- Department of Cardiology, The Quzhou Affiliated Hospital of Wenzhou Medical University, Quzhou People’s Hospital, Quzhou, China
Hypertension is a significant risk factor for cardiovascular and cerebrovascular diseases, and its development involves multiple mechanisms. Gut microbiota has been reported to be closely linked to hypertension. Short-chain fatty acids (SCFAs)—the metabolites of gut microbiota—participate in hypertension development through various pathways, including specific receptors, immune system, autonomic nervous system, metabolic regulation and gene transcription. This article reviews the possible mechanisms of SCFAs in regulating blood pressure and the prospects of SCFAs as a target to prevent and treat hypertension.
Introduction
Hypertension is a global public health problem as well as an important risk factor for cardiovascular and cerebrovascular diseases (Rossier et al., 2017; Mills et al., 2020). Increase in the ageing population and lifestyle risk factors consequently increases the global prevalence of hypertension. As of 2010, 1.38 billion people (31.1% of the global adult population) suffer from hypertension (Mills et al., 2016). Consistent with the trend of hypertension prevalence, the number of cardiovascular and cerebrovascular deaths increased significantly from 1990 to 2015; hypertension is responsible for 40.1 and 40.4% of deaths caused by heart disease deaths and stroke deaths, respectively (Forouzanfar et al., 2017; Furie, 2020; Mills et al., 2020). Hypertension is a complex and multifactorial disease affected by both genetic and environmental factors (Rossier et al., 2017; Mills et al., 2020). In the genome-wide association study of Ehret et al. involving 342,415 individuals, numerous single gene variants related to blood pressure control and hypertension were identified, but these specific causal genes only explained a small proportion of the systolic pressure variation between individuals (<5%; Ehret et al., 2016). Therefore, identification of new diagnosis and treatment targets based on hypertension pathogenesis is urgently needed.
Gut microbiota and its metabolites play an important role in human health and diseases by affecting the body’s metabolism, immunity, nervous system and endocrine homeostasis (Fenneman et al., 2020; Verhaar et al., 2020; Yoo et al., 2020; Wu et al., 2021). Hypertension occurrence is often accompanied with gut microbiota imbalance, including decreased diversity, altered enterotype distribution and variation in bacterial populations (Li et al., 2017). It is mainly characterised by the increase in Klebsiella, Prevotella, Coprobacillus and Enterobacter populations and the decrease in Anaerotruncus, Coprococcus, Ruminococcus, Clostridium, Roseburia, Blautia and Bifidobacterium populations, which reduce ratio of Firmicutes/Bacteroidetes and the production of short-chain fatty acid (SCFA; Li et al., 2017; Verhaar et al., 2020). These observations provide a new perspective for hypertension diagnosis and treatment. SCFAs, which are the main metabolites produced by intestinal bacteria, ferment dietary fibre in the gastrointestinal tract. SCFAs can be effectively absorbed by the intestinal mucosa; they act as a source of energy, a regulator of gene expression, a participant in cell metabolism and a signal molecule recognised by specific receptors, thereby having an important impact on blood pressure regulation (Jin et al., 2019; Poll et al., 2020; Naqvi et al., 2021). This article summarises the possible mechanisms of SCFAs in regulating blood pressure and reviews the prospect of SCFAs as a target for hypertension prevention and treatment.
SCFAs and Blood Pressure
Short-chain fatty acids are an important link between the host and gut microbiota, which comprise different bacteria in the intestine, especially anaerobic bacteria, through dietary fibre fermentation (Kumar et al., 2020; Ge et al., 2021). SCFAs are organic fatty acids with fewer than six carbon atoms, and acetate, propionate and butyrate are considered the most important and biologically effective ones, accounting for 95% of the SCFAs produced by the gut microbiota (He et al., 2020). SCFAs are mainly produced in the colon and cecum, with a total concentration of approximately 150mmol/l. As the most abundant anion in the colon, SCFAs are absorbed in a concentration-dependent manner, transported to the portal vein by various transporters and then migrated to other organs through blood circulation (Nicholson et al., 2012; Liu et al., 2021). The proportion of the three main SCFAs (acetate, propionate and butyrate) is roughly 3:1:1, and they differ in their sources, distribution and potential effects on host physiology (He et al., 2020; Kumar et al., 2020; Liu et al., 2021). Acetate is the main SCFA in the colon produced by most of the Enterococcus species and is easily absorbed and transported to the liver. Propionate, produced by Bacteroidetes, Acidaminococcus and Salmonella, is also absorbed and transported to the liver, promoting intrahepatic gluconeogenesis. Furthermore, butyrate, which is produced by Clostridium, Eubacterium and Roseburia, can be used as the energy source of the intestinal mucosa and regulates cell proliferation and differentiation, with the hydroxylation product β-hydroxybutyrate (BHB) as its main effective component in the circulation (Wong et al., 2006; Nicholson et al., 2012; Louis and Flint, 2017; Sasaki et al., 2020; Liu et al., 2021).
Despite their low peripheral circulation concentration (0.1–10mM), as signal molecules, SCFAs are involved in different physiological and pathological processes of the host (Natarajan and Pluznick, 2014). Several animal model studies revealed that SCFAs can regulate blood pressure. In spontaneously hypertensive rats (SHR) and deoxycorticosterone acetate salt induced hypertensive rats (DHR), both high-fibre diet and acetate and propionate supplementation can significantly reduce the blood pressure levels (Pluznick et al., 2013; Marques et al., 2017). Yang et al. analysed the intestinal bacterial genome of stool samples and observed that the SCFA-producing microbiota was significantly less abundant in SHR than in the normal controls (Yang et al., 2015). Similarly, Holmes et al. found that SCFAs significantly correlated with blood pressure levels in East Asian and western population samples (Holmes et al., 2008). In a case report of patients with refractory hypertension, minocycline administration to inhibit intestinal microbiota could produce a powerful anti-hypertensive effect (Qi et al., 2015), which may be closely related to Firmicutes/Bacteroidetes reduction by minocycline (Pluznick, 2013; Yang et al., 2015). SCFAs not only directly affect hypertension progression but also regulate several hypertension-related syndromes, such as obesity, insulin sensitivity and diabetes (de la Cuesta-Zuluaga et al., 2018; Mandaliya and Seshadri, 2019; Zhi et al., 2019). Acetate and butyrate can be used as substrates for lipid synthesis, whereas propionate can serve as the medium of liver gluconeogenesis (Liu et al., 2021). Compared with the normal-weight group, the obesity group had an altered SCFA composition in the faeces in which the proportion of acetate was relatively lower (Schwiertz et al., 2010). SCFAs are increasingly proven to be widely involved in body-weight regulation, energy metabolism balance, lipid metabolism and other pathophysiological processes (den Besten et al., 2015; Hu et al., 2018; Barrea et al., 2019), which cross-correlated with hypertension.
Possible Mechanism of SCFAs Regulating Blood Pressure
Short-chain fatty acids have an important impact on blood pressure regulation, whereas hypertension occurrence is typically accompanied with the decrease in SCFAs production. Moreover, a number of potential mechanisms have been proposed to explain this association, including specific receptors, immune system, autonomic nervous system, metabolic regulation, cell senescence and gene transcription.
G-Protein-Coupled Receptors
Through receptor binding, SCFAs can directly regulate blood pressure. The currently discovered SCFA receptors are mainly G-protein-coupled receptors (GPR), such as GPR41, GPR43, GPR109A and olfactory receptor (Olfr) 78 (Priyadarshini et al., 2018; Muralitharan et al., 2020; Poll et al., 2020; Yao et al., 2020). Interestingly, SCFAs binding to different receptors play a diametrically opposite role in blood pressure regulation (Bolognini et al., 2016; Pluznick, 2017).
GPR41 and GPR43 are widely distributed throughout the body. They are activated when they bind to acetate, propionate and butyrate (Kim et al., 2013; Kimura et al., 2020; Muralitharan and Marques, 2021), whereas GPR109A specifically binds to butyrate and BHB (Thangaraju et al., 2009). GPR41 could be expressed in vascular smooth muscle cells and endothelial cells; its expression is necessary for SCFA-mediated vasodilation (Natarajan et al., 2016). Natarajan et al. added propionate to the diet of GPR41−/− and GPR41+/− mice; consequently, propionate produced an anti-hypertensive effect in the latter and an opposite effect in the former (Natarajan et al., 2016). Blood pressure was significantly higher in GPR41-knockout mice than in wild-type mice, with a more pronounced systolic pressure level (Natarajan et al., 2016). After GPR41 knockout, mice exhibited thickening of aorta and increase in vascular collagen, consequently causing vascular fibrosis and hypertension (Natarajan et al., 2016). Similarly, Onyszkiewicz et al. demonstrated that butyrate can pass into the bloodstream through the gut–vascular barrier and act on GPR41/GPR43 to relax the mesenteric artery, thereby significantly mitigating hypertension (Onyszkiewicz et al., 2019).
The Olfr is a seven-pass transmembrane GPR (known as Olfr78 in mice and OR51E2 in humans) that also serves as a receptor for SCFAs, especially acetate and propionate (Segers et al., 2019; Kotlo et al., 2020). In mice, Olfr78 is mainly distributed in the kidneys and blood vessels; when activated, it can increase blood pressure, possibly because it affects the vascular smooth muscle cells in renal afferent arterioles and peripheral blood vessels (Pluznick et al., 2013; Miyamoto et al., 2016). The renal afferent arteriole is the main site for renin secretion and storage. In Olfr78-knockout mice, plasma renin and blood pressure levels are decreased (Pluznick, 2014; Miyamoto et al., 2016). Treating Olfr78-knockout and non-knockout mice with an identical dose of propionate, the former showed lower blood pressure levels; this may be caused by the stimulation of cyclic adenosine monophosphate (cAMP) production in glomerular cells, resulting in renin release (Pluznick et al., 2013; Pluznick, 2014). In peripheral vascular smooth muscle cells, Olfr78 expression may affect the baseline blood pressure (Hsu et al., 2018). Moreover, propionate mediated by Olfr78 receptors can produce a blood pressure-boosting effect, mainly by resisting its powerful anti-hypertensive effect caused by other receptors or pathways (Pluznick et al., 2013; Pluznick, 2017).
Olfr78 and GPR41 produce effects through different G protein-α subunits and second-messenger systems; Olfr78 activates adenylate cyclase type 3 (AC3) and Golf in the olfactory signalling pathway to induce cAMP production, while GPR41 and GPR43 activate Gαi and/or Gαo to decrease cAMP (Pluznick et al., 2013). After being activated by SCFAs, these receptors are coupled with different second messengers and generate opposite effects on blood pressure (Pluznick et al., 2013). Therefore, the mechanisms and physiological effects of SCFAs on blood pressure regulation are complex and diverse, and the abovementioned contrasting effect may explain the blood pressure fluctuations mediated by SCFA level alteration (Figure 1). Pluznick et al. reported that exogenous propionate injection (0.1mmol) into mice could cause a large (20mm Hg) and rapid (within 1–2min) blood pressure drop, which would then return to the normal level within 5min; after the injection, the blood pressure level was lower in Olfr78−/− mice than in wild-type mice (11.9±1.6mm Hg vs. 5.5±0.5mm Hg; p<0.000013). In addition, to verify the role of GPR41 receptor in blood pressure regulation, they used the same method and found that after exogenous propionate injection (10mmol, maximum physiological dose), the blood pressure level slightly decreased in GPR41+/− mice (2.9±1.6mmHg) and moderately increased in GPR41−/− mice (4.5±2.4mmHg; Pluznick et al., 2013; Pluznick, 2014, 2017). Thus, after exogenous SCFA administration, the blood pressure of Olfr78−/− mice would be greatly reduced. The reason could be that SCFAs could only bind to GPR41 to exert an anti-hypertensive effect, with no Olfr78 antagonism; in contrast, SCFAs in GPR41−/− mice could only bind to Olfr78, with no GPR41, thereby increasing blood pressure (Pluznick et al., 2013; Pluznick, 2014, 2017). The opposite effect of SCFAs on blood pressure regulation may be related to the different sensitivity of Olfr78 and GPR41 towards SCFAs. Plasma SCFAs at basal concentrations (0.1–0.9mmol) could activate GPR41 to induce vasodilation and lower blood pressure; conversely, SCFAs with a higher concentration (0.9mmol) could activate Olfr78 to increase renin release and blood pressure levels (Miyamoto et al., 2016). Given that Olfr78 can adjust the inappropriate GPR41/GPR43-mediated hypotension resulting from excessively elevated circulating SCFA levels, the acute hypotensive effect of propionate is accentuated at low physiological doses (Pluznick, 2014). Hence, the role of different SCFA concentrations in different tissues must be determined.
