- 1LabEx Lermit, Faculty of Medicine, INSERM UMR 1184—Team RESIST, Université Paris-Sud, Université Paris-Saclay, Le Kremlin-Bicêtre, France
- 2Associated French National Reference Center for Antibiotic Resistance: Carbapenemase-Producing Enterobacteriaceae, Le Kremlin-Bicêtre, France
- 3Department of Hormonal Biochemistry, Hôpital de Bicêtre, Assistance Publique—Hôpitaux de Paris, Le Kremlin-Bicêtre, France
- 4Bacteriology-Hygiene Unit, Assistance Publique - Hôpitaux de Paris, Bicêtre Hospital, Le Kremlin-Bicêtre, France
The differential expression of VIM-1 in Atlantibacter hermannii WEB-2 and Enterobacter hormaechei ssp. hoffmannii WEB-1 clinical isolates from a rectal swab of a hospitalized patient in France was investigated. A. hermannii WEB-2 was resistant to all β-lactams except carbapenems. It produced ESBL SHV-12, but the Carba NP test failed to detect any carbapenemase activity despite the production of VIM-1. Conversely, E. hormaechei WEB-1, previously recovered from the same patient, was positive for the detection of carbapenemase activity. The blaVIM–1 gene was located on a plasmid and embedded within class 1 integron. Both plasmids were of the same IncA incompatibility group and conferred the same resistance pattern when electroporated in Escherichia coli TOP10 or Enterobacter cloacae CIP7933. Quantitative RT-PCR experiments indicated a weaker replication of pWEB-2 in A. hermannii as compared to E. hormaechei. An isogenic mutant of A. hermannii WEB-2 selected after sequential passages with increased concentrations of imipenem possessed higher MICs for carbapenems and cephalosporins including cefiderocol, higher levels of the blaVIM–1 gene transcripts, and detectable carbapenemase activity using the Carba NP test. Assessment of read coverage demonstrated that a duplication of the region surrounding blaVIM–1 gene occurred in the A. hermannii mutant with detectable carbapenemase activity. The lack of detection of the VIM-1 carbapenemase activity in A. hermannii WEB-2 isolate was likely due to a weak replication of the IncA plasmid harboring the blaVIM–1 gene. Imipenem as selective pressure led to a duplication of this gene on the plasmid and to the restoration of a significant carbapenem-hydrolyzing phenotype.
Introduction
Atlantibacter (formerly Escherichia) hermannii now belongs to the distinct genus Atlantibacter in Enterobacterales (Brenner et al., 1982; Hata et al., 2016). A. hermannii has rarely been implicated in clinical ocular infections (Poulou et al., 2008) or colonization of human wounds (Pien et al., 1985). A. hermannii harbors a chromosome-encoded class A β-lactamase (HER-1-like), conferring resistance to amoxicillin and ticarcillin, which is reversed by clavulanate, and a moderate susceptibility to piperacillin. But it remains susceptible to all cephalosporins and carbapenems (Fitoussi et al., 1995; Beauchef-Havard et al., 2003).
The VIM-1 enzyme is a metallo-β-lactamase (MBL) identified for the first time in 1997, in a carbapenem-resistant Pseudomonas aeruginosa isolate at the Verona University Hospital (Lauretti et al., 1999). This enzyme exhibits a very broad substrate specificity, including carbapenems, and was found to be encoded by a determinant carried on a mobile gene cassette inserted into an integron located on the chromosome or on plasmids (Di Pilato et al., 2014, 2015). Infection with VIM-1-producing Klebsiella pneumoniae has become endemic in some European countries, especially in intensive care units of tertiary care hospitals in Greece (Souli et al., 2008), and sporadic cases of infection due to multidrug-resistant Enterobacterales carrying blaVIM–1 have also been reported (Perilli et al., 2002; Souli et al., 2008).
PCR-based replicon typing allowed a rapid and accurate classification of plasmid families (Carattoli et al., 2005). The IncA/C plasmid family is one the largest resistance plasmid families along with IncFI, IncFII, IncN, IncH, IncX, IncL, and IncM (Carattoli, 2009). This plasmid family is strongly associated with resistance genes such as blaCMY–2 (Carattoli et al., 2012; Martin et al., 2012) and MBL encoding genes like blaNDM–1 or blaVIM–1 (Drieux et al., 2013; Esposito et al., 2017). This plasmid family was recently divided into two plasmid families IncA and IncC, formerly IncA/C1 and IncA/C2, respectively (Harmer and Hall, 2015). Compatibility of IncA and IncC has been recently demonstrated, reinforcing the fact that IncA and IncC plasmids belong to distinct groups (San Millan et al., 2015; Ambrose et al., 2018).
Several studies have shown that, despite the presence of the VIM-1 MBL in Enterobacterales, carbapenem MICs may remain under resistance thresholds (Psichogiou et al., 2008; Souli et al., 2008; Falcone et al., 2009, 2010). However, these isolates containing VIM-1 usually possess detectable carbapenemase activity using the Carba NP test (Dortet et al., 2012). The aim of this study was to decipher the discrepancy between the molecular detection of blaVIM–1 and carbapenem susceptibility confirmed by the absence of carbapenem-hydrolyzing activity in A. hermannii.
Materials and Methods
Bacterial Strains, Antimicrobial Susceptibility, and Carbapenemase Detection
Enterobacter cloacae complex WEB-1 and A. hermannii WEB-2 clinical isolates were collected from a rectal swab of a hospitalized patient in France, in 2015. Both isolates were first identified by MALDI-TOF mass spectrometry (MALDI Biotyper, Bruker, Wissembourg, France) and then by whole-genome sequencing (WGS) and comparison with genomes in GenBank data. Isogenic imipenem-resistant A. hermannii WEB-3 mutant was obtained by repeated cultures with increased concentrations of imipenem from 0.5 to 10 mg/L (0.5, 1, 2, 4, and 10 mg/L) of the A. hermannii WEB-2 clinical isolate. These repeated cultures correspond to one overnight culture in each imipenem-supplemented broth that was inoculated in the next twofold-dilution imipenem-supplemented broth. Except for cefiderocol, the MICs were determined by E-test (bioMérieux, France) and interpreted according to the CLSI breakpoints (Humphries et al., 2018). Cefiderocol MICs were performed according to EUCAST guidelines as updated in 2019 using broth microdilution plates (EUMDROXF Plate, Thermo Fisher). Escherichia coli TOP10 (Thermo Fisher Scientific, France) and E. cloacae CIP 7933 (Pasteur Institute Collection, Paris, France) were used as hosts for electroporation experiments and analysis of antimicrobial patterns. The updated Carba NP test (Nordmann et al., 2012; Dortet et al., 2014; Dortet et al., 2018) and MALDI-TOF mass spectrometry-based assays (MBT STAR®-Carba, Bruker) (Hrabák et al., 2012; Dortet et al., 2018) were performed as previously described to monitor carbapenem-hydrolyzing activity.
