- 1College of Resources and Environmental Science, China Agricultural University, Beijing, China
- 2College of Natural Resources and Environment, Northwest A&F University, Xianyang, China
- 3Institute of Plant Protection, Jiangsu Academy of Agricultural Sciences (JAAS), Nanjing, China
Tomato wilt disease, caused by the Fusarium oxysporum is an ever-increasing threat for agricultural production, and unreasonable fertilization and pesticide abuse caused environmental challenge. Increasing evidence suggested that microbiomes or those associated with crops, played key roles on plant health. Plant disease dynamics were affected by multiple biotic and abiotic factors including phytopathogen population density, the genetic type of the pathogen and the host, in particular, the composition and assembly of the host-associated microbiome. However, it was unclear how pathogen invasion interaction and correlate with endophytic bacterial communities in natural field conditions. To study this, we sampled temporally the tomato plants that were exposed to F. oxysporum invasions over one crop season. High-throughput sequencing were performed to explore the correlation between agricultural practice, pathogen invasion, and endophytic microbiota communities. Results showed that pathogen invasion had clear effect on the endophytic and a strong link between increased pathogen densities and reduced abundance of Bacillus sp., which are crucial taxonomy for suppressiveness to F. oxysporum in vitro and in greenhouse condition. In summary, monitoring the dynamics of endophytic bacteria communities and densities of pathogen could thus open new avenue for more accurate disease diagnostics and high-efficiency screening antagonisms methods in the future, and our results will broaden the agricultural view of beneficial microbiota as biological control agents against plant pathogen.
Introduction
Tomato wilt disease, caused by the Fusarium oxysporum, poses a growing threat to agricultural production, and the unreasonable fertilization and pesticide abuse used to control this disease has caused an environmental challenge. With the demand for policy regarding the reduction of fertilizer and pesticides, there is an urgent need to reduce the use of environmentally unfriendly pesticides and agrochemicals (Bautista-Baños et al., 2013; Wei et al., 2019). Application of beneficial microbial inoculants can increase crop yields and resist the invasion of pathogens. In addition, increasing cases illustrate that soil microbiomes or those associated with plants play key roles in plant health (Shen, 1997; Curtis et al., 2004; Chen et al., 2018). Endophytic bacteria that can inhabit plant tissues without causing disease has been suggested as a viable alternative to reduce crop disease level (Mazurier et al., 2009). Increasing evidence suggests that taxonomic groups such as Bacteroidetes, Acidobacteria, Firmicutes, and Proteobacteria are involved in biocontrol of soil-borne diseases (Zhang et al., 2009; Mendes et al., 2011; Trivedi et al., 2012; Garciasalamanca et al., 2013; Donn et al., 2015). Various suppressive mechanisms were reported, mainly including the improvement of microbe-mediated pathogen suppression and inducing the plant immune practice (Hu et al., 2016). Specifically, antibiosis, competition, and elicitation of induced systemic resistance (ISR) contributes indirectly to disease suppression (Niu et al., 2017; Kwak et al., 2018). Especially, many antagonistic taxonomies including Bacillus spp., Streptomyces spp., Trichoderma spp., and Pseudomonads aeruginosa inhibited soil borne diseases by producing the antibiotics: 2,4-diacetylphloroglucinol, surfactin, fengycin, and antitoxin, etc. (Arrebola et al., 2010; Sandhya et al., 2010; Wang et al., 2019). Fan et al. (2017) found that inoculation of Bacillus subtilis 9407 can suppress the bacterial fruit blotch, for instance, surfactin antibiotic mediated the pathogen suppression.
With the development of next-generation sequencing technology, the composition and assembly of plant microbiome dynamics on plant health have been studied for several decades, which might be generally influenced by the genetic type of the host, and temporal and meteorological variation (Dodds and Rathjen, 2010). However, little is still known about how endophytic bacterial communities, pathogen density dynamics, and agricultural manipulation affect how plant disease takes place, that is, how these biotic and abiotic factors interact and correlate with each other in regulating plant disease dynamics in greenhouse conditions. Given the complex interaction between the endophytic bacterial, soil physical and chemical properties, and pathogen dynamics above mentioned, we set out to study the following two key hypotheses:
(i) To what extent do plant pathogen invasion and agricultural practice influence endophytic bacterial communities?
(ii) What are the underlying suppressive taxonomics in the endophytic bacterial communities?
Here, we used comprehensive methods, including greenhouse experiments, high throughput sequencing, and artificially created co-culturing of select (two or more) species assay to study the correlation between agricultural practice, pathogen invasion, and endophytic microbiota communities, and constructing suppressive endophytic bacterial communities. The endophytic bacterial communities under four different crop phases (seedling phase, flowering phase, fruiting phase, and harvesting phase) and different healthy conditions (initial health, final health, and diseased), agricultural practices (conventional practice, organic practice) were explored by using 16S amplicon sequencing in this study. Results showed that pathogen invasion had a clear effect on the endophytic bacterial communities, and Bacillus sp. were enriched in the healthy tomato samples at the seedling phase, which implied a preventive-protective effect of Bacillus. We also found an antagonism effect on the F. oxysporum both in the vitro and vivo conditions. Our results will help to broaden the agricultural applications of synthetic microbial communities as biological control agents against plant pathogens.
Materials and Methods
Experimental Field Site, Sampling Regime, and Soil Physicochemical Properties
The field experiment was located in Quzhou county, Hebei province (36°52′N, 115°01′E). The long-term trail treatment included organic, integrated, and conventional farming practices. Details on the agricultural management in each greenhouse were as described by Han et al. (2019), briefly, organic farming practices were characterized by the application of compost, as well as biocontrol for the plant disease, while conventional farming followed the local traditional farming style for greenhouse vegetable production and used chemical fertilizers. Both organic (ORG) and conventional (CON) greenhouse practices were selected with relatively high disease incidence of Tomato Fusarium oxysporum wilt (30% disease incidence for organic greenhouse during crop season, and 50% disease incidence for conventional greenhouse during crop season). Ten healthy tomato samples were randomly collected at the organic and conventional greenhouses at the seedling phase. Tomato showed symptoms of wilting and approximately 50% of plants showed clear symptoms of Fusarium wilt by the end of the crop season (from 10 weeks from the transplantation), thus, 10 healthy and 10 diseased plants were randomly collected from the organic and conventional greenhouse, and sampling finished after 12 weeks. Each sample was divided into two parts, aboveground (stem) and belowground (root), after the transplantation, thus 30 tomato plants (60 samples) were collected in total (Supplementary Figure 1). Physicochemical properties of soil such as the content total nitrogen, organic matter, ammonia, and pH were determined according to standard protocols (Wang et al., 2020), and soil physicochemical properties were ordinated by Excel (v.2019) and SPSS (v.19).
qPCR Quantification of Fusarium oxysporum Densities
Fusarium oxysporum densities were determined with qPCR by using primers targeting the foC gene (forward primer: 5′-AACMGGATTAGATACCCKG-3′ and reverse primer: 5′-GCACTGGCATATA-3′, KamaID, Sharma et al., 2004). The qPCR was carried out with Applied Biopractices 7500 Real-Time PCR Practice (Applied Biopractices, CA, United States) by using the SYBR Green I fluorescent dye detection in 20-μl volumes containing 10 μl of SYBR Premix Ex Taq (TaKaRa Biotech. Co., Japan), 2 μl of template, and 0.5 μl of both forward and reverse primers (10 mM each). The qPCR was performed by initially denaturing for 30 s at 95°C with subsequent cycling 40 times with a 5 s denaturizing step at 95°C. The protocol was followed by a 34 s elongation/extension step at 60°C and with a melt curve analysis for 15 s at 95°C followed by 1 min at 60°C and finally for 15 s at 95°C. Melting curves were obtained based on a standard protocol and used to identify the characteristic peak of PCR product (160 bp). Three independent technical replicates were used for each sample.
DNA Extraction and 16S rRNA Gene Amplicon High Throughput Sequencing
All samples’ surface sterilization for removing the epiphyte was performed as previously described (McPherson et al., 2018), until no bacterial growth was detected after inoculating the roots on R2A agar plate at 30°C for 7 days, as well as no amplification of 16S rRNA gene observed in the final wash water as template DNA.
Total microbial community DNA from samples was extracted using a Fast DNA spin Kit for soil (MP, Biomedicals, Santa Ana, Carlsbad, CA, United States). 16S rRNA gene fragments was amplified with the universal primers 799F (5′-GTGCCAGCMGC CGCGGTAA-3′) and 1193R (5′-CCCCGYCAATTCMTTTRA GT-3′) fused with a unique barcode (Liu et al., 2021). Gel-purified PCR products were mixed with equal molar following illumina sequencing using the platform of Hiseq2500.
Bioinformatic Analysis
The concentrations of the purified amplicon products were determined using QuantiFluor-ST (Promega, WI, United States) and sequenced on an Illumina MiSeq platform at Guangzhou Meige Biological Technology Co., Ltd.
