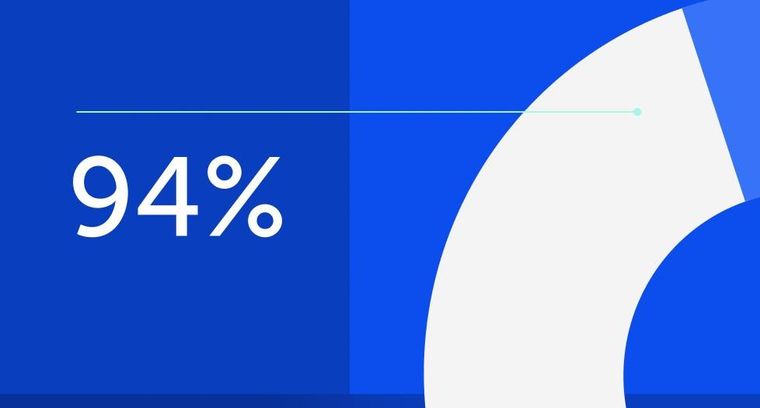
94% of researchers rate our articles as excellent or good
Learn more about the work of our research integrity team to safeguard the quality of each article we publish.
Find out more
ORIGINAL RESEARCH article
Front. Microbiol., 18 October 2021
Sec. Aquatic Microbiology
Volume 12 - 2021 | https://doi.org/10.3389/fmicb.2021.726138
This article is part of the Research TopicResponses of Marine Microbes to Multiple Environmental Drivers of Global Change: the Interplay of Abiotic and Biotic FactorsView all 23 articles
Cellulose and chitin are the most abundant polymeric, organic carbon source globally. Thus, microbes degrading these polymers significantly influence global carbon cycling and greenhouse gas production. Fungi are recognized as important for cellulose decomposition in terrestrial environments, but are far less studied in marine environments, where bacterial organic matter degradation pathways tend to receive more attention. In this study, we investigated the potential of fungi to degrade kelp detritus, which is a major source of cellulose in marine systems. Given that kelp detritus can be transported considerable distances in the marine environment, we were specifically interested in the capability of endophytic fungi, which are transported with detritus, to ultimately contribute to kelp detritus degradation. We isolated 10 species and two strains of endophytic fungi from the kelp Ecklonia radiata. We then used a dye decolorization assay to assess their ability to degrade organic polymers (lignin, cellulose, and hemicellulose) under both oxic and anoxic conditions and compared their degradation ability with common terrestrial fungi. Under oxic conditions, there was evidence that Ascomycota isolates produced cellulose-degrading extracellular enzymes (associated with manganese peroxidase and sulfur-containing lignin peroxidase), while Mucoromycota isolates appeared to produce both lignin and cellulose-degrading extracellular enzymes, and all Basidiomycota isolates produced lignin-degrading enzymes (associated with laccase and lignin peroxidase). Under anoxic conditions, only three kelp endophytes degraded cellulose. We concluded that kelp fungal endophytes can contribute to cellulose degradation in both oxic and anoxic environments. Thus, endophytic kelp fungi may play a significant role in marine carbon cycling via polymeric organic matter degradation.
Coastal habitats have been recognized as carbon (C) cycling hotspots with the potential to influence climate through organic matter (OM) decomposition (Wang and D’Odorico, 2013) controlling the balance between atmospheric CO2 and O2 (Guenet et al., 2010). Coastal vegetation, including mangroves, seagrasses, salt marshes, and macroalgae supply a significant portion of polymeric, organic C (lignin, cellulose, and hemicellulose) to coastal sediments (Duarte et al., 2005; McLeod et al., 2011; Ortega et al., 2020). Furthermore, macroalgae form the largest vegetated habitats in the coastal ocean (ca. 3.4 million km2) with a total annual primary production of ∼ 1.5 Pg C y–1 (Krause-Jensen and Duarte, 2016; Krause-Jensen et al., 2018; Raven, 2018). A significant amount (82%) of macroalgae biomass enters the detrital pathway (Krumhansl and Scheibling, 2012), forming an essential component of the marine C cycle. Of all macroalgae, brown macroalgae (kelp) deposit the largest quantities of detritus, globally, in a variety of receiver habitats from sandy beaches to the deep ocean (Krause-Jensen and Duarte, 2016). This detritus is predominantly comprised of cellulose, hemicellulose (mannan, xylan) and sulfated polysaccharides (carrageenan) (Trevathan-Tackett et al., 2015; Deniaud-Bouët et al., 2017), which may ultimately be buried in the sediment, leached into groundwater (Queirós et al., 2019), or degraded through biological processes (Wang and D’Odorico, 2013). Whether kelp detritus is stored as blue carbon or remineralized depends mainly on microbial communities (Raghukumar, 2004), temperature (Passerini et al., 2016), and oxygen concentration; these are fundamental variables controlling biogeochemical processes (Glud, 2008). Degradation pathways can have a significant influence on coastal and marine C cycling, and the global C budget (Guenet et al., 2010; Worden et al., 2015; Jeewon et al., 2017; Grossart et al., 2019; Chrismas and Cunliffe, 2020). Although the role of bacteria in marine detritus degradation is reasonably well known, the potential role of marine fungi has received relatively little attention.
In terrestrial systems, research has shown that fungi (Ascomycota and Basidiomycota) are the major microbial organisms responsible for degradation of refractory polymers (lignin, cellulose, and chitin) (Van der Wal et al., 2013; Berlemont, 2017; Amend et al., 2019; Weinberger et al., 2020). Fungi are known to occur in marine (Hyde et al., 1998; Bonugli-Santos et al., 2015; Behera et al., 2017), and coastal environments (Alsheikh-Hussain et al., 2014; Lakshmi et al., 2017; Amend et al., 2019), however, studies in marine systems have so far mainly focused on characterizing the fungal community rather than assessing its function (Grossart et al., 2019). The ocean floor is a key marine habitat receiving a substantial fraction of the sinking marine detritus that could be degraded by fungi. However large sections of the ocean floor and deeper coastal sediments (below the upper few centimeters of sediment) are anoxic (Watson, 2016) and the potential for fungal degradation to occur in anoxic sediments has been recognized only since the past decade (Ivarsson et al., 2016; Drake and Ivarsson, 2018; Zain UI Arifeen et al., 2019). Marine macroalgae, which are a major contributor of marine detritus, provide an excellent habitat for endophytic fungi, where fungi live in symbiosis within the macroalgae tissues (Suryanarayanan et al., 2010; Suryanarayanan, 2012). Once the macroalgae dies, these endophytic fungi have the ability to turn in to saprophytes and degrade the dead macroalgae biomass (Hyde and Soytong, 2008; Balabanova et al., 2018). Thus, endophytic fungi may be transported within the macroalgae tissue over long distances and become ultimately buried within deep marine sediments or in coastal sediments. This gives fungi the opportunity to remineralize the detritus to a large extent before colonization by any other decomposer, and the ability to potentially influence colonization by other decomposers (Cline et al., 2018; Wolfe and Ballhorn, 2020). Endophytic fungi may therefore directly and indirectly affect degradation of macroalgae detritus.
Fungi produce a range of versatile enzymes to degrade refractory, polymeric compounds such as lignin, chitin, cellulose, hemicellulose, and phenolics (Harms et al., 2011; Wang and D’Odorico, 2013; Khatoon et al., 2017), some of which are found in macroalgae detritus. The primary fungal degradation enzymes are laccases (Janusz et al., 2017), manganese-dependent peroxidase (MnP), manganese-independent peroxidase (MiP), lignin peroxidase (LiP) (Hammel, 1997), and protocatechuate-3,4 dioxygenase (Leonowicz et al., 2001). These enzymes are assisted by secondary enzymes (aryl alcohol oxidase, and glyoxal oxidase) which produce H2O2 and ⋅OH (Shao et al., 2020). A third group of enzymes [glucose oxidase and cellobiose dehydrogenase (quinone oxidoreductase)] play a crucial role in degrading high-molecular-weight woody material, acting as a feedback system for the primary and secondary enzymes. While these enzymes work synergistically to enhance microbial degradation (Wood and Garcia-Campayo, 1990; Chen et al., 2021), certain enzymes such as laccases, because of their multi-copper oxidases, can also act separately to break down lignin (Leonowicz et al., 2001). Fungi can also potentially break down organic C by inducing cellulose degradation via short-lived reactive oxygen species (ROS), including hydroxyl radicals (⋅OH), superoxide anion radicals (O2–⋅), and singlet oxygen (1O2) formation (Ślesak et al., 2019; Smirnoff and Arnaud, 2019). The short-lived ROS are often formed from the longer-lived ROS H2O2, which is commonly produced in eukaryotic mitochondria (Ivarsson et al., 2016). Hydroxyl radicals and singlet oxygen, in particular, are potent oxidants that can degrade a variety of polymeric, organic C compounds and whose activity can be catalyzed by the presence of other redox-active compounds, such as Cu, Fe, or specific organic moieties (Rose et al., 2010). These same degradation pathways are known to exist in marine and terrestrial fungi, but the knowledge of their ability to degrade polymers in anoxic marine sediments can be improved.
Fungal polymer degradation under oxic conditions has been well studied, where oxygen is used as a terminal electron acceptor (Canfield et al., 2005). Fungal degradation under anoxic conditions is less well known, although yeasts are active under anoxic conditions and can carry out anaerobic respiration, oxic and/or anoxic fermentation and can switch their metabolism (Rangel-Porras et al., 2019). Research into the ability of fungi to degrade OM under anoxic conditions has been expanding over the last decade within the deep biosphere (Ivarsson et al., 2016; Drake and Ivarsson, 2018; Zain UI Arifeen et al., 2019), groundwater (Perkins et al., 2019), and marine environments (Cathrine and Raghukumar, 2009; Lovelock et al., 2017). Certain fungi can function as facultative anaerobes (Ivarsson et al., 2016), using nitrate or nitrite as the terminal electron acceptor (Canfield et al., 2005), or as facultative chemolithotrophs, where metabolic energy is gained from sulfur oxidation (Xu et al., 2018). Given that sulfate reduction rapidly consumes oxygen during kelp degradation (Van Erk et al., 2020) and given that kelp is transported to anoxic/hypoxic environments (buried, or in the deep sea) (Krause-Jensen and Duarte, 2016), understanding oxygen limitation for a potentially facultative decomposing fungi is important for assessing the ability of fungi to contribute to kelp degradation.