Cloning of Naturally Occurring HER-9 Class A β-Lactamase of A. hermannii
The class A β-lactamase gene blaHER–9 was PCR amplified by PCR and sequenced with primers HER-9F and HER-9R, designed using the whole sequence of the WEB-2 isolate (Table 1). The obtained amplicon was cloned into the pCR-Blunt II-TOPO plasmid (Invitrogen). The recombinant pTOPO-HER-9 plasmid was electroporated into the E. coli TOP10, and the resulting recombinant E. coli TOP10 p(HER-9) was selected on ampicillin 100 mg/L containing trypticase soy agar (TSA) plates.
Plasmid Extraction and Transformation
Plasmid DNA was extracted from both E. hoffmannii WEB-1 and A. hermannii WEB-2 isolates with the Kieser technique as previously described (Kieser, 1984) and electroporated in E. coli TOP10 and E. cloacae CIP 7933. Recombinant strains were selected on TSA supplemented with 100 mg/L of ticarcillin (Sigma, St-Quentin-Fallavier, France).
Nucleic Acid Extractions, PCR, and qRT-PCR
Total DNA was extracted using the QIAGEN TipG500 genomic extraction kit (Qiagen, Courtaboeuf, France). The DNA concentration and purity were controlled by a Qubit® 2.0 fluorometer using the dsDNA HS and/or BR assay kit (Life Technologies). Transcription of the carbapenemase gene was further studied by qRT-PCR using CFX96 (Bio-Rad) on RNA extracts from exponential phase (RNA minikit, Qiagen, Courtaboeuf, France) treated with DNAse. For each reaction, 20 ng of RNA was used. Transcription levels were standardized relative to the transcription level of the constitutive rpoB gene or to the repA gene located on the IncA plasmid (Table 1). The Rotor Gene SYBR Green OneStep RT-PCR kit (Qiagen) was used in qRT-PCR experiments, and all experiments were performed in triplicate. After the reverse transcription step for 10 min at 55°C, qPCR included an initial denaturation step of 3 min at 95°C, 40 cycles of denaturation (95°C/5 s) and annealing and extension (60°C/10 s) as previously described (Naas et al., 2012). The transcriptional levels were interpreted using 2–ΔΔCt (Falcone et al., 2009; Girlich et al., 2017).
Whole-Genome Sequencing and Genomic Analyses
Whole-genome sequencing was performed with two different techniques: short-read Illumina sequencing and long-read Oxford Nanopore technology. The DNA library was prepared either (i) by using the NEB Ultra II FS DNA library prep kit for Illumina (NEB, Evry, France) and run as 75- or 150-bp paired-end reads with V2 chemistry on a MiSeq sequencer or (ii) by using a R9.4 flow cell and the 1D native barcoding genomic DNA kit (SQK-LSK109) on a MinION sequencer (Oxford Nanopore Technologies, ONT). De novo assembly of the reads obtained with the libraries and comparison of the coverage of blaVIM–1, repA (IncA), traI, parA, blaSHV–12, and intI1 genes from the plasmid and rpoB, recA, dnaA, G6Pdh, and gyrB genes from the chromosome were performed with CLC Genomics Workbench v12.1 (Qiagen, Les Ulis, France). Demultiplexing and assembly of the Nanopore reads were done with Guppy and Canu v1.8 workflows, respectively. The acquired antimicrobial resistance genes were identified using ResFinder server v2.1 (Center for Genomic Epidemiology (CGE1), and the genome was annotated using the RAST server. The plasmid content was analyzed by using PlasmidFinder (CGE) and manually searched by homology.
Representative members of all Enterobacter species have been used to classify Enterobacter spp. WEB-1, and a phylogenetic tree was created using the CSI Phylogeny 1.4 server2 (Kaas et al., 2014). Phylogenetic tree was represented using Figtree v1.4.33.
GenBank Accession Number
Genomes of E. hormaechei ssp. hoffmannii WEB-1 and A. hermannii WEB-2 and WEB-3 have been deposited in GenBank under GenBank accession number JAEVEU00000000, JAEVEV00000000, and JAEVEW00000000, respectively.
Results and Discussion
Bacterial Strains and Antimicrobial Susceptibility
Phylogenetic analysis revealed that E. cloacae complex WEB-1 actually corresponds to E. hormaechei ssp. hoffmannii WEB-1 (hereafter E. hoffmannii WEB-1) (Supplementary Figure 1). A. hermannii WEB-2 showed resistance to amoxicillin, ticarcillin, ceftazidime, and aztreonam, which was only slightly reversed by clavulanate; a moderate susceptibility to piperacillin and other cephalosporins; and susceptibility to carbapenems and cefiderocol (MICs at 0.12 mg/L). In contrast, E. hoffmannii WEB-1 was resistant to all β-lactams (Table 2). Regarding non-β-lactams, A. hermannii WEB-2 showed resistance to quinolones, sulfonamides, and trimethoprim–sulfamethoxazole and susceptibility to fosfomycin, gentamicin, chloramphenicol, and tigecycline (data not shown). The global susceptibility pattern suggested the production of an extended-spectrum-β-lactamase in addition to the chromosomal class A HER-type β-lactamase. WGS data confirmed the identification of the blaSHV–12 ESBL encoding gene. Analysis of the resistome of E. hoffmannii WEB-1 indicated the presence of three β-lactamase encoding genes: (i) blaVIM–1, (ii) blaSHV–12, and (iii) the cephalosporinase encoding gene blaACT–67. Several aminoglycoside-modifying enzymes (AMEs) have been identified including aadA1, aadA2-like, aph(3″)-Ib-like, aph(6′)-Id, and aac(6′)-Ib. Acquired quinolone resistance qnrS1 gene was also identified along with dfrA14, sul1, sul2, catB2, cmlA1-like, mph(A), and fosA, encoding resistance to trimethoprim, sulfamethoxazole, chloramphenicol, macrolides, and fosfomycin, respectively. Noticeably, the fosA gene is naturally occurring in Enterobacter spp. The resistome of A. hermannii WEB-2 was similar to that of E. hoffmannii WEB-1, with the exception of the presence of blaHER–like β-lactamase, the intrinsic class A β-lactamase of A. hermannii, and the absence of cmlA1-like and blaACT–67. These close resistomes may indicate a probable plasmid location of the common genes and a conjugative transfer from one clinical isolate to the another (E. hoffmannii WEB-1 to A. hermannii WEB-2 or vice versa).
