of the material containing spores was heat treated at 90°C for 5 min. One milliliter of water was added to the material and allowed to soak for 15–30 min. The suspension was vortexed for 30 s and serially diluted 10-fold for colony counts on agar plates.
Study Design
A total of 2,500 1-day-old male broiler chicks (Cobb 500) were randomly allocated to two treatment groups on Study Day (SD) 0. The control group received only the basal diet, while the treated group received the basal diet plus 1.5 × 105 CFU of Ba PTA84 per gram of final feed. The control group consisted of 30 pens of 50 birds per pen, and the Ba PTA84 group consisted of 20 pens of 50 birds per pen.
Birds were housed in floor pens in a single environmentally controlled room with ad libitum access to treatment diets and water. Basal diets were formulated to be iso-nutritive, and to meet or exceed the nutrient requirements recommended for broilers (Supplementary Table 1). Feed was issued in four study phases: Starter Phase I (SD 0–12); Grower Phase II (SD 12–26); Finisher Phase III (SD 26–35), Withdrawal Phase IV (SD 35–42). Diets did not contain antibiotics, anticoccidials or growth promoters and were fed to the birds as a mash in all phases.
Bird weights (pen weight) were measured and recorded at SD 0, 12, 26, 35, and 42. Feed issued and weighed back were recorded for each feeding phase. Bird general health, mortality and environmental temperature were recorded daily.
Statistical Analysis
The experimental unit was the pen. All statistical analysis was conducted using the SAS system version 9.4 (SAS Institute, Cary, NC, United States) and all tests were performed comparing the control group to the treated group using a one-sided test at P < 0.05 level of significance.
Performance variables of interest for each feeding period and overall included: live final body weight (LFBW), average daily gain (ADG), average daily feed intake (ADFI), gain to feed efficiency (GF), feed to gain efficiency (FCR), mortality, and the European Broiler Index (EBI). These variables were calculated and evaluated for each study phase [Starter, Grower, Finisher, Withdrawal and Overall (SD 0–42)] and both adjusted for mortality and unadjusted.
Microbiome Profiling of Cecal Content From Birds Treated With Ba PTA84
DNA Extraction, Library Preparation and Sequencing
Total DNA from cecal content samples, collected from 20 animals from each Bacillus-fed and control group, were extracted employing the Lysis and Purity kit (Shoreline Biome, Farmington, CT, United States) following manufacturer’s protocol. The resulting DNA was used as template for library preparation using Shoreline Biome’s V4 16S DNA Purification and Library Prep Kit (Shoreline Biome, Farmington, CT, United States). Briefly, PCR amplification of the V4 region of the 16S rRNA gene was performed using the extracted DNA and the primers 515F (5′GTGGCCAGCMGCCGCGGTAA) and 806R (5′-GGACTACHVHHHTWTCTAAT). The resulting amplicons were then sequenced using 2 bp × 150 bp paired-end kits on the Illumina iSeq platform. To increase diversity, PhiX 50 pM was added to a final concentration of 5% into the amplicon library.
Bioinformatic Analysis
Forward and reverse reads were processed with cutadapt (v 2.5) (Martin, 2011) to remove primer sequences. Read pairs without primer sequences present or more than 15% primer mismatches were discarded. The DADA2 pipeline (v. 1.12.1) (Callahan et al., 2016) was used to generate a count matrix of amplicon sequence variants (ASVs) across samples. Due to the short length of iSeq reads, forward and reverse reads were trimmed to a length of 110 bp and merged with DADA2’s justConcatenate option. The DADA2 parameters parameters maxN = 0, truncQ = 2, rm.phix = TRUE and maxEE = 2 were used. Taxonomic labels were assigned to each ASV using the DADA2 assignTaxonomy method and the Silva v. 138 database (Yilmaz et al., 2014). Diversity and richness per sample were quantified from the ASV matrix using the Simpson, Shannon and Chao indices (Simpson, 1949; Chao, 1984; Shannon, 1997) and compared across treatments with the Mann–Whitney U test. Comparison of microbiome structures across treatments was performed using PERMANOVA and ANOSIM analysis based on the Bray–Curtis dissimilarity between samples. PERMANOVA and ANOSIM were performed using code in the scikit-bio python package1. Principal component analysis of the Bray–Curtis dissimilarity matrix was used to analyze sample clustering according to treatment group.
Results
Isolation and Identification Bacillus spp. From Healthy Animals
Bacillus spp. strains were isolated from the cecal contents and fecal materials of healthy chickens. The taxonomic identities of the isolates were determined by 16S-rRNA amplicon sequencing. These isolates belonged to 30 different Bacillus species with the top hits of B. velezensis, B. amyloliquefaciens, Bacillus haynesii, Bacillus pumilus, B. subtilis, and B. licheniformis, Supplementary Table 2.
Due to safety considerations, Bacillus spp. isolates chosen for further screening included only those that belong to the species listed as DFMs in the Association of American Feed Control Officials, Inc. (AAFCO) Official Publication since they “were reviewed by FDA Center for Veterinary Medicine and found to present no safety concerns when used in direct-fed microbial products” (AAFCO, 2018), and to the species listed as Qualified Presumption of Safety (QPS) status according to the European Food Safety Authority (EFSA) BIOHAZ Panel (Koutsoumanis et al., 2020). These were B. subtilis, B. amyloliquefaciens, B. pumilus, and B. licheniformis.
In vitro Screening for Probiotic Properties of Bacillus spp. Strains
Bacillus spp. strains were tested to determine their effect on selected microorganisms and their ability to secrete selected enzymes (Latorre et al., 2016). For the former, Gram-negative and Gram-positive microorganisms (E. coli O2, O18, O78, C. perfringens NAH 1314-JP1011, and S. enterica serovar Typhimurium ATCC 14028) were used. For the latter, plate-based assays for determining the secretion of amylase, protease, and β-mannanase were performed (Figure 1).
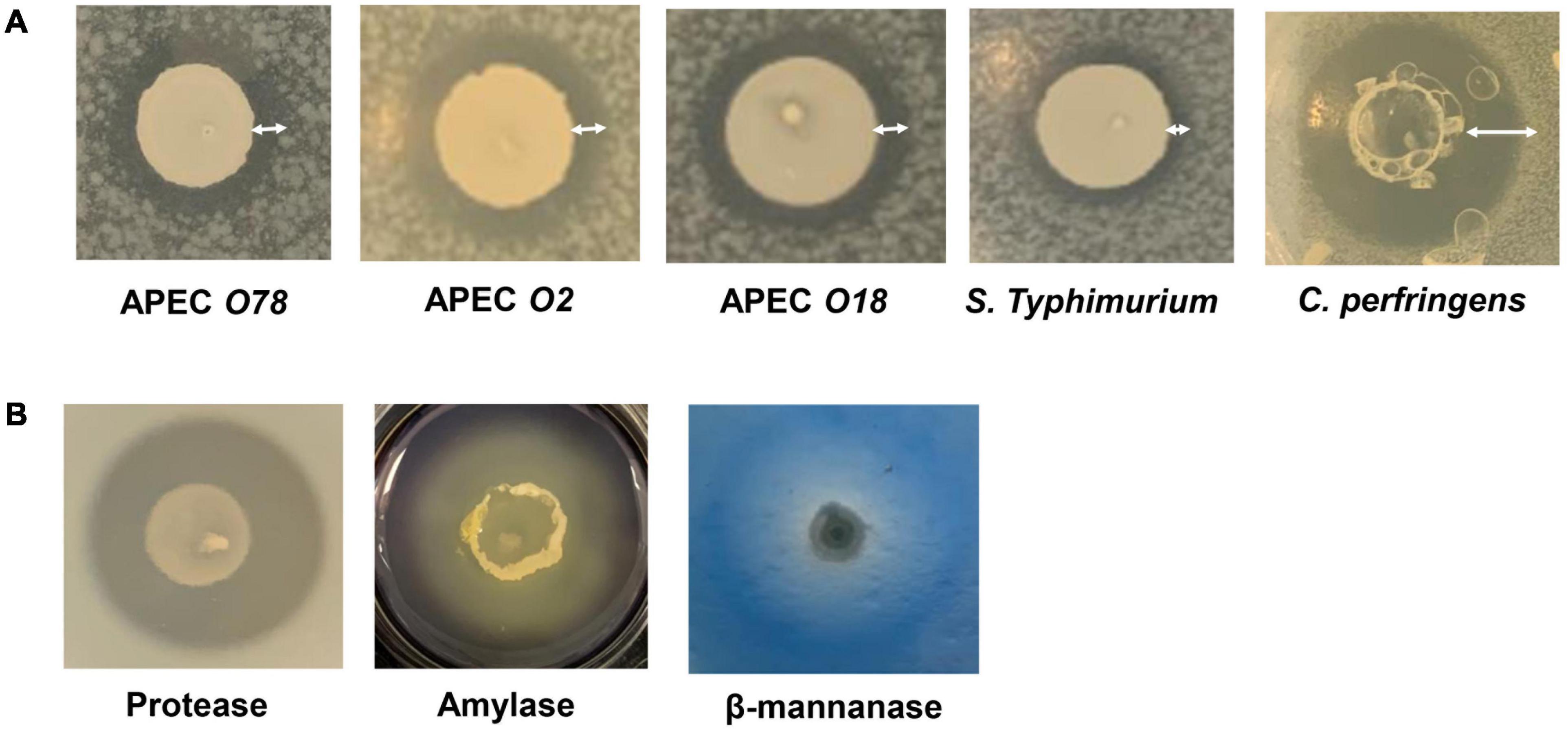
Figure 1. Screening of Bacillus spp. DFM candidates for pathogen growth inhibition and enzyme activities. (A) Microorganism growth inhibition. Representative examples of microorganism overlay assays were presented with the following microorganisms, Avian Pathogenic Escherichia coli serotype O78, O2, and O18, Salmonella enterica serovar Typhimurium ATCC 14028, and Clostridium perfringens NAH 1314-JP1011. Overlay microorganism inhibition assays were performed in duplicate for each of the Bacillus spp. strains. A zone of inhibition was measured from the edge of Bacillus spp. until the end of clearance zone as shown as white double-edge arrows. (B) Screening of digestive enzyme activities of Bacillus spp. strains. The assay was performed in duplicate for each Bacillus spp.
A total of 266 Bacillus strains were first screened against E. coli O2, and 71% of the strains showing positive E. coli O2 inhibition were selected for a second-round of assays targeting E. coli O18, then E. coli O78, S. Typhimurium and lastly C. perfringens JP1011. The top 8 Bacillus strain candidates were selected according to their cumulative inhibition scores, Supplementary Table 3. These included 6 B. amyloliquefaciens (Ba): Ba ELA006, Ba ELA071, Ba ELA151, Ba ELA175, Ba PTA84, Ba PTA85, and 2 B. subtilis (Bs) isolates; Bs ELA082 and Bs PTA86.
The cumulative inhibition score was calculated as the sum of the inhibition score values of a Bacillus strain against the five microorganisms tested. The data showed that six Ba strains had better cumulative microbial inhibition scores compared to two Bs strains. The average cumulative inhibition scores of 8.5 and 5.5 for six of Ba and two of Bs, respectively.
The eight Bacillus strain candidates were also evaluated for their ability to secrete enzymes. Bacillus strains are known to produce a variety of enzymes (Contesini et al., 2018; Danilova and Sharipova, 2020). In vitro plate-based assays for protease, amylase, and β-mannanase activities showed that six Ba and two Bs showed comparable amylase, protease, and β-mannanase activities, Supplementary Table 3 Ba ELA071 and Ba PTA85 showed the lowest and highest cumulative REA values of 4.73 and of 6.1, respectively.
Safety Assessment of Bacillus spp. Probiotic Candidates
To evaluate the safety of Bacillus spp. as microbial feed ingredients, the Bacillus candidates were tested for antimicrobial susceptibility to medically relevant antimicrobials.
The results from antimicrobial susceptibility tests for 8 Bacillus strains showed that all strains were susceptible to the tested antibiotics. The only exception was Bs ELA082 which exhibited a borderline resistance to streptomycin. The MIC of streptomycin for this strain was 16 μg/mL, which is twofold higher than the EFSA threshold cut-off of streptomycin for Bacillus as DFM, Figure 2A.