The kelp genus Ecklonia occurs globally, and the species Ecklonia radiata is the principal macroalgal species in the temperate reefs of Australia (Kelaher et al., 2013). Here, we cultivated 11 endophytic fungi (10 individual species, with one species having 2 strains) from the brown macroalgal kelp species E. radiata and assessed their ability to degrade various organic polymeric compounds. Organic polymers can be used as a quick indicator for degradation potential and can identify microbial enzymatic activities, aiding to understand microbial degradation potentials (Caldwell et al., 2000; Promputtha et al., 2010; Perkins et al., 2019) and the function of endophytes. We compared the polymeric organic matter decomposition abilities of these kelp endophytic fungi with four common terrestrial fungal species (3 Basidiomycota and 1 Ascomycota). To determine the ability of endophytic fungi to degrade kelp in low oxygen environments (buried in coastal sediments, or transported to the deep sea), we investigated organic polymer degradation indicated through dye decolorization under oxic and anoxic conditions for marine and terrestrial Ascomycota, Basidiomycota, and Mucoromycota. We hypothesized that (1) kelp endophytic fungi can degrade a variety of organic polymeric substrates under oxic conditions, (2) some kelp endophytic fungi can also degrade organic polymeric substrates under anoxic conditions, (3) the degradation potential under oxic and anoxic conditions differs between kelp endophyte species, and (4) there are functional differences between the degradation abilities of Ascomycota, Basidiomycota, and Mucoromycota. If kelp endophytic fungi that are symbiotic within living kelp have the capacity to degrade organic polymers, particularly under anoxic conditions, this suggests that they may become saprophytes of kelp detritus even when buried in the anoxic sediments, representing a currently unquantified coastal carbon cycling process.
Whole and healthy (with no apparent symptoms of disease) Ecklonia radiata specimens were collected from water at 2–4 m depth in Charlesworth Bay, NSW, Australia (30°18′15″S, 153°9′5″E) then immediately transported in fresh seawater to the laboratory. The fresh kelp material was rinsed with sterile tap water and then blotted dry with autoclaved paper towels in a biosafety cabinet, equipped with a laminar flow-hood (Flewelling et al., 2013a). Surface sterilization was tested with 70% ethanol dip for varying durations (5, 10, 15, and 20s), and with Metrex cavicide (for the detailed surface sterilization method, please refer to Supplementary Material). A flame sterilized scalpel was used to cut the kelp material into discs for cultivation. For equipment sterilization, 70% ethanol was used.
From five surface-sterilized E. radiata, approximately 200 circular discs (5–8 mm diameter) were cut with a flame sterilized scalpel. These were taken from different sections (stipe, blade, and holdfast) of E. radiata, to represent the whole E. radiata organism. Discs from the various kelp sections were cultivated on separate plates. The discs were placed onto 70 Petri dishes (9 cm diameter, vented; 3 discs per Petri dish) containing either (1) 2% malt extract agar (MEA), (2) 2% MEA with sterilized seawater (MEA-SW), (3) selective agar for pathogenic fungi (SA) (Sigma Aldrich, BCBN8597TV), or (4) selective agar for pathogenic fungi with sterilized seawater (SA-SW). Seawater was collected during high tide at Seven Mile Beach (NSW, Australia 28.7906102S, 153.5940488E). These plates were incubated for up to 2 weeks at 22–24°C, and all emerging fungal endophytes were isolated onto 2% MEA. To prepare a stock library of each fungus, all isolates were further cultivated on 2% MEA.
To characterize each fungal isolate, ca. 250 mg DNA was extracted from the fungal mycelia of each isolate using the cetrimonium bromide (CTAB)-phenol-chloroform-isoamylalcohol/bead-beating protocol (Nercessian et al., 2005). Briefly, mycelial fragments were homogenized in a bead beater with zirconia/glass beads (0.1, 0.7, and 3 mm) and the following was added: (1) 600 μL 10% CTAB buffer in 1.6 M NaCl with a 1:1 ratio with 240 mM K2HPO4/KH2PO4 buffer, (2) 600 μL phenol:chloroform:isoamylalcohol (25:24:1), (3) 60 μL 10% N-lauryol sarcosine, and (4) 60 μL 10% sodium dodecyl sulfate (SDS). Following shaking (2,850 rpm for 10 min) and centrifugation (16,000× for 10 min at 4°C), the upper aqueous phase was removed and centrifuged in a new reaction tube with chloroform-isoamylacohol (24:1, 650 μL) to remove residual phenol. This solution was then incubated in 30% PEG 6000 prepared in 1.6 M NaCl (1.1 mL) to precipitate the DNA and was centrifuged again. The subsequent pellet was removed and cleaned with 1 mL icy 70% ethanol and centrifuged again. The ethanol was removed with a pipette, and the supernatant was dried at 37°C for up to 10 min. Finally, the recovered genetic material was dissolved in 50 μL PCR water and stored at −18°C until further processing.
For PCR, 2 μL of the stored DNA was mixed in 31.75 μL PCR water, 10 μL buffer, 2 μL bovine serum albumin (BSA), 1 μL each ITS1 and ITS4 (ITS1-5.8S-ITS2 region was amplified; White et al., 1990), 2 μL MgCl2 and 0.2 μL MyTaq Red DNA Polymerase (Bioline, Germany) and the PCR conditions were set at: (i) 95°C for 2 min, (ii) 35 cycles at 95°C for 30 s, (iii) 53°C for 30 s, (iv) 72°C for 45 s, and (v) 72°C for 5 s. The amplification was terminated at 10°C. PCR products were sequenced with Sanger technology at Macrogen Europe. Sequences were assembled using BioEdit (Hall, 1999). The taxonomic assignment of isolates was determined by comparing the ITS sequences against reference databases such as UNITE1 and the NCBI GenBank. Taxonomy was further curated using the Index fungorum.2 For the phylogenetic analysis, we aligned the sequences with MAFFT v7 (Katoh et al., 2018). We generated a maximum likelihood phylogenetic tree with FastTree version 2.1.9 (Price et al., 2010), using a GTR + G + I model of evolution. The resulting phylogenetic tree was displayed and edited in MEGA-X (Kumar et al., 2018). The fungal sequencing data was deposited to NCBI website (ID: 4751, MW999952-66). The link is included in Supplementary Material. For long term preservation, all isolates were freeze-dried at −80°C < 0.1 mBar (Labconco model 7934032) over 3 days.
For organic polymer degradation testing, the culture medium was prepared with, per liter, 0.94 g KH2PO4, 1.9 g K2HPO4, 1.6 g KCl, 1.43 g NaCl, 0.15 g NH4Cl, 0.037 g MgSO4, 0.1 g yeast, 10 g light-malt sugar, and 15 g agar. The solution was aliquoted into six sterile 1-liter Schott Duran glass bottles, kept in a water bath at 55°C, and supplemented with 100 mg streptomycin and penicillin to prevent bacterial contamination. To test for fungal organic polymer utilization, each of the following organic polymers were added to one of five aliquots: (1) 0.1% wt./vol 2,2’-Azino-bis(3-ethylbenzothiazoline-6-sulfonic acid) diammonium salt (ABTS), (2) 0.02% wt./vol Congo Red (CR), (3) 0.02% wt./vol Remazol Brilliant Blue (RBBR), (4) 0.02% wt./vol Toluidine Blue 199 (Tol), or (5) 0.02% wt./vol Bromocresol Green (Bromo). The degradation potential and/or bioactive compound production indicated by each of these substrates is detailed in Table 1. The sixth aliquot was used as a control, with no addition of any organic polymers allowing it to be compared to the growth of fungi with organic polymeric substrates.
Table 1. Isolated kelp endophytic fungi from E. radiata and their occurrence in different plant tissues(HF-holdfast, S-stipe, B-blade).
Once the aliquots were mixed, the media was poured into 9 cm diameter vented Petri dishes, and once solidified the media was inoculated with one of 11 cultivated kelp endophytic fungi or one out of four terrestrial fungi (one Ascomycota, cultivated from local soils, and three commercially available Basidiomycota [Oyster (Pleurotus sp.), Shiitake (Lentinula sp.), and Tasman Reishi (Ganoderma sp. from “Aussie mushroom supplies”), no strain number available)]. The total number of Petri dishes was 192 [16 (15 fungi + 1 control) times 12 (5 organic polymer + 1 MEA control plates), duplicated for oxic and anoxic treatments]. To ensure that each Petri dish was inoculated with a similar mass of fungi, a flame sterilized molded wire was used. For each fungus, material for inoculations was taken from a single initial plate to ensure the same organism was used across treatments. The terrestrial fungi were chosen to gain a deeper knowledge of dissimilarities or similarities in degradation functions of the three fungal phyla and of kelp endophytic fungi from marine sources with common terrestrial fungi. Of the 192 plates, one set (96) was sealed with parafilm in front of the laminar flow hood and was packed into a clear still air box. To assess the degradation during anoxic conditions, the second set (96) was placed into a pre-sterilized anaerobic chamber. Any specimen transferred to the chamber was first purged with high purity N2. The anaerobic chamber was a COY model 10 gas analyzer, which automatically measures H2 and O2. Anoxic conditions were maintained at under 1–5% H2 in a N2 atmosphere at ambient temperature, using a Pd catalyst that consumes O2. This was monitored by an inbuilt alarm system that would be triggered if the gas concentration fell outside the chosen range. Due to the requirement for N2 purging, the second set of inoculates could only be sealed inside the anaerobic chamber. Any tool used in the chamber was first sterilized and purged with high purity N2. Kelp degradation where detritus was collected occurs at highly variable temperatures (in Australia 5–40°C); Petri dishes were kept at a median temperature (22–24°C) and under natural light conditions.
Each of the isolates was assessed weekly to determine growth and color change. Changes in color indicate catalytic conversion through the splitting of organic polymeric bonds in the substrates (Saroj and Narasimhulu, 2018). This enzymatic activity, known as hydrolysis, occurs through the production of acids or bases by the hydroxide anion and hydrogen cation of water during fungal OM degradation. The production of degrading enzymes changes the color of the media, and the type of enzymes determines the color change during hydrolysis (e.g., for CR loss of red color, or the media becoming clear would indicate acidic enzymatic production, whereas darkening of the media indicates alkaline enzymatic production). Hydrolysis was visually observed and was assessed (+, or ++) depending on the area of the specimen (diameter in cm) with changes in color to the media (Saroj and Narasimhulu, 2018) (if the color change did not exceed the area under the specimen received + and if the color change exceeded the area under the specimen received ++). Once a specimen received ++, or the specimen reached 90% growth on the Petri dish, it was kept for further observation but was not re-assessed. The isolates in oxic conditions were maintained for 6 weeks. As the anaerobic chamber acts as a laminar flow hood and as anaerobic degradation takes longer (Prasad et al., 2007), the isolates under anoxia were kept there for 16 weeks. After week 16, the fungi were transferred to a still air box and kept there for another week to observe any response following the change from anoxic to oxic conditions. Bacterial or mold contamination was not observed on any media throughout the experiments.