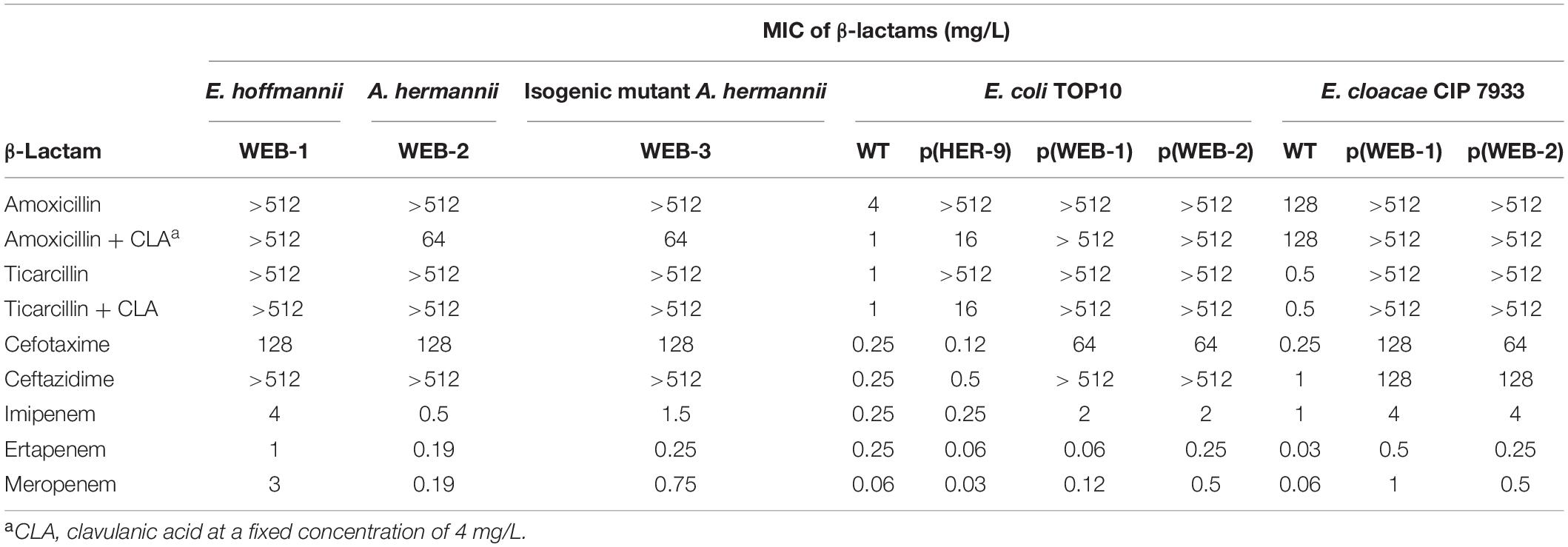
Table 2. MIC of β-lactams for E. hoffmannii WEB-1, A. hermannii WEB-2, isogenic mutant A. hermannii WEB-3, E. coli TOP10 wild type (WT), and recombinant E. coli isolates with plasmids from E. hoffmannii WEB-1 and from A. hermannii WEB-2 and for E. cloacae CIP7933 and recombinant E. cloacae isolates with plasmids from E. hoffmannii WEB-1 and from A. hermannii WEB-2.
Cloning and Sequencing of the HER-Type Class A β-Lactamase Gene
Cloning of the blaHER–9 gene in the pTOPO plasmid allowed us to obtain the recombinant E. coli TOP10 p(HER-9) strain expressing a narrow-spectrum resistance pattern limited to penicillins and susceptibility to β-lactamase/inhibitor combinations and to other β-lactams (Table 2). The deduced penicillinase HER-9 showed 79% amino acid identity with the closest relative, HER-6 from A. hermannii 2-89 (Beauchef-Havard et al., 2003). Contrary to the eight previously reported variants showing little inter-strain variability (≥98% identity) (Beauchef-Havard et al., 2003), this β-lactamase exhibited lower identity of 77.2–78.6%.
Carbapenemase Activity
A significant carbapenemase activity could be detected for the E. hoffmannii WEB-1 using both the Carba NP test and the MALDI-TOF mass spectrometry-based assay, MBT STAR®-Carba assay. Intriguingly, on the A. hermannii WEB-2 isolate, the Carba NP test failed to detect any carbapenemase activity. Of note, the MBT STAR®-Carba assay was still able to detect a slight imipenem hydrolysis. Although not quantitative, this test already showed a higher sensitivity for the detection of carbapenemase with low hydrolytic activity (e.g., OXA-244) compared to biochemical tests (i.e., Carba NP test) (Hoyos-Mallecot et al., 2017).
Plasmid Extraction, Transformation, and Expression
Plasmid DNAs from both E. hoffmannii WEB-1 and A. hermannii WEB-2 were extracted and transformed into either E. coli TOP10 or E. cloacae CIP7933 in order to compare the susceptibility profiles. Both recombinant strains expressed a phenotype compatible with the production of VIM-1 and SHV-12 β-lactamases and similar MICs to β-lactams (Table 2). In both strains, imipenem hydrolysis was successfully detected using the Carba NP test and MBT STAR®-Carba assay. This result demonstrated that the lack of detection of carbapenemase activity using the Carba NP test in A. hermannii WEB-2 was not due to the structure of the plasmid p(WEB-2) but rather to the genetic background of the parental strain.
The blaVIM–1 Gene and the Genetic Context
In order to verify that differential expression was not due to different promoter sequences, upstream regions of blaVIM–1 were analyzed. In E. hoffmannii WEB-1 and A. hermannii WEB-2, the blaVIM–1 gene was enclosed in a common class 1 integron in first position. In both isolates, the blaVIM–1 gene was preceded by the PcH1 promoter [−35 (TGGACA) and −10 (TAAACT) regions spaced by 17 bp] and a P2 promoter, located 90 bp downstream of Pc [−35 (TTGTTA) and −10 (TACAGT) regions]. However, the promoter P2 might be inactive since −35 and −10 boxes were separated by only 14 bp. The third promoter Pint (TTGCTG-n = 17-TAGACT) is responsible for the expression of intI1. An inverse correlation has been demonstrated between the strength of promoter PC+P2 and excision activity of integrase (Jové et al., 2010). The association of PcH1 and inactive P2 gives rise to a weak promoter combination and thus may lead to a high excision activity of class 1 integrase. The same genetic structure was present at the promoter site in E. hormaechei WEB-1 and A. hermannii WEB-2 excluding any promoter-dependent differential expression of blaVIM–1 in these two isolates. Moreover, comparison of the same region between A. hermannii WEB-2 and its isogenic mutant A. hermannii WEB-3 showed no difference as well.
