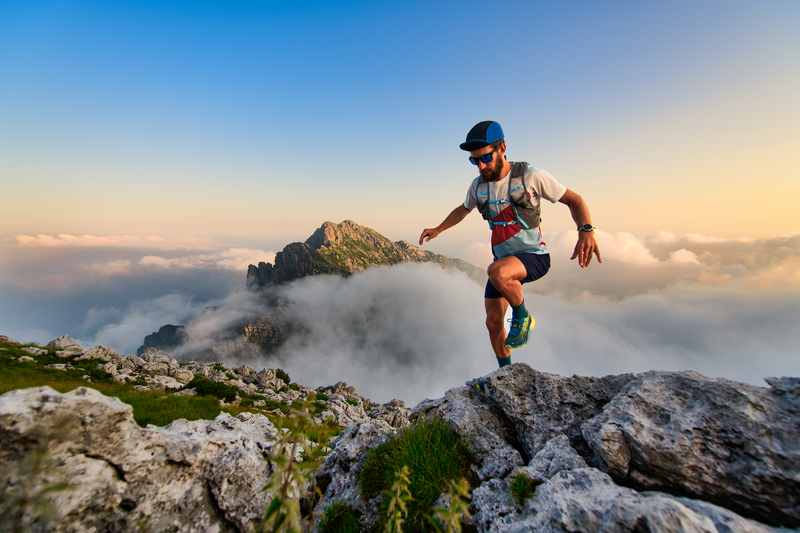
95% of researchers rate our articles as excellent or good
Learn more about the work of our research integrity team to safeguard the quality of each article we publish.
Find out more
ORIGINAL RESEARCH article
Front. Microbiol. , 17 September 2021
Sec. Microorganisms in Vertebrate Digestive Systems
Volume 12 - 2021 | https://doi.org/10.3389/fmicb.2021.741616
Diet and host genetics influence the composition of intestinal microbiota, yet few studies have compared the function of intestinal microbiota in the diet- or genotype-induced lipid deposition, which limits our understanding of the role of intestinal bacteria in metabolic disorders. The lipid accumulation in wild-type zebrafish fed with control (CON) or high-fat (HF) diet and two gene-knockout zebrafish lines (cpt1b–/– or pparab–/–) fed with control diet was measured after a 4-week feeding experiment. The intestinal microbiota composition of these groups was investigated using 16S ribosomal RNA (rRNA) gene sequencing (DNA-based) and 16S rRNA sequencing (RNA-based). The HF diet or deficiency of two genes induced more weight gain and higher triglyceride content in the liver compared with their control group. 16S rRNA gene sequencing (DNA-based) indicated the decreased abundance of Proteobacteria in the HF group compared with CON, but there was no significant difference in bacterial α diversity among treatments. 16S rRNA sequencing (RNA-based) confirmed the decreased abundance of Proteobacteria and the bacterial α diversity in the HF group compared with CON. Deficiency of cpt1b or pparab showed less change in microbiota composition compared with their wild-type group. Intestinal microbiota of each group was transferred to germ-free zebrafish, and the quantification of Nile red staining indicated that the intestinal microbiota of the HF group induced more lipid accumulation compared with CON, whereas intestinal microbiota of cpt1b–/– and pparab–/– zebrafish did not. The results showed that RNA-based bacterial sequencing revealed more bacterial alteration than DNA-based bacterial sequencing. HF diet had a more dominant role in shaping gut microbiota composition to induce lipid accumulation compared with the gene-knockout of cpt1b or pparab in zebrafish, and the transplant of intestinal microbiota from HF-fed fish induced more lipid deposition in germ-free zebrafish. Together, these data suggested that a high-fat diet exerted a more dominant role over the deletion of cpt1b or pparab on the intestinal bacterial composition, which corresponded to lipid accumulation.
Obesity is one of the most prevalent global challenges, as it increases the risk of a wide range of chronic diseases (Brial et al., 2018; Indiani et al., 2018; Moen et al., 2018). It is well-known that both diet components and genetic factors play important roles in determining an individual’s predisposition to weight gain and being obese (Goodarzi, 2018). Increasing pieces of evidence also suggested that intestinal microbiota was indispensable for the development of obesity. The conventionalization of germ-free mice with the microbiota from obese mice caused substantially higher adiposity than those conventionalized with the microbiota from lean ones (Bäckhed et al., 2004; Turnbaugh et al., 2008). Several bacteria have been found to contribute to lipid accumulation by different mechanisms in mammals. For example, Lactobacillus paracasei produces L-lactate, which is converted by intestinal epithelial cells into fatty acids and, subsequently, increases lipid storage in intestinal epithelial cells, whereas Escherichia coli produces acetate, which activates genes involved in fatty acids oxidation (Araujo et al., 2020).
Being the substrates for the host and microbiota, diet components influence the intestinal microbial composition and lipid deposition (Landgraf et al., 2017; Araujo et al., 2020). Except for affording more energy, it was found that a high-fat diet diminished gut barrier-protecting bacteria and enriched endotoxin producers (Chassaing et al., 2015) to induce lipid accumulation (Faillaci et al., 2018). Although the intestinal microbiota is influenced by diet components, there is a substantial interindividual variation in the intestinal bacterial composition in response to diets, suggesting the genetic factor should not be ignored when we evaluate the metabolic characteristics (Ussar et al., 2016; Singh et al., 2019). Kreznar et al. (2017) characterized the metabolic phenotypes of eight genetically distinct inbred mouse strains in response to a high energy diet and found that different mouse strains showed great variation in the intestinal microbiota as well as the diabetes-related phenotypes, showing the dominant role of genetic factors in shaping the intestinal microbiota. Gut microbiota, which acts as a “second genome” for modulating the nutrition, metabolism, and immunity phenotypes of the superorganism host (Jia et al., 2008), is likely indispensable for obesity development. It has been shown that the presence of diet enriched the bacteria from the phylum Firmicutes, which were sufficient to increase the number of intestinal epithelial lipid droplets in zebrafish (Semova et al., 2012). However, few studies have compared the function of intestinal microbiota in diet-induced or genotype-induced lipid deposition.
