- 1Interfaculty Bioinformatics Unit and Swiss Institute of Bioinformatics, University of Bern, Bern, Switzerland
- 2Agroscope, Bern, Switzerland
- 3Institute of Veterinary Bacteriology, University of Bern, Bern, Switzerland
The bacterium Morganella morganii can produce the biogenic amines (BA) cadaverine, putrescine, and histamine in vitro and is responsible for high histamine concentrations in fish products. These BA can have toxic effects upon ingestion and are undesired in food. The purpose of this study was to characterize the phenotype and genotype of 11 M. morganii isolated from cheese in regard to the BA formation. In addition, we investigated the phylogeny, trehalose fermentation ability, and antibiotic resistance of the cheese isolates. To do so, we sequenced their genomes using both long and short read technologies. Due to the presence of the trehalose operon and the ability to ferment trehalose, the cheese isolates can be assigned to the subsp. sibonii. Comparative genomics with public available M. morganii genomes shows that the genomes of the cheese isolates cluster together with other subsp. sibonii genomes. All genomes between subsp. morganii and subsp. sibonii are separated by an average nucleotide identity (ANI) of less than 95.0%. Therefore, the subspecies could represent two distinct species. Nine of the strains decarboxylated lysine yielding cadaverine in vitro. This metabolic activity is linked to a previously unknown gene cluster comprising genes encoding a lysine-tRNA ligase (lysS), an HTH-transcriptional regulator (argP), a cadaverine-lysine antiporter (cadB), and a lysine decarboxylase (cadA). The formation of putrescine is linked to the speF gene encoding an ornithine decarboxylase. The gene is disrupted in five strains by an insertion sequence, and these strains only exhibit a weak putrescine production. Antimicrobial susceptibility profiling revealed that all cheese strains are resistant to tetracycline, chloramphenicol, tigecycline, colistin, and ampicillin. These phenotypes, except for colistin which is intrinsic, could be linked to antimicrobial resistance genes located on the chromosome.
Introduction
Morganella morganii (formerly Proteus morganii) is a facultative anaerobic Gram-negative bacterium (Janda and Abbott, 2015). The bacterium is divided into two subspecies (Jensen et al., 1992). Trehalose fermenting strains are designated as M. morganii subsp. sibonii. The strains unable to ferment trehalose are named M. morganii subsp. morganii. The bacterium is present in the environment and the gastrointestinal tract of mammals and reptiles (Janda and Abbott, 2015). M. morganii was also found in fish products, where it produces the biogenic amine (BA) histamine (Kim et al., 2003; Kanki et al., 2007). Histamine can provoke allergic-like reactions upon consumption and is therefore undesired in food (Linares et al., 2011). In addition, various strains can decarboxylate ornithine (ODC+) and/or lysine (LDC+) to form the BA putrescine and cadaverine, respectively (Janda et al., 1996). Because cadaverine and putrescine enhance the toxicity of histamine, they are also undesired in food (Linares et al., 2011). M. morganii was also detected in cheese and the biogenic amine formation of this species could therefore have an impact on the cheese quality (Amato et al., 2012; Coton et al., 2012). The gene encoding the histidine decarboxylase of M. morganii was cloned and characterized by Kanki et al. (2007). Furthermore, de las Rivas et al. (2007, 2008) cloned two genes encoding ornithine decarboxylases and showed that the gene products decarboxylate ornithine. In contrast, the genetic elements responsible for cadaverine formation in M. morganii have not been described.
Foodborne bacteria with antibiotic resistances are undesired in cheese because they have the potential to disseminate their resistances to human pathogenic bacteria (Huddleston, 2014). M. morganii possesses intrinsic resistance to oxacillin, ampicillin, amoxicillin, most of the first- and second-generation cephalosporins, macrolides, lincosamides, glycopeptides, fosfomycin, fusidic acid, and colistin (Liu et al., 2016). The bacterium is currently gaining more and more attention because isolates with acquired antibiotic resistances emerge (Liu et al., 2016).
With this study, we aim to gain knowledge about M. morganii isolated from dairy products. Eleven strains were isolated from cheese and sequenced using both long and short read technologies. We could link phenotypic traits regarding biogenic amine formation, trehalose fermentation and antibiotic resistances to the genomic data. In addition, we examined the phylogenetic relationships of the cheese isolates with publicly available Morganella genomes.
Materials and Methods
Bacterial Strains and Cultivation
In a routine analysis, we isolated 11 M. morganii strains as follows: 10 grams of cheese were homogenized in 90 mL of 40°C warm peptone water (10 g L–1 peptone from casein, 5 g L–1 sodium chloride, 20 g L–1 trisodium citrate dihydrate, pH 7.0) for 3 min using a stomacher (Masticator, IUL instruments GmbH, Königswinter, Germany). We plated serial dilutions of the homogenate either on ECC agar (CHROMagar, Paris, France) or on agar prepared using the protocol of improved decarboxylase medium containing 10 g L–1 of L-lysine (Bover-Cid and Holzapfel, 1999). After an incubation at 37°C overnight, we determined the bacterial species of individual colonies on the agar plates using a MALDI Biotyper instrument (Bruker Daltonics GmbH, Bremen, Germany).
We obtained the type strain of M. morganii subsp. morganii DSM 30164 from the German Collection of Microorganisms and Cell Cultures GmbH (Braunschweig, Germany).
The M. morganii strains (Table 1) were stored at −80°C in Trypticase Soy Broth (TSB) (BD, Dr. Grogg Chemie AG, Stettlen-Deisswil, Switzerland) containing 30% (v/v) glycerol and were cultivated in TSB at 37°C for 20 h under aerobic conditions.
We used API 20E strips (Biomérieux, Geneva, Switzerland) to determine the capability to ferment trehalose. To assess if the M. morganii cheese isolates show hemolytic activity, we streaked them on Trypticase Soy agar plates containing 5% sheep blood (bioMérieux). The plates were incubated at 37°C up to 3 days and inspected for lysed red blood cells.
Library Preparation and Whole Genome Sequencing
We sequenced 11 M. morganii cheese isolates (Table 1) and the M. morganii subsp. morganii type strain DSM 30164 using Oxford Nanopore Technologies (ONT, Oxford, United Kingdom) and Ion Torrent (Thermo Fisher Scientific, Baar, Switzerland). The strain DSM 30164 was included as a control.
For library preparation, we collected the bacteria of 10 mL culture in TSB by centrifugation and extracted the DNA from the pellet using the EZ1 DNA Tissue Kit on the BioRobot EZ1 (Qiagen, Basel, Switzerland) according to the manufacturer’s instructions. The DNA concentration was determined using the Qubit DNA assay kit (Thermo Fisher Scientific).
We performed the Nanopore long read sequencing as follows: 4 μg of DNA were sheared using Covaris g-TUBES. The DNA fragments were used for the library preparation using the ligation sequencing kit SQK-LSK109 (ONT) and native barcoding expansion 1–12 EXP-NBD104 (ONT). We sequenced the libraries with a Spot-ON Flow Cell (FLO-MIN110, R10) on a MinION sequencer (ONT).