All sequence reads were assigned to each sample based on designed primer and barcodes and the technical regions were trimmed for the following analyses. Sequence data were processed with the UPARSE pipeline (Edwards et al., 2015). High quality sequences (Chimeras were removed using UCHIME, Liu et al., 2021) were used for downstream analyses. Generation of the representative sequences and taxonomic OTU feature table obtained were then analyzed using Mothur (Schloss et al., 2009). Classification and identification of representative OTUs were processed with RDP naïve bayes classifier version 16 (Liu et al., 2019). Shannon and Ace indices were calculated in an algorithm similar to analysis to mitigate the biases caused by low quality sequences (Zhang et al., 2018; Ding et al., 2019; Wang et al., 2020). Community composition of endophytic bacterial visualized by PCoA analysis based on pairwise Bray-Curtis distance was calculated from the level of relative abundance of OTUs, and the effects of plant growth phase, treatment, and plant health condition on bacterial communities based on weighted_unifrac distance by PERMANOVA test, and all statistical analyses were performed with the R 3.1.21 software (R Core Team, 2013). Redundancy analysis (RDA) was used to analyze the correlation between the endophytic bacterial community, soil physicochemical properties, and environmental factors, and the correlation network was performed to compare the bacterial interactions at the OTU level in different treatments. Network analysis and visualization was performed based on Cytoscape software (version 3.4.0, Segata et al., 2011).
Linear discriminant analysis and a significance test were used to explore the most discriminating OTUs between health and diseased samples (Bastian et al., 2009). Three screening criteria were used:
(i) Linear discriminant analysis with a score of ≥4 (health condition relative to diseased condition).
(ii) Fold change ≥ 3.5 (health condition relative to diseased condition).
(iii) Significance test with P < 0.05.
The raw sequencing reads were deposited in the NCBI Sequence Read 186 Archive (SRA) under the accession number PRJNA764343 (SAMN 187 21500483).
Isolation, Screening of Endophytic Antagonists for Tomato Wilt Disease (Fusarium oxysporum)
To isolate the endospheric microbes, samples were washed thrice with sterilized PBS buffer (Na2HPO4 1.42 g/L; KH2PO4 0.24 g/L; NaCl 8 g/L; KCl 0.2 g/L; 0.01%, pH 7.4) shaken (170 rpm) for 2 h at 30°C. After centrifugation at 1,200 rpm at 4°C, all samples were washed until soil particles were completely removed. Then the washed roots and stems were cut up in pieces and a subsample of root tissue, representative of whole plant system, was collected and placed on the R2A medium. All bacteria were isolated by picking colonies, and each isolate was randomly selected by Sanger sequencing analysis, and sequence reads were aligned using BLASTn1, and the closest match was identified. Antagonism bacterial screening for F. oxysporum was based on co-culture in the plate as described (Liu et al., 2011).
Culture-Dependent Assessment of Antimicrobial Activity of Bacillus and Pseudomonas Bacteria Against Fusarium oxysporum Under Greenhouse Condition
Five antagonists (Bacilllus megaterium, Bacillus subtilis, Bacillus amyloliquefaciens, Pseudomonas aeruginosa and Pseudomonas putida, Supplementary Table 1) were selected from healthy tomato samples in this study. Co-cultures were performed to demonstrate antagonistic effects of each of the five selected isolates against F. oxysporum according to Paidhungat et al. (2000). When tomato seedlings were 20 days old, ten milliliters of the suspension antagonists (approximately 108 spores/mL) were inoculated, then challenged with F. oxysporum spores (approximately 107 spores/mL) and the disease incidence was evaluated 7 days after inoculation according to the Li et al. (2019). Three pots were taken and all six seedlings in each pot were used as a replicate for each treatment.
Results
Changes in the Soil Physiochemical Properties During the Field Experiment
To explore the variation of soil physiochemical properties in the greenhouse, four physicochemical properties were measured during the seedling phase and fruiting phase. In addition, soil physiochemical properties were different in two soil types. The results showed that concentration of total nitrogen and organic content of the organic greenhouse were significantly higher than those in the conventional greenhouse (p < 0.05, ANOVA, Tukey HSD; Figures 1A–D).
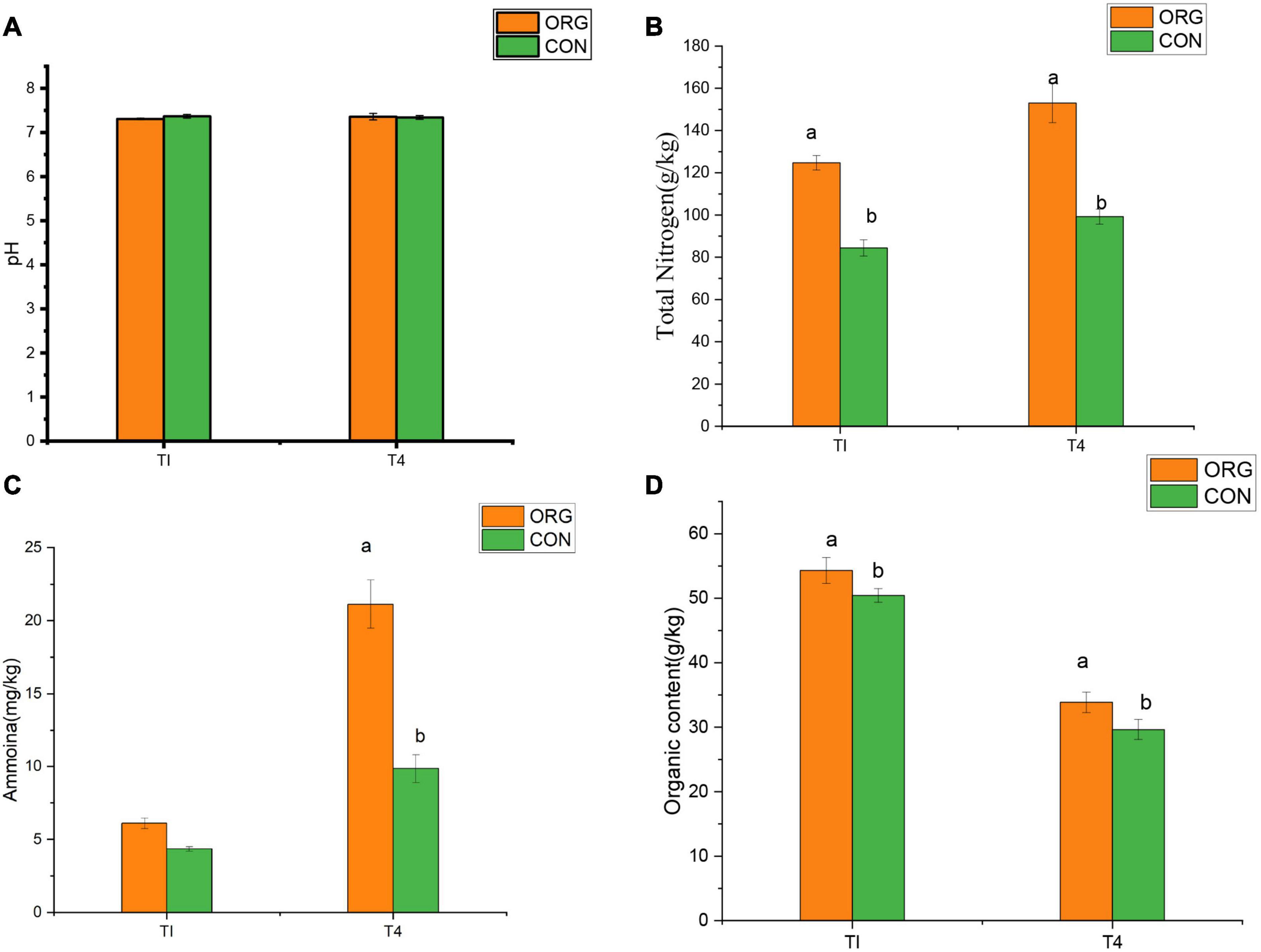
Figure 1. Discrimination of physiochemical soil properties during the field experiment. (A–D) Differences in major physiochemical soil properties between seedling phase and fruiting phase (averaged over time). (A) pH, (B) total nitrogen, (C) ammonia content, and (D) organic content. Different letters denote dramatically significant (p < 0.01). TI, seedling phase; T4, fruiting phase.
Disease Development and Fusarium oxysporum Density Dynamics During the Field Experiment
Pathogens could be detected in all tomato rhizosphere soil samples since the beginning of the sampling and pathogen abundance varied from 101.45 to 109.23 copy of foC gene per gram of soil. As tomato Fusarium wilt disease showed visible signs at fruiting and harvesting phase, thus disease incidence was observed in June, July, and August. Results showed that disease incidence in the organic greenhouse was about 31.2%, while that of the conventional greenhouse was about 49.8% (Figure 2A), and disease incidence was less severe in the organic practice greenhouse compared to that in the conventional practice greenhouse (p < 0.05, ANOVA, Tukey HSD; Figure 2). Intriguingly, a relatively high density of pathogens was observed in either the organic or conventional diseased tomato rhizosphere root soil, specifically, the density of F. oxysporum (foC gene copy number/g rhizosphere soil) reached about 108 copy number/g in the diseased tomato rhizosphere root soil, while 102 copy number/g rhizosphere root soil in the healthy tomato samples (Table 1).