As in mammals, fish also accumulate extra lipid when fed with a high-fat diet (Zhang et al., 2020) and the knockout of genes related to lipid catabolism also cause lipid accumulation in zebrafish (Li et al., 2020). Identifying the contribution of intestinal microbiota in diet- or genetic-induced obesity in fish, which are more primitive than mammals, may afford more evolutionary information on the complex relationship among nutrients intake, genetic factors, intestinal microbiota, and the development of obesity.
16S ribosomal RNA (rRNA) gene amplicon sequencing is widely used to reflect the bacterial composition, but it should be noticed that a high abundance of certain bacteria not necessarily implies the high activity of these bacteria (De Vrieze et al., 2018). 16S rRNA sequencing is also used to estimate the active bacterial members, and plenty of studies indicate the existence of a difference between the DNA and the RNA profiles, suggesting alternative techniques including intestinal microbiota transplant or metabolomics should be involved to identify the function of microbiota (Franchi et al., 2018).
In the present study, the relative contributions of intestinal microbiota in genotype- or diet-induced lipid accumulation were detected in zebrafish. Two gene-knockout models (cpt1b–/– and pparab–/–) were generated. Carnitine acyltransferase 1b (cpt1b) is a limiting enzyme of fatty acid β-oxidation, which plays a crucial role in transferring long-chain fatty acid from CoA to carnitine for oxidation in the mitochondrion (Li et al., 2018). Peroxisome proliferator-activated receptor α is one of the nuclear receptors that promotes fatty acid β-oxidation and regulates lipid homeostasis (Madureira et al., 2017). Wild-type (WT) zebrafish were fed with different energy diets (control diet or high-fat diet) and different genotypes (cpt1b–/– and pparab–/–). Zebrafish were fed with the control diet for 4 weeks. The lipid accumulation phenotypes were measured, and the intestinal microbiota composition was analyzed based on 16S rRNA gene (DNA-based) and 16S rRNA (RNA-based) sequencing. To detect the bacterial function, the intestinal microbiota of adult zebrafish was collected from each experimental group (CON vs. HF, WT vs. cpt1b–/–, WT vs. pparab–/–) and transplanted to larval germ-free zebrafish to identify whether intestinal microbiota reshaped by different diet components or genetic background could cause lipid accumulation in germ-free zebrafish.
All experiments were performed under the Guidance for the Care and Use of Laboratory Animals in China. Laboratory Animals in China. This study was approved by the Committee on the Ethics of Animal Experiments of East China Normal University (No. F20190101).
The WT adult AB line zebrafish (4–5 months) used in the experiment were obtained from the Chinese National Zebrafish Resource Center (Wuhan, China). Zebrafish were reared in a zebrafish breeding recirculating system under a 14-h light–10-h dark cycle at 28°C. Dissolved oxygen and nitrogen were 6.07 ± 0.01 and 0.11 ± 0.01 mg/L, respectively. The fish were fed twice daily (09:00 and 17:00) using a commercial diet (Shengsuo, Yantai, China) containing 50% protein and 7% lipid. One week before the experiment, the male fish were selected for the treatments to avoid the sex differences in metabolism.
The cpt1b–/– and pparab–/– zebrafish were generated using CRISPR/Cas9 gene-editing technology as described in our previous studies (Lu D. L. et al., 2019; Li et al., 2020).
The WT zebrafish were randomly divided into two groups. They were fed with a CON diet (7% fat) and HF diet (15% fat) (Table 1). The cpt1b–/–, pparab–/– zebrafish and their matrilineal WT fish were fed with the control diet. Each treatment had three replicate tanks (40 fish per tank). Fish were fed twice daily at 9:00 am and 5:00 pm at a ratio of 4% of the fish weight of each tank for 4 weeks. Fish were weighed every week to plot the weight gain curve. At the end of the feeding trial, all fish were fasted for 24 h and then sampled. Fifteen fish of each treatment were randomly collected to measure the body length and body weight to determine the condition factor (CF; CF = body weight/body length3) and then used for the total lipid extraction. Twelve fish of each treatment were dissected on ice to obtain the liver and muscle for the subsequent biochemical analysis.
Total lipid content was measured using the classic methanol–chloroform method (Bligh and Dyer, 1959). The triglyceride (TG) contents of the liver and muscle were determined with commercial kits according to the manufacturer’s instructions (Nanjing Jiancheng Bioengineering Institute, Nanjing, China). Protein concentration was determined with the Enhanced BCA Protein Assay Kit (Beyotime, Biotechnology, Shanghai, China).
Bacterial DNA was extracted from approximately 0.1 g of intestinal contents of a mixture of five fish using QlAamp DNA Stool Mini Kit 51604 (QIAGEN, Hilden, Germany) following the manufacturer’s instructions. RNA was extracted from approximately 0.1 g of intestinal content of a mixture of five fish, according to a previously described method (Zhang Z. et al., 2019) with some modifications. Co-extracted DNA was removed using RNase-free DNase (QIAGEN, Hilden, Germany) according to the manufacturer’s instructions. The resulting RNA was purified using an RNeasy® Mini Kit (QIAGEN, Hilden, Germany). Purified RNA of intestinal microbiota was used for complementary DNA synthesis using a PrimeScriptTM RT-PCR Kit (Takara, Dalian, China) according to the instruction of the manufacturers. The final quality of the complementary DNA and DNA was checked by 1% agarose gel electrophoresis. The amplicons were subsequently purified and analyzed on an Illumina Miseq Platform (Personal Biotechnology Co., Ltd., Shanghai, China) by sequencing the V3-V4 16S rRNA region, using the primers 338F 5-ACTCCTACGGGAGGCAGCA-3 and 806R 5-GGACTACHVGGGTWTCTAAT-3. To distinguish DNA and RNA samples, each reverse primer was tagged with a unique 6-bp unique barcode. The amplification reaction and thermal cycles were described in a previous study (Lu H. F. et al., 2019). Microbiota sequencing data were submitted to National Center for Biotechnology Information with the accession number PRJNA632454.