Genomic and Transcriptomic Analysis of E. hoffmannii WEB-1, A. hermannii WEB-2, and A. hermannii WEB-3
An isogenic mutant of A. hermannii WEB-2, named A. hermannii WEB-3, was obtained after sequential passages of the parental strain in imipenem-containing broth. Although A. hermannii WEB-3 was able to grow on agar plates and in broth supplemented with 10 mg/L of imipenem; the carbapenem MICs were only slightly increased as compared to the parental strain and remained in the susceptible range (Table 2). Noticeably, the MIC of cefiderocol was increased in the mutant from 0.12 to 1 mg/L for A. hermannii WEB-2 and A. hermannii WEB-3, respectively. The addition of EDTA restored the susceptibility to cefiderocol at 0.25 mg/L (Supplementary Figure 2). Another crucial difference between A. hermannii WEB-2 and A. hermannii WEB-3 resides in the fact that A. hermannii WEB-3 mutant now possesses a detectable carbapenem-hydrolyzing activity using the Carba NP test.
WGS was performed simultaneously with DNA extracts from E. hoffmannii WEB-1, A. hermannii WEB-2, and the isogenic mutant A. hermannii WEB-3 using Illumina technology. Raw data are summarized in Supplementary Table 1.
Analysis of the genetic background (chromosome sequence) of the A. hermannii WEB-3 mutant strain vs. its parental A. hermannii WEB-2 isolates showed only one SNP in the fliC gene (T136 G) conferring an amino acid change in the flagellin (FliC) protein (Ser46Ala).
PlasmidFinder identified an IncA plasmid that is 98.32% identical to the reference IncA/C pRA-1 sequence (accession number FJ705807) in both E. hoffmannii WEB-1 and A. hermannii WEB-2 clinical isolates. Mapping of the reads of p(WEB-2) to p(WEB-1) used as the reference showed no difference between those IncA plasmids. In E. hoffmannii WEB-1, an additional plasmid belonging to the IncFIB subgroup was identified (data not shown). Analysis of the promoter sequence of blaVIM–1 on p(WEB-3) showed no mutation that could be involved in this differential expression of blaVIM–1 in the WEB-3 isogenic strain. Quantitative RT-PCR experiments performed on E. hoffmannii WEB-1, A. hermannii WEB-2, and A. hermannii WEB-3 isolates showed that the blaVIM–1 transcription level was similar in E. hoffmannii WEB-1 and A. hermannii WEB-2 when the repA(IncA) replicase gene was used as reference (22.1- vs. 25.4-fold of the expression level of repA). This result suggested a similar expression in isolates WEB-1 and WEB-2 when compared to repA expression. However, when rpoB is used as control, the expression of blaVIM–1 is 10-fold lower in WEB-2, indicating an overall lower expression of the carbapenemase in A. hermannii WEB-2. This result showed a weaker transcription relative to chromosomal genes in the parental A. hermannii WEB-2 strain (Table 3). Moreover, comparison of the transcription of repA(IncA/C) vs. that of rpoB showed that it was 10-fold higher in E. hoffmannii WEB-1 than in the A. hermannii WEB-2 isolate. On the other hand, the expression level of blaVIM–1 was 20-fold higher (406.7-fold of the expression level of repA) in A. hermannii WEB-3 (Table 4). Altogether, these qRT-PCR results showed that a weaker expression of VIM-1 in A. hermannii WEB-2 might be due to a lower replication of the IncA plasmid in A. hermannii (Table 4).
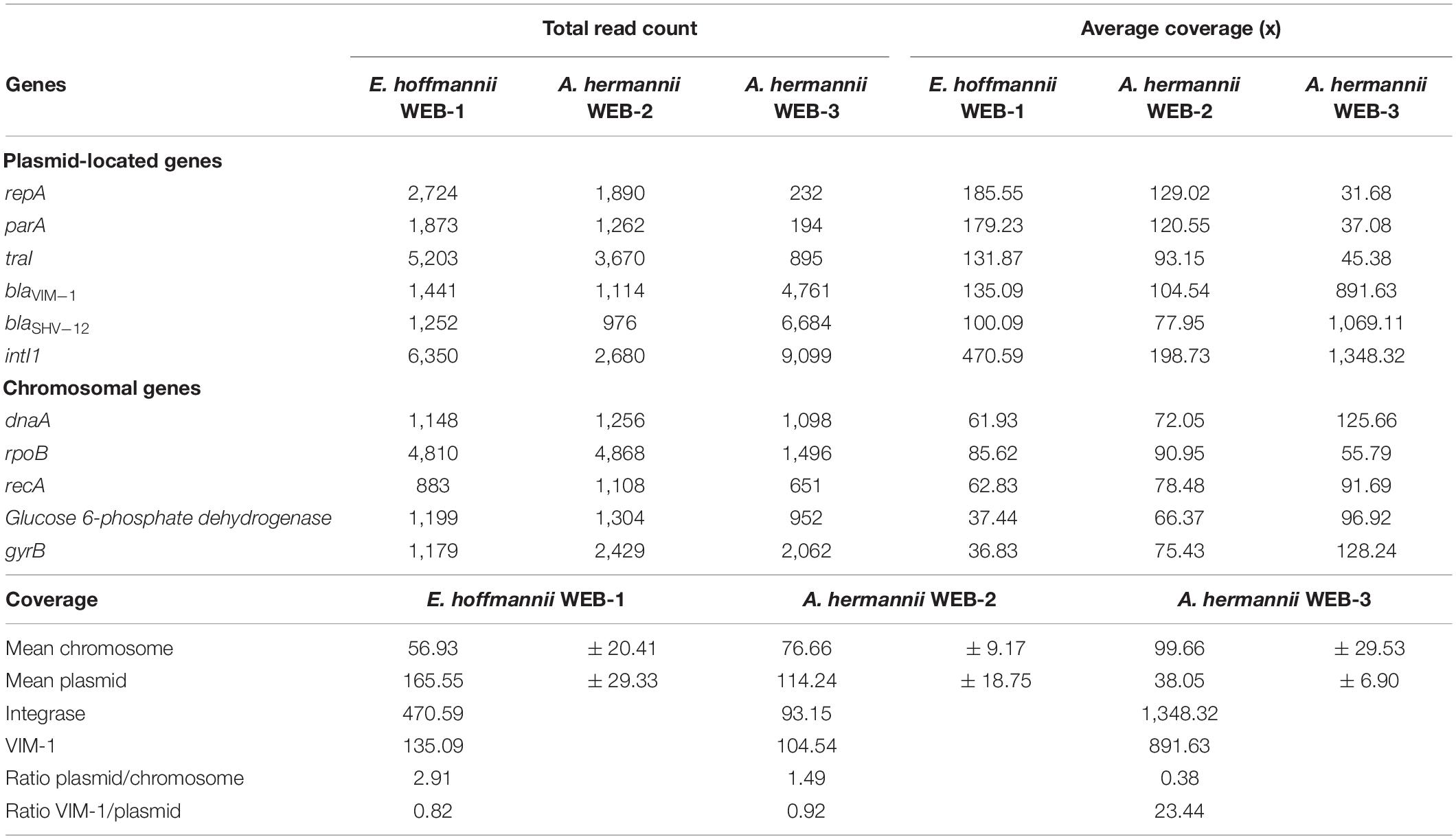
Table 3. Total read count and average coverage for chromosomal and plasmid-located genes in E. hoffmannii WEB-1, A. hermannii WEB-2, and isogenic mutant A. hermannii WEB-3.