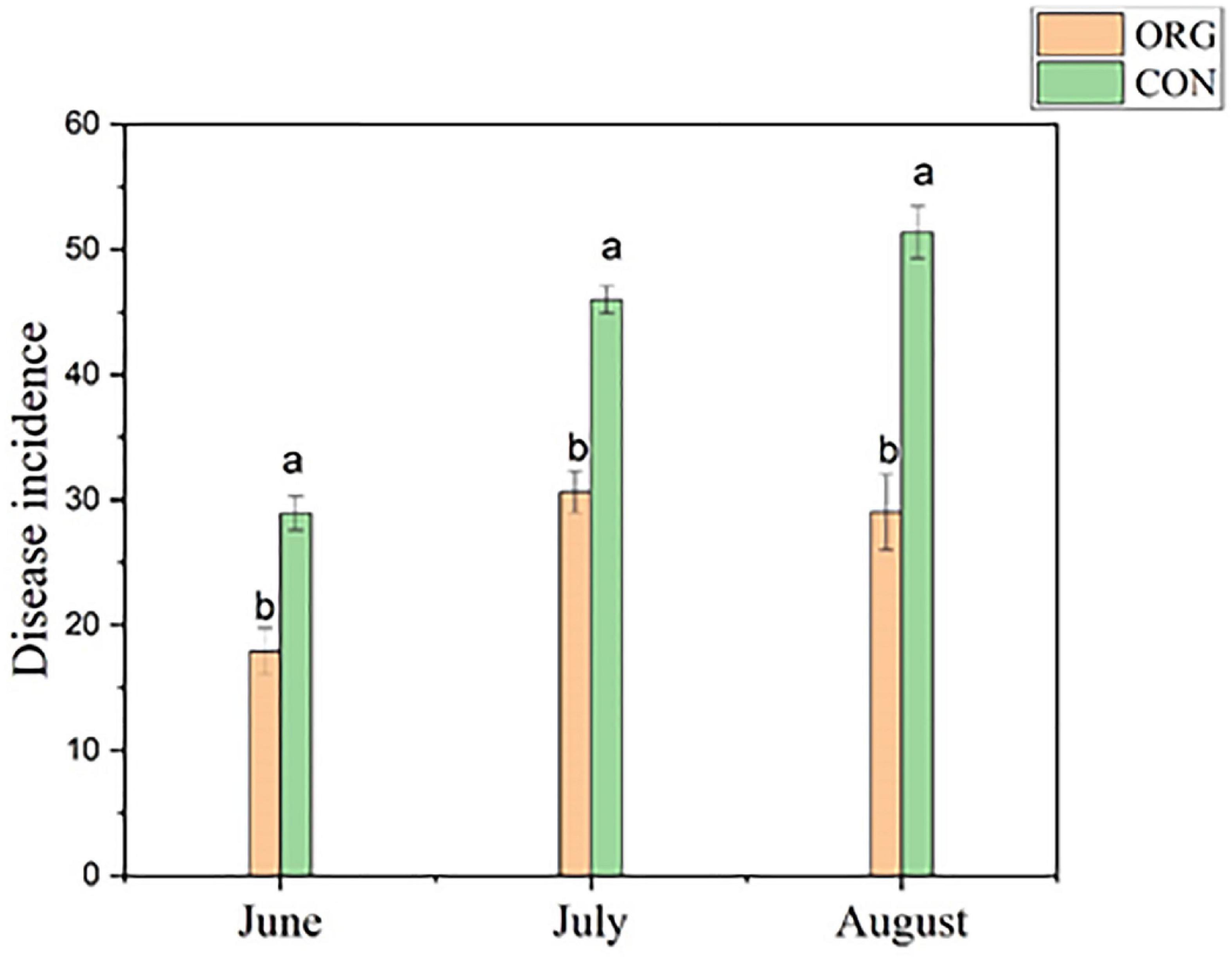
Figure 2. Tomato Fusarium wilt disease incidence from June to August in 2019 during the field experiment (N = 15).
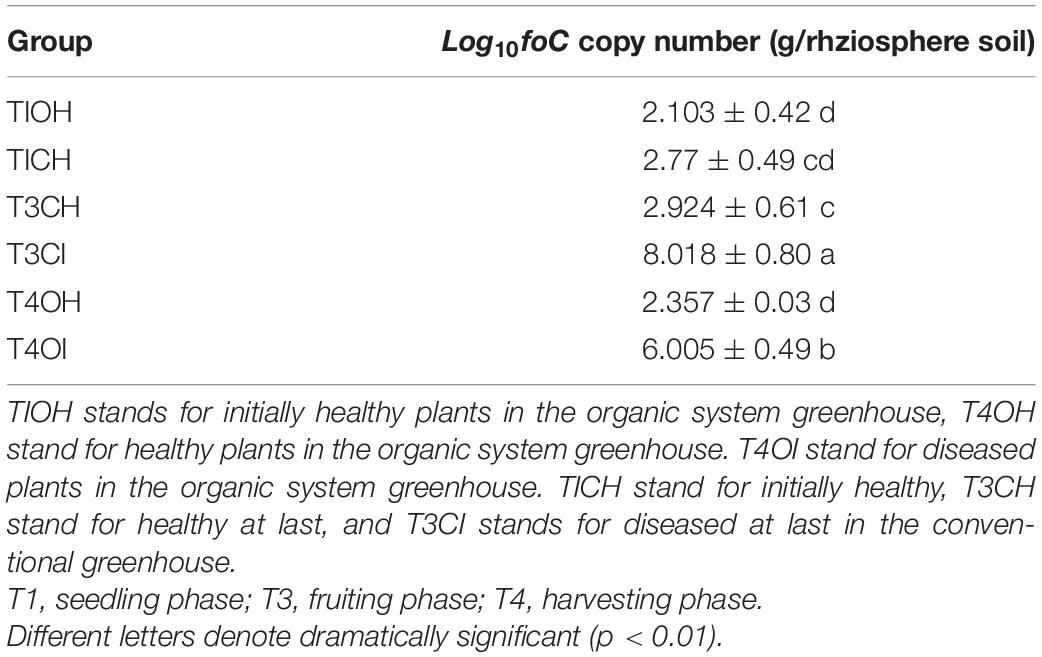
Table 1. Tomato rhizosphere Fusarium oxysporum pathogen density dynamics from seedling phase (March) to harvesting phase (August) in 2019 during the field experiment (N = 10).
Healthy Tomato Endosphere Microbiota Have a Distinct Structure
The density dynamics of the most abundant endophytic bacterial phyla differed significantly between the healthy and diseased samples, and results showed that the majority of phyla belonged to Proteobacteria accounting for 41.58%, followed by Firmicutes (26.15%), while Bacteroidetes and Actinobacteria accounted for 20.2 and 11.82%, respectively, in the initial healthy tomato samples, and the most striking results showed that Firmicutes decreased intensely to 3.55% at diseased samples under the organic practice greenhouse. The similar results were also observed at the conventional manipulation greenhouse. Proteobacteria (42.20%) and Firmicutes (29.15%) are the main phyla in the initial healthy tomato samples, and Firmicutes decreased dramatically to 6.43% in the diseased samples in the conventional practice greenhouse (Supplementary Figures 2, 3).
Taxonomic dynamics of the most abundant endophytic bacterial genera differed significantly between the healthy and diseased samples. In general, the relative abundance of the Bacillus, Cronbacter, and Weeksellaceae clearly decreased in the diseased compared to healthy plant samples, while Sphingobacterium, Flavobacterium, Burkholderiaceae, and Xanthobacteraceae increased in the diseased plant samples in the organic greenhouse. Similar trends occurred in the conventional greenhouse, crucially, Bacillus sp. were observed in the relative high abundance of bacterial genus at healthy samples in the seedling phase, and the density of which decreased in the diseased tomato samples (Figures 3A,B). Interestingly, low abundance of Bacillus was observed in the healthy samples at the late crop season (Figure 3C). Diversity decreased in diseased samples compared with healthy samples regardless of organic or conventional greenhouse (p < 0.05, ANOVA, Tukey HSD), Specifically, the increase in pathogen densities was associated with reduced endophytic bacterial diversity both in the organic and conventional greenhouse (Shannon index, Figures 4C,D).