A nonparametric Mann–Whitney U test was used to test the significant differences among groups. Principal component analyses based on unweighted UniFrac distance metrics were conducted, and the R package was used to visualize interactions among the bacterial communities from different samples. Bacterial richness and diversity across the samples were calculated using Chao1, ACE, Shannon, and Simpson richness estimators (Oksanen et al., 2014; Lu H. F. et al., 2019). Spearman’s correlation analyses were used to assess the potential association between the bacterial genera and the lipid deposition phenotype in zebrafish using Hmisc package in R (McMurdie and Holmes, 2013).
The production of germ-free zebrafish was performed following established protocols as previously described (Pham et al., 2008; Tan et al., 2019). To determine the function of gut microbiota in lipid accumulation, the intestinal bacterial cells collected from zebrafish in different treatments were added to the gnotobiotic zebrafish medium containing 3 days post-fertilization (dpf) germ-free zebrafish at a concentration of 104 CFUs/ml (Tan et al., 2019) and the inoculation lasted for 7 days. The zebrafish were fasted for 12 h before Nile Red staining.
Nile Red powder (Sangon Biotech, Shanghai, China) was dissolved in acetone at a concentration of 1 mg/ml as the stock solution. Larvae were immersed in system water containing 0.1 mg/ml Nile Red stock for 1 h at room temperature in the dark. Afterward, the stained larvae were washed with fresh medium three times to remove Nile red adhering to the surface. Images were obtained using an ECLIPSE Ti2 fluorescence microscope (Nikon, Tokyo, Japan) at an excitation wavelength of 543 nm. The images were converted to an 8-bit grayscale for measuring mean gray value using ImageJ software (National Institutes of Health). The fluorescent area and intensity of images were quantified using ImageJ to evaluate the lipid accumulation degree of larvae.
All data were presented as mean ± SEM. Student’s unpaired t-test analyzed the significant difference. All statistical analyzes were performed using the Statistical Package for the Social Sciences version 20.0 software for Windows (IBM, Armonk, NY, United States). Results with a p-value < 0.05 were considered statistically significant. Correlations with p-values lower than 0.05 were considered significant.
During the feeding trial, WT AB line zebrafish fed with the HF diet gained more weight and showed significantly increased values of CF, which was supposed to be higher in fatter fish (Landgraf et al., 2017), compared with those fed with the CON diet (Figures 1A,B). Furthermore, the HF group had higher contents of total lipid of whole fish and TG in the liver and muscle compared with the CON group (Figures 1C,D), suggesting that a high-fat diet induced extra lipid accumulation in zebrafish. After ingesting the control diet for 4 weeks, cpt1b–/– zebrafish gained more bodyweight (Figure 1E) and showed increased values of CF relative to WT zebrafish (Figure 1F). Total lipid content of whole fish showed no significant difference between WT and cpt1b–/– zebrafish (Figure 1G), whereas cpt1b–/– zebrafish presented higher TG level in the liver (Figure 1H). The growth curve revealed that pparab–/– zebrafish gained higher body weight than WT zebrafish (Figure 1I). Both the values of CF and the contents of total lipid were higher in pparab–/– zebrafish, but no significant difference was found (Figures 1J,K). Furthermore, pparab–/– zebrafish showed higher TG contents in the liver and muscle (Figure 1L). These data indicated that compared with WT zebrafish, cpt1b–/– and pparab–/– zebrafish were characterized by a significantly higher degree of lipid accumulation.
Figure 1. Phenotypes of zebrafish with different genotypes or fed on different diets. (A) Growth curve established using average bodyweight of two dietary groups (CON and HF) every week for 4 weeks (n = 3 per group). (B) Condition factor (CF) of two dietary groups (n = 15 per group). (C) Total lipid of whole fish of two dietary groups (n = 15 per group). (D) Triglyceride contents of liver and muscle of two groups (n = 8 per group). (E) Growth curve established using average bodyweight of WT and cpt1b–/– zebrafish every week for 4 weeks (n = 3 per group). (F) Condition factor of WT and cpt1b–/– zebrafish (n = 15 per group). (G) Total lipid of whole fish of WT and cpt1b–/– zebrafish (n = 15 per group). (H) Triglyceride contents in liver and muscle of WT and cpt1b–/– zebrafish (n = 8 per group). (I) Growth curve established using average bodyweight of WT and pparab–/– zebrafish every week for 4 weeks (n = 3 per group). (J) Condition factor of WT and pparab–/– zebrafish (n = 15 per group). (K) Total lipid of whole fish of WT and pparab–/– zebrafish (n = 15 per group). (L) Triglyceride contents in liver and muscle of WT and pparab–/– zebrafish (n = 8 per group). All data are normalized to control group (100%) and presented as means ± SEM. Significance difference was analyzed by Student’s t-test (*p < 0.05, **p < 0.01, ***p < 0.001).
To investigate the responses of intestinal microbiota to different diets or gene mutants, the microbial composition of each experimental group was analyzed by high-throughput sequencing based on microbial 16S rRNA gene and 16S rRNA. In total, samples based on total microbial analysis yielded between 60,423 to 75,919 reads per sample with an average of 66,031 sequences per sample [2,200 operational taxonomic units (OTUs) per sample]. For samples of 16S rRNA analysis, we obtained 61,599 to 74,862 OTUs per sample in total, with an average of 70,282 reads per sample (around 1,400 OTUs per sample). DNA-based analyses indicated no significant difference in α diversity between the dietary challenge groups or manipulating genetic groups according to ACE, Chao1, Shannon, and Simpson richness estimators (Supplementary Figure 1). RNA-based microbial data showed a less diverse microbial community in the HF group relative to the CON group (p < 0.001) (Figures 2A–C). The HF group showed a significantly higher level of diversity based on the Simpson richness indices (Figure 2D). In contrast, no significant difference was found among these diversity indexes when WT was compared with the cpt1b–/– group (p > 0.05) (Figures 2E–H) or WT relative to the pparab–/– group (p > 0.05) (Figures 2I–L). In summary, the active microbial community captured the decline of gut microbial α diversity in the HF group compared with the CON group, whereas DNA-based microbial sequence reflected no evident difference in α diversity among all experimental groups (Supplementary Figure 1).
