- Department of Microecology, College of Basic Medical Sciences, Dalian Medical University, Dalian, China
Mounting evidence suggests that probiotics can be used to treat allergic asthma by modulating the gut microbiota, and that the effects of probiotics may be influenced by environmental factors such as diet. We conducted a rat model with allergic asthma (AA) modulated by Lactobacillus paracasei, feeding up with high-fat or high-fiber diets based on collecting data from 85 questionnaires. The systemic proinflammatory cytokines were detected by ELISA and the overall structure of fecal microbiota was analyzed via 16S rRNA gene sequencing. The results showed consumption of a high-fiber diet alleviated the allergic symptoms and airway inflammation, and led to improving the imbalance of T-helper type 1 (Th1)/Th2 cells with increased expression of interferon-γ and decreased expression of interleukin-4. Whereas, the high-fat diet had deteriorating implications and skewed the inflammatory perturbation. Furthermore, abundances of phylum Bacteroidetes, families Muribaculaceae, Tannerellaceae, Prevotellaceae, Enterococcaceae, genera Allobaculum, Parabacteroides, and Enterococcus were enriched in L. paracasei-modulating rats fed with high-fiber diet. Firmicutes and Proteobacteria, families Lachnospiraceae, Ruminococcaceae and Desulfovibrionaceae, genera Blautia, unidentified_Ruminococcaceae, unidentified_Clostridiales and Oscillibacter were in relatively high abundance in the rats administered high-fat diet. Association between changed microbiota and inflammatory cytokines was also conferred. These data indicated that the efficacy of L. paracasei in allergic asthma was influenced by different dietary patterns. Hence, diet is important for probiotic therapy when managing allergic asthma.
Introduction
There has been an increase in the prevalence of allergic asthma (AA) globally, particularly in children and in Western countries, which has led to substantial financial and medical burdens (Colombo et al., 2019). The recommended resolution for AA is to use high-dose inhaled corticosteroids (ICs) alone or combine them with long-acting bronchodilators. Unfortunately, besides the undesirable effects of long-term treatment with ICs, a significant number of asthmatic patients fail to respond to IC therapy.
A disturbed balance of Th1/Th2 cells and Th17/T-regulatory cells (Tregs) imbalance in AA have been reported. Th2 cytokines [e.g., interleukin(IL)-4, IL-5, and IL-13] and potent proinflammatory cytokines (e.g., IL-1β, IL-6, IL-17, IL-25, and tumor necrosis factor (TNF)-α) orchestrate mucosal inflammation. Inflammatory mediators have been shown to impair or boost mucosal inflammation in the airways. Over recent decades, increased perturbation of the microbiota has been demonstrated to contribute to the development of allergic inflammation. Mounting evidence suggests that low diversity of the gut microbiota (GM) in early infancy is a vital risk factor for the development of immune-mediated allergic diseases (Abrahamsson et al., 2014; Tsabouri et al., 2014; Dzidic et al., 2017). Furthermore, microbial colonization in germ-free mice within the first days of life or in antibiotic-treated mice has been indicated to protect against increased IgE levels (Herbst et al., 2011) and promote tolerance to aeroallergens via Tregs induction (Gollwitzer et al., 2014).
Beneficial bacteria modulate the intestinal microbiota and mucosal immune responses. A significantly decreased profile of Th2 cytokines in response to probiotic treatment has been found in animal experiments (Choi et al., 2018; Makino et al., 2019). Oral administration of mixed strains of Clostridium species to BALB/c mice has been shown to stimulate the allergen-induced expansion of Tregs in the colonic mucosa and decreased systemic production of IgE (Atarashi et al., 2011). Lactobacillus paracasei, commonly used in dairy-product fermentation, has been shown to mitigate respiratory tract allergies in various studies applying in mice and humans (Fujiwara et al., 2004; Schabussova et al., 2012; Wang et al., 2017; Lin et al., 2020). However, clinical trials have conflicting results in assessing L. paracasei prevents allergy (Kuitunen et al., 2009; Fiocchi et al., 2012; Jensen et al., 2012; West et al., 2013).
Several studies have underlined the critical role of diet in AA pathogenesis. High consumption of fat (e.g., Western diet) is likely related to an increased risk of asthma (Hou et al., 2011; Protudjer et al., 2012; Manzel et al., 2014; Myles, 2014; Thorburn et al., 2014; Julia et al., 2015). Diets high in fiber (e.g., fruit and vegetables) protect from allergies and are associated with decreased inflammation in the airways (Ellwood et al., 2001; Maslowski et al., 2009; Frei et al., 2012; Grimshaw et al., 2014; Trompette et al., 2014). The effect of diets on allergic asthma might have a close association with the intestinal microbiota. Different diets yield numerous metabolites that can influence the immune response, such as short-chain fatty acids (SCFAs) fermented by colonic commensal, responsible for the effect of anti-inflammation, and the saturated fatty acids or cholesterol displayed exacerbation for allergic disease. However, how to promote the effect of probiotics in people with different diets is not known.
Herein, we collected data (via questionnaires) from 85 human participants to design specific different dietary patterns and established a rat model of AA (using ovalbumin[OVA]) fed up with the designed diets to assess the efficacy of different diets on L. paracasei-modulated allergic inflammation. Our findings emphasize the importance of diet to explain the effect of L. paracasei on AA prevention.
Materials and Methods
Collection of Dietary Data
Collection of dietary data was conducted from 25 November to 2 December 2017. The food inventory was documented according to the Chinese Dietary Guidelines (fourth edition, 2016) and AA history. Food (including edible oils and salt) consumption was determined by weighing, individuals aged >18 years, and excluding those unable to complete data collection.
Preparation of Probiotics
Lactobacillus paracasei DMLA16017 (Department of Microecology, Dalian Medical University) was grown in MRS broth (Hopebio, Qingdao, China) at pH 5.7 ± 0.2 and under anaerobic conditions. Bacteria were harvested in the early stationary phase, washed with phosphate-buffered saline (PBS; Solarbio, Beijing, China), and stored at −80°C. Cell counts were determined by plating serial dilutions.
Animals and Intervention Design
The study protocol was approved by the Animal Ethics Committee of Dalian Medical University (No. L201312). Specific pathogen-free (SPF) three-week-old male Wistar rats were purchased from the Animal Center of Dalian Medical University and allowed to acclimatize to their surroundings for ≥24 h before experimentation. Rats were housed under standard temperature conditions and humidity with a 12-h light-dark schedule with free access to sterile food and autoclaved water.
An AA model was established according to a modification of airway inflammation described by Zhang and Shi (Zhang et al., 2016). After acclimatization, rats were assigned randomly to five groups (n = 8/group): the control group (Con), the model group (Model), the probiotics-modulated group (Probio), the high-fat diet intervented probiotics-modulated group (Hfat), and the high-fiber diet intervented probiotics-modulated group (Hfiber). Sensitizations were undertaken on days 1, 5, 8, and 12 in all groups except the Con group. Rats were sensitized to OVA (egg-white albumin of chickens ≥98%; Sigma-Aldrich, Saint Louis, MO, United States) by intraperitoneal injection of 0.2 mL of alum-precipitated antigen [comprising 10 μg of OVA absorbed into 1 mg of aluminum hydroxide (Sigma-Aldrich)]. From day 15,rats were administered (i.n.) 20 μL of 1% OVA diluted in PBS for 7 consecutive days and exposed daily to 1% OVA aerosol in PBS in an aerosol cabin for 30 min between day 28 and day 32 in the challenge phase. Rats in the Con group received PBS alone (Figure 1).