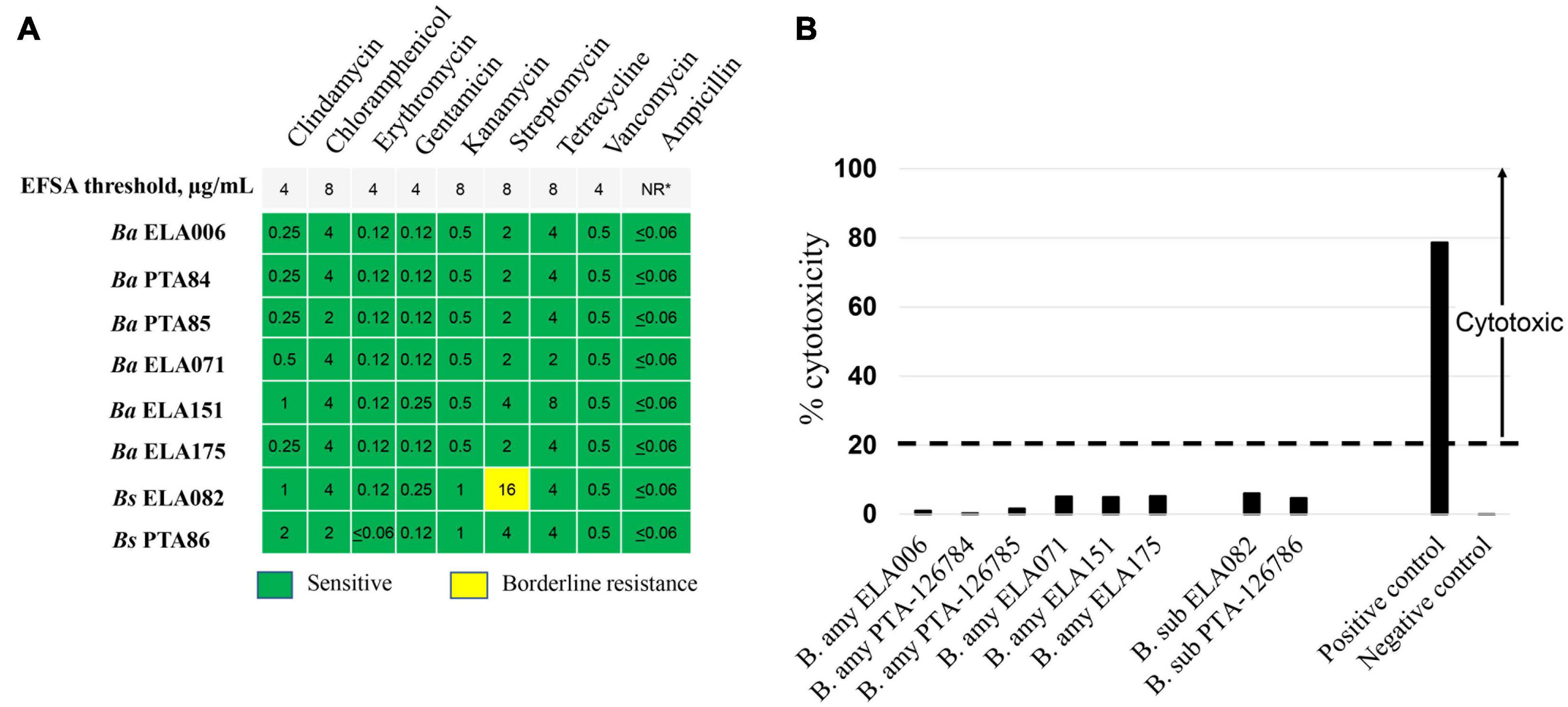
Figure 2. A safety assessment of Bacillus spp. DFM candidates. (A) Antimicrobial susceptibility tests of Bacillus spp. MIC (μg/mL) values for each antibiotic tested of respective Bacillus spp. were shown. Nine medically important antibiotics at a concentration range of 0.06–32 μg/mL were tested and the respective antimicrobial susceptibility cut-off concentrations required for Bacillus spp. were shown at the top panel. (B) Cytotoxicity assessment of Bacillus spp. culture supernatant toward Vero cells. Culture supernatant of B. cereus ATCC 14579 and B. licheniformis ATCC14580 were used as positive and negative controls, respectively. Cytotoxicity level above 20%, dashed line, is considered cytotoxic. *, NR, not required by EFSA guidelines.
To determine the potential toxicity of Bacillus strains on the host cells, culture supernatants of Bacillus spp. were tested for cytotoxicity toward Vero cells according to EFSA Panel on Additives and Products or Substances used in Animal Feed [FEEDAP] et al. (2018). The cytotoxicity assay was performed by monitoring the lactate dehydrogenase (LDH) enzyme originated from compromised Vero cells as described in Fagerlund et al. (2008). Figure 2B shows the cytotoxicity levels of each Bacillus strain tested. The results suggested that all 8 Bacillus strains were non-cytotoxic with toxicity levels far below 20%, a percentage that is considered cytotoxic according to the EFSA guidelines. Of all isolates, the cytotoxicity level of Ba PTA84 was closest to that of the negative control. In general, Ba strains exhibited toxicity levels that were lower than those of Bs strains. Within the B. subtilis group, the cytotoxicity level of Bs PTA86 was the lowest, 5%.
Selection of Bacillus spp. as Direct Fed Microbial Candidates
Based on their performance on microorganism inhibition, enzymatic activities, antimicrobial susceptibility, and low toxicity against Vero cells, the strains Ba PTA84, Ba PTA85, and Bs PTA86 were chosen for more detailed characterization employing genomic and metabolomic approaches described in the following sections.
Untargeted Global Metabolomic Analysis of Cell Pellets and Culture Supernatants of Ba PTA84, Ba PTA85, and Bs PTA86 as Single Strains and as Consortia
Untargeted metabolomics analysis of cell pellets and culture supernatants of the three candidate strains was performed to assess metabolite profiles of each strain and strains-in consortia when grown in two different media. Cells were cultured in both rich and minimal media as individual strains, as well as a consortium of Ba PTA84 and Ba PTA85, and a consortium including all three strains. In total, 436 and 457 named metabolites were identified in the supernatant and pellet samples, respectively (Supplementary Tables 4–7).
A principal component analysis (PCA) of the normalized metabolite abundances showed a clear separation between samples from rich and minimal media along the first principal component (∼70% explained variance), both in the supernatant and pellet (Figures 3A,B). Additionally, the separation between samples along the two first principal components suggested that under the tested growth conditions the strains and consortia differ in their profiles of secreted/consumed metabolites as well as their cell-pellet small-molecule content.
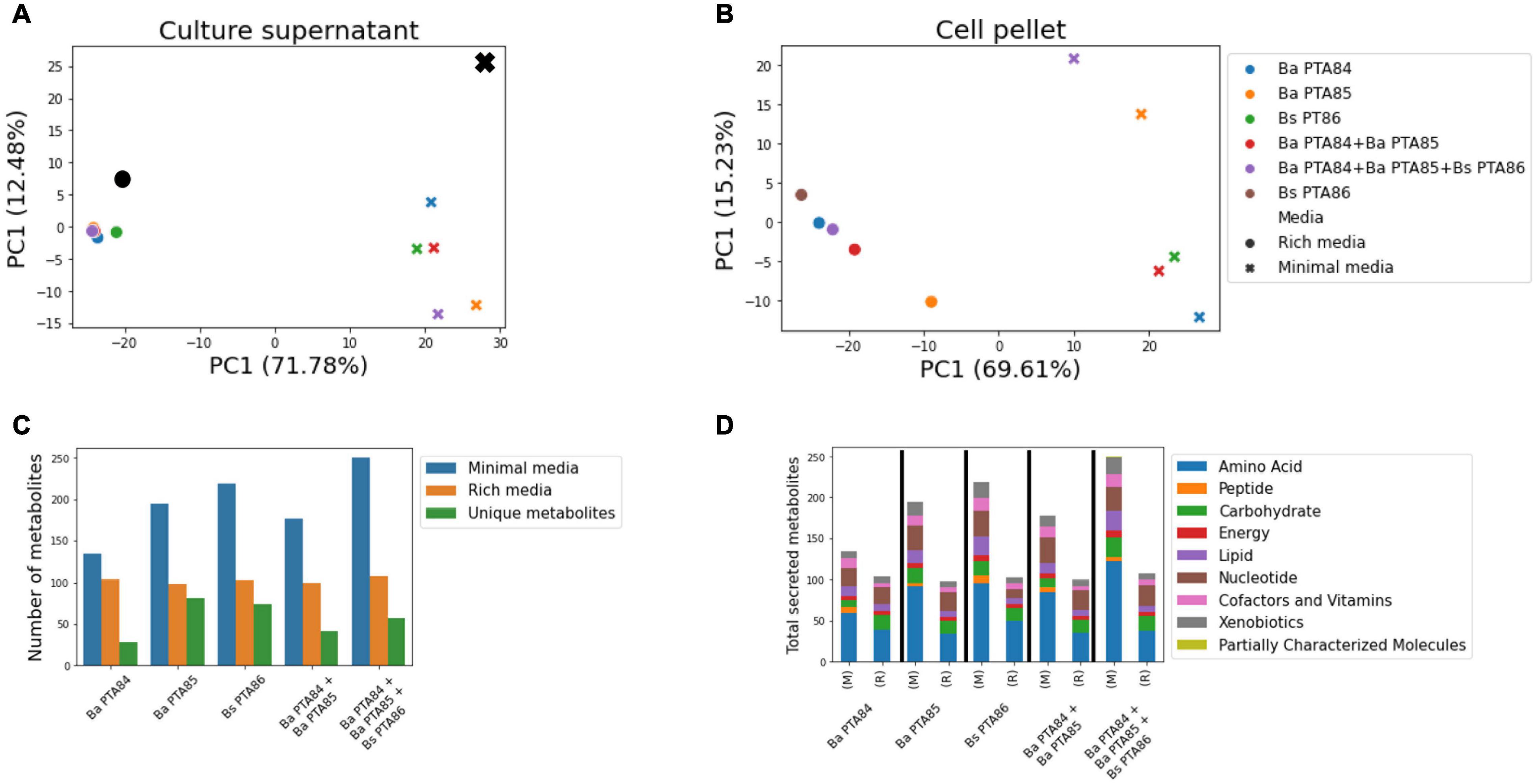
Figure 3. Global untargeted metabolomic analysis of Ba PTA84, Ba PTA85, and Bs PTA86. (A) Principal component analysis of scaled metabolite intensities for culture supernatants in two different media. Black symbols represent media controls; numbers in parentheses indicate the variance explained by the first two principal components. (B) Like (A), but for the culture cell pellets. (C) Number of identified secreted metabolites in culture supernatants in two different media (M) and (R), minimal and rich media, respectively. Secreted metabolites were defined as having a scaled intensity at least 1.5-fold higher than observed in media controls. Unique metabolites represent secreted compounds with abundances at least 1.5-fold higher than observed for other single strains (in the case of individual strain cultures) or corresponding individual strains (in the case of co-cultures). (D) Pathway representation of metabolites secreted by strains or strain combinations under minimal and rich media culture conditions.
Interestingly, looking at the metabolite profiles in the culture supernatant (Figure 3A), all strains and strain combinations tightly clustered in the rich media samples while they separated under minimal media conditions. Indeed, looking at the number of secreted metabolites under each condition (abundance > 1.5-fold above media controls, Figure 3C), cells in all cultures secreted approximately 100 named metabolites in rich media with a median Jaccard similarity of 0.8, compared to a range of 134–250 secreted metabolites in minimal media with only 0.54 median overlap (Mann–Whitney p-value = 0.01). This shows that especially under minimal media conditions, each strain or consortia secretes distinct sets of small molecules.
In rich media, Ba PTA84 secreted the largest number of metabolites (104 metabolites) and it had the fewest uniquely secreted metabolites (abundance > 1.5-fold higher than other individual strains) across both media. Thirteen unique metabolites related to amino acid, central carbon, nucleotide, and lipid metabolisms as well as some xenobiotic compounds (i.e., quinate, 4-hydroxybenzyl alcohol, and 3-dehydroshikimate) were detected.