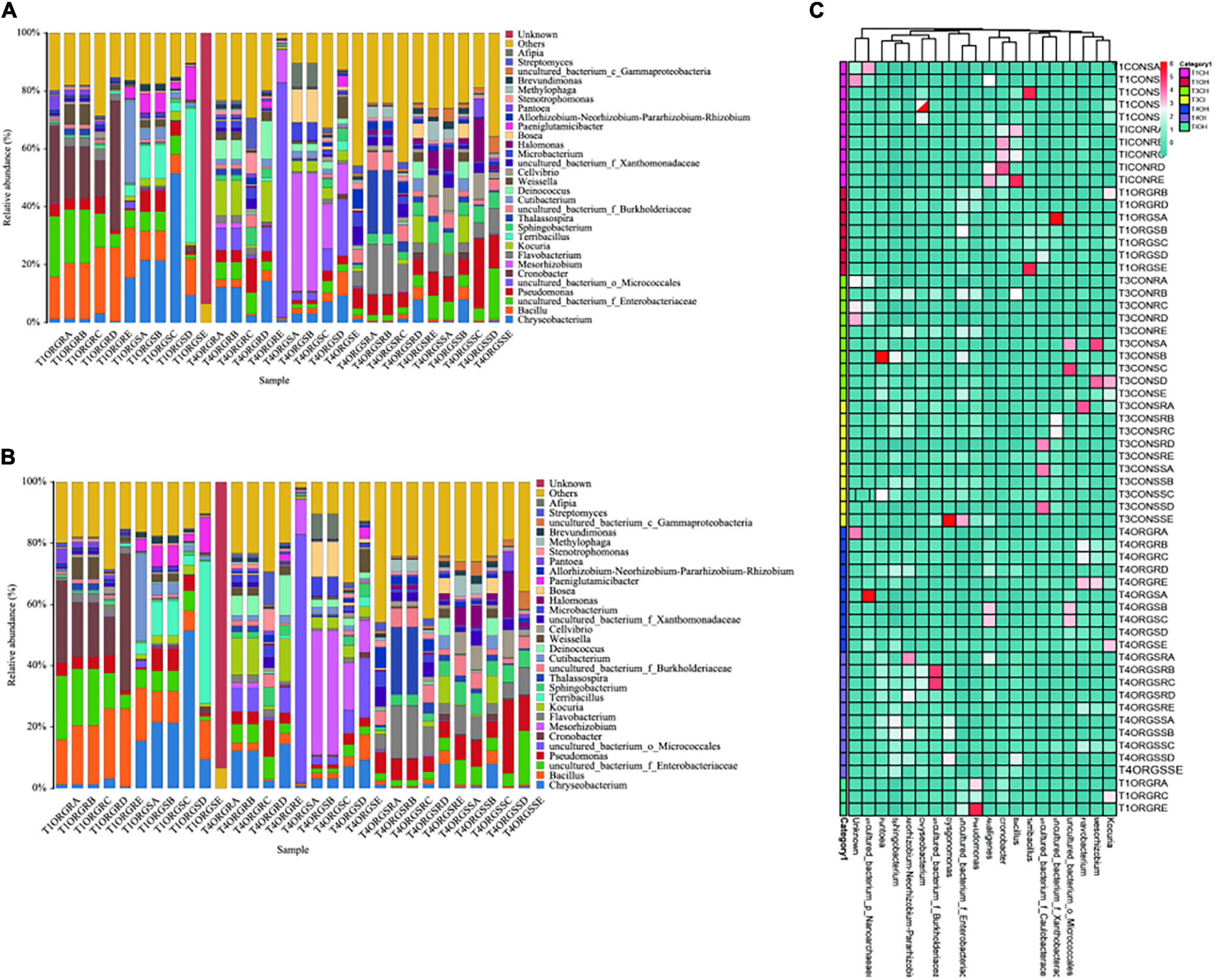
Figure 3. Changes in the endophytic microbiome composition and diversity during the field experiment. (A) The relative bacterial density dynamics in initial, healthy, and diseased tomato endophytic samples in the organic system greenhouse. (B) The relative bacterial density dynamics in initial, healthy, and diseased tomato endophytic samples in the conventional system greenhouse. (C) Heatmap analysis of endophytic microbiome composition during the field experiment. T1OH stands for initially healthy, T4OH stands for healthy at last, T4OI stands for diseased at last. TICH stands for initially healthy, T3CH stands for healthy at last, T3CI stands for diseased at last in the conventional greenhouse, TIOH stands for initially healthy plants in the organic system greenhouse, T4OH stands for healthy plants in the organic system greenhouse, and T4OI stands for diseased plants in the organic system greenhouse.
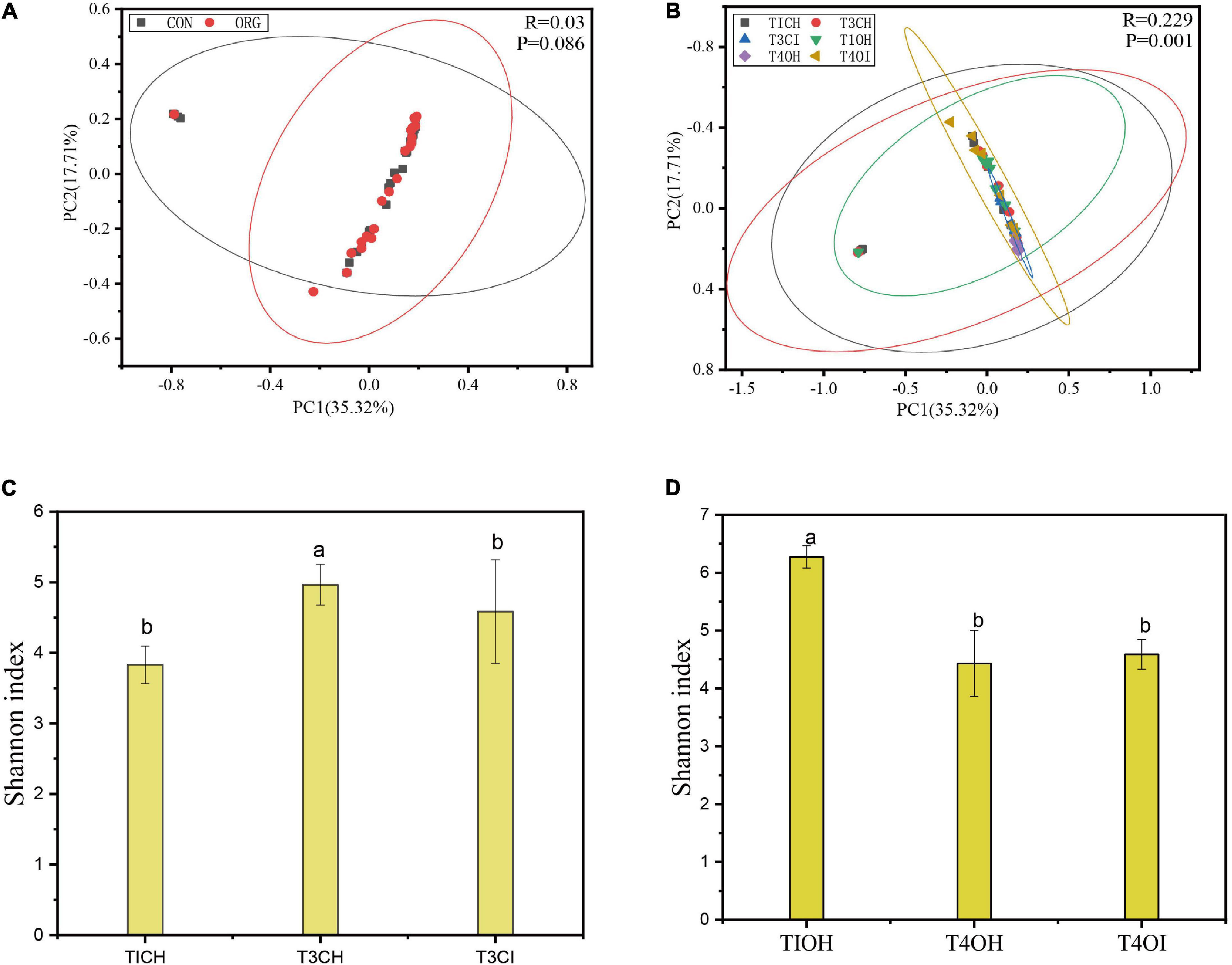
Figure 4. (A) PCoA of bacterial microbiota using Bray–Curtis distance for organic and conventional agricultural practice. ORG stands for organic greenhouse. CON stands for conventional greenhouse. (B) PCoA of bacterial microbiota using Bray–Curtis distance for healthy and diseased plants. (C) OTU bacterial Shannon diversity index for different treatments at organic greenhouse. (D) OTU bacterial Shannon diversity index for different treatments at conventional greenhouse. TIOH stands for initially healthy plants in the organic system greenhouse, T4OH stands for healthy plants in the organic system greenhouse. T4OI stands for diseased plants in the organic system greenhouse. TICH stands for initially healthy, T3CH stands for healthy at last, and T3CI stands for diseased at last in the conventional greenhouse. T1, seedling phase; T3, fruiting phase; T4, harvesting phase. Different letters denote dramatically significant (p < 0.01).
Pathogen Invasion Disrupts the Endophytic Bacterial Composition
PCoA analysis showed that pathogen invasion had strong effects on endophytic bacteria composition, healthy and diseased samples clustered with two axes regardless of organic or conventional agricultural practice (R2 = 0.229, P = 0.001, PERMANOVA analysis; Figures 4A,B). To investigate the diversity of changes occurring in microbial community structures at the phylum level, redundancy analysis (RDA) was conducted. Results showed that all of the edaphic variables explained up to 56.28% of the variance, with the first axis explaining 36.73% of variation and the second axis explaining another 19.55% (Figure 5A). RDA plots showed that pathogens might be key physicochemical factors in assembling the microbial community structure of the tomato endophytic microbiome, and Firmicutes showed positive reaction to pathogen invasion. In addition, the correlation heatmap showed that the content of total nitrogen and organic had a positive influence on Proteabacteria and Gemmatimonadetes (Figure 5B).