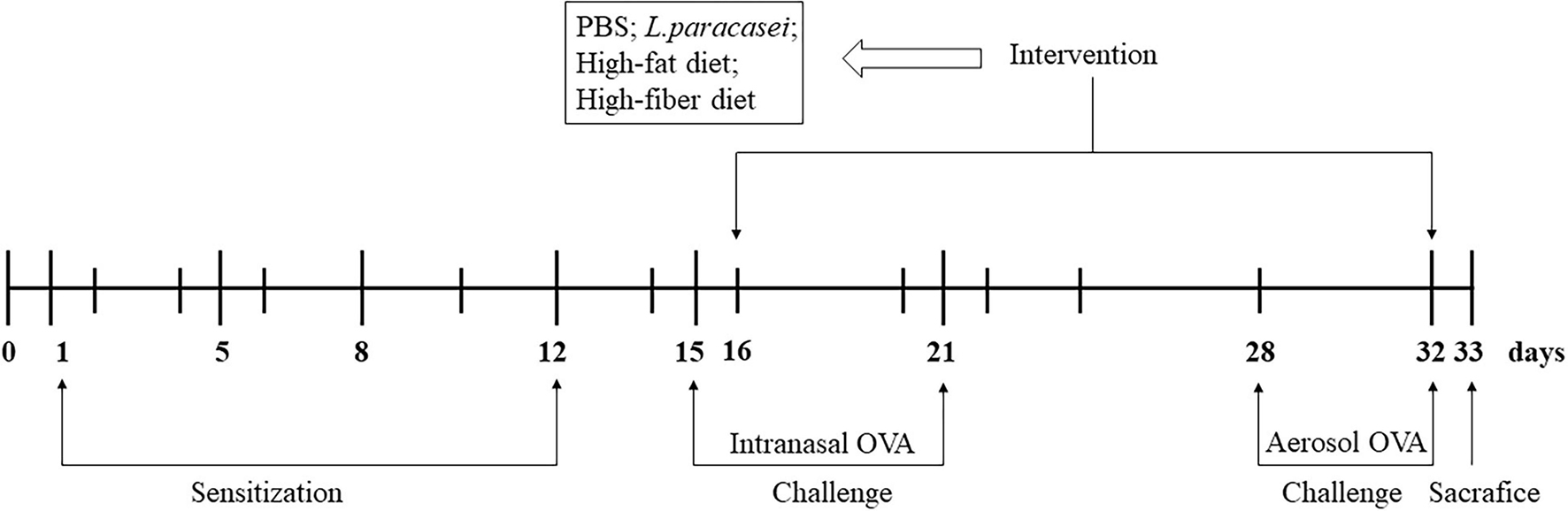
Figure 1. Experimental design of a model of allergic asthma in rats. Male Wistar rats were sensitized and challenged with OVA, as described in “Animals and Intervention Design” section. Groups were allocated as Con, Model, Probio, Hfat, and Hfiber. From day 16 until day 32, rats were treated daily with Lactobacillus paracasei, and then randomly assigned to receive basic chow, high-fat chow or high-fiber chow. Rats in the Con group and Model group received PBS only. All rats were sacrificed on day 33 after documentation of allergic symptoms.
After developing airway inflammation, other groups except Con and Model group were gavage with 1.0 × 109 colony-forming units of L. paracasei suspended in 0.2 mL of PBS per day, 1 h before the challenge. Rats that underwent gavage with L. paracasei were received high-fat (Hfat), high-fiber (Hfiber), or basic chow (Con, Model and Probio), respectively, that we purchased from Huafukang Bioscience (Beijing, China) (see Supplementary Table 1 for nutritional parameters). The intervention was from day 16 until day 32 (Figure 1). Twenty-four hours after the final challenge, all animals were sacrificed.
Evaluation of Allergy Symptoms
For each rat, the frequency of two symptoms of allergy (nose rubbing and sneezing) was recorded immediately for 15 min after the last challenge with OVA aerosol on day 32 in a blinded manner, as described previously (Shinmei et al., 2009).
Cell Counts for Bronchoalveolar Lavage Fluid (BALF)
After sacrifice on day 33, lungs were perfused with 1 mL of PBS containing 10% fetal bovine serum through a tracheal cannula to collect BALF. The latter was centrifuged at 1000 × g for 10 min at room temperature, and the supernatant was separated and stored until use. The pellet was resuspended in PBS for cell counting. BALF cells contain leukocytes and provide a “snapshot” of airway disease.
Enzyme-Linked Immunosorbent Assay (ELISA) for Total Level of OVA-Specific IgE and Cytokines
Twenty-four hours after the final challenge with OVA, rats were anesthetized and blood samples were withdrawn by cardiac puncture. Serum was separated by centrifugation (1000 × g, 10 min, room temperature) after resting for 1 h. The OVA-specific IgE level in serum was determined with an ELISA kit (No. RX-D302482R) according to manufacturer (Ruixinbio, Quanzhou, China) instructions. Absorbance was measured at 450 nm.
The level of IL-4, IL-17, interferon(IFN)-γ, and TGF-β in BALF and serum prepared as stated above were measured by ELISA kits (No. SEA077Ra, No. SEA063Ra, No. SEA049Ra; and No. RX302048R) following manufacturer (Cloud-Clone, Wuhan, China; Ruixinbio, Quanzhou, China) instructions.
Histology of Lung Tissue
After the sacrifice of rats on day 33, the left-lung lobes were removed rapidly and fixed in 4% paraformaldehyde for ≥24 h at room temperature. Then, lung tissues were dehydrated in a graded series of ethanol solutions, cleared in xylene, and embedded in paraffin. Histology slices (thickness = 5 μm) were prepared using a microtome. These slices were stained with hematoxylin and eosin (H&E) for morphology evaluation and inflammation using a microscope (BX50; Olympus, Tokyo, Japan) equipped with a digital camera (DFC 320, Leica, Wetzlar, Germany).
DNA Extraction and 16S rRNA Gene Sequencing of Bacteria
Thirty-five fresh feces from rats (seven from Con, five from Model, seven from Probio, eight from Hfat, and eight from Hfiber groups) were harvested aseptically with 2-mL sterilized Eppendorf tubes, respectively, and stored at −80°C immediately.
DNA isolation and 16S rRNA gene sequencing were provided by Novogene (Beijing, China), as described previously (Wang et al., 2016; Zhang et al., 2018), to ascertain the composition of the bacteria in the feces of rats in different groups. DNA was extracted using the cetyl trimethyl ammonium bromide/sodium dodecyl sulfonate (CTAB/SDS) method. The V3-V4 sequences of 16S rRNA genes were amplified by polymerase chain reaction (PCR) using specific primers (341 forward: 5′-CCTAYGGGRBGCASCAG-3′; 806 reverse: 5′-GGACTACNNGGGTATCTAAT-3′). The PCR-amplified product was purified with a gel extraction kit (Qiagen, Hilden, Germany). After quantification, amplicons were normalized to generate a sequencing library and sequenced on a NovaSeqTM platform (Illumina, San Diego, CA, United States).
Alpha diversity, including Chao1 and ACE, was analyzed with Quantitative Insights Into Microbial Ecology (QIIME) 1.7.01. Beta diversity was ascertained using the non-metric multi-dimensional scaling (NMDS) of QIIME 1.7.0 to determine the similarity of species diversity in different samples. Statistical significance was evaluated according to stress. Significant differences in the relative abundance of genera among strains were obtained by linear discriminant analysis (LDA) effect size (LEfSe).
Statistical Analyses
Continuous variables with a normal distribution were represented as the mean ± SEM, and comparisons between groups were determined by the parametric Student’s t-test. For parameters with a non-normal distribution, median and interquartile range (IQR) values are provided, and males were compared with females using the non-parametric Wilcoxon rank-sum test. Absolute and relative frequencies were calculated for qualitative data, and tested by the chi-square test or Fisher’s exact test. Pearson’s correlation was computed between intestinal microbiota and cytokines. p < 0.05 was considered significant. Missing values were not replaced and did not contribute to the analysis of the variable. Experiments were carried out at least twice. Data were analyzed using Prism 8.0 (GraphPad, San Diego, CA, United States).
Accession Number
The sequence data from this study are deposited in the GenBank Sequence Read Archive with the accession number SUB9930073.
Results
Collection of Dietary Data
The data [age, body mass index (BMI), allergy history, and daily food intake] of 85 study participants [40 (47.1%) men and 45 (52.9%) women] are shown in Table 1. The median (IQR) age at study inclusion was 35 (27-48) years in men and 34 (26-43) years in women. Forty-nine (57.6%) participants reported a normal BMI (18.5-23.9 kg/m2).
Table 2 shows the percentages of major-diet components in study participants. A significant difference was registered in the intake of vegetables, soybeans, animal-based food, and condiments. Excessive intake of soybeans (58.18 ± 49.45 vs. 39.34 ± 34.41 g), animal-based food (meat: 220.77 ± 117.58 vs. 108.51 ± 103.15 g; fish and shrimps: 103.75 ± 73.86 vs. 62.61 ± 33.88 g; eggs: 47.20 ± 29.13 vs. 34.20 ± 25.88 g) and condiments (oil: 38.27 ± 16.29 vs. 23.85 ± 9.36 g; salt: 7.86 ± 2.95 vs. 5.20 ± 1.79 g) was common in people suffering from allergy compared with that in participants not suffering from allergy. An inadequate intake of vegetables was common in people suffering from allergy (319.43 ± 125.15 g) compared with that in participants not suffering from allergy (387.83 ± 124.62 g) (Table 2).