As expected, the three-strain consortium was found to have the largest number of secreted metabolites in minimal media, a total of 250 metabolites. These include potential host beneficial metabolites such as betaine, kynurenine, indolactate, tyrosol, citrulline, tricarballylate, vitamins B5 and B6, hippurate, and kestose. Of all three single strains, the culture supernatant of Bs PTA86 carried the highest number of metabolites in minimal media (219 metabolites), followed by Ba PTA85 and Ba PTA84 with 195 and 130 metabolites, respectively. Ba PTA85 had the greatest number of unique secreted metabolites, a total 78 metabolites. The larger number of secreted metabolites under minimal media conditions was partly due to a higher number of amino acid metabolism intermediates, followed by nucleotide and carbohydrate metabolites (Figure 3D). Thus, our results suggest that the different strains may use different metabolic strategies to synthesize amino acids/proteins, nucleotide, and carbohydrate molecules given a limited nutrient availability.
Genome Properties of Ba PTA84 and PTA85, and Bs PTA86
The genomes of Ba PTA84, Ba PTA85, and Bs PTA86 were sequenced by PacBio sequencing. Assembly of Ba PTA84 and Bs PTA86 genomes yielded 1 contig each while the Ba PTA85 assembly contained 2 contigs – a large 4,084,681 bp contig and a smaller 231,132 bp long contig. The genome properties and annotation of different features are summarized in Table 1. The whole-genome sequences were deposited at DDBJ/ENA/GenBank under BioProject numbers PRJNA701126 and PRJNA701127.
Core-Genomes of Ba PTA84, Ba PTA85, and Bs PTA86
Ortholog analysis was performed to identify paralogous and/or orthologous relationships between the genomes of Ba PTA84, Ba PTA85, and Bs PTA86. Ba PTA84 shared the highest number of genes with 99.4% gene presentation in orthogroups while Ba PTA85, and Bs PTA86 shared 93.6% and 90.1%, respectively (Supplementary Table 8). A total of 3,024 orthologs were shared among all three strains while 586 orthologs were shared only between Ba PTA84 and Ba PTA85, 60 orthologs between Ba PTA84 and Bs PTA86, and 34 orthologs were shared only between Ba PTA85, and Bs PTA86 (Figures 4A,B).
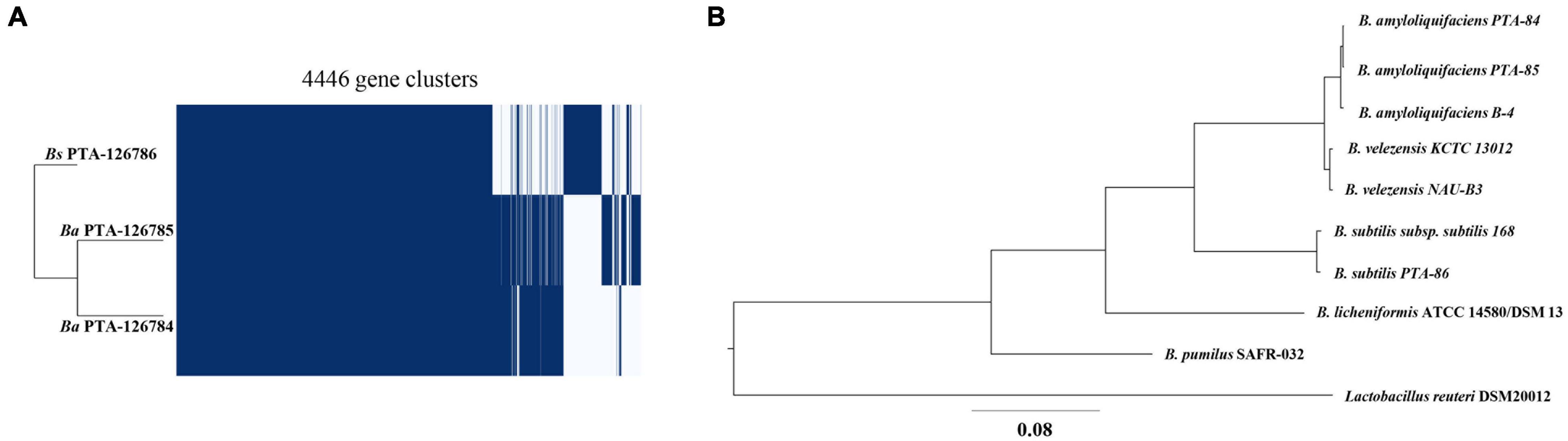
Figure 4. Phylogenetic analysis of poultry origin Bacillus spp. (A) Core genome analysis of Ba PTA84 and PTA85, and Bs PTA86. (B) Phylogenetic relationship of Ba PTA84 and PTA85, and Bs PTA86 to other Bacillus species along with Lactobacillus reuteri as an outgroup.
Phylogenetic Analysis of Ba PTA84, Ba PTA85, and Bs PTA86
Phylogenetic relationships of the three genomes were explored with UBCG v3.0 which employs a set of 92 single-copy core genes commonly present in all bacterial genomes. Ba PTA84, Ba PTA85 and Bs PTA86 genomes were compared against the genomes of B. amyloliquefaciens, B. velezensis, and B. subtilis strains along with Lactobacillus reuteri as an outgroup (Accession numbers: AL009126, CP000560, CP002627, CP002634, CP002927, HE617159, HG514499, JMEF01000001, CP005997, CP009748, CP009749, CP011115, LHCC01000001, CP014471, and QVMX01000001). As shown in Figure 4, both strains Ba PTA84 and Ba PTA85 showed closest relationship to B. amyloliquefaciens B4 while Bs PTA86 showed closest relationship to B. subtilis subsp. subtilis 168.
Genome Analyses of Ba PTA84, Ba PTA85, and Bs PTA86
The assembled genome sequences of 3 Bacillus strains were annotated for the following potential probiotic properties, enzymes, antioxidants, bacteriocins, and secondary metabolites, and for the presence of genes of potential safety concerns such as genes encoding toxins, virulence factors, and antimicrobial resistance genes. A detailed description of each of the above-mentioned features is described below.
Selected Enzymes Analyses
Figure 5 illustrates the presence and absence of genes encoding selected digestive enzymes identified in the Bacillus genomes. All three Bacillus genomes encode lipase, 3-phytase, alpha-amylase, endo-1,4-β-xylanase A, β-glucanase, β-glucanase, β-mannanase, pectin lyase, and alpha galactosidase. Bs PTA86 carried two copies of β-mannanase genes, Supplementary Table 9. β-mannanase catalyzes the hydrolysis of β-1,4-linkage of glucomannan releasing mannan oligosaccharide (McCleary, 1978; Saeed et al., 2019). This enzyme along with phytase, xylanase, amylase are added as feed ingredients to improve feed digestibility (Fayek et al., 1995; Sherif, 2009; Zhou et al., 2009). Of the three Bacillus genomes, only Bs PTA86 possessed pullulanase, oligo-1,6-glucosidase, and glycogen degrading enzymes such as 1,4-alpha-glucan branching enzyme. A complete list of enzymes in the three Bacillus genomes were presented in Supplementary Table 9.
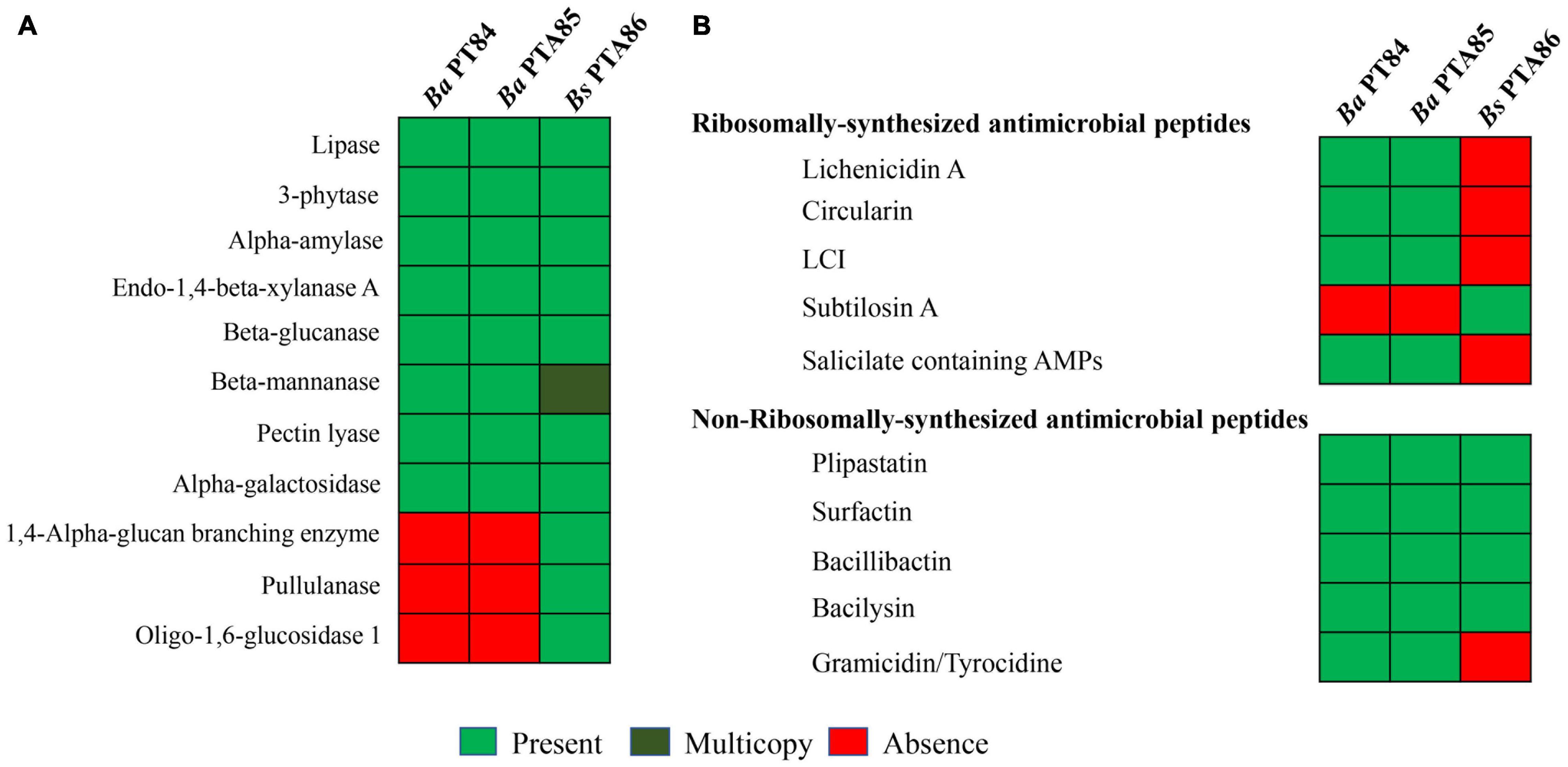
Figure 5. In silico analysis of genes encoding enzymes and antimicrobial peptides in Bacillus spp. genomes. Genes encoding enzyme (A) and antimicrobial peptides (B) were compared among the genomes of three Bacillus spp., Ba PTA84 and PTA85, and Bs PTA86.
Secondary Metabolites
Secondary metabolite clusters accounted for 20, 20, and 12% of the genomes of Bacillus Ba PTA84, Ba PTA85, and Bs PTA86, respectively. Table 2 illustrates the respective clusters for each Bacillus genome. Ba PTA84 and Ba PTA85 genomes contained 13 secondary metabolite clusters whereas Bs PTA86 genome encoded for 10 clusters. More than half of the clusters were contributed by biosynthetic genes for antimicrobial peptides (AMPs) (Figure 5B).
Genes of Safety Concern
To search for genes encoding known virulence factors, toxins, and antimicrobial resistance (AMR), we applied a screening approach using cutoff values according to an EFSA guideline (EFSA, 2021), sequence identity and coverage values higher than 80 and 70%, respectively. According to the analysis, genes for known virulence factors or toxins were not identified in the genomes of three Bacillus strains, Ba PTA84, Ba PTA85, and Bs PTA86. Supplementary Table 10 presents genes for putative genes encoding for antimicrobial resistance (AMR). Ba PTA84 and 85 had a similar set of putative AMR genes identified, namely putative genes encoding methyl transferase (cfr/cfr-like, clbA) (EFSA Panel on Additives and Products or Substances used in Animal Feed [FEEDAP] et al., 2018), tetracyclin efflux protein [tet(L)] (World, 2021), Streptothricin-N-acetyltransferase (satA), and rifamycin-inactivating phosphotransferase (rphC) (Laslett and Canback, 2004; Hyatt et al., 2010). Bs PTA86 genome carried putative genes that encoded macrolide 2′phosphotransferase (mphK), ABC-F type ribosomal protection protein (vmlR), Streptothricin-N-acetyltransferase (satA), tetracyclin efflux protein [tet(L)], aminoglycoside 6-adenylyltransferase (aadK) (Seemann, 2014), and rifamycin-inactivating phosphotransferase (rphC). The aadK gene from B. subtilis was originally found in susceptible derivatives of Marburg 168 strains. Heterologous expression of the gene in a plasmid in E. coli resulted in resistance phenotype toward rifamycin suggesting the need for high gene copies to confer resistance (Suzek et al., 2001).