Table 4. Fold change RNA transcription of blaVIM–1 relative to that of repA(IncA) and rpoB in E. hormaechei WEB-1, A. hermannii WEB-2, and isogenic mutant A. hermannii WEB-3.
Genomic Analysis of E. hoffmannii WEB-1, A. hermannii WEB-2, and Its Mutant: Role of Gene Amplification and Plasmid Copy Number
To confirm qRT-PCR results, comparison of the coverage of the plasmid-located genes (blaVIM–1, repA(IncA), traI (IncA), and blaSHV–12 and intI1) vs. that of chromosomal genes (rpoB, recA, dnaA, g6-pdh, and gyrB) highlighted that plasmid genes were covered 2.91-fold more than those located on the chromosome in E. hoffmannii WEB-1, whereas this coverage was only 1.49-fold in A. hermannii WEB-2 (Table 3). It suggests that E. hoffmannii WEB-1 harbors twofold more copies of the blaVIM–1-carrying IncA-type plasmid compared to A. hermannii WEB-2. Of note, the coverage ratio blaVIM–1/plasmid is nearly identical in E. hoffmannii WEB-1 and A. hermannii WEB-2 (0.82 vs. 0.92), suggesting that only one copy of blaVIM–1 was present on p(WEB-1) and p(WEB-2) plasmids. Quantitative RT-PCR experiments showed that the transcription level of blaVIM–1 was similar in E. hoffmannii WEB-1 and A. hermannii WEB-2 when the repA(IncA) replicase gene was used as reference (22.1 vs. 25.4-fold the expression level of repA) (Table 4). It confirms an equal copy number of blaVIM–1 on both plasmids p(WEB-1) and p(WEB-2), previously deciphered as one copy per plasmid by coverage analysis (Table 3). On the contrary, the expression level of blaVIM–1 was ∼20-fold higher (406.7-fold the expression level of repA) in A. hermannii WEB-3 compared to E. hoffmannii WEB-1 and A. hermannii WEB-2 (Table 4 and Figure 1). It might be due to (i) ∼20 duplications of blaVIM–1 containing elements on the p(WEB-2) plasmid, (ii) to duplication of such element on the (WEB-3) plasmid as well as on the chromosome, or (iii) to the higher copy number of the blaVIM–1-carrying plasmid in the A. hermannii WEB-3 strain. As shown in Table 4, no increase of plasmid copy number was evidenced in the A. hermannii WEB-3 strain but rather a decrease of copy number. In addition, a striking increase of blaVIM–1/plasmid coverage was observed from 0.92 to 23.44 X (Table 3). Accordingly, this result is in favor of ∼20 duplications of blaVIM–1-containing elements on the p(WEB-3) plasmid including the whole integron and surrounding region. In addition, the long-read WGS analysis confirmed that blaVIM–1 was absent from the chromosomes of A. hermannii WEB-2 and A. hermannii WEB-3.
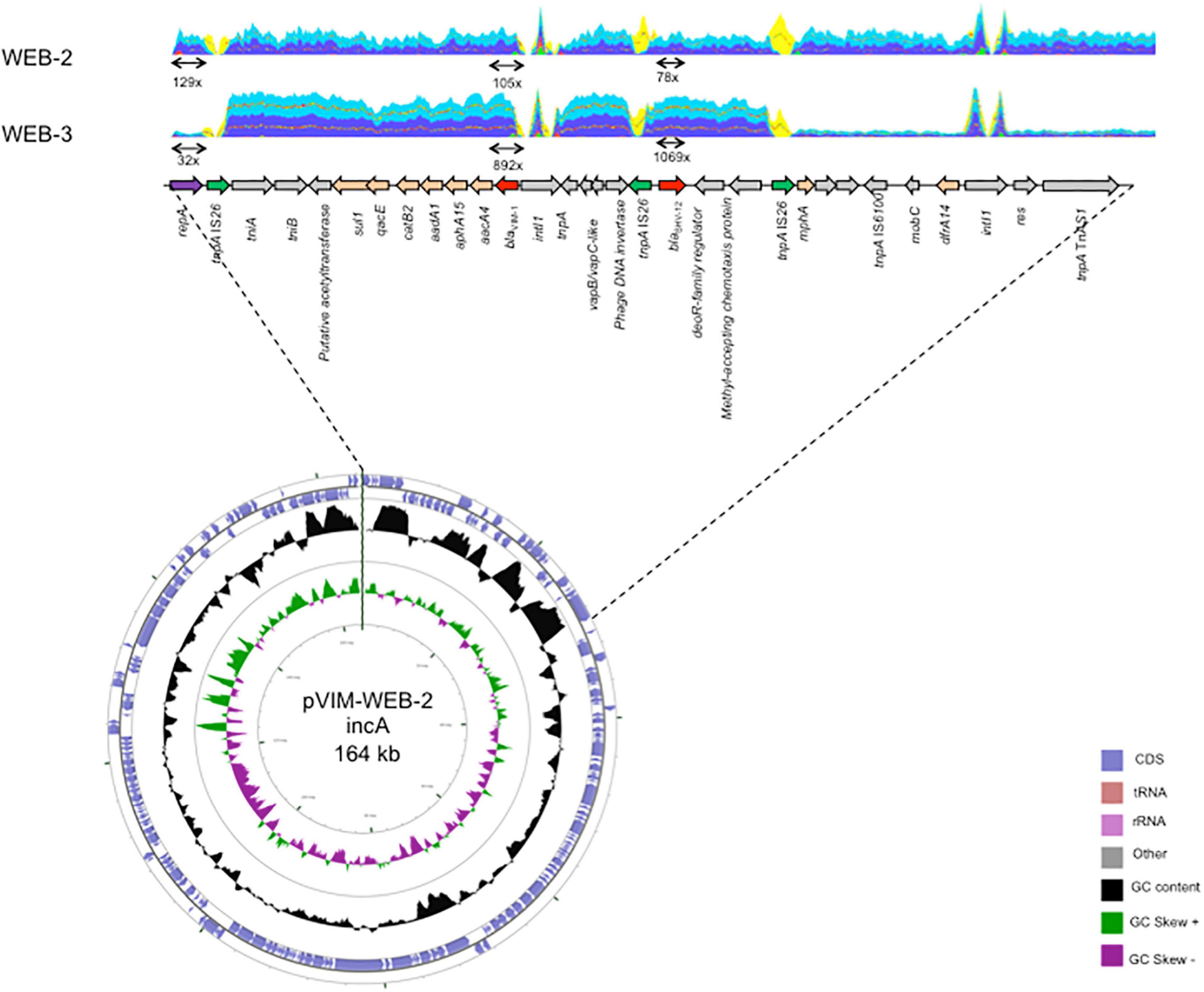
Figure 1. Schematic representation of the p(WEB-2) plasmid and the structure carrying the blaVIM–1 carbapenemase gene in A. hermannii WEB-2 and WEB-3. Open reading frames are indicated by arrows. Gene coverage was indicated at scale with the genetic organization. Gene coverage is indicated on selected features and determined using Illumina read sequencing.