Different Diet Influences the Effect of L. paracasei on Allergic Symptoms
To evaluate allergy symptoms in rats, we measured the frequency of nasal rubs and sneezes per rat during the 15 min observation period after the last OVA challenge immediately. Rats in the Model group showed increased nasal rubs (vs. Con group, p < 0.001). With probiotics modulation, the number of nasal rubs decreased (vs. Model group, p < 0.01). High-fat diet intervention caused a significantly higher number of nasal rubs in probiotics-modulation AA rats (p < 0.001), compared with that in the Probio group, along with a reduced nasal rubs in Hfiber group but not significantly (Figures 2A,B). Whereas, the allergic symptoms of rats in Hfiber group was inhibited significantly (vs. Model group, p < 0.001). The incidence of sneezes in rats in all the groups maintained no significance. These results indicated that a high-fiber diet acted synergistically with L. paracasei intervention to alleviate allergic symptoms.

Figure 2. Allergy symptoms resulting from different diets influencing the effect of L. paracasei. (A) Number of nose rubs. (B) Number of sneezes. (C) Altered serum level of OVA-specific IgE. (D) Absolute number of WBCs in BALF 24 h after final challenge with OVA. Each bar represents the mean ± SEM; *p < 0.05, **p < 0.01, and ***p < 0.001.
The level of OVA-specific IgE in serum was investigated to elucidate the effect of different diets on L. paracasei that modulated the allergic response. The serum OVA-specific IgE level was higher in the Model group than in the Con group (p < 0.01). There was a trend toward reducing OVA-specific IgE with high-fiber diet treatment (p = 0.063), although not significantly (Figure 2C).
We counted the number of inflammatory cells obtained from BALF 24 h after the final challenge with OVA (Figure 2D). An increased total number of white blood cells (WBCs) in BALF was detected in the Model group compared with that in the Con group (p < 0.001). Level of WBCs after administered L. paracasei was lower in Probio group, although this difference was not statistically significant. However, when the rats consumed the high-fiber diet, the total number of inflammatory cells reduced markedly compared with that in the Model group (p < 0.05). These data suggested that the total number of WBCs in BALF was inhibited significantly by dietary consumption of fiber, whereas a high-fat diet aggravated the inflammatory response of AA.
Airway Inflammation in L. paracasei-Modulating AA Rats Fed Different Diets
To examine altered inflammation in allergic airways, histological examination of the lung tissue of AA rats was undertaken. Eosinophilic cytoplasm stained red with H&E (Figure 3). The typical pathological features of AA were observed in Model group as compared to Con group, along with discernible damage, edema, and a thickened mucosa. Probiotics treatment was effective in reducing the inflammation. Compared with the Model group, a more robust inflammatory response occurred in the Hfat group: extensive infiltration by eosinophils in peribronchial and alveolar septa, along with markedly thickened alveolar and bronchial walls. However, infiltration of inflammatory cells was suppressed significantly in the Hfiber group.

Figure 3. Histology of H&E-stained lung tissue (original magnification: ×100). Photomicrographs were captured to detect eosinophil infiltration. Eosinophil infiltration was significantly higher in the Hfat group than the other groups. Interestingly, eosinophil inflammation was suppressed dramatically consumption of a high-fiber diet. n = 5-8 rat/group.
Alteration of Cytokine Levels From T Cells by Consumption of Different Diets Influenced L. paracasei Intervention
We measured cytokine levels in BALF and serum after the OVA challenge to further investigate the skewing of Th1/Th2 and Th17/Tregs immune responses after consuming different diets and how this influenced L. paracasei intervention. As shown in Figure 4, discrepancy is apparent in applying the L. paracasei modulating AA when there are different diets intervented. Notably, compared with the Hfat group, IL-4 was dropped significantly in Hfiber group (p < 0.05). The Hfiber group contained apparently lower Th17 cytokine IL-17 level in serum compared with the Model group (p < 0.05). Increased production of IL-17 was observed intervented with high-fat diet, compared with Hfiber group (p < 0.05). Th1 cytokine IFN-ɤ in both BALF and serum were on the trend opposite from IL-4, although the differences were not statistical significant. The ratio of IL-4/IFN-γ was significantly different in Hfat group from that in Hfiber group (P < 0.05) (see Supplementary Figure 1). Based on these results, it was indicated that different diets affect the profile of cytokines in AA during the modulation of probiotics. The high-fiber diet blockaded the allergic inflammation significantly by regulating the levels of multiple inflammatory mediators therein, while the high-fat diet exacerbated it.
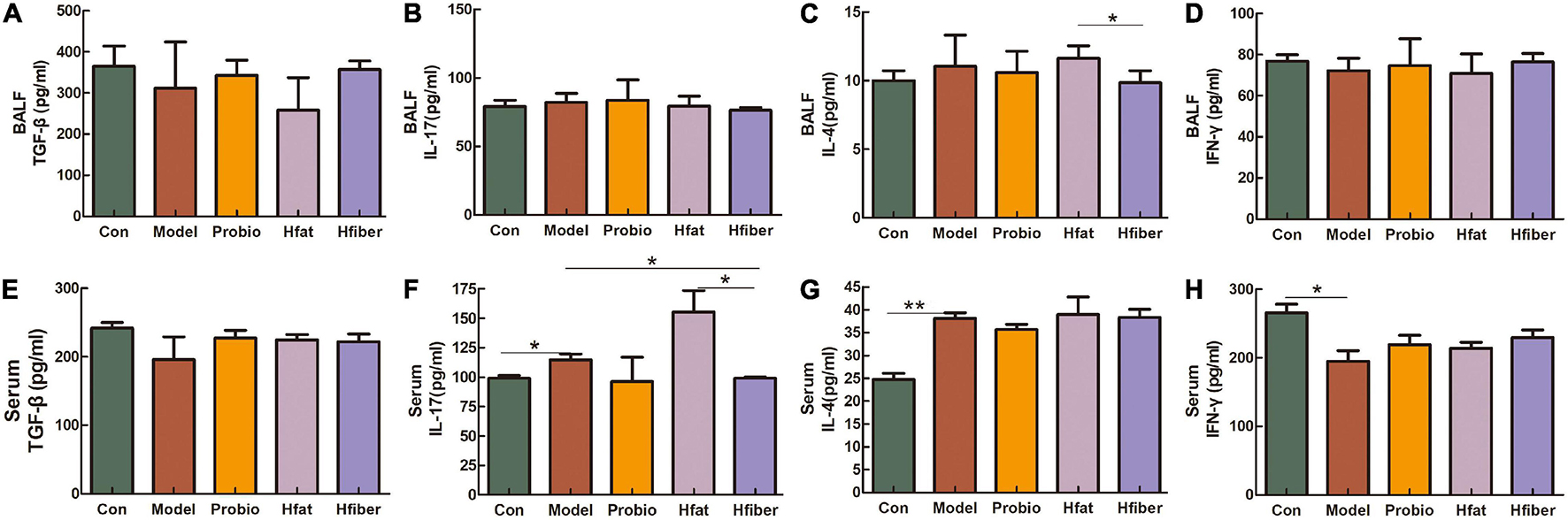
Figure 4. Altered levels of the cytokines after OVA challenge. (A) TGF-β in BALF. (B) IL-17 in BALF. (C) IL-4 in BALF. (D) IFN-γ in BALF. (E) TGF-β in serum. (F) IL-17 in serum. (G) IL-4 in serum. (H) IFN-γ in serum. Data are the mean secretion (pg/mL) ± SEM, n = 4; ∗p < 0.05, ∗∗p < 0.001.
GM Composition of AA Rats Using 16S rRNA Gene Sequencing
A total of 1,915 operational taxonomic units (OTUs) were shared out of sequences obtained from 35 fecal samples. Nineteen phyla, 240 genera, and 162 species of gut microbes were annotated for subsequent analyses.
We wished to evaluate changes in the structure of the microbiota community among groups fed a different diet. Hence, microbial alpha diversity was measured using ACE and Chao1. As a result, we discovered a significantly higher alpha diversity for the Hfat group compared with that in the Probio group (p < 0.01) (Figures 5A,B). Furthermore, ordination with NMDS showed distinct spatial clustering between five groups (stress = 0.142) (Figure 5C).