Antioxidants, Adhesion, and Folate Biosynthesis
Genes encoding primary redox enzymes such as superoxide dismutase and catalase that scavenge reactive oxygen species were found in the three Bacillus genomes, Supplementary Table 11. A thioredoxin system and genes for bacillithiol biosynthesis were also identified. All three genomes encoded for a thioredoxin reductase (locus tag for Ba PTA84, Ba PTA85, and Bs PTA86: JS608_03853, JTE87_00428, and JS609_03503, respectively) and two cognate thioredoxins for Ba PTA84 and Ba PTA85 (locus tags, JS608_02520 and JTE87_01059; JS609_02844 and JS608_03225) and a Trx for Bs PTA86 (locus tag, JTE87_01767). Thioredoxin systems maintain cellular redox homeostasis (Meyer et al., 2009). Interestingly, despite lacking glutathione-glutaredoxin system, several genes for glutathione transport were found suggesting the potential transport of redox proteins, possibly bacillithiol, to the extracellular environment maintaining redox potential of the surroundings. Two genes for bacillithiol biosynthesis (Gaballa et al., 2010), bshA and B, were identified in genomes of Ba PTA84 and PTA85, and Bs PTA86, Supplementary Table 11.
Two genes each encoding for elongation factor Tu and 60 kDa chaperonin involved in adhesion of Bacillus species to intestinal epithelium were identified in all three genomes.
Probiotic bacteria confer several health benefits to the host, including vitamin production. We searched for key components of folate production pathways in Bacillus strains using the Enzyme Commission (EC) numbers associated with folate biosynthetic pathway. The analysis of genome sequences of Bacillus strains identified genes involved para-aminobenzoic acid (PABA) synthesis in all three strains (Table 3). However, strain Ba PTA84 has a frameshift mutation in pabB gene. The enzymes necessary for chorismate conversion into PABA are present in all three Bacillus probiotic strains. Bacillus probiotic strains also contain the genes of DHPPP de novo biosynthetic pathway. Previous studies have shown that B. subtilis genome harbor all the pathways components and have been engineered for folate production (Salem et al., 1972; Zhu et al., 2005; de Crecy-Lagard, 2014).
Screening for Prophages, Insertion Sequences and Transposases
All three strains were scanned for the presence of mobile genetic elements such as prophages, insertion sequences (IS) and transposases. Both Ba PTA84 and Ba PTA85 have 3 transposases while Bs PTA86 has 4 transposases. Ba PTA84, Ba PTA85 and Bs PTA86 share 2 copies of IS21 insertion sequence.
Effects of In-Feed Administration of Ba PTA84 on Growth Performance of Broiler Chickens
As a proof of principle, Ba PTA84 was selected in an in vivo pilot study as a DFM to evaluate its probiotic efficacy in supporting improved broiler growth performance. To accomplish this, 2,500 1-day old broiler chickens (Cobb 500) were randomly assigned to 50 pens of 50 birds each and split between two treatment groups; an untreated Control group and a group receiving 1.5 × 105 CFU of Ba PTA84 per gram of feed for the full 42-day production period. Despite similar feed intake, birds fed the DFM had 3.5% higher final body weight compared to the control (2.16 vs. 2.23 kg for Control and DFM, respectively; p = 0.0018). This translated to a 3.3% improvement in feed conversion ratio (FCR) for the DFM-fed group compared to Control (1.50 vs. 1.45 for Control and DFM, respectively; p = 0.011) and a 6.2% increase in the European Broiler Index (EBI), a metric of overall production efficiency (337 vs. 358 for Control and DFM, respectively; p < 0.0001), Figure 6. These results indicated that use of Ba PTA84 as a DFM can significantly improve weight gain and feed efficiency in broiler chickens.
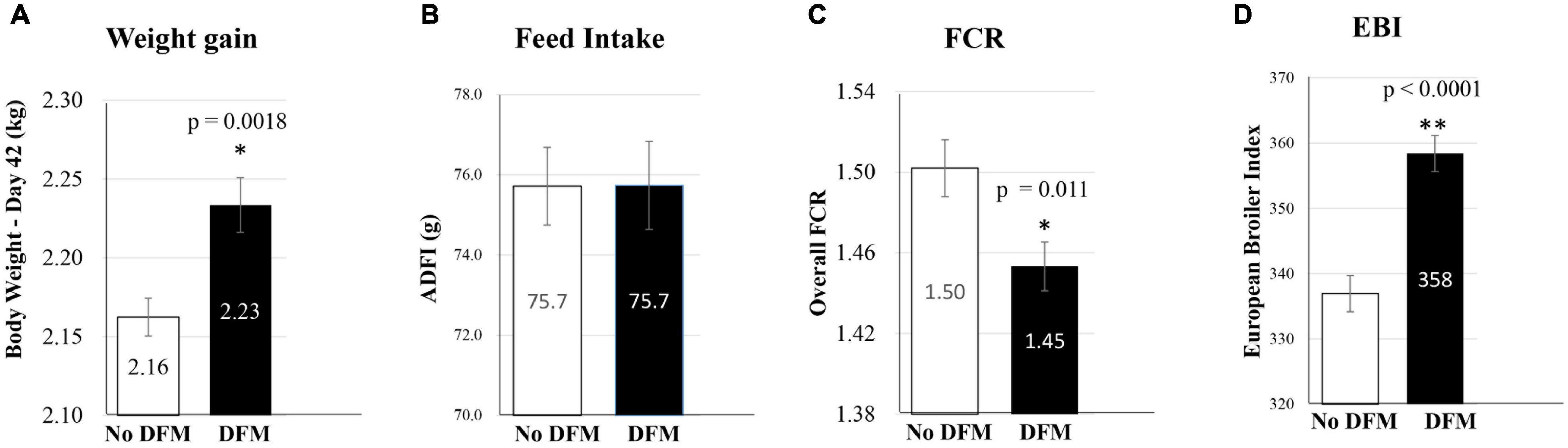
Figure 6. Effect of in-feed administration of Ba PTA-84 on growth performance of broiler chicken. Growth performance as measured by four parameters, (A) weight gain, (B) feed intake, (C) Feed Conversion Ratio (FCR), and (D) European Broiler Index (EBI).
Cecal Microbiome Structure Analysis From Chicken With and Without In-Feed Administration of Ba PTA84
To gather insights into the in vivo effects of in-feed administration of B. amyloliquefaciens on broiler chickens, we analyzed the cecal microbiomes of animals in the control and treatment groups of our clinical study. Samples from twenty 42-day old animals from each treatment group were used to build 16S rRNA amplicon libraries for sequencing, corresponding to one randomly chosen animal per pen. The median coverage was ∼36,000 read pairs per sample, and 1,945 ASVs were identified across samples.
Samples in the control and treatment groups showed similar values for ASV richness and diversity (Figures 7A,B, p-values = 0.07, 0.44–0.36, respectively). Additionally, ANOSIM and PERMANOVA analyses based on the Bray–Curtis dissimilarity between samples did not support significant differences in community structures between treatment groups (ANOSIM p-value = 0.66. PERMANOVA p-value = 0.44). In addition, using ANCOM, we did not observe differentially abundant ASVs between treatment groups, including those classified to the genera of pathogens used for in vitro screening (Mandal et al., 2015). These results, which are evident by the lack of clustering of samples by treatment following principal components analysis (Figure 7C), indicate that supplementation of Ba PTA84 at a dose sufficient to induce a positive effect on performance (Figure 7) had minor effects on the diversity and structure of the cecal microbiome.
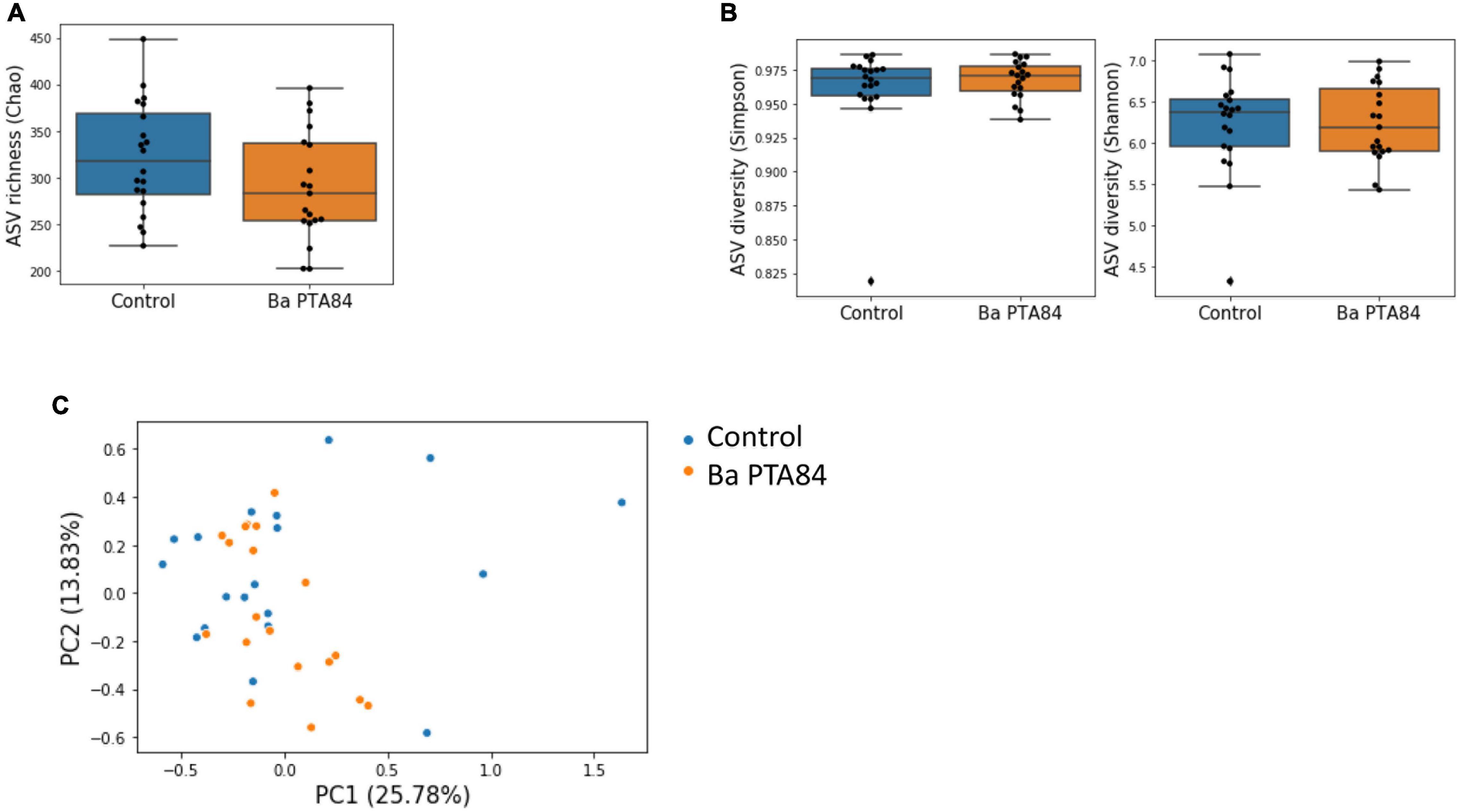
Figure 7. Microbiome analysis of cecal content from birds fed with or without Ba PTA84. (A) ASV richness in the cecum of control birds and birds treated with Ba PTA84. Richness is quantified using the Chao index (Mann–Whitney U p-value = 0.07). (B) ASV diversity quantified with the Simpson (left) and Shannon indexes (right) for control birds and birds treated with Ba PTA84. (p-value = 0.44, 0.36). (C) Principal component analysis of the Bray–Curtis dissimilarity matrix between microbiome profiles for control birds and birds fed with Ba PTA84. Each dot represents a cecal sample. Numbers in parentheses indicate the variance explained by each principal component.