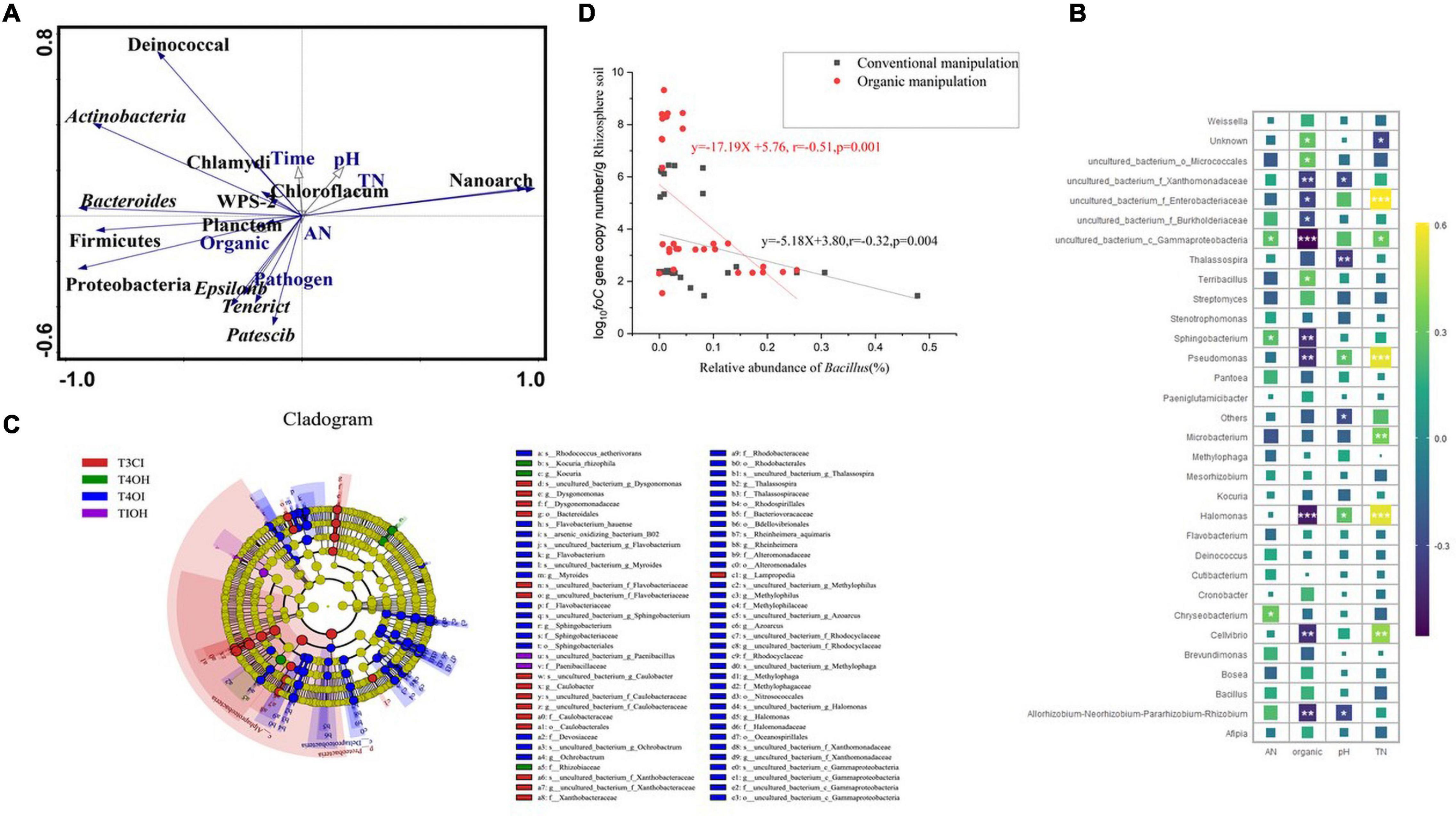
Figure 5. (A) The relationship among the bacterial community, environmental parameters. Redundancy analyses (RDA) of the correlation between the bacterial community (Phylum level) and soil physicochemical properties. Gray lines represent significant factors and Blue lines represent key Phyla. (B) Correlation (Pearson’s coefficient) heat map between key rhizosphere soil bacterial community (genus level) and soil physicochemical properties of rhizosphere soil. *Denotes significance (P < 0.05, FDR adjust), and ** denotes significance (P < 0.01, FDR adjust). (C) Lefse analysis discrepancy in Endophytic bacterial taxonomy of Tomato between Healthy and Diseased Plants in the organic system greenhouse (LDA > 3.5, FD > 2, P < 0.05). (D) Correlation between log10foCgene copy number/g rhizosphere soil and relative abundance of Bacillus sp. in the organic greenhouse and conventional greenhouse.
Microbial Biomarkers in Different Treatments and Healthy Conditions
Lefse analysis discrepancy based on datasets of healthy and diseased plants revealed that Bacillus sp. were enriched in the healthy tomato samples during the seedling phase (LDA > 3.5, FD > 2, p < 0.05), while Sphingobacterium, Flavobacterium, Burkholderiaceae, and Xanthobacteraceae were enriched in the diseased tomato samples. Similar results occurred in the conventional greenhouse, and Lefse analysis also showed that discrepancy decreased with plant growth between each treatment (Figure 5C). Negative correlation between pathogen density dynamics and relative abundance of Bacillus sp. were observed both in the organic and conventional greenhouse based on linear regression (Figure 5D).
Seedling Phase Healthy Tomato Steered a Highly Connected Association Network
Microbial networks were generated to depict the co-occurrence patterns of the microbial community in different treatments and plant healthy statuses. The results showed that pathogen invasion disrupted significantly simplified microbial associations as compared with the healthy tomato which had a complexity of microbial associations (Figure 6). Specifically, the average degrees in networks markedly reduced from 39.272 in the seeding phase organic endophyte group (T1OH) to 4.186 and 2.664 in the fruiting phase healthy organic endophyte group (T4OH) and diseased organic endophyte group (T4OI) respectively. Similarly, the average degrees in networks markedly reduced from 7.708 in the seeding phase organic endophyte group (T1CH) to 7.269 and 2.93 in fruiting phase healthy organic endophyte group (T3CH) and diseased organic endophyte group (T3CI) respectively (Table 2). In comparison to the diseased and healthy plants, we further found that the dominant keystone taxa in the diseased and healthy treatments were mainly represented by potential plant beneficial or antagonistic bacterial taxa like Actinobacteria, Proteobacteria, and Firmicutes (Table 2).
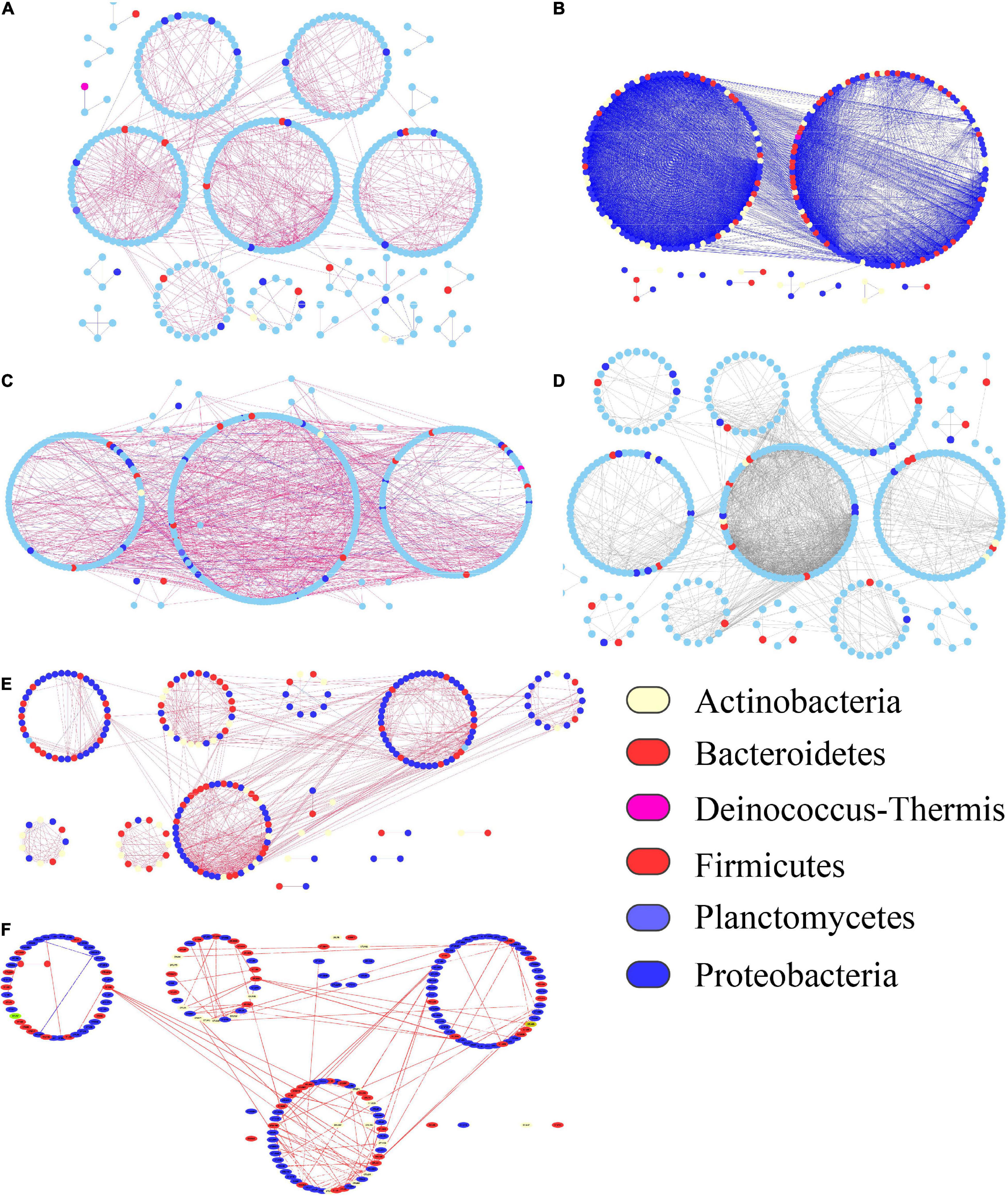
Figure 6. Visualized networks of microbial co-occurrence patterns. (A) T1OH, (B) T4OH, (C) T4OI, (D) TICH, (E) T3CH, and (F) T3CI. TIOH stands for initially healthy plants in the organic system greenhouse, T4OH stands for healthy plants in the organic system greenhouse, T4OI stands for diseased plants in the organic system greenhouse, TICH stands for initially healthy, T3CH stands for healthy at last, and T3CI stands for diseased at last in the conventional greenhouse. T1, seedling phase; T3, fruiting phase; T4, harvesting phase.