Figure 2. Boxplots of α diversity indices of bacterial community based on 16S rRNA sequencing. (A–D) Gut microbial α diversity of dietary groups based on (A) ACE, (B) Chao1, (C) Shannon, and (D) Simpson. (E–H) Gut microbial α diversity of WT and cpt1b–/– zebrafish groups based on (E) ACE, (F) Chao1, (G) Shannon, and (H) Simpson. (I–L) Gut microbial α diversity of WT and pparab–/– zebrafish based on (I) ACE, (J) Chao1, (K) Shannon, and (L) Simpson. All data are normalized to control group (100%) (**p < 0.01).
According to DNA-based analysis, all identified reads from the intestinal samples belonged to 20 phyla, and over 70% of sequences belonged to two phyla (Proteobacteria and Fusobacteria), followed by Bacteroidetes and Firmicutes (Figures 3A–C). Intriguingly, the abundance of Proteobacteria in the HF group remarkably decreased compared with the CON group (Figure 3D). The abundance of Proteobacteria showed no difference between genes manipulated groups (cpt1b–/– or pparab–/–) and the WT group (Figures 3E,F). The effects of host genetics and dietary components on the ratio of Bacteroidetes to Firmicutes were also estimated, and no significant difference was found (Figures 3G–I).
Figure 3. Relative phyla abundance of microbiome based on 16S rRNA gene sequencing. (A) Relative abundances of phylum level among dietary groups (CON and HF). (B) Relative abundances of phylum level between WT and cpt1b–/– zebrafish. (C) Relative abundances of phylum level between WT and pparab–/– zebrafish. (D–F) Ratio of Firmicutes/Bacteroidetes in gut microbiota of (D) dietary groups, (E) WT and cpt1b–/– zebrafish, and (F) WT and pparab–/– zebrafish. (G–I) Ratio of Fusobacteria/Proteobacteria in gut microbiota of (G) dietary groups, (H) WT and cpt1b–/– zebrafish, and (I) WT and pparab–/– zebrafish. All data are normalized to control group (100%) and presented as means ± SEM (**p < 0.01, ***p < 0.001).
At the RNA level, 17 bacterial phyla were found in the zebrafish gut, and most of the identified reads belong to two phyla, Proteobacteria and Fusobacteria, followed by Bacteroidetes and Firmicutes, which was similar to DNA-based sequencing analysis (Figures 4A–C). A significant decrease in the abundance of Proteobacteria was also observed in the HF group compared with the CON group, whereas there was no significant distinction of that between genes manipulated groups (cpt1b–/– or pparab–/–) and the WT group (Figures 4D–F). Inconsistent with the total microbial analysis, the active microbial analysis illustrated that HF diet significantly reduced the ratio of Firmicutes to Bacteroidetes vs. CON diet (Figure 4G), but no dramatic difference of that was detected in cpt1b–/– or pparab–/– compared with the WT group (Figures 4H,I). These results demonstrated that although dietary interruption and gene manipulation all induced extra lipid accumulation in zebrafish, only the HF diet reduced the abundance of Proteobacteria.
Figure 4. Relative phyla abundances of microbiome based on 16S rRNA sequencing. (A) Relative abundances of phylum level of two dietary groups (CON and HF). (B) Relative abundances of phylum level between WT and cpt1b–/– zebrafish. (C) Relative abundances of phylum level between WT and pparab–/– zebrafish. (D–F) Ratio of Firmicutes/Bacteroidetes in gut microbiota of (D) dietary groups, (E) WT and cpt1b–/– zebrafish, and (F) WT and pparab–/– zebrafish. (G–I) Ratio of Fusobacteria/Proteobacteria in gut microbiota of (G) dietary groups, (H) WT and cpt1b–/– zebrafish, and (I) WT and pparab–/– zebrafish. All data are normalized to control group (100%) and presented as means ± SEM (**p < 0.01, ***p < 0.001).
The intestinal microbiota in the HF diet group differed from the control group (Figures 5A,B), whereas it showed no separation between genes-manipulated groups and WT based on active or total microbial community (Figures 5C–F). Considering that dietary interruption caused more significant changes of intestinal microbiota, Spearman’s rank correlation was performed to identify the correlation between the gut bacterial genus and lipid overaccumulation parameters between the control and high-fat diet groups. At the DNA level, excessive lipid accumulation or weight gain induced by the HF diet was positively related to Dechloromonas (Spearman’s correlation > 0.3, p < 0.05) and negatively associated with Enhydrobacte, Paracoccus, and Pseudomonas (Spearman’s correlation < −0.3, p < 0.05) (Figure 5G). At the RNA level, three genus-level taxa Cetobacteria, Aeromonadaceae, and Thauera were positively correlated with lipid accumulation or higher weight phenotype, whereas 24 genus-level taxa, including Bifidobacterium, Bacteroides, and Prevotella, were negatively relevant to lipid overaccumulation (Figure 5H), suggesting the active microbial sequencing revealed more bacteria genus associated with the lipid deposition in dietary treatments. Cetobacteria and Pseudomonas were the most abundant among 27 microbial genera, and the abundance of Cetobacteria was negatively linked with Pseudomonas (Figure 5I).
Figure 5. Comparison of gut microbiota composition among different dietary groups and different genotypes on same diets. (A) Principal components analysis (PCA) scores plot of dietary groups (CON and HF) based on 16S rRNA genes (n = 5 per group). (B) PCA scores plot of dietary groups (CON and HF) based on 16S rRNA (n = 5 per group). (C) PCA scores plot of WT and cpt1b–/– zebrafish based on 16S rRNA genes (n = 5 per group). (D) PCA scores plot of WT and cpt1b–/– zebrafish based on 16S rRNA (n = 5 per group). (E) PCA scores plot of WT and pparab–/– zebrafish based on 16S rRNA genes (n = 5 per group). (F) PCA scores plot of WT and pparab–/– zebrafish based on 16S rRNA (n = 5 per group). (G,H) Heatmap illustrates Spearman’s correlation between microbial genus and lipid overaccumulation traits measured between control and high-fat diet group based on (G) total microbial analysis and (H) active microbial analysis. (I) Correlation between abundance of microbial genus and lipid overaccumulation traits in HF groups based on active microbial analysis. *p < 0.05, **p < 0.01, ***p < 0.001.