Discussion
A clear understanding of the physiology and safety of probiotic strains as well as their interactions with target host, and hosts’ gut microbiota are essential to rationally develop the next generation of probiotics with improved safety and efficacy, and increased reproducibility. Here, we employed comprehensive multi-omics, biochemical, and microbiological approaches for the selection and characterization of Bacillus spp. strains to improve growth performance in poultry. Our data showed that the selected strain Ba PTA84 significantly improved growth performance indicating that the screening workflow helped to rationally design promising DFM candidates. Moreover, we generated copious data that we expect will guide future efforts to decipher the gene clusters, metabolites, phenotypic traits, and microbiome impact of spore-formers as important characteristics of probiotic strains.
Figure 8 illustrates the screening and characterization workflow. This bottom-up approach ensures selection of the best candidates at each screening step. Strains that did not meet safety criteria were not selected. Only the best candidates that met phenotypic selection criteria moved forward to the next screening step. Genomic analysis of the top three Bacillus strains helped to create a link between phenotypic observations with genomic traits. Furthermore, genomic and metabolomic analyses of three candidate strains pointed to potential outcome differences when combining these three candidate strains in a consortium. Details from our findings are described below.
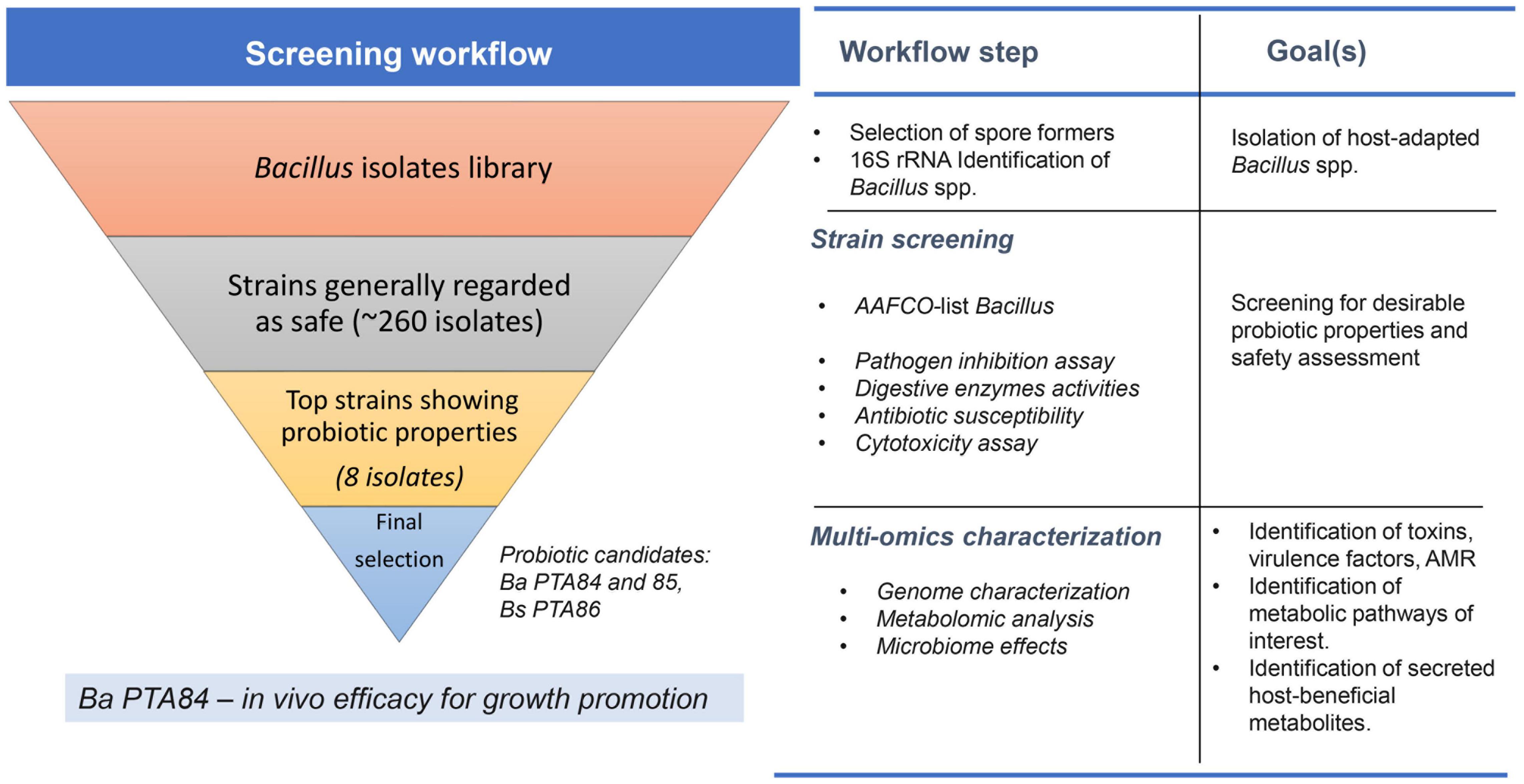
Figure 8. A screening workflow diagram for selection of Bacillus spp. probiotic candidate. Bacillus spp. isolates were screened for their activities to inhibit poultry pathogens and ability to secrete digestive enzymes in vitro. The best candidates were further selected based on their safety profiles (i.e., antimicrobial resistance profile and cytotoxicity level). Genomic and metabolomic analyses were performed on the select isolates to further investigate potential host-benefit properties and possible health/safety concerns. The top candidate was then tested for its effects on growth promotion in vivo.
Host-Adapted Bacillus Strains
We expected that host-adapted Bacillus strains to exert better probiotic effects in the host environment than those isolated from other sources, thus, we targeted our isolation to those Bacillus spp. from animal GIT content or fecal samples of healthy animals (Barbosa et al., 2005). A higher diversity of isolates was obtained from the ethanol-treated samples compared to heat-treated samples, as reported previously (Koransky et al., 1978; Barbosa et al., 2005). Despite the general heat resistance feature of Bacillus spores, spore core, cortex, coat, and membrane composition determines the degree of the spores’ heat resistance (Setlow, 2006; Brul et al., 2011; Berendsen et al., 2015) resulting in different responses of spores toward heat stresses.
Desirable Probiotic Properties
With the continuing reduction in use of antibiotics in poultry farms, as driven by regulations, and some customer preferences, the development of microbial feed additives that support maintenance of poultry health in the face of undesirable organisms would be beneficial. Our screening results showed that Bacillus spp. controlled the growth of undesirable E. coli O2, O18, and O78, C. perfringens- and Salmonella Typhimurium. APEC strains cause colibacillosis, which is a major problem in commercial production (Dziva and Stevens, 2008; Mellata, 2013). Colibacillosis occurs when APEC originating from fecal materials translocate into the lung epithelium during fecal aerosolization. Thus, reducing the APEC load in feces as a potential effect of Bacillus spp. in the feed could help reduce the incidence of colibacillosis (Duan et al., 2008; Chien et al., 2011). C. perfringens is a pathogen that causes necrotic enteritis in poultry (Cooper and Songer, 2009) by the production of alpha toxin and NetB (Keyburn et al., 2006, 2008). Necrotic enteritis is a multi-factorial disease that cost poultry farmers 6 billion dollar annually (Wade and Keyburn, 2015). Salmonella Typhimurium, a poultry gut commensal, is the major cause of salmonellosis in humans. This infection is facilitated by the consumption of Salmonella-containing poultry products (Foley and Lynne, 2008; Batz et al., 2012). The ability of Bacillus spp. to suppress the growth of these undesirable organisms might be due to the production of AMPs (bacteriocins). Genome analysis of Ba PTA84, Ba PTA85, and Bs PTA86 suggested that Ba and Bs genomes encoded distinct AMPs (Figure 5B). Ba PTA84 and Ba PTA85 genomes encoded for ribosomally-synthesized lichenicidin A, circularin, LCI, and salicylate containing AMPs that were not found in Bs PTA86 genome. The latter possessed subtilosin A, a cyclic antimicrobial peptide that are potent against some Gram-positive and Gram-negative bacteria such as Listeria monocytogenes, Enterococcus faecalis, Porphyromonas gingivalis, Klebsiella rhizophila, Streptococcus pyogenes and Shigella sonnei, Pseudomonas aeruginosa and Staphylococcus aureus (Babasaki et al., 1985; Stein et al., 2004; Shelburne et al., 2007). For non-ribosomally synthesized AMPs, Ba PTA84 and Ba PTA85 carried plipastatin, surfactin, bacillibactin, bacilysin, and gramicidin. Only the latter was absent in Bs PTA 86.
Bacillus species are known to secrete host beneficial enzymes such as cellulase, xylanase, amylase, protease, β-mannanase, phytase (Latorre et al., 2016; Singh and Bajaj, 2016; Danilova and Sharipova, 2020). These enzymes, when fed to animals, improve digestion of low-calorie diets or reduce intestinal inflammation by breaking down non-starch polysaccharides (NSPs). Some NSPs are anti-nutritional factors, and increase the gut content viscosity, slow down feed retention time in the gut, and thus reduce nutrient absorption (Raza et al., 2019). An accumulation of undigested NSPs can lead to the growth of pathogens that cause subclinical infection challenges (Kaldhusdal, 2000; Van Immerseel et al., 2004). Production of pro-inflammatory cytokines as a response to NSPs demands a significant amount of energy, which otherwise could be preserved for growth, lowering food efficiency and growth performance [reviewed in Cardoso Dal Pont et al. (2020)]. Ba and Bs showed comparable protease, amylase, and β-mannanase activities. These activities were supported by our genomic analysis showing that Ba and Bs possess genes encoding for amylase, protease, β-mannanase, and phytase. Bs PTA86 genome contained more genes for enzymes compared to those of Ba PTA84 and PTA 85.
It is noteworthy that genome analyses revealed other potential benefits of top three Bacillus candidates for animals. Genes encoding a wide array of antioxidant proteins were identified, superoxide dismutase, catalase, thioredoxin, and methionine sulfoxide, and bacillithiol. These proteins when expressed and secreted in the GIT could provide protection toward oxidative stress (Abudabos et al., 2016; Wang et al., 2017; Inatomi and Otomaru, 2018). Oxidative stress occurs in the GIT when the level of free radicals generated by reactive oxygen/nitrogen species (RO/NS) is much higher than the level of antioxidant proteins for neutralization of these toxic compounds (Sherif, 2009). This event is triggered by various factors including nutritional or environmental heat stress, or pathological factors which ultimately decrease growth performance and quality of meat and eggs (Sherif, 2009). Another key desirable traits in a probiotic candidate is the ability to adhere to epithelial cells. The two genes identified in all three strains encode proteins potentially involved in adhesion to mucus, epithelial cells and are known to be involved in host immunomodulation and unwanted microorganism aggregation, providing stability to the strains and the ability to compete with other undesirable resident gut bacteria, thereby enabling effective colonization of the gut and exclusion of pathogens (Antelmann et al., 2000; Sánchez et al., 2008).
Among proposed functions of probiotic bacteria are the reduction of potential pathogenic bacteria, immune modulation, removal of harmful metabolites in the intestine and/or providing bioactive or otherwise regulatory metabolites. Folate-producing probiotic bacteria enable better nutrient digestion and energy recovery. Folate-producing probiotic strains could potentially confer protection against cancer, inflammation, stress, and digestive disturbances (White et al., 1997; Fuchs et al., 2002; Zhu et al., 2005; Rossi et al., 2011; Zhang et al., 2020). Several studies exploring the commercial utility of probiotic strains for folate production have been reported (Sauer et al., 1998; Schallmey et al., 2004; Rossi et al., 2011). Genes encoding essential enzymes in the biosynthetic pathways of folate were also found in the genome of three Bacillus strains. The products of these pathways supply important cofactors which once secreted would be absorbed by the host improving health status (Sauer et al., 1998; Schallmey et al., 2004; Rossi et al., 2011).