Bacillus sp. Exhibited High Biocontrol Efficacy on Fusarium oxysporum
Among the antagonists, 14 Bacillus strains, 11 Pseudomonas including Bacillus Megaterium, Bacillus subtilis, Bacillus amyloliquefaciens Pseudomonas aeruginosa, and Pseudomonas putida belonging to species (Supplementary Table 1) that are usually reported as biocontrol agents in the specialized literature were observed to have a direct inhibition effect on F. oxysporum. Specifically, the inhibition zone of Bacillus subtilis 6 reached 2.0 ± 0.26 cm (p < 0.05, Figure 7A). Bacillus Megaterium 5, Bacillus subtilis 6, Bacillus amyloliquefaciens 24, Pseudomonas aeruginosa 142, and Pseudomonas putida 271 were selected to evaluate their antagonistic capacity against pathogens in the greenhouse condition for their desirable inhibition effect on F. oxysporum in vitro, and results of the greenhouse bioassay showed that biocontrol effect reached 92.8% (P < 0.05, Figure 7B and Supplementary Figure 4).
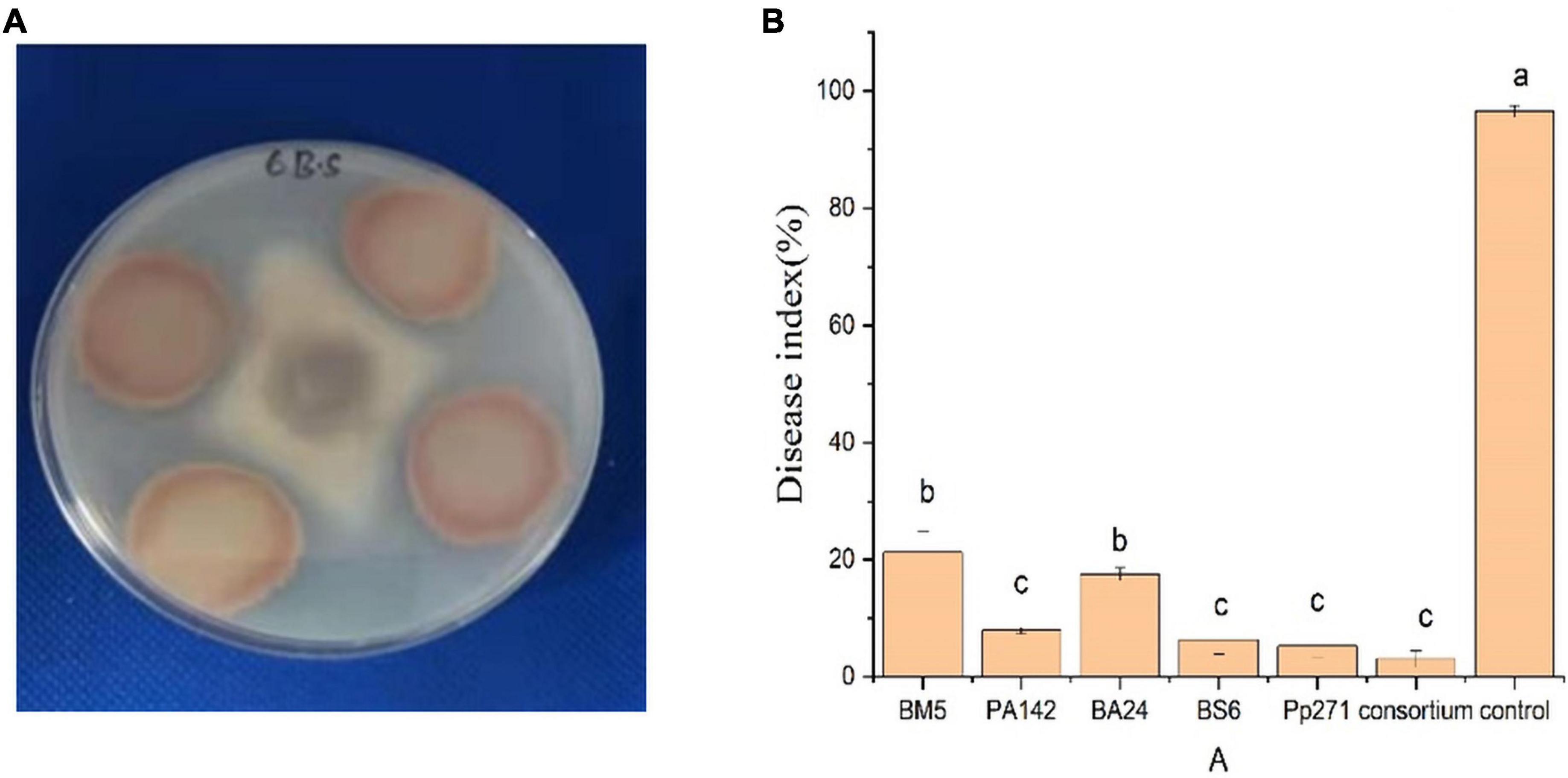
Figure 7. (A) Antagonism effect of endophytic bacterial in vitro. Strain inoculated on PDA plates, incubated at 30°C for 2 days and then sprayed with the isolated bacteria. (B) Bioassay against F. oxysporum in the greenhouse condition, n = 6, significant difference is denoted by different letters (P < 0.05).
Discussion
Here we explored how agricultural practice (organic and conventional agricultural manipulation system), crop season (plant development time), and endophytic bacterial community composition interact with the invasion of F. oxysporum in tomato under field conditions. Host-associated microbiome communities have repeatedly been observed, especially between healthy and diseased plant individuals of which discriminative taxonomies are hotspots in the related research (Adesina et al., 2007; van der Heijden and Schlaeppi, 2015; Perez-Jaramillo et al., 2019).
Wei et al. (2018) found no difference in the rhizosphere soil physicochemical properties between healthy and diseased plants, suggesting that abiotic factor may not drive the outbreak of disease. The soil’s physiochemical properties showed clear discrepancy patterns with the agricultural practice in this study, however, only bulk soil physical properties instead of rhizosphere soil of each sampled tomato were determined in this study, and the correlation between rhizosphere soil properties and each sample’s F. oxysporum dynamics still needs to be explored.
Clearly, decline was found in the relative abundances of Firmcutes, Bacterioidetes, and Actinomycetes (Supplementary Figures 1, 2), which is in line with other similar results, that is, Firmcutes decline leads to disruption of host-associated microbiome exacerbated F. oxysporum invasion (Walters et al., 2018). The final health status of tomato is significantly related to its initial (seedling phase) endophytic bacterial community composition based on Lefse analysis. Temporal pathogen dynamics and endophyte associated with healthy and diseased plants were analyzed with linear regression, and a negative correlation was observed between pathogen density and abundance of Bacillus sp., demonstrating that relative abundance of Bacillus, especially in the initial growing phase, makes a contribution to the suppression of pathogens (Figure 5B). Among the antagonistic bacteria isolated at the seedling phase in this study, the abundance of Bacillus was enriched, reaching 71%, while reaching 29% in the late crop season (Fruiting phase) (Supplementary Figure 5). We conclude that the host endophyte Bacillus can provide protection from plant disease in the early crop phase.