To further identify the causal relationship of the microbiota in the lipid accumulation, we colonized germ-free zebrafish at 3 dpf with the intestinal microbiota of adult zebrafish collected from each experimental group (CON vs. HF, WT vs. cpt1b–/–, WT vs. pparab–/–). The lipid accumulation phenotype of zebrafish larvae was detected by Nile Red staining. The statistical analyses of fluorescent area and intensity showed that the microbiome of the HF group induced a significantly higher degree of lipid accumulation in germ-free zebrafish than that of the CON group (Figures 6A,D,E). The colonization of microbiota with the intestinal microbiota from cpt1b–/– zebrafish or pparab–/– zebrafish caused no significant difference in lipid accumulation compared with WT zebrafish (Figures 6B–E), implying that intestinal microbes reshaped by HF diet donated more to lipid accumulation in zebrafish larvae compared with microbiota from groups of gene manipulation.
Figure 6. Nile red staining visualizes deep tissue fat deposition in zebrafish. (A–C) Fluorescent images of adipose tissue using Nile red staining of (A) CON and HF zebrafish, (B) WT and cpt1b–/– zebrafish, and (C) WT and pparab–/– zebrafish. (D,E) Quantitative evaluation of fluorescent area (D) and intensity (E) of Nile red staining (n = 6 per group). All data are presented as means ± SEM (***p < 0.001).
Identifying the interaction among the host genetics, diet, or intestinal microbiota is indispensable for revealing the pathogenesis of obesity. Differences in host genotypes that affect the carbohydrate landscape altered the intestinal bacterial composition in a diet-dependent manner (Kashyap et al., 2013). ob/ob gene mutant caused a major change to the mouse gut microbiota composition than dietary composition (Kashani et al., 2019). Zhang et al. (2010) assessed the relative contributions of host genetics and diets in shaping the intestinal microbiota in mice and indicated that diet could explain 57% of the total structure variation in microbiota, whereas genetic mutation explained for <12%, but the contribution of intestinal microbiota in diet or genotype induced obesity remains unclear. In the present study, we compared the intestinal bacteria in different diet treatments and gene-knockout zebrafish, and we found high-fat diet had a more dominant role in shaping microbiota community to increase the lipid accumulation relative to cpt1b and pparab knockout, and the follow-up microbiota transplant experiment also confirmed this conclusion. These results suggested that high-fat-diet induced lipid accumulation dependent on the intestinal microbiota, whereas two gene-knockout zebrafish accumulated more lipid mainly by inhibiting lipid catabolism instead of the alteration of intestinal microbiota.
The characteristics of intestinal microbiota in the different types of obesity have been addressed; for example, the decrease of microbial diversity is related to the development of obesity in mammals (Walters et al., 2014). In the present study, active microbial analyses indicated that HF diet had remarkably decreased microbial diversity, but the knockout of cpt1b and pparab caused no significant difference in microbial diversity, revealing lower bacterial diversity was more prone to exist in a high-fat diet that induced lipid accumulation. Another characteristic related to obesity is the increased ratio of Firmicutes to Bacteroidetes in mammals (Ley et al., 2006), although different views exist (Schwiertz et al., 2010). In the present study, no increase of Firmicutes/Bacteroidetes ratio was observed in zebrafish with diet-induced or genotype-induced lipid accumulation based on 16S rRNA gene sequencing. However, 16S rRNA sequencing indicated that HF diet had reduced Firmicutes/Bacteroidetes ratio, suggesting different sequencing methods also influenced the results. Furthermore, we also observed that the abundance of Proteobacteria was significantly declined in the HF group compared with the control group according to both total and active microbial sequencing. Proteobacteria is the dominant member in the intestine of numerous aquatic organisms, and it is one of the most responsive groups when the host health is affected by environmental factors such as diet (Navarro-Barron et al., 2019). These results suggested that decreased bacterial diversity and alteration of some responsive bacterial groups to the diet component might be related to diet-induced obesity.
A recent study found nuclear factor-kB/Relish was vital to adapt gut microbiota species composition to host diet macronutrient composition, suggesting the importance of host genetic factors and diet components in shaping microbiota diversity (Vandehoef et al., 2020). The fecal bacteria of Fut2– mice that lack fucosylated host glycans showed decreased alpha diversity relative to Fut2+ mice and exhibited significant differences in community composition (Kashyap et al., 2013). The present study showed the intestinal bacteria of cpt1b–/– or pparab–/– zebrafish exhibit no significant difference relative to WT zebrafish. These results suggested that different genes played different roles in shaping the intestinal microbiota composition and the response of different bacteria to the environmental or genetic factors also varied. More gene manipulation models should be conducted to reveal the influence of host genetics on intestinal microbiota and host metabolism.
The correlation analyses based on DNA level suggested Enhydrobacter, Paracoccus, Pseudomonas, and Dechloromonas (p < 0.05, Spearman’s rank correlation) were related to lipid accumulation, and among them, Enhydrobacter was proved to be able to ferment glucose and carbohydrates in the intestines of shrimp and crabs (Premalatha et al., 2015), but the function of the other three bacterial genera in nutrients metabolism is unknown. The correlation analyses based on 16S rRNA sequencing showed that Bacteroides and Prevotella negatively related to TG in the liver and weight gain. It was found that Bacteroides was negatively correlated with body weight in mice (Sidiropoulos et al., 2020), and the abundance of Prevotellaceae was negatively related to a high-fat diet in pigs and humans (Zhang C. et al., 2019; Zhou et al., 2019). Additionally, Bifidobacterium and Faecalibacterium were negatively related to TG in the liver. These results suggested that the relationship of Bacteroides, Prevotella, Bifidobacterium, and Faecalibacterium to lipid accumulation was conserved among different animals. Cetobacteria was positively correlated with lipid accumulation or higher weight phenotype. It has been found that Cetobacteria is the producer of acetic acid from peptones or carbohydrates in the fish gut (Qin et al., 2017; Siriyappagouder et al., 2018), which may account for harvesting more energy from the diet.