Safety Profiles
In addition, Bacillus DFM candidates must have acceptable safety profiles as expected by regulatory authorities. Some Bacillus spp. are known to produce AMPs and enterotoxins that might exert deleterious effects on the host cells (EFSA Panel on Additives and Products or Substances used in Animal Feed [FEEDAP] et al., 2018). Lack of detailed characterizations of probiotic strains resulted in the use of B. cereus (Bactisubtil, Biosubtyl, and Subtyl) probiotic strains harboring structural genes of known enterotoxins (Duc et al., 2004). Cytotoxicity assessment of our Bacillus spp. strains suggested that Bacillus spp. did not cause cytotoxicity of Vero cells. Moreover, genome analysis of Ba PTA84, Ba PTA85, and Bs PTA86 suggested that enterotoxins and other known virulence factors were absent in the subject Bacillus spp. Another important safety criterion is that Bacillus DFM genomes must be devoid of transferable antimicrobial resistance genotypes (Ciorba et al., 2015). The data suggested that almost all of the tested Bacillus isolates were sensitive to the antimicrobials tested and the apparent MIC values were below the recommended cut-off values. Genomic analysis of three Bacillus spp. identified putative genes for antimicrobial resistance to tetracycline, lincosamide, and streptothricin. In the genome of Bs PTA86, putative genes conferring resistance to rifampicin and macrolides were found. However, these genes have been reported present in Ba and Bs isolates from the environment (Phelan et al., 2011; Adimpong et al., 2012), suggesting these genes may be intrinsic properties of Ba and Bs strains. Furthermore, transferable mobile genetic elements such as transposons, insertion sequences were absent in the proximity of these genes indicating the very low risk of these genes being horizontally transferred to other gut microbes pose little to no risk to public health safety.
Metabolomic Analysis
To investigate the potential host beneficial metabolites secreted by the three Bacillus strains, we performed global untargeted metabolomic analyses of Ba PTA84 and PTA85, and Bs PTA86. The use of rich and minimal media, as well as the co-culture experiments, allowed us to identify a collection of global secreted molecules of potential value. A metabolite of particular interest was 1-kestose that was identified in the culture supernatants of all strains. 1-Kestose, the smallest fructooligosaccharide (FOS), is a trisaccharide molecule composed of a glucose and two fructose residues linked by glycosidic bonds. Kestose is a prebiotic that, when consumed, enriches the growth of gut commensals such as Bifidobacteria, Lactobacillus, and Faecalibacterium prausnitzii promoting gut health (Patterson et al., 1997). Of the three strains, Ba PTA84 produced the highest amount of 1-kestose. Thioproline, an antioxidant molecule, was identified in the culture supernatant of Ba PTA84 and Bs PTA86. Thioproline was reported to inhibit carcinogenesis in humans, and is expected to act as a nitrite scavenger (Patterson et al., 2020). Pantothenate (Vitamin B5) and pyridoxine (Vitamin B6) were found in the culture supernatants of Ba PTA85 and Bs PTA86, respectively. Betaine and choline were possibly secreted by Bs PTA86. These molecules are methyl donors required for the biosynthesis of acetylcholine and phosphatidylcholine, for neural transmission and cell membrane integrity, respectively (Zempleni et al., 2007). Betaine, when supplemented in feed, has shown improved growth performance of birds during heat stresses (He et al., 2015; Liu et al., 2019). Inclusion of choline has been associated with reduced FCR in broiler chickens (Santiago et al., 2020).
Genomic analysis of Ba PTA84, PTA85, and Bs PTA86 suggested that these three strains harbor genes with complimentary activities (i.e., AMPs and enzymes). Thus, we hypothesize that inclusion of these three strains in a consortium would exert a greater benefit to the animal than that of any single strain. It is noteworthy that the subject Bacillus spp. generated more unique metabolites when grown in consortia of two or three Bacillus strains, suggesting that a combination of strains would generate distinct outcomes compared to that of single isolate (Figure 5). Indoleacetate was detected only in the culture supernatant of consortia of Ba PTA84-Ba PTA85, and Ba PTA84-Ba PTA85-Bs PTA86, but not in the individual strains. Indolacetate is an intermediate of microbial tryptophan biosynthesis that serves as a ligand for aryl hydrocarbon receptors (AhRs) enhances intestinal integrity and modulates host immune systems by exerting anti-inflammatory activities (Nawrocki et al., 2009; Tjaden, 2020). A higher abundance of tryptophan metabolites was also observed in animals treated with sub-therapeutic level of antibiotic Bacitracin Methylene Disalicylate (BMD) (Xie and Tang, 2017).
Feed Inclusion of Ba PTA84 Supported Improved Poultry Growth Performance
An in vivo efficacy study employing daily feed inclusion of Ba PTA84 resulted in significantly improved overall growth performance of broiler chickens as shown by a significant increase of ADG, production efficiency, and a reduction in feed conversion ratio. To better understand the mechanism of action underlying the effect of Ba PTA84 supplementation on animal health, we investigated the effects of supplementation of Ba PTA84 on the modulation of intestinal microbiota. The chicken gut microbiota plays prominent roles in bioavailability of nutrients, immune system development, intestinal integrity, and exclusion of unwanted microorganisms (Shang et al., 2018). Growth promotion effects of probiotics have been linked to changes in cecal microbiome structure and function (Al-Fataftah and Abdelqader, 2014; Ma et al., 2018). Interestingly, microbiome taxonomic profiling analyses of cecal contents from a control group and that with dietary supplement of Ba PTA84 suggested no significant differences between the cecal microbiome structures of the two groups according to both alpha and β-diversity parameters. Thus, it is likely that Ba PTA84 supports growth performance without altering the normal cecum microbiome. It is still possible that microbial communities in other organs are affected by supplementation of this strain. It is noteworthy that metabolomic analysis of culture supernatant of Ba PTA84 showed that it had a potential to produce 1-kestose, a microbiome modulator (Patterson et al., 1997). In the future, it would be interesting to test whether 1-kestose is indeed produced in vivo.
Conclusion
Ba PTA84 was selected via a comprehensive screening workflow as a potential probiotic for supporting improvement of growth performance in broiler chickens. Inclusion of Ba PTA84 in one study resulted in significantly improved weight gain and overall animal growth performance. More detailed studies on the mechanisms of action of Ba PTA84 are yet to be done. This includes investigating the effects of Ba PTA84 on intestinal structure, immune system function, oxidative response, microorganism exclusions, and nutrient digestibility. It is interesting to see in the future whether in-feed inclusion of other well-characterized probiotic candidates such as Ba PTA85 and Bs PTA86 or multi-strain consortia harboring a combination of genes and traits will have better outcomes than that of Ba PTA84. With the advances of sequencing technologies allowing analysis of large number of samples with relatively low cost, it is possible to use genomic analysis as an initial high-throughput screening step to eliminate candidate strains harboring genes that might have negative impacts to the animal or to public health, and importantly to enable investigating the impact of individual genes and molecules on the observed clinical outcomes.
Data Availability Statement
The raw sequencing reads, genome assemblies and annotations in this study were deposited in the NCBI BioProject database under projects PRJNA701126 and PRJNA701127. The accession numbers of CP071042, JAFMSQ000000000, and CP071043 were assigned to B. amyloliquefaciens ATCC PTA-126784 and ATCC PTA-126785, and B. subtilis ATCC PTA787 126786, respectively. Raw reads for the 16S rRNA cecal microbiome analysis were submitted to the NCBI Sequence Read Archive with accession number: PRJNA721687.
Ethics Statement
The animal study was reviewed and approved by Elanco Institutional Animal Care and Use Committee (Protocol #1395).
Author Contributions
DS, NB, DG, TH, SPM, and AK conceived and designed the experiments. DS, AV, NB, and DG performed the experiments. DS, AV, NT, NB, DG, GP, AN, SPM, and AK analyzed and interpreted data. DS, AV, NB, GP, and SPM wrote the manuscript. All authors read, reviewed, and approved the final manuscript.
Conflict of Interest
All authors are employees of Elanco Animal Health. Elanco Animal Health manufactures and markets probiotics.
Publisher’s Note
All claims expressed in this article are solely those of the authors and do not necessarily represent those of their affiliated organizations, or those of the publisher, the editors and the reviewers. Any product that may be evaluated in this article, or claim that may be made by its manufacturer, is not guaranteed or endorsed by the publisher.
Acknowledgments
The authors would like to thank Byungjoon Kwon for help with Vero cells preparation.
Supplementary Material
The Supplementary Material for this article can be found online at: https://www.frontiersin.org/articles/10.3389/fmicb.2021.747845/full#supplementary-material
Abbreviations
DFM, direct fed microbials; Ba, Bacillus amyloliquefaciens; Bs, Bacillus subtilis; ADG, average daily gain; FCR, feed conversion ration; EBI, European Broiler Index; APEC, Avian Pathogenic Escherichia coli; MIC, minimum inhibitory concentration; EFSA, European Food Safety Association; AAFCO, Association of American Feed Control Officials; ASV, amplicon sequence variant; PCA, principal component analysis.
Footnotes
References
Abudabos, A., Alyemni, A., and Zakaria, H. (2016). Effect of two strains of probiotics on the antioxidant capacity, oxidative stress, and immune responses Salmonella-challenged broilers. Brazil. J. Poult. Sci. 18, 175–180. doi: 10.1590/18069061-2015-0052
Adimpong, D. B., Sorensen, K. I., Thorsen, L., Stuer-Lauridsen, B., Abdelgadir, W. S., Nielsen, D. S., et al. (2012). Antimicrobial susceptibility of Bacillus strains isolated from primary starters for African traditional bread production and characterization of the bacitracin operon and bacitracin biosynthesis. Appl. Environ. Microbiol. 78, 7903–7914. doi: 10.1128/AEM.00730-12
Akhter, S., Aziz, R. K., and Edwards, R. A. (2012). PhiSpy: a novel algorithm for finding prophages in bacterial genomes that combines similarity- and composition-based strategies. Nucleic Acids Res. 40:e126. doi: 10.1093/nar/gks406
Al-Fataftah, A.-R., and Abdelqader, A. (2014). Effects of dietary Bacillus subtilis on heat-stressed broilers performance, intestinal morphology and microflora composition. Anim. Feed Sci. Technol. 198, 279–285. doi: 10.1016/j.anifeedsci.2014.10.012
Antelmann, H., Scharf, C., and Hecker, M. (2000). Phosphate starvation-inducible proteins of Bacillus subtilis: proteomics and transcriptional analysis. J. Bacteriol. 182, 4478–4490. doi: 10.1128/JB.182.16.4478-4490.2000
Babasaki, K., Takao, T., Shimonishi, Y., and Kurahashi, K. (1985). Subtilosin A, a new antibiotic peptide produced by Bacillus subtilis 168: isolation, structural analysis, and biogenesis. J. Biochem. 98, 585–603. doi: 10.1093/oxfordjournals.jbchem.a135315
Barbosa, T. M., Serra, C. R., La Ragione, R. M., Woodward, M. J., and Henriques, A. O. (2005). Screening for Bacillus isolates in the broiler gastrointestinal tract. Appl. Environ. Microbiol. 71, 968–978. doi: 10.1128/AEM.71.2.968-978.2005
Batz, M. B., Hoffmann, S., and Morris, J. G. Jr. (2012). Ranking the disease burden of 14 pathogens in food sources in the United States using attribution data from outbreak investigations and expert elicitation. J. Food Prot. 75, 1278–1291. doi: 10.4315/0362-028X.JFP-11-418
Berendsen, E. M., Zwietering, M. H., Kuipers, O. P., and Wells-Bennik, M. H. J. (2015). Two distinct groups within the Bacillus subtilis group display significantly different spore heat resistance properties. Food Microbiol. 45, 18–25. doi: 10.1016/j.fm.2014.04.009
Bernardeau, M., Lehtinen, M. J., Forssten, S. D., and Nurminen, P. (2017). Importance of the gastrointestinal life cycle of Bacillus for probiotic functionality. J. Food Sci. Technol. 54, 2570–2584. doi: 10.1007/s13197-017-2688-3
Bremges, A., Fritz, A., and Mchardy, A. C. (2020). CAMITAX: taxon labels for microbial genomes. GigaScience 9:giz154. doi: 10.1093/gigascience/giz154
Brul, S., Van Beilen, J., Caspers, M., O’brien, A., De Koster, C., Oomes, S., et al. (2011). Challenges and advances in systems biology analysis of Bacillus spore physiology; molecular differences between an extreme heat resistant spore forming Bacillus subtilis food isolate and a laboratory strain. Food Microbiol. 28, 221–227. doi: 10.1016/j.fm.2010.06.011
Callahan, B. J., Mcmurdie, P. J., Rosen, M. J., Han, A. W., Johnson, A. J., and Holmes, S. P. (2016). DADA2: high-resolution sample inference from Illumina amplicon data. Nat. Methods 13, 581–583. doi: 10.1038/nmeth.3869
Cardoso Dal Pont, G., Farnell, M., Farnell, Y., and Kogut, M. H. (2020). Dietary factors as triggers of low-grade chronic intestinal inflammation in poultry. Microorganisms 8:139. doi: 10.3390/microorganisms8010139
Casula, G., and Cutting, S. M. (2002). Bacillus probiotics: spore germination in the gastrointestinal tract. Appl. Environ. Microbiol. 68, 2344–2352. doi: 10.1128/AEM.68.5.2344-2352.2002
Chao, A. (1984). Nonparametric estimation of the number of classes in a population. Scand. J. Stat. 11, 265–270.
