- 1Department of Chemistry, Bioscience and Environmental Engineering, Faculty of Science and Technology, University of Stavanger, Stavanger, Norway
- 2Department of Electrical Engineering and Computer Science, Faculty of Science and Technology, University of Stavanger, Stavanger, Norway
- 3Department of Chemistry and Research Centre, Aditanar College of Arts and Science, Tiruchendur, India
- 4Department of Natural Resource Management, College of Forestry, Banda University of Agricultural and Technology, Banda, India
This paper reviews current knowledge on sources, spread and removal mechanisms of antibiotic resistance genes (ARGs) in microbial communities of wastewaters, treatment plants and downstream recipients. Antibiotic is the most important tool to cure bacterial infections in humans and animals. The over- and misuse of antibiotics have played a major role in the development, spread, and prevalence of antibiotic resistance (AR) in the microbiomes of humans and animals, and microbial ecosystems worldwide. AR can be transferred and spread amongst bacteria via intra- and interspecies horizontal gene transfer (HGT). Wastewater treatment plants (WWTPs) receive wastewater containing an enormous variety of pollutants, including antibiotics, and chemicals from different sources. They contain large and diverse communities of microorganisms and provide a favorable environment for the spread and reproduction of AR. Existing WWTPs are not designed to remove micropollutants, antibiotic resistant bacteria (ARB) and ARGs, which therefore remain present in the effluent. Studies have shown that raw and treated wastewaters carry a higher amount of ARB in comparison to surface water, and such reports have led to further studies on more advanced treatment processes. This review summarizes what is known about AR removal efficiencies of different wastewater treatment methods, and it shows the variations among different methods. Results vary, but the trend is that conventional activated sludge treatment, with aerobic and/or anaerobic reactors alone or in series, followed by advanced post treatment methods like UV, ozonation, and oxidation removes considerably more ARGs and ARB than activated sludge treatment alone. In addition to AR levels in treated wastewater, it examines AR levels in biosolids, settled by-product from wastewater treatment, and discusses AR removal efficiency of different biosolids treatment procedures. Finally, it puts forward key-points and suggestions for dealing with and preventing further increase of AR in WWTPs and other aquatic environments, together with a discussion on the use of mathematical models to quantify and simulate the spread of ARGs in WWTPs. Mathematical models already play a role in the analysis and development of WWTPs, but they do not consider AR and challenges remain before models can be used to reliably study the dynamics and reduction of AR in such systems.
Introduction
Antibiotic substances are by far the most powerful tools available for the treatment of infectious diseases by inhibition of bacterial cell growth. In addition to being used for the treatment of infections in human patients and farm animals, antibiotics are also routinely given to healthy farm animals to promote growth and proactively prevent disease outbreaks. Antibiotic resistance (AR) is the ability of bacteria to overcome and resist exposure to antibiotic substances, this is made possible by the acquisition of antibiotic resistance genes (ARGs) (Davison et al., 2000; Wright, 2010). Extensive use of antibiotics since the successful purification and mass production of penicillin in the middle of the twentieth century until today has led to an increase in antibiotic resistance, compromising the effectiveness of antibiotics (Davies and Davies, 2010).
Antibiotic resistance is a global and challenging issue (Walsh, 2003; Deurenberg and Stobberingh, 2008; Livermore, 2012). The risk it poses needs to be tackled in a context that combines environmental, and human health, which focuses on the mechanisms that drive biological (growth and exchange) and physiochemical (transport and conversion) spread. Considering human health issues like AR in a context that combines human, animal and environmental factors is the essence of the One Health initiative’s perspective, endorsed by the World Health Organisation (WHO) (One Health Initiative, 2020) and AR has large implications for half a dozen of the United Nation’s (UN’s) sustainable development goals (WHO, 2020). Part of the issue is to understand how ARGs spread in different environments [wastewater, wastewater treatment plants (WWTPs), soil, and receiving aquatic eco-system] to prevent the spread existing and the development of new ARGs (Brooks et al., 2008).
For more than 100 years, the Activated Sludge Process has been and still is among the most widespread wastewater treatment technologies used for the removal of key pollutants from municipal wastewater (Stensel and Makinia, 2014; van Loosdrecht and Brdjanovic, 2014). By bacterial uptake and metabolic conversions of organics and nutrients, cellular growth provides for an auto-catalytical removal process which is further enhanced by settling and recirculation of active biomass as originally proposed by Ardern and Lockett (1914). While bacterial densities in wastewater are normally in the range of 105–108 cells per ml (Tchobanoglous et al., 2014), the enhancement of biomass in modern biological WWTPs increases the bacterial density in the bioreactors by 3 orders of magnitude and selection by sedimentation results in dense bacterial aggregates. Additionally, depending on operating conditions and temperature, there can be very high material turnover (up to 90%) and much higher specific heterotrophic growth rates (up to 13.2 day–1) in WWTPs bioreactors than in natural water systems. In WWTP bioreactors, microbial diversity and interactions are ubiquitous and frequent (Daims et al., 2006; Nielsen and McMahon, 2014). High abundance, density, diversity, activity, and interactions in the activated sludge bioreactors would suggest an increased rate of gene transfer, including horizontal and vertical exchange of ARG. Mechanisms and rates at which exchange occur in the microbiome of these systems are now under intense study, and resistomes (all ARG’s in a microbial community) of activated sludge systems are currently being mapped (Manaia et al., 2018).
Established effluent standards set the quality of WWTP effluents based on environmental effect parameters such as the chemical and biochemical oxygen demand, the amount of suspended solids, total nitrogen, number of coliform bacteria, etc. (Directive 91/271/Eec, 1991). It is not known how, or whether at all, these parameters indicate the prevalence of antibiotic resistant bacteria (ARB) and ARGs. Lately, more attention has been paid to examine detection and elimination techniques for ARB and ARGs, in addition to removal techniques for other micropollutants like detergents, pesticides, pharmaceuticals, and personal care products (Łuczkiewicz et al., 2010; Luo et al., 2014). Some WWTPs use extra disinfection units at the end of the biological treatment process, which include chlorination, UV radiation and ozonation, or quaternary advanced treatment techniques such as advanced oxidation processes (AOPs) or membrane filtration. Such unit processes can as this review will show reduce the number of ARB and possibly also ARGs in the WWTP effluent but are costly to operate and may not be as effective as observed in laboratory studies (Auerbach et al., 2007; Zhang et al., 2015, 2016a; Zhuang et al., 2015). Biological removal of organic material from wastewater is linked to the fast growth of microorganisms in the WWTP, and since the biomass builds up some is continuously discarded as excess biological sludge. ARB and ARGs that are present in the biomass of the reactor will also be present in the sludge, therefore further treatment processes of excess sludge need to be considered. We will in the last part of section four of this review go through the current knowledge of how effective the different sludge treatment methods are able in reducing ARB and ARGs.
This review will present the major groups of antibiotics, the major groups of mechanisms for antibiotic resistance in bacteria, and the general bacterial mechanisms for genetic exchange, but only briefly as other reviews already have covered this in general (Wright, 2010, 2011; Pazda et al., 2019; Zhu et al., 2021) and in the context of wastewater treatment. More space is instead given to go through what is known about which wastewater sources show the high occurrence of antibiotic resistance, how antibiotic resistance persists and spreads through WWTPs, and what contributes to this persistence. Previous works in the literature already focused on complete lists of every specific type of ARGs that have been found in WWTPs (Pazda et al., 2019), and on the strength and weaknesses of different methods used to measure and analyze ARB and ARGs content in wastewater (Manaia et al., 2018). Therefore, this review will focus on documenting what is known about the removal efficiency of different treatment processes or technologies, i.e., what are the reported elimination efficiencies of ARGs and ARB for different treatment technologies for both wastewater and sludge, and whether the reported results are consistent. Moreover, in this work special efforts have been put into gathering and reviewing results from studies of elimination of ARGs and ARB in different sludge and biosolids treatment processes, as this has been more or less overlooked in other reviews (Barancheshme and Munir, 2018; Pazda et al., 2019; Bairán et al., 2020; Zhu et al., 2021).
In essence, this systematic review aims to describe the factors that affect the persistence and spread of antibiotic resistance in wastewater treatment and to evaluate current and emerging treatment technologies. For completeness this review documents removal efficiencies for antibiotic substances for different treatment technologies, but it does not aim to discuss the pathways and mechanisms for the breakdown of these substances at length, which has been addressed in a recent review by Zhu et al. (2021). Additionally, this review will also discuss how mathematical models can be used to better understand the dynamics of antibiotic resistance spread in WWTPs. It has been suggested that mathematical modeling can help to quantify and simulate the spread of ARGs in WWTPs, but as this review will show only a few models have been proposed and even fewer have been sufficiently parameterized and validated. It will discuss why, and which challenges remain to be tackled before mathematical models can be used to their full potential. Finally, this review concludes with future directions and some key points that should be prioritized for improving the current state of antibiotic resistance in WWTPs.
Antibiotic Resistance: Mechanisms, Sources, and Transfer
Antibiotics are classified into five major groups, according to their mode of action (Figure 1): (i) Cell wall synthesis inhibition (vancomycin, cephlosporins, β–lactams, bacitracin); (ii) Protein synthesis inhibition (aminoglycosides, chloramphenicol, tetracycline, linezolid); (iii) Nucleic acid synthesis inhibition (rifampin, metronidazole, quinolones, fluoroquinolones); (iv) Antimetabolites (trimethoprim, dapsone, sulphonamide) and; (v) Cell membrane disintegration (polymyxin, daptomycin). Note that some sources use a coarser division into only four groups (Calderón and Sabundayo, 2007; Kapoor et al., 2017) whereas other use a bit finer division into six (Wanger et al., 2017).
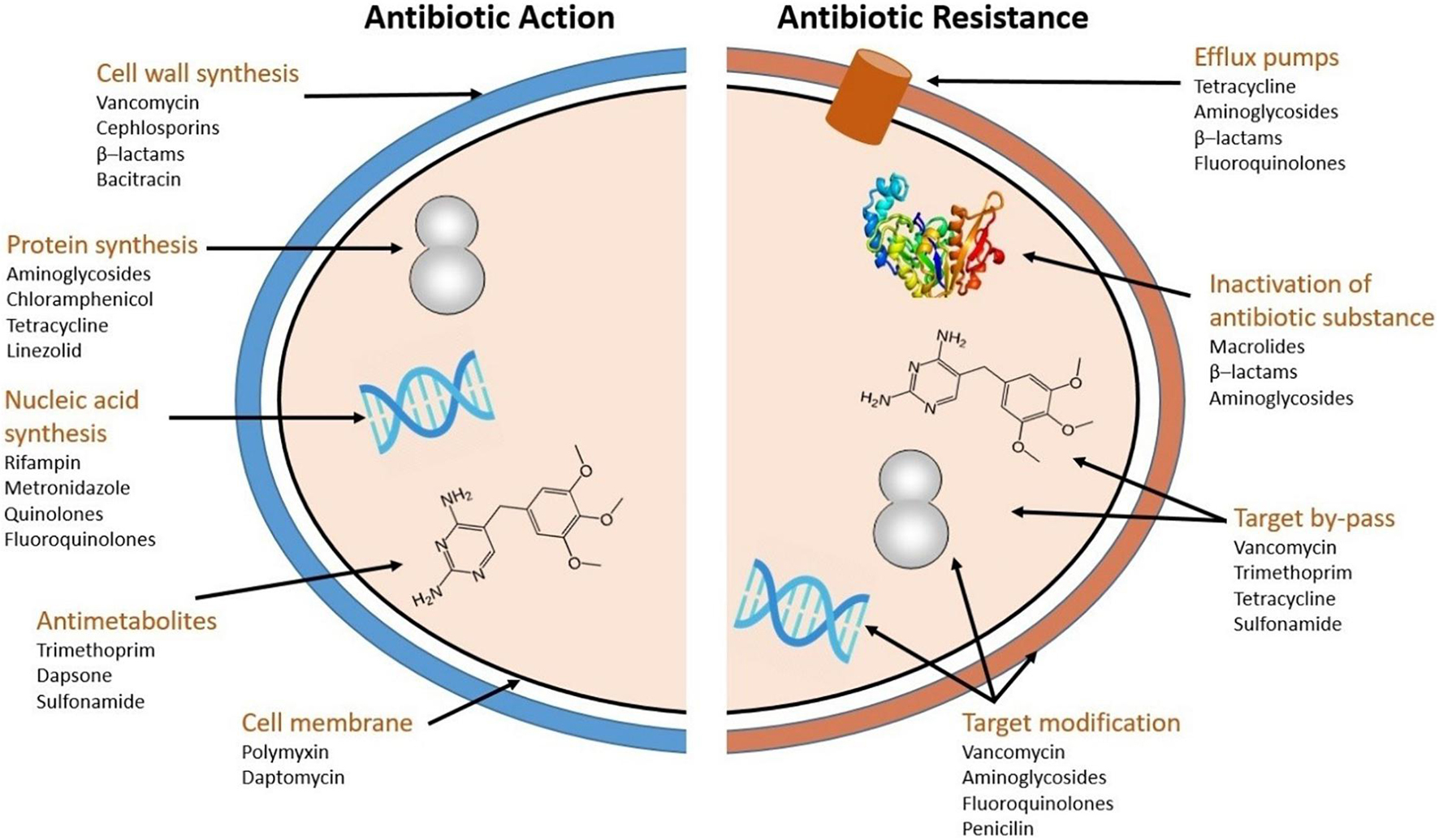
Figure 1. Antibiotic action and resistance mechanisms (adapted from Wright, 2010).
Bacteria have developed four main types of resistance mechanisms against antibiotics (Figure 1; Zhang et al., 2009; Wright, 2010): (i) Efflux pumps, which effectively excrete antibiotics from the cell (Wright, 2011). There are five efflux protein families: ATP-binding cassette (ABC), multidrug and toxic compound extrusion (MATE), major facilitators (MFs), resistance nodulation cell division (RND), and small multidrug resistance (SMR) (Nishino and Yamaguchi, 2001). (ii) Inactivation of antibiotics occurs when the activity of the antibiotic substance is directly hindered by hydrolysis, or by conversion of functional groups etc. (Wright, 2005; Diaz et al., 2014). (iii) Target by-pass: strategies for target by-pass includes creating new pathways to circumvent the originally targeted enzyme, overproduction of the target compound (Munita and Arias, 2016), structural changes in the cell wall (Vila et al., 2007), and prevention of the antibiotic to bind to its target (Wright, 2010). (iv) Target modification: occurs through modification of the antibiotic targets themselves (Wright, 2010). Multiple types of resistance mechanisms may simultaneously confer resistance against the same family of antibiotics (de Sousa Oliveira et al., 2016). Conversely, one type of resistance mechanism can also confer resistance against more than one type of antibiotics.
Wastewater from hospitals and wastewater and waste from animal husbandry together with runoff from manure amended fields are essential ARB and ARGs sources in aquatic ecosystems (Marti and Balcazar, 2013). Hospital wastewaters have especially been shown to contain many ARGs (Zhang et al., 2009; Rowe et al., 2017). One of the first reports dates back to the early 1970s, Grabow and Prozesky (1973) studied the presence of resistant coliforms in hospital wastewater in Pietermaritzburg in South Africa. They found that 26% of coliform bacteria in hospital wastewater had transferable resistance while only 4% of coliform bacteria in municipal wastewater had transferable resistance (Grabow and Prozesky, 1973). The same trend is seen today. Based on several studies done in Europe and Asia, the total ARGs and ARB concentrations in hospital wastewater were 2–9 orders of magnitude higher than municipal wastewater (Li et al., 2015; Lamba et al., 2017; Hutinel et al., 2019). Rowe et al. (2017) showed that the normalized abundance of ARGs in hospital wastewater samples from the Cambridge University Hospitals was 9-folds greater than in samples collected from the effluent lagoon of the University of Cambridge dairy farm and 34-folds greater than in samples from the River Cam source water, which served as background samples for the environment. Another detailed study of wastewater from three different hospitals in Romania showed the presence of genes encoding for resistance for tetracyclines, aminoglycosides, chloramphenicol, β-lactams, sulphonamides, quaternary ammonium, and macrolide-lincosamide-streptogramin B antibiotics with abundance levels in as high ranges as 0.01–0.1 copies per 16S rRNA gene copies measured by qPCR (Szekeres et al., 2017). Moreover, in a recent review by Hassoun-Kheir et al. (2020), 37 studies on the occurrence of AR in hospital wastewaters were examined. The review found that 30 (81%) of the studies reported that hospital wastewater contains higher amounts of AR than community wastewater. Furthermore, in a subset of studies where the impact of hospital wastewater on the dissemination of AR in the environment was considered, 25 out of 32 (78%) studies held that hospital wastewaters had an important role as a source of AR to the environment.
Apart from the vertical inheritance, antibiotic resistance can be obtained in two ways, through mutation or by horizontal gene transfer (HGT) (Jury et al., 2010). The latter is the most concerning regarding the spread of antibiotic resistance in WWTPs since ARGs can potentially be transferred between organisms effectively and much faster than resistance development through mutations. HGT is a non-reproductive intra- and inter-species transfer of genetic information by means of mobile genetic elements (MGE), such as plasmids and transposons (Barlow, 2009; Huang et al., 2017a). The movement of genes from chromosomes to and between MGEs are mostly facilitated by integrons (Mazel, 2006; Davies and Davies, 2010; Gillings, 2014). There are three different HGT mechanisms for the spread of MGEs. (i) Conjugation: transfer mechanism that requires cell-to-cell contact, where a recipient bacterium acquires genetic material from a donor bacterium, usually in the form of a plasmid (Madigan et al., 2006; Figure 2). (ii) Transformation: intra- and inter-species exchange of genetic information by uptake of, free extracellular suspended DNA, which can only be received by a competent bacterium. Following uptake and translocation to the cytoplasm, it is incorporated into the recipient’s chromosome or into a plasmid (Madigan et al., 2006; Heuer and Smalla, 2007). Finally; (iii) Transduction: involves bacteriophages that transport different types of genetic elements from their host to the receiver (Cano and Colomé, 1988; Snyder and Champness, 2007; Modi et al., 2013), whereupon this is incorporated into the genome of the new host by recombination (Figure 2). There are two types of this mode of transfer, namely generalized and specialized transductions. In generalized transduction, only a segment of bacterial DNA is randomly packed into the bacteriophage head, and bacterial host DNA becomes a part of the DNA of the phage whereas in specialized transduction, both phage and specific bacterial DNA are packed into the head (Chiang et al., 2019). Transduction may also occur via gene transfer agents (GTAs), which are DNA carrying structures that resemble bacteriophages, but which do not self-replicate. Although GTAs exact impact has not yet been determined, their potential to act as carriers of resistance in the environment continues their attention (von Wintersdorff et al., 2016).
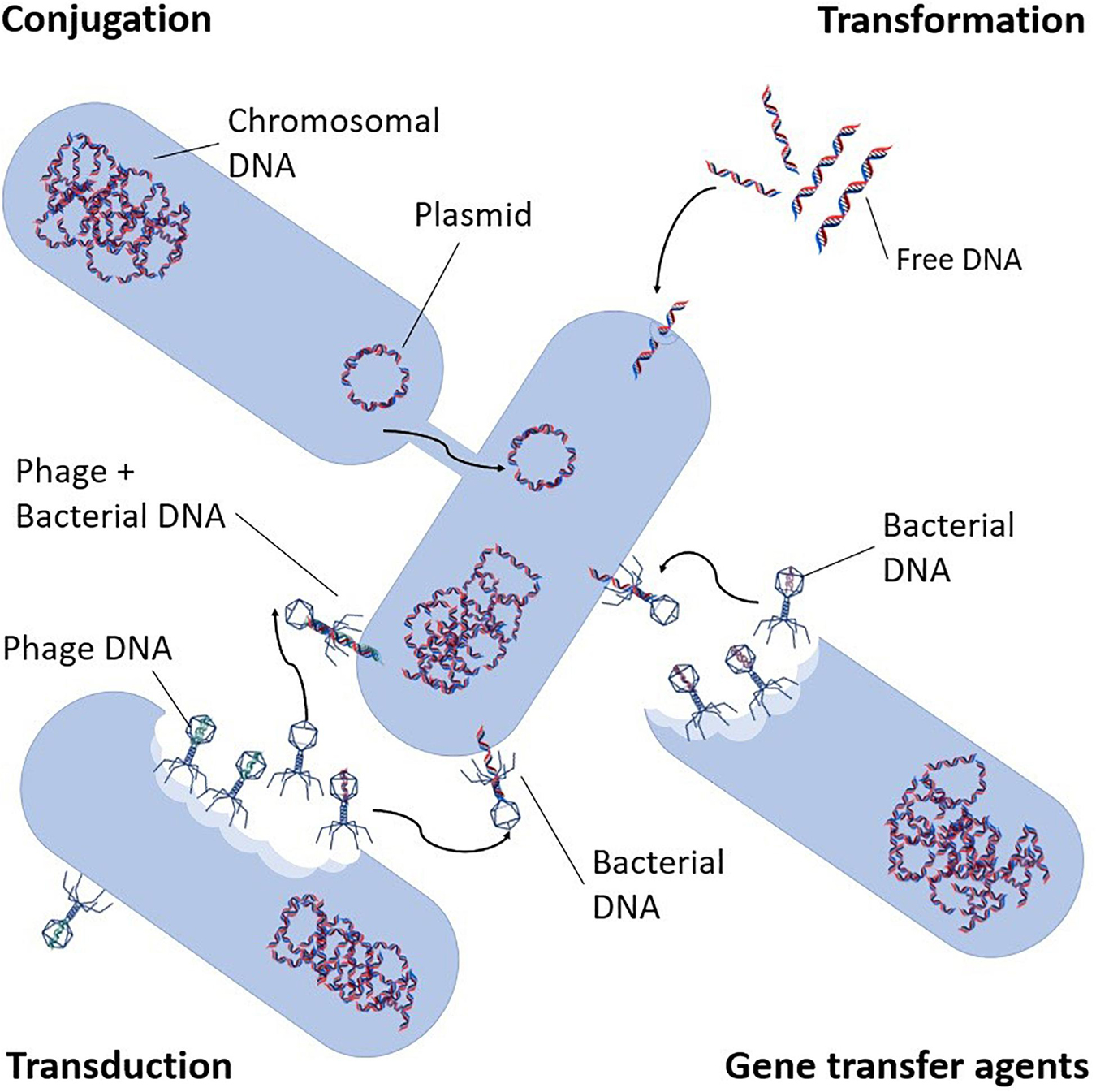
Figure 2. Antibiotic resistance transport mechanisms (adapted from von Wintersdorff et al., 2016).
Occurrence and Spread of Antibiotic Resistance Genes in Wastewater Treatment Plants
Although antibiotic resistance (AR) occurs naturally at low levels in most ecosystems, the occurrence of ARB and ARGs at high levels is associated with anthropogenic activities. Table 1 shows an overview of resistance genes found in bacteria from wastewater effluents and in aquatic ecosystems. ARGs are frequently detected in WWTPs (Chen and Zhang, 2013; Novo et al., 2013; Rizzo et al., 2013; Manaia, 2014; Table 1), and studies have shown that the ARGs found in wastewaters often reside in clinically relevant pathogenic bacteria (Figueira et al., 2011; Marti et al., 2013a; Hembach et al., 2017). Samples from three different stages of a WWTP in Poland showed that approximately 22, 5, and 9% of Enterobacteriaceae strains isolated from (i) the raw sewage in the primary sedimentation tank, (ii) the aeration tank, and (iii) from the effluent, respectively, carried the intI integron; and that all strains which carried this integron were resistant to at least three unrelated antibiotics (Mokracka et al., 2012). Note that, although a significant fraction of bacteria in the effluent of this WWTP were still resistant, the above percentages must be interpreted with care as the total number of culturable coliform bacteria in the effluent was reduced with a factor of as much as 103 in the effluent as compared to in the raw sewage (Mokracka et al., 2012). Many of the Enterobacteriaceae isolated from a wastewater treatment plant in the study by Amador et al. (2015) were also found to be resistant, and even multi-resistant. The isolates showed resistance against β-lactam group antibiotics, including cefoxitin, amoxicillin, cefotaxime, and non β-lactam groups antibiotics, including trimethoprim/sulfamethoxazole, ciprofloxacin, and tetracycline. Other studies (Mokracka et al., 2012; Szekeres et al., 2017; Karkman et al., 2018) have also shown that resistance genes against antibiotics, including tetracycline, methicillin and sulphonamide are present in WWTPs. Based on a review of many studies, tetracycline (tet) resistance genes have been found to be one of the most commonly occurring ARGs in wastewater treatment systems in many countries (Zhang et al., 2009).
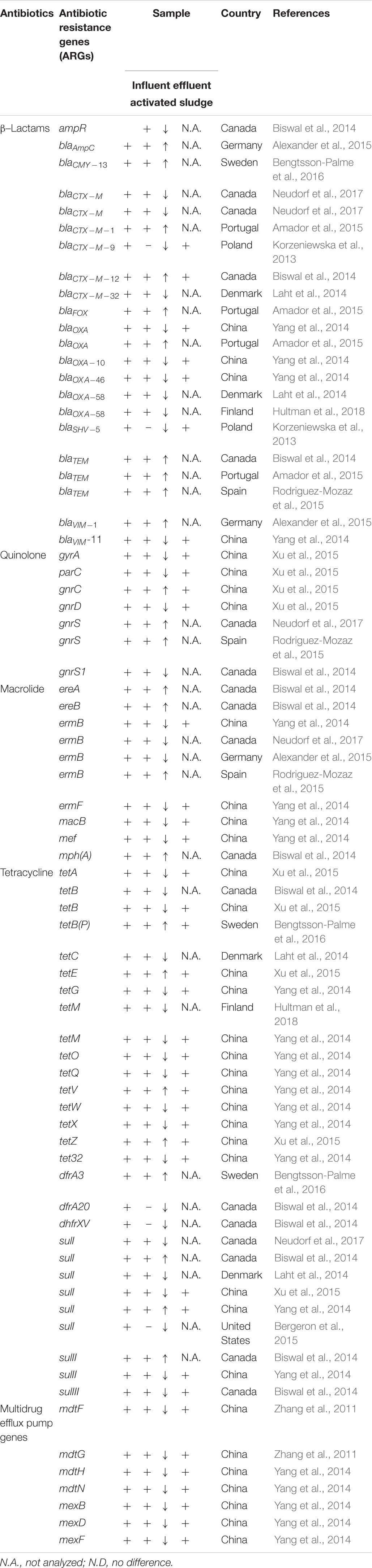
Table 1. Overview of antibiotic resistance genes (ARGs) found in influent, effluent, and activated sludge in wastewater treatment plants (WWTPs) and if their concentrations increase (↑) or decrease (↓) from influent to effluent [adapted from Pazda et al. (2019) and shortened to only include studies that have measured changes in concentration from influent to effluent].
Hospital wastewater is a particular risk as it may contain not only pathogenic single- and multi-drug resistant (MDR) bacteria, as detailed in the previous section but also relatively high concentrations of antibiotic compounds. A high percentage of administered antibiotics are not metabolized in humans and are thus excreted into the sewerage (Sabri et al., 2018). Rodriguez-Mozaz et al. (2015) analyzed a broad range of antibiotics including β-lactams, lincosamides, macrolides, quinolones/fluoroquinolones, sulfonamides, tetracyclines, dihydrofolate reductase inhibitors, and nitroimidazoles and ARGs released from hospitals and urban wastewaters, their removal by a WWTP effluent and their influence on a receiving river. The results show that antibiotics were detected at high concentrations in downstream river samples with antibiotics such as ofloxacin reaching concentrations up to 131.0 ng/L while not being detected upstream of the WWTP discharge. Moreover, ciprofloxacin and sulfamethoxazole had almost 10-fold higher concentrations in downstream than upstream of the WWTP discharge. Studies indicate that the presence of incompletely degraded antibiotic compounds may exert biological selection pressure for the development of ARGs and provide a breeding ground in WWTPs for HGT between bacteria (Zhang et al., 2009; McKinney and Pruden, 2012; Bouki et al., 2013; Sharma et al., 2014) and propagation of resistance genes (Davies and Davies, 2010).
During wastewater treatment, antibiotics, other pharmaceutical residues, and heavy metals present in the wastewater are in continuous contact with bacteria, leading to the potential selection pressure for resistance genes (Zhang et al., 2009; Ding and He, 2010; Bouki et al., 2013). It is difficult to determine a safe concentration of antibiotics in wastewater as results disagree on whether or not antibiotic concentrations lower than the minimum inhibitory concentrations (MIC) cause selection of ARGs. Gullberg et al. (2011) competed for resistant strains against susceptible strains in monoculture with different antibiotic concentrations. The result showed that the resistant strains have a selection advantage even in subminimal inhibitory concentrations and outperform the susceptible strains (Gullberg et al., 2011; Andersson and Hughes, 2014). On the other hand, a recent study by Klümper et al. (2019) suggests that a diverse bacterial community in a mixed culture may select against resistance. Resistant and non-resistant (otherwise isogenic) focal strains (Escherichia coli) cultivated together with a pig fecal community, exhibited more than one order of magnitude higher minimal selection concentration for gentamicin or kanamycin. For the gentamicin resistant focal strain, reduced growth was observed due to higher fitness cost for a range of gentamicin concentrations (0–10 μg/ml), indicating that resource limitations have a stronger impact on resistant phenotypes (Gómez and Buckling, 2011; Wale et al., 2017; Klümper et al., 2019). However, at very high gentamicin concentrations (100 μg/ml) only resistant strains could grow. The same behavior was observed under intermediate kanamycin concentrations (0–20 μg/ml), the susceptible strain did again show improved growth compared to the resistant strain when co-cultured with the pig fecal community. These findings are in accordance with results from the study of Galera-Laporta and Garcia-Ojalvo (2020), where susceptible Bacillus subtilis and E. coli were cultivated exposed to ampicillin separately and in mixed culture. Cultivated separately, B. subtilis was able to grow after a lag phase, while E. coli died. Cultivated in a mixed culture the two strains displayed reversed reactions to ampicillin. The protective effect of the community might play a role and further experimental effort to evaluate the risk of sub-minimal inhibitory concentrations are required.
Heavy metals and some organic compounds, such as quaternary ammonium compounds (QAC), monoaromatic hydrocarbons (MACH), anti-fouling agents and detergents can increase the selective pressure for ARGs through co-selection (Schlüter et al., 2007; Tuckfield and McArthur, 2008; Di Cesare et al., 2016). Two mechanisms for co-selection are normally distinguished: Co-resistance and cross-resistance. Co-resistance refers to the presence of resistance against more than one class of antibiotics in the same bacterial strain. It occurs due to the physical link between different resistance genes, that are placed together, for example on a plasmid, where the selection of resistance to one of the genes leads to resistance to others. Heavy metal resistance genes (HMRGs), especially against zinc and copper, have been shown to increase the rate of AR dissemination by co-resistance (Yazdankhah et al., 2014). Another example is the co-resistance of qac genes encoding for efflux pumps against QAC and MACH; the qac genes are typically located on MGEs (plasmids and transposons), often together with ARGs (Jiao et al., 2017). In cross-resistance, however, one single resistance mechanism confers resistance to an entire class of compounds, antibiotics and/or other toxicants (Baker-Austin et al., 2006). For example, if two different antimicrobials are present and both have a common strategy to attack the cell, resistance developed against one will be effective against both i.e., the resistance gained for one compound confers resistance for another compound. An example of cross resistance is multi-drug resistance pumps that can export both metals and antibiotics (Baker-Austin et al., 2006). Thus, co-selection is a plausible explanation for the persistence of some ARGs even when antibiotics are not present (Zhang et al., 2018) and both co- and cross-resistance have an important impact on the antibiotic resistance selection in different environments (Stepanauskas et al., 2005; Knapp et al., 2017).
Antibiotic Resistance Gene Removal in Wastewater Treatment Plants
There are many treatment techniques used in WWTPs that have varying potential to remove organic matter, nitrogen, phosphorous, pollutants and pathogens from wastewater. However, the mechanisms and efficacy of these techniques to remove antibiotics, ARB and ARGs remain mostly unexplored. This section aims to look at the existing situation for the removal of ARB and ARGs from wastewater and sludge in WWTPs.
Removal From Wastewater
The operation of redox gradient aerobic, anoxic and anaerobic activated sludge reactors and their sequence in a WWTP affects the removal of ARB and ARGs (Christgen et al., 2015; Du et al., 2015; Szekeres et al., 2017). Du et al. (2015) found that anoxic and anaerobic treatment reduced the concentration of ARGs in wastewater, whereas aerobic treatment increased the concentration. The same has been observed by Pei et al. (2007) who proposed that the difference is related to lower growth rates in anaerobic and anoxic compartments compared to aerobic. However, Christgen et al. (2015) have compared three different wastewater treatment strategies; anaerobic, aerobic, and anaerobic–aerobic sequence bioreactors (AAS) in terms of energy use, treatment performance, and ARG abundance. They reported an opposite effect that aerobic bioreactors and AAS bioreactors had higher ARG removal efficiencies than anaerobic bioreactors alone. The AAS bioreactors showed higher removal of ARGs (>85%), compared to separate aerobic (83%) and anaerobic (62%) treatment systems (Christgen et al., 2015). The authors concluded that even though none of these systems were perfect for ARG removal aerobic and AAS were superior to anaerobic bioreactors. Additionally, results suggested that due to lower energy consumption (32% less) AAS systems were seen to be a promising treatment alternative. Moreover, temperature also plays a role in the removal of ARGs showing higher removal at 20°C than at 4°C (Pei et al., 2007), and aerobic treatment may remove more of some types of ARGs than anaerobic at 20°C.
Membrane bioreactors (MBRs) are potentially much better at removing ARB and ARGs than traditional activated sludge reactors. This is because MBRs are better at removing bacteria in general, due to the extra filtration of the effluent through the membrane (Pauwels et al., 2006). The previously mentioned study by Du et al. (2015) reported that the concentration of ARGs throughout a sequence of treatment steps declined proportionally more in the final treatment in an MBR than it did in any of the prior treatment steps in aerobic and anoxic/anaerobic reactors. The MBR showed more than 5 log10 units gene copies/100 ml removal of tetG, tetW, tetX, and sulI resistance genes, mostly due to filtration (pore size 0.1–0.4 μm) (Du et al., 2015; Hiller et al., 2019). Research by Kappell et al. (2018) has similarly shown the effectiveness of anaerobic MBRs with ARG removals of up to 3.6 log10.
Schwermer et al. (2018) investigated the efficiency of two WWTPs in the removal of ampicillin, sulfamethoxazole, ciprofloxacin, and tetracycline resistant E. coli. The two WWTPs employed a biofilm process and a conventional activated sludge treatment process, respectively. By physical and chemical treatment strategies in WWTP, the percentage of resistant E. coli was reduced but full disinfection was not achievable. However, in both conventional activated sludge and the biofilm processes, the percentage of cultivable resistant E. coli did not show a considerable decrease in addition to the physical and chemical treatment steps. Moreover, the effluents were also subjected to ultrafiltration (UF) and the total removal effectiveness of E. coli in both WWTPs with UF was >4.2 log. Although the ability of DNA to pass through membranes was mentioned by the authors, they stated that membrane filtration processes can provide an additional barrier and post-treatment alternative for the effluent in order to reduce ARB and ARG release by WWTP effluents. Other membrane filtration processes that can be used as post treatment methods include microfiltration (MF) and reverse osmosis (RO). While the effectiveness of MF efficiency against ARB and ARGs has been studied (Riquelme Breazeal et al., 2013), the application of RO, alone or combined with other methods, has yet to be investigated in detail (Schwermer et al., 2018).
Constructed wetlands (CWs) are engineered aquatic systems with very diverse microbial communities and are used to treat wastewater by the same biogeochemical processes dominant in natural wetlands (Doherty et al., 2015; Lv et al., 2017). They are, however, mostly relevant for cases where the total amount of wastewater is relatively low, or for wastewater with lower amounts of organic matter, e.g., urban and agricultural runoff or post treatment of effluents from conventional treatment plants, rather than raw sewage (Zhang et al., 2018; Liu et al., 2019). Their ability to remove ARB and ARGs have brought CWs to attention. CW’s removal mechanisms are dependent on different conditions such as phyta and substrate types together with the physical design of the CW itself (Liu et al., 2019). Li et al. (2019) investigated removal efficiencies for antibiotic and ARG in riverine constructed wetlands. Their results showed that one constructed wetland had 46 and 80% removal efficiency for antibiotics and ARGs, respectively, while another wetland had 70 and 88% removal efficiency, respectively. The difference in efficiencies was associated with antibiotic concentrations in the influent into both wetlands and the scale of the wetland, indicating that the presence of sub-inhibitory levels of antibiotics increases the selective pressure for resistance (Li et al., 2019). In a different study, Chen et al. (2019) designed four different hybrid constructed wetlands. Two horizontal sub-surface flow (HSSF) CWs, one with and one without artificial aeration, and two vertical sub-surface flow (VSSF) CWs, again one with and one without artificial aeration. These four CWs were tested for their ability to remove antibiotics and ARGs. Efficiencies between 87 and 95% for total antibiotic removal, and between 88 and 99% for total ARG removal, were reported. The authors found that the hybrid constructed wetlands with artificial aeration compared to CWs without artificial aeration had higher removal efficiencies of ARB and ARGs, together with higher removal rates of organic carbon, ammonia, nitrogen, and phosphorous.
Several recent studies have investigated the removal of ARGs in WWTPs by chemical disinfection processes, such as chlorination and advanced oxidation processes (AOPs) including ozonation and UV. It showed that these processes can significantly decrease the occurrence of ARGs and pathogenic microorganisms in WWTP effluents (Luczkiewicz et al., 2011; Zhuang et al., 2015; Hiller et al., 2019). Zhuang et al. (2015) reported chlorine disinfection resulted in 1.654–2.28 log10 reduction, and UV irradiation resulted in 0.80–1.21 log10 reduction of ARGs under economically suitable operational conditions. Although ozonation disinfection achieved 1.68–2.55 log10 reduction of ARGs, the authors in the same study advised against the use of this process due to excessive operational costs. Contrary to this, Alexander et al. (2016) indicated that even though ozone treatment can reduce the erythromycin resistance gene (ermB) by 2 orders of magnitude, ARGs against vancomycin (vanA) and imipemem (blaVIM) increased within the surviving wastewater bacterial population. Luczkiewicz et al. (2011) showed that ultrafiltration, ozonation, and UV irradiation can reduce the amount of fecal coliform bacteria in wastewater by more than 99%, but there was a slightly higher percentage of ARGs containing bacteria among the bacteria surviving disinfection. They found that of coliforms grown from water samples taken before and after disinfection, 47–60% of E. coli isolates were resistant after disinfection compared to 42–50% of isolates before and that 68–90% of Enterrococus spp. isolates had resistance after treatment compared to 68–85% before. Recently, using a the combination of two or more AOPs (like Fenton’s oxidation reaction, UV/H2O2, solar/H2O2, photo-Fenton process, TiO2 photocatalyst and ionizing radiation) have been shown to be effective in the removal of refractory organic compounds (like antibiotics) in secondary effluents (Rizzo et al., 2013; Zhang et al., 2016b). Karaolia et al. (2014) investigated a solar-driven Fenton oxidation that may eliminate ARB. Mccullagh et al. (2007) reported that the utilization of a UV-TiO2 photocatalyst AOP inactivated a diverse array of bacterial, viral, and protozoal organisms from water and wastewater. While AOPs represent a potential way to remove antibiotics and thus prevent antibiotic resistance, they are not widely used due to their operational costs (Qiao et al., 2018). Table 2 shows different treatment techniques and their effectiveness in removing different ARGs and pathogens from wastewater under different conditions. Additionally, Table 3 summarizes the antibiotic elimination efficiencies in different wastewater treatment units. Both tables include information on different treatment techniques categorized in physical, biological, and chemical processes.
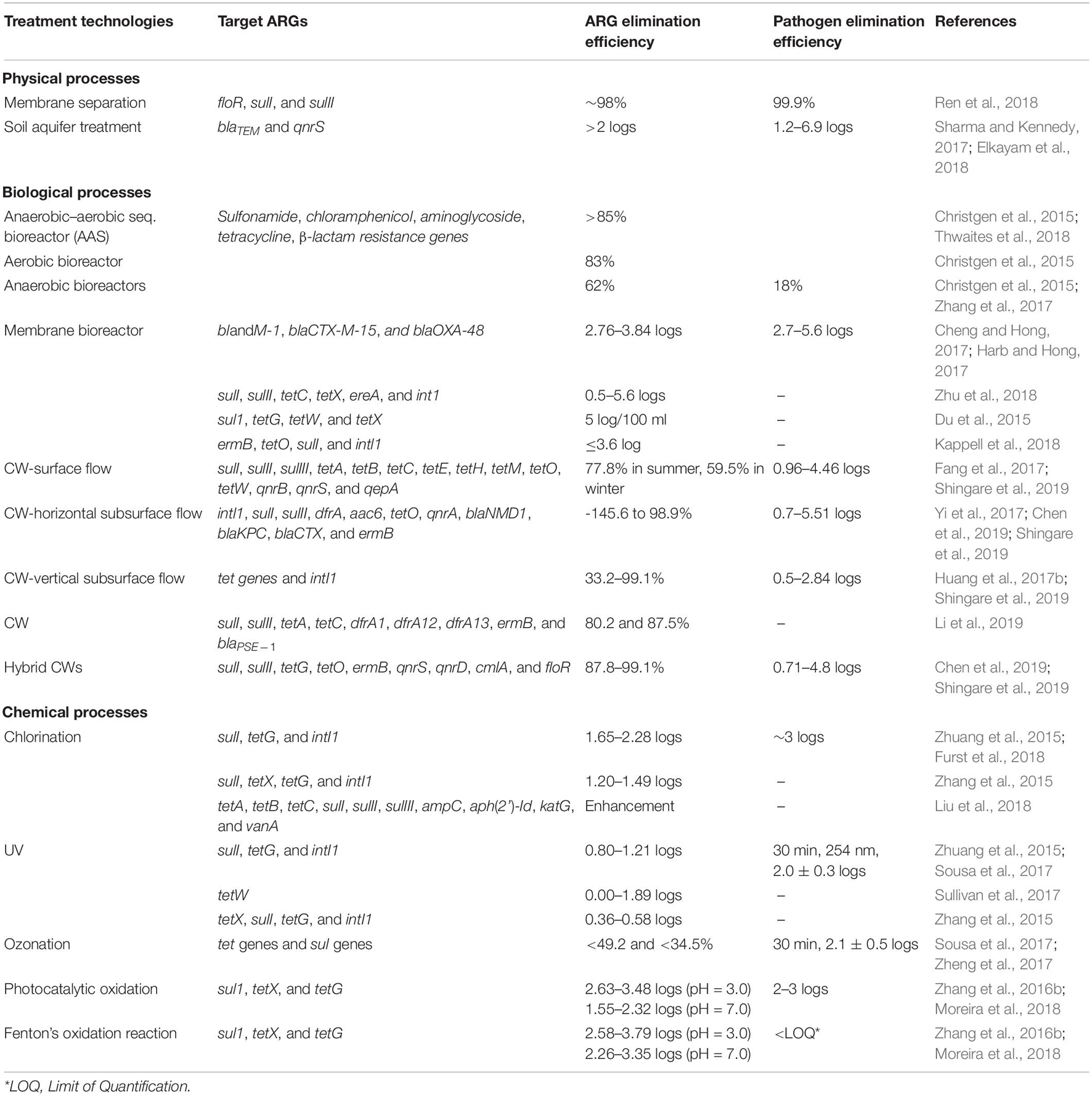
Table 2. Antibiotic resistance gene (ARG) and pathogen elimination efficiencies of different treatment technologies.
All the studies conducted in the literature together with the information presented in Tables 2, 3, suggest that the wastewater to be treated should be analyzed for antibiotics and ARGs, in addition to the standard wastewater characterization parameters. The WWTP should be designed using this characterization specially tailored for the needs of the specific wastewater ensuring the removal of antibiotics and ARGs to avoid the spread of antibiotic resistance in the receiving water body. However, even though the removal efficiency of disinfection processes is very high it is not possible to avoid secondary treatment to cut cost, since the organic matter in the wastewater act as precursors of disinfection by-products. Additionally, the secondary treatment also decreases the suspended solids concentration, which is a key parameter for UV disinfection. As a further treatment step, membrane filtration systems might be used to remove the remaining ARG and ARB, and CW can be considered as a post treatment step for effluents in smaller settings. Finally, MBRs that combine biological treatment and membrane filtration make good alternatives for ARB and ARG removal.
Removal From Sludge and Biosolids
Biological wastewater treatment relies on the growth of bacteria and other microorganisms and subsequent flocculation and settling of aggregated biomass. At a steady state, excessive biomass is removed (so called sludge wasting usually done through the underflow from secondary clarifiers) together with other solids that are collected in skimmers and primary clarifiers. Several unit operations reduce water content, stabilize, and treat the discarded sludge before it is disposed or recycled. Biosolids from WWTPs are typically applied to agricultural land as fertilizer, disposed of to landfills, or incinerated (United States Environmental Protection Agency, 2003; Tchobanoglous et al., 2014; Collivignarelli et al., 2019).
Unsurprisingly, most of the resistant bacteria and resistance genes that arrives with the sewage and that grows and propagates through a treatment plant end up in the settled sludge (Munir et al., 2011; Calero-Cáceres et al., 2014; Yuan et al., 2019). Studies examining municipal WWTPs without advanced sludge treatment in the United States (Munir et al., 2011; Gao et al., 2012) and in China (Chen and Zhang, 2013; Wen et al., 2016; Yuan et al., 2019) have shown that although the plants are able to reduce the abundance of resistance genes and resistant bacteria in their effluents by 2–4 orders of magnitude, the amount of resistance genes and resistant bacteria in the biosolids from these plants are of the same order of magnitude as in the inflow sewage (around 108–1010 copies of tetW and tetO resistance genes per 100 ml sample and 106–108 CFU of tetracycline resistant bacteria per 100 ml sample).
Supplementary Table 1 gives an overview of reported levels of bacteria, resistant bacteria, and resistance genes in biosolids after sludge treatment at WWTPs around the world. The table also includes extended information about plant type, treatment process, sludge sources, and final application of the biosolids. Conventional sludge treatment methods that simply thickens and dewaters sludge by gravity thickening, belt pressing, centrifugation, or other mechanical methods are not effective at removing resistance genes (Supplementary Table 1). Further anaerobic or aerobic digestion of the sludge is also in many cases not enough to substantially reduce the number of resistant bacteria and genes.
Heat drying, which involves reducing the moisture content to below 10% by direct or indirect contact with hot gases (Tchobanoglous et al., 2014), and advanced lime stabilization, which involves the addition of alkali (lime) to increase pH in combination with other treatments like pasteurization or heat drying, are more effective at removing resistance. Heat drying and advanced lime stabilization reduce the density of bacteria in biosolids, and thus also the density of resistant bacteria, to levels similar to and in most cases below what is typical for soil (Supplementary Table 1). This is due to the high temperatures and/or pH that are reached in the processes. The density of resistance genes is on the other hand in many cases still higher than what is typical for unfertilized soil. However, it has been suggested and observed that the genes have lower stability after treatment as many are trapped within dead microorganisms (Lau et al., 2017; Murray et al., 2019). In a study that measured the density of resistance genes in the soil directly after application of biosolids, the authors found that the abundance of resistance genes 2 h after application was remarkably low in soil amended with heat-dried biosolids (Lau et al., 2017). Similar results have also been found for soil amended with biosolids pasteurized at more than 70°C for a period of 30 min in a lab scale experiment (Burch et al., 2017). It seems that many of the resistance genes are rapidly destroyed when they come in contact with soil and moisture.
The N-rich biosolids produced through the N-Viro treatment used at Thorold, Ontario (Supplementary Table 1) have particularly low levels of resistance genes. Murray et al. (2019) found that out of 41 selected genes associated with resistance and HGT, 38 were below the detection limit and the remaining 3 were below the quantification limit. The reason is that the pH is so high that double stranded DNA denatures (Murray et al., 2019).
Pyrolysis is another treatment method that consistently reduces the density of resistance genes in biosolids to below what is found in pristine soil in nature. Pyrolysis is not a common biosolid treatment technique today and has many of the same disadvantages as incineration. It has high capital and operating cost, and requires highly skilled operating and maintenance staff, compared to the simpler dewatering, stabilization, and heat drying methods (Tchobanoglous et al., 2014; Carey et al., 2016). It is also energy intensive, but it can potentially be used as a refinement step in treatment plants that already use heat drying, as the added energy cost of pyrolysis is reported to be low compared to the energy already invested in drying the biosolids (McNamara et al., 2016). The benefit of pyrolysis over incineration is that more organic content and nutrients remain in biochar than in incinerated ash, giving biochar a higher fertilizer potential—biochar has an NKP content of 6-13-0 vs. 0-6-2 for incinerated ash (Carey et al., 2016). They both, however, have the risk of containing high levels of heavy metals, which are concentrated in the product during the production process (Carey et al., 2016).
From the results combined in Supplementary Table 1, it is worth noting that the density of remaining resistance genes after a specific biosolids treatment method can vary with more than an order of magnitude between facilities (Supplementary Table 1). This may be due to differences in the sludge loading or their operation, but also because the methods and protocols for quantification have different sensitivities and efficiencies for extracting and measuring the absolute concentration of genes (Feinstein et al., 2009; Taylor et al., 2019; Yuan et al., 2019).
The trend seen from the numbers in Supplementary Table 1 is that further treatment beyond digestion is needed to reduce the density of resistant bacteria to levels comparable to or below what is found in soils. The trend coincides comparatively well with the grouping of sludge treatment methods used in the biosolids regulation of the United States (40 CFR Part 503) (United States Environmental Protection Agency, 1994, 2003). Treatment processes are categorized into “processes to significantly reduce pathogens” (PSRP) and “processes to further reduce pathogens” (PFRP). PSRP includes the first set of treatment methods after thickening/dewatering, i.e., aerobic digestion, anaerobic digestion, air drying, composting, and limes stabilization, with specific requirements to process parameters such as time, temperature, and pH (United States Environmental Protection Agency, 1994, 2003). PFRP includes further treatments that use heat or radiation to purposefully kill pathogens, i.e., heat drying, heat treatment, pasteurization, beta- or gamma-ray irradiation, and also composting and thermophilic digestion if the temperature is kept over 55°C for a specified number of days (United States Environmental Protection Agency, 1994, 2003). The PSRP and PFRP grouping are in the United States are used together with bacteria density limits (fecal coliforms or Salmonella) to regulate land application of biosolids. However, the current regulations only require PSRP treatment or an average fecal coliform density below 2⋅106 CFU/g for agricultural use (class B biosolids), and there is no specific mention of either resistant bacteria or resistance genes (United States Environmental Protection Agency, 1994, 2003). Similarly, there are currently no specific limits on resistant bacteria or resistance genes for biosolids in the European Union (Eur-Lex, 2018; Collivignarelli et al., 2019). The European Union directive 86/278/EEC (2018), which regulates the application of biosolids in the EU, does not specify any limits on pathogen content, but several member states have national regulations with limit values for indicator bacteria [typically Salmonella and some type(s) of fecal bacteria] (Collivignarelli et al., 2019).
More and more studies are linking the application of biosolids to higher levels of resistant bacteria and genes in agricultural soil (Ross and Topp, 2015; Gondim-Porto et al., 2016; Burch et al., 2017; Lau et al., 2017; Murray et al., 2019). However, the resistance levels decrease with time after application (Marti et al., 2014; Rahube et al., 2014; Ross and Topp, 2015; Burch et al., 2017; Lau et al., 2017; Murray et al., 2019), and the current evidence for gene transfer to crops and animals remains inconclusive (Marti et al., 2013b; Rahube et al., 2014; Lau et al., 2017; Murray et al., 2019; You et al., 2020). Current regulations in the US and the EU do include time restrictions from application to harvesting and/or grazing (United States Environmental Protection Agency, 1994, 2003; Eur-Lex, 2018; Collivignarelli et al., 2019). Implementation of limits for the density of resistant bacteria and resistance genes to the regulations for biosolids should also be considered. Limits on the density of resistance genes can be difficult to implement, as measurement methods for gene amounts have varying sensitivity and accuracy (Feinstein et al., 2009; Taylor et al., 2019; Yuan et al., 2019). The density of resistance genes can furthermore be an inconsistent factor for risk alone because of the difference in stability and transfer potential between genes in living bacteria, genes in dead bacteria, and free and adsorbed genes outside of bacteria. Significant risk reduction can be achieved merely by stricter limits on the general density of bacteria, e.g., as for biosolids of class A today (Murray et al., 2019). Treatment operations that consistently reach these limits are already implemented technologies at many WWTPs. Stricter limits must, however, be weighed against the implications they will have for the overall use of biosolids as fertilizer and soil improvement. Moreover, limits and regulations for biosolids must be harmonized with other biological fertilizers such as manure, which is also known to contain high levels of resistance (Marti et al., 2013b, 2014; Ross and Topp, 2015; Nõlvak et al., 2016). Stricter regulations can lead to more incineration and less reuse, an effect that cannot be disregarded in the context of a sustainable and circular economy.
Modeling Antibiotic Resistance in Wastewater Treatment Plants
Mathematical models formulated from a mechanistic or holistic understanding of microbial and biogeochemical interactions in aquatic systems have advanced our understanding of dynamics in technical and natural systems (Simon et al., 2002; Benedetti et al., 2013). Mathematical models describing the processes involved in the treatment and biodegradation of wastewater have already successfully been developed and established as standard, well used tools within the WWTP community (Gernaey et al., 2004; Solon et al., 2019). Such models are, as mathematical models in general, functional tools for a priori model and hypothesis testing, and a posteriori data analysis and performance evaluation. The standard WWTP models are the so-called activated sludge models (ASM1, ASM2, ASM2d, ASM3, and variants), which have been applied for research and process performance evaluations, as well as for the design of new WWTPs (Henze et al., 2000; Van Loosdrecht et al., 2015). These models include the major WWTP processes of biomass growth, carbon oxidation, nitrification, denitrification, and phosphorus removal. None of the standard models, however, include the occurrence or spread of antibiotic resistance among bacteria in WWTPs.
Several mathematical models for the spread of antibiotic resistance in bacteria populations have been proposed, although mainly in theoretical, or simplified, environmental settings (Birkegård et al., 2018). This includes models of the spread of resistance in axenic cultures of bacteria (Tremblay and Rose, 1985; Imran and Smith, 2007; Svara and Rankin, 2011), and a few models that include spread through more than a single strain (Clewlow et al., 1990). There are also models that deal with the dynamics of antibiotic resistance in relation to antibiotic concentrations and distinguish the type of resistance mechanism (Bootsma et al., 2012; Krzyzanski and Rao, 2017); and finally, models that deal with the spread between hosts of bacteria, i.e., in an epidemiological setting (Spicknall et al., 2013; Levin et al., 2014).
The development of mathematical models that combine the biodegradation processes and population dynamics of microorganisms in a WWTP with the presence of antibiotic compounds, ARGs, and the spread of antibiotic resistance in the populations through HGT is still in its early stages. Attempts to combine the use of WWTP and ARG models are few and limited to early-stage developments. There have, however, been attempts to combine antibiotic degradation dynamics with the traditional activated sludge models (ASM-X by Polesel et al., 2016) to assess degradation kinetics of antibiotics and other pharmaceuticals in WWTPs. Few examples exist of models that have been set up to address the effect of antibiotic resistance in realistic environments that are partly similar to WWTPs (Hellweger et al., 2011; Hellweger, 2013; Baker et al., 2016). Baker et al. (2016) modeled the spread of antimicrobial resistance in a slurry tank that collects and stores fecal and urinary waste from cows at a dairy farm. Their model includes most processes that should be considered to capture both population dynamic and resistance spread, i.e., cellular growth and death processes, HGT, segregation loss, antibiotic concentration (to capture selection pressure), slurry inflow and fitness cost. We think that this model structure with the addition of the treatment processes from the ASM models can serve as a basis for a model suitable for a WWTP environment. Baker et al. (2016) parameterized their model based on their experimental data and data from the literature and showed through sensitivity analysis that gene transfer rate is one of the most important parameters for the spread of resistance. Hellweger et al. (2011) and Hellweger (2013) used a mathematical model to test if observed concentrations of antibiotics and densities of tetracycline resistant bacteria in the Poudre River in Colorado could be explained by different scenarios for how resistant exogenous bacteria that arrive at the river grow and exchanges genes with indigenous bacteria. They showed that the observed data could not be explained by a scenario with high input of exogenous resistant bacteria to the river without growth in the river itself; their model suggested that there has to be the growth of resistant bacteria and thus maintenance of the resistance gene in the river itself, is most likely due to soft selection pressure from low concentrations of tetracycline (Hellweger et al., 2011).
Wastewater treatment plants are highly complex systems with mixed cultures of microorganisms, and a wide range of modeling approaches, including individual-based models (IbMs), are needed to understand the functioning of such plants from micro to macro scale. Deterministic population-level models, like the classical ASM models, allow for studying the average behavior of systems, e.g., the overall dynamics of populations and concentrations in the plant reactors. However, they may miss some important individual effects on biological processes rates in the bacterial community. Population-level models do not account for individual heterogeneity, local interactions, or adaptive behavior. IbMs do on the other hand treat bacteria as single cells, as discrete entities, and might be better suited to account for the spread of resistance and can potentially overcome these limitations that arise from population model design. HGT is a micro-level process. For example, conjugation of resistance plasmids, as this is a discrete event between individual cells, happens when an individual donor bacterium and a recipient bacterium are close enough in space that a pilus from the donor can attach to the recipient and bring them together (Seoane et al., 2011). IbM of conjugation mechanism allows presenting the intrapopulation variability, to capture the changes that occur during the coupling process (Merkey et al., 2011; Seoane et al., 2011). Moreover, local variations in population density, e.g., flocculation, plays a role in the spread of resistance (Merkey et al., 2011), and the description of actions on the level of the single organism in the model may thus be needed to explain the total population development (Breckling, 2002; Hellweger et al., 2016). IbMs can determine the relevance of a specific interaction or location for the overall behavior of the biofilm Therefore, IbMs can give insights into the emergence of antibiotic resistance from biofilms to aquatic environments. Moreover, the combination of different level models, population and individual, can provide quantitative analysis of the spread of antibiotic-resistant bacteria.
Although mathematical models can be powerful tools and the ASM models have been very successful for understanding and developing treatment processes, mathematical modeling approaches have so far not yet been able to help improve our understanding of the conditions that drive maintenance, spread or extinction of ARGs or ARB in WWTPs. The main weakness of many of the proposed models of antimicrobial resistance is the lack of experimental data available to parameterize and validate the models (Birkegård et al., 2018). Any extensions of the standard WWTP models to include resistant and non-resistant bacteria, the presence of ARGs, different mechanisms for HGT, and concentrations of antibiotics, should include considerations on how to experimentally measure associated process rates and concentrations. An integrated AR-WWTP model designed -with this in mind- can become a promising tool for theoretical and diagnostic studies of ARG spreading, and it can be of help in identifying which mechanisms and factors that are the most important for the spread of resistance under different circumstances. That is, in evaluating which operational conditions or parameter values that can minimize spread, and which parameters are key drivers.
Future Directions and Conclusion
In this review, we assessed the causes and the mechanisms for the spread of ARGs, together with their occurrence, transfer, and potential removal in WWTPs. While the issue of antibiotic resistance could never have completely been prevented, the current universal problem of resistant bacteria is solely due to anthropogenic activities. Moreover, the absence of regulations and strict monitoring regimes have contributed to the escalation of the occurrence of antibiotic resistance in the environment. The research shows that neither conventional nor advanced WWTPs are efficient enough to completely remove ARGs and ARB from water environments, but that more advanced treatment methods perform better. Advanced post treatment methods like UV, ozonation and oxidation of water effluents, and heat drying, lime stabilization and pyrolysis of biosolids, remove considerably more ARGs and ARB than activated sludge treatment alone but are not without disadvantages like more difficult and complex operation and higher cost. Finally, the following key points are proposed to improve current WWTPs and provide guidance for future application:
(i) In order to reduce the threat of antibiotic resistance, it is advisable to set strict threshold limits for antibiotic release from point sources like hospitals and animal husbandries, together with the thresholds for release of metal residues, biocides, and other pharmaceuticals that drive co-selection of resistance.
(ii) Plans for implementation of more advanced treatment processes should consider the economy and ecology of the whole waterway. It may be more cost effective to employ smaller scale treatment plants with disinfection units at point sources than to redesign and rebuild larger municipal WWTPs.
(iii) Efforts should be made to devise and agree upon standard methods to measure and report ARB and ARG levels to make it easier to compare resistance levels between different countries and at different treatment plants. This will also make it easier to evaluate removal efficiencies of treatment methods and to evaluate the performance of already established treatment plants, which can facilitate the decision process of operators and regulatory agencies of whether additional post-treatment steps are necessary.
(iv) Experimental studies should be combined with mathematical modeling to further examine the mechanisms for the spread and population growth of resistant and non-resistant bacteria in wastewater treatment environments. The effect of different treatment methods and plant operation strategies on the spread of resistance genes should be further studied, including the effect of operating conditions (pH, temperature, COD, BOD) on HGT.
These approaches can provide a further understanding of the processes and mechanisms of spread and can therefore help in the design of WWTPs that are less likely to become breeding grounds for antibiotic resistance, and which function better as final barriers.
Author Contributions
CU, KMK, and IP-O researched, wrote, and edited the manuscript. KT researched and wrote section “Removal From Sludge and Biosolids” and edited the manuscript. DB researched and designed Table 3. SS, MJ, and GK conceived the review outline. RK provided significant input on wastewater treatment and operation of WWTPs and edited the manuscript. All authors reviewed the manuscript.
Funding
This work was supported by the European Union JPI-AMR grant Wastewater treatment plants as critical reservoirs for resistance genes (Gene-gas) through the Norwegian Research Council grant NFR 271177/H10. Open access publication funds were received from the University of Stavanger.
Conflict of Interest
The authors declare that the research was conducted in the absence of any commercial or financial relationships that could be construed as a potential conflict of interest.
Publisher’s Note
All claims expressed in this article are solely those of the authors and do not necessarily represent those of their affiliated organizations, or those of the publisher, the editors and the reviewers. Any product that may be evaluated in this article, or claim that may be made by its manufacturer, is not guaranteed or endorsed by the publisher.
Supplementary Material
The Supplementary Material for this article can be found online at: https://www.frontiersin.org/articles/10.3389/fmicb.2021.717809/full#supplementary-material
References
Alexander, J., Bollmann, A., Seitz, W., and Schwartz, T. (2015). Microbiological characterization of aquatic microbiomes targeting taxonomical marker genes and antibiotic resistance genes of opportunistic bacteria. Sci. Total Environ. 512–513, 316–325. doi: 10.1016/j.scitotenv.2015.01.046
Alexander, J., Knopp, G., Dötsch, A., Wieland, A., and Schwartz, T. (2016). Ozone treatment of conditioned wastewater selects antibiotic resistance genes, opportunistic bacteria, and induce strong population shifts. Sci. Total Environ. 559, 103–112. doi: 10.1016/j.scitotenv.2016.03.154
Amador, P. P., Fernandes, R. M., Prudêncio, M. C., Barreto, M. P., and Duarte, I. M. (2015). Antibiotic resistance in wastewater: occcurrence and fate of Enterobacteriaceae producers of class A and class C β-lactamases. J. Environ. Sci. Heal. - Part A Toxic/Hazardous Subst. Environ. Eng. 50, 26–39. doi: 10.1080/10934529.2015.964602
Andersson, D. I., and Hughes, D. (2014). Microbiological effects of sublethal levels of antibiotics. Nat. Rev. Microbiol. 12, 465–478. doi: 10.1038/nrmicro3270
Ardern, E., and Lockett, W. T. (1914). Experiments on the oxidation of sewage without the aid of filters. J. Soc. Chem. Ind. 33, 523–539. doi: 10.1002/jctb.5000331005
Auerbach, E. A., Seyfried, E. E., and McMahon, K. D. (2007). Tetracycline resistance genes in activated sludge wastewater treatment plants. Water Res. 41, 1143–1151. doi: 10.1016/j.watres.2006.11.045
Ávila, C., Matamoros, V., Reyes-Contreras, C., Piña, B., Casado, M., Mita, L., et al. (2014). Attenuation of emerging organic contaminants in a hybrid constructed wetland system under different hydraulic loading rates and their associated toxicological effects in wastewater. Sci. Total Environ. 47, 1272–1280. doi: 10.1016/j.scitotenv.2013.10.065
Bairán, G., Rebollar-Pérez, G., Chávez-Bravo, E., and Torres, E. (2020). Treatment processes for microbial resistance mitigation: the technological contribution to tackle the problem of antibiotic resistance. Int. J. Environ. Res. Public Health 17, 1–20. doi: 10.3390/ijerph17238866
Baker, M., Hobman, J. L., Dodd, C. E. R., Ramsden, S. J., and Stekel, D. J. (2016). Mathematical modelling of antimicrobial resistance in agricultural waste highlights importance of gene transfer rate. FEMS Microbiol. Ecol. 92:fiw040. doi: 10.1093/femsec/fiw040
Baker-Austin, C., Wright, M. S., Stepanauskas, R., and McArthur, J. V. (2006). Co-selection of antibiotic and metal resistance. Trends Microbiol. 14, 176–182. doi: 10.1016/j.tim.2006.02.006
Barancheshme, F., and Munir, M. (2018). Strategies to combat antibiotic resistance in the wastewater treatment plants. Front. Microbiol. 8:2603. doi: 10.3389/fmicb.2017.02603
Barlow, M. (2009). “What antimicrobial resistance has taught us about horizontal gene transfer,” in Horizontal Gene Transfer. Methods in Molecular Biology, eds M. B. Gogarten, J. P. Gogarten, and L. C. Olendzenski (Totowa, NJ: Humana Press), 14151–14154. doi: 10.1007/978-1-60327-853-9
Benedetti, L., Langeveld, J., Comeau, A., Corominas, L., Daigger, G., Martin, C., et al. (2013). Modelling and monitoring of integrated urban wastewater systems: review on status and perspectives. Water Sci. Technol. 68, 1203–1215. doi: 10.2166/wst.2013.397
Bengtsson-Palme, J., Hammarén, R., Pal, C., Östman, M., Björlenius, B., Flach, C. F., et al. (2016). Elucidating selection processes for antibiotic resistance in sewage treatment plants using metagenomics. Sci. Total Environ. 572, 697–712. doi: 10.1016/j.scitotenv.2016.06.228
Bergeron, S., Boopathy, R., Nathaniel, R., Corbin, A., and LaFleur, G. (2015). Presence of antibiotic resistant bacteria and antibiotic resistance genes in raw source water and treated drinking water. Int. Biodeterior. Biodegrad. 102, 370–374. doi: 10.1016/j.ibiod.2015.04.017
Birkegård, A. C., Halasa, T., Toft, N., Folkesson, A., and Græsbøll, K. (2018). Send more data: a systematic review of mathematical models of antimicrobial resistance. Antimicrob. Resist. Infect. Control 7:117. doi: 10.1186/s13756-018-0406-401
Biswal, B. K., Mazza, A., Masson, L., Gehr, R., and Frigon, D. (2014). Impact of wastewater treatment processes on antimicrobial resistance genes and their co-occurrence with virulence genes in Escherichia coli. Water Res. 50, 245–253. doi: 10.1016/j.watres.2013.11.047
Bootsma, M. C. J., van der Horst, M. A., Guryeva, T., ter Kuile, B. H., and Diekmann, O. (2012). Modeling non-inherited antibiotic resistance. Bull. Math. Biol. 74, 1691–1705. doi: 10.1007/s11538-012-9731-9733
Bouki, C., Venieri, D., and Diamadopoulos, E. (2013). Detection and fate of antibiotic resistant bacteria in wastewater treatment plants: a review. Ecotoxicol. Environ. Saf. 91, 1–9. doi: 10.1016/j.ecoenv.2013.01.016
Breckling, B. (2002). Individual-based modelling: potentials and limitations. ScientificWorldJournal 2, 1044–1062. doi: 10.1100/tsw.2002.179
Brooks, B. W., Maul, J. D., and Belden, J. B. (2008). “Antibiotics in aquatic and terrestrial ecosystems,” in Encyclopedia of Ecology, eds S. E. Jørgensen, B. D. Fath (Academic Press), 210–217. doi: 10.1016/B978-008045405-4.00370-0
Burch, T. R., Sadowsky, M. J., and LaPara, T. M. (2017). Effect of different treatment technologies on the fate of antibiotic resistance genes and class 1 integrons when residual municipal wastewater solids are applied to soil. Environ. Sci. Technol. 51, 14225–14232. doi: 10.1021/acs.est.7b04760
Calderón, C. B., and Sabundayo, B. P. (2007). “Antimicrobial classifications: drugs for bugs,” in Antimicrobial Susceptibility Testing Protocols, eds R. Schwalbe, L. Steele-Moore, and A. C. Goodwin (Boca Raton FL: CRC Press), 7–53.
Calero-Cáceres, W., Melgarejo, A., Colomer-Lluch, M., Stoll, C., Lucena, F., Jofre, J., et al. (2014). Sludge as a potential important source of antibiotic resistance genes in both the bacterial and bacteriophage fractions. Environ. Sci. Technol. 48, 7602–7611. doi: 10.1021/es501851s
Carey, D. E., Yang, Y., McNamara, P. J., and Mayer, B. K. (2016). Recovery of agricultural nutrients from biorefineries. Bioresour. Technol. 215, 186–198. doi: 10.1016/j.biortech.2016.02.093
Chen, H., and Zhang, M. (2013). Effects of advanced treatment systems on the removal of antibiotic resistance genes in wastewater treatment plants from Hangzhou. China. Environ. Sci. Technol. 47, 8157–8163. doi: 10.1021/es401091y
Chen, J., Deng, W. J., Liu, Y. S., Hu, L. X., He, L. Y., Zhao, J. L., et al. (2019). Fate and removal of antibiotics and antibiotic resistance genes in hybrid constructed wetlands. Environ. Pollut. 249, 894–903. doi: 10.1016/j.envpol.2019.03.111
Cheng, D., Ngo, H. H., Guo, W., Chang, S. W., Nguyen, D. D., Liu, Y., et al. (2020). Removal process of antibiotics during anaerobic treatment of swine wastewater. Bioresour. Technol. 300:122707. doi: 10.1016/j.biortech.2019.122707
Cheng, H., and Hong, P. Y. (2017). Removal of antibiotic-resistant bacteria and antibiotic resistance genes affected by varying degrees of fouling on anaerobic microfiltration membranes. Environ. Sci. Technol. 51, 12200–12209. doi: 10.1021/acs.est.7b03798
Chiang, Y. N., Penadés, J. R., and Chen, J. (2019). Genetic transduction by phages and chromosomal islands: the new and noncanonical. PLoS Pathog. 15:e1007878. doi: 10.1371/journal.ppat.1007878
Christgen, B., Yang, Y., Ahammad, S. Z., Li, B., Rodriquez, D. C., Zhang, T., et al. (2015). Metagenomics shows that low-energy anaerobic-aerobic treatment reactors reduce antibiotic resistance gene levels from domestic wastewater. Environ. Sci. Technol. 49, 2577–2584. doi: 10.1021/es505521w
Clewlow, L. J., Cresswell, N., and Wellington, E. M. H. (1990). Mathematical model of plasmid transfer between strains of streptomycetes in soil microcosms. Appl. Environ. Microbiol. 56, 3139–3145. doi: 10.1128/aem.56.10.3139-3145.1990
Collivignarelli, M. C., Abbà, A., Frattarola, A., Miino, M. C., Padovani, S., Katsoyiannis, I., et al. (2019). Legislation for the reuse of biosolids on agricultural land in Europe: overview. Sustain 11, 1–26. doi: 10.3390/su11216015
Daims, H., Taylor, M. W., and Wagner, M. (2006). Wastewater treatment: a model system for microbial ecology. Trends Biotechnol. 24, 483–489. doi: 10.1016/j.tibtech.2006.09.002
Davies, J., and Davies, D. (2010). Origins and evolution of antibiotic resistance. Microbiologia 74, 417–433. doi: 10.1128/MMBR.00016-10
Davison, H. C., Woolhouse, M. E. J., and Low, J. C. (2000). What is antibiotic resistance and how can we measure it? Trends Microbiol. 8, 554–559.
De la Cruz, N., Giménez, J., Esplugas, S., Grandjean, D., De Alencastro, L. F., and Pulgarín, C. (2012). Degradation of 32 emergent contaminants by UV and neutral photo-fenton in domestic wastewater effluent previously treated by activated sludge. Water Res. 46, 1947–1957. doi: 10.1016/j.watres.2012.01.014
Deurenberg, R. H., and Stobberingh, E. E. (2008). The evolution of Staphylococcus aureus. Infect. Genet. Evol. 8, 747–763. doi: 10.1016/j.meegid.2008.07.007
Di Cesare, A., Eckert, E. M., D’Urso, S., Bertoni, R., Gillan, D. C., Wattiez, R., et al. (2016). Co-occurrence of integrase 1, antibiotic and heavy metal resistance genes in municipal wastewater treatment plants. Water Res. 94, 208–214. doi: 10.1016/j.watres.2016.02.049
Diaz, L., Tran, T. T., Munita, J. M., Miller, W. R., Rincon, S., Carvajal, L. P., et al. (2014). Whole-genome analyses of Enterococcus faecium isolates with diverse daptomycin MICs. Antimicrob. Agents Chemother. 58, 4527–4534. doi: 10.1128/AAC.02686-2614
Ding, C., and He, J. (2010). Effect of antibiotics in the environment on microbial populations. Appl. Microbiol. Biotechnol. 87, 925–941. doi: 10.1007/s00253-010-2649-2645
Directive 91/271/Eec (1991). Council Directive of 21 May 1991 Concerning Urban Waste Water Treatment. Brussels: European Union.
Doherty, L., Zhao, Y., Zhao, X., Hu, Y., Hao, X., Xu, L., et al. (2015). A review of a recently emerged technology: constructed wetland - microbial fuel cells. Water Res. 85, 38–45. doi: 10.1016/j.watres.2015.08.016
Du, J., Geng, J., Ren, H., Ding, L., Xu, K., and Zhang, Y. (2015). Variation of antibiotic resistance genes in municipal wastewater treatment plant with A 2O-MBR system. Environ. Sci. Pollut. Res. 22, 3715–3726. doi: 10.1007/s11356-014-3552-x
Elkayam, R., Aharoni, A., Vaizel-Ohayon, D., Sued, O., Katz, Y., Negev, I., et al. (2018). Viral and microbial pathogens, indicator microorganisms, microbial source tracking indicators, and antibiotic resistance genes in a confined managed effluent recharge system. J. Environ. Eng. (United States) 144, 5017011–5017013.
Elmolla, E., and Chaudhuri, M. (2009). Optimization of Fenton process for treatment of amoxicillin, ampicillin and cloxacillin antibiotics in aqueous solution. J. Hazard. Mater. 170, 666–672. doi: 10.1016/j.jhazmat.2009.05.013
Espíndola, J. C., Cristóvão, R. O., Santos, S. G. S., Boaventura, R. A. R., Dias, M. M., Lopes, J. C. B., et al. (2019). Intensification of heterogeneous TiO2 photocatalysis using the NETmix mili-photoreactor under microscale illumination for oxytetracycline oxidation. Sci. Total Environ. 681, 467–474. doi: 10.1016/j.scitotenv.2019.05.066
Eur-Lex (2018). Council Directive of 12 June 1986 on the Protection of the Environment, and in Particular of the Soil, When Sewage Sludge is Used in Agriculture (86/278/EEC). Available online at: https://eur-lex.europa.eu/eli/dir/1986/278/2018-07-04 (accessed September 27, 2021).
Fang, H., Zhang, Q., Nie, X., Chen, B., Xiao, Y., Zhou, Q., et al. (2017). Occurrence and elimination of antibiotic resistance genes in a long-term operation integrated surface flow constructed wetland. Chemosphere 173, 99–106. doi: 10.1016/j.chemosphere.2017.01.027
Feinstein, L. M., Woo, J. S., and Blackwood, C. B. (2009). Assessment of bias associated with incomplete extraction of microbial DNA from soil. Appl. Environ. Microbiol. 75, 5428–5433. doi: 10.1128/AEM.00120-129
Figueira, V., Vaz-Moreira, I., Silva, M., and Manaia, C. M. (2011). Diversity and antibiotic resistance of Aeromonas spp. in drinking and waste water treatment plants. Water Res. 45, 5599–5611. doi: 10.1016/j.watres.2011.08.021
Furst, K. E., Pecson, B. M., Webber, B. D., and Mitch, W. A. (2018). Tradeoffs between pathogen inactivation and disinfection byproduct formation during sequential chlorine and chloramine disinfection for wastewater reuse. Water Res. 143, 579–588. doi: 10.1016/j.watres.2018.05.050
Galera-Laporta, L., and Garcia-Ojalvo, J. (2020). Antithetic population response to antibiotics in a polybacterial community. Sci. Adv. 6:eaaz5108. doi: 10.1126/sciadv.aaz5108
Gao, P., Munir, M., and Xagoraraki, I. (2012). Correlation of tetracycline and sulfonamide antibiotics with corresponding resistance genes and resistant bacteria in a conventional municipal wastewater treatment plant. Sci. Total Environ. 421–422, 173–183. doi: 10.1016/j.scitotenv.2012.01.061
Gernaey, K. V., Van Loosdrecht, M. C. M., Henze, M., Lind, M., and Jørgensen, S. B. (2004). Activated sludge wastewater treatment plant modelling and simulation: state of the art. Environ. Model. Softw. 19, 763–783. doi: 10.1016/j.envsoft.2003.03.005
Gillings, M. R. (2014). Integrons: past, present, and future. Microbiol. Mol. Biol. Rev. 78, 257–277. doi: 10.1128/mmbr.00056-13
Gómez, P., and Buckling, A. (2011). Bacteria-phage antagonistic coevolution in soil. Science 332, 106–109. doi: 10.1126/science.1198767
Gondim-Porto, C., Platero, L., Nadal, I., and Navarro-García, F. (2016). Fate of classical faecal bacterial markers and ampicillin-resistant bacteria in agricultural soils under Mediterranean climate after urban sludge amendment. Sci. Total Environ. 565, 200–210. doi: 10.1016/j.scitotenv.2016.04.160
Grabow, W. O., and Prozesky, O. W. (1973). Drug resistance of coliform bacteria in hospital and city sewage. Antimicrob. Agents Chemother. 3, 175–180. doi: 10.1128/AAC.3.2.175
Gullberg, E., Cao, S., Berg, O. G., Ilbäck, C., Sandegren, L., Hughes, D., et al. (2011). Selection of resistant bacteria at very low antibiotic concentrations. PLoS Pathog 7:e1002158. doi: 10.1371/journal.ppat.1002158
Harb, M., and Hong, P. Y. (2017). Molecular-based detection of potentially pathogenic bacteria in membrane bioreactor (MBR) systems treating municipal wastewater: a case study. Environ. Sci. Pollut. Res. 24, 5370–5380. doi: 10.1007/s11356-016-8211-y
Hassoun-Kheir, N., Stabholtz, Y., Kreft, J.-U., de la Cruz, R., Romalde, J. L., Nesme, J., et al. (2020). Comparison of antibiotic-resistant bacteria and antibiotic resistance genes abundance in hospital and community wastewater: a systematic review. Sci. Total Environ. 5:140804. doi: 10.1016/j.scitotenv.2020.140804
Hellweger, F. L. (2013). Simple model of tetracycline antibiotic resistance in aquatic environment: accounting for metal coselection. J. Environ. Eng. (United States) 139, 913–921.
Hellweger, F. L., Clegg, R. J., Clark, J. R., Plugge, C. M., and Kreft, J. U. (2016). Advancing microbial sciences by individual-based modelling. Nat. Rev. Microbiol. 14, 461–471. doi: 10.1038/nrmicro.2016.62
Hellweger, F. L., Ruan, X., and Sanchez, S. (2011). A simple model of tetracycline antibiotic resistance in the aquatic environment (with application to the Poudre River). Int. J. Environ. Res. Public Health 8, 480–497. doi: 10.3390/ijerph8020480
Hembach, N., Schmid, F., Alexander, J., Hiller, C., Rogall, E. T., and Schwartz, T. (2017). Occurrence of the mcr-1 colistin resistance gene and other clinically relevant antibiotic resistance genes in microbial populations at different municipal wastewater treatment plants in Germany. Front. Microbiol. 8:1282. doi: 10.3389/fmicb.2017.01282
Henze, M., Gujer, W., Mino, T., and van Loosdrecht, M. (2000). Activated Sludge Models ASM1, ASM2, ASM2d and ASM3. Philippines: I. T. Group.
Heuer, H., and Smalla, K. (2007). Horizontal gene transfer between bacteria. Environ. Biosafety Res. 6, 3–13. doi: 10.1051/ebr:2007034
Hiller, C. X., Hübner, U., Fajnorova, S., Schwartz, T., and Drewes, J. E. (2019). Antibiotic microbial resistance (AMR) removal efficiencies by conventional and advanced wastewater treatment processes: a review. Sci. Total Environ. 685, 596–608. doi: 10.1016/j.scitotenv.2019.05.315
Huang, W., Tsai, L., Li, Y., Hua, N., Sun, C., and Wei, C. (2017a). Widespread of horizontal gene transfer in the human genome. BMC Genomics 18:274. doi: 10.1186/s12864-017-3649-y
Huang, X., Zheng, J., Liu, C., Liu, L., Liu, Y., and Fan, H. (2017b). Removal of antibiotics and resistance genes from swine wastewater using vertical flow constructed wetlands: effect of hydraulic flow direction and substrate type. Chem. Eng. J. 308, 692–699. doi: 10.1016/j.cej.2016.09.110
Hultman, J., Tamminen, M., Pärnänen, K., Cairns, J., Karkman, A., and Virta, M. (2018). Host range of antibiotic resistance genes in wastewater treatment plant influent and effluent. FEMS Microbiol. Ecol. 94:fiy038. doi: 10.1093/femsec/fiy038
Hutinel, M., Huijbers, P. M. C., Fick, J., Åhrén, C., Larsson, D. G. J., and Flach, C. F. (2019). Population-level surveillance of antibiotic resistance in Escherichia coli through sewage analysis. Eurosurveillance 24:1800497. doi: 10.2807/1560-7917.ES.2019.24.37.1800497
Imran, M., and Smith, H. L. (2007). “A mathematical model of gene transfer in a biofilm,” in Mathematics for Ecology and Environmental Sciences, eds Y. Takeuchi, Y. Iwasa, and K. Sato (Berlin: Springer), 93–125.
Jiao, Y. N., Chen, H., Gao, R. X., Zhu, Y. G., and Rensing, C. (2017). Organic Compounds Stimulate Horizontal Transfer of Antibiotic Resistance Genes in Mixed Wastewater Treatment Systems. Amsterdam: Elsevier Ltd, doi: 10.1016/j.chemosphere.2017.05.149
Jury, K. L., Vancov, T., Stuetz, R. M., and Khan, S. J. (2010). Antibiotic resistance dissemination and sewage treatment plants. Curr. Res. Technol. Educ. Appl. Microbiol. Microb. Biotechnol. 2, 509–519.
Kansal, S. K., Kundu, P., Sood, S., Lamba, R., Umar, A., and Mehta, S. K. (2014). Photocatalytic degradation of the antibiotic levofloxacin using highly crystalline TiO2 nanoparticles. New J. Chem. 38, 3220–3226. doi: 10.1039/c3nj01619f
Kapoor, G., Saigal, S., and Elongavan, A. (2017). Action and resistance mechanisms of antibiotics: a guide for clinicians. J. Anaesthesiol. Clin. Pharmacol. 33, 300–305. doi: 10.4103/joacp.JOACP
Kappell, A. D., Kimbell, L. K., Seib, M. D., Carey, D. E., Choi, M. J., Kalayil, T., et al. (2018). Removal of antibiotic resistance genes in an anaerobic membrane bioreactor treating primary clarifier effluent at 20 °C. Environ. Sci. Water Res. Technol. 4, 1783–1793. doi: 10.1039/c8ew00270c
Karaolia, P., Michael, I., García-Fernández, I., Agüera, A., Malato, S., Fernández-Ibáñez, P., et al. (2014). Reduction of clarithromycin and sulfamethoxazole-resistant Enterococcus by pilot-scale solar-driven Fenton oxidation. Sci. Total Environ. 46, 19–27. doi: 10.1016/j.scitotenv.2013.08.027
Karkman, A., Do, T. T., Walsh, F., and Virta, M. P. J. (2018). Antibiotic-resistance genes in waste water. Trends Microbiol. 26, 220–228. doi: 10.1016/j.tim.2017.09.005
Klümper, U., Recker, M., Zhang, L., Yin, X., Zhang, T., Buckling, A., et al. (2019). Selection for antimicrobial resistance is reduced when embedded in a natural microbial community. ISME J. 13, 2927–2937. doi: 10.1038/s41396-019-0483-z
Knapp, C. W., Callan, A. C., Aitken, B., Shearn, R., Koenders, A., and Hinwood, A. (2017). Relationship between antibiotic resistance genes and metals in residential soil samples from Western Australia. Environ. Sci. Pollut. Res. 24, 2484–2494. doi: 10.1007/s11356-016-7997-y
de Sousa Oliveira, K., de Lima, L. A., Cobacho, N. B., Dias, S. C., and Franco, O. L. (2016). “Chapter 2 - mechanisms of antibacterial resistance: shedding some light on these obscure processes?,” in Antibiotic Resistance, eds K. Kon and M. Rai (Academic Press), 19–35. doi: 10.1016/B978-0-12-803642-6.00002-2
Korzeniewska, E., Korzeniewska, A., and Harnisz, M. (2013). Antibiotic resistant Escherichia coli in hospital and municipal sewage and their emission to the environment. Ecotoxicol. Environ. Saf. 91, 96–102. doi: 10.1016/j.ecoenv.2013.01.014
Krzyzanski, W., and Rao, G. G. (2017). Multi-scale model of drug induced adaptive resistance of Gram-negative bacteria to polymyxin B. PLoS One 12:e0171834. doi: 10.1371/journal.pone.0171834
Laht, M., Karkman, A., Voolaid, V., Ritz, C., Tenson, T., Virta, M., et al. (2014). Abundances of tetracycline, sulphonamide and beta-lactam antibiotic resistance genes in conventional wastewater treatment plants (WWTPs) with different waste load. PLoS One 9:e103705. doi: 10.1371/journal.pone.0103705
Lamba, M., Graham, D. W., and Ahammad, S. Z. (2017). Hospital wastewater releases of carbapenem-resistance pathogens and genes in urban India. Environ. Sci. Technol. 51, 13906–13912. doi: 10.1021/acs.est.7b03380
Lau, C. H. F., Li, B., Zhang, T., Tien, Y. C., Scott, A., Murray, R., et al. (2017). Impact of pre-application treatment on municipal sludge composition, soil dynamics of antibiotic resistance genes, and abundance of antibiotic-resistance genes on vegetables at harvest. Sci. Total Environ. 58, 214–222. doi: 10.1016/j.scitotenv.2017.02.123
Levin, B. R., Baquero, F., and Johnsen, P. J. (2014). A model-guided analysis and perspective on the evolution and epidemiology of antibiotic resistance and its future. Curr. Opin. Microbiol. 19, 83–89. doi: 10.1016/j.mib.2014.06.004
Li, B., and Zhang, T. (2011). Mass flows and removal of antibiotics in two municipal wastewater treatment plants. Chemosphere 83, 1284–1289. doi: 10.1016/j.chemosphere.2011.03.002
Li, J., Cheng, W., Xu, L., Strong, P. J., and Chen, H. (2015). Antibiotic-resistant genes and antibiotic-resistant bacteria in the effluent of urban residential areas, hospitals, and a municipal wastewater treatment plant system. Environ. Sci. Pollut. Res. 22, 4587–4596. doi: 10.1007/s11356-014-3665-3662
Li, S., Zhang, R., Hu, J., Shi, W., Kuang, Y., Guo, X., et al. (2019). Occurrence and removal of antibiotics and antibiotic resistance genes in natural and constructed riverine wetlands in Beijing. China. Sci. Total Environ. 664, 546–553. doi: 10.1016/j.scitotenv.2019.02.043
Liu, S. S., Qu, H. M., Yang, D., Hu, H., Liu, W. L., Qiu, Z. G., et al. (2018). Chlorine disinfection increases both intracellular and extracellular antibiotic resistance genes in a full-scale wastewater treatment plant. Water Res. 136, 131–136. doi: 10.1016/j.watres.2018.02.036
Liu, X., Guo, X., Liu, Y., Lu, S., Xi, B., Zhang, J., et al. (2019). A review on removing antibiotics and antibiotic resistance genes from wastewater by constructed wetlands: performance and microbial response. Environ. Pollut. 254:112996. doi: 10.1016/j.envpol.2019.112996
Livermore, D. M. (2012). Fourteen years in resistance. Int. J. Antimicrob. Agents 39, 283–294. doi: 10.1016/j.ijantimicag.2011.12.012
Luczkiewicz, A., Jankowska, K., Bray, R., Kulbat, E., Quant, B., Sokolowska, A., et al. (2011). Antimicrobial resistance of fecal indicators in disinfected wastewater. Water Sci. Technol. 64, 2352–2361. doi: 10.2166/wst.2011.769
Łuczkiewicz, A., Jankowska, K., Fudala-Ksiazek, S., and Olańczuk-Neyman, K. (2010). Antimicrobial resistance of fecal indicators in municipal wastewater treatment plant. Water Res. 44, 5089–5097. doi: 10.1016/j.watres.2010.08.007
Luo, Y., Guo, W., Ngo, H. H., Nghiem, L. D., Hai, F. I., Zhang, J., et al. (2014). A review on the occurrence of micropollutants in the aquatic environment and their fate and removal during wastewater treatment. Sci. Total Environ. 47, 619–641. doi: 10.1016/j.scitotenv.2013.12.065
Lv, T., Carvalho, P. N., Zhang, L., Zhang, Y., Button, M., Arias, C. A., et al. (2017). Functionality of microbial communities in constructed wetlands used for pesticide remediation: influence of system design and sampling strategy. Water Res. 110, 241–251. doi: 10.1016/j.watres.2016.12.021
Macku’ak, T., Nagyová, K., Faberová, M., Grabic, R., Koba, O., Gál, M., et al. (2015). Utilization of Fenton-like reaction for antibiotics and resistant bacteria elimination in different parts of WWTP. Environ. Toxicol. Pharmacol. 40, 492–497. doi: 10.1016/j.etap.2015.07.002
Madigan, M. T., Bender, K. S., Buckley, D. H., Sattley, W. M., and Stahl, D. A. (2006). Brock Biology of Microorganisms, Global Edition. London: Pearson.
Manaia, C. M. (2014). Antibiotic resistance