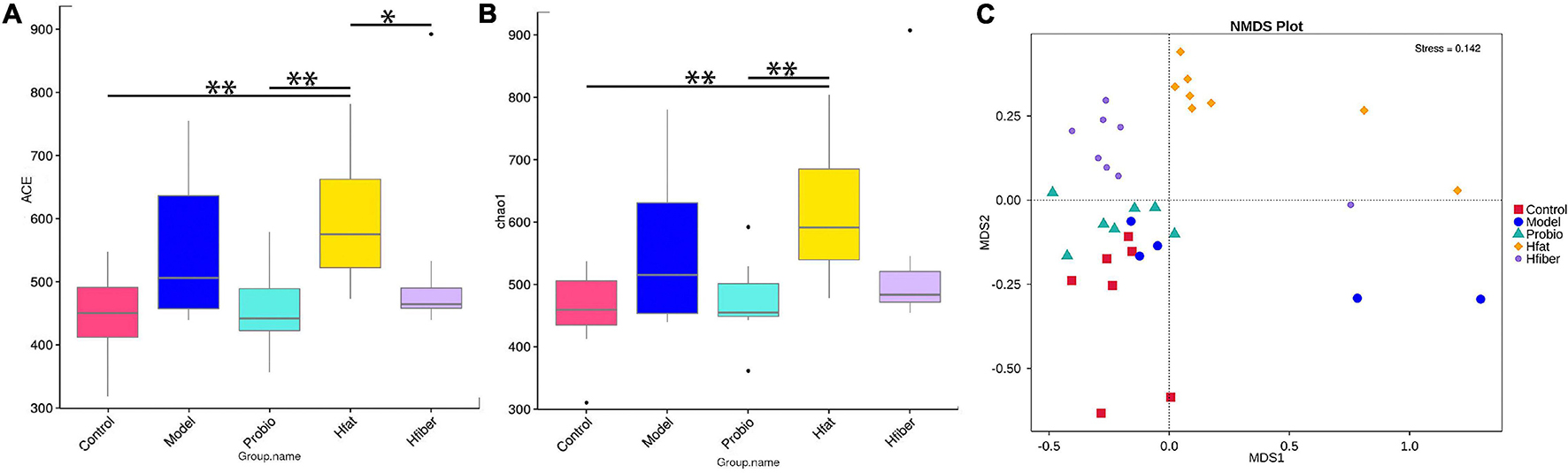
Figure 5. Diversity in microbiota-community structure in different rat groups. (A) ACE indice. (B) Chao1 indice. *p < 0.05, **p < 0.01. (C) NMDS. Stress < 0.2, p < 0.05.
To further illustrate the differences in gut microbiota composition among groups, we applied the LEfSe method to discover biomarkers in high-dimensional data and reveal genomic characteristics (Figure 6). Differentially enriched bacterial colonizers with LDA > 4 were identified among the five groups. Phylum Actinobacteria, families Bifidobacteriaceae and Peptostreptococcaceae, genera Bifidobacterium, Turicibacter, and Romboutsia were enriched in Con group. Family Erysipelotrichaceae and genus Dubosiella were enriched in Model group. Abundances of family Lactobacillaceae and genus Lactobacillus were enriched in Probio group. Abundances of phylum Firmicutes and Proteobacteria, families Lachnospiraceae, Ruminococcaceae and Desulfovibrionaceae, genera Blautia, unidentified_Ruminococcaceae, unidentified_Clostridiales and Oscillibacter were enriched in Hfat group. Biomarkers in Hfiber group including phylum Bacteroidetes, families Muribaculaceae, Tannerellaceae, Prevotellaceae, Enterococcaceae, genera Allobaculum, Parabacteroides, and Enterococcus.
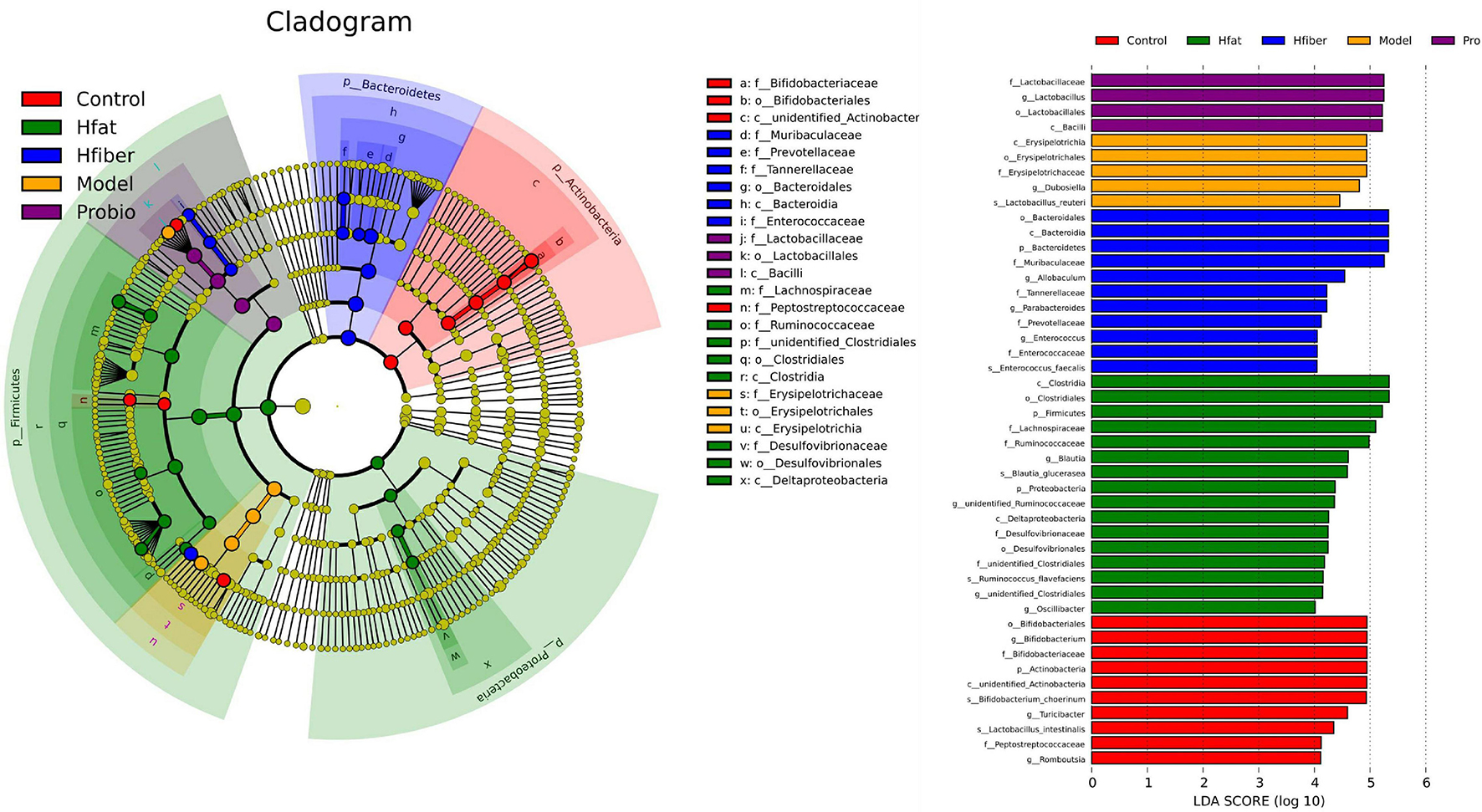
Figure 6. Gut-microbiota comparison analyzed by LEfSe. In the cladogram, changes in the relative abundance of bacterial taxa identified by LEfSe are shown in phylogenetic trees from the phylum to the genus (from the inner to the outer ring, respectively). In the cladogram, the size of the node circle is proportional to the relative abundance of taxa. The node size corresponds to the average relative abundance of the OTU. Yellow indicates no significant difference between groups, and other colors (red, blue, purple, green, and orange) denote that OTUs have a significant difference between groups. Histogram of the LDA scores for differentially abundant genera. LDA scores were calculated by LEfSe using linear discriminant analysis to assess the effect size of each differentially abundant bacterial taxa. Only taxa meeting LDA > 4 are shown.