For Ion Torrent sequencing we used the Ion Xpress Plus Fragment Library Kit (Thermo Fisher Scientific) to prepare bar-coded libraries out of 1 μg of DNA according to the manufacturer’s instructions. The DNA libraries were size-selected for 400-bp fragments using E-Gel SizeSelect II Agarose Gels, 2% (Thermo Fisher Scientific). The libraries were pooled and sequenced on an Ion S5 System using Ion 530 Chips (Thermo Fisher Scientific) according to the manufacturer’s protocols.
Genome Assembly
We performed the base calling and demultiplexing of the long reads using Guppy basecaller (v3.2.4; “–config dna_r10_450bps_fast.cfg –cpu_threads_per_caller 4 –num_callers 2 –trim_strategy dna –trim_barcodes –barcode_kits EXP-NBD104’’).1 Afterward, we assembled the long reads using flye (v2.3.4; –“nano-raw, –genome-size 4 m”) (Kolmogorov et al., 2019) and corrected the consensus sequence of the draft genome with long reads using minimap2 (v2.17; “-ax map-ont”) (Li, 2018), racon (v1.3.1; default) (Vaser et al., 2017) and medaka (v0.10.0; ‘‘medaka_consensus’’).2 Base calling of short reads was performed with the Ion Torrent Suite software 5.4 (Thermo Fisher Scientific) using standard settings. We used Trimmomatic (v0.36; “CROP: 350, HEADCROP: 30, MINLEN:10”) for a quality trimming of the reads (Bolger et al., 2014). To find plasmids which were not assembled by flye, we reconstructed additional plasmids from the Ion Torrent reads with PlasmidSPAdes (v3.12.0; default) (Antipov et al., 2016). We further polished the consensus sequence of the assembly using the short reads and the tools bowtie 2 (v2.3.4.1; default) (Langmead and Salzberg, 2012) and pilon (v1.22; “–unpaired, -vcf, –changes, –tracks”) (Walker et al., 2014). We uploaded the genomes to the GenBank database where they were annotated by the NCBI prokaryotic genome annotation pipeline (Tatusova et al., 2016). GenBank accession numbers of the whole genome sequences are listed in Table 1. The average GC-content was calculated using Quast (v4.6.0; default) (Gurevich et al., 2013). For bioinformatics analyses, we retrieved 88 M. morganii and 4 Morganella psychrotolerans genome sequences from the GenBank database (November 2020, excluding assemblies with the status “anomalous” and “genome length too small,” Supplementary Table 1). Insertion sequences (IS) of interest were classified using ISfinder (Siguier et al., 2006).
Calculation of Average Nucleotide Identity
We calculated the pairwise whole genome average nucleotide identity (ANI) of the 104 above mentioned Morganella spp. genomes with fastANI (v1.32; default) (Jain et al., 2018). Heat maps were generated using the python seaborn package.3
Construction of Phylogenetic Tree
We constructed a phylogenetic tree based on core genes to investigate the relationship of the cheese isolates, DSM 30164 and the 88 above mentioned M. morganii genomes deposited in the GenBank database (Supplementary Table 1, November 2020). The four M. psychrotolerans genomes served as outgroup and were included in the selection of the core genes. M. psychrotolerans is the most closely related species of M. morganii (Emborg et al., 2006). The core genes were defined and aligned with Roary (v3.13.0; “-e –mafft”) (Page et al., 2015). To avoid differences in gene prediction caused by different annotation tools, we re-annotated all 104 strains (including cheese isolates) using Prokka (v1.14.6; “-mincontiglen 200, -rfam, –addgenes”) (Seemann, 2014). The phylogenetic relationship of the alignments was inferred based on maximum likelihood using RAxML including 500 bootstrap analysis (v8.2.12; “-f a -# 500 –m GTRGAMMA”) (Stamatakis, 2014) and visualized with the ETE3 Toolkit (v3.1.1) (Huerta-Cepas et al., 2016). Branch lengths were ignored and branches with a bootstrap value below 70% were collapsed.
Determination of Cadaverine and Putrescine Formation in vitro
To determine the capability to produce cadaverine and putrescine, we incubated the cheese strains in IDM broth under aerobic conditions (adapted from Bover-Cid and Holzapfel, 1999; without the addition of bromocresol purple and agar) at 37°C for 20 h. The broth was supplemented with 10 g L–1 L-lysine and 10 g L–1 L-ornithine (Merck, Darmstadt, Germany). A non-inoculated medium was used as a negative control and standard solutions of cadaverine (Sigma-Aldrich, Dr. Grogg Chemie AG, Stettlen-Deisswil, Switzerland) and putrescine (Merck, Darmstadt, Germany) served as positive controls. The cell suspension was centrifuged at room temperature with 4,000 g for 5 min.
We determined the content of biogenic amines using high-pressure liquid chromatography (HPLC). Therefore, we mixed 500 μL of the culture supernatant with 100 μL of 20 mM 1,7-diaminoheptane which served as internal standard. Then, 5 mL of extraction solution (0.1 M perchloric acid in 50% acetonitrile) was added. The mixture was centrifuged (890 × g for 10 min at 10°C). A 200 μL volume of the supernatant was then treated with dansyl chloride and analyzed using HPLC as described in Ascone et al. (2017).
Determination of Antimicrobial Susceptibility
We determined the antimicrobial susceptibility by measuring the minimal inhibitory concentration (MIC) in cation-adjusted Müller-Hinton broth using Sensititre® susceptibility MIC plate EUVSEC (Thermo Fisher Scientific). The following antibiotics were tested: sulfamethoxazole (SMX), trimethoprim (TMP), ciprofloxacin (CFX), tetracycline (TET), meropenem (MERO), azithromycin (ATM), nalidixic acid (NLX), cefotaxime (CTX), chloramphenicol (CHL), tigecycline (TGC), ceftazidime (CEF), colistin (COL), ampicillin (AMP), and gentamicin (GEN). We evaluated the sensitivity according to EUCAST (AMP, CEF, CTX, MERO, CFX, GEN, TGC, CHL, COL, TMP) (The European Committee on Antimicrobial Susceptibility Testing. Breakpoint tables for interpretation of MICs and zone diameters. Version 11.0, 2021)4 and Clinical and Laboratory Standards Institute (NLX, TET, SMX) (CLSI, 2020) breakpoint tables for Enterobacterales. We searched antibiotic resistance genes in the cheese isolate genomes with Abricate (v1.0.1; “–minid 80, --mincov 80’’)5 using the CARD database (v3.0.8) (Jia et al., 2017).
Results
Isolation, Identification, and Genome Sequencing
During routine analyses of various cheeses for the presence of E. coli and other coliforms using chromogenic media, individual colonies were identified as M. morganii using the MALDI Biotyper (data not shown).
We sequenced the genomes of 11 cheese isolates and the type strain M. morganii subsp. morganii DSM 30164 using both short read and long read sequencing technologies. This mix of sequencing technologies allowed us to assemble complete chromosomes and plasmids. The chromosome size of the cheese isolates is between 4.0 and 4.3 Mb, whereas the genome size of the DSM 30164 is 3.8 Mb (Table 1). The GC-content ranges from 50.16 to 50.45%, whereas the genome of DSM 30164 has a GC-content of 51.16% (Table 1).
Trehalose Fermentation
The capability to ferment trehalose is used as the criterion to classify M. morganii into subsp. sibonii (trehalose fermenter) and subsp. morganii (no trehalose fermenter) (Jensen et al., 1992). This phenotype is also linked to the presence of the trehalose operon (treR, treB, and treP) (Palmieri et al., 2020). This operon is present in the genome of all cheese isolates. All cheese isolates except FAM24678 fermented trehalose in the API assay. The treB gene of FAM24678 is disrupted by an IS5 family element, which likely explains the trehalose-negative phenotype of this strain (Figure 1). We identified the trehalose operon also in all other seven genomes of cluster III and in the genomes of the strains GCSL-TSO-24, H1r (both cluster II), and MMSCG (cluster IV) (see section “phylogenetic relationship”). The locus is absent in all genomes from cluster I and in the genomes of the M. psychrotolerans strains.
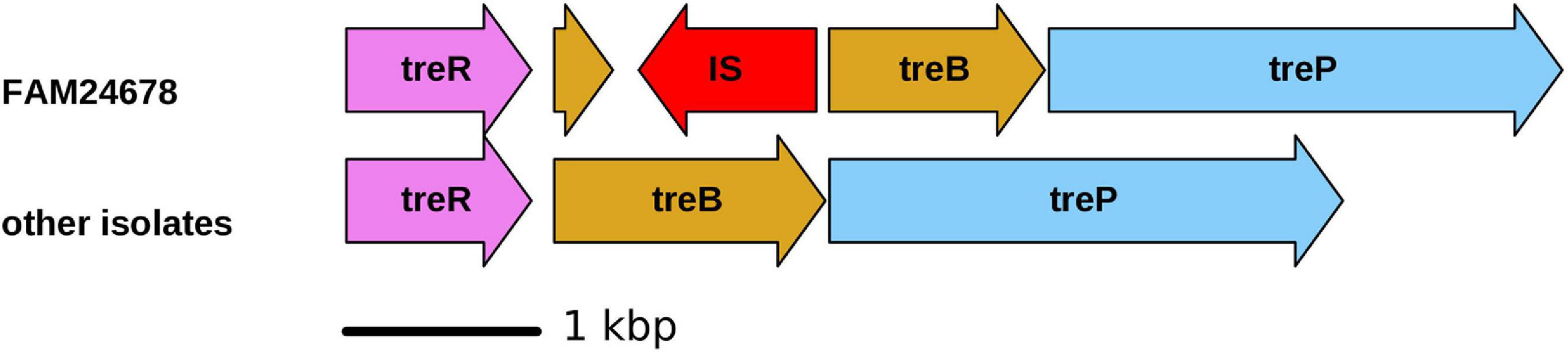
Figure 1. Schematic illustration of the trehalose operon of FAM24678 (not fermenting trehalose) and the other 10 cheese isolates (fermenting trehalose). TreR, HTH-type transcriptional regulator; treB, PTS system trehalose-specific EIIBC component; treP, trehalose 6-phosphate phosphorylase; IS, insertion sequence.
Phylogenetic Relationship
The ability of the cheese isolates to ferment trehalose and the presence of the trehalose operon suggest that they belong to the subsp. sibonii. To gain a detailed insight into the phylogenetic relationship of the cheese isolates, DSM 30164 and 92 Morganella genomes from GenBank, we generated a phylogenetic tree based on 559 core genes using maximum likelihood (Figure 2).
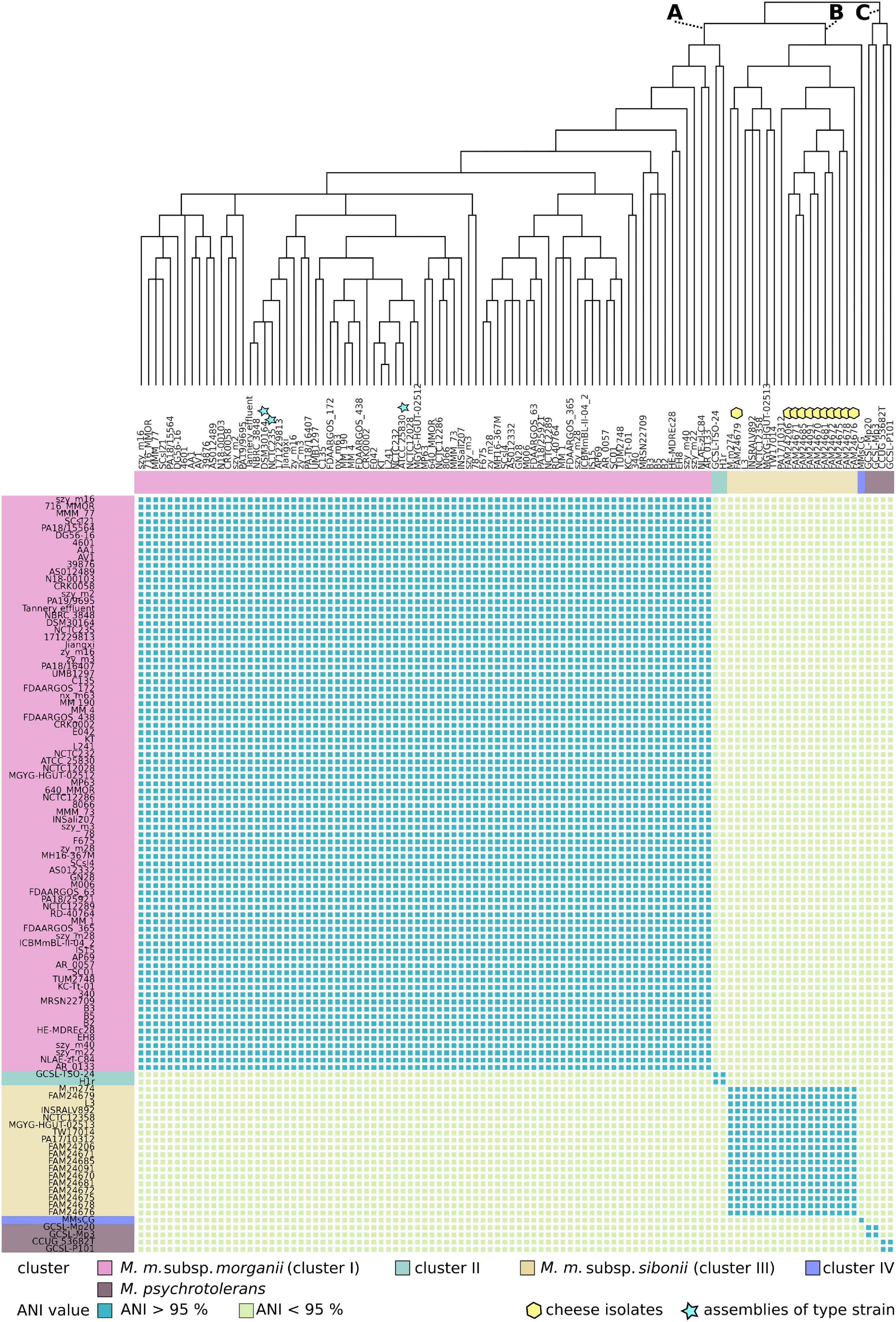
Figure 2. The upper part of the figure displays a phylogenetic core gene tree of 100 M. morganii (M. m.) and four M. psychrotolerans genomes. The tree was inferred based on maximum likelihood and branches with bootstrap values below 70% were collapsed. A, B, and C mark the three main branches of the tree. The heat map in the lower part of the figure shows the pairwise whole genome average nucleotide identity (ANI) values. An ANI value cut-off of 95% separates the M. morganii genomes into the clusters I, II, III, and IV.
The tree branches out into three main groups (A, B, and C in Figure 2). All strains of M. psychrotolerans cluster together on branch C, whereas the strains of M. morganii are located either on branch A or B. The cheese isolates group together and are located on branch B. The DSM 30164 strain is present in branch A and shows proximity to NCTC 235, which represents the type strain of M. morganii deposited in the National Collection of Type Cultures (Salisbury, United Kingdom). A boundary of 95% ANI is proposed to distinguish between bacterial species (Richter and Rosselló-Móra, 2009; Chun et al., 2018; Jain et al., 2018). When we applied an ANI cut-off value of 95%, the M. morganii genomes revealed four different clusters (I, II, III, and IV in Figure 2). The largest cluster I belongs to branch A and contains 79 genomes including the three assemblies of the M. morganii subsp. morganii type strain. The genomes of the strains GCSL-TSO-24 and H1r which also belong to branch A form together a small cluster (cluster II). The second largest cluster (cluster III) comprises 18 genomes including all 11 cheese isolates and belongs to branch B. This branch also includes the genome of strain MMSCG. However, the ANI value of this genome was lower than 95% when compared to the ones of branch B. In addition to the separation based on the ANI values, the mean GC-content of cluster I (51.0%) and cluster III (50.4%) genomes is statistically significant different (P < 0.05, unpaired two-samples t-test) (Supplementary Figure 1).
Biogenic Amine Formation
Ingested histamine can provoke allergic-like reactions in humans. The toxicity of histamine is enhanced by the presence of cadaverine and putrescine (Linares et al., 2011). Therefore, bacteria producing cadaverine, putrescine, or histamine are undesirable in cheese. We determined the capability of the M. morganii cheese isolates to produce biogenic amines.
All cheese isolates produced histamine (data not shown), whereas the formation of cadaverine and putrescine was strain dependent. Nine cheese isolates (FAM24670, FAM24681, FAM24671, FAM24672, FAM24678, FAM24685, FAM24091, FAM24206, and FAM24675) produced cadaverine in the range of 4.6–7.4 g L–1 (strong producers) and two isolates (FAM24679 and FAM24676) produced a maximum of 0.5 g L–1 cadaverine (weak producers) when incubated in broth containing L-lysine (Figure 3A), respectively. The strong cadaverine producer possess a gene that is annotated as lysine decarboxylase ldcC. The translated amino acid sequence of this gene is identical among the strong producers. Because the percent identity of the protein sequence is higher to the Escherichia coli inducible lysine decarboxylase CadA (68.5%, Swiss-Prot accession number P0A9H3) than to the E. coli LdcC (61.8%, UniProtKB accession number P52095), we refer to the lysine decarboxylase gene of M. morganii as cadA in this report.
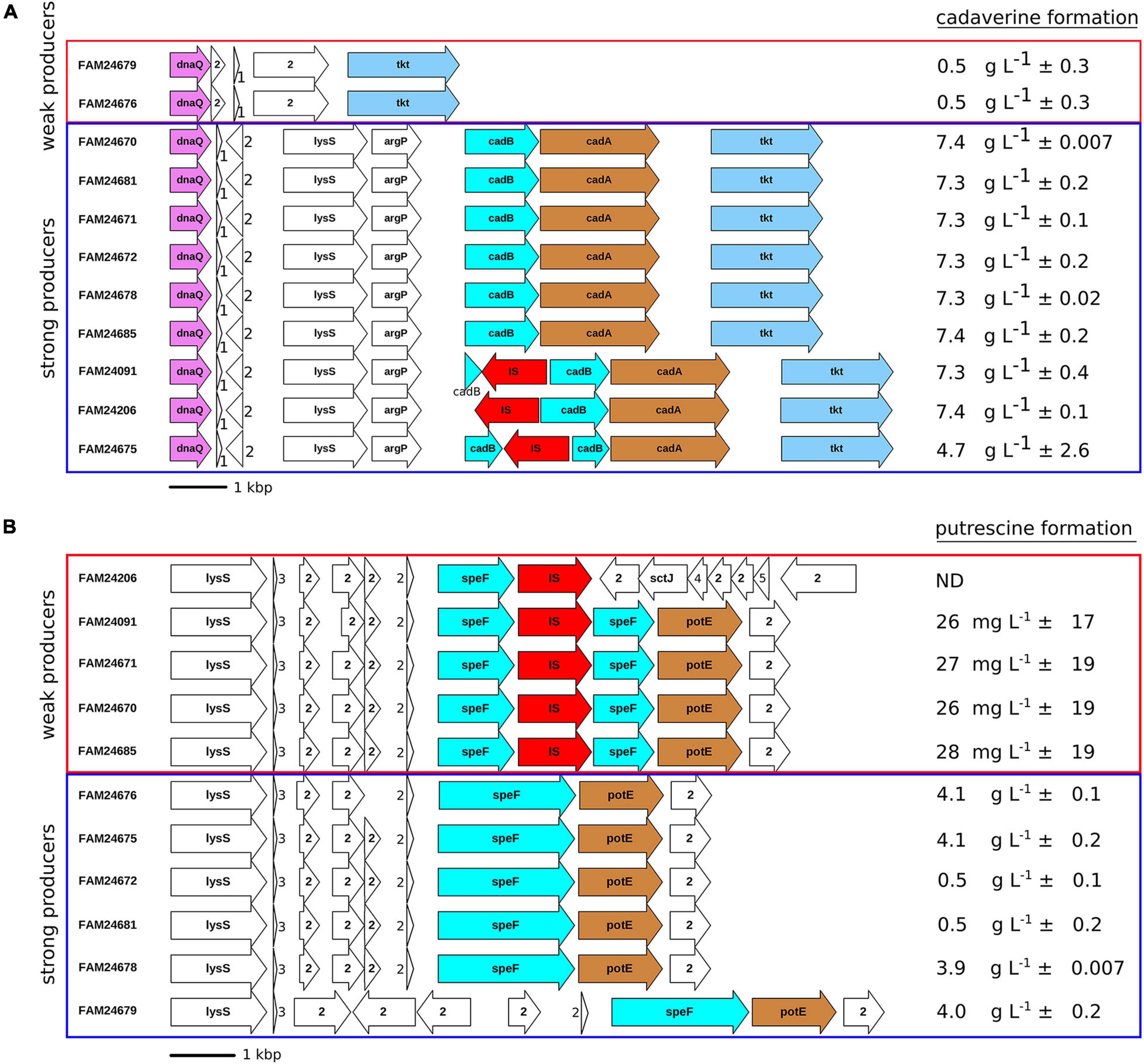
Figure 3. Genetic organization of loci containing the genes involved in (A) cadaverine formation and (B) putrescine formation. Arrows indicate open reading frames. DnaQ, DNA polymerase III subunit epsilon; tkt, transketolase; lysS, lysine-tRNA ligase; argP, HTH-transcriptional regulator; cadB, cadaverine-lysine antiporter; cadA, lysine decarboxylase; IS, insertion sequence; speF, ornithine decarboxylase; potE, putrescine-ornithine antiporter; sctJ, type III secretion inner membrane ring lipoprotein, 1: tRNAAsp, 2: hypothetical protein, 3: tRNAGly, 4(sctI): type III secretion system inner rod subunit, 5 (sctF): type III secretion system needle filament subunit. The mean ± standard deviation of biogenic amine formation of two replicates determined by HPLC is shown (mg of biogenic amines per L broth). Rectangles mark strong (blue) and weak (red) biogenic amine producers. Some orthologs are colored in order to increase readability. ND: below limit of quantification.
The genomic region surrounding cadA revealed the three genes lysS, argP, and cadB (Figure 3A). The result of BLASTp searches of the protein sequences against the UniProtKB database propose that the genes encode for a lysine-tRNA ligase (LysS), an HTH-transcriptional regulator (ArgP), and a cadaverine-lysine antiporter (CadB) (data not shown). The cadB gene of FAM24091, FAM24206, and FAM24675 is disrupted by an IS3 element. Interestingly, the disruption of this gene did not affect the capability to produce cadaverine as these three strains still exhibited strong production of cadaverine.
When L-ornithine was present in the medium, six of the cheese isolates (FAM24676, FAM24675, FAM24672, FAM24681, FAM24678, and FAM24679) produced putrescine in the range of 0.5–4.2 g L–1 (strong producers) (Figure 3B) and five cheese isolates (FAM24206, FAM24091, FAM24671, FAM24670, and FAM24685) produced less than 30 mg L–1 putrescine (weak producers). de las Rivas et al. (2007, 2008) showed that both genes speF and speC encode an ornithine decarboxylase in M. morganii and are involved in putrescine formation. All study strains possess both speF and speC. While the nucleotide sequence of speC is identical in all strains (data not shown), an IS3 family insertion sequence disrupts the speF gene in all five weak putrescine producers (Figure 3B). A putrescine-ornithine antiporter (potE) is located downstream of speF (de las Rivas et al., 2007). The antiporter is present in all but one cheese isolate. This indicates that the potE is not affected by the IS in speF. Only one weak putrescine producer (FAM24206) misses the antiporter.
Antibiotic Susceptibility Testing and Resistance Genes
Foodborne bacteria exhibiting antibiotic resistances may act as an antibiotic resistance gene reservoir and can pass their resistances to other bacteria in the human intestinal tract (Huddleston, 2014). We performed antibiotic sensitivity tests with all M. morganii cheese isolates and could show that all strains exhibited resistance against tetracycline, chloramphenicol, tigecycline, colistin, and ampicillin (Table 2). Additionally, the strains FAM24679, FAM24675, and FAM24678 were resistant against trimethoprim.
Screening of the genomes for known antibiotic resistance genes revealed that that all M. morganii cheese isolates possess an ampC gene (DHA family class C beta-lactamase), a tet(D) gene (tetracycline efflux transporter), a catA gene (chloramphenicol O-acetyltransferase), and an acrA gene (multidrug efflux pump subunit) (Table 3). Ruzin et al. (2005) showed that acrA is associated with tigecycline resistance in M. morganii. Interestingly, all antibiotic resistance genes are located on the chromosome and not on plasmids. M. morganii has an intrinsic resistance to colistin (Liu et al., 2016).
Discussion
Taxonomic Assignment
Since the initial description of Morganella morganii (Proteus morganii) the species has undergone several taxonomic reclassifications (O’Hara et al., 2000). In the current classification, M. morganii forms together with Morganella psychrotolerans the genus Morganella in the family Morganellaceae (Emborg et al., 2006). Based on trehalose metabolism, M. morganii is divided into two subspecies (Jensen et al., 1992). Trehalose fermenting M. morganii are designated as M. morganii subsp. sibonii, whereas M. morganii unable to ferment trehalose belong to M. morganii subsp. morganii. Ten out of the 11 M. morganii cheese isolates of this study fermented trehalose. The genome data showed that all cheese isolates possess the trehalose operon. The absence of trehalose fermentation in one strain is likely caused by the disruption of the treB gene of the trehalose operon by an IS element. Considering phenotypic and genotypic characteristics, all cheese isolates belong to M. morganii subsp. sibonii. Therefore, we recommend to perform both genetic and physiological characterization to determine the subspecies of new isolates to avoid wrong classifications.
The core genome-based clustering of the Morganella spp. genomes retrieved from GenBank and the cheese isolates confirm that M. morganii and M. psychrotolerans can be distinguished using genomic analysis. Palmieri et al. (2020) showed that the trehalose operon negative (subsp. morganii) and the trehalose operon positive (subsp. sibonii) strains group separately. Only the trehalose operon positive strain H1R clustered together with the subsp. morganii strains. Remarkably, in the current study, all strains between subsp. morganii (cluster I) and subsp. sibonii (cluster III) are separated by an ANI of less than 95.0%. This ANI cutoff score of less than 95.0% is typically used to separate prokaryotic organisms into distinct species (Richter and Rosselló-Móra, 2009; Chun et al., 2018; Jain et al., 2018). Consequently, this suggests that the bacteria of cluster III constitute a distinct bacterial species. The strains H1R, GCSL-TSO-24 (cluster II) and MMSCG (cluster IV) are separated by ANI of less than 95% from both cluster I and III, respectively. This could indicate that even more putative Morganella species exist. However, more strains belonging to cluster II and IV would be required to confirm this hypothesis. The existence of more Morganella species would also explain why the trehalose operon positive H1R strain does not cluster together with the other subsp. sibonii strains (Palmieri et al., 2020). DNA-DNA hybridization experiments of Emborg et al. (2006) also revealed that two trehalose positive strains analyzed in their study did not group together with the strains from subsp. sibonii. The presence of multiple clusters of trehalose operon positive strains implies that the phenotype with respect to trehalose fermentation alone is not sufficient to distinguish the clusters.
The capability of M. morganii to metabolize trehalose may have an impact on its growth in cheese. González-Hernández et al. (2005) demonstrated that Debaryomyces hansenii, which can be part of the cheese microbiome, can produce trehalose in response to salt stress. Consequently, trehalose-degrading M. morganii may benefit from this additional energy source in cheese. This could explain why all 11 cheese isolates belong to the subsp. sibonii.
Formation of Biogenic Amines
Both subspecies are further divided into biogroups based on their ability to decarboxylate ornithine (ODC+) and lysine (LDC+) to the biogenic amines putrescine and cadaverine, respectively (Jensen et al., 1992). Both cadaverine and putrescine are undesirable in cheese because they may provoke toxic reactions when ingested together with histamine (Linares et al., 2011). Several studies showed that M. morganii can produce toxic histamine concentrations in fish products (Kim et al., 2003; Kanki et al., 2007). In our study, all M. morganii cheese isolates produced histamine in vitro (data not shown) and exhibited strain-dependent capability to produce cadaverine and putrescine in vitro. The ability to form biogenic amines makes this bacterium undesirable in cheese. In situ experiments with M. morganii will clarify if the cheese isolates also produce biogenic amines in cheese.
With regard to cheese, in which the carbohydrate sources become exhausted during ripening, M. morganii could generate energy by amino acid decarboxylation. During the decarboxylation reaction, protons are consumed (Barbieri et al., 2019) which could lead to a proton gradient across the cell membrane. In fact, this was shown by Molenaar et al. (1993) for Lactobacillus (para)buchneri with the decarboxylation of histidine. The resulting proton motive force could be used for ATP synthesis. Additionally, the formation of amines increases the pH in cheese, which may be a protective measure against acidic stress in the cheese environment.
Locus Involved in Cadaverine Formation
To the best of our knowledge, the gene encoding a lysine decarboxylase has not yet been described in M. morganii. In this study, we found that the locus containing the four genes lysS (lysine-tRNA ligase), argP (trancriptional regulator), cadB (cadaverine-lysine antiporter), and cadA (lysine decarboxylase) is responsible for strong lysine decarboxylation.
Escherichia coli also possesses a cad locus that is responsible for cadaverine formation. However, the genetic organization of the E. coli cad locus is different from the cadA locus of M. morganii (Meng and Bennett, 1992). Upstream of the E. coli cadB is an additional gene cadC that encodes for a DNA-binding transcriptional activator and the lysine-tRNA ligase (lysU) is located downstream of cadA.
As in E. coli, the genes responsible for lysine decarboxylation are located on the chromosome of the M. morganii cheese strains. This apparently contradicts observations made by Cornelis et al. (1981) who concluded that the lysine-decarboxylating phenotype (called lysine-positive character) is plasmid-encoded. The researchers observed that the lysine-positive character could be transferred to lysine-negative strains. It is noteworthy that the GC-content of the cadB and cadA genes of the cheese isolates is 44.4 and 38.7%, respectively. This is lower than the mean GC-content of the chromosome (50.3%). This indicates that both genes may have been acquired by horizontal gene transfer and could indeed be transferred between strains.
Remarkably, the disruption of the cadaverine-lysine antiporter cadB in FAM24091, FAM24206, and FAM24675 did not affect the capability to produce cadaverine. We did not find any other gene that shows similarities to cadB or cadA (lysine decarboxylase). It suggests that the lysine/cadaverine antiporter function may be taken over by other transporters in M. morganii.
SpeF Is Mainly Responsible for Putrescine Formation
M. morganii possess the two genes speF and speC that encode for ornithine decarboxylases (de las Rivas et al., 2007, 2008). Both genes are present in all cheese isolates of our study. However, five cheese strains showed weak putrescine production. In these strains, an IS element disrupts the speF gene, whereas the speC gene is intact. This indicates that speF is the gene mainly responsible for putrescine formation observed in vitro. The production of cheese inoculated with strains having both the intact and the disrupted speF will help to understand the putrescine formation in situ.
Antibiotic Resistances of the Cheese Isolates
Bacteria showing antibiotic resistances are undesirable in food because they can disseminate their resistances to human pathogenic bacteria (Huddleston, 2014). Therefore, we analyzed antibiotic resistances of the M. morganii cheese isolates. All strains were resistant against ampicillin, tetracycline, colistin, and tigecycline. M. morganii possesses an ampC β-lactamase which makes the species naturally resistant to ampicillin (Liu et al., 2016). We detected the gene in all cheese isolates. We could link the tetracycline resistance to the tetracycline efflux transporter tet(D) gene (Henriques et al., 2008). The resistance to tetracycline is widespread in M. morganii subsp. sibonii. However, it can also occur in M. morganii subsp. morganii strains (Stock and Wiedermann, 1998). M. morganii has an intrinsic colistin resistance, which is conferred by the structure, composition and modifications of lipid A that prevents the binding of the antibiotic to the lipopolysaccharides of the outer membrane (Olaitan et al., 2014). M. morganii, as do all our cheese isolates, possess an AcrAB efflux pump that decreases the susceptibility to tigecycline (Ruzin et al., 2005). M. morganii is normally sensitive to trimethoprim (Liu et al., 2016). However, three cheese isolates were resistant against this antibiotic. We could not identify the resistance mechanism because the three strains do not possess known resistance genes described in the literature (Tsakris et al., 2007; Schultz et al., 2017) or CARD database. Furthermore, the amino acid sequence of the dihydrofolate reductase (DHFR), the target enzyme of trimethoprim, is identical to the DHFR sequence of sensitive cheese isolates (Watson et al., 2007).
The characteristic of resistance to chloramphenicol is not uniformly described in the literature. On the one hand, there are reports that M. morganii is sensitive to chloramphenicol (Janda and Abbott, 2015) and on the other hand, that cat genes conferring resistance to chloramphenicol are located on chromosomes (Chen et al., 2012; Liu et al., 2016). In our case, all cheese isolates show chloramphenicol resistance and possess a cat gene located on the chromosome. These findings indicate that chloramphenicol resistance is presumably more widespread than previously thought.
Conclusion
In the present study we isolated M. morganii strains from different cheese samples. Our genomic and phenotypic analyses show that the isolates belong to subsp. sibonii. The differences in ANI values between subsp. morganii and subsp. sibonii suggest that they may represents distinct bacterial species. Various strains can metabolize trehalose, histidine, lysine, and ornithine, which serve as energy sources in cheese environments. We linked the metabolic activities to genetic loci, and, to the best of our knowledge, describe the locus involved in cadaverine formation for the first time. Antibiotic resistance profiles did not exhibit unusual resistances. Taken together, in this study we describe M. morganii cheese isolates both genetically and phenotypically, providing a useful resource for the field of comparative genomics. In situ experiments will clarify the impact of M. morganii on cheese quality.
Data Availability Statement
The datasets presented in this study can be found in online repositories. The names of the repository/repositories and accession number(s) can be found below: https://www.ncbi.nlm.nih.gov/genbank/, CP066777, CP066778, CP066142, CP066140, CP066141, CP066138, CP066139, CP066137, CP066133, CP066134, CP066135, CP066136, CP066132, CP066130, CP066131, CP066129, CP068562, CP066127, and CP069157.
Author Contributions
LR, SI, and VP conducted the experiments. LR and RB performed the bioinformatic analysis. LR, SI, and RB wrote the manuscript. EA-R critically revised and discussed the manuscript. All authors read and approved the final manuscript, conceived and designed the study, and analyzed the data.
Funding
Part of this study was funded by the Innosuisse Project 18791.2_PFLS-LS and the Canton of Bern.
Conflict of Interest
The authors declare that the research was conducted in the absence of any commercial or financial relationships that could be construed as a potential conflict of interest.
Publisher’s Note
All claims expressed in this article are solely those of the authors and do not necessarily represent those of their affiliated organizations, or those of the publisher, the editors and the reviewers. Any product that may be evaluated in this article, or claim that may be made by its manufacturer, is not guaranteed or endorsed by the publisher.
Acknowledgments
We thank Tharmatha Bavan, Monika Haueter, Cédric Brügger, Dieter Weik, and Alexandra Collaud for excellent technical support.
Supplementary Material
The Supplementary Material for this article can be found online at: https://www.frontiersin.org/articles/10.3389/fmicb.2021.738492/full#supplementary-material
Footnotes
- ^ https://community.nanoporetech.com/
- ^ https://github.com/nanoporetech/medaka
- ^ https://github.com/mwaskom/seaborn
- ^ http://www.eucast.org
- ^ https://github.com/tseemann/abricate
References
Amato, L., Ritschard, J. S., Kurtz, O., Arias-Roth, E., Lacroix, C., Schuppler, M., et al. (2012). Microbial composition of defect smear – a problem evolving during foil-prepacked storage of red-smear cheeses. Int. Dairy J. 27, 77–85. doi: 10.1016/j.idairyj.2012.07.012
Antipov, D., Hartwick, N., Shen, M., Raiko, M., Lapidus, A., and Pevzner, P. A. (2016). PlasmidSPAdes: assembling plasmids from whole genome sequencing data. Bioinformatics 32, 3380–3387. doi: 10.1093/bioinformatics/btw493
Ascone, P., Maurer, J., Haldemann, J., Irmler, S., Berthoud, H., Portmann, R., et al. (2017). Prevalence and diversity of histamine-forming Lactobacillus parabuchneri strains in raw milk and cheese – a case study. Int. Dairy J. 70, 26–33. doi: 10.1016/j.idairyj.2016.11.012
Barbieri, F., Montanari, C., Gardini, F., and Tabanelli, G. (2019). Biogenic amine production by lactic acid bacteria: a review. Foods 8:17. doi: 10.3390/foods8010017
Bolger, A. M., Lohse, M., and Usadel, B. (2014). Trimmomatic: a flexible trimmer for Illumina sequence data. Bioinformatics 30, 2114–2120. doi: 10.1093/bioinformatics/btu170
Bover-Cid, S., and Holzapfel, W. H. (1999). Improved screening procedure for biogenic amine production by lactic acid bacteria. Int. J. Food Microbiol. 53, 33–41. doi: 10.1016/S0168-1605(99)00152-X
Chen, Y. T., Peng, H. L., Shia, W. C., Hsu, F. R., Ken, C. F., Tsao, Y. M., et al. (2012). Whole-genome sequencing and identification of Morganella morganii KT pathogenicity-related genes. BMC Genomics 13:S4. doi: 10.1186/1471-2164-13-S7-S4
Chun, J., Oren, A., Ventosa, A., Christensen, H., Arahal, D. R., da Costa, M. S., et al. (2018). Proposed minimal standards for the use of genome data for the taxonomy of prokaryotes. Int. J. Syst. Evol. Microbiol. 68, 461–466. doi: 10.1099/ijsem.0.002516
CLSI (2020). Performance Standards for Antimicrobial Susceptibility Testing. CLSI supplement M100. 30th ed. Wayne, PA: Clinical and Laboratory Standards Institute.
Cornelis, G., Van Bouchaute, M., and Wauters, G. (1981). Plasmid-encoded lysine decarboxylation in Proteus morganii. J. Clin. Microbiol. 14, 365–369. doi: 10.1128/jcm.14.4.365-369.1981
Coton, M., Delbés-Paus, C., Irlinger, F., Desmasures, N., Le Fleche, A., Stahl, V., et al. (2012). Diversity and assessment of potential risk factors of Gram-negative isolates associated with French cheeses. Food Microbiol. 29, 88–98. doi: 10.1016/j.fm.2011.08.020
de las Rivas, B., Gonzalez, R., Landete, J. M., and Munoz, R. (2008). Characterization of a second ornithine decarboxylase Isolated from Morganella morganii. J. Food Prot. 71, 657–661. doi: 10.4315/0362-028x-71.3.657
de las Rivas, B., Marcobal, A., and Munoz, R. (2007). Gene organization of the ornithine decarboxylase-encoding region in Morganella morganii. J. Appl. Microbiol. 102, 1551–1560. doi: 10.1111/j.1365-2672.2006.03188.x
Emborg, J., Dalgaard, P., and Ahrens, P. (2006). Morganella psychrotolerans sp. nov., a histamine-producing bacterium isolated from various seafoods. Int. J. Syst. Evol. Microbiol. 56, 2473–2479. doi: 10.1099/ijs.0.64357-0
González-Hernández, J. C., Jimenez-Estrada, M., and Pena, A. (2005). Comparative analysis of trehalose production by Debaryomyces hansenii and Saccharomyces cerevisiae under saline stress. Extremophiles 9, 7–16. doi: 10.1007/s00792-004-0415-2
Gurevich, A., Saveliev, V., Vyahhi, N., and Tesler, G. (2013). QUAST: quality assessment tool for genome assemblies. Bioinformatics 29, 1072–1075. doi: 10.1093/bioinformatics/btt086
Henriques, I. S., Fonseca, F., Alves, A., Saavedra, M. J., and Correia, A. (2008). Tetracycline-resistance genes in Gram-negative isolates from estuarine waters. Lett. Appl. Microbiol. 47, 526–533. doi: 10.1111/j.1472-765X.2008.02452.x
Huddleston, J. R. (2014). Horizontal gene transfer in the human gastrointestinal tract: potential spread of antibiotic resistance genes. Infect. Drug Resist. 7, 167–176. doi: 10.2147/IDR.S48820
Huerta-Cepas, J., Serra, F., and Bork, P. (2016). ETE 3: reconstruction, analysis, and visualization of phylogenomic data. Mol. Biol. Evol. 33, 1635–1638. doi: 10.1093/molbev/msw046
Jain, C., Rodriguez-R, L. M., Phillippy, A. M., Konstantinidis, K. T., and Aluru, S. (2018). High throughput ANI analysis of 90K prokaryotic genomes reveals clear species boundaries. Nat. Commun. 9:5114. doi: 10.1038/s41467-018-07641-9
Janda, J. M., and Abbott, S. L. (2015). “Morganella,” in Bergey’s Manual of Systematics of Archaea and Bacteria, eds M. E. Trujillo, S. Dedysh, P. DeVos, B. Hedlund, P. Kämpfer, F. A. Rainey, et al. (Hoboken, NJ: John Wiley & Sons, Inc), doi: 10.1002/9781118960608.gbm01155
Janda, J. M., Abbott, S. L., Khashe, S., and Robin, T. (1996). Biochemical investigations of biogroups and subspecies of Morganella morganii. J. Clin. Microbiol. 34, 108–113. doi: 10.1128/jcm.34.1.108-113.1996
Jensen, K. T., Frederiksen, W., Hickman-Brenner, F. W., Steigerwalt, A. G., Riddle, C. F., and Brenner, D. J. (1992). Recognition of Morganella subspecies, with proposal of Morganella morganii subsp. morganii subsp. nov. and Morganella morganii subsp. sibonii subsp. nov. Int. J. Syst. Microbiol. 42, 613–620. doi: 10.1099/00207713-42-4-613
Jia, B., Raphenya, A. R., Alcock, B., Waglechner, N., Guo, P., Tsang, K. K., et al. (2017). CARD 2017: expansion and model-centric curation of the comprehensive antibiotic resistance database. Nucleic Acids Res. 45, D566–D573. doi: 10.1093/nar/gkw1004
Kanki, M., Yoda, T., Tsukamoto, T., and Baba, E. (2007). Histidine decarboxylases and their role in accumulation of histamine in tuna and dried saury. Appl. Environ. Microbiol. 73, 1467–1473. doi: 10.1128/AEM.01907-06
Kim, S. H., An, H., Wei, C. I., Visessanguan, W., Benjakul, S., Morrissey, M. T., et al. (2003). Molecular detection of a histamine former. Morganella morganii, in albacore, mackerel, sardine, and a processing plant. J. Food Sci. 68, 453–457. doi: 10.1111/j.1365-2621.2003.tb05693.x
Kolmogorov, M., Yuan, J., Lin, Y., and Pevzner, P. A. (2019). Assembly of long, error-prone reads using repeat graphs. Nat. Biotechnol. 37, 540–546. doi: 10.1038/s41587-019-0072-8
Langmead, B., and Salzberg, S. L. (2012). Fast gapped-read alignment with Bowtie 2. Nat. Methods 9, 357–359. doi: 10.1038/nmeth.1923
Li, H. (2018). Minimap2: pairwise alignment for nucleotide sequences. Bioinformatics 34, 3094–3100. doi: 10.1093/bioinformatics/bty191
Linares, D. M., Martín, M. C., Ladero, V., Alvarez, M. A., and Fernandez, M. (2011). Biogenic amines in dairy products. Crit. Rev. Food Sci. Nutr. 51, 691–703. doi: 10.1080/10408398.2011.582813
Liu, H., Zhu, J., Hu, Q., and Rao, X. (2016). Morganella morganii, a non-negligent opportunistic pathogen. Int. J. Infect. Dis. 50, 10–17. doi: 10.1016/j.ijid.2016.07.006
Meng, S. Y., and Bennett, G. N. (1992). Nucleotide sequence of the Escherichia coli cad operon: a system for neutralization of low extracellular pH. J. Bacteriol. 174, 2659–2669. doi: 10.1128/jb.174.8.2659-2669.1992
Molenaar, D., Bosscher, J. S., TenBrink, B., Driessen, A. J., and Konings, W. N. (1993). Generation of a proton motive force by histidine decarboxylation and electrogenic histidine/histamine antiport in Lactobacillus buchneri. J. Bacteriol. 175, 2864–2870. doi: 10.1128/jb.175.10.2864-2870.1993
O’Hara, C. M., Brenner, F. W., and Miller, J. M. (2000). Classification, identification, and clinical significance of Proteus, Providencia, and Morganella. Clin. Microbiol. Rev. 13, 534–546. doi: 10.1128/CMR.13.4.534
Olaitan, A. O., Diene, S. M., Gupta, S. K., Adler, A., Assous, M. V., and Rolain, J.-M. (2014). Genome analysis of NDM-1 producing Morganella morganii clinical isolate. Expert Rev. Anti. Infect. Ther. 12, 1297–1305. doi: 10.1586/14787210.2014.944504
Page, A. J., Cummins, C. A., Hunt, M., Wong, V. K., Reuter, S., Holden, M. T. G., et al. (2015). Roary: rapid large-scale prokaryote pan genome analysis. Bioinformatics 31, 3691–3693. doi: 10.1093/bioinformatics/btv421
Palmieri, N., Hess, C., Hess, M., and Alispahic, M. (2020). Sequencing of five poultry strains elucidates phylogenetic relationships and divergence in virulence genes in Morganella morganii. BMC Genomics 21:579. doi: 10.1186/s12864-020-07001-2
Richter, M., and Rosselló-Móra, R. (2009). Shifting the genomic gold standard for the prokaryotic species definition. Proc. Natl. Acad. Sci. USA 106, 19126–19131. doi: 10.1073/pnas.0906412106
Ruzin, A., Keeney, D., and Bradford, P. A. (2005). AcrAB efflux pump plays a role in decreased susceptibility to tigecycline in Morganella morganii. Antimicrob. Agents Chemother. 49, 791–793. doi: 10.1128/AAC.49.2.791-793.2005
Schultz, E., Barraud, O., Madec, J., Haenni, M., Cloeckaert, A., Ploy, M., et al. (2017). Multidrug resistance Salmonella Genomic Island 1 in a Morganella morganii subsp. morganii human clinical isolate from France. mSphere 2, e117–e118. doi: 10.1128/mSphere.00118-17
Seemann, T. (2014). Prokka: rapid prokaryotic genome annotation. Bioinformatics 30, 2068–2069. doi: 10.1093/bioinformatics/btu153
Siguier, P., Pérochon, J., Lestrade, L., Mahillon, J., and Chandler, M. (2006). ISfinder: the reference centre for bacterial insertion sequences. Nucleic Acids Res. 34, D32–D36. doi: 10.1093/nar/gkj014
Stamatakis, A. (2014). RAxML version 8: a tool for phylogenetic analysis and post-analysis of large phylogenies. Bioinformatics 30, 1312–1313. doi: 10.1093/bioinformatics/btu033
Stock, I., and Wiedermann, B. (1998). Identification and natural antibiotic susceptibility of Morganella morganii. Diagn. Microbiol. Infect. Dis. 30, 153–165. doi: 10.1016/S0732-8893(97)00243-5
Tatusova, T., DiCuccio, M., Badretdin, A., Chetvernin, V., Nawrocki, E. P., Zaslavsky, L., et al. (2016). NCBI Prokaryotic Genome Annotation Pipeline. Nucleic Acids Res. 44, 6614–6624. doi: 10.1093/nar/gkw569
Tsakris, A., Ikonomidis, A., Spanakis, N., Poulou, A., and Pournaras, S. (2007). Characterization of In3Mor, a new integron carrying VIM-1 metallo-β-lactamase and sat1 gene, from Morganella morganii. J. Antimicrob. Chemother. 59, 739–741. doi: 10.1093/jac/dkm020
Vaser, R., Sovic, I., Nagarajan, N., and Sikic, M. (2017). Fast and accurate de novo genome assembly from long uncorrected reads. Genome Res. 27, 737–746. doi: 10.1101/gr.214270.116
Walker, B. J., Abeel, T., Shea, T., Priest, M., Abouelliel, A., Sakthikumar, S., et al. (2014). Pilon: an integrated tool for comprehensive microbial variant detection and genome assembly improvement. PLoS One 9:e112963. doi: 10.1371/journal.pone.0112963
Keywords: Morganella morganii, biogenic amines, lysine decarboxylase, antibiotic resistances, histamine, cadaverine, putrescine, trehalose
Citation: Ryser LT, Arias-Roth E, Perreten V, Irmler S and Bruggmann R (2021) Genetic and Phenotypic Diversity of Morganella morganii Isolated From Cheese. Front. Microbiol. 12:738492. doi: 10.3389/fmicb.2021.738492
Received: 08 July 2021; Accepted: 13 October 2021;
Published: 17 November 2021.
Edited by:
Mutamed Ayyash, United Arab Emirates University, United Arab EmiratesReviewed by:
Tam Tran, University of Science and Technology of Hanoi (USTH), VietnamShabarinath Srikumar, United Arab Emirates University, United Arab Emirates
Copyright © 2021 Ryser, Arias-Roth, Perreten, Irmler and Bruggmann. This is an open-access article distributed under the terms of the Creative Commons Attribution License (CC BY). The use, distribution or reproduction in other forums is permitted, provided the original author(s) and the copyright owner(s) are credited and that the original publication in this journal is cited, in accordance with accepted academic practice. No use, distribution or reproduction is permitted which does not comply with these terms.
*Correspondence: Rémy Bruggmann, cmVteS5icnVnZ21hbm5AYmlvaW5mb3JtYXRpY3MudW5pYmUuY2g=