Since the mean size of MinION reads was 6 kb (Supplementary Table 1), these multiple duplications (∼20 duplications) did not allow us to reconstruct the entire p(WEB-3) plasmid despite long-read WGS having been performed. Thus, to decipher if only blaVIM–1 or the complete integron was duplicated on the p(WEB-3) plasmid, Illumina reads of A. hermannii WEB-3 were mapped against p(WEB-2), which was easily reconstructed from the long-read WGS data of A. hermannii WEB-2 (Figure 1). Coverage analysis indicated that the blaVIM–1 gene cassette was not the sole duplicated gene. The duplicated region was a 17-kbp fragment bracketed by two copies of IS26 (Figure 1). Of note, due to the size of MinION reads, only two copies of this entire region were evidenced on a single read. To verify that the p(WEB-3) plasmid corresponded to an increased size of 391 kbp (corresponding to 23 kbp × 17 kbp) compared to the p(WEB-2) plasmid, an extraction was performed followed by gel electrophoresis. Unfortunately, despite repeated attempts, no size estimation was possible. Indeed, the p(WEB-3) plasmid extracted by the Kieser method did not migrate properly in the 0.7% agar gel likely due to its huge size.
Conclusion
Here, we described the identification of the blaVIM–1 gene in A. hermannii that weakly expressed its carbapenemase. In the same patient, an E. hoffmannii was previously recovered and correctly identified as a VIM-1 producer. Analysis of the plasmid content of these two isolates revealed a similar blaVIM–1-carrying plasmid belonging to the IncA-incompatibility group. Both plasmids were transferred in E. coli and showed similar resistance profiles when introduced in the same genetic background. The analysis of genetic coverage coupled with qRT-PCR experiments led us to decipher that the weaker expression of blaVIM–1 in A. hermannii WEB-2 as compared to E. hoffmannii WEB-1 was the consequence of a lower blaVIM–1-carrying plasmid copy number in A. hermannii WEB-2. Upon imipenem exposure, we selected an isogenic mutant with higher carbapenem MICs and a significant carbapenemase activity. In this mutant, the plasmid copy number was lower than that in the parental strain, but the region encompassing the carbapenemase gene, bracketed by copies of IS26, was duplicated several times. It highlights the ability of A. hermannii to respond to antibiotic selective pressure by duplicating antimicrobial resistance gene copy numbers. Of note, we identified that these multiple duplications occurred in a region bracketed by IS26 inside the parental plasmid. As previously described, it highlights the role of IS26 in gene amplification through hot spot duplication (Hubbard et al., 2020; Rodríguez-Villodres et al., 2020).
Finally, despite the fact that A. hermannii and E. hoffmannii belong to the family of Enterobacteriaceae (Enterobacterales order), the expression and replication of plasmids may vary from one genus to another, leading to altered phenotypes that result in the misidentification of carbapenemase-encoding genes. In addition, we demonstrated that under specific antibiotic selective pressure, clinical isolates possessing carbapenemase-encoding genes without phenotypic expression could easily convert to true carbapenemase producers (increase of MICs and carbapenemase activity) through gene duplication as observed for ceftazidime/avibactam resistance in K. pneumoniae (Coppi et al., 2020).
Data Availability Statement
The datasets presented in this study can be found in online repositories. The names of the repository/repositories and accession number(s) can be found below: https://www.ncbi.nlm.nih.gov/genbank/, JAEVEU00000000; https://www.ncbi.nlm.nih.gov/genbank/, JAEVEV00000000; and https://www.ncbi.nlm.nih.gov/genbank/, JAEVEW00000000.
Author Contributions
DG and RB: study design, analysis, and proofreading of manuscript. AP: data analysis. TN: proofreading of manuscript. LD: study design and proofreading of manuscript. All authors contributed to the article and approved the submitted version.
Funding
This work was partially funded by the University Paris-Saclay, France, and by grants from the French National Research Agency (ANR-10-LABX-33 and ANR-17-ASTR-0018-03). LD, TN, and RB are members of the Laboratory of Excellence in Research on Medication and Innovative Therapeutics (LERMIT).
Conflict of Interest
The authors declare that the research was conducted in the absence of any commercial or financial relationships that could be construed as a potential conflict of interest.
Publisher’s Note
All claims expressed in this article are solely those of the authors and do not necessarily represent those of their affiliated organizations, or those of the publisher, the editors and the reviewers. Any product that may be evaluated in this article, or claim that may be made by its manufacturer, is not guaranteed or endorsed by the publisher.
Acknowledgments
We would like to thank Thomas Huby and Linda Falgenhauer (Justus Liebig University Giessen, Germany) for their help.
Supplementary Material
The Supplementary Material for this article can be found online at: https://www.frontiersin.org/articles/10.3389/fmicb.2021.741972/full#supplementary-material
Supplementary Figure 1 | Phylogenetic tree of Enterobacter species. Accession number of representative isolates of each species is indicated within brackets. Enterobacter WEB-1 is indicated in bold. This phylogenetic tree was obtained using CSI Phylogeny.
Supplementary Figure 2 | Impact of blaVIM–1 gene amplification on susceptibility to cefiderocol, mecillinam, moxalactam, and cefoxitin of A. hermannii WEB-2 and WEB-3. Seven microliters of EDTA at 0.5 M was used for inhibition.