Chien, Y. C., Chen, C. J., Lin, T. H., Chen, S. H., and Chien, Y. C. (2011). Characteristics of microbial aerosols released from chicken and swine feces. J. Air. Waste. Manag. Assoc. 61, 882–889. doi: 10.3155/1047-3289.61.8.882
Ciorba, V., Odone, A., Veronesi, L., Pasquarella, C., and Signorelli, C. (2015). Antibiotic resistance as a major public health concern: epidemiology and economic impact. Ann. Ig. 27, 562–579.
Contesini, F. J., Melo, R. R. D., and Sato, H. H. (2018). An overview of Bacillus proteases: from production to application. Crit. Rev. Biotechnol. 38, 321–334. doi: 10.1080/07388551.2017.1354354
Cooper, K. K., and Songer, J. G. (2009). Necrotic enteritis in chickens: a paradigm of enteric infection by Clostridium perfringens type A. Anaerobe 15, 55–60. doi: 10.1016/j.anaerobe.2009.01.006
Danilova, I., and Sharipova, M. (2020). The practical potential of bacilli and their enzymes for industrial production. Front. Microbiol. 11:1782. doi: 10.3389/fmicb.2020.01782
de Crecy-Lagard, V. (2014). Variations in metabolic pathways create challenges for automated metabolic reconstructions: examples from the tetrahydrofolate synthesis pathway. Comput. Struct. Biotechnol. J. 10, 41–50. doi: 10.1016/j.csbj.2014.05.008
Duan, H., Chai, T., Cai, Y., Zhong, Z., Yao, M., and Zhang, X. (2008). Transmission identification of Escherichia coli aerosol in chicken houses to their environments using ERIC-PCR. Sci. China C Life Sci. 51, 164–173. doi: 10.1007/s11427-008-0021-0
Duc, L. H., Hong, H. A., Barbosa, T. M., Henriques, A. O., and Cutting, S. M. (2004). Characterization of Bacillus probiotics available for human use. Appl. Environ. Microbiol. 70, 2161–2171. doi: 10.1128/AEM.70.4.2161-2171.2004
Dziva, F., and Stevens, M. P. (2008). Colibacillosis in poultry: unravelling the molecular basis of virulence of avian pathogenic Escherichia coli in their natural hosts. Avian. Pathol. 37, 355–366. doi: 10.1080/03079450802216652
EFSA (2021). EFSA statement on the requirements for whole genome sequence analysis of microorganisms intentionally used in the food chain. EFSA J. 19:e06506. doi: 10.2903/j.efsa.2021.6506
EFSA Panel on Additives and Products or Substances used in Animal Feed [FEEDAP], Rychen, G., Aquilina, G., Azimonti, G., Bampidis, V., Bastos, M. L., et al. (2018). Guidance on the characterisation of microorganisms used as feed additives or as production organisms. EFSA J. 16:e05206. doi: 10.2903/j.efsa.2018.5206
Emms, D. M., and Kelly, S. (2019). OrthoFinder: phylogenetic orthology inference for comparative genomics. Genome Biol. 20:238. doi: 10.1186/s13059-019-1832-y
Fagerlund, A., Lindbäck, T., Storset, A. K., Granum, P. E., and Hardy, S. P. (2008). Bacillus cereus Nhe is a pore-forming toxin with structural and functional properties similar to the ClyA (HlyE, SheA) family of haemolysins, able to induce osmotic lysis in epithelia. Microbiology 154, 693–704. doi: 10.1099/mic.0.2007/014134-0
FAO-UN (2018). The Future of Food and Agriculture, Alternative Pathways to 2050. Rome: Food and Agriculture Organization of the United Nations.
Fayek, H., Abou El-Ella, M., Attia, M., and Mady, Y. (1995). Evaluation of using some feed additives in layer diets. Sci. Conf. Anim. Nutr. 5, 313–321.
Foley, S. L., and Lynne, A. M. (2008). Food animal-associated Salmonella challenges: pathogenicity and antimicrobial resistance. J. Anim. Sci. 86, E173–E187. doi: 10.2527/jas.2007-0447
Fuchs, C. S., Willett, W. C., Colditz, G. A., Hunter, D. J., Stampfer, M. J., Speizer, F. E., et al. (2002). The influence of folate and multivitamin use on the familial risk of colon cancer in women. Cancer Epidemiol. Biomark. Prevent. 11, 227–234.
Gaballa, A., Newton, G. L., Antelmann, H., Parsonage, D., Upton, H., Rawat, M., et al. (2010). Biosynthesis and functions of bacillithiol, a major low-molecular-weight thiol in Bacilli. Proc. Natl. Acad. Sci. U.S.A. 107, 6482–6486. doi: 10.1073/pnas.1000928107
Grant, A. Q., Gay, C. G., and Lillehoj, H. S. (2018). Bacillus spp. as direct-fed microbial antibiotic alternatives to enhance growth, immunity, and gut health in poultry. Avian Pathol. 47, 339–351. doi: 10.1080/03079457.2018.1464117
Green, D. H., Wakeley, P. R., Page, A., Barnes, A., Baccigalupi, L., Ricca, E., et al. (1999). Characterization of two Bacillus probiotics. Appl. Environ. Microbiol. 65, 4288–4291. doi: 10.1128/AEM.65.9.4288-4291.1999
Guo, X., Li, D., Lu, W., Piao, X., and Chen, X. (2006). Screening of Bacillus strains as potential probiotics and subsequent confirmation of the in vivo effectiveness of Bacillus subtilis MA139 in pigs. Antonie Van Leeuwenhoek 90, 139–146. doi: 10.1007/s10482-006-9067-9
He, S., Zhao, S., Dai, S., Liu, D., and Bokhari, S. G. (2015). Effects of dietary betaine on growth performance, fat deposition and serum lipids in broilers subjected to chronic heat stress. Anim. Sci. J. 86, 897–903. doi: 10.1111/asj.12372
Hill, C., Guarner, F., Reid, G., Gibson, G. R., Merenstein, D. J., Pot, B., et al. (2014). The International Scientific Association for Probiotics and Prebiotics consensus statement on the scope and appropriate use of the term probiotic. Nat. Rev. Gastroenterol. Hepatol. 11, 506–514. doi: 10.1038/nrgastro.2014.66
Hoa, T. T., Duc, L. H., Isticato, R., Baccigalupi, L., Ricca, E., Van, P. H., et al. (2001). Fate and dissemination of Bacillus subtilis spores in a murine model. Appl. Environ. Microbiol. 67, 3819–3823. doi: 10.1128/AEM.67.9.3819-3823.2001
Hyatt, D., Chen, G. L., Locascio, P. F., Land, M. L., Larimer, F. W., and Hauser, L. J. (2010). Prodigal: prokaryotic gene recognition and translation initiation site identification. BMC Bioinformatics 11:119. doi: 10.1186/1471-2105-11-119
Inatomi, T., and Otomaru, K. (2018). Effect of dietary probiotics on the semen traits and antioxidative activity of male broiler breeders. Sci. Rep. 8:5874. doi: 10.1038/s41598-018-24345-8
Jha, R., Das, R., Oak, S., and Mishra, P. (2020). Probiotics (direct-fed microbials) in poultry nutrition and their effects on nutrient utilization, growth and laying performance, and gut health: a systematic review. Animals 10:1863. doi: 10.3390/ani10101863
Kaldhusdal, M. (2000). Necrotic enteritis as affected by dietary ingredients. World Poultry 16, 42–43.
Keyburn, A. L., Boyce, J. D., Vaz, P., Bannam, T. L., Ford, M. E., Parker, D., et al. (2008). NetB, a new toxin that is associated with avian necrotic enteritis caused by Clostridium perfringens. PLoS Pathog. 4:e26. doi: 10.1371/journal.ppat.0040026
Keyburn, A. L., Sheedy, S. A., Ford, M. E., Williamson, M. M., Awad, M. M., Rood, J. I., et al. (2006). Alpha-toxin of Clostridium perfringens is not an essential virulence factor in necrotic enteritis in chickens. Infect. Immun. 74, 6496–6500. doi: 10.1128/IAI.00806-06
Kingsford, C. L., Ayanbule, K., and Salzberg, S. L. (2007). Rapid, accurate, computational discovery of Rho-independent transcription terminators illuminates their relationship to DNA uptake. Genome Biol. 8:R22. doi: 10.1186/gb-2007-8-2-r22
Koransky, J. R., Allen, S. D., and Dowell, V. R. (1978). Use of ethanol for selective isolation of sporeforming microorganisms. Appl. Environ. Microbiol. 35, 762–765. doi: 10.1128/aem.35.4.762-765.1978
Koutsoumanis, K., Allende, A., Alvarez-Ordonez, A., Bolton, D., Bover-Cid, S., Chemaly, M., et al. (2020). Update of The List of QPS-Recommended Biological Agents Intentionally Added to Food or Feed as Notified to EFSA 13: Suitability of Taxonomic Units Notified to EFSA until September 2020. Parma: EFSA.