Isolated strains were identified by visual morphological assessment (Supplementary Figure 1), and their identity confirmed via genetic analysis. A phylogenetic tree of the 11 fungal isolates from E. radiata kelp (Figure 1) assigned the fungi to three phyla (Ascomycota, Mucoromycota, and Basidiomycota), five classes (Eurotiomycetes, Sordariomycetes, Dothideomycetes, Mucoromycetes, and Agaricomycetes), six orders (Eurotiales, Xylariales, Pleosporales, Capnodiales, Mucorales, and Polyporales), and seven families (Aspergillaceae, Sporocadaceae, Didymellaceae, Trichocomaceae, Mucoraceae, and Polyporaceae) (Supplementary Table 2). From E. radiata tissues (blade, stipe, and holdfast), the species more frequently isolated were M. circinelloides (2 strains identified from 1 species on over 30 plates, although there may be other strains), Penicillium sp. (3 species on 23 plates, although there may be other species or strains), and Cladosporium sp. (15 plates). In contrast, T. assiuntensis was isolated only on four plates. Each plant section from E. radiata was separately cultivated to determine tissue specificity, particularly for the holdfast (Table 1).
Figure 1. Maximum likelihood tree of all tested fungi in this study. The ITS1-5.8S-ITS2 sequences were aligned with MAFFT, the phylogenetic tree was generated with FastTree using a GTR + G + I model and visualized with MEGA X. Bootstrap values are indicated on the nodes. The red squares represent terrestrial fungi that were not derived from E. radiata. The scale represents the sequence divergence between the number of nucleotide substitutions per site.
All E. radiata endophytic fungal isolates presented activities related with the degradation (change of color) of the polymeric compounds analyzed under oxic conditions, with varying effectiveness over time (Table 2 and Supplementary Table 1). CR is commonly used as a sensitive assay for the cellulase enzyme; this enzyme breaks down cellulose (Sazci et al., 1986; Florencio et al., 2012), which is the major component of E. radiata detritus. Strong decolorization of CR, which is associated with degradation of cellulose, was observed in A. proliferans, E. sorghinum, Cladosporium sp., P. corylophilum, Penicillium sp., P. fagi, and M. circinelloides, all of which produced significant (++) color change within 1 week. G. sessile and T. tephropora (endophytic Basidiomycota) changed the color of CR, but only by week three.
Table 2. Degradation of organic polymers by endophytic fungal isolates from kelp after 3 and 6 weeks under oxic conditions.
Hydrolysis (color change) of Tol is indicative of sulfur-containing lignin peroxidase activity (Hamza et al., 2012) and was observed within 1 week for B. pondoensis, E. sorghinum, Cladsporium sp. A. proliferans, B. pondoensis, E. sorghinum, Cladosporium sp., P. fagi, and M. circinelloides. No color change on Tol was detected for any Basidiomycota at this time and, overall, Basidiomycota showed a stronger color change of ABTS, RBBR, and Bromo than Ascomycota and Mucoromycota.
Within the first week, all Basidiomycota fully changed the color of ABTS, a compound associated with laccase activity (Leonowicz et al., 2001), whereas all Ascomycota required more time and showed less intensity in the color change. The change of color in RBBR by Basidiomycota, linked to ligninolytic and laccase activities (Sing et al., 2017), was slower than the color change on ABTS. Ascomycota and Mucoromycota showed a color change on RBBR only after 4 weeks. The color change of Bromo (associated with sulfonephthaleins lignin degrading enzymes) first occurred in week three for Basidiomycota, and in general, Ascomycota and Mucoromycota showed less activity. Overall, the Fusarium sp. patterns in changing the color of the media under oxic conditions were similar to Mucoromycota strains.
In general, growth or activity on polymers without any atmospheric oxygen was slower and no polymer changed color within the first 3 weeks (Table 3). CR was the first substrate to change color by week six under anoxic conditions; by this time four of the E. radiata endophytic fungal isolates (+) had changed the color of the media. G. sessile was the only Basidiomycota that changed color of any media, being ABTS by week 12. The control (MEA) was the least favored substrate for all fungi under anoxic conditions. The last 4 weeks of incubation resulted in more color changes for the same isolates. In contrast to Basidiomycota, endophytic Ascomycota successfully survived and revived after exposure to oxic conditions following 16 weeks of anoxia.
Table 3. Degradation of organic polymers by endophytic fungal isolates from kelp after 3, 6, and 12 weeks under anoxic conditions.
E. radiata had a diverse mycobiome of endophytic fungi and the 10 cultivated species and two strains represented three phyla: Ascomycota, Basidiomycota, and Mucoromycota (Supplementary Table 2). The phylum Ascomycota is the largest endophytic phylum (over 70,000 species in terrestrial and marine environments; Rashmi, 2019), and ca. 70% of endophytic fungi from E. radiata belong to this phylum. Eurotiomycetes, Dothideomycetes (Ascomycota), and Agaricomycetes (Basidiomycota) are the most commonly recorded endophyte classes (Rashmi, 2019) and were also dominant in E. radiata (eight of eleven isolates) (Supplementary Table 2). Pleosporales, Eurotiales, and Xylariales are the most commonly recorded endophytic orders (Rashmi, 2019), with seven of our eight isolates belonging to these orders (Supplementary Table 2). Here we cultivated five isolates (Penicillium sp., Aspergillus sp., and Cladosporium sp.; Supplementary Table 2), which are frequently isolated endophytes (Rashmi, 2019), from various macroalgae (Zuccaro et al., 2003, 2008; Suryanarayanan et al., 2010; Flewelling et al., 2013b; Sarasan et al., 2020). Endophytic fungi from E. radiata were cosmopolitan, found in other hosts or different environments (Table 1). Endophytic fungi are a diverse group, inhabiting and degrading terrestrial (Arnold, 2007; Kusari et al., 2012; Rana et al., 2019; Yan et al., 2019; Manganyi and Ateba, 2020) and marine vegetation (Hamzah et al., 2018; Noorjahan et al., 2019; Teixeira et al., 2019; Parthasarathy et al., 2020; Calado et al., 2021), linking ecology to plant pathology (Shipunov et al., 2008; Behie and Bidochka, 2014; Leach et al., 2017; Latz et al., 2018; Poveda et al., 2020), and evolution (Miyauchi et al., 2020; Tiwari and Bae, 2020), as well as plant communication and nutrient distribution (Kothe and Turnau, 2018; Sharifi and Ryu, 2021). Endophytic fungi live in symbiosis within the macroalgae, as in terrestrial vegetation (Jia et al., 2016), or in marine vegetation (Hamzah et al., 2018; Noorjahan et al., 2019; Calado et al., 2021) and are likely to regulate many biochemical pathways through their life cycle (Navarro-Meléndez and Heil, 2014; Latz et al., 2018). Clearly, endophytic fungi are likely of considerable importance, potentially for OM degradation (macrophytes, or other vegetation sources) and C cycling in terrestrial and marine environments.
This study focused on endophytic fungi from marine kelp, and we confirmed their presence. Our degradation assay demonstrates that kelp endophytic fungi can potentially turn to saprophytes, and we show that these fungi have the ability to decolorize several polymeric compounds found within the kelp detritus. Kelp detritus often travels great distances and our assay suggest that, after the death of E. radiata saprophytic endophytic fungi may be as important in marine sediments as has been reported for terrestrial soils (Mohamed and Martiny, 2011; Worden et al., 2015; Dini-Andreote et al., 2016; Ortega-Arbulú et al., 2019). Furthermore, endophytic fungi may influence kelp degradation in both oxic and anoxic environments (Cline et al., 2018; Wolfe and Ballhorn, 2020).
Fungal oxic degradation may be important in the water column, or on top of the kelp pile on the beach. The ability of the three phyla (Ascomycota, Basidiomycota, and Mucoromycota) to decolorize the various polymeric substrates differed significantly. Ascomycota and Mucoromycota primarily decolorized CR and Tol, which could indicate their cellulose degradation potential (pointing to the production of MnP, MiP, and sulfur-containing LiP), whereas Basidiomycota decolorized ABTS, RBBR, and Bromo (reflecting the potential production of laccase and LiP) (Supplementary Table 1). Importantly, although this study highlights the potential role of endophytic fungi in kelp degradation, the ability of fungi to degrade kelp detritus cannot be categorically determined through their dye decolorization abilities alone. However, the considerable differences in the dye decolorization ability of the various fungal species tested suggest that the decolorization was not simply caused by biosorption but was rather related to some difference in the biodegradation ability among the fungal species. These differences in degradation potential supports the ability of fungi to co-exist through niche-partitioning, as previously reported (Asgher et al., 2008). The composition of the fungal community within kelp may therefore be important for kelp detritus degradation.
Unlike in terrestrial environments, anoxic conditions are relatively common in coastal and marine sediments, where kelp detritus is deposited. The anoxic degradation potential of kelp endophytes is therefore particularly important. In this study we showed that some of the endophytic fungal isolates were able to decolorize CR in the absence of oxygen, indicating that they may have the potential to play a significant role in kelp (cellulose) degradation within anoxic sediments. This is in line with recent reports of anoxic fungal degradation in terrestrial (Ivarsson et al., 2016; Drake and Ivarsson, 2018) and marine environments (Barone et al., 2019; Zain UI Arifeen et al., 2019). CR was the substrate that was most decolorized by fungi under anoxia; this is not surprising, as CR is the most soluble and least recalcitrant of all substrates tested (Costa et al., 2012). Under oxic conditions the color change of CR media varied between species, from a loss in color to the media becoming dark (red, gray, or black) and, in some cases, later becoming losing color again after initially darkening. Under anoxic conditions, however, the media turned dark during hydrolysis. One single species of Basidiomycota (G. sessile) indicated activity on ABTS (associated with laccase production) under anoxic conditions. These results suggest that Ascomycota and Mucoromycota may have the potential to degrade cellulose under anoxic conditions.