Amplicon sequencing methods targeting the 16S rRNA gene and 16S rRNA have been extensively applied to investigate the microbial community composition and dynamics in multiple places, including anaerobic digestion (De Vrieze et al., 2018), the rumen of beef cattle (Li F. et al., 2019), a coastal microbial mat (Cardoso et al., 2017), or soils (Bastida et al., 2017; Li H. et al., 2019). Although some differences exist between the total and the active microbial communities, active microbial communities are more sensitive to address community compositions and microbial function predictions (Li F. et al., 2019; Li H. et al., 2019). The present study showed that 16S rRNA-based sequencing result was more consistent with the intestinal bacteria transplantation, suggesting multiple sequencing platform and bacterial transplantation was indispensable for identifying the bacterial function. Bacteria transplantation combined with Nile red staining was used to show the lipid accumulation in zebrafish, but due to the small size of zebrafish (10 dpf), the lack of physiological and biochemical indexes was the limitation of the present study.
In summary, we found that the intestinal microbiota composition shaped by a high-fat diet played a more important role in lipid accumulation compared with those of cpt1b–/– and pparab–/–. 16S rRNA sequencing provided more consistent and accurate information in reflecting the function of the intestinal microbiome relative to 16S rRNA gene sequencing. The interactions among the host genotypes, intestinal microbiota, and diet components should be considered when we identify the development of obesity and its related metabolic syndrome.
Microbiota sequencing data was submitted to the National Center for Biotechnology Information (NCBI) with the accession number PRJNA632454.
This study was approved by the Committee on the Ethics of Animal Experiments of East China Normal University (No. F20190101).
M-LZ and Z-YD conceived the study, designed the experiments, and revised the manuscript. FQ, FT, and L-YL performed the experiments and analyzed the data. L-YL generated the cpt1b knockout zebrafish. L-YL and H-BL generated the pparab knockout zebrafish. LQC provided the essential materials and technical support. M-LZ and FT wrote the manuscript. All authors contributed experimental assistance, intellectual input to this study, and read and approved the final manuscript.
This work was supported by the National Key R&D program (Grant Number 2018YFD0900400) and the National Natural Science Foundation of China (Grant Number 31972798).
The authors declare that the research was conducted in the absence of any commercial or financial relationships that could be construed as a potential conflict of interest.
All claims expressed in this article are solely those of the authors and do not necessarily represent those of their affiliated organizations, or those of the publisher, the editors and the reviewers. Any product that may be evaluated in this article, or claim that may be made by its manufacturer, is not guaranteed or endorsed by the publisher.
The Supplementary Material for this article can be found online at: https://www.frontiersin.org/articles/10.3389/fmicb.2021.741616/full#supplementary-material
Araujo, J. R., Tazi, A., Burlen-Defranoux, O., Vichier-Guerre, S., Nigro, G., Licandro, H., et al. (2020). Fermentation products of commensal bacteria alter enterocyte lipid metabolism. Cell Host Microbe 27, 358–375 e357. doi: 10.1016/j.chom.2020.01.028
Bäckhed, F., Ding, H., Wang, T., Hooper, L. V., Koh, G. Y., Nagy, A., et al. (2004). The gut microbiota as an environmental factor that regulates fat storage. Proc. Natl. Acad. Sci. U.S.A. 101, 15718–15723.
Bastida, F., Torres, I. F., Andres-Abellan, M., Baldrian, P., Lopez-Mondejar, R., Vetrovsky, T., et al. (2017). Differential sensitivity of total and active soil microbial communities to drought and forest management. Glob. Change Biol. 23, 4185–4203. doi: 10.1111/gcb.13790
Bligh, E. G., and Dyer, W. J. (1959). A rapid method of total lipid extaction and purification. Can. J. Biochem. Physiol. 37, 911–917.
Brial, F., Le Lay, A., Dumas, M. E., and Gauguier, D. (2018). Implication of gut microbiota metabolites in cardiovascular and metabolic diseases. Cell. Mol. Life Sci. 75, 3977–3990. doi: 10.1007/s00018-018-2901-1
Cardoso, D. C., Sandionigi, A., Cretoiu, M. S., Casiraghi, M., Stal, L., and Bolhuis, H. (2017). Comparison of the active and resident community of a coastal microbial mat. Sci. Rep. 7:10. doi: 10.1038/s41598-017-03095-z
Chassaing, B., Koren, O., Goodrich, J. K., Poole, A. C., Srinivasan, S., Ley, R. E., et al. (2015). Dietary emulsifiers impact the mouse gut microbiota promoting colitis and metabolic syndrome. Nature 519, 92–96. doi: 10.1038/nature14232
De Vrieze, J., Pinto, A. J., Sloan, W. T., and Ijaz, U. Z. (2018). The active microbial community more accurately reflects the anaerobic digestion process: 16S rRNA (gene) sequencing as a predictive tool. Microbiome 6:63. doi: 10.1186/s40168-018-0449-9
Faillaci, F., Milosa, F., Critelli, R. M., Turola, E., Schepis, F., and Villa, E. (2018). Obese zebrafish: a small fish for a major human health condition. Anim. Model Exp. Med. 1, 255–265. doi: 10.1002/ame2.12042
Franchi, O., Bovio, P., Ortega-Martínez, E., Rosenkranz, F., and Chamy, R. (2018). Active and total microbial community dynamics and the role of functional genes bamA and mcrA during anaerobic digestion of phenol and p-cresol. Bioresour. Technol. 264, 290–297.