To determine whether specific bacteria were differentially abundant along with the probiotics modulation intervented by different diets, we conducted a further analyses. After the AA model had been established, family Erysipelotrichaceae and genera Dubosiella and Paraprevotella were more abundant, compared with that in the Con group (see Supplementary Figure 2). After intervention with L. paracasei, the dominant bacteria changed into phylum Bacteroidetes, and there were significant decrease in family Erysipelotrichaceae and genus Dubosiella (see Supplementary Figure 3). Upon consumption of different diets, the intestinal community altered significantly. In the Hfat group, phylum Proteobacteria, families unidentified_Clostridiales, Desulfovibrionaceae, Ruminococcaceae and Lachnospiraceae, and genera Anaerotruncus, Oscillibacter, Candidatus_Soleaferrea and Blautia were dominant, while phylum Actinobacteria, families Lactobacillaceae, Bifidobacteriaceae and Muribaculaceae, genera Lactobacillus, Bifidobacterium, and Turicibacter decreased significantly (see Supplementary Figure 4). In the Hfiber group, the phylum Bacteroidetes, families Tannerellaceae, Lachnospiraceae and Muribaculaceae, genera Angelakisella, Parabacteroides, and Allobaculum were in relatively high abundance, phyla Firmicutes and Actinobacteria, families Lactobacillaceae, Bifidobacteriaceae, and Corynebacteriaceae, genera Lactobacillus Bifidobacterium, Turicibacter, and Corynebacterium decreased (see Supplementary Figure 5). Taken together, these data demonstrated that different diets promoted distinct microbial communities that influenced L. paracasei-modulated AA.
Correlation Analysis for the Intestinal Microbiota and Cytokine Expression in BALF
The Pearson correlation coefficients between the predominant intestinal microbiota and cytokines in BALF were displayed in Table 3. The BALF level of IL-4 was positively correlated with the abundance of Lachnospiraceae, Deltaproteobacteria, Desulfovibrionaceae, and Clostridiales, whereas TGF-β was negatively correlated with the abundance of Deltaproteobacteria and Desulfovibrionaceae, respectively (p < 0.05). The BALF level of IFN-ɤ was negatively associated with Erysipelotrichales abundance (r = −0.483, p = 0.031). A strong positive association and high significance between the IL-17 and abundance of Turicibacter, Peptostreptococcaceae, and Romboutsia, respectively, were found (p < 0.05).
Discussion
Diet affects the GM, and dietary habits can impact overall intestinal health (Nakaji et al., 2002; Gophna, 2011; Wu et al., 2011; Bernaud and Rodrigues, 2013; Trompette et al., 2014). However, little is known of the role of diet in probiotic-modulating AA. One might speculate an interplay of diet, intestinal microflora, and immune cells. The diet pattern could be critical in regulating the effect of probiotics given to people suffering from AA.
The dietary patterns of 85 individuals were obtained. We found that people suffering from AA had unbalanced diets and inadequate intake of vegetables, and excessive intake of animal-based food was typical. According to a World Health Report from 2014, low intake of vegetables and fruit is associated with ischemic heart disease, gastrointestinal cancer, and stroke (World Health Organization (WHO), 2014). Consistent with the notion that a plant-rich diet is associated with positive health outcomes and reduced risk of disease, Alison et al. highlighted the importance of a high-fiber diet in protection against asthma development (Thorburn et al., 2015). Saadeh et al. (2015) observed that consumption of fast food and butter intake were associated with an increased prevalence of asthma symptoms among atopic children. A high-fat diet has been shown to increase airway inflammation in asthma (Wood et al., 2011). Hence, diet could be an environmental factor that influences how probiotics prevent AA.
We monitored systemic symptoms (nasal rubbing and sneezing), cytokines levels, inflammatory cell infiltration, and fecal bacterial composition in a dietary-intervention study of AA (induced by OVA) rats with modulation by L. paracasei. We discovered that different diets influenced the effect of L. paracasei on modulating AA. Moreover, a high fiber diet plus L. paracasei as a rescue medication showed a synergistic effect and led to a decreased frequency of rubbing and sneezes, whereas a high-fat diet exacerbated these symptoms. A reduced serum level of OVA-specific IgE and total counts of inflammatory cells in BALF in the Hfiber group suggested an instinctive response to remission of allergic inflammation, but the group that consumed a high-fat diet showed the opposite results. IgE on the surface of immune cells binds to specific airway allergens followed by IgE cross-linking, cell activation, and release of preformed mediators (Komi and Bjermer, 2019; Salomonsson et al., 2019; Liu et al., 2020). In this regard, the lower IgE level also reflected attenuation of the Th2 immune response and the immune tolerance to allergic antigens (Ciprandi et al., 2015; Strokes and Casale, 2015). AA is characterized by eosinophils accumulation. The decreased number of eosinophils infiltrating the lungs was a response to the inhibition of allergic inflammation by consumption of a high-fiber diet. A high-fat diet induced eosinophil accumulation in the lungs of AA rats.
Th2 cells have crucial roles in AA pathogenesis, and imbalance of Th1/Th2 cells and disturbed balance of Th17/Treg cells has been reported in asthma patients. Hence, to discriminate the state of Th1/Th2 and Th17/Treg subsets in allergic airways, we measured the levels of IFN-ɤ (Th1 cytokine), IL-4 (Th2 cytokine), IL-17 (Th17 cytokine), and TGF-β (Treg cytokine) by ELISA. The Probio group given a high-fiber diet inhibited expression of IL-4 and IL-17 and increased expression of IFN-ɤ, but the group that consumed a high-fat diet had the opposite results. Th2 cytokines are believed to regulate IgE synthesis, and eosinophil numbers/activity are thought to play a significant part in driving AA pathogenesis (Dent et al., 1990; Savelkoul et al., 1991). IL-4 can upregulate expression of chemotactic factors such as eotaxins to promote eosinophil infiltration. IL-17 can drive airway remodeling (Quan et al., 2012). Indeed, our study found that the Hfiber diet assists the L. paracasei skewed the immune balance toward Th1, with increased IFN-ɤ and conversely decreased IL-4, in commitment with the previous presentation of reduced IgE and fewer eosinophils infiltration. Alteration in the balance of Th1/Th2 cytokines is an essential indicator of functional changes in suppressing the aberrant immune response in allergic diseases (Skapenko and Schulze-Koops, 2007; Cho et al., 2009). Although increased expression of IFN-ɤ has been found in individuals with severe asthma and acute exacerbations (Kumar et al., 2006; Barnes, 2009), there is evidence that probiotics promote IFN-ɤ production to reduce allergic inflammation (Giudice et al., 2010). Higher expression of IFN-ɤ in the Hfiber group with decreased expression of IL-4 compared with that in the Hfat group, and the ratio of IL-4/IFN-ɤ implied skewing of the balance of Th1/Th2 cells. The balance between Th17 and Tregs cells also suggests a crucial role in asthma pathogenesis (Vroman et al., 2018; Wang et al., 2018; Xu et al., 2018). Various studies have indicated that the homeostatic balance between Tregs and Th17 cells was altered markedly in asthma exacerbations, and correlates with asthma severity (Zou et al., 2018). TGF-β is produced by Tregs, which have been implicated to inhibit the immune response. They suppress effector T cells of Th1 or Th2 phenotypes. Therefore, increased expression of TGF-β in BALF in the Hfiber group seems rational.
Several studies have associated a changed intestinal microbiota with the etiology of various diseases, and changes in gut microflora composition, in response to diet change (Kau et al., 2011; David et al., 2014). We discovered that consumption of different diet led to alterations in the structure of microbial communities in the gut as well as the composition of intestinal bacteria as evidenced by NMDS (Giloteaus et al., 2016). There was a significant difference in the alpha diversity of microbiota in different diet groups, and the bacterial diversity of the Hfiber group was decreased dramatically.
We revealed that a diet rich in fiber assisted the way that L. paracasei modified the GM. This finding corroborates the data from a study by Salonen et al. (2014), who reported that a diet high in fiber was associated with decreased bacterial diversity relative to that of other diets. Comparison of 16S rRNA gene sequencing among groups revealed that a high-fiber diet significantly improved GM structure by enriching bacteria of the phylum Bacteroidetes, as reported by Trompette et al. (2014). Consumption of a high-fat diet enhanced the proportions of Proteobacteria and Firmicutes, which indicated the enrichment of specific bacteria in the colon is diet-specific (Filippo et al., 2010; Walker et al., 2011; Campo et al., 2014; Cruz et al., 2014; Ramos-Romero et al., 2018). Phylum Bacteroidetes was increased markedly in the Hfiber group. Salyers and coworkers showed that the bacteria of phylum Bacteroidetes have the functions of carbohydrate fermentation, polysaccharide metabolism, and maintaining the normal physiological function of the intestinal tract (Salyers, 1984). The influence of a high-fat diet led to significant induction of Clostridiales, Lachnospiraceae, Ruminococcaceae, Deltaproteobacteria, and Desulfovibrionaceae. Studies have shown that Clostridiales can be pathogenic, leading to infectious diseases or mild cognitive impairment in mice (Antharam et al., 2013; Vogt et al., 2017). The members of the family Lachnospiraceae are enriched by a low-fiber Western-style diet, are associated with inflammatory diseases, and have been reported to protect against allergy by modulating the immune system (Png et al., 2010; Pascal et al., 2018). The relative abundance of bacteria of the family Ruminococcaceae is lower in IgE-associated eczema compared with that in people not suffering from allergies. Enriched abundance of Deltaproteobacteria is related to AA in mice (Hirota et al., 2019). Moreover, bacteria of the family Desulfovibrionaceae (which are opportunistic pathogens) have been linked to inflammatory diseases of the gut and chronic disorders. Hence, the alterations induced by a high-fat diet suggest inflammation exacerbation.