Footnotes
- ^ https://cge.cbs.dtu.dk/services/
- ^ https://cge.cbs.dtu.dk/services/CSIPhylogeny/
- ^ http://tree.bio.ed.ac.uk
References
Ambrose, S. J., Harmer, C. J., and Hall, R. M. (2018). Compatibility and entry exclusion of IncA and IncC plasmids revisited: IncA and IncC plasmids are compatible. Plasmid 9, 7–12. doi: 10.1016/j.plasmid.2018.02.002
Beauchef-Havard, A., Arlet, G., Gautier, V., Labia, R., Grimont, P., and Philippon, A. (2003). Molecular and biochemical characterization of a novel class A beta-lactamase (HER-1) from Escherichia hermannii. Antimicrob. Agents Chemother. 47, 2669–2673. doi: 10.1128/AAC.47.8.2669-2673.2003
Brenner, D. J., Davis, B. R., Steigerwalt, A. G., Riddle, C. F., McWhorter, A. C., Allen, S. D., et al. (1982). Atypical biogroups of Escherichia coli found in clinical specimens and description of Escherichia hermannii sp. nov. J. Clin. Microbiol. 15, 703–713. doi: 10.1128/jcm.15.4.703-713.1982
Carattoli, A. (2009). Resistance plasmid families in Enterobacteriaceae. Antimicrob. Agents Chemother. 53, 2227–2238.
Carattoli, A., Bertini, A., Villa, L., Falbo, V., Hopkins, K. L., and Threlfall, E. J. (2005). Identification of plasmids by PCR-based replicon typing. J. Microbiol. Methods 63, 219–228.
Carattoli, A., Villa, L., Poirel, L., Bonnin, R. A., and Nordmann, P. (2012). Evolution of IncA/C blaCMY-2-carrying plasmids by acquisition of the blaNDM-1 carbapenemase gene. Antimicrob. Agents Chemother. 56, 783–786. doi: 10.1128/AAC.05116-11
Coppi, M., Di Pilato, V., Monaco, F., Giani, T., Conaldi, P. G., and Rossolini, G. M. (2020). Ceftazidime-avibactam resistance associated with increased blaKPC-3 gene copy number mediated by pKpQIL plasmid derivatives in sequence type 258 Klebsiella pneumoniae. Antimicrob. Agents Chemother. 64, e1816–e1819. doi: 10.1128/AAC.01816-19
Di Pilato, V., Pollini, S., and Rossolini, G. M. (2014). Characterization of plasmid pAX22, encoding VIM-1 metallo-β-lactamase, reveals a new putative mechanism of In70 integron mobilization. J. Antimicrob. Chemother. 69, 67–71. doi: 10.1093/jac/dkt311
Di Pilato, V., Pollini, S., and Rossolini, G. M. (2015). Tn6249, a new Tn6162 transposon derivative carrying a double-integron platform and involved with acquisition of the blaVIM-1 metallo-β-lactamase gene in Pseudomonas aeruginosa. Antimicrob. Agents Chemother. 59, 1583–1587. doi: 10.1128/AAC.04047-14
Dortet, L., Brechard, L., Poirel, L., and Nordmann, P. (2014). Impact of the isolation medium for detection of carbapenemase-producing Enterobacteriaceae using an updated version of the Carba NP test. J. Med. Microbiol. 63, 772–776. doi: 10.1099/jmm.0.071340-0
Dortet, L., Poirel, L., and Nordmann, P. (2012). Rapid identification of carbapenemase types in Enterobacteriaceae and Pseudomonas spp. by using a biochemical test. Antimicrob. Agents Chemother. 56, 6437–6440. doi: 10.1128/AAC.01395-12
Dortet, L., Tandé, D., de Briel, D., Bernabeu, S., Lasserre, C., Gregorowicz, G., et al. (2018). MALDI-TOF for the rapid detection of carbapenemase-producing Enterobacteriaceae: comparison of the commercialized MBT STAR® -Carba IVD Kit with two in-house MALDI-TOF techniques and the RAPIDEC® CARBA NP. J. Antimicrob. Chemother. 73, 2352–2359. doi: 10.1093/jac/dky209
Drieux, L., Decré, D., Frangeul, L., Arlet, G., Jarlier, V., and Sougakoff, W. (2013). Complete nucleotide sequence of the large conjugative pTC2 multireplicon plasmid encoding the VIM-1 metallo-β-lactamase. J. Antimicrob. Chemother. 68, 97–100. doi: 10.1093/jac/dks367
Esposito, E. P., Gaiarsa, S., Del Franco, M., Crivaro, V., Bernardo, M., Cuccurullo, S., et al. (2017). A Novel IncA/C1 Group Conjugative Plasmid, Encoding VIM-1 Metallo-Beta-Lactamase, Mediates the Acquisition of Carbapenem Resistance in ST104 Klebsiella pneumoniae isolates from neonates in the intensive care unit of V. Monaldi Hospital in Naples. Front. Microbiol. 8:2135. doi: 10.3389/fmicb.2017.02135
Falcone, M., Mezzatesta, M. L., Perilli, M., Forcella, C., Giordano, A., Cafiso, V., et al. (2009). Infections with VIM-1 metallo-beta-lactamase-producing enterobacter cloacae and their correlation with clinical outcome. J. Clin. Microbiol. 47, 3514–3519. doi: 10.1128/JCM.01193-09
Falcone, M., Perilli, M., Mezzatesta, M. L., Mancini, C., Amicosante, G., Stefani, S., et al. (2010). Prolonged bacteraemia caused by VIM-1 metallo-beta-lactamase-producing Proteus mirabilis: first report from Italy. Clin. Microbiol. Infect. Off. Public Eur. Soc. Clin. Microbiol. Infect. Dis. 16, 179–181. doi: 10.1111/j.1469-0691.2009.02781.x
Fitoussi, F., Arlet, G., Grimont, P. A., Lagrange, P., and Philippon, A. (1995). Escherichia hermannii: susceptibility pattern to beta-lactams and production of beta-lactamase. J. Antimicrob. Chemother. 36, 537–543. doi: 10.1093/jac/36.3.537
Girlich, D., Bonnin, R. A., Jousset, A., and Naas, T. (2017). Promoter characterization and expression of the blaKPC-2 gene in Escherichia coli, Pseudomonas aeruginosa and Acinetobacter baumannii. J. Antimicrob. Chemother. 72, 1597–1601. doi: 10.1093/jac/dkx044
Harmer, C. J., and Hall, R. M. (2015). The A to Z of A/C plasmids. Plasmid 80, 63–82. doi: 10.1016/j.plasmid.2015.04.003
Hata, H., Natori, T., Mizuno, T., Kanazawa, I., Eldesouky, I., Hayashi, M., et al. (2016). Phylogenetics of family Enterobacteriaceae and proposal to reclassify Escherichia hermannii and Salmonella subterranea as Atlantibacter hermannii and Atlantibacter subterranea gen. nov., comb. nov. Microbiol. Immunol. 60, 303–311. doi: 10.1111/1348-0421.12374
Hoyos-Mallecot, Y., Naas, T., Bonnin, R. A., Patino, R., Glaser, P., Fortineau, N., et al. (2017). OXA-244-Producing Escherichia coli isolates, a challenge for clinical microbiology laboratories. Antimicrob. Agents Chemother. 61:e00818-17. doi: 10.1128/AAC.00818-17