Goodarzi, M. O. (2018). Genetics of obesity: what genetic association studies have taught us about the biology of obesity and its complications. Lancet Diabetes Endocrinol. 6, 223–236. doi: 10.1016/s2213-8587(17)30200-0
Indiani, C., Rizzardi, K. F., Castelo, P. M., Ferraz, L. F. C., Darrieux, M., and Parisotto, T. M. (2018). Childhood obesity and firmicutes/bacteroidetes ratio in the gut microbiota: a systematic review. Child. Obes. 14, 501–509. doi: 10.1089/chi.2018.0040
Jia, W., Li, H., Zhao, L., and Nicholson, J. K. (2008). Gut microbiota: a potential new territory for drug targeting. Nat. Rev. Drug Discov. 7, 123–129.
Kashani, A., Brejnrod, D., Jin, C., Kern, T., Madsen, A. N., Holm, L. A., et al. (2019). Impaired glucose metabolism and altered gut microbiome despite calorie restriction of ob/ob mice. Anim. Microb. 1:11. doi: 10.1186/s42523-019-0007-1
Kashyap, P. C., Marcobal, A., Ursell, L. K., Smits, S. A., Sonnenburg, E. D., Costello, E. K., et al. (2013). Genetically dictated change in host mucus carbohydrate landscape exerts a diet-dependent effect on the gut microbiota. Proc. Natl. Acad. Sci.U.S.A. 110, 17059–17064. doi: 10.1073/pnas.1306070110
Kreznar, J. H., Keller, M. P., Traeger, L. L., Rabaglia, M. E., Schueler, K. L., Stapleton, D. S., et al. (2017). Host genotype and gut microbiome modulate insulin secretion and diet-induced metabolic phenotypes. Cell Rep. 18, 1739–1750. doi: 10.1016/j.celrep.2017.01.062
Landgraf, K., Schuster, S., Meusel, A., Garten, A., Riemer, T., Schleinitz, D., et al. (2017). Short-term overfeeding of zebrafish with normal or high-fat diet as a model for the development of metabolically healthy versus unhealthy obesity. BMC Physiol. 17:4. doi: 10.1186/s12899-017-0031-x
Ley, R. E., Peterson, D. A., and Gordon, J. I. (2006). Ecological and evolutionary forces shaping microbial diversity in the human intestine. Cell 124, 837–848. doi: 10.1016/j.cell.2006.02.017
Li, F., Hitch, T. C. A., Chen, Y., Creevey, C. J., and Guan, L. L. (2019). Comparative metagenomic and metatranscriptomic analyses reveal the breed effect on the rumen microbiome and its associations with feed efficiency in beef cattle. Microbiome 7:6. doi: 10.1186/s40168-019-0618-5
Li, H., Su, J. Q., Yang, X. R., and Zhu, Y. G. (2019). Distinct rhizosphere effect on active and total bacterial communities in paddy soils. Sci. Total Environ. 649, 422–430. doi: 10.1016/j.scitotenv.2018.08.373
Li, L. Y., Li, J. M., Ning, L. J., Lu, D. L., Luo, Y., Ma, Q., et al. (2020). Mitochondrial fatty acid β-oxidation inhibition promotes glucose utilization and protein deposition through energy homeostasis remodeling in fish. J. Nutr. 150, 2322–2335. doi: 10.1093/jn/nxaa187
Li, L. Y., Limbu, S. M., Ma, Q., Chen, L. Q., Zhang, M. L., and Du, Z. Y. (2018). The metabolic regulation of dietary L-carnitine in aquaculture nutrition: present status and future research strategies. Rev. Aquacult. 11, 1228–1257.
Lu, D. L., Ma, Q., Wang, J., Li, L. Y., Han, S. L., Limbu, S. M., et al. (2019). Fasting enhances cold resistance in fish through stimulating lipid catabolism and autophagy. J. Physiol. 597, 1585–1603. doi: 10.1113/jp277091
Lu, H. F., Ren, Z. G., Li, A., Zhang, H., Xu, S. Y., Jiang, J. W., et al. (2019). Fecal microbiome data distinguish liver recipients with normal and abnormal liver function from healthy controls. Front. Microbiol. 10:1518. doi: 10.3389/fmicb.2019.01518
Madureira, T. V., Pinheiro, I., de Paula Freire, R., Rocha, E., Castro, L. F., and Urbatzka, R. (2017). Genome specific PPARalphaB duplicates in salmonids and insights into estrogenic regulation in brown trout. Comp. Biochem. Physiol. B Biochem. Mol. Biol. 208-209, 94–101. doi: 10.1016/j.cbpb.2017.04.005
McMurdie, P. J., and Holmes, S. (2013). phyloseq: an R package for reproducible interactive analysis and graphics of microbiome census data. PLoS One 8:e61217. doi: 10.1371/journal.pone.0061217
Moen, A. E. F., Lindstrom, J. C., Tannaes, T. M., Vatn, S., Ricanek, P., Vatn, M. H., et al. (2018). The prevalence and transcriptional activity of the mucosal microbiota of ulcerative colitis patients. Sci. Rep. 8:17278. doi: 10.1038/s41598-018-35243-4
Navarro-Barron, E., Hernandez, C., Llera-Herrera, R., Garcia-Gasca, A., and Gomez-Gil, B. (2019). Overfeeding a high-fat diet promotes sex-specific alterations on the gut microbiota of the zebrafish (Danio rerio). Zebrafish 16, 268–279. doi: 10.1089/zeb.2018.1648
Oksanen, J., Blanchet, F. G., Kindt, R., Legendre, P., Minchin, P. R., O’Hara, R. B., et al. (2014). Vegan: Community Ecology Package. R Package Version 2.2-0. Available online at: http://CRAN.Rproject.org/package=vegan
Pham, L. N., Kanther, M., Semova, I., and Rawls, J. F. (2008). Methods for generating and colonizing gnotobiotic zebrafish. Nat. Protocols 3, 1862–1875. doi: 10.1038/nprot.2008.186
Premalatha, N., Gopal, N. O., Jose, P. A., Anandham, R., and Kwon, S. W. (2015). Optimization of cellulase production by Enhydrobacter sp. ACCA2 and its application in biomass saccharification. Front. Microbiol. 6:1046.