Three endophytes were active under anoxia (P. corylophilum, M. circinelloides, and B. pondoensis) and of the isolated fungi, these three species showed the highest potential to degrade kelp in anoxic environments. The ability of these species to contribute to anoxic kelp degradation is not surprising. Under anoxic conditions P. corylophilum can reduce the chemical oxygen demand of activated sludge (Mannan et al., 2007), B. pondoensis produces cellulase (Yadav and Reddy, 2013) and emits volatile organic compounds (Navarro-Meléndez and Heil, 2014), and M. circinelloides has been shown to have the ability to use numerous C sources (e.g., xylose, arabinose, glycerol, starch, cellulose, or chitin) (Xia et al., 2011). M. circinelloides was the most active isolate under anoxic conditions, producing more cellulose-degrading and lignin-degrading enzymes (RBBR and Bromo). M. circinelloides is a yeast-mold dimorphic species, where dimorphism is influenced by C metabolism (Díaz-Pérez et al., 2020), thus it can switch between fermentative and oxidative processes (Crabtree effect—aerobic fermentation) (Rangel-Porras et al., 2019). Aerobic fermentation was found during macroalgae degradation (Van Erk et al., 2020). A likely pathway for fungi to break down macroalgal cellulose, whether in oxic or anoxic conditions, is via MnP or MiP production (Dos Santos et al., 2007) and subsequent splitting of cellulose bonds via hydrolysis (Conrad, 2006; Jung et al., 2015). MnP acts as a catalyst in the degradation of cellulose or phenolic and non-phenolic compounds in macroalgae, using H2O2 as an oxidant (Shin et al., 2005). The three endophytic fungi that were active under anoxic conditions in the current study probably work on charges alone and as such may not require oxygen. Aerobic fermentation during kelp degradation offers potential for more fungi to capitalize on the rich C source from kelp even in anoxic environments.
The productivity of kelp is the highest amongst all coastal vegetation (Krause-Jensen and Duarte, 2016; Raven, 2018). Of all kelp, the widely distributed genus Ecklonia has moderately high productivity and detritus production (Krumhansl and Scheibling, 2012). Therefore, it is important to understand how C is cycled through the lifecycle Ecklonia sp., how that relates to marine carbon cycling, and to establish if the rich endophytic mycobiome has the ability to influence kelp degradation. In marine pelagic systems or coastal ecosystems, fungi have received far less attention than in terrestrial systems (∼1,900 documented marine species compared to over 100,000 documented terrestrial species) (Hyde et al., 1998; Ortega-Arbulú et al., 2019), and marine fungal degradation has received far less attention than marine bacterial OM degradation. Fungal pathways for kelp degradation are yet to be explored, whereas kelp degradation by bacteria (Bacteroidetes, Verrucomicrobia, Planctomycetes, Gammaproteobacteria, and Chlamydiae) has been intensively investigated (Sakai et al., 2003; Bengtsson et al., 2011; Silchenko et al., 2013; Sichert et al., 2020; Sun et al., 2021). Bacteria account for 60% (Sichert et al., 2020) to 90% (Koop et al., 1982) of kelp detritus degradation. Kelp detritus degradation by fungal degradation has been largely disregarded (Koop et al., 1982; Bengtsson et al., 2011; Van Erk et al., 2020), despite the dominant role of terrestrial fungi in refractory OM degradation (Gooday, 1990a; Tedersoo et al., 2014; Treseder and Lennon, 2015; Beaton et al., 2019). As has been reported previously, (Ivarsson et al., 2016; Drake and Ivarsson, 2018; Barone et al., 2019) the current study demonstrates that, like bacteria, some fungi may be active under anoxic conditions and have the capacity to break down kelp cellulose in various habitats. In terrestrial environments, an antagonistic interaction between bacteria and fungi is reported (Gooday, 1990b; Mille-Lindblom and Tranvik, 2003), and this may also be true for the marine environment. As cellulose is the most abundant polymer in marine systems, cellulose degradation is an important process for global C cycling (Leschine, 1995; Hyde et al., 1998; Worden et al., 2015; Ortega-Arbulú et al., 2019; Chrismas and Cunliffe, 2020). The role of both fungi and bacteria for kelp detritus degradation processes needs to be evaluated in further detail. Given that fungal polymeric OM degradation in terrestrial systems outcompetes bacterial degradation (Malik et al., 2016; Fabian et al., 2017), fungi in anoxic marine sediments may also contribute significantly to global C cycling (Worden et al., 2015; Lakshmi et al., 2017; Balabanova et al., 2018).
To date, kelp degradation by fungi has received little attention and this study highlights that endophytic fungi in kelp have the potential to contribute to kelp detritus degradation. Cultivation based techniques would be complemented with further studies; DNA analysis to confirm the endophytic fungal community within the macroalgae and genomics to confirm the specific role of fungi in kelp detritus degradation with the underlying mechanisms, such as the relative contribution of specific enzymes (e.g., MnP, MiP, and LiP). Kelp degradation can be assessed by using a single dye or a mixture of dyes coupled with different environmental factors (e.g., pH, temperature, oxygen, and agitation) (Rani et al., 2014; Sen et al., 2016). Biosorption is a major mechanism in dye decolorization and can address the differences (e.g., deposition, entrapment in inner spaces, surface ion-exchange, complexation, precipitation, adsorption, and the formation of hydrogen bonds) within living and dead fungal cells (Yeddou-Mezenner, 2010). Moreover, coastal contamination (e.g., agriculture, or waste processing) can influence macroalgal community dynamics and seedling bioassays using different dyes can define how these contaminants affect growth rate, or the physiological fitness of the macroalgal community. Pollution also holds the potential to impact the kelp endophytic fungal community, their kelp degradation rates and thus marine C cycling.
The datasets presented in this study can be found in online repositories. The names of the repository/repositories and accession number(s) can be found below: https://www.ncbi.nlm.nih.gov/, ID: 4751, MW999952-66.
AP helped conceive the project, collected, analyzed, and interpreted the data, and drafted the manuscript. AR, H-PG, and JO helped conceive the project and contributed substantially to the manuscript. KR-J contributed substantially to data analysis and interpretation. SB contributed to collecting specimens and data and assisted with the manuscript. All listed authors have contributed substantially to the preparation and drafting of this manuscript and have approved the final submitted manuscript.
Funding for this research was provided by German Research Foundation (DFG) project GR1540/30-1.
The authors declare that the research was conducted in the absence of any commercial or financial relationships that could be construed as a potential conflict of interest.
All claims expressed in this article are solely those of the authors and do not necessarily represent those of their affiliated organizations, or those of the publisher, the editors and the reviewers. Any product that may be evaluated in this article, or claim that may be made by its manufacturer, is not guaranteed or endorsed by the publisher.
We thank Kirsten Benkendorff (the director of the National Marine Science Center) for her full support of this project. Nick Ward, Matthew Tonge, Roslyn Hagan (Southern Cross University, Geoscience) for their laboratory support. We acknowledge the support of the Deutsche Forschungsgemeinschaft and Open Access Publishing Fund of University of Potsdam.
The Supplementary Material for this article can be found online at: https://www.frontiersin.org/articles/10.3389/fmicb.2021.726138/full#supplementary-material
Abdel-Hafez, S. I. I., Abo-Elyousr, K. A. M., and Abdel-Rahim, I. R. (2015). Leaf surface and endophytic fungi associated with onion leaves and their antagonistic activity against Alternaria porri. Czech. Mycol. 67, 1–22. doi: 10.33585/cmy.67101
Alsheikh-Hussain, A., Altenaiji, E. M., and Yousef, L. F. (2014). Fungal cellulases from mangrove forests-A short review. J. Biochem. Tech. 5, 765–774.
Amend, A., Burgaud, G., Cunliffe, M., Edgcomb, V. P., Ettinger, C. L., Gutiérrez, M. H., et al. (2019). Fungi in the marine environment: open questions and unsolved problems. mBio 10, e1189–e1118. doi: 10.1128/mBio.01189-18
Arnold, A. E. (2007). Understanding the diversity of foliar endophytic fungi: progress, challenges, and frontiers. Fungal Biol. Rev. 21, 51–66. doi: 10.1016/j.fbr.2007.05.003
Arzanlou, M. (2012). Contribution to the knowledge of pestalotioid fungi of Iran. Mycosphere. 3:12 doi: 10.5943/mycosphere/3/5/12
Asgher, M., Bhatti, H. N., Ashraf, M., and Legge, R. L. (2008). Recent developments in biodegradation of industrial pollutants by white rot fungi and their enzyme system. Biodegradation 19:771. doi: 10.1007/s10532-008-9185-3
Balabanova, L., Slepchenko, L., Son, O., and Tekutyeva, L. (2018). Biotechnology potential of marine fungi degrading plant and algae polymeric substrates. Front. Microbiol. 9:1527. doi: 10.3389/fmicb.2018.01527
Barone, G., Varrella, S., Tangherlini, M., Rastelli, E., Dell’Anno, A., Danovaro, R., et al. (2019). Marine fungi: Biotechnological perspectives from deep-hypersaline anoxic basins. Diversity. 11:113. doi: 10.3390/d11070113
Beaton, D., Pelletier, P., and Goulet, R. R. (2019). Microbial degradation of cellulosic material and gas generation: implications for the management of low-and intermediate-level radioactive waste. Front. Microbiol. 10:204. doi: 10.3389/fmicb.2019.00204
Behera, B. C., Sethi, B. K., Mishra, R. R., Dutta, S. K., and Thatoi, H. N. (2017). Microbial cellulases – Diversity and biotechnology with reference to mangrove environment: a review. J. Genet. Eng. Biotechnol. 15, 197–210. doi: 10.1016/j.jgeb.2016.12.001
Behie, S. W., and Bidochka, M. J. (2014). Nutrient transfer in plant–fungal symbioses. Trends Plant Sci. 19, 734–740. doi: 10.1016/j.tplants.2014.06.007
Ben Younes, S., Mechichi, T., and Sayadi, S. (2007). Purification and characterization of the laccase secreted by the white rot fungus Perenniporia tephropora and its role in the decolourization of synthetic dyes. J. Appl. Microbiol. 102, 1033–1042. doi: 10.1111/j.1365-2672.2006.03152.x
Bengtsson, M. M., Sjøtun, K., Storesund, J. E., and Øvreås, J. (2011). Utilization of kelp-derived carbon sources by kelp surface-associated bacteria. Aquat. Microb. Ecol. 62, 191–199. doi: 10.3354/ame01477
Berlemont, R. (2017). Distribution and diversity of enzymes for polysaccharide degradation in fungi. Sci. Rep. 7:222. doi: 10.1038/s41598-017-00258-w
Bonugli-Santos, R. C., Dos Santos Vasconcelos, M. R., Passarini, M. R., Vieira, G. A., Lopes, V. C., Mainardi, P. H., et al. (2015). Marine-derived fungi: diversity of enzymes and biotechnological applications. Front. Microbiol. 6:269. doi: 10.3389/fmicb.2015.00269
Bouhri, Y., Askun, T., Tunca, B., Deniz, G., Ak Aksoy, S., and Mutlu, M. (2020). The orange-red pigment from Penicillium mallochii: pigment production, optimization, and pigment efficacy against Glioblastoma cell lines. Biocatal. Agric. Biotechnol. 23:101451. doi: 10.1016/j.bcab.2019.101451
Braga, R. M., Padilla, G., and Araújo, W. L. (2018). The biotechnological potential of Epicoccum spp.: diversity of secondary metabolites. Crit. Rev. Microbiol. 44, 759–778. doi: 10.1080/1040841X.2018.1514364
Cai, J., Yhou, X. M., Yang, X., Tang, M. M., Liao, Q. Y., Meng, B. Z., et al. (2020). Three new bioactive natural products from the fungus Talaromyces assiutensis JTY2. Bioorg. Chem. 94:103362. doi: 10.1016/j.bioorg.2019.103362
Calado, M., da, L., Silva, J., Alves, C., Susano, P., Santos, D., et al. (2021). Marine endophytic fungi associated with Halopteris scoparia (Linnaeus) Sauvageau as producers of bioactive secondary metabolites with potential dermocosmetic application. PLoS One 16:e0250954. doi: 10.1371/journal.pone.0250954
Caldwell, B. A., Jumpponen, A., and Trappe, J. M. (2000). Utilization of major detrital substrates by dark-septate, root endophytes. Mycologia. 92, 230–232. doi: 10.2307/3761555
Canfield, D. E., and Bo Thamdrup Erik Kristensen. (2005). Heterotrophic Carbon Metabolism. Adv. Mar. Biol. 48, 129–166. doi: 10.1016/S0065-2881(05)48005-0
Cathrine, S. J., and Raghukumar, C. (2009). Anaerobic denitrification in fungi from the coastal marine sediments off Goa. India Mycol. Res. 113, 100–109. doi: 10.1016/j.mycres.2008.08.009
Chen, A. J., Hubka, V., Frisvad, J. C., Visagie, C. M., Houbraken, J., Meijer, M., et al. (2017). Polyphasic taxonomy of Aspergillus (formerly Eurotium), and its occurrence in indoor environments and food. Stud. Mycol. 88, 37–135. doi: 10.1016/j.simyco.2017.07.001
Chen, X., Cheng, W., Li, S., Tang, X., and Wei, Z. (2021). The “quality” and “quantity” of microbial species drive the degradation of cellulose during composting. Bioresour. Technol. 320:124425. doi: 10.1016/j.biortech.2020.124425
Chrismas, N., and Cunliffe, M. (2020). Depth-dependent mycoplankton glycoside hydrolase gene activity in the open ocean—evidence from the Tara Oceans eukaryote metatranscriptomes. ISME J. 14, 2361–2365. doi: 10.1038/s41396-020-0687-2
Cline, L. C., Schilling, J. S., Menke, J., Groenhof, E., and Kennedy, P. G. (2018). Ecological and functional effects of fungal endophytes on wood decomposition. Funct. Ecol. 32, 181–191. doi: 10.1111/1365-2435.12949
Conrad, R. (2006). Contribution of hydrogen to methane production and control of hydrogen concentrations in methanogenic soils and sediments. FEMS Microbiol. Ecol. 28, 193–202. doi: 10.1111/j.1574-6941.1999.tb00575.x
Costa, M. C., Mota, F. S., Dos Santos, A. B., Farias Mendonca, G. L., and Do Nascimento, R. F. (2012). Effect of dye structure and redox mediators on anaerobic azo and anthraquinone dye reduction. Quim. Nova. 35, 482–486. doi: 10.1590/S0100-40422012000300008
Crous, P. W., Wingfield, M. J., Le Roux, J. J., Richardson, D. M., Strasberg, D., Shivas, R. G., et al. (2015). Fungal planet description sheets. Persoonia 2015, 371–399. doi: 10.3767/003158515X690269
Deka, D., and Jha, D. K. (2020). Bioactivity assessment of endophytic fungi associated with Citrus macroptera Montr.: an endangered ethnomedicinal plant used in folk medicines in North-East India. Indian Phytopathol. 73, 21–33. doi: 10.1007/s42360-019-00179-w
Deniaud-Bouët, E., Hardouin, K., Potin, P., Kloareg, B., and Hervé, C. (2017). A review about brown algal cell walls and fucose-containing sulfated polysaccharides: Cell wall context, biomedical properties and key research challenges. Carbohydr. Pol. 175, 395–408. doi: 10.1016/j.carbpol.2017.07.082
Díaz-Pérez, S. P., Patino-Medina, A., Valle-Maldonado, M., López-Torres, A., Jácome-Galarza, I. E., Anaya-Martinez, V., et al. (2020). Alteration of fermentative metabolism enhances Mucor circinelloides virulence. Infect. Immun. 88, e434–e419. doi: 10.1128/IAI.00434-19
Dini-Andreote, F., Satler Pylro, V., Baldrian, P., Van Elsas, J. D., and Falcão Salles, J. (2016). Ecological succession reveals potential signatures of marine-terrestrial transition in salt marsh fungal communities. ISME J. 10, 1984–1997. doi: 10.1038/ismej.2015.254
Dos Santos, A. B., Cervantes, F. J., and van Lier, J. B. (2007). Review paper on current technologies for decolourisation of textile wastewaters: perspectives for anaerobic biotechnology. Bioresourc. Technol. 98, 2369–2385. doi: 10.1016/j.biortech.2006.11.013
Drake, H., and Ivarsson, M. (2018). The role of anaerobic fungi in fundamental biogeochemical cycles in the deep biosphere. Fungal Biol. Rev. 32, 20–25. doi: 10.1016/j.fbr.2017.10.001
Duarte, C. M., Middelburg, J. J., and Caraco, N. (2005). Major role of marine vegetation on the oceanic carbon cycle. Biogeosciences 2, 1–8. doi: 10.5194/bg-2-1-2005
Fabian, J., Zlatanovic, S., Mutz, M., and Premke, K. (2017). Fungal-bacterial dynamics and their contribution to terrigenous carbon turnover in relation to organic matter quality. ISME J. 2, 415–425. doi: 10.1038/ismej.2016.131
Flewelling, A. J., Currie, J., Gray, C. A., and Johnson, J. A. (2015). Endophytes from marine macroalgae: Promising sources of novel natural products. Curr. Sci. 109, 88–11.
Flewelling, A. J., Johnson, J. A., and Gray, C. A. (2013a). Isolation and bioassay screening of fungal endophytes from North Atlantic marine macroalgae. Bot. Mar. 56:224. doi: 10.1515/bot-2012-0224
Flewelling, A., Ellsworth, K. T., Sanford, J., Forward, E., Johnson, J. A., and Gray, C. A. (2013b). Macroalgal endophytes from the atlantic coast of canada: a potential source of antibiotic natural products? Microorganisms 1, 175–187. doi: 10.3390/microorganisms1010175
Florencio, C., Couri, S., and Farinas, C. S. (2012). Correlation between agar plate screening and solid-state fermentation for the prediction of cellulase production by trichoderma strains. Enzyme. Res. 2012:793708. doi: 10.1155/2012/793708
Garcia Silva, M., Furtado, N. A., Pupo, M. T., Fonseca, M. J., Said, S., and Da Silva (2004). Antibacterial activity from Penicillium corylophilum Dierckx. Microbiol. Res. 159, 317–322. doi: 10.1016/j.micres.2004.06.003
Glud, R. N. (2008). Oxygen dynamics of marine sediments. Mar. Biol. Res. 4, 243–289. doi: 10.1080/17451000801888726
Gómez-Toribio, V., Garcia-Martin, A. B., Martinez, M. J., Martinez, A. T., and ad Guillén, F. (2009). Induction of extracellular hydroxyl radical production by white-rot fungi through quinone redox cycling. Appl. Environ. Microbiol. 75, 3944–3953. doi: 10.1128/AEM.02137-08
Gong, B., Yao, X. H., Zhang, Y. Q., Fang, H. Y., Pang, T. C., and Dong, Q. L. (2015). A cultured endophyte community is associated with the plant Clerodendrum inerme and antifungal activity. Gen. Mol. Res. 14, 6084–6093. doi: 10.4238/2015.June.8.6
Gooday, G. W. (1990a). Physiology of microbial degradation of chitin and chitosan. Biodegradation 1, 177–190. doi: 10.1007/BF00058835
Gooday, G. W. (1990b). The ecology of chitin degradation. Adv. Microb. Ecol. 11:10. doi: 10.1007/978-1-4684-7612-5_10
Grossart, H. P., Van den Wyngaert, S., Kagami, M., Wurzbacher, C., Cunliffe, M., and Rojas-Jimenez, K. (2019). Fungi in aquatic ecosystems. Nat. Revi. Microbiol. 17, 339–354. doi: 10.1038/s41579-019-0175-8
Guenet, B., Danger, M., Abbadie, L., and Lacroix, G. (2010). Priming effect: bridging the gap between terrestrial and aquatic ecology. J. Ecol. 91, 2850–2861. doi: 10.1890/09-1968.1
Hall, T. A. (1999). BioEdit: a user-friendly biological sequence alignment editor and analysis program for Windows 95/98/NT. Nucleic Acids Sympo. Ser. 41, 95–98.
Hammel, K. E. (1997). “Fungal degradation of lignin,” in Driven by Nature: Plant Litter Quality and Decomposition, eds G. Cadisch and K. E. Giller (Wallingford: CAB International), 33–45.
Hamza, S. A., Iyun, J. F., and Idris, S. O. (2012). Kinetics and mechanism of the redox reaction of toluidine blue and nitrite ions in aqueous acidic medium. Arch. Appl. Sci. Res. 4, 10–18.
Hamzah, T. N. T., Lee, S. Y., Hidayat, A., Terhem, R., Faridah-Hanum, I., and Mohamed, R. (2018). Diversity and characterization of endophytic fungi isolated from the tropical mangrove species, rhizophora mucronata, and identification of potential antagonists against the soil-borne fungus, fusarium solani. Front. Microbiol. 9:1707. doi: 10.3389/fmicb.2018.01707
Harms, H., Schlosser, D., and Wick, L. Y. (2011). Untapped potential: Exploiting fungi in bioremediation of hazardous chemicals. Nat. Revi. Microbiol. 9, 177–192. doi: 10.1038/nrmicro2519
Hassan, A. A., Abdel-Sater, M. A., and Soliman, Z. (2018). Biodiversity of filamentous and yeast fungi in citrus and grape fruits and juices in Assiut area, Egypt. J. Microbiol. Biotechnol. Food Sci. 7, 353–365. doi: 10.15414/jmbfs.2018.7.4.353-365
Hyde, K. D., Jones, E. B. G., Leaño, E., Pointing, S. B., Poonyth, A. D., and Vrijmoed, L. L. P. (1998). Role of fungi in marine ecosystems. Biodivers. Conserv. 7, 1147–1161. doi: 10.1023/A:1008823515157
Ivarsson, M., Schnürer, A., Bengtson, S., and Neubeck, A. (2016). Anaerobic fungi: A potential source of biological H2 in the oceanic crust. Front. Microbiol. 7:674. doi: 10.3389/fmicb.2016.00674
Janusz, G., Pawlik, A., Sulej, J., Swiderska-Burek, U., Jarosz-Wilkolazka, A., and Paszczynski, A. (2017). Lignin degradation: Microorganisms, enzymes involved, genomes analysis and evolution. FEMS Microbiol. Rev. 41, 941–962. doi: 10.1093/femsre/fux049
Jeewon, R., Wanasinghe, D. N., Rampadaruth, S., Puchooa, D., and Zhou Li-Gang (2017). Nomenclatural and identification pitfalls of endophytic mycota based on DNA sequence analyses of ribosomal and protein genes phylogenetic markers: A taxonomic dead end? Mycosphere 8, 1802–1817. doi: 10.5943/mycosphere/8/10/7
Jia, M., Chen, L., Xin, H.-L., Zheng, C.-J., Rahman, K., Han, T., et al. (2016). A Friendly relationship between endophytic fungi and medicinal plants: a systematic review. Front. Microbiol. 7:906. doi: 10.3389/fmicb.2016.00906
Jin, Z., Li, D., Liu, T., Yu, F., Zhang, Z., Su, C., et al. (2017). Cultural endophytic fungi associated with Dendrobium officinale: identification, diversity estimation and their antimicrobial potential. Curr. Sci. 112, 1690–1697. doi: 10.18520/cs/v112/i08/1690-1697
Jung, S. J., Kim, S. H., and Chung, I. M. (2015). Comparison of lignin, cellulose, and hemicellulose contents for biofuels utilization among 4 types of lignocellulosic crops. Biomass Bioenergy 83, 322–327. doi: 10.1016/j.biombioe.2015.10.007
Kanmani, P., Satish Kumar, R., Yuvaraj, N., Paari, K. A., Pattukumar, V., and Arul, V. (2011). Microbial decolorization of synthetic dyes and reactive dyes of industrialeffluents by using a novel fungus aspergillus proliferans. Water Environ. Res. 83, 2099–2106. doi: 10.2175/106143011X12928814444655
Katoh, K., Rozewicki, J., and Yamada, K. D. (2018). MAFFT online service: Multiple sequence alignment, interactive sequence choice and visualization. Brief Bioinform. 20, 1160–1166. doi: 10.1093/bib/bbx108
Kelaher, B. P., Bishop, M. J., Potts, J., Scanes, P., and Skilbeck, G. (2013). Detrital diversity influences estuarine ecosystem performance. Glob. Chang. Biol. 19, 1909–1918. doi: 10.1111/gcb.12162
Khatoon, H., Solanki, P., Naravan, M., Tewari, L., and Rai, J. (2017). Role of microbes in organic carbon decomposition and maintenance of soil ecosystem. Int. J. Chem. Stud. 5, 1648–1656.
Kim, K. S., Cui, X., Lee, D.-S., Sohn, J. H., Yim, J. H., Kim, Y.-C., et al. (2013). Anti-inflammatory effect of neoechinulin A from the marine fungus Eurotium sp. SF-5989 through the suppression of NF-?B and p38 MAPK Pathways in Lipopolysaccharide-Stimulated RAW264.7 Macrophages. Molecules 18, 13245–13259. doi: 10.3390/molecules181113245
Koop, K., Newell, R. C., and Lucas, M. I. (1982). Biodegradation and carbon flow based on kelp (Ecklonia maxima) debris in a sandy beach microcosm. Mar. Ecol. Prog. Ser. 7, 315–326. doi: 10.3354/meps007315
Kothe, E., and Turnau, K. (2018). Editorial: mycorrhizosphere communication: mycorrhizal fungi and endophytic fungus-plant interactions. Front. Microbiol. 9:3015. doi: 10.3389/fmicb.2018.03015
Krause-Jensen, D., and Duarte, C. M. (2016). Substantial role of macroalgae in marine carbon sequestration. Nat. Geosci. 9, 737–742. doi: 10.1038/ngeo2790
Krause-Jensen, D., Lavery, P., Serrano, O., Marba, N., Masque, P., and Duarte, C. M. (2018). Sequestration of macroalgal carbon: the elephant in the blue carbon room. Biol. Let. 14:236. doi: 10.1098/rsbl.2018.0236
Krumhansl, K. A., and Scheibling, R. E. (2012). Production and fate of kelp detritus. Mar. Ecol. Pro. Ser. 497, 281–302. doi: 10.3354/meps09940
Kumar, S., Stecher, G., Li, M., Knyaz, C., and Tamura, K. (2018). MEGA X: Molecular evolutionary genetics analysis across computing platforms. Mol. Biol. Evol. 35, 1547–1549. doi: 10.1093/molbev/msw054
Kusari, S., Hertweck, C., and Spiteller, M. (2012). Chemical ecology of endophytic fungi: Origins of secondary metabolites. Chem. Biol. 19, 792–798. doi: 10.1016/j.chembiol.2012.06.004
Lakshmi, D. S., Trivedi, N., and Reddy, C. R. K. (2017). Synthesis and characterization of seaweed cellulose derived carboxymethyl cellulose. Carbohydr. Polym. 157, 1604–1610. doi: 10.1016/j.carbpol.2016.11.042
Latz, M. A. C., Jensen, B., Collinge, D. B., and Jørgensen, H. J. L. (2018). Endophytic fungi as biocontrol agents: elucidating mechanisms in disease suppression. Plant Ecol. Divers 11, 555–567. doi: 10.1080/17550874.2018.1534146
Leach, J. E., Triplett, L. R., Argueso, C. T., and Trivedi, P. (2017). Communication in the Phytobiome. Cel 169, 587–596. doi: 10.1016/j.cell.2017.04.025
Leonowicz, A., Cho, N. S., Luterek, J., Wilkolazka, A., Wojtas-Wasilewska, M., Matuszewska, A., et al. (2001). Fungal laccase: properties and activity on lignin. Basic Microbiol. 41, 185–127. doi: 10.1002/1521-4028(200107)41:3/4<185::AID-JOBM185>3.0.CO;2-T
Leschine, S. B. (1995). Cellulose degradation in anaerobic environments. Annu. Rev. Microbiol. 49, 399–426. doi: 10.1146/annurev.micro.49.1.399
Li, C., Sarotti, A. M., Yang, B., Turkson, J., and Cao, S. (2017). A new N-methoxypyridone from the co-cultivation of Hawaiian endophytic fungi Camporesia sambuci FT1061 and Epicoccum sorghinum FT1062. Molecules 22:1166. doi: 10.3390/molecules22071166
Lovelock, C. E., Fourqurean, J. W., and Morris, J. T. (2017). Modeled CO2 emissions from coastal wetland transitions to other land uses: tidal marshes, mangrove forests, and seagrass beds. Front. Mar. Sci. 4:143. doi: 10.3389/fmars.2017.00143
Malik, A. A., Chowdhury, S., Schlager, V., Oliver, A., Puissant, J., Vazquez, P. G. M., et al. (2016). Soil fungal: bacterial ratios are linked to altered carbon cycling. Front Microbiol. 7:1247. doi: 10.3389/fmicb.2016.01247
Manganyi, M. C., and Ateba, C. N. (2020). Untapped potentials of endophytic fungi: A review of novel bioactive compounds with biological applications. Microorganisms 8, 1–25. doi: 10.3390/microorganisms8121934
Mannan, S., Fakhru’l-Razi, A., and Alam, M. Z. (2007). Optimization of process parameters for the bioconversion of activated sludge by Penicillium corylophilum, using response surface methodology. J. Environ. Sci. 19, 23–28. doi: 10.1016/S1001-0742(07)60004-7
Mapari, S. A. S., Nielsen, K. F., Larsen, T. O., Frisvad, J. C., Meyer, A. S., and Thrane, U. (2005). Exploring fungal biodiversity for the production of water-soluble pigments as potential natural food colorants. Curr. Opin. Biotechnol. 16, 231–238. doi: 10.1016/j.copbio.2005.03.004
Martinez, A. T., and Ramirez, C. (1978). Penicillium fagi sp. nov., isolated from beech leaves. Mycopathologia 63, 57–59. doi: 10.1007/BF00473161
McLeod, E., Chmura, G. L., Bouillon, S., Salm, R., Björk, M., Duarte, C. M., et al. (2011). A blueprint for blue carbon: toward an improved understanding of the role of vegetated coastal habitats in sequestering CO2. Front. Ecol. Environ. 9:552–560. doi: 10.1890/110004
Mille-Lindblom, C., and Tranvik, L. J. (2003). Antagonism between bacteria and fungi on decomposing aquatic plant litter. Microbiol. Ecol. 45, 173–182. doi: 10.1007/s00248-002-2030-z
Miyauchi, S., Kiss, E., Kuo, A., Drula, E., Kohler, A., Sánchez-Garcia, M., et al. (2020). Large-scale genome sequencing of mycorrhizal fungi provides insights into the early evolution of symbiotic traits. Nat. Commun. 11, 1–17. doi: 10.1038/s41467-020-18795-w
Mohamed, D. J., and Martiny, J. B. H. (2011). Patterns of fungal diversity and composition along a salinity gradient. ISME J. 5, 379–388. doi: 10.1038/ismej.2010.137
Navarro-Meléndez, A. L., and Heil, M. (2014). Symptomless endophytic fungi suppress endogenous levels of salicylic acid and interact with the jasmonate-dependent indirect defense traits of their host, Lima Bean (Phaseolus lunatus). J. Chem. Ecol. 40, 816–825. doi: 10.1007/s10886-014-0477-2
Nercessian, O., Noyes, E., Kalyuzhnaya, M. G., Lidstrom, M. E., and Chistoserdova, L. (2005). Bacterial populations active in metabolism of C1 compounds in the sediment of Lake Washington, a freshwater lake. Appl. Environ. Microbiol. 71, 6885–6899. doi: 10.1128/AEM.71.11.6885-6899.2005
Nicoletti, R., Fiorentino, A., and Scognamiglio, M. (2014). Endophytism of Penicillium Species in Woody Plants. Open Mycol. J. 8, 1–26. doi: 10.2174/1874437001408010001
Noorjahan, A., Aiyamperumal, B., and Anantharaman, P. (2019). Isolation and charecterisation of seaweed endophytic fungi as an efficient phosphate solubiizers. Biosci. Biotechnol. Res. Asia 16, 33–39. doi: 10.13005/bbra/2718
Oliveira, R. C., Nguyen, H. N., Mallmann, C. A., Freitas, R. S., Correa, B., and Rodrigues, D. F. (2018). Influence of environmental factors on tenuazonic acid production by Epicoccum sorghinum: An integrative approach of field and laboratory conditions. Sci. Total Environ. 64, 1132–1138. doi: 10.1016/j.scitotenv.2018.05.293
Ortega, A., Geraldi, N. R., and Duarte, C. M. (2020). Environmental DNA identifies marine macrophyte contributions to Blue Carbon sediments. Limnol. Oceanogr. 65, 3139–3149. doi: 10.1002/lno.11579
Ortega-Arbulú, A. S., Pichler, M., Vuillemin, A., and Orsi, W. D. (2019). Effects of organic matter and low oxygen on the mycobenthos in a coastal lagoon. Environ. Microbiol. 1, 374–388. doi: 10.1111/1462-2920.14469
Parthasarathy, R., Chandrika, M., Yashavantha, R., Kamalraj, S., Jayabaskaran, C., and Pugazhendhi, A. (2020). Molecular profiling of marine endophytic fungi from green algae: assessment of antibacterial and anticancer activities. Process Biochem. 96, 11–20. doi: 10.1016/j.procbio.2020.05.012
Passerini, M. D., Cunha-Santino, M. B., and Bianchini, I. (2016). Oxygen availability and temperature as driving forces for decomposition of aquatic macrophytes. Aquat. Bot. 130, 1–10. doi: 10.1016/j.aquabot.2015.12.003
Perkins, A. K., Ganzert, L., Rojas-Jimenez, K., Fonvielle, J., Hose, G. C., and Grossart, H.-P. (2019). Highly diverse fungal communities in carbon-rich aquifers of two contrasting lakes in Northeast Germany. Fungal Ecol. 41, 116–125. doi: 10.1016/j.funeco.2019.04.004
Peter, S., Shen, Y., Kaiser, K., Benner, R., and Durisch-Kaiser, E. (2012). Bioavailability and diagenetic state of dissolved organic matter in riparian groundwater. J. Geophys. Res. Biogeosci. 117:72. doi: 10.1029/2012JG002072
Phonrod, U., Aramsirirujiwet, Y., Sri-indrasutdhi, V., Ueapattanakit, J., Chuaseeharonnachai, C., and Suwannarit, P. (2016). Biodiversity of fungi in seawater and sediment from mangrove forest at Andaman coastal research station for development, Ranong province. Asia Pac. J. Sci. Technol. 21, 77–85.
Poveda, J., Abril-Urias, P., and Escobar, C. (2020). Biological control of plant-parasitic nematodes by filamentous fungi inducers of resistance: trichoderma, mycorrhizal and endophytic fungi. Front. Microbiol. 9:992. doi: 10.3389/fmicb.2020.00992
Prasad, D., Arun, S., Murugesan, M., Padmanaban, S., Satyanarayanan, R. S., Berchmans, S., et al. (2007). Direct electron transfer with yeast cells and construction of a mediatorless microbial fuel cell. Biosens. Bioelectron. 22, 2604–2610. doi: 10.1016/j.bios.2006.10.028
Price, M. N., Dehal, P. S., and Arkin, A. P. (2010). FastTree 2 - Approximately maximum-likelihood trees for large alignments. PLoS One 5:e9490. doi: 10.1371/journal.pone.0009490
Promputtha, I., Hyde, K. D., McKenzie, E. H. C., Peberdy, J. F., and Lumyong, S. (2010). Can leaf degrading enzymes provide evidence that endophytic fungi becoming saprobes? Fungal Diver 41, 89–99. doi: 10.1007/s13225-010-0024-6
Queirós, A. M., Stephens, N., Widdicombe, S., Tait, K., McCoy, S. J., Ingels, J., et al. (2019). Connected macroalgal-sediment systems: blue carbon and food webs in the deep coastal ocean. Ecol. Monogr. 89:e01366. doi: 10.1002/ecm.1366
Raghukumar, S. (2004). The role of fungi in marine detrital processes, in marine microbiology: facets and opportunities. Biol. Oceanogr. Div. 403, 91–101.
Rai, M., Rathod, D., Agarkar, G., Dar, M., Brestic, M., Pastore, G. M., et al. (2014). Fungal growth promotor endophytes: a pragmatic approach towards sustainable food and agriculture. Symbiosis 62, 63–79. doi: 10.1007/s13199-014-0273-3
Rana, K. L., Kour, D., Sheikh, I., Dhiman, A., Yadav, N., Yadav, A. N., et al. (2019). “Endophytic Fungi: Biodiversity, Ecological Significance, and Potential Industrial Applications,” in Recent Advancement in White Biotechnology Through Fungi. Fungal Biology, eds A. Yadav, S. Mishra, S. Singh, and A. Gupta (New York, NY: Springer), 1–62. doi: 10.1007/978-3-030-10480-1_1
Rangel-Porras, R. A., Diaz-Pérez, S. P., Mendoza-Hernández, J. M., Romo-Rodriguez, P., Alejandre-Castaneda, V., Valle-Maldonado, M. I., et al. (2019). Alcoholdehydrogenase 1 participates in the Crabtree effect and connects fermentative and oxidative metabolism in the Zygomycete Mucor circinelloides. J. Microbiol. 57, 606–617. doi: 10.1007/s12275-019-8680-z
Rani, B., Kumar, V., Singh, J., Bisht, S., Teotia, P., Sharma, S., et al. (2014). Bioremediation of dyes by fungi isolated from contaminated dye effluent sites for bio-usability. Braz. J. Microbiol. 45, 1055–1063. doi: 10.1590/S1517-83822014000300039
Rashmi, M. (2019). A worldwide list of endophytic fungi with notes on ecology and diversity. Mycosphere 10:19. doi: 10.5943/mycosphere/10/1/19
Raven, J. (2018). Blue carbon: past, present and future, with emphasis on macroalgae. Biol. Lett. 14:336. doi: 10.1098/rsbl.2018.0336
Rose, A. L., Godrant, A., Furnas, M., and Waite, T. D. (2010). Dynamics of nonphotochemical superoxide production and decay in the Great Barrier Reef lagoon. Limnol. Oceanogr. 55:1521. doi: 10.4319/lo.2010.55.4.1521
Sakai, T., Ishizuka, K., and Kato, I. (2003). Isolation and characterization of a fucoidan-degrading marine bacterium. Mar. Biotechnol. 6, 335–346. doi: 10.1007/s10126-002-0118-6
Sarasan, M., Job, N., Puthumana, J., Ravinesh, R., Prabhakaran, M. P., Lathika, C. T., et al. (2020). Exploration and profiling of hidden endophytic mycota of marine macroalgae with potential drug leads. FEMS Microbiol. Lett. 367:fnaa078. doi: 10.1093/femsle/fnaa078
Saroj, P. P. M., and Narasimhulu, K. (2018). Characterization of thermophilic fungi producing extracellular lignocellulolytic enzymes for lignocellulosic hydrolysis under solid-state fermentation. Bioresour. Bioprocess 5, 1–14. doi: 10.1186/s40643-018-0216-6
Sazci, A., Erenler, K., and Radford, A. (1986). Detection of cellulolytic fungi by using Congo red as an indicator: a comparative study with the dinitrosalicyclic acid reagent method. J. Appl. Bacteriol. 61, 559–562. doi: 10.1111/j.1365-2672.1986.tb01729.x
Sen, S. K., Raut, S., Bandyopadhyay, P., and Raut, S. (2016). Fungal decolouration and degradation of azo dyes: a review. Fungal Biol. Rev. 30, 112–133. doi: 10.1016/j.fbr.2016.06.003
Shao, C., Shao, Q., Wang, X., Ling, J., Guo, X., Ning, Y., et al. (2020). Study on cellulose degradation induced by hydroxyl radical with cellobiose as a model using GC–MS, ReaxFF simulation and DFT computation. Carbohydr. Polym. 233:115677. doi: 10.1016/j.carbpol.2019.115677
Sharifi, R., and Ryu, C.-M. (2021). Social networking in crop plants: Wired and wireless cross-plant communications. Plant Cell Environ. 44, 1095–1110. doi: 10.1111/pce.13966
Shin, K. S., Young, H. K., and Lim, J. S. (2005). Purification and characterization of manganese peroxidase of the white-rot fungus Irpex lacteus. J. Microbiol. 43, 503–509.
Shipunov, A., Newcombe, G., Raghavendra, A. K. H., and Anderson, C. L. (2008). Hidden diversity of endophytic fungi in an invasive plant. Am. J. Bot. 95, 1096–1108. doi: 10.3732/ajb.0800024
Sichert, A., Corzett, C. H., Schechter, M. S., Unfried, F., Markert, S., Becher, D., et al. (2020). Verrucomicrobia use hundreds of enzymes to digest the algal polysaccharide fucoidan. Nat. Microbiol. 5, 1026–1039. doi: 10.1038/s41564-020-0720-2
Silchenko, A. S., Kusaykin, M. I., Kurilenko, V. V., Zakharenko, A. M., Isakov, V. V., Zaporozhets, T. S., et al. (2013). Hydrolysis of fucoidan by fucoidanase isolated from the marine bacterium, formosa algae. Mar. Drugs. 11, 2413–2430. doi: 10.3390/md11072413
Sing, N. N., Husaini, A., Zulkharnain, A., and Roslan, H. A. (2017). Decolourisation capabilities of ligninolytic enzymes produced by marasmius cladophyllus UMAS MS8 on remazol brilliant blue r and other azo dyes. Bio. Med. Res. Int. 2017:1325754. doi: 10.1155/2017/1325754
Ślesak, I., Kula, M., Ślesak, H., Miszalki, Z., and Strzalka, K. (2019). How to define obligatory anaerobiosis? an evolutionary view on the antioxidant response system and the early stages of the evolution of life on Earth. Free Radical Biol. Med. 140, 61–73. doi: 10.18388/abp.2007_3267
Smirnoff, N., and Arnaud, D. (2019). Hydrogen peroxide metabolism and functions in plants. New Phytol. 221, 1197–1214. doi: 10.1111/nph.15488
Sun, Z. Z., Ji, B.-W., Zheng, N., Wang, M., Cao, Y., Wan, L., et al. (2021). Phylogenetic distribution of polysaccharide-degrading enzymes in marine bacteria. Front. Microbiol. 6:584. doi: 10.3389/fmicb.2021.658620
Suryanarayanan, T. S. (2012). Fungal endosymbionts of seaweeds. Prog. Mol. Subcell Biol. 53, 53–69. doi: 10.1007/978-3-642-23342-5_3
Suryanarayanan, T. S., Venkatachalam, A., Thirunavukkarasu, N., Ravishankar, J. P., Dobbie, M., and Geetha, V. (2010). Internal mycobiota of marine macroalgae from the Tamilnadu coast: Distribution, diversity and biotechnological potential. Bot. Mar. 53, 457–468. doi: 10.1515/bot.2010.045
Tedersoo, L., Bahram, M., Pölme, S., Köljalg, U., Zorou, N. S., Wijesundera, R., et al. (2014). Global diversity and geography of soil fungi. Sci 346:1256688. doi: 10.1126/science.1256688
Teixeira, T. R., Santos, G. S., dos, Armstrong, L., Colepicolo, P., and Debonsi, H. M. (2019). Antitumor potential of seaweed derived-endophytic fungi. Antibiotics 8:205. doi: 10.3390/antibiotics8040205
Tiwari, P., and Bae, H. (2020). Horizontal gene transfer and endophytes: An implication for the acquisition of novel traits. Plants 9:305. doi: 10.3390/plants9030305
Treseder, K. K., and Lennon, J. T. (2015). Fungal traits that drive ecosystem dynamics on land. Microbiol. Mol. Biol. Rev. 79, 243–262. doi: 10.1128/MMBR.00001-15
Trevathan-Tackett, S. M., Kelleway, J., Macreadie, P. I., Beardall, J., Ralph, P., and Bellgrove, A. (2015). Comparison of marine macrophytes for their contributions to blue carbon sequestration. Ecol 96, 3043–3057. doi: 10.1890/15-0149.1.sm
Van der Wal, A., Geydan, T. D., Kuyper, T. W., and De Boer, W. (2013). A thready affair: Linking Fungal diversity and community dynamics to terrestrial decomposition processes. FEMS Microbiol. Rev. 37, 477–494. doi: 10.1111/1574-6976.12001
Van Erk, M. R., Meier, D. V., Ferdelman, T., Harder, J., Bussmann, I., and De Beer, D. (2020). Kelp deposition changes mineralization pathways and microbial communities in a sandy beach. Limnol. Oceanogr. 65, 3066–3084. doi: 10.1002/lno.11574
Vellanki, S., Navarro-Mendoza, M. I., Garcia, A., Murcia, L., Perez-Arques, C., Garre, V., et al. (2018). Mucor circinelloides: growth, maintenance, and genetic manipulation. Curr. Protoc. Microbiol. 49:e53. doi: 10.1002/cpmc.53
Wang, L., and D’Odorico, P. (2013). Decomposition and Mineralization. Reference Module in Earth Systems and Environmental Sciences. Encyclopedia of Ecology, 2nd Edn. Amsterdam: Elsevier, 280–285. doi: 10.1016/B978-0-12-409548-9.00688-6
Wang, X. C., Wang, X.-C., Zhuang, W.-Y., Cheng, X.-H., and Zhao, P. (2016). A new species of Talaromyces (Trichocomaceae) from the Xisha Islands, Hainan, China. Phytotaxa 267, 187–200. doi: 10.11646/phytotaxa.267.3.2
Watson, A. J. (2016). Oceans on the edge of anoxia. Sci. Adv. 354, 1529–1530. doi: 10.1126/science.aaj2321
Weinberger, S., Beyer, R., Schüller, C., Strauss, J., Pellis, A., Ribitsch, D., et al. (2020). High throughput screening for new fungal polyester hydrolyzing enzymes. Front. Microbiol. 9:554. doi: 10.3389/fmicb.2020.00554
White, T. J., Bruns, T., Lee, S., and Taylor, J. (1990). Amplification and direct sequencing of fungal ribosomal RNA genes for phylogenetics, in PCR Protocols’, PCR protocols: a guide to methods and applications.1990, 315–322. doi: 10.1016/B978-0-12-372180-8.50042-1
Wolfe, E. R., and Ballhorn, D. J. (2020). Do foliar endophytes matter in litter decomposition? Microorganisms 8:446. doi: 10.3390/microorganisms8030446
Wood, T. M., and Garcia-Campayo, V. (1990). Enzymology of cellulose degradation. Biodegradation 1, 147–161. doi: 10.1007/BF00058833
Worden, A. Z., Follows, M. J., Giovannoni, S. J., Wilken, S., Zimmerman, A. E., and Keeling, P. J. (2015). Rethinking the marine carbon cycle: factoring in the multifarious lifestyles of microbes. Sci. Adv. 347:1257594. doi: 10.1126/science.1257594
Wu, L. S., Hu, C.-L., Han, T., Zheng, C.-J., Ma, X.-Q., Rahman, K., et al. (2013). Cytotoxic metabolites from Perenniporia tephropora, an endophytic fungus from Taxus chinensis var. mairei. Appl. Microbiol. Biotechnol. 97, 305–315. doi: 10.1007/s00253-012-4189-7
Xia, C., Zhang, J., Zhang, W., and Hu, B. (2011). A new cultivation method for microbial oil production: Cell pelletization and lipid accumulation by Mucor circinelloides. Biotechnol. Biofuels. 15:15. doi: 10.1186/1754-6834-4-15
Xu, H. B., Tsukuda, M., Takahara, Y., Sato, T., Gu, J. D., and Katayama, Y. (2018). Lithoautotrophical oxidation of elemental sulfur by fungi including Fusarium solani isolated from sandstone Angkor temples. Int. Biodeterior Biodegradation. 126, 95–102. doi: 10.1016/j.ibiod.2017.10.005
Yadav, R., and Reddy, M. S. (2013). Characterization of thermostable and alkali-tolerant cellulase from endophytic fungus Bartalinia sp. Biores. Technol. 101, 8798–8806.
Yan, L., Zhu, J., Zhao, X., Shi, J., Jiang, C., and Shao, D. (2019). Beneficial effects of endophytic fungi colonization on plants. Appl. Microbiol. Biotechnol. 103, 3327–3340. doi: 10.1007/s00253-019-09713-2
Yeddou-Mezenner, N. (2010). Kinetics and mechanism of dye biosorption onto an untreated antibiotic waste. Desalination 262, 251–259. doi: 10.1016/j.desal.2010.06.023
You, Y.-H., Lee, M.-C., and Kim, J. G. (2014). Endophytic fungal diversity isolated from the root of halophytes in taean peninsula. Kor. J. Mycol. 42, 269–275. doi: 10.4489/KJM.2014.42.4.269
Zain Ul Arifeen, M., Ma, Y. N., Xue, Y. R., and Liu, C. H. (2019). Deep-sea fungi could be the new arsenal for bioactive molecules. Mar. Drugs. 18:9. doi: 10.3390/md18010009
Zhang, Y. Z., Sundaram, S. T., Sharma, A., and Brodman, B. W. (1997). Biodegradation of glyceryl trinitrate by Penicillium corylophilum Dierckx. Appl. Environ. Microbiol. 63, 1712–1714. doi: 10.1128/aem.63.5.1712-1714.1997
Zhao, P., Xia, W., Lei, C., Omer, S. H. S., Zhang, X., Zhuang, Y., et al. (2016). Isolation, identification and physiological activity of endophytes from the roots of Vitis vinifera. Nanosci. Nanotechnol. Lett. 8, 532–538. doi: 10.1166/nnl.2016.2164
Zuccaro, A., Schoch, C. L., Spatafora, J. W., Kohlmeyer, J., Draeger, S., and Mitchell, J. I. (2008). Detection and identification of fungi intimately associated with the brown seaweed Fucus serratus. Appl. Environ. Microbiol. 74, 931–941. doi: 10.1128/AEM.01158-07
Keywords: kelp, fungi, endophytes, carbon cycling, extracellular enzymes, cellulose, polymeric organic matter
Citation: Perkins AK, Rose AL, Grossart H-P, Rojas-Jimenez K, Barroso Prescott SK and Oakes JM (2021) Oxic and Anoxic Organic Polymer Degradation Potential of Endophytic Fungi From the Marine Macroalga, Ecklonia radiata. Front. Microbiol. 12:726138. doi: 10.3389/fmicb.2021.726138
Received: 16 June 2021; Accepted: 31 August 2021;
Published: 18 October 2021.
Edited by:
Michael Yu Roleda, University of the Philippines Diliman, PhilippinesReviewed by:
Brandon T. Hassett, Arctic University of Norway, NorwayCopyright © 2021 Perkins, Rose, Grossart, Rojas-Jimenez, Barroso Prescott and Oakes. This is an open-access article distributed under the terms of the Creative Commons Attribution License (CC BY). The use, distribution or reproduction in other forums is permitted, provided the original author(s) and the copyright owner(s) are credited and that the original publication in this journal is cited, in accordance with accepted academic practice. No use, distribution or reproduction is permitted which does not comply with these terms.
*Correspondence: Hans-Peter Grossart, aGdyb3NzYXJ0QGlnYi1iZXJsaW4uZGU=
†These authors have contributed equally to this work and share senior authorship
‡These authors have contributed equally to this work and share last authorship
Disclaimer: All claims expressed in this article are solely those of the authors and do not necessarily represent those of their affiliated organizations, or those of the publisher, the editors and the reviewers. Any product that may be evaluated in this article or claim that may be made by its manufacturer is not guaranteed or endorsed by the publisher.
Research integrity at Frontiers
Learn more about the work of our research integrity team to safeguard the quality of each article we publish.