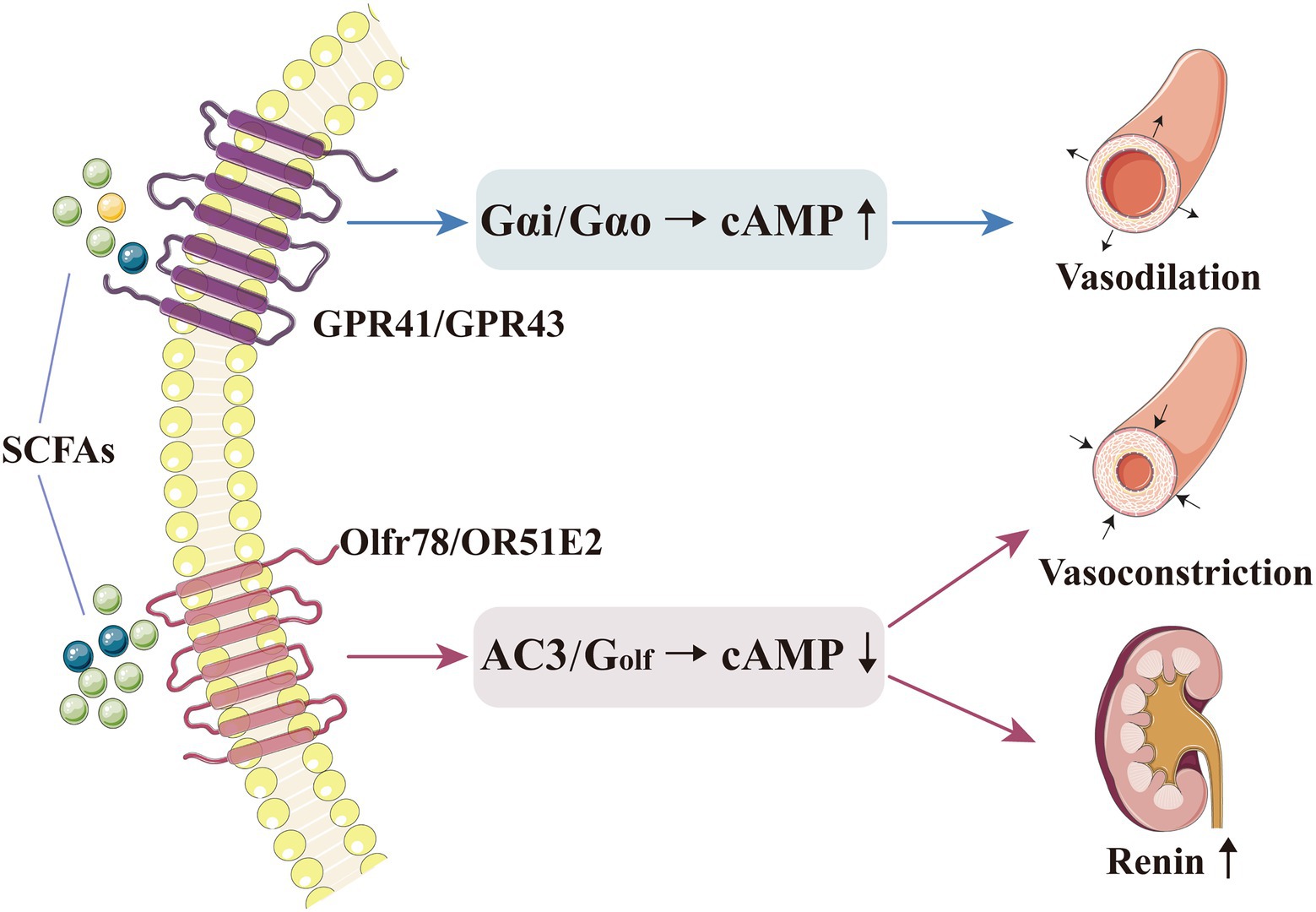
Figure 1. SCFAs can directly regulate blood pressure by binding to their receptors. Basal concentration of SCFAs could activate GPR41/GPR43, which triggers Gαi and/or Gαo to decrease cAMP, thereby inducing vasodilation and lowering the blood pressure level. A higher concentration of SCFAs could activate Olfr78, which triggers AC3 and Golf in the olfactory signalling pathway to induce cAMP production, thereby increasing renin release and inducing vasoconstriction. AC3, adenylate cyclase type 3; cAMP, cyclic adenosine monophosphate; GPR, G-protein-coupled receptor; Olfr, olfactory receptor; and SCFA, short-chain fatty acid.
Immunoregulation
The immune system and excessive inflammation have been increasingly proven to participate in hypertension development. T-lymphocyte subsets, such as T helper (Th) 1, Th2, Th17, regulatory T (Treg) and CD8+ T cells, are involved in regulating blood pressure and tissue damage (Wenzel et al., 2016; Ren and Crowley, 2019). SCFAs are important regulators of immune pathways, including intestinal and immune homeostasis, inflammatory cell biology and inflammatory response (Li et al., 2018b; Parada Venegas et al., 2019; Ratajczak et al., 2019). Bartolomaeus et al. observed that in mice with hypertension induced by angiotensin (Ang) II, propionate attenuated the response of various T cells to Ang II, such as Th17 and memory T-cell reduction, thereby lowering the blood pressure level; this process was confirmed to be Treg-dependent (Bartolomaeus et al., 2019). Moreover, butyrate can regulate hypertension occurrence and development through the immune response. In vitro, butyrate can decrease the interleukin (IL)-6 and tumour necrosis factor-α (TNF-α) levels caused by Ang II and induce Treg differentiation in vivo and in vitro; this SCFA can also reverse the elevated Th17 and IL-17 levels in patients with hypertension (Furusawa et al., 2013; Ohira et al., 2013; Singh et al., 2014; Wang et al., 2017; Kim et al., 2018). In mice, a high-fibre diet or acetate supplementation can significantly reduce systolic and diastolic pressure and improve cardiac fibrosis and left-ventricular hypertrophy, related to the downregulation of the signal transduction of the proinflammatory cytokine IL-1 in the kidney (Marques et al., 2017). For salt-sensitive hypertension, the exogenous supplementation of BHB precursor inhibits the formation of the renal inflammasome NOD-, LRR- and pyrin domain-containing protein 3, thereby attenuating hypertension (Chakraborty et al., 2018).
The anti-inflammatory effects of SCFAs (especially butyrate) may be mediated by histone deacetylase (HDAC) inhibition in vascular endothelial cells (Chang et al., 2014; Li et al., 2018a; Yang et al., 2020). HDAC inhibition contributes to the prevention of vascular inflammation and related diseases; in SHR, HDAC activation is closely related to hypertension (Cardinale et al., 2010; Chun, 2020; Li et al., 2020). Verdin et al. proved that as an HDAC inhibitor, BHB could increase the histone acetylation level, promote the expression of the antioxidants factor forkhead box O3a (FOXO3a) and metallothionein 2 (MT2) and ultimately protect the body from oxidative stress (Shimazu et al., 2013). Furthermore, the butyrate and valerate levels in the faeces of patients with preeclampsia were significantly decreased; correspondingly, butyrate directly downregulated lipopolysaccharide-induced hypertension in preeclampsia rats by regulating macrophage function and inhibiting HDAC (Chang et al., 2014). In addition, butyrate injection in Npr1+/− mice significantly lowered the blood pressure levels and reduced renal inflammation and fibrosis by inhibiting HDAC (Kumar et al., 2017).
In addition to providing energy to the intestinal epithelium, SCFAs promote the integrity of the intestinal epithelium and help repair the damaged epithelium (D’Souza et al., 2017). Thus, inhibition of these mechanisms are inhibited would lead to uncontrolled infiltration between the lumen and adjacent vessels, thereby inducing systemic inflammation and subsequently participating in hypertension pathogenesis (Jama et al., 2019). SCFAs can also promote the secretion of anti-inflammatory intestinal hormones, including glucagon-like peptide 2 (GLP-2; Onal et al., 2019). As a specific intestinal growth factor, GLP-2 can promote the growth of normal intestinal mucosa, repair the damaged intestinal epithelium and protect the intestinal mucosal barrier (Brubaker, 2018; Chang et al., 2021). Moreover, SCFAs, especially butyrate, can enhance the β-oxidation process of the cells in the intestinal mucosa, consume oxygen in the intestinal lumen and then create a favourable intestinal microenvironment to promote the growth of beneficial bacteria and inhibit the proliferation of potential pathogenic microorganisms; ultimately, the immune inflammatory response related to hypertension is alleviated, and target organ damage is relieved (Cani, 2017; Guan et al., 2020). Similarly, Kim et al. confirmed that butyrate treatment in mice with Ang II-induced hypertension can ameliorate microbial imbalance and intestinal barrier dysfunction, thereby reducing the mean arterial pressure (Kim et al., 2018). Therefore, the metabolites of gut microbiota can protect host’s health locally by regulating the intestinal barrier and immune response (Figure 2).
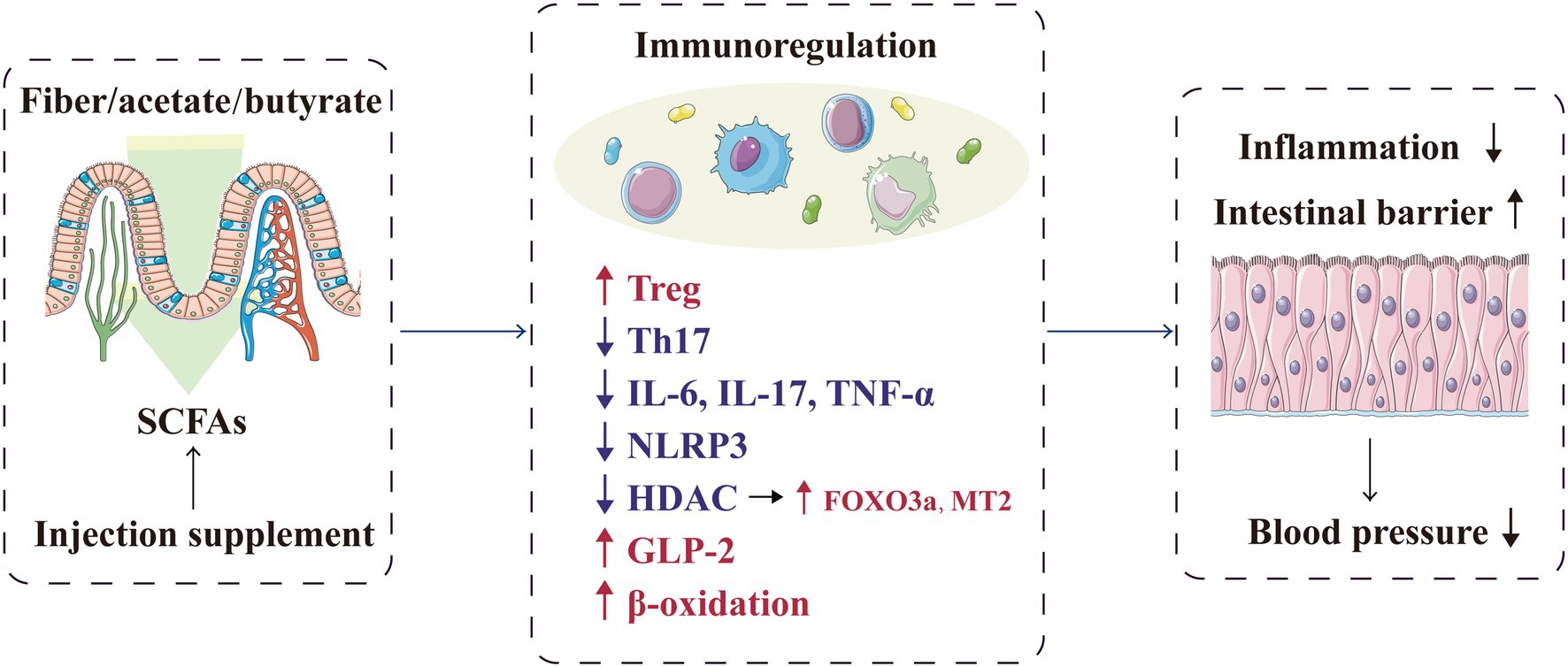
Figure 2. SCFAs play a local protective role in blood pressure regulation by regulating the intestinal barrier and immune response. FOXO3a, forkhead box O-3; GLP-2, glucagon-like peptide 2; HDAC, histone deacetylase; IL, interleukin; MT2, metallothionein-2; NLRP3, NOD-, LRR- and pyrin domain-containing protein 3; SCFA, short-chain fatty acid, TH17, T helper cell 17; TNF-α, tumour necrosis factor-α; and Treg, regulatory T cell.
Autonomic Nervous System
Hypertension occurrence is closely related to autonomic nervous dysfunction (Mancia and Grassi, 2014; Kalla et al., 2016). In SHR, long-term stimulation of cardiac vagus nerve preganglionic neurons could lower blood pressure (Moreira et al., 2018), whereas short-term vagus nerve stimulation could improve the prognosis of rats with salt-sensitive hypertension (Annoni et al., 2019). The microbiota–gut–brain axis is a complex neuro–humoral communication network that maintains body homeostasis; it consists of gut microbiota, enteric nervous system, central nervous system and autonomic nervous system and its related sympathetic and parasympathetic branches. Through this axis, SCFAs can regulate blood pressure (Dalile et al., 2019; Zubcevic et al., 2019; Figure 3). The sympathetic and parasympathetic ganglia express SCFA receptors, such as Olfr78, GPR41 and GPR43. By acting on the receptors expressed in the sympathetic ganglia, SCFAs can directly regulate the sympathetic nervous system (Kimura et al., 2011; Nohr et al., 2015), and through the receptors expressed in the parasympathetic ganglia, SCFAs can affect the neural feedback in the gut (Zubcevic et al., 2019), Therefore, SCFAs participate in the neural regulation mechanism of blood pressure.