Laslett, D., and Canback, B. (2004). ARAGORN, a program to detect tRNA genes and tmRNA genes in nucleotide sequences. Nucleic Acids Res. 32, 11–16. doi: 10.1093/nar/gkh152
Latorre, J. D., Hernandez-Velasco, X., Kallapura, G., Menconi, A., Pumford, N. R., Morgan, M. J., et al. (2014). Evaluation of germination, distribution, and persistence of Bacillus subtilis spores through the gastrointestinal tract of chickens. Poult. Sci. 93, 1793–1800. doi: 10.3382/ps.2013-03809
Latorre, J. D., Hernandez-Velasco, X., Wolfenden, R. E., Vicente, J. L., Wolfenden, A. D., Menconi, A., et al. (2016). Evaluation and selection of Bacillus species based on enzyme production, antimicrobial activity, and biofilm synthesis as direct-fed microbial candidates for poultry. Front. Vet. Sci. 3:95. doi: 10.3389/fvets.2016.00095
Liu, W., Yuan, Y., Sun, C., Balasubramanian, B., Zhao, Z., and An, L. (2019). Effects of dietary betaine on growth performance, digestive function, carcass traits, and meat quality in indigenous yellow-feathered broilers under long-term heat stress. Animals 9:506. doi: 10.3390/ani9080506
Ma, Y., Wang, W., Zhang, H., Wang, J., Zhang, W., Gao, J., et al. (2018). Supplemental Bacillus subtilis DSM 32315 manipulates intestinal structure and microbial composition in broiler chickens. Sci. Rep. 8:15358. doi: 10.1038/s41598-018-33762-8
Mandal, S., Van Treuren, W., White, R. A., Eggesbø, M., Knight, R., and Peddada, S. D. (2015). Analysis of composition of microbiomes: a novel method for studying microbial composition. Microb. Ecol. Health Dis. 26:27663. doi: 10.3402/mehd.v26.27663
Martin, M. (2011). Cutadapt removes adapter sequences from high-throughput sequencing reads. EMBnet J. 17:3. doi: 10.14806/ej.17.1.200
McCleary, B. V. (1978). A simple assay procedure for β-d-mannanase. Carbohydr. Res. 67, 213–221. doi: 10.1016/S0008-6215(00)83743-X
Mellata, M. (2013). Human and avian extraintestinal pathogenic Escherichia coli: infections, zoonotic risks, and antibiotic resistance trends. Foodborne Pathog. Dis. 10, 916–932. doi: 10.1089/fpd.2013.1533
Meyer, Y., Buchanan, B. B., Vignols, F., and Reichheld, J.-P. (2009). Thioredoxins and glutaredoxins: unifying elements in redox biology. Annu. Rev. Genet. 43, 335–367. doi: 10.1146/annurev-genet-102108-134201
Mingmongkolchai, S., and Panbangred, W. (2018). Bacillus probiotics: an alternative to antibiotics for livestock production. J. Appl. Microbiol. 124, 1334–1346. doi: 10.1111/jam.13690
Moore, P. R., Evenson, A., Luckey, T. D., Mccoy, E., Elvehjem, C. A., and Hart, E. B. (1946). Use of sulfasuxidine, streptothricin, and streptomycin in nutritional studies with the chick. J. Biol. Chem. 2, 437–441. doi: 10.1016/S0021-9258(17)41154-9
Na, S. I., Kim, Y. O., Yoon, S. H., Ha, S. M., Baek, I., and Chun, J. (2018). UBCG: up-to-date bacterial core gene set and pipeline for phylogenomic tree reconstruction. J. Microbiol. 56, 280–285. doi: 10.1007/s12275-018-8014-6
Nawrocki, E. P., Kolbe, D. L., and Eddy, S. R. (2009). Infernal 1.0: inference of RNA alignments. Bioinformatics 25, 1335–1337. doi: 10.1093/bioinformatics/btp157
Patterson, J. A., He, H., Folz, J. S., Li, Q., Wilson, M. A., Fiehn, O., et al. (2020). Thioproline formation as a driver of formaldehyde toxicity in Escherichia coli. Biochem. J. 477, 1745–1757. doi: 10.1042/BCJ20200198
Patterson, J. A., Orban, J. I., Sutton, A. L., and Richards, G. N. (1997). Selective enrichment of Bifidobacteria in the intestinal tract of broilers by thermally produced kestoses and effect on broiler performance. Poult. Sci. 76, 497–500. doi: 10.1093/ps/76.3.497
Phelan, R. W., Clarke, C., Morrissey, J. P., Dobson, A. D., O’gara, F., and Barbosa, T. M. (2011). Tetracycline resistance-encoding plasmid from Bacillus sp. strain #24, isolated from the marine sponge Haliclona simulans. Appl. Environ. Microbiol. 77, 327–329. doi: 10.1128/AEM.01239-10
Price, M. N., Dehal, P. S., and Arkin, A. P. (2010). FastTree 2–approximately maximum-likelihood trees for large alignments. PLoS One 5:e9490. doi: 10.1371/journal.pone.0009490
Rao, J., Susanti, D., Childress, J. C., Mitkos, M. C., Brima, J. K., Baffoe-Bonnie, A. W., et al. (2018). Tn2008-driven carbapenem resistance in Acinetobacter baumannii isolates from a period of increased incidence of infections in a Southwest Virginia hospital (USA). J. Glob. Antimicrob. Resist. 12, 79–87. doi: 10.1016/j.jgar.2017.08.017
Raza, A., Bashir, S., and Tabassum, R. (2019). An update on carbohydrases: growth performance and intestinal health of poultry. Heliyon 5:e01437. doi: 10.1016/j.heliyon.2019.e01437
Rossi, M., Amaretti, A., and Raimondi, S. (2011). Folate production by probiotic bacteria. Nutrients 3, 118–134. doi: 10.3390/nu3010118
Saeed, M., Aya_An, T., Alagawany, M., El-Hack, M., Abdel-Latif, M., and Patra, A. (2019). The role of ß-mannanase (hemicell) in improving poultry productivity, health and environment. Brazil. J. Poult. Sci. 21:1001. doi: 10.1590/1806-9061-2019-1001
Salem, A. R., Pattison, J. R., and Foster, M. A. (1972). Folic acid and the methylation of homocysteine by Bacillus subtilis. Biochem. J. 126, 993–1004. doi: 10.1042/bj1260993
Sánchez, B., Bressollier, P., and Urdaci, M. C. (2008). Exported proteins in probiotic bacteria: adhesion to intestinal surfaces, host immunomodulation and molecular cross-talking with the host. FEMS Immunol. Med. Microbiol. 54, 1–17. doi: 10.1111/j.1574-695X.2008.00454.x
Santiago, G. S., Vieira, S. L., Stefanello, C., Simões, C. T., Kindlein, L., Maria, D. D., et al. (2020). Dietary choline affects field performance and broiler leg deviations. Livestock Sci. 240:104127. doi: 10.1016/j.livsci.2020.104127
Sauer, U., Cameron, D. C., and Bailey, J. E. (1998). Metabolic capacity of Bacillus subtilis for the production of purine nucleosides, riboflavin, and folic acid. Biotechnol. Bioeng. 59, 227–238. doi: 10.1002/(SICI)1097-0290(19980720)59:2<227::AID-BIT10>3.0.CO;2-B
Sayers, E. W., Beck, J., Bolton, E. E., Bourexis, D., Brister, J. R., Canese, K., et al. (2021). Database resources of the National Center for Biotechnology Information. Nucleic Acids Res. 49, D10–D17. doi: 10.1093/nar/gkaa892
Schallmey, M., Singh, A., and Ward, O. P. (2004). Developments in the use of Bacillus species for industrial production. Can. J. Microbiol. 50, 1–17. doi: 10.1139/w03-076
Seemann, T. (2014). Prokka: rapid prokaryotic genome annotation. Bioinformatics 30, 2068–2069. doi: 10.1093/bioinformatics/btu153
Setlow, P. (2006). Spores of Bacillus subtilis: their resistance to and killing by radiation, heat and chemicals. J. Appl. Microbiol. 101, 514–525. doi: 10.1111/j.1365-2672.2005.02736.x
Shang, Y., Kumar, S., Oakley, B., and Kim, W. K. (2018). Chicken gut microbiota: importance and detection technology. Front. Vet. Sci. 5:254. doi: 10.3389/fvets.2018.00254
Shannon, C. E. (1997). The mathematical theory of communication. 1963. MD Comput. 14, 306–317. doi: 10.2307/3498901
Shelburne, C. E., An, F. Y., Dholpe, V., Ramamoorthy, A., Lopatin, D. E., and Lantz, M. S. (2007). The spectrum of antimicrobial activity of the bacteriocin subtilosin A. J. Antimicrob. Chemother. 59, 297–300. doi: 10.1093/jac/dkl495
Sherif, K. (2009). Performance of broiler chicks fed plant protein diets supplemented with commercial enzymes. J. Anim. Poult. Prod. 34, 2819–2834. doi: 10.21608/jappmu.2009.116931
Shivaramaiah, S. P., Pumford, N. R., Morgan, M. J., Wolfenden, R. E., Wolfenden, A. D., Hargis, B. M., et al. (2011). Evaluation of Bacillus species as potential candidates for direct-fed microbials in commercial poultry. Poult. Sci. 90, 1574–1580. doi: 10.3382/ps.2010-00745
Singh, S., and Bajaj, B. K. (2016). Bioprocess optimization for production of thermoalkali-stable protease from Bacillus subtilis K-1 under solid-state fermentation. Preparat. Biochem. Biotechnol. 46, 717–724. doi: 10.1080/10826068.2015.1135455
Stein, T., Düsterhus, S., Stroh, A., and Entian, K. D. (2004). Subtilosin production by two Bacillus subtilis subspecies and variance of the sbo-alb cluster. Appl. Environ. Microbiol. 70, 2349–2353. doi: 10.1128/AEM.70.4.2349-2353.2004
Suzek, B. E., Ermolaeva, M. D., Schreiber, M., and Salzberg, S. L. (2001). A probabilistic method for identifying start codons in bacterial genomes. Bioinformatics 17, 1123–1130. doi: 10.1093/bioinformatics/17.12.1123
Tjaden, B. (2020). A computational system for identifying operons based on RNA-seq data. Methods 176, 62–70. doi: 10.1016/j.ymeth.2019.03.026
US-FDA (2013). New Animal Drugs and New Animal Drug Combination Products Administered in or on Medicated Feed or Drinking Water of Food-Producing Animals: Recommendations for Drug Sponsors for Voluntarily Aligning Product Use Conditions with GFI #209. Rome: FAO.
Van Immerseel, F., De Buck, J., Pasmans, F., Huyghebaert, G., Haesebrouck, F., and Ducatelle, R. (2004). Clostridium perfringens in poultry: an emerging threat for animal and public health. Avian. Pathol. 33, 537–549. doi: 10.1080/03079450400013162
Wang, Y., Wu, Y., Wang, Y., Fu, A., Gong, L., Li, W., et al. (2017). Bacillus amyloliquefaciens SC06 alleviates the oxidative stress of IPEC-1 via modulating Nrf2/Keap1 signaling pathway and decreasing ROS production. Appl. Microbiol. Biotechnol. 101, 3015–3026. doi: 10.1007/s00253-016-8032-4
Weese, J. S. (2002). Microbiologic evaluation of commercial probiotics. J. Am. Vet. Med. Assoc. 220, 794–797. doi: 10.2460/javma.2002.220.794
Weese, J. S., and Martin, H. (2011). Assessment of commercial probiotic bacterial contents and label accuracy. Can. Vet. J. 52, 43–46.
White, E., Shannon, J. S., and Patterson, R. E. (1997). Relationship between vitamin and calcium supplement use and colon cancer. Cancer Epidemiol. Biomarkers Prev. 6, 769–774.
Xie, Z., and Tang, H. (2017). ISEScan: automated identification of insertion sequence elements in prokaryotic genomes. Bioinformatics 33, 3340–3347. doi: 10.1093/bioinformatics/btx433
Yilmaz, P., Parfrey, L. W., Yarza, P., Gerken, J., Pruesse, E., Quast, C., et al. (2014). The SILVA and “All-species Living Tree Project (LTP)” taxonomic frameworks. Nucleic Acids Res. 42, D643–D648. doi: 10.1093/nar/gkt1209
Zempleni, J. R. R., Mccormick, D. B., and Suttie, J. W. (2007). Handbook of Vitamins. Boca Raton, FL: CRC Press.
Zhang, J., Cai, D., Yang, M., Hao, Y., Zhu, Y., Chen, Z., et al. (2020). Screening of folate-producing lactic acid bacteria and modulatory effects of folate-biofortified yogurt on gut dysbacteriosis of folate-deficient rats. Food Funct. 11, 6308–6318. doi: 10.1039/D0FO00480D
Zhou, Y., Jiang, Z., Lv, D., and Wang, T. (2009). Improved energy-utilizing efficiency by enzyme preparation supplement in broiler diets with different metabolizable energy levels. Poult. Sci. 88, 316–322. doi: 10.3382/ps.2008-00231
Keywords: Bacillus, probiotics, poultry, growth performance, broiler chickens, multi-omics
Citation: Susanti D, Volland A, Tawari N, Baxter N, Gangaiah D, Plata G, Nagireddy A, Hawkins T, Mane SP and Kumar A (2021) Multi-Omics Characterization of Host-Derived Bacillus spp. Probiotics for Improved Growth Performance in Poultry. Front. Microbiol. 12:747845. doi: 10.3389/fmicb.2021.747845
Received: 26 July 2021; Accepted: 24 September 2021;
Published: 20 October 2021.
Edited by:
Arun K. Bhunia, Purdue University, United StatesReviewed by:
Henrique César Pereira Figueiredo, Federal University of Minas Gerais, BrazilZabdiel Alvarado-Martínez, University of Maryland, College Park, United States
Copyright © 2021 Susanti, Volland, Tawari, Baxter, Gangaiah, Plata, Nagireddy, Hawkins, Mane and Kumar. This is an open-access article distributed under the terms of the Creative Commons Attribution License (CC BY). The use, distribution or reproduction in other forums is permitted, provided the original author(s) and the copyright owner(s) are credited and that the original publication in this journal is cited, in accordance with accepted academic practice. No use, distribution or reproduction is permitted which does not comply with these terms.
*Correspondence: Dwi Susanti, ZHdpLnN1c2FudGlAZWxhbmNvYWguY29t; Arvind Kumar, YXJ2aW5kLmt1bWFyQGVsYW5jb2FoLmNvbQ==