Our correlation analysis of intestinal bacteria and cytokine expression (section “Correlation Analysis for the Intestinal Microbiota and Cytokine Expression in BALF”) demonstrated that GM alterations might be associated with inflammation during AA. Therefore, L. paracasei, with the assistance of a high-fiber diet, ameliorated allergy symptoms to a greater extent than use of L. paracasei alone in an inflammatory-modulation manner. Thus, a high fiber diet seems to support the effect of AA medication as an add-on therapy.
Our study had two main limitations. First, our findings are limited to an AA model induced by OVA; the effect a high-fiber or high-fat diet on an AA sensitized by other antigens (e.g., house dust mite) is not known. Second, associations between the most relevant taxa and a high-fiber diet or high-fat diet, respectively, were not validated by real-time reverse transcription-quantitative PCR.
Conclusion
We provided new insights into consumption of different diets influenced the effect of L. paracasei on suppressing AA. In particular, the intestinal microbiota altered by different dietary patterns were associated with allergic inflammation.
Data Availability Statement
The datasets presented in this study can be found in online repositories. The names of the repository/repositories and accession number(s) can be found in the article/Supplementary Material.
Ethics Statement
The studies involving human participants were reviewed and approved by the Ethics Committee of Dalian Medical University. The patients/participants provided their written informed consent to participate in this study. The animal study was reviewed and approved by the Animal Ethics Committee of Dalian Medical University.
Author Contributions
SW conceived and designed the experiments and supervised. AX, JS, and SL performed the experiments. JS and YL analyzed the data. AX wrote the manuscript. SW and LT reviewed the manuscript. All authors have read and given approval to the final version of the manuscript.
Funding
This work was funded by National Natural Science Foundation of China (No. 81370113).
Conflict of Interest
The authors declare that the research was conducted in the absence of any commercial or financial relationships that could be construed as a potential conflict of interest.
Publisher’s Note
All claims expressed in this article are solely those of the authors and do not necessarily represent those of their affiliated organizations, or those of the publisher, the editors and the reviewers. Any product that may be evaluated in this article, or claim that may be made by its manufacturer, is not guaranteed or endorsed by the publisher.
Supplementary Material
The Supplementary Material for this article can be found online at: https://www.frontiersin.org/articles/10.3389/fmicb.2021.737622/full#supplementary-material
Supplementary Figure 1 | The ratio of IL-4/IFN-γ.
Supplementary Figure 2 | Gut microbiota comparisons between Con group and Model group.
Supplementary Figure 3 | Gut microbiota comparisons between Model group and Probio group.
Supplementary Figure 4 | Gut microbiota comparisons between Probio group and Hfat group.
Supplementary Figure 5 | Gut microbiota comparisons between Probio group and Hfiber group.
Supplementary Table 1 | Diet composition.
Footnote
References
Abrahamsson, T. R., Jakobsson, H. E., Andersson, A. F., Björkstén, B., Engstrand, L., and Jenmalm, M. C. (2014). Low gut microbiota diversity in early infancy precedes asthma at school age. Clin. Exp. Allergy. 44, 842–850.
Antharam, V. C., Li, E. C., Ishmael, A., Sharma, A., Mai, V., Rand, K. H., et al. (2013). Intestinal dysbiosis and depletion of butyrogenic bacteria in Clostridium difficile infection and nosocomial diarrhea. J. Clin. Microbiol. 51, 2884–2892. doi: 10.1128/jcm.00845-13
Atarashi, K., Tanoue, T., Shima, T., Imaoka, A., Kuwahara, T., Momose, Y., et al. (2011). Induction of colonic regulatory T cells by indigenous Clostridium species. Science 331, 337–341. doi: 10.1126/science.1198469
Barnes, P. J. (2009). The cytokine network in chronic obstructive pulmonry disease. Am. J. Respir. Cell Mol. Biol. 41, 631–638. doi: 10.1165/rcmb.2009-0220tr
Bernaud, F. S. R., and Rodrigues, T. C. (2013). Dietary fiber – adequate intake and effects on metabolism health. Arq. Bras. Endocrinol. Metabol. 57, 397–405. doi: 10.1590/s0004-27302013000600001
Campo, R., Garriga, M., Pérez-Aragón, A., Guallarte, P., Lamas, A., Máiz, L., et al. (2014). Improvement of digestive health and reduction in protebobacterial populations in the gut microbiota of cystic fibrosis patients using a Lactobacillus reuteri probiotic preparation: a double blind prospective study. J. Cyst. Fibros. 13, 716–722. doi: 10.1016/j.jcf.2014.02.007
Cho, K.-S., Park, H.-K., Park, H.-Y., Jung, J. S., Jeon, S.-G., Kim, Y.-K., et al. (2009). IFATS collection: immunomodulatory effects of adipose tissue-derived stem cells in an allergic rhinitis mouse model. Stem Cells 27, 259–265. doi: 10.1634/stemcells.2008-0283
Choi, S.-P., Oh, H.-N., Choi, C.-Y., Ahn, H., Yun, H. S., Chung, Y. M., et al. (2018). Oral administration of Lactobacillus plantarum CJLP133 and CJLP243 alleviates birch pollen-induced allergic rhinitis in mice. J. Appl. Microbiol. 124, 821–828. doi: 10.1111/jam.13635
Ciprandi, G., Marseglia, G. L., Castagnoli, R., Valsecchi, C., Tagliacarne, C., Caimmi, S., et al. (2015). From IgE to clinical trials of allergic rhinitis. Expert Rev. Clin. Immunol. 11, 1321–1333.