Our results support the hypothesis that agricultural practice and pathogen invasion had a clear effect on the endophytic bacteria communities in line with other research (Trivedi et al., 2012). Complexity of microbial associations, and the resulting higher percentage of co-occurrence in the networks during pathogen invasion, indicates lower potential competition for resources and predators and an increased cooperation within the network (Figure 6).
Similar results showed that development of dysbiosis, a microbial imbalance associated with the increased density of bacterial pathogen, could thus also be important in explaining the bacterial wilt disease dynamics (Trivedi et al., 2017; Shi et al., 2020). Related research showed that dysbiosis of the phyllosphere microbiota was recently reported to cause disease on Arabidopsis leaves, and reduction of Firmicutes caused by an increase of the Proteobacteria population was the main driver of this dysbiosis (Chen et al., 2020). Dysbiosis of microbiota communities can also trigger loss predicted community functioning module based on metagenomics analysis (Wei et al., 2018). Our results also demonstrated that Bacillus sp. and pseudomonas sp. have an antagonist effect on the F. oxysporum both in the vivo and vitro conditions (Figure 7), which is in line with previous study which found that Firmicutes taxa, Bacillus, Flavobacterium, and Streptomyces establish disease suppression by a single strain or SynCom method via antagonistic effect (Kim et al., 2016; Xu et al., 2018; Wang et al., 2020). However, mechanisms of Bacillus sp. and Pseudomonas sp. in the suppression of F. oxysporum still need to be explored.
This study was based on a long-term greenhouse experiment in which several agricultural practices, including crop rotation, tillage, and irrigation, were the same for all three farming systems since 2002. On the basis of our results, we recommend that the current method to control plant diseases in agricultural systems be reconsidered. First, by modifying the composition of the microbial as a whole, instead of focusing on controlling pathogens directly, thus a better solution to control the outbreak of plant diseases can be achieved.
Conclusion
Pathogen invasion can drive community-wide dynamics in tomato endophytic bacterial composition. Bacillus sp. which is enriched in the seedling phase may play key role for the suppression of F. oxysporum based on Lefse analysis. Healthy tomato steered a higher connected association network than that in diseased tomato regardless of organic or conventional agricultural treatment. Bacillus and Pseudomonas are the dominant taxa in the antagonists of F. oxysporum in both of the field conditions.
Data Availability Statement
The datasets presented in this study can be found in online repositories. The names of the repository/repositories and accession number(s) can be found below: NCBI (accession: PRJNA764343).
Author Contributions
ZyZ and JL: methodology, validation, formal analysis, writing–original draft, and writing–review and editing. ZqZ, YL, and YW: revising and editing. All authors contributed to the article and approved the submitted version.
Funding
This work was supported by the National Natural Science Foundation of China (Grant Nos: 31570441 and 31400095).
Conflict of Interest
The authors declare that the research was conducted in the absence of any commercial or financial relationships that could be construed as a potential conflict of interest.
Publisher’s Note
All claims expressed in this article are solely those of the authors and do not necessarily represent those of their affiliated organizations, or those of the publisher, the editors and the reviewers. Any product that may be evaluated in this article, or claim that may be made by its manufacturer, is not guaranteed or endorsed by the publisher.
Supplementary Material
The Supplementary Material for this article can be found online at: https://www.frontiersin.org/articles/10.3389/fmicb.2021.731764/full#supplementary-material
Supplementary Figure 1 | Schematic figure of the field sampling system and the experimental design. Treatments have organic and conventional two manipulation greenhouses. Initial healthy samples were collected when the experiment was conducted (4 weeks), and diseased samples were collected when the experiment was conducted (10 and 12 weeks after planting).
Supplementary Figure 2 | Changes in the endophytic microbiome composition at the phylum level during the field experiment. The relative bacterial density dynamics in initial, healthy, and diseased tomato endophytic samples in the organic system greenhouse.
Supplementary Figure 3 | Changes in the endophytic microbiome composition at the phylum level during the field experiment. The relative bacterial density dynamics in initial, healthy, and diseased tomato endophytic samples in the conventional system greenhouse.
Supplementary Figure 4 | Evaluation of disease suppression effect by greenhouse bioassay (N = 18).
Supplementary Figure 5 | Percentage of different antagonistic bacteria in the Seedling and Fruiting phases.
Footnotes
References
Adesina, M. F., Lembke, A., Costa, R., Speksnijder, A., and Smalla, K. (2007). Screening of bacterial isolates from various European soils for in vitro antagonistic activity towards Rhizoctonia solani and Fusarium oxysporum: site-dependent composition and diversity revealed. Soil Biol. Biochem. 39, 2818–2828. doi: 10.1016/j.soilbio.2007.06.004
Arrebola, E., Jacobs, R., and Korsten, L. (2010). Iturin A is the principal inhibitor in the biocontrol activity of Bacillus amyloliquefaciens PPCB004 against postharvest fungal pathogens. J. Appl. Microbiol. 108, 386–395. doi: 10.1111/j.1365-2672.2009.04438.x
Bastian, M., Heymann, S., and Jacomy, M. (2009). “Gephi: an open source software for exploring and manipulating networks,” in Proceedings of the International Conference on Weblogs and Social Media, eds E. Adar, M. Hurst, T. Finin, N. Glance, N. Nicolov, and B. Tseng (Menlo Park, CA: The AAAI Press).
Bautista-Baños, S., Sivakumar, D., Bello-P’erez, A., and Villanueva-Arce, R. (2013). A review of the management alternatives for controlling fungi on papaya fruit during the postharvest supply chain. Crop Protect. 49, 8–20. doi: 10.1016/j.cropro.2013.02.011
Chen, T., Nomura, K., Wang, X., Sohrabi, R., and He, S. Y. (2020). A plant genetic network for preventing dysbiosis in the phyllosphere. Nature 580, 653–657. doi: 10.1038/s41586-020-2185-0
Chen, Y., Wang, J., Yang, N., Wen, Z., Sun, X., Chai, Y., et al. (2018). Wheat microbiome bacteria can reduce virulence of a plant pathogenic fungus by altering histone acetylation. Nat. Commun. 9:3429.
Curtis, H., Noll, U., Stormann, J., and Slusarenko, A. J. (2004). Broad-spectrum activity of the volatile phytoanticipin allicin in extracts of garlic (Allium sativum L.) against plant pathogenic bacteria, fungi and Oomycetes. Physiol. Mol. Plant Pathol. 65, 79–89. doi: 10.1016/j.pmpp.2004.11.006
Ding, G. C., Bai, M., Han, H., Li, H., Ding, X., Yang, H., et al. (2019). Microbial taxonomic, nitrogen cycling and phosphorus recycling community composition during longterm organic greenhouse farming. FEMS Microbiol. Ecol. 95:fiz042.
Dodds, P. N., and Rathjen, J. P. (2010). Plant immunity: towards an integrated view of plant–pathogen interactions. Nat. Rev. Genet. 11, 539–548. doi: 10.1038/nrg2812
Donn, S., Kirkegaard, J. A., Perera, G., Richardson, A. E., and Watt, M. (2015). Evolution of bacterial communities in the wheat crop rhizosphere. Environ. Microbiol. 17, 610–621. doi: 10.1111/1462-2920.12452
Edwards, J., Johnson, C., Santos-Medellin, C., Lurie, E., Podishetty, N. K., and Bhatnagar, S. (2015). Structure, variation, and assembly of the root-associated microbiomes of rice. Proc. Natl. Acad. Sci. U.S.A. 112, 911–920. doi: 10.1073/pnas.1414592112
Fan, H., Zhang, Z., Li, Y., Zhang, X., Duan, Y., and Wang, Q. (2017). Biocontrol of bacterial fruit blotch by bacillus subtilis 9407 via surfactin-mediated antibacterial activity and colonization. Front. Microbiol. 8:1973. doi: 10.3389/fmicb.2017.0197
Garciasalamanca, A., Molinahenares, M. A., Van Dillewijn, P., Solano, J., Pizarrotobias, P., and Roca, A. (2013). Bacterial diversity in the rhizosphere of maize and the surrounding car bonate-rich bulk soil. Microb. Biotechnol. 6, 36–44. doi: 10.1111/j.1751-7915.2012.00358.x
Han, H., Ding, G. C., Li, X. X., Hu, K., Yang, H., Xu, T., et al. (2019). Erratum to “Organic vegetable cultivation reduces N leaching while increasing the relative soil N budget” Agric. Water Manag. 213 (2019) 803–808. Agric. Water Manag. 223:105607. doi: 10.1016/j.agwat.2019.04.024
Hu, J., Wei, Z., Friman, V. P., Gu, S. H., Wang, X. F., and Eisenhauer, N. (2016). Probiotic diversity enhances rhizosphere microbiome function and plant disease suppression. MBio 7:e01790-16.
Kim, B., Song, G. C., and Ryu, C.-M. (2016). Root exudation by aphid leaf infestation recruits root-associated Paenibacillus spp. to lead plant insect susceptibility. J. Microbiol. Biotechnol. 26, 549–557. doi: 10.4014/jmb.1511.11058
Kwak, M.-J., Kong, H. G., Choi, K., Kwon, S.-K., Song, J. Y., Lee, J., et al. (2018). Rhizosphere microbiome structure alters to enable wilt resistance in tomato. Nat. Biotechnol. 36, 1100–1109. doi: 10.1038/nbt.4232
Li, H., Cai, X., and Gong, J. (2019). Long-term organic farming manipulated rhizospheric microbiome and Bacillus antagonism against pepper blight (Phytophthora capsici). Front. Microbiol. 10:342. doi: 10.3389/fmicb.2019.00342
Liu, Y., Chen, Z., Liu, Y., Wang, X., Luo, C., Nie, Y., et al. (2011). Enhancing bio efficacy of bacillus subtilis with sodium bicarbonate for the control of ring rot in pear during storage. Biol. Control 57, 110–117. doi: 10.1016/j.biocontrol.2011.01.008
Liu, Y. X., Qin, Y., and Bai, Y. (2019). Reductionist synthetic community approaches in root microbiome research. Curr. Opin. Microbiol. 49:97. doi: 10.1016/j.mib.2019.10.010
Liu, Y. X., Qin, Y., Chen, T., Lu, M., Qian, X., Guo, X., et al. (2021). A practical guide to amplicon and metagenomic analysis of microbiome data. Protein Cell 12:315. doi: 10.1007/s13238-020-00724-8
Mazurier, S., Corberand, T., Lemanceau, P., and Raaijmakers, J. M. (2009). Phenazine antibiotics produced by fluorescent pseudomonads contribute to natural soil suppressiveness to Fusarium wilt. ISME J. 3, 977–991. doi: 10.1038/ismej.2009.33
McPherson, M. R., Wang, P., Marsh, E. L., Mitchell, R. B., and Schachtman, D. P. (2018). Isolation and analysis of microbial communities in soil, rhizosphere, and roots in perennial grass experiments. J. Vis. Exp. 24:57932. doi: 10.3791/57932
Mendes, R., Kruijt, M., De Bruijn, I., Dekkers, E., van derVoort, M., and Schneider, J. H. (2011). Deciphering the rhizosphere microbiome for disease-suppressive bacteria. Science 332, 1097–1100. doi: 10.1126/science.1203980
Niu, B., Paulson, J. N., Zheng, X., and Kolter, R. (2017). Simplified and representative bacterial community of maize roots. Proc. Natl. Acad. Sci. U.S.A. 114, E2450–E2459.
Paidhungat, M., Setlow, B., Driks, A., and Setlow, P. (2000). Characterization of spores of Bacillus subtilis which lack dipicolinic acid. J. Bacteriol. 182, 5505–5512. doi: 10.1128/JB.182.19.5505-5512.2000
Perez-Jaramillo, J. E., de Hollander, M., Ramirez, C. A., Mendes, R., Raaijmakers, J. M., and Carrion, V. J. (2019). Deciphering rhizosphere microbiome assembly of wild and modern common bean (Phaseolus vulgaris) in native and agricultural soils from Colombia. Microbiome 7:114.
R Core Team (2013). R: A Language and Environment for Statistical Computing. Vienna: R Foundation for Statistical Computing.
Sandhya, V., Ali, S. Z., Grover, M., Reddy, G., and Venkateswarlu, B. (2010). Effect of plant growth promoting Pseudomonas spp. on compatible solutes, antioxidant status and plant growth of maize under drought stress. Plant Growth Regul. 62, 21–30. doi: 10.1007/s10725-010-9479-4
Schloss, P. D., Westcott, S. L., Ryabin, T., Hall, J. R., Hartmann, M., Hollister, E. B., et al. (2009). Introducing mothur: open-source, platform634 independent, community-supported software for describing and comparing microbial communities. Appl. Environ. Microbiol. 75, 7537–7541. doi: 10.1128/AEM.01541-09
Segata, N., Izard, J., Waldron, L., Gevers, D., Miropolsky, L., Garrett, W. S., et al. (2011). Metagenomic biomarker discovery and explanation. Genome Biol. 12:R60.
Sharma, K. D., Winter, P., and Kahl, G. (2004). Molecular mapping of Fusarium oxysporum f. sp. ciceris race 3 resistance gene in chickpea. Theor. Appl. Genet. 108, 1243–1248. doi: 10.1007/s00122-003-1561-0
Shen, D. (1997). Microbial diversity and application of microbial products for agricultural purposes in China. Agric. Ecosyst. Environ. 62, 237–245. doi: 10.1016/s0167-8809(96)01132-2
Shi, X., Wang, S., and Duan, X. (2020). Biocontrol strategies for the management of Colletotrichum species in postharvest fruits. Crop Protect. 141:105454. doi: 10.1016/j.cropro.2020.105454
Trivedi, P., Delgado-Baquerizo, M., Trivedi, C., Hamonts, K., Anderson, I. C., and Singh, B. K. (2017). Keystone microbial taxa regulate the invasion of a fungal pathogen in agro-ecosystems. Soil Biol. Biochem. 111, 10–14. doi: 10.1016/j.soilbio.2017.03.013
Trivedi, P., He, Z., Van Nostrand, J. D., Albrigo, G., Zhou, J., and Wang, N. (2012). Huanglongbing alters the structure and functional diversity of microbial communities associated with citrus rhizosphere. ISME J. 6, 363–383. doi: 10.1038/ismej.2011.100
van der Heijden, M. G., and Schlaeppi, K. (2015). Root surface as a frontier for plant microbiome research. Proc. Natl. Acad. Sci. U.S.A. 112, 2299–2300. doi: 10.1073/pnas.1500709112
Walters, W. A., Jin, Z., Youngblut, N., Wallace, J. G., Sutter, J., and Zhang, W. (2018). Large-scale replicated field study of maize rhizosphere identifies heritable microbes. Proc. Natl. Acad. Sci. U.S.A. 115, 7368–7373. doi: 10.1073/pnas.1800918115
Wang, Y., Gong, J., Li, J., Xin, Y., Hao, Z., Chen, C., et al. (2020). Insights into bacterial diversity in compost: core microbiome and prevalence of potential pathogenic bacteria. Sci. Total Environ. 718:137304. doi: 10.1016/j.scitotenv.2020.137304
Wang, Z., Li, Y., Zhuang, L., Yu, Y., Liu, J., and Zhang, L. (2019). A rhizosphere-derived consortium of Bacillus subtilis and Trichoderma harzianum suppresses commonscab of potato and increases yield. Comput. Struct. Biotechnol. J. 17, 645–653. doi: 10.1016/j.csbj.2019.05.003
Wei, Z., Gu, Y., Friman, V.-P., Kowalchuk, G. A., Xu, Y., and Shen, Q. (2019). Initial soil microbiome composition and functioning predetermine future plant health. Sci. Adv. 5:eaaw0759. doi: 10.1126/sciadv.aaw0759
Wei, Z., Hu, J., Gu, Y., Yin, S., Xu, Y., Jousset, A., et al. (2018). Ralstonia solanacearum pathogen disrupts bacterial rhizosphere microbiome during an invasion. Soil Biol. Biochem. 118, 8–17. doi: 10.1016/j.soilbio.2017.11.012
Xu, J., Zhang, Y., Zhang, P., Trivedi, P., Riera, N., and Wang, Y. (2018). The structure and function of the global citrus rhizosphere microbiome. Nat. Commun. 9:4894.
Zhang, H., Sun, Y., Xie, X., Kim, M. S., Dowd, S. E., and Pare, P. W. (2009). A soil bacterium regulates plant acquisition of iron via deficiency-inducible mechanisms. Plant J. 58, 568–577. doi: 10.1111/j.1365-313x.2009.03803.x
Keywords: endophytic bacteria, 16S rRNA gene, Bacillus antagonism, Fusarium oxysporum, suppressiveness
Citation: Zhang Z, Li J, Zhang Z, Liu Y and Wei Y (2021) Tomato Endophytic Bacteria Composition and Mechanism of Suppressiveness of Wilt Disease (Fusarium oxysporum). Front. Microbiol. 12:731764. doi: 10.3389/fmicb.2021.731764
Received: 29 June 2021; Accepted: 16 September 2021;
Published: 15 October 2021.
Edited by:
Spyridon Ntougias, Democritus University of Thrace, GreeceReviewed by:
Dewa Ngurah Suprapta, Udayana University, IndonesiaRonaldo Dalio, Ideelab Biotecnologia, Brazil
Copyright © 2021 Zhang, Li, Zhang, Liu and Wei. This is an open-access article distributed under the terms of the Creative Commons Attribution License (CC BY). The use, distribution or reproduction in other forums is permitted, provided the original author(s) and the copyright owner(s) are credited and that the original publication in this journal is cited, in accordance with accepted academic practice. No use, distribution or reproduction is permitted which does not comply with these terms.
*Correspondence: Ji Li, NjQyNTY5NzI1QHFxLmNvbQ==