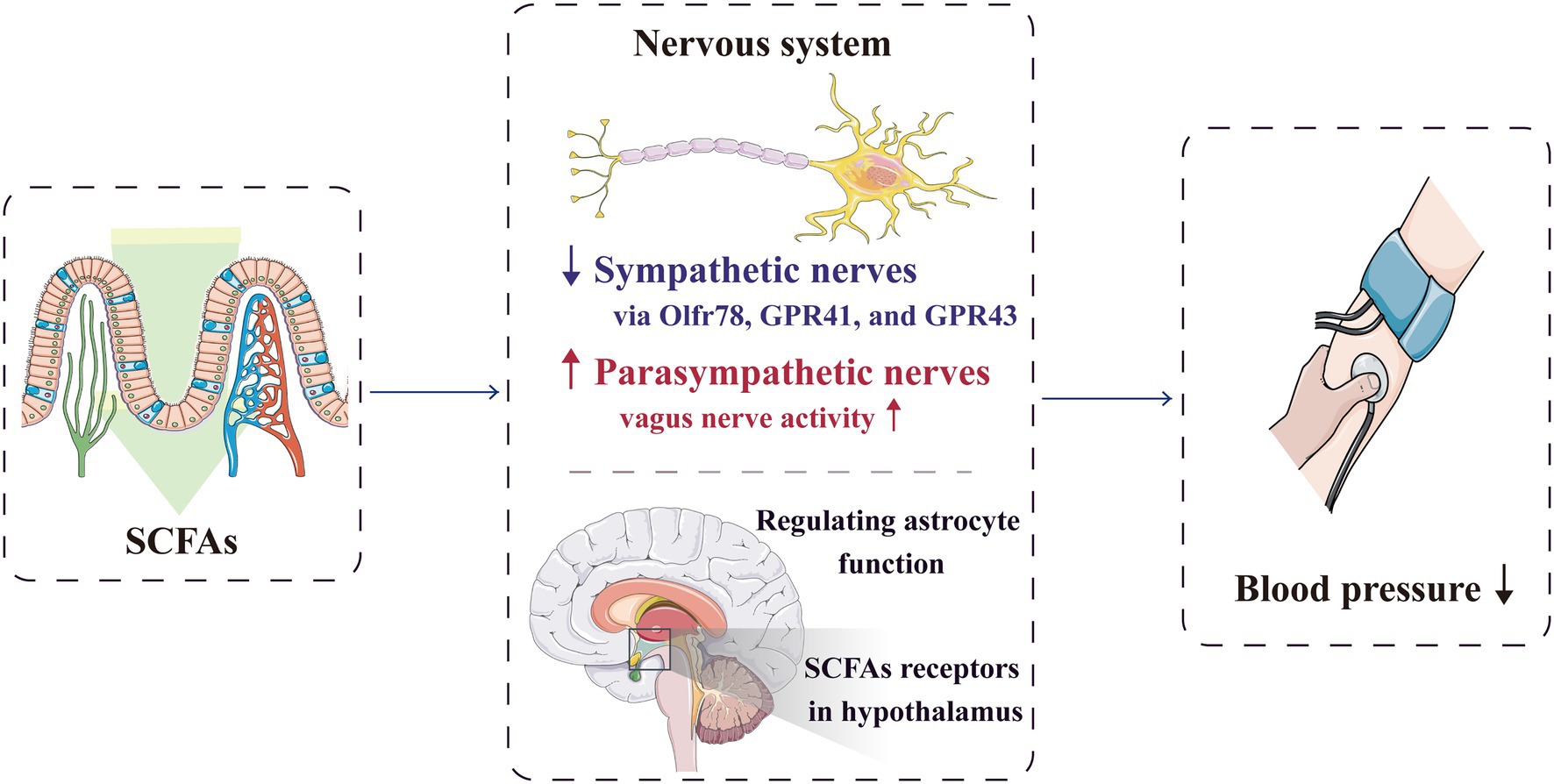
Figure 3. SCFAs can regulate blood pressure through the nervous system. By acting on the receptors expressed in the sympathetic ganglia, SCFAs can directly regulate the sympathetic nervous system. They can also significantly activate vagal afferent neurons, which facilitate SCFAs to regulate blood pressure. Furthermore, SCFAs can directly act on the central nervous system to reduce the blood pressure. GPR, G-protein-coupled receptors; Olfr, olfactory receptor; and SCFA, short-chain fatty acid.
Lal et al. reported that SCFAs, especially butyrate, can directly activate the afferent nerve fibres of vagus nerve after being absorbed into the nerve endings of intestinal mucosal lamina propria, which help SCFAs in blood pressure regulation (Lal et al., 2001). Moreover, Goswami et al. showed that SCFAs can significantly activate vagal afferent neurons by increasing phosphorylation, with the butyrate>propionate>acetate effect (Goswami et al., 2018). After transection of the sub-phrenic vagus nerve and pre-treatment of the colon with a nonspecific antagonist of GPR41/GPR43, the anti-hypertensive effect of butyrate is weakened; therefore, the stimulation of butyrate on the vagal afferent fibres may partly cause the anti-hypertensive effect (Onyszkiewicz et al., 2019).
Additionally, butyrate can cross the blood–brain barrier through specific transporters and directly act on the central nervous system to regulate blood pressure; for instance, butyrate improves the function of astrocytes in the central nucleus (Vijay and Morris, 2014; Yang et al., 2018). SCFA receptors are also present in the para-ventricular nucleus, and injecting butyrate into the lateral ventricle can significantly reduce blood pressure levels in both the SHR and control groups (Yang et al., 2019). In addition, butyrate receptors in the hypothalamus of SHR are less expressed, resulting in decreased reactivity; therefore, the role of butyrate in blood pressure regulation is affected (Yang et al., 2019).
Metabolism
According to epidemiological and animal data analyses, hypertension and other metabolic disorders, such as diabetes and obesity, have an extremely close and reciprocal causal relationship (Velarde and Berk, 2005; Katsimardou et al., 2020; Litwin and Kulaga, 2021). The role of SCFAs in obesity and metabolic regulation (glucose and lipid metabolism) has attracted increasing attention, and these metabolites may indirectly regulate blood pressure by participating in metabolism (Figure 4; Frampton et al., 2020; He et al., 2020; Machate et al., 2020). After being produced by gut microbiota, SCFAs are initially used by intestinal epithelial cells as an energy source, and they can activate intestinal gluconeogenesis (IGN), which is important in maintaining normal blood glucose level and energy homeostasis (De Vadder et al., 2014). SCFAs also circulate through the blood vessels and enter the liver and muscles to regulate energy metabolism. The SCFA propionate is a good precursor for glycolipid and protein syntheses (Wilson et al., 2017). Acetate, which is also an SCFA, is a matrix for cholesterol synthesis (den Besten et al., 2013); it can directly cross the blood–brain barrier and act on the hypothalamus to inhibit appetite (Frost et al., 2014; Hartstra et al., 2015). Moreover, oral (rather than intravenous) butyrate can reduce food intake and improve glucose and lipid distribution through the gut–brain axis in animals (Li et al., 2018c), whereas oral propionate can increase fat oxidation in humans (Chambers et al., 2018).
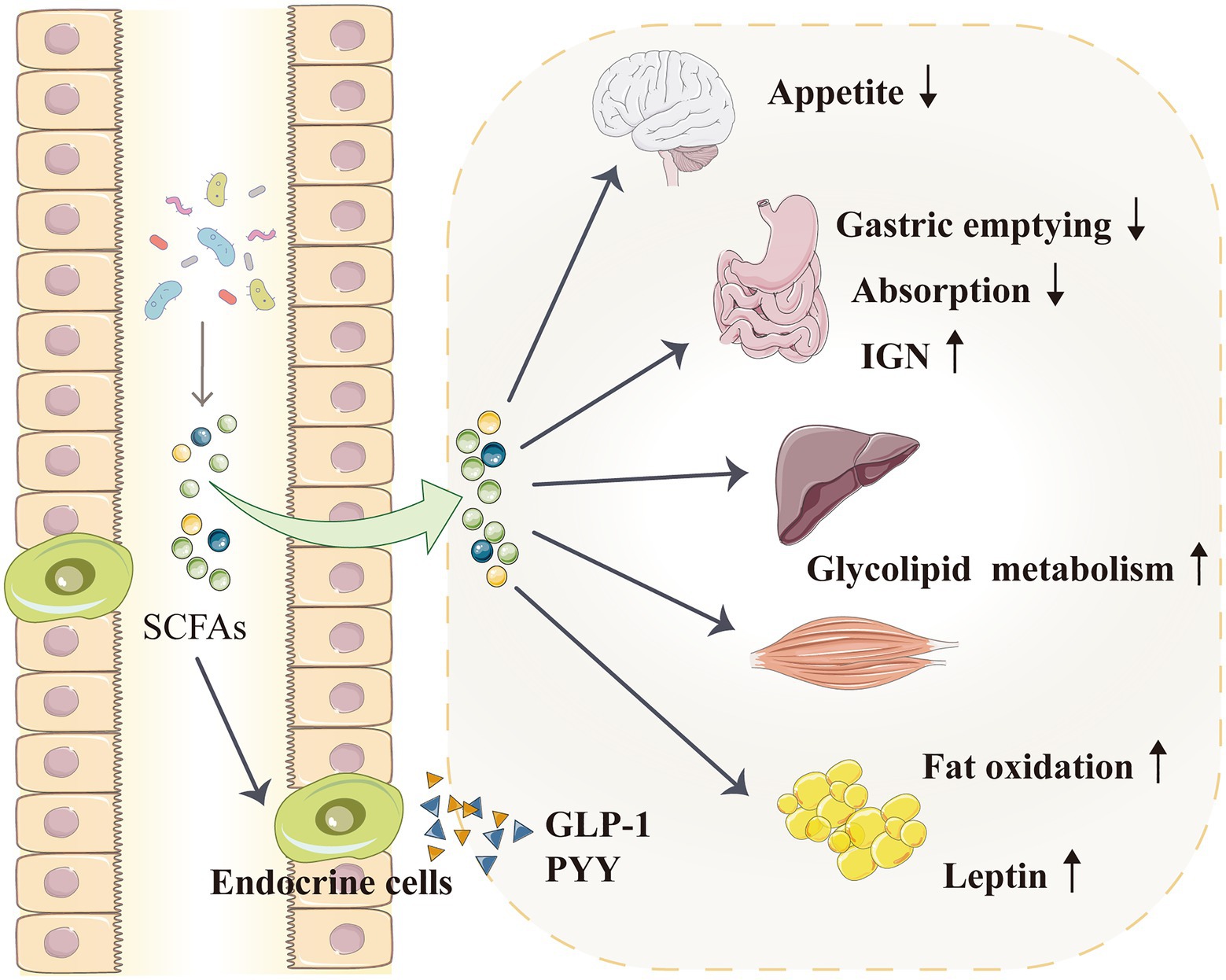
Figure 4. SCFAs indirectly regulate blood pressure by affecting the metabolism. SCFAs can activate IGN, which is involved in maintaining normal blood glucose levels and energy homeostasis. SCFAs enter the liver and muscles to improve glycolipid metabolism. SCFAs can also directly enter the blood–brain barrier and act on the hypothalamus to inhibit appetite. Furthermore, SCFAs promote the secretion of intestinal hormones, such as GLP-1 and PYY, which can slow down gastric emptying and reduce food energy absorption. SCFAs can also increase fat oxidation and promote the secretion of leptin from adipocytes; leptin is a typical metabolic hormone that reduces food intake, increases energy release and reduces body mass. GLP-1, glucagon-like peptide 1; IGN, intestinal gluconeogenesis; PYY, peptide tyrosine tyrosine; and SCFA, short-chain fatty acid.
Through the GPRs on the surface of intestinal endocrine cells, SCFAs promote the secretion of intestinal hormones, such as glucagon-like peptide-1 (GLP-1) and peptide tyrosine tyrosine (PYY; Zhang et al., 2019a; Ren et al., 2020; Wang et al., 2020; Nishida et al., 2021). In particular, GLP-1 enhances glucose tolerance and regulate metabolism (Suzuki and Aoe, 2021), whereas PYY increases satiety, reduces food intake, regulates intestinal movement and slows down gastric emptying to improve body metabolism (Freire and Alvarez-Leite, 2020; Nishida et al., 2021). GPRs affect the influence of gut microbiota on the body’s energy, i.e. when intestinal GPR activation is inhibited, food energy absorption would be reduced (Samuel et al., 2008). In overweight people, propionate promotes PYY and GLP-1 secretion, improves insulin sensitivity and reduces food intake; its long-term use can control weight gain and reduce abdominal fat (Chambers et al., 2015b).
Short-chain fatty acids can also promote leptin secretion from adipocytes; leptin is a typical metabolic hormone that reduces food intake, increases energy release and reduces body mass (Chambers et al., 2015a; Wang et al., 2020). Insulin signalling in adipocytes can also be inhibited by SCFAs, leading to the prevention of insulin-mediated fat accumulation and promotion of the metabolism of unbound lipids and glucose in other tissues (Kimura et al., 2013; Jiao et al., 2020). A pig model study suggested that oral SCFAs—acetic, propionic and butyric acids—can decrease serum triglyceride, total cholesterol and low-density lipoprotein cholesterol levels and increase serum GLP-1, PYY and leptin levels, thereby reducing fat deposition (Jiao et al., 2018, 2020). Evidence from mouse and human research also supports that SCFAs regulate lipogenesis and attenuate lipolysis (Ohira et al., 2016; Ivan et al., 2017; Aguilar et al., 2018).