Colombo, D., Zagni, E., Ferri, F., and Canonica, G. W. Proxima study centers. (2019). Gender differences in asthma perception and its impact on quality of life: a post hoc analysis of the PROXIMA(Patient Reported Outcomes and Xolair® In the Management of Asthma) study. Allergy Asthma Clin. Immunol. 15:65. doi: 10.1186/s13223-019-0380-z
Cruz, P. D., Kang, S., Wagner, J., Buckley, M., Sim, W. H., Prideaus, L., et al. (2014). Specific mucosa-associated microbiota in Crohn’s disease at the time of resection are associated with early disease recurrence: a Pilot Study. J. Gastroenterol. Hepatol. 30, 268–278. doi: 10.1111/jgh.12694
David, L. A., Maurice, C. F., Carmody, R. N., Gootenberg, D. B., Button, J. E., Wolfe, B. E., et al. (2014). Diet rapidly and reproducibly alters the human gut microbiome. Nature 505, 559–563. doi: 10.1038/nature12820
Dent, L. A., Strath, M., Mellor, A. L., and Sanderson, C. J. (1990). Eosinophilia in transgenic mice expressing interleukin 5. J. Exp. Med. 172, 1425–1431. doi: 10.1084/jem.172.5.1425
Dzidic, M., Abrahamsson, T. R., Artacho, A., Björkstén, B., Collado, M. C., Mira, A., et al. (2017). Aberrant IgA responses to the gut microbiota during infancy precede asthma and allergy development. J. Allergy Clin. Immunol. 139, 1017–1025. doi: 10.1016/j.jaci.2016.06.047
Ellwood, P., Asher, M. I., Björkstén, B., Burr, M., Pearce, N., and Robertson, C. F. (2001). Diet and asthma, allergic rhinoconjunctivitis and atopic eczema symptom prevalence: an ecological analysis of the International Study of Asthma and Allergies in Childhood(ISAAC) data. Eur. Respir. J. 17, 436–443. doi: 10.1183/09031936.01.17304360
Filippo, C. D., Cavalieri, D., Paola, M. D., Ramazzotti, M., Poullet, J. B., Massart, S., et al. (2010). Impact of diet in shaping gut microbiota revealed by a comparative study in children from Europe and rural Africa. Proc. Natl. Acad. Sci. U. S. A. 107, 14691–14696. doi: 10.1073/pnas.1005963107
Fiocchi, A., Burks, W., Bahna, S. L., Bielory, L., Boyle, R. J., Cocco, R., et al. (2012). Clinical use of probiotics in pediatric allergy(CUPPA): a World Allergy Organization position paper. World Allergy Organ J. 5, 148–167. doi: 10.1097/wox.0b013e3182784ee0
Frei, R., Lauener, R. P., Crameri, R., and O’Mahony, L. (2012). Microbiota and dietary interactions: an update to the hygiene hypothesis? Allergy 67, 451–461. doi: 10.1111/j.1398-9995.2011.02783.x
Fujiwara, D., Inoue, S., Wakabayashi, H., and Fujii, T. (2004). The anti-allergic effects of lactic acid bacteria are strain dependent and mediated by effects on both Th1/Th2 cytokine expression and balance. Int. Arch. Allergy Immunol. 135, 205–215. doi: 10.1159/000081305
Giloteaus, L., Goodrich, J. K., Walters, W. A., Levine, S. M., Ley, R. E., and Hanson, M. R. (2016). Reduced diversity and altered composition of the gut microbiome in individuals with myalgic encephalomyelitis/chronic fatigue syndrome. Microbiome 4:30. doi: 10.1186/s40168-016-0171-4
Giudice, M. M., Leonardi, S., Maiello, N., and Brunese, F. P. (2010). Food allergy and probiotics in childhood. J. Clin. Gastroenterol. 44, S22–S25. doi: 10.1097/mcg.0b013e3181e102a7
Gollwitzer, E. S., Saglani, S., Trompette, A., Yadava, K., Sherburn, R., McCoy, K. D., et al. (2014). Lung microbiota promotes tolerance to allergens in neonates via PD-L1. Nat. Med. 20, 642–647. doi: 10.1038/nm.3568
Gophna, U. (2011). Microbiology. The guts of dietary habits. Science 334, 45–46. doi: 10.1126/science.1213799
Grimshaw, K. E. C., Maskell, J., Oliver, E. M., Morris, R. C. G., Foote, K. D., Mills, E. N. C., et al. (2014). Diet and food allergy development during infancy: birth cohort study findings using prospective food diary data. J. Allergy Clin. Immunol. 133, 511–519. doi: 10.1016/j.jaci.2013.05.035
Herbst, T., Sichelstiel, A., Schär, C., Yadava, K., Bürki, K., Cahenzli, J., et al. (2011). Dysregulation of allergic airway inflammation in the absence of microbial colonization. Am. J. Respir. Crit. Care Med. 184, 198–205. doi: 10.1164/rccm.201010-1574OC
Hirota, R., Ohya, Y., Yamamoto-Hanada, K., Fukutomi, Y., Muto, G., Ngatu, N. R., et al. (2019). Triclosan-induced alteration of gut microbiome and aggravation of asthmatic airway response in aeroallergen-sensitized mice. Allergy 74, 996–999. doi: 10.1111/all.13639
Hou, J. K., Abraham, B., and El-Serag, H. (2011). Dietary intake and risk of developing inflammatory bowel disease: a systematic review of the literature. Am. J. Gastroenterol. 106, 563–573. doi: 10.1038/ajg.2011.44
Jensen, M. P., Meldrum, S., Taylor, A. L., Dunstan, J. A., and Prescott, S. L. (2012). Early probiotic supplementation for allergy prevention: long-term outcomes. J. Allergy Clin. Immunol. 130, 1209–1211. doi: 10.1016/j.jaci.2012.07.018
Julia, V., Macia, L., and Dombrowicz, D. (2015). The impact of diet on asthma and allergic diseases. Nat. Rev. Immunol. 15, 308–322. doi: 10.1038/nri3830
Kau, A. L., Ahern, P. P., Griffin, N. W., Goodman, A. L., and Gordon, J. I. (2011). Human nutrition, the gut microbiome and the immune system. Nature 474, 327–336. doi: 10.1038/nature10213
Komi, D. E. A., and Bjermer, L. (2019). Mast cell-mediated orchestration of the immune responses in human allergic asthma: current insights. Clin. Rev. Allergy Immunol. 56, 234–247. doi: 10.1007/s12016-018-8720-1
Kuitunen, M., Kukkonen, K., Juntunen-Backman, K., Korpela, R., Poussa, T. T., Haahtela, T., et al. (2009). Probiotics prevent IgE-associated allergy until age 5 years in cesarean-delivered children but not in the total cohort. J. Allergy Clin. Immunol. 123, 335–341. doi: 10.1016/j.jaci.2008.11.019
Kumar, R. K., Webb, D. C., Herbert, C., and Foster, P. S. (2006). Interferon-gamma as a possible target in chronic asthma. Inflamm. Allergy Drug Targets 5, 253–256. doi: 10.2174/187152806779010909
Lin, C.-H., Tseng, C.-Y., and Chao, M.-W. (2020). Administration of Lactobacillus paracasei HB89 mitigates PM2.5-induced enhancement of inflammation and allergic airway response in murine asthma model. PLoS One 15:e0243062. doi: 10.1371/journal.pone.0243062
Liu, H., Tan, J., Liu, J., Feng, H., and Pan, D. (2020). Altered mast cell activity in response to rhinovirus infection provides novel insight into asthma. J. Asthma 57, 459–467. doi: 10.1080/02770903.2019.1585870
Makino, T., Yamashita, M., Takeuchi, N., Kabuki, T., Hattori, M., and Yoshida, T. (2019). Lactobacillus helveticus SBT2171 alleviates allergic symptoms in a murine model for pollen allergy. Biosci. Biotechnol. Biochem. 83, 2298–2306. doi: 10.1080/09168451.2019.1654847
Manzel, A., Muller, D. N., Hafler, D. A., Erdman, S. E., Linker, R. A., and Kleinewietfeld, M. (2014). Role of “western diet” in inflammatory autoimmune diseases. Curr. Allergy Asthma Rep. 14:404. doi: 10.1007/s11882-013-0404-6
Maslowski, K. M., Vieira, A. T., Ng, A., Kranich, J., Sierro, F., Yu, D., et al. (2009). Regulation of inflammatory responses by gut microbiota and chemoattractant receptor GPR43. Nature 461, 1282–1286. doi: 10.1038/nature08530
Myles, I. A. (2014). Fast food fever: reviewing the impacts of the Western diet on immunity. Nutr. J. 13:61. doi: 10.1186/1475-2891-13-61
Nakaji, S., Sugawara, K., Saito, D., Yoshioka, Y., MacAuley, D., Bradley, T., et al. (2002). Trends in dietary fiber intake in Japan over the last century. Eur. J. Nutr. 41, 222–227. doi: 10.1007/s00394-002-0379-x
Pascal, M., Perez-Gordo, M., Caballero, T., Escribese, M. M., Longo, M. N. L., Luengo, O., et al. (2018). Microbiome and allergic diseases. Front. Immunol. 9:1584. doi: 10.3389/fimmu.2018.01584
Png, C. W., Lindén, S. K., Gilshenan, K. S., Zoetendal, E. G., McSweeney, C. S., Sly, L. I., et al. (2010). Mucolytic bacteria with increased prevalence in IBD mucosa augment in vitro utilization of mucin by other bacteria. Am. J. Gastroenterol. 105, 2420–2428. doi: 10.1038/ajg.2010.281
Protudjer, J. L. P., Sevenhuysen, G. P., Ramsey, C. D., Kozyrskyj, A. L., and Becker, A. B. (2012). Low vegetable intake is associated with allergic asthma and moderate-to-severe airway hyperresponsiveness. Pediatr. Pulmonol. 47, 1159–1169. doi: 10.1002/ppul.22576
Quan, S.-H., Zhang, Y.-L., Han, D. H., Iwakura, Y., and Rhee, C.-S. (2012). Contribution of interleukin 17A to the development and regulation of allergic inflammation in a murine allergic rhinitis model. Ann. Allergy Asthma Immunol. 108, 342–350. doi: 10.1016/j.anai.2012.02.014
Ramos-Romero, S., Hereu, M., Atienza, L., Casas, J., Jáuregui, O., Amézqueta, S., et al. (2018). Mechanistically different effects of fat and sugar on insulin resistance, hypertension, and gut microbiota in rats. Am. J. Physiol. Endocrinol. Metab. 314, E552–E563. doi: 10.1152/ajpendo.00323.2017
Saadeh, D., Salameh, P., Caillaud, D., Charpin, D., Blay, F. D., Kopferschmitt, C., et al. (2015). Prevalence and association of asthma and allergic sensitization with dietary factors in schoolchildren: data from the french six cities study. BMC Public Health 15:993. doi: 10.1186/s12889-015-2320-2
Salomonsson, M., Malinovschi, A., Kalm-Stephens, P., Dahlin, J. S., Janson, C., Alving, K., et al. (2019). Circulating mast cell progenitors correlate with reduced lung function in allergic asthma. Clin. Exp. Allergy 49, 874–882. doi: 10.1111/cea.13388
Salonen, A., Lahti, L., Salojärvi, J., Holtrop, G., Korpela, K., Duncan, S. H., et al. (2014). Impact of diet and individual variation on intestinal microbiota composition and fermentation products in obese men. ISME J. 8, 2218–2230. doi: 10.1038/ismej.2014.63
Salyers, A. A. (1984). Bacteroides of the human lower intestinal tract. Annu. Rev. Microbiol. 38, 293–313. doi: 10.1146/annurev.mi.38.100184.001453
Savelkoul, H. F., Seymour, B. W., Sullivan, L., and Coffman, R. L. (1991). IL-4 can correct defective IgE production in SJA/9 mice. J. Immunol. 146, 1801–1805.