Qin, C., Zhang, Z., Wang, Y., Li, S., Ran, C., Hu, J., et al. (2017). EPSP of L. casei BL23 protected against the infection caused by aeromonas veronii via enhancement of immune response in zebrafish. Front. Microbiol. 8:2406. doi: 10.3389/fmicb.2017.02406
Schwiertz, A., Taras, D., Schafer, K., Beijer, S., Bos, N. A., Donus, C., et al. (2010). Microbiota and SCFA in lean and overweight healthy subjects. Obesity (Silver Spring) 18, 190–195. doi: 10.1038/oby.2009.167
Semova, I., Carten, J. D., Stombaugh, J., Mackey, L. C., Kningt, R., Farber, S. T., et al. (2012). Microbiota regulate intestinal absorption and metabolism of fatty acids in the zebrafish. Cell Host Microbe. 12, 277–288. doi: 10.1016/j.chom.2012.08.003
Sidiropoulos, D. N., Al-Ghalith, G. A., Shields-Cutler, R. R., Ward, T. L., Johnson, A. J., Vangay, P., et al. (2020). Wild primate microbiomes prevent weight gain in germ-free mice. Anim. Microbiome 2:16. doi: 10.1186/s42523-020-00033-9
Singh, A., Zapata, R. C., Pezeshki, A., Workentine, M. L., and Chelikani, P. K. (2019). Host genetics and diet composition interact to modulate gut microbiota and predisposition to metabolic syndrome in spontaneously hypertensive stroke-prone rats. FASEB J. 33, 6748–6766.
Siriyappagouder, P., Galindo-Villegas, J., Lokesh, J., Mulero, V., Fernandes, J. M. O., and Kiron, V. (2018). Exposure to yeast shapes the intestinal bacterial community assembly in zebrafish larvae. Front. Microbiol. 9:1868. doi: 10.3389/fmicb.2018.01868
Tan, F., Limbu, S. M., Qian, Y., Qiao, F., Du, Z.-Y., and Zhang, M. (2019). The responses of germ-free zebrafish (Danio rerio) to varying bacterial concentrations, colonization time points, and exposure duration. Front. Microbiol. 10:2156. doi: 10.3389/fmicb.2019.02156
Turnbaugh, P. J., Backhed, F., Fulton, L., and Gordon, J. I. (2008). Diet-induced obesity is linked to marked but reversible alterations in the mouse distal gut microbiome. Cell Host Microbe 3, 213–223. doi: 10.1016/j.chom.2008.02.015
Ussar, S., Fujisaka, S., and Kahn, C. R. (2016). Interactions between host genetics and gut microbiome in diabetes and metabolic syndrome. Mol. Metab. 5, 795–803. doi: 10.1016/j.molmet.2016.07.004
Vandehoef, C., Molaei, M., and Karpac, J. (2020). Dietary adaptation of microbiota in drosophila requires NF-κB-dependent control of the translational regulator 4E-BP. Cell Rep. 31:107736. doi: 10.1016/j.celrep.2020.107736
Walters, W. A., Xu, Z., and Knight, R. (2014). Meta-analyses of human gut microbes associated with obesity and IBD. FEBS Lett. 588, 4223–4233. doi: 10.1016/j.febslet.2014.09.039
Zhang, C., Jing, Y., Zhang, W., Zhang, J., Yang, M., Du, L., et al. (2019). Dysbiosis of gut microbiota is associated with serum lipid profiles in male patients with chronic traumatic cervical spinal cord injury. Am. J. Transl. Res. 11, 4817–4834.
Zhang, C., Zhang, M., Wang, S., Han, R., Cao, Y., Hua, W., et al. (2010). Interactions between gut microbiota, host genetics and diet relevant to development of metabolic syndromes in mice. ISME J. 4, 232–241. doi: 10.1038/ismej.2009.112
Zhang, M. L., Li, M., Sheng, Y., Tan, F., Chen, L., Cann, I., et al. (2020). Citrobacter species increase energy harvest by modulating intestinal microbiota in fish: nondominant species play important functions. mSystems 5:e00303–20. doi: 10.1128/mSystems.00303-20
Zhang, Z., Ran, C., Ding, Q. W., Liu, H. L., Xie, M. X., Yang, Y. L., et al. (2019). Ability of prebiotic polysaccharides to activate a HIF1alpha-antimicrobial peptide axis determines liver injury risk in zebrafish. Commun. Biol. 2:274. doi: 10.1038/s42003-019-0526-z
Keywords: gut microbiota, lipid accumulation, diet, host genetics, 16S rRNA sequencing, obesity
Citation: Qiao F, Tan F, Li L-Y, Lv H-B, Chen L, Du Z-Y and Zhang M-L (2021) Alteration and the Function of Intestinal Microbiota in High-Fat-Diet- or Genetics-Induced Lipid Accumulation. Front. Microbiol. 12:741616. doi: 10.3389/fmicb.2021.741616
Received: 15 July 2021; Accepted: 18 August 2021;
Published: 17 September 2021.
Edited by:
Klibs N. Galvao, University of Florida, United StatesReviewed by:
Chenyang Lu, Ningbo University, ChinaCopyright © 2021 Qiao, Tan, Li, Lv, Chen, Du and Zhang. This is an open-access article distributed under the terms of the Creative Commons Attribution License (CC BY). The use, distribution or reproduction in other forums is permitted, provided the original author(s) and the copyright owner(s) are credited and that the original publication in this journal is cited, in accordance with accepted academic practice. No use, distribution or reproduction is permitted which does not comply with these terms.
*Correspondence: Mei-Ling Zhang, bWx6aGFuZ0BiaW8uZWNudS5lZHUuY24=; Zhen-Yu Du, enlkdUBiaW8uZWNudS5lZHUuY24=
†These authors have contributed equally to this work
Disclaimer: All claims expressed in this article are solely those of the authors and do not necessarily represent those of their affiliated organizations, or those of the publisher, the editors and the reviewers. Any product that may be evaluated in this article or claim that may be made by its manufacturer is not guaranteed or endorsed by the publisher.
Research integrity at Frontiers
Learn more about the work of our research integrity team to safeguard the quality of each article we publish.