Cell Senescence
Decreased vascular elasticity and compliance lead to increased vascular wall stiffness, which is the most important feature of hypertension; furthermore, hypertension occurs when endothelial cell senescence leads to the increase in intimal stiffness (Kaess et al., 2012; Tian and Li, 2014; Li et al., 2019). In cardiovascular diseases, P53 is a key regulator of the senescence of endothelial cells and vascular smooth muscle cells (Men et al., 2021). As a vascular protective factor, BHB increases p53 acetylation via HDAC inhibition and weakens its activity; consequently, the expression of the downstream genes p21 and PUMA decreases to attenuate cellular apoptosis (Newman and Verdin, 2014; Liu et al., 2019). BHB also significantly inhibits stress-induced premature ageing and replicative senescence through the p53-independent pathway. Furthermore, BHB indirectly increases lamin B1 level by enhancing the expression of the transcription factor octamer-binding transcriptional factor (OCT) 4, which is important for preventing senescence induced by DNA damage (Han et al., 2018; Mendelsohn and Larrick, 2018). Therefore, the protective effect of BHB on hypertension is possibly mediated by delaying vascular stiffness associated with endothelial cell senescence (Figure 5).
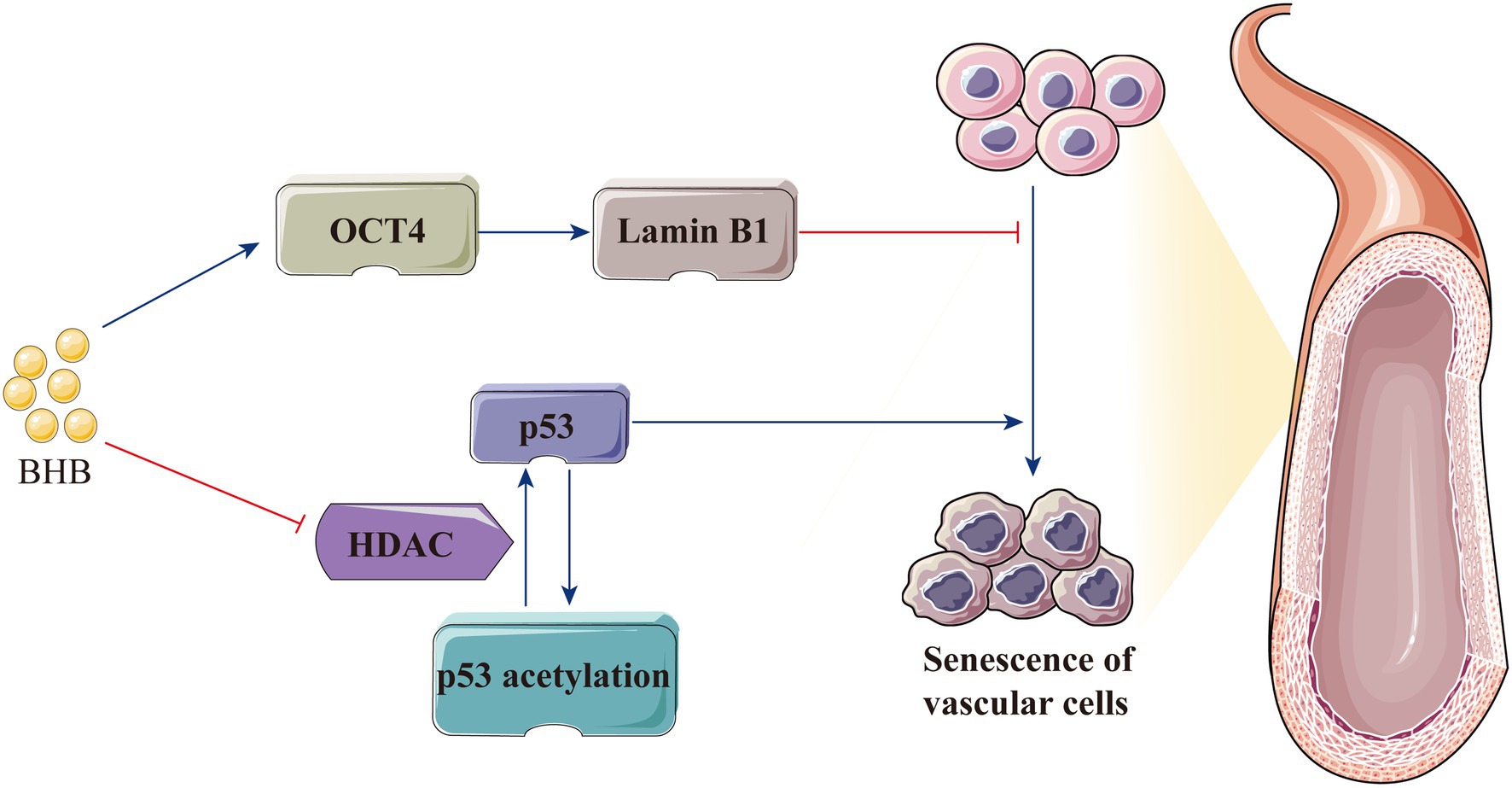
Figure 5. BHB protects the body against hypertension by delaying vascular stiffness associated with endothelial cell senescence. BHB indirectly increases the lamin B1 level by enhancing the expression of OCT4 and increases p53 acetylation by HDAC inhibition, thereby attenuating cellular apoptosis and delaying endothelial cell senescence. BHB, β-hydroxybutyrate; HDAC, histone deacetylase; and OCT4, octamer-binding transcriptional factor 4.
Gene Transcription
Short-chain fatty acids can prevent hypertension by regulating the hypertension-associated gene transcription in the gut–heart–renal axis (Figure 6). Marques et al. conducted RNA sequencing on the heart and kidney transcriptomes of mice fed with a standard fibre diet, a high-fibre diet or an acetate diet for 3weeks. They found that high-fibre and acetate intake affected the expression of genes associated with heart disease and hypertension, including Rasal1 [associated with renal fibrosis (Bechtel et al., 2010)], Cyp4a14 [encoding a protein that regulates body fluid absorption through sodium channels (Nakagawa et al., 2006)] and Cck [associated with the anti-inflammatory process (Miyamoto et al., 2012)], and genes that regulate the renin–angiotensin–aldosterone system (Marques et al., 2017). SCFAs also upregulated genes related to circadian rhythm and downregulated genes related to the mitogen-activated protein kinase signalling pathway (Marques et al., 2017; Segers et al., 2019). Cardiovascular pathology is mainly regulated by the gene encoding early growth response-1 (Wang et al., 2014; Ho et al., 2016), which is significantly downregulated in the kidney and heart with fibre intake or acetate supplementation (Marques et al., 2017). Furthermore, SCFAs can promote the expression of genes encoding proteins involved in blood pressure regulation; these genes include atrial natriuretic peptide and brain natriuretic peptide (Thorburn et al., 2015).
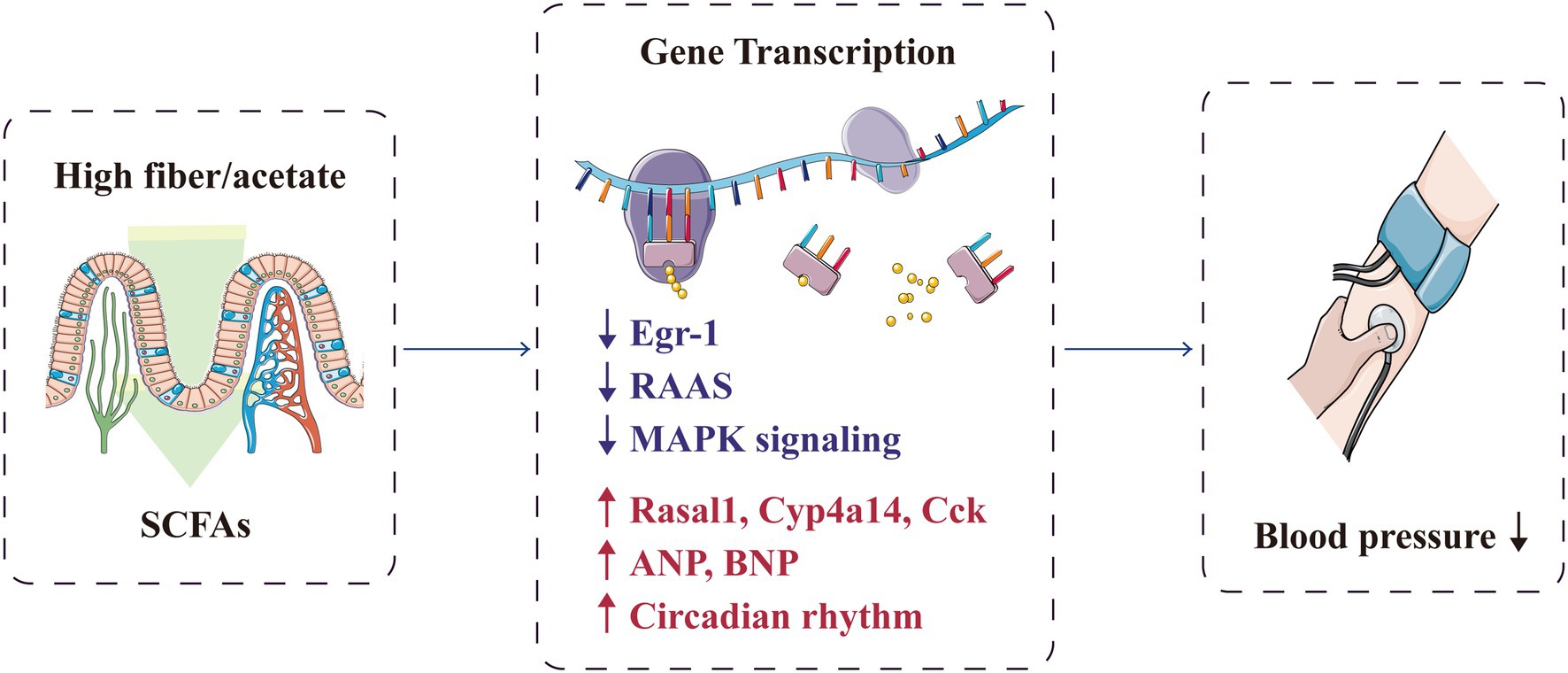
Figure 6. SCFAs protectively regulate the transcription of genes associated with hypertension. ANP, atrial natriuretic peptide; BNP, brain natriuretic peptide; Egr-1, early growth response-1; MAPK, mitogen-activated protein kinase; RAAS, renin–angiotensin–aldosterone system; and SCFA, short-chain fatty acid.
SCFAs as a Target for the Prevention and Treatment of Hypertension
Short-chain fatty acids are closely related to hypertension occurrence and prognosis, and increasing the production of SCFAs can improve cardiovascular homeostasis, suggesting a potential target for reducing cardiovascular risk (Jadoon et al., 2018; Almeida et al., 2021; Figure 7). Although the available data are still very preliminary, SCFAs have been found to be related to the metabolism of anti-hypertensive drugs, and regulation of the gut microbiota metabolites, such as SCFAs, may help attenuate drug resistance in patients with refractory hypertension (Qi et al., 2015; Zhernakova et al., 2016). Internal and external factors, such as gut microbiota, diet and intracellular regulatory factors, dynamically regulate SCFAs and the corresponding acylation (Chen et al., 2020). The current main methods for intervening and regulating SCFA production are as follows:
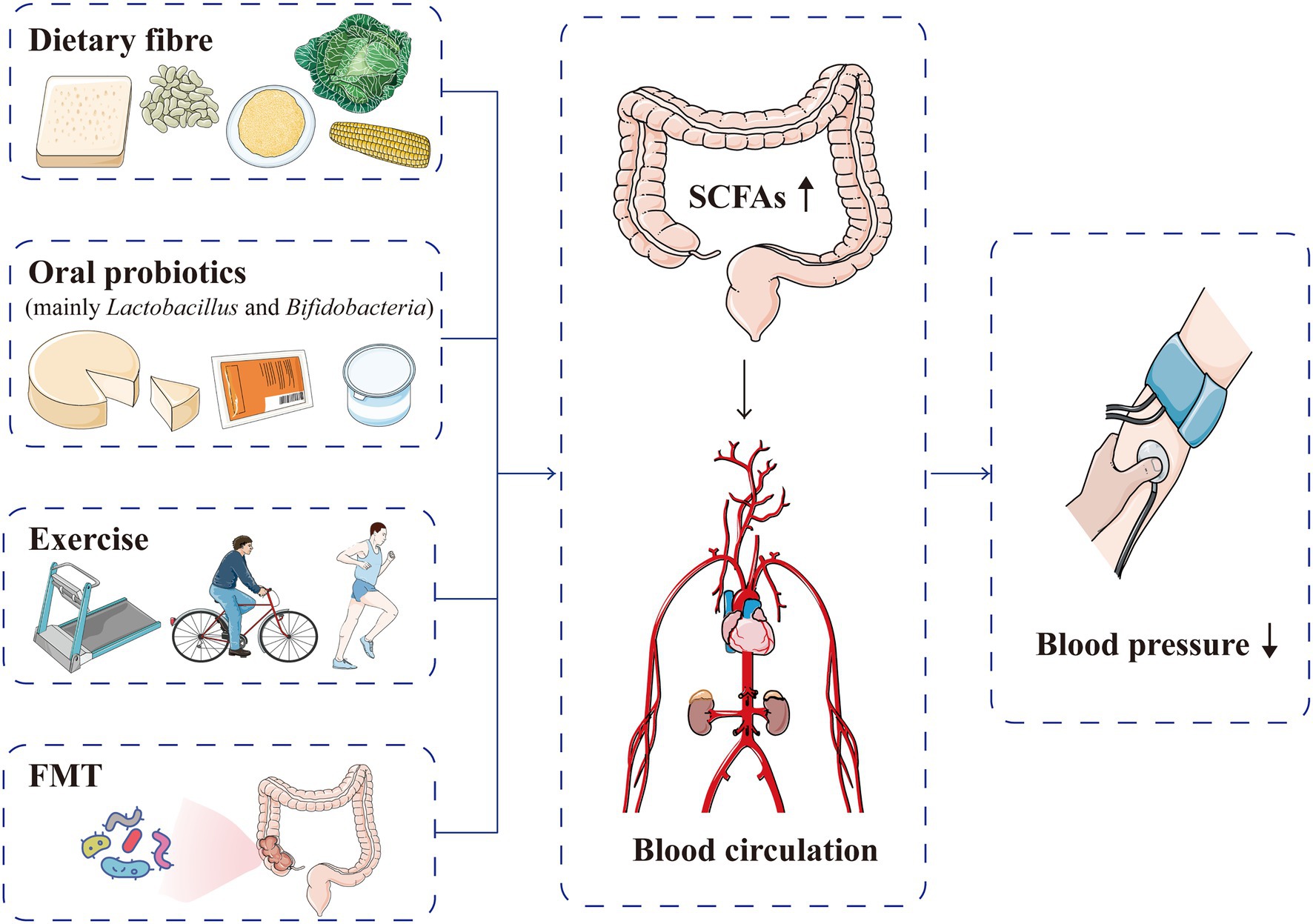
Figure 7. Current methods used to intervene and regulate SCFA production for blood pressure normalisation. Dietary fibre, oral probiotics, exercise and FMT can increase the production of SCFAs, which are absorbed into the blood circulation and subsequently lower blood pressure levels. FMT, faecal microflora transplantation; SCFA, short-chain fatty acid.
Dietary Adjustment
The effect of diet on blood pressure has been investigated in recent decades. A high-salt diet alters the production of SCFAs by disrupting the composition of gut microbiota; thus, hypertension develops (Bier et al., 2018). Meanwhile, a high-fibre diet and dietary fibre supplementation could increase the circulating levels of SCFAs and subsequently reduce blood pressure (Myint et al., 2018; Zhai et al., 2018; Zhao et al., 2018). In an experiment using a hypertensive rat model, Marques et al. indicated that the systolic and diastolic pressure levels were significantly lower in DHR with high-fibre diet or acetate supplementation than in the control group (Marques et al., 2017). A high-fibre diet can reduce systolic pressure from 116±19mmHg (baseline) to 91±5mmHg and diastolic pressure from 75±5mmHg to 58±5mmHg, whereas acetate supplementation can reduce systolic pressure from 116±19mmHg to 85±9mmHg and diastolic pressure from 75±5mmHg to 54±5mmHg (Marques et al., 2017). Hence, a high-fibre diet can change the composition of gut microbiota by increasing the level of Bacteroidetes and acetate-producing enterobacteria, which exert a hypotensive effect through acetate production (Marques et al., 2017). Similarly, in clinical studies, dietary supplementation with SCFAs has also achieved beneficial effects. Streppel et al. systematically reviewed 24 clinical trials (published from 1966 to 2003) investigating on blood pressure reduction by fibre diet. They found that an average daily intake of 11.5g of fibre food could reduce systolic pressure by 1.13mm Hg (95% CI, −2.49 to 0.23) and diastolic pressure by 1.26mm Hg (95% CI, −2.04 to −0.48); these results were more significantly observed in people aged over 40years and those with hypertension (Streppel et al., 2005). Although the specific effects and mechanisms of dietary structure on SCFAs and hypertension remain obscure, increasing the intake of dietary fibre generally increases the proportion of SCFA-producing bacteria in the intestinal microbes and relatively augments SCFA production, thereby reducing the risk of hypertension.
Oral Probiotics
Probiotics are living microorganisms that are beneficial to the health of the host; clinically, they mainly consist of Lactobacillus and Bifidobacteria (Wegh et al., 2019; Markowiak-Kopec and Slizewska, 2020). When carbohydrate is lacking, Bifidobacteria can produce acetate and formate through glycolysis; when carbohydrate is sufficient, acetate and lactate are produced (Aoki et al., 2017). In rat models, Lactobacillus intake could lower blood pressure levels, related to the improvement of the intestinal barrier function and the production of peptides to inhibit angiotensin-converting enzyme (Nakamura et al., 1995; Robles-Vera et al., 2020). In a recent meta-analysis of 23 randomised controlled studies, probiotics could lower systolic pressure levels by 3.05mmHg and diastolic pressure levels by 1.51mmHg in 2037 adults with or without hypertension (Qi et al., 2020). However, the anti-hypertensive effect of probiotics is inconsistent in different populations; this effect is more evident in Japanese patients and patients with hypertension, suggesting a relationship with genetic or environmental factors (Dong et al., 2013; Qi et al., 2020). The anti-hypertensive effect of probiotics can only last for a short period of time (8 or 10weeks), with only a slight reduction in blood pressure (Qi et al., 2020); hence, probiotic supplementation may be used as an adjuvant therapy. Currently, the regulation of SCFA production by probiotics for hypertension treatment has not yet been investigated. Thus, future studies should focus on clarifying how probiotics influence blood pressure by gut microbiota metabolites.
Exercise
Exercise is an effective and safe nondrug treatment for many metabolic diseases. Moderate exercise combined with diet control can improve cardiovascular disorders, such as hypertension (Keating et al., 2020; Saco-Ledo et al., 2020). Through exercise, the composition of gut microbiota and the metabolites changes (Monda et al., 2017; Mohr et al., 2020). Allen et al. demonstrated that compared with the non-exercise group, the SCFAs in stool samples increased significantly after 6weeks of endurance exercise in lean participants, but not in those with obesity; when the exercise training was stopped, the exercise-induced changes of microbiota were basically reversed (Allen et al., 2018). The effect of moderate exercise on SCFAs provides a new possible mechanism to explain how exercise prevents and treats hypertension, suggesting an interesting research topic.
Faecal Microflora Transplantation
Faecal microflora transplantation (FMT) has been applied in the clinical treatment of inflammatory bowel disease and metabolic syndrome; it increases the diversity of gut microbiota, thereby changing the composition of metabolites (Weingarden and Vaughn, 2017; Costello et al., 2019; Zhang et al., 2019b). Animal model studies have explored the effect of FMT on hypertension. Li et al. transplanted stool samples separately from healthy and hypertensive individuals into sterile mice and reported that the systolic and diastolic pressure levels of mice receiving faecal transplants from patients with hypertension were higher than those of mice receiving faecal transplants from healthy individuals; hence, blood pressure increase could be transmitted through gut microbiota (Li et al., 2017). Although relevant clinical reports on hypertension treatment with FMT remain unavailable, recent studies have shown that patients with FMT have a significantly increased production of SCFAs (Seekatz et al., 2018). With further clarification of the relationship between SCFAs and blood pressure, FMT could be a new treatment for hypertension.
Conclusion
As dietary habits and the living environment change, the prevalence of hypertension increases. Gut microbiota has been gaining considerable attention. SCFAs, which are gut microbiota metabolites, can be involved in hypertension occurrence and development through various pathways, such as specific receptors, the immune system and the autonomic nervous system. However, most of the studies on SCFAs in hypertension are phenotypic descriptions, and their specific molecular mechanisms remain unclear. Considering the differences in intestinal and body functions between rodents and humans, the research findings, which are mostly from rodents, cannot always be extrapolated to humans. Additional studies are needed to clarify the potential effects and internal mechanisms of SCFAs on hypertension, which could provide basic information for the novel prevention and treatment methods of hypertension.
Author Contributions
XT and ZG: conceptualisation. YW: writing—original draft preparation. YW, HX, XT, and ZG: writing—review and editing. ZG: supervision. All authors have read and agreed to the published version of the manuscript.
Funding
This work was funded by Clinical Research Fund Project of Zhejiang Medical Association (2021ZYC-A46) to XMT; Guiding Scientific and Technological Project of Quzhou (2021007) to ZG.
Conflict of Interest
The authors declare that the research was conducted in the absence of any commercial or financial relationships that could be construed as a potential conflict of interest.
Publisher’s Note
All claims expressed in this article are solely those of the authors and do not necessarily represent those of their affiliated organizations, or those of the publisher, the editors and the reviewers. Any product that may be evaluated in this article, or claim that may be made by its manufacturer, is not guaranteed or endorsed by the publisher.
Acknowledgments
We would like to thank Servier Medical Art (https://smart.servier.com) for providing the professionally designed medical elements to the manuscript.
References
Aguilar, E. C., da Silva, J. F., Navia-Pelaez, J. M., Leonel, A. J., Lopes, L. G., Menezes-Garcia, Z., et al. (2018). Sodium butyrate modulates adipocyte expansion, adipogenesis, and insulin receptor signaling by upregulation of PPAR–gamma in obese Apo E knockout mice. Nutrition 47, 75–82. doi: 10.1016/j.nut.2017.10.007
Allen, J. M., Mailing, L. J., Niemiro, G. M., Moore, R., Cook, M. D., White, B. A., et al. (2018). Exercise alters gut microbiota composition and function in lean and obese humans. Med. Sci. Sports Exerc. 50, 747–757. doi: 10.1249/MSS.0000000000001495
Almeida, C., Barata, P., and Fernandes, R. (2021). The influence of gut microbiota in cardiovascular diseases–a brief review. Porto Biomed. J. 6:e106. doi: 10.1097/j.pbj.0000000000000106
Annoni, E. M., Van Helden, D., Guo, Y., Levac, B., Libbus, I., KenKnight, B. H., et al. (2019). Chronic low–level vagus nerve stimulation improves long–term survival in salt–sensitive hypertensive rats. Front. Physiol. 10:25. doi: 10.3389/fphys.2019.00025
Aoki, R., Kamikado, K., Suda, W., Takii, H., Mikami, Y., Suganuma, N., et al. (2017). A proliferative probiotic Bifidobacterium strain in the gut ameliorates progression of metabolic disorders via microbiota modulation and acetate elevation. Sci. Rep. 7:43522. doi: 10.1038/srep43522
Barrea, L., Muscogiuri, G., Annunziata, G., Laudisio, D., Pugliese, G., Salzano, C., et al. (2019). From gut microbiota dysfunction to obesity: could short–chain fatty acids stop this dangerous course? Hormones (Athens) 18, 245–250. doi: 10.1007/s42000-019-00100-0
Bartolomaeus, H., Balogh, A., Yakoub, M., Homann, S., Marko, L., Hoges, S., et al. (2019). Short–chain fatty acid propionate protects from hypertensive cardiovascular damage. Circulation 139, 1407–1421. doi: 10.1161/CIRCULATIONAHA.118.036652
Bechtel, W., McGoohan, S., Zeisberg, E. M., Muller, G. A., Kalbacher, H., Salant, D. J., et al. (2010). Methylation determines fibroblast activation and fibrogenesis in the kidney. Nat. Med. 16, 544–550. doi: 10.1038/nm.2135
Bier, A., Braun, T., Khasbab, R., Di Segni, A., Grossman, E., Haberman, Y., et al. (2018). A high salt diet modulates the gut microbiota and short chain fatty acids production in a salt–sensitive hypertension rat model. Nutrients 10:1154. doi: 10.3390/nu10091154
Bolognini, D., Tobin, A. B., Milligan, G., and Moss, C. E. (2016). The pharmacology and function of receptors for short–chain fatty acids. Mol. Pharmacol. 89, 388–398. doi: 10.1124/mol.115.102301
Brubaker, P. L. (2018). Glucagon–like peptide–2 and the regulation of intestinal growth and function. Compr. Physiol. 8, 1185–1210. doi: 10.1002/cphy.c170055
Cani, P. D. (2017). Gut cell metabolism shapes the microbiome. Science 357, 548–549. doi: 10.1126/science.aao2202
Cardinale, J. P., Sriramula, S., Pariaut, R., Guggilam, A., Mariappan, N., Elks, C. M., et al. (2010). HDAC inhibition attenuates inflammatory, hypertrophic, and hypertensive responses in spontaneously hypertensive rats. Hypertension 56, 437–444. doi: 10.1161/HYPERTENSIONAHA.110.154567
Chakraborty, S., Galla, S., Cheng, X., Yeo, J. Y., Mell, B., Singh, V., et al. (2018). Salt–responsive metabolite, beta–hydroxybutyrate, attenuates hypertension. Cell Rep 25, 677.e674–689.e674. doi: 10.1016/j.celrep.2018.09.058
Chambers, E. S., Byrne, C. S., Aspey, K., Chen, Y., Khan, S., Morrison, D. J., et al. (2018). Acute oral sodium propionate supplementation raises resting energy expenditure and lipid oxidation in fasted humans. Diabetes Obes. Metab. 20, 1034–1039. doi: 10.1111/dom.13159
Chambers, E. S., Morrison, D. J., and Frost, G. (2015a). Control of appetite and energy intake by SCFA: what are the potential underlying mechanisms? Proc. Nutr. Soc. 74, 328–336. doi: 10.1017/S0029665114001657
Chambers, E. S., Viardot, A., Psichas, A., Morrison, D. J., Murphy, K. G., Zac-Varghese, S. E., et al. (2015b). Effects of targeted delivery of propionate to the human colon on appetite regulation, body weight maintenance and adiposity in overweight adults. Gut 64, 1744–1754. doi: 10.1136/gutjnl-2014-307913
Chang, Y., Deng, Q., Zhang, Z., Zhao, H., Tang, J., Chen, X., et al. (2021). Glucagon–like peptide 2 attenuates intestinal mucosal barrier injury through the MLCK/pMLC signaling pathway in a piglet model. J. Cell. Physiol. 236, 3015–3032. doi: 10.1002/jcp.30068
Chang, P. V., Hao, L., Offermanns, S., and Medzhitov, R. (2014). The microbial metabolite butyrate regulates intestinal macrophage function via histone deacetylase inhibition. Proc. Natl. Acad. Sci. U. S. A. 111, 2247–2252. doi: 10.1073/pnas.1322269111
Chen, X. F., Chen, X., and Tang, X. (2020). Short–chain fatty acid, acylation and cardiovascular diseases. Clin. Sci. 134, 657–676. doi: 10.1042/CS20200128
Chun, P. (2020). Therapeutic effects of histone deacetylase inhibitors on heart disease. Arch. Pharm. Res. 43, 1276–1296. doi: 10.1007/s12272-020-01297-0
Costello, S. P., Hughes, P. A., Waters, O., Bryant, R. V., Vincent, A. D., Blatchford, P., et al. (2019). Effect of fecal microbiota transplantation on 8–week remission in patients with ulcerative colitis: A randomized clinical trial. JAMA 321, 156–164. doi: 10.1001/jama.2018.20046
Dalile, B., Van Oudenhove, L., Vervliet, B., and Verbeke, K. (2019). The role of short–chain fatty acids in microbiota–gut–brain communication. Nat. Rev. Gastroenterol. Hepatol. 16, 461–478. doi: 10.1038/s41575-019-0157-3
de la Cuesta-Zuluaga, J., Mueller, N. T., Alvarez-Quintero, R., Velasquez-Mejia, E. P., Sierra, J. A., Corrales-Agudelo, V., et al. (2018). Higher fecal short–chain fatty acid levels are associated with gut microbiome dysbiosis, obesity, hypertension and cardiometabolic disease risk factors. Nutrients 11:51. doi: 10.3390/nu11010051
De Vadder, F., Kovatcheva-Datchary, P., Goncalves, D., Vinera, J., Zitoun, C., Duchampt, A., et al. (2014). Microbiota–generated metabolites promote metabolic benefits via gut–brain neural circuits. Cell 156, 84–96. doi: 10.1016/j.cell.2013.12.016
den Besten, G., Bleeker, A., Gerding, A., van Eunen, K., Havinga, R., van Dijk, T. H., et al. (2015). Short–chain fatty acids protect against high–fat diet–induced obesity via a PPARgamma–dependent switch from lipogenesis to fat oxidation. Diabetes 64, 2398–2408. doi: 10.2337/db14-1213
den Besten, G., Lange, K., Havinga, R., van Dijk, T. H., Gerding, A., van Eunen, K., et al. (2013). Gut–derived short–chain fatty acids are vividly assimilated into host carbohydrates and lipids. Am. J. Physiol. Gastrointest. Liver Physiol. 305, G900–G910. doi: 10.1152/ajpgi.00265.2013
Dong, J. Y., Szeto, I. M., Makinen, K., Gao, Q., Wang, J., Qin, L. Q., et al. (2013). Effect of probiotic fermented milk on blood pressure: a meta–analysis of randomised controlled trials. Br. J. Nutr. 110, 1188–1194. doi: 10.1017/S0007114513001712
D'Souza, W. N., Douangpanya, J., Mu, S., Jaeckel, P., Zhang, M., Maxwell, J. R., et al. (2017). Differing roles for short chain fatty acids and GPR43 agonism in the regulation of intestinal barrier function and immune responses. PLoS One 12:e0180190. doi: 10.1371/journal.pone.0181594
Ehret, G. B., Ferreira, T., Chasman, D. I., Jackson, A. U., Schmidt, E. M., Johnson, T., et al. (2016). The genetics of blood pressure regulation and its target organs from association studies in 342, 415 individuals. Nat. Genet. 48, 1171–1184. doi: 10.1038/ng.3667
Fenneman, A. C., Rampanelli, E., Yin, Y. S., Ames, J., Blaser, M. J., Fliers, E., et al. (2020). Gut microbiota and metabolites in the pathogenesis of endocrine disease. Biochem. Soc. Trans. 48, 915–931. doi: 10.1042/BST20190686
Forouzanfar, M. H., Liu, P., Roth, G. A., Ng, M., Biryukov, S., Marczak, L., et al. (2017). Global burden of hypertension and systolic blood pressure of at least 110 to 115 mm hg, 1990–2015. JAMA 317, 165–182. doi: 10.1001/jama.2016.19043
Frampton, J., Murphy, K. G., Frost, G., and Chambers, E. S. (2020). Short–chain fatty acids as potential regulators of skeletal muscle metabolism and function. Nat. Metab. 2, 840–848. doi: 10.1038/s42255-020-0188-7
Freire, R. H., and Alvarez-Leite, J. I. (2020). Appetite control: hormones or diet strategies? Curr. Opin. Clin. Nutr. Metab. Care 23, 328–335. doi: 10.1097/MCO.0000000000000675
Frost, G., Sleeth, M. L., Sahuri-Arisoylu, M., Lizarbe, B., Cerdan, S., Brody, L., et al. (2014). The short–chain fatty acid acetate reduces appetite via a central homeostatic mechanism. Nat. Commun. 5:3611. doi: 10.1038/ncomms4611
Furie, K. (2020). Epidemiology and primary prevention of stroke. Continuum 26, 260–267. doi: 10.1212/CON.0000000000000831
Furusawa, Y., Obata, Y., Fukuda, S., Endo, T. A., Nakato, G., Takahashi, D., et al. (2013). Commensal microbe–derived butyrate induces the differentiation of colonic regulatory T cells. Nature 504, 446–450. doi: 10.1038/nature12721
Ge, Y., Ahmed, S., Yao, W., You, L., Zheng, J., and Hileuskaya, K. (2021). Regulation effects of indigestible dietary polysaccharides on intestinal microflora: An overview. J. Food Biochem. 45:e13564. doi: 10.1111/jfbc.13564
Goswami, C., Iwasaki, Y., and Yada, T. (2018). Short–chain fatty acids suppress food intake by activating vagal afferent neurons. J. Nutr. Biochem. 57, 130–135. doi: 10.1016/j.jnutbio.2018.03.009
Guan, Y., Chen, K., Quan, D., Kang, L., Yang, D., Wu, H., et al. (2020). The combination of Scutellaria baicalensis Georgi and Sophora japonica L. ameliorate renal function by regulating gut microbiota in spontaneously hypertensive rats. Front. Pharmacol. 11:575294. doi: 10.3389/fphar.2020.575294
Han, Y. M., Bedarida, T., Ding, Y., Somba, B. K., Lu, Q., Wang, Q., et al. (2018). Beta–hydroxybutyrate prevents vascular senescence through hnRNP A1–mediated upregulation of Oct4. Mol. Cell 71, 1064.e1065–1078.e1065. doi: 10.1016/j.molcel.2018.07.036
Hartstra, A. V., Bouter, K. E., Backhed, F., and Nieuwdorp, M. (2015). Insights into the role of the microbiome in obesity and type 2 diabetes. Diabetes Care 38, 159–165. doi: 10.2337/dc14-0769
He, J., Zhang, P., Shen, L., Niu, L., Tan, Y., Chen, L., et al. (2020). Short–chain fatty acids and their association with signalling pathways in inflammation, glucose and lipid metabolism. Int. J. Mol. Sci. 21:6356. doi: 10.3390/ijms21176356
Ho, L. C., Sung, J. M., Shen, Y. T., Jheng, H. F., Chen, S. H., Tsai, P. J., et al. (2016). Egr–1 deficiency protects from renal inflammation and fibrosis. J. Mol. Med. (Berl) 94, 933–942. doi: 10.1007/s00109-016-1403-6
Holmes, E., Loo, R. L., Stamler, J., Bictash, M., Yap, I. K., Chan, Q., et al. (2008). Human metabolic phenotype diversity and its association with diet and blood pressure. Nature 453, 396–400. doi: 10.1038/nature06882
Hsu, C. N., Lin, Y. J., Hou, C. Y., and Tain, Y. L. (2018). Maternal administration of probiotic or prebiotic prevents male adult rat offspring against developmental programming of hypertension induced by high fructose consumption in pregnancy and lactation. Nutrients 10:1229. doi: 10.3390/nu1009122
Hu, J., Lin, S., Zheng, B., and Cheung, P. C. K. (2018). Short–chain fatty acids in control of energy metabolism. Crit. Rev. Food Sci. Nutr. 58, 1243–1249. doi: 10.1080/10408398.2016.1245650
Ivan, J., Major, E., Sipos, A., Kovacs, K., Horvath, D., Tamas, I., et al. (2017). The short–chain fatty acid propionate inhibits adipogenic differentiation of human chorion–derived mesenchymal stem cells through the free fatty acid receptor 2. Stem Cells Dev. 26, 1724–1733. doi: 10.1089/scd.2017.0035
Jadoon, A., Mathew, A. V., Byun, J., Gadegbeku, C. A., Gipson, D. S., Afshinnia, F., et al. (2018). Gut microbial product predicts cardiovascular risk in chronic kidney disease patients. Am. J. Nephrol. 48, 269–277. doi: 10.1159/000493862
Jama, H. A., Beale, A., Shihata, W. A., and Marques, F. Z. (2019). The effect of diet on hypertensive pathology: is there a link via gut microbiota–driven immunometabolism? Cardiovasc. Res. 115, 1435–1447. doi: 10.1093/cvr/cvz091
Jiao, A. R., Diao, H., Yu, B., He, J., Yu, J., Zheng, P., et al. (2018). Oral administration of short chain fatty acids could attenuate fat deposition of pigs. PLoS One 13:e0196867. doi: 10.1371/journal.pone.0196867
Jiao, A., Yu, B., He, J., Yu, J., Zheng, P., Luo, Y., et al. (2020). Short chain fatty acids could prevent fat deposition in pigs via regulating related hormones and genes. Food Funct. 11, 1845–1855. doi: 10.1039/C9FO02585E
Jin, M., Qian, Z., Yin, J., Xu, W., and Zhou, X. (2019). The role of intestinal microbiota in cardiovascular disease. J. Cell. Mol. Med. 23, 2343–2350. doi: 10.1111/jcmm.14195
Kaess, B. M., Rong, J., Larson, M. G., Hamburg, N. M., Vita, J. A., Levy, D., et al. (2012). Aortic stiffness, blood pressure progression, and incident hypertension. JAMA 308, 875–881. doi: 10.1001/2012.jama.10503
Kalla, M., Herring, N., and Paterson, D. J. (2016). Cardiac sympatho–vagal balance and ventricular arrhythmia. Auton. Neurosci. 199, 29–37. doi: 10.1016/j.autneu.2016.08.016
Katsimardou, A., Imprialos, K., Stavropoulos, K., Sachinidis, A., Doumas, M., and Athyros, V. (2020). Hypertension in metabolic syndrome: novel insights. Curr. Hypertens. Rev. 16, 12–18. doi: 10.2174/1573402115666190415161813
Keating, S. E., Coombes, J. S., Stowasser, M., and Bailey, T. G. (2020). The role of exercise in patients with obesity and hypertension. Curr. Hypertens. Rep. 22:77. doi: 10.1007/s11906-020-01087-5
Kim, S., Goel, R., Kumar, A., Qi, Y., Lobaton, G., Hosaka, K., et al. (2018). Imbalance of gut microbiome and intestinal epithelial barrier dysfunction in patients with high blood pressure. Clin. Sci. 132, 701–718. doi: 10.1042/CS20180087
Kim, M. H., Kang, S. G., Park, J. H., Yanagisawa, M., and Kim, C. H. (2013). Short–chain fatty acids activate GPR41 and GPR43 on intestinal epithelial cells to promote inflammatory responses in mice. Gastroenterology 145, 396.e391–406.e391. doi: 10.1053/j.gastro.2013.04.056
Kimura, I., Ichimura, A., Ohue-Kitano, R., and Igarashi, M. (2020). Free fatty acid receptors in health and disease. Physiol. Rev. 100, 171–210. doi: 10.1152/physrev.00041.2018
Kimura, I., Inoue, D., Maeda, T., Hara, T., Ichimura, A., Miyauchi, S., et al. (2011). Short–chain fatty acids and ketones directly regulate sympathetic nervous system via G protein–coupled receptor 41 (GPR41). Proc. Natl. Acad. Sci. U. S. A. 108, 8030–8035. doi: 10.1073/pnas.1016088108
Kimura, I., Ozawa, K., Inoue, D., Imamura, T., Kimura, K., Maeda, T., et al. (2013). The gut microbiota suppresses insulin–mediated fat accumulation via the short–chain fatty acid receptor GPR43. Nat. Commun. 4:1829. doi: 10.1038/ncomms2852
Kotlo, K., Anbazhagan, A. N., Priyamvada, S., Jayawardena, D., Kumar, A., Chen, Y., et al. (2020). The olfactory G protein–coupled receptor (Olfr–78/OR51E2) modulates the intestinal response to colitis. Am. J. Physiol. Cell Physiol. 318, C502–C513. doi: 10.1152/ajpcell.00454.2019
Kumar, P., Gogulamudi, V. R., Periasamy, R., Raghavaraju, G., Subramanian, U., and Pandey, K. N. (2017). Inhibition of HDAC enhances STAT acetylation, blocks NF–kappaB, and suppresses the renal inflammation and fibrosis in Npr1 haplotype male mice. Am. J. Physiol. Renal Physiol. 313, F781–F795. doi: 10.1152/ajprenal.00166.2017
Kumar, J., Rani, K., and Datt, C. (2020). Molecular link between dietary fibre, gut microbiota and health. Mol. Biol. Rep. 47, 6229–6237. doi: 10.1007/s11033-020-05611-3
Lal, S., Kirkup, A. J., Brunsden, A. M., Thompson, D. G., and Grundy, D. (2001). Vagal afferent responses to fatty acids of different chain length in the rat. Am. J. Physiol. Gastrointest. Liver Physiol. 281, G907–G915. doi: 10.1152/ajpgi.2001.281.4.G907
Li, P., Ge, J., and Li, H. (2020). Lysine acetyltransferases and lysine deacetylases as targets for cardiovascular disease. Nat. Rev. Cardiol. 17, 96–115. doi: 10.1038/s41569-019-0235-9
Li, R., Mi, X., Yang, S., Yang, Y., Zhang, S., Hui, R., et al. (2019). Long–term stimulation of angiotensin II induced endothelial senescence and dysfunction. Exp. Gerontol. 119, 212–220. doi: 10.1016/j.exger.2019.02.012
Li, M., van Esch, B., Henricks, P. A. J., Folkerts, G., and Garssen, J. (2018a). The anti–inflammatory effects of short chain fatty acids on lipopolysaccharide– or tumor necrosis factor alpha–stimulated endothelial cells via activation of GPR41/43 and inhibition of HDACs. Front. Pharmacol. 9:533. doi: 10.3389/fphar.2018.00533
Li, M., van Esch, B., Wagenaar, G. T. M., Garssen, J., Folkerts, G., and Henricks, P. A. J. (2018b). Pro– and anti–inflammatory effects of short chain fatty acids on immune and endothelial cells. Eur. J. Pharmacol. 831, 52–59. doi: 10.1016/j.ejphar.2018.05.003
Li, Z., Yi, C. X., Katiraei, S., Kooijman, S., Zhou, E., Chung, C. K., et al. (2018c). Butyrate reduces appetite and activates brown adipose tissue via the gut–brain neural circuit. Gut 67, 1269–1279. doi: 10.1136/gutjnl-2017-314050
Li, J., Zhao, F., Wang, Y., Chen, J., Tao, J., Tian, G., et al. (2017). Gut microbiota dysbiosis contributes to the development of hypertension. Microbiome 5:14. doi: 10.1186/s40168-016-0222-x
Litwin, M., and Kulaga, Z. (2021). Obesity, metabolic syndrome, and primary hypertension. Pediatr. Nephrol. 36, 825–837. doi: 10.1007/s00467-020-04579-3
Liu, K., Li, F., Sun, Q., Lin, N., Han, H., You, K., et al. (2019). p53 beta–hydroxybutyrylation attenuates p53 activity. Cell Death Dis. 10:243. doi: 10.1038/s41419-019-1463-y
Liu, P., Wang, Y., Yang, G., Zhang, Q., Meng, L., Xin, Y., et al. (2021). The role of short–chain fatty acids in intestinal barrier function, inflammation, oxidative stress, and colonic carcinogenesis. Pharmacol. Res. 165:105420. doi: 10.1016/j.phrs.2021.105420
Louis, P., and Flint, H. J. (2017). Formation of propionate and butyrate by the human colonic microbiota. Environ. Microbiol. 19, 29–41. doi: 10.1038/s41419-019-1463-y
Machate, D. J., Figueiredo, P. S., Marcelino, G., Guimaraes, R. C. A., Hiane, P. A., Bogo, D., et al. (2020). Fatty acid diets: regulation of gut microbiota composition and obesity and its related metabolic dysbiosis. Int. J. Mol. Sci. 21:4093. doi: 10.3390/ijms21114093
Mancia, G., and Grassi, G. (2014). The autonomic nervous system and hypertension. Circ. Res. 114, 1804–1814. doi: 10.1161/CIRCRESAHA.114.302524
Mandaliya, D. K., and Seshadri, S. (2019). Short chain fatty acids, pancreatic dysfunction and type 2 diabetes. Pancreatology 19, 280–284. doi: 10.1016/j.pan.2019.01.021
Markowiak-Kopec, P., and Slizewska, K. (2020). The effect of probiotics on the production of short–chain fatty acids by human intestinal microbiome. Nutrients 12:1107. doi: 10.3390/nu12041107
Marques, F. Z., Nelson, E., Chu, P. Y., Horlock, D., Fiedler, A., Ziemann, M., et al. (2017). High–fiber diet and acetate supplementation change the gut microbiota and prevent the development of hypertension and heart failure in hypertensive mice. Circulation 135, 964–977. doi: 10.1161/CIRCULATIONAHA.116.024545
Men, H., Cai, H., Cheng, Q., Zhou, W., Wang, X., Huang, S., et al. (2021). The regulatory roles of p53 in cardiovascular health and disease. Cell. Mol. Life Sci. 78, 2001–2018. doi: 10.1007/s00018-020-03694-6
Mendelsohn, A. R., and Larrick, J. W. (2018). Prevention of senescence in vasculature through quiescence. Rejuvenation Res. 21, 477–481. doi: 10.1089/rej.2018.2138
Mills, K. T., Bundy, J. D., Kelly, T. N., Reed, J. E., Kearney, P. M., Reynolds, K., et al. (2016). Global disparities of hypertension prevalence and control: A systematic analysis of population–based studies from 90 countries. Circulation 134, 441–450. doi: 10.1161/CIRCULATIONAHA.115.018912
Mills, K. T., Stefanescu, A., and He, J. (2020). The global epidemiology of hypertension. Nat. Rev. Nephrol. 16, 223–237. doi: 10.1038/s41581-019-0244-2
Miyamoto, J., Kasubuchi, M., Nakajima, A., Irie, J., Itoh, H., and Kimura, I. (2016). The role of short–chain fatty acid on blood pressure regulation. Curr. Opin. Nephrol. Hypertens. 25, 379–383. doi: 10.1097/MNH.0000000000000246
Miyamoto, S., Shikata, K., Miyasaka, K., Okada, S., Sasaki, M., Kodera, R., et al. (2012). Cholecystokinin plays a novel protective role in diabetic kidney through anti–inflammatory actions on macrophage: anti–inflammatory effect of cholecystokinin. Diabetes 61, 897–907. doi: 10.2337/db11-0402
Mohr, A. E., Jager, R., Carpenter, K. C., Kerksick, C. M., Purpura, M., Townsend, J. R., et al. (2020). The athletic gut microbiota. J. Int. Soc. Sports Nutr. 17:24. doi: 10.1186/s12970-020-00353-w
Monda, V., Villano, I., Messina, A., Valenzano, A., Esposito, T., Moscatelli, F., et al. (2017). Exercise modifies the gut microbiota with positive health effects. Oxidative Med. Cell. Longev. 2017, 3831972. doi: 10.1155/2017/3831972
Moreira, T. S., Antunes, V. R., Falquetto, B., and Marina, N. (2018). Long–term stimulation of cardiac vagal preganglionic neurons reduces blood pressure in the spontaneously hypertensive rat. J. Hypertens. 36, 2444–2452. doi: 10.1097/HJH.0000000000001871
Muralitharan, R. R., Jama, H. A., Xie, L., Peh, A., Snelson, M., and Marques, F. Z. (2020). Microbial peer pressure: The role of the gut microbiota in hypertension and its complications. Hypertension 76, 1674–1687. doi: 10.1161/HYPERTENSIONAHA.120.14473
Muralitharan, R. R., and Marques, F. Z. (2021). Diet–related gut microbial metabolites and sensing in hypertension. J. Hum. Hypertens. 35, 162–169. doi: 10.1038/s41371-020-0388-3
Myint, H., Kishi, H., Iwahashi, Y., Saburi, W., Koike, S., and Kobayashi, Y. (2018). Functional modulation of caecal fermentation and microbiota in rat by feeding bean husk as a dietary fibre supplement. Benef Microbes 9, 963–974. doi: 10.3920/BM2017.0174
Nakagawa, K., Holla, V. R., Wei, Y., Wang, W. H., Gatica, A., Wei, S., et al. (2006). Salt–sensitive hypertension is associated with dysfunctional Cyp4a10 gene and kidney epithelial sodium channel. J. Clin. Invest. 116, 1696–1702. doi: 10.1172/JCI27546
Nakamura, Y., Yamamoto, N., Sakai, K., and Takano, T. (1995). Antihypertensive effect of sour milk and peptides isolated from it that are inhibitors to angiotensin I–converting enzyme. J. Dairy Sci. 78, 1253–1257. doi: 10.3168/jds.S0022-0302(95)76745-5
Naqvi, S., Asar, T. O., Kumar, V., Al-Abbasi, F. A., Alhayyani, S., Kamal, M. A., et al. (2021). A cross–talk between gut microbiome, salt and hypertension. Biomed. Pharmacother. 134:111156. doi: 10.1016/j.biopha.2020.111156
Natarajan, N., Hori, D., Flavahan, S., Steppan, J., Flavahan, N. A., Berkowitz, D. E., et al. (2016). Microbial short chain fatty acid metabolites lower blood pressure via endothelial G protein–coupled receptor 41. Physiol. Genomics 48, 826–834. doi: 10.1152/physiolgenomics.00089.2016
Natarajan, N., and Pluznick, J. L. (2014). From microbe to man: the role of microbial short chain fatty acid metabolites in host cell biology. Am. J. Physiol. Cell Physiol. 307, C979–C985. doi: 10.1152/ajpcell.00228.2014
Newman, J. C., and Verdin, E. (2014). Ketone bodies as signaling metabolites. Trends Endocrinol. Metab. 25, 42–52. doi: 10.1016/j.tem.2013.09.002
Nicholson, J. K., Holmes, E., Kinross, J., Burcelin, R., Gibson, G., Jia, W., et al. (2012). Host–gut microbiota metabolic interactions. Science 336, 1262–1267. doi: 10.1126/science.1223813
Nishida, A., Miyamoto, J., Shimizu, H., and Kimura, I. (2021). Gut microbial short–chain fatty acids–mediated olfactory receptor 78 stimulation promotes anorexigenic gut hormone peptide YY secretion in mice. Biochem. Biophys. Res. Commun. 557, 48–54. doi: 10.1016/j.bbrc.2021.03.167
Nohr, M. K., Egerod, K. L., Christiansen, S. H., Gille, A., Offermanns, S., Schwartz, T. W., et al. (2015). Expression of the short chain fatty acid receptor GPR41/FFAR3 in autonomic and somatic sensory ganglia. Neuroscience 290, 126–137. doi: 10.1016/j.neuroscience.2015.01.040
Ohira, H., Fujioka, Y., Katagiri, C., Mamoto, R., Aoyama-Ishikawa, M., Amako, K., et al. (2013). Butyrate attenuates inflammation and lipolysis generated by the interaction of adipocytes and macrophages. J. Atheroscler. Thromb. 20, 425–442. doi: 10.5551/jat.15065
Ohira, H., Tsutsui, W., Mamoto, R., Yamaguchi, S., Nishida, M., Ito, M., et al. (2016). Butyrate attenuates lipolysis in adipocytes co–cultured with macrophages through non–prostaglandin E2–mediated and prostaglandin E2–mediated pathways. Lipids Health Dis. 15:213. doi: 10.1186/s12944-016-0387-0
Onal, E. M., Afsar, B., Covic, A., Vaziri, N. D., and Kanbay, M. (2019). Gut microbiota and inflammation in chronic kidney disease and their roles in the development of cardiovascular disease. Hypertens. Res. 42, 123–140. doi: 10.1038/s41440-018-0144-z
Onyszkiewicz, M., Gawrys-Kopczynska, M., Konopelski, P., Aleksandrowicz, M., Sawicka, A., Kozniewska, E., et al. (2019). Butyric acid, a gut bacteria metabolite, lowers arterial blood pressure via colon–vagus nerve signaling and GPR41/43 receptors. Pflugers Arch. 471, 1441–1453. doi: 10.1007/s00424-019-02322-y
Parada Venegas, D., De la Fuente, M. K., Landskron, G., Gonzalez, M. J., Quera, R., Dijkstra, G., et al. (2019). Short chain fatty acids (SCFAs)–mediated gut epithelial and immune regulation and its relevance for inflammatory bowel diseases. Front. Immunol. 10:277. doi: 10.3389/fimmu.2019.00277
Pluznick, J. L. (2013). Renal and cardiovascular sensory receptors and blood pressure regulation. Am. J. Physiol. Renal Physiol. 305, F439–F444. doi: 10.1152/ajprenal.00252.2013
Pluznick, J. (2014). A novel SCFA receptor, the microbiota, and blood pressure regulation. Gut Microbes 5, 202–207. doi: 10.4161/gmic.27492
Pluznick, J. L. (2017). Microbial short–chain fatty acids and blood pressure regulation. Curr. Hypertens. Rep. 19:25. doi: 10.1007/s11906-017-0722-5
Pluznick, J. L., Protzko, R. J., Gevorgyan, H., Peterlin, Z., Sipos, A., Han, J., et al. (2013). Olfactory receptor responding to gut microbiota–derived signals plays a role in renin secretion and blood pressure regulation. Proc. Natl. Acad. Sci. U. S. A. 110, 4410–4415. doi: 10.1073/pnas.1215927110
Poll, B. G., Cheema, M. U., and Pluznick, J. L. (2020). Gut microbial metabolites and blood pressure regulation: focus on SCFAs and TMAO. Physiology 35, 275–284. doi: 10.1152/physiol.00004.2020
Priyadarshini, M., Kotlo, K. U., Dudeja, P. K., and Layden, B. T. (2018). Role of short chain fatty acid receptors in intestinal physiology and pathophysiology. Compr. Physiol. 8, 1091–1115. doi: 10.1002/cphy.c170050
Qi, Y., Aranda, J. M., Rodriguez, V., Raizada, M. K., and Pepine, C. J. (2015). Impact of antibiotics on arterial blood pressure in a patient with resistant hypertension – A case report. Int. J. Cardiol. 201, 157–158. doi: 10.1016/j.ijcard.2015.07.078
Qi, D., Nie, X. L., and Zhang, J. J. (2020). The effect of probiotics supplementation on blood pressure: a systemic review and meta–analysis. Lipids Health Dis. 19:79. doi: 10.1186/s12944-020-01259-x
Ratajczak, W., Ryl, A., Mizerski, A., Walczakiewicz, K., Sipak, O., and Laszczynska, M. (2019). Immunomodulatory potential of gut microbiome–derived short–chain fatty acids (SCFAs). Acta Biochim. Pol. 66, 1–12. doi: 10.18388/abp.2018_2648
Ren, J., and Crowley, S. D. (2019). Role of T–cell activation in salt–sensitive hypertension. Am. J. Physiol. Heart Circ. Physiol. 316, H1345–H1353. doi: 10.1152/ajpheart.00096.2019
Ren, M., Zhang, H., Qi, J., Hu, A., Jiang, Q., Hou, Y., et al. (2020). An almond–based low carbohydrate diet improves depression and glycometabolism in patients with type 2 diabetes through modulating gut microbiota and GLP–1: A randomized controlled trial. Nutrients 12:3036. doi: 10.3390/nu12103036
Robles-Vera, I., de la Visitacion, N., Toral, M., Sanchez, M., Romero, M., Gomez-Guzman, M., et al. (2020). Probiotic Bifidobacterium breve prevents DOCA–salt hypertension. FASEB J. 34, 13626–13640. doi: 10.1096/fj.202001532R
Rossier, B. C., Bochud, M., and Devuyst, O. (2017). The hypertension pandemic: An evolutionary perspective. Physiology (Bethesda) 32, 112–125. doi: 10.1152/physiol.00026.2016
Saco-Ledo, G., Valenzuela, P. L., Ruiz-Hurtado, G., Ruilope, L. M., and Lucia, A. (2020). Exercise reduces ambulatory blood pressure in patients with hypertension: A systematic review and meta–analysis of randomized controlled trials. J. Am. Heart Assoc. 9:e018487. doi: 10.1161/JAHA.120.018487
Samuel, B. S., Shaito, A., Motoike, T., Rey, F. E., Backhed, F., Manchester, J. K., et al. (2008). Effects of the gut microbiota on host adiposity are modulated by the short–chain fatty–acid binding G protein–coupled receptor, Gpr41. Proc. Natl. Acad. Sci. U. S. A. 105, 16767–16772. doi: 10.1073/pnas.0808567105
Sasaki, K., Sasaki, D., Hannya, A., Tsubota, J., and Kondo, A. (2020). In vitro human colonic microbiota utilises D–beta–hydroxybutyrate to increase butyrogenesis. Sci. Rep. 10:8516. doi: 10.1038/s41598-020-65561-5
Schwiertz, A., Taras, D., Schafer, K., Beijer, S., Bos, N. A., Donus, C., et al. (2010). Microbiota and SCFA in lean and overweight healthy subjects. Obesity 18, 190–195. doi: 10.1038/oby.2009.167
Seekatz, A. M., Theriot, C. M., Rao, K., Chang, Y. M., Freeman, A. E., Kao, J. Y., et al. (2018). Restoration of short chain fatty acid and bile acid metabolism following fecal microbiota transplantation in patients with recurrent clostridium difficile infection. Anaerobe 53, 64–73. doi: 10.1016/j.anaerobe.2018.04.001
Segers, A., Desmet, L., Thijs, T., Verbeke, K., Tack, J., and Depoortere, I. (2019). The circadian clock regulates the diurnal levels of microbial short–chain fatty acids and their rhythmic effects on colon contractility in mice. Acta Physiol (Oxf.) 225:e13193. doi: 10.1111/apha.13193
Shimazu, T., Hirschey, M. D., Newman, J., He, W., Shirakawa, K., Le Moan, N., et al. (2013). Suppression of oxidative stress by beta–hydroxybutyrate, an endogenous histone deacetylase inhibitor. Science 339, 211–214. doi: 10.1126/science.1227166
Singh, N., Gurav, A., Sivaprakasam, S., Brady, E., Padia, R., Shi, H., et al. (2014). Activation of Gpr109a, receptor for niacin and the commensal metabolite butyrate, suppresses colonic inflammation and carcinogenesis. Immunity 40, 128–139. doi: 10.1016/j.immuni.2013.12.007
Streppel, M. T., Arends, L. R., van’t Veer, P., Grobbee, D. E., and Geleijnse, J. M. (2005). Dietary fiber and blood pressure: a meta–analysis of randomized placebo–controlled trials. Arch. Intern. Med. 165, 150–156. doi: 10.1001/archinte.165.2.150
Suzuki, S., and Aoe, S. (2021). High beta–glucan barley supplementation improves glucose tolerance by increasing GLP–1 secretion in diet–induced obesity mice. Nutrients 13:527. doi: 10.3390/nu13020527
Thangaraju, M., Cresci, G. A., Liu, K., Ananth, S., Gnanaprakasam, J. P., Browning, D. D., et al. (2009). GPR109A is a G–protein–coupled receptor for the bacterial fermentation product butyrate and functions as a tumor suppressor in colon. Cancer Res. 69, 2826–2832. doi: 10.1158/0008-5472.CAN-08-4466
Thorburn, A. N., McKenzie, C. I., Shen, S., Stanley, D., Macia, L., Mason, L. J., et al. (2015). Evidence that asthma is a developmental origin disease influenced by maternal diet and bacterial metabolites. Nat. Commun. 6:7320. doi: 10.1038/ncomms8320
Tian, X. L., and Li, Y. (2014). Endothelial cell senescence and age–related vascular diseases. J. Genet. Genomics 41, 485–495. doi: 10.1016/j.jgg.2014.08.001
Velarde, G., and Berk, B. C. (2005). Role of hypertension in the metabolic syndrome: who is affected? Curr. Hypertens. Rep. 7, 418–426. doi: 10.1007/s11906-005-0036-x
Verhaar, B. J. H., Prodan, A., Nieuwdorp, M., and Muller, M. (2020). Gut microbiota in hypertension and atherosclerosis: A review. Nutrients 12:2982. doi: 10.3390/nu12102982
Vijay, N., and Morris, M. E. (2014). Role of monocarboxylate transporters in drug delivery to the brain. Curr. Pharm. Des. 20, 1487–1498. doi: 10.2174/13816128113199990462
Wang, N. P., Pang, X. F., Zhang, L. H., Tootle, S., Harmouche, S., and Zhao, Z. Q. (2014). Attenuation of inflammatory response and reduction in infarct size by postconditioning are associated with downregulation of early growth response 1 during reperfusion in rat heart. Shock 41, 346–354. doi: 10.1097/SHK.0000000000000112
Wang, S. Z., Yu, Y. J., and Adeli, K. (2020). Role of gut microbiota in neuroendocrine regulation of carbohydrate and lipid metabolism via the microbiota–gut–brain–liver axis. Microorganisms 8:527. doi: 10.3390/microorganisms8040527
Wang, L., Zhu, Q., Lu, A., Liu, X., Zhang, L., Xu, C., et al. (2017). Sodium butyrate suppresses angiotensin II–induced hypertension by inhibition of renal (pro)renin receptor and intrarenal renin–angiotensin system. J. Hypertens. 35, 1899–1908. doi: 10.1097/HJH.0000000000001378
Wegh, C. A. M., Geerlings, S. Y., Knol, J., Roeselers, G., and Belzer, C. (2019). Postbiotics and their potential applications in early life nutrition and beyond. Int. J. Mol. Sci. 20:4673. doi: 10.3390/ijms20194673
Weingarden, A. R., and Vaughn, B. P. (2017). Intestinal microbiota, fecal microbiota transplantation, and inflammatory bowel disease. Gut Microbes 8, 238–252. doi: 10.1080/19490976.2017.1290757
Wenzel, U., Turner, J. E., Krebs, C., Kurts, C., Harrison, D. G., and Ehmke, H. (2016). Immune mechanisms in arterial hypertension. J. Am. Soc. Nephrol. 27, 677–686. doi: 10.1681/ASN.2015050562
Wilson, K. A., Han, Y., Zhang, M., Hess, J. P., Chapman, K. A., Cline, G. W., et al. (2017). Inter–relations between 3–hydroxypropionate and propionate metabolism in rat liver: relevance to disorders of propionyl–CoA metabolism. Am. J. Physiol. Endocrinol. Metab. 313, E413–E428. doi: 10.1152/ajpendo.00105.2017
Wong, J. M., de Souza, R., Kendall, C. W., Emam, A., and Jenkins, D. J. (2006). Colonic health: fermentation and short chain fatty acids. J. Clin. Gastroenterol. 40, 235–243. doi: 10.1097/00004836-200603000-00015
Wu, J., Wang, K., Wang, X., Pang, Y., and Jiang, C. (2021). The role of the gut microbiome and its metabolites in metabolic diseases. Protein Cell 12, 360–373. doi: 10.1007/s13238-020-00814-7
Yang, T., Magee, K. L., Colon-Perez, L. M., Larkin, R., Liao, Y. S., Balazic, E., et al. (2019). Impaired butyrate absorption in the proximal colon, low serum butyrate and diminished central effects of butyrate on blood pressure in spontaneously hypertensive rats. Acta Physiol (Oxf.) 226:e13256. doi: 10.1111/apha.13256
Yang, T., Rodriguez, V., Malphurs, W. L., Schmidt, J. T., Ahmari, N., Sumners, C., et al. (2018). Butyrate regulates inflammatory cytokine expression without affecting oxidative respiration in primary astrocytes from spontaneously hypertensive rats. Physiol. Rep. 6:e13732. doi: 10.14814/phy2.13732
Yang, T., Santisteban, M. M., Rodriguez, V., Li, E., Ahmari, N., Carvajal, J. M., et al. (2015). Gut dysbiosis is linked to hypertension. Hypertension 65, 1331–1340. doi: 10.1161/HYPERTENSIONAHA.115.05315
Yang, W., Yu, T., Huang, X., Bilotta, A. J., Xu, L., Lu, Y., et al. (2020). Intestinal microbiota–derived short–chain fatty acids regulation of immune cell IL–22 production and gut immunity. Nat. Commun. 11:4457. doi: 10.1038/s41467-020-18262-6
Yao, Y., Yan, L., Chen, H., Wu, N., Wang, W., and Wang, D. (2020). Cyclocarya paliurus polysaccharides alleviate type 2 diabetic symptoms by modulating gut microbiota and short–chain fatty acids. Phytomedicine 77:153268. doi: 10.1016/j.phymed.2020.153268
Yoo, J. Y., Groer, M., Dutra, S. V. O., Sarkar, A., and McSkimming, D. I. (2020). Gut microbiota and immune system interactions. Microorganisms 8:1587. doi: 10.3390/microorganisms8101587
Zhai, X., Lin, D., Zhao, Y., Li, W., and Yang, X. (2018). Effects of dietary fiber supplementation on fatty acid metabolism and intestinal microbiota diversity in C57BL/6J mice fed with a high–fat diet. J. Agric. Food Chem. 66, 12706–12718. doi: 10.1021/acs.jafc.8b05036
Zhang, Z., Mocanu, V., Cai, C., Dang, J., Slater, L., Deehan, E. C., et al. (2019b). Impact of fecal microbiota transplantation on obesity and metabolic syndrome–A systematic review. Nutrients 11:2291. doi: 10.3390/nu11102291
Zhang, J. M., Sun, Y. S., Zhao, L. Q., Chen, T. T., Fan, M. N., Jiao, H. C., et al. (2019a). SCFAs–induced GLP–1 secretion links the regulation of gut microbiome on hepatic lipogenesis in chickens. Front. Microbiol. 10:2176. doi: 10.3389/fmicb.2019.02176
Zhao, L., Zhang, F., Ding, X., Wu, G., Lam, Y. Y., Wang, X., et al. (2018). Gut bacteria selectively promoted by dietary fibers alleviate type 2 diabetes. Science 359, 1151–1156. doi: 10.1126/science.aao5774
Zhernakova, A., Kurilshikov, A., Bonder, M. J., Tigchelaar, E. F., Schirmer, M., Vatanen, T., et al. (2016). Population–based metagenomics analysis reveals markers for gut microbiome composition and diversity. Science 352, 565–569. doi: 10.1126/science.aad3369
Zhi, C., Huang, J., Wang, J., Cao, H., Bai, Y., Guo, J., et al. (2019). Connection between gut microbiome and the development of obesity. Eur. J. Clin. Microbiol. Infect. Dis. 38, 1987–1998. doi: 10.1007/s10096-019-03623-x
Keywords: hypertension, blood pressure, short-chain fatty acids, gut microbiota, mechanism, treatment
Citation: Wu Y, Xu H, Tu X and Gao Z (2021) The Role of Short-Chain Fatty Acids of Gut Microbiota Origin in Hypertension. Front. Microbiol. 12:730809. doi: 10.3389/fmicb.2021.730809
Edited by:
Daniela De Biase, Sapienza University of Rome, ItalyReviewed by:
Zhaolai Dai, China Agricultural University, ChinaJiangxin Wang, Shenzhen University, China
Copyright © 2021 Wu, Xu, Tu and Gao. This is an open-access article distributed under the terms of the Creative Commons Attribution License (CC BY). The use, distribution or reproduction in other forums is permitted, provided the original author(s) and the copyright owner(s) are credited and that the original publication in this journal is cited, in accordance with accepted academic practice. No use, distribution or reproduction is permitted which does not comply with these terms.
*Correspondence: Zhenyan Gao, Z2FvemhlbnlhbjgwQDE2My5jb20=