Hrabák, J., Studentová, V., Walková, R., Zemlicková, H., Jakubu, V., Chudácková, E., et al. (2012). Detection of NDM-1, VIM-1, KPC, OXA-48, and OXA-162 carbapenemases by matrix-assisted laser desorption ionization-time of flight mass spectrometry. J. Clin. Microbiol. 50, 2441–2443. doi: 10.1128/JCM.01002-12
Hubbard, A. T. M., Mason, J., Roberts, P., Parry, C. M., Corless, C., van Aartsen, J., et al. (2020). Piperacillin/tazobactam resistance in a clinical isolate of Escherichia coli due to IS26-mediated amplification of blaTEM-1B. Nat. Commun. 11:4915. doi: 10.1038/s41467-020-18668-2
Humphries, R. M., Ambler, J., Mitchell, S. L., Castanheira, M., Dingle, T., Hindler, J. A., et al. (2018). CLSI methods development and standardization working group best practices for evaluation of antimicrobial susceptibility tests. J. Clin. Microbiol. 56:e01934-17. doi: 10.1128/JCM.01934-17
Jové, T., Da Re, S., Denis, F., Mazel, D., and Ploy, M.-C. (2010). Inverse correlation between promoter strength and excision activity in class 1 integrons. PLoS Genet. 6:e1000793. doi: 10.1371/journal.pgen.1000793
Kaas, R. S., Leekitcharoenphon, P., Aarestrup, F. M., and Lund, O. (2014). Solving the problem of comparing whole bacterial genomes across different sequencing platforms. PLoS One 9:e104984. doi: 10.1371/journal.pone.0104984
Kieser, T. (1984). Factors affecting the isolation of CCC DNA from Streptomyces lividans and Escherichia coli. Plasmid 12, 19–36. doi: 10.1016/0147-619x(84)90063-5
Lauretti, L., Riccio, M. L., Mazzariol, A., Cornaglia, G., Amicosante, G., Fontana, R., et al. (1999). Cloning and characterization of blaVIM, a new integron-borne metallo-beta-lactamase gene from a Pseudomonas aeruginosa clinical isolate. Antimicrob. Agents Chemother. 43, 1584–1590. doi: 10.1128/AAC.43.7.1584
Martin, L. C., Weir, E. K., Poppe, C., Reid-Smith, R. J., and Boerlin, P. (2012). Characterization of blaCMY-2 plasmids in Salmonella and Escherichia coli isolates from food animals in Canada. Appl. Environ. Microbiol. 78, 1285–1287. doi: 10.1128/AEM.06498-11
Naas, T., Cuzon, G., Truong, H.-V., and Nordmann, P. (2012). Role of ISKpn7 and deletions in blaKPC gene expression. Antimicrob. Agents Chemother. 56, 4753–4759. doi: 10.1128/AAC.00334-12
Nordmann, P., Poirel, L., and Dortet, L. (2012). Rapid detection of carbapenemase-producing Enterobacteriaceae. Emerg. Infect. Dis. 18, 1503–1507.
Perilli, M., Dell’Amico, E., Segatore, B., de Massis, M. R., Bianchi, C., Luzzaro, F., et al. (2002). Molecular characterization of extended-spectrum β-lactamases produced by nosocomial isolates of Enterobacteriaceae from an Italian Nationwide Survey. J. Clin. Microbiol. 40, 611–614. doi: 10.1128/JCM.40.2.611-614.2002
Pien, F. D., Shrum, S., Swenson, J. M., Hill, B. C., Thornsberry, C., and Farmer, J. J. (1985). Colonization of human wounds by Escherichia vulneris and Escherichia hermannii. J. Clin. Microbiol. 22, 283–285. doi: 10.1128/jcm.22.2.283-285.1985
Poulou, A., Dimitroulia, E., Markou, F., and Tsakris, A. (2008). Escherichia hermannii as the sole isolate from a patient with purulent conjunctivitis. J. Clin. Microbiol. 46, 3848–3849. doi: 10.1128/JCM.01119-08
Psichogiou, M., Tassios, P. T., Avlamis, A., Stefanou, I., Kosmidis, C., Platsouka, E., et al. (2008). Ongoing epidemic of blaVIM-1-positive Klebsiella pneumoniae in Athens, Greece: a prospective survey. J. Antimicrob. Chemother. 61, 59–63. doi: 10.1093/jac/dkm443
Rodríguez-Villodres, Á, Gil-Marqués, M. L., Álvarez-Marín, R., Bonnin, R. A., Pachón-Ibáñez, M. E., Aguilar-Guisado, M., et al. (2020). Extended-spectrum resistance to β-lactams/β-lactamase inhibitors (ESRI) evolved from low-level resistant Escherichia coli. J. Antimicrob. Chemother. 75, 77–85. doi: 10.1093/jac/dkz393
San Millan, A., Toll-Riera, M., Escudero, J. A., Cantón, R., Coque, T. M., and MacLean, R. C. (2015). Sequencing of plasmids pAMBL1 and pAMBL2 from Pseudomonas aeruginosa reveals a blaVIM-1 amplification causing high-level carbapenem resistance. J. Antimicrob. Chemother. 70, 3000–3003. doi: 10.1093/jac/dkv222
Souli, M., Kontopidou, F. V., Papadomichelakis, E., Galani, I., Armaganidis, A., and Giamarellou, H. (2008). Clinical experience of serious infections caused by Enterobacteriaceae producing VIM-1 metallo-beta-lactamase in a Greek University Hospital. Clin. Infect. Dis. Off. Public Infect. Dis. Soc. Am. 46, 847–854. doi: 10.1086/528719
Keywords: β-lactamase, carbapenemase, transcription, Enterobacter, plasmid
Citation: Girlich D, Bonnin RA, Proust A, Naas T and Dortet L (2021) Undetectable Production of the VIM-1 Carbapenemase in an Atlantibacter hermannii Clinical Isolate. Front. Microbiol. 12:741972. doi: 10.3389/fmicb.2021.741972
Received: 15 July 2021; Accepted: 17 November 2021;
Published: 20 December 2021.
Edited by:
Miklos Fuzi, Semmelweis University, HungaryReviewed by:
Teresa M. Coque, Ramón y Cajal Institute for Health Research, SpainSanda Sardelic, University Hospital Split, Croatia
Copyright © 2021 Girlich, Bonnin, Proust, Naas and Dortet. This is an open-access article distributed under the terms of the Creative Commons Attribution License (CC BY). The use, distribution or reproduction in other forums is permitted, provided the original author(s) and the copyright owner(s) are credited and that the original publication in this journal is cited, in accordance with accepted academic practice. No use, distribution or reproduction is permitted which does not comply with these terms.
*Correspondence: Laurent Dortet, bGF1cmVudC5kb3J0ZXRAYXBocC5mcg==