Schabussova, I., Hufnagl, K., Tang, M. L. K., Hoflehner, E., Wagner, G. L., Nutten, S., et al. (2012). Perinatal maternal administration of Lactobacillus paracasei NCC 2461 prevents allergic inflammation in a mouse model of birch pollen allergy. PLoS One 7:e40271. doi: 10.1371/journal.pone.0040271
Shinmei, Y., Yano, H., Kagawa, Y., Izawa, K., Akagi, M., Inoue, T., et al. (2009). Effect of Brazilian propolis on sneezing and nasal rubbing in experimental allergic rhinitis of mice. Immunopharmacol. Immunotoxicol. 31, 688–693. doi: 10.3109/08923970903078443
Skapenko, A., and Schulze-Koops, H. (2007). Analysis of Th1/Th2 T-cell subsets. Methods Mol. Med. 136, 87–96. doi: 10.1007/978-1-59745-402-5_7
Strokes, J. R., and Casale, T. B. (2015). The use of anti-IgE therapy beyond allergic asthma. J. Allergy Clin. Immunol. Pract. 3, 162–166. doi: 10.1016/j.jaip.2014.10.010
Thorburn, A. N., Macia, L., and Mackay, C. R. (2014). Diet, metabolites, and “western-lifestyle” inflammatory diseases. Immunity 40, 833–842. doi: 10.1016/j.immuni.2014.05.014
Thorburn, A. N., McKenzie, C. I., Shen, S., Stanley, D., Macia, L., Masson, L. J., et al. (2015). Evidence that asthma is a developmental origin disease influenced by maternal diet and bacterial metabolites. Nat. Commun. 6:7320. doi: 10.1038/ncomms8320
Trompette, A., Gollwitzer, E. S., Yadava, K., Sichelstiel, A. K., Sprenger, N., Ngom-Bru, C., et al. (2014). Gut microbiota metabolism of dietary fiber influences allergic airway disease and hematopoiesis. Nat. Med. 20, 159–166. doi: 10.1038/nm.3444
Tsabouri, S., Priftis, K. N., Chaliasos, N., and Siamopoulou, A. (2014). Modulation of gut microbiota downregulates the development of food allergy in infancy. Allergol. Immunopathol. 42, 69–77. doi: 10.1016/j.aller.2013.03.010
Vogt, N. M., Kerby, R. L., Harding, S., Merluzzi, A. P., Koenig, L., Beilfuss, M., et al. (2017). Gut microbiome alterations in Alzheimer’s disease and relationship with Csf Biomarkers. Alzheimers Dement. 13:563. doi: 10.1016/j.jalz.2017.07.172
Vroman, H., Bergen, I. M., Hulst, J. A. C., Nimwegen, M., Uden, D., Schuijs, M. J., et al. (2018). TNF-α-induced protein 3 levels in lung dentritic cells instruct TH2 or TH17 cell differentiation in eosinophilic or neutrophilic asthma. J. Allergy Clin. Immunol. 141, 1620–1621. doi: 10.1016/j.jaci.2017.08.012
Walker, A. W., Ince, J., Duncan, S. H., Webster, L. M., Holtrop, G., Ze, X., et al. (2011). Dominant and diet-responsive groups of bacteria within the human colonic microbiota. ISME J. 5, 220–230. doi: 10.1038/ismej.2010.118
Wang, L., Li, P., Tang, Z., Yan, X., and Feng, B. (2016). Structural modulation of the gut microbiota and the relationship with body weight: compared evaluation of liraglutide and saxagliptin treatment. Sci. Rep. 6:33251. doi: 10.1038/srep33251
Wang, L., Wan, H., Tang, W., Ni, Y., Hou, X., Pan, L., et al. (2018). Critical roles of adenosine A2A receptor in regulating the balance of Treg/Th17 cells in allergic asthma. Clin. Respir. J. 12, 149–157. doi: 10.1111/crj.12503
Wang, X., Hui, Y., Zhao, L., Hao, Y., and Guo, H. (2017). Oral administration of Lactobacillus paracasei L9 attenuates PM2.5-induced enhancement of airway hyperresponsiveness and allergic airway response in murine model of asthma. PLoS One 12:e0171721. doi: 10.1371/journal.pone.0171721
West, C. E., Hammarström, M.-L., and Hernell, O. (2013). Probiotics in primary prevention of allergic disease - follow-up at 8-9 years of age. Allergy 68, 1015–1020. doi: 10.1111/all.12191
Wood, L. G., Garg, M. L., and Gibson, P. G. (2011). A high-fat challenge increases airway inflammation and impairs bronchodilator recovery in asthma. J. Allergy Clin. Immunol. 127, 1133–1140. doi: 10.1016/j.jaci.2011.01.036
World Health Organization (WHO). (2014). Promoting Fruit and Vegetable Consumption Around the World. Switzerland: WHO.
Wu, G. D., Chen, J., Hoffmann, C., Birringer, K., Chen, Y.-Y., and Keilbaugh, S. A. (2011). Linking long-term dietary patterns with gut microbial enterotypes. Science 334, 105–108. doi: 10.1126/science.1208344
Xu, L., Sun, W. J., Jia, A. J., Qiu, L. L., Xiao, B., Mu, L., et al. (2018). MBD2 regulates differentiation and function of Th17 cells in neutrophils-dominant asthma via HIF-1 alpha. J. Inflamm. 15:15. doi: 10.1186/s12950-018-0191-x
Zhang, B., Sun, W., Yu, N., Sun, J., Yu, X., Li, X., et al. (2018). Anti-diabetic effect of baicalein is associated with the modulation of gut microbiota in streptozotocin and high-fat-diet induced diabetic rats. J. Funct. Foods 46, 256–267. doi: 10.1016/j.jff.2018.04.070
Zhang, Z., Shi, L., Pang, W., Liu, W., Li, J., Wang, H., et al. (2016). Dietary fiber intake regulates intestinal microflora and inhibits ovalbumin-induced allergic airway inflammation in a mouse model. PLoS One 11:e0147778. doi: 10.1371/journal.pone.0147778
Keywords: diet pattern, intestinal microbiota, Lactobacillus paracasei, allergic asthma, airway inflammation
Citation: Xie A, Song J, Lu S, Liu Y, Tang L and Wen S (2021) Influence of Diet on the Effect of the Probiotic Lactobacillus paracasei in Rats Suffering From Allergic Asthma. Front. Microbiol. 12:737622. doi: 10.3389/fmicb.2021.737622
Received: 07 July 2021; Accepted: 31 August 2021;
Published: 27 September 2021.
Edited by:
Tingtao Chen, Nanchang University, ChinaReviewed by:
Hong Wei, Army Medical University, ChinaJiaming Liu, Wenzhou Medical University, China
Longxian Lv, Zhejiang University, China
Copyright © 2021 Xie, Song, Lu, Liu, Tang and Wen. This is an open-access article distributed under the terms of the Creative Commons Attribution License (CC BY). The use, distribution or reproduction in other forums is permitted, provided the original author(s) and the copyright owner(s) are credited and that the original publication in this journal is cited, in accordance with accepted academic practice. No use, distribution or reproduction is permitted which does not comply with these terms.
*Correspondence: Shu Wen, c2h1d2VuQGRtdS5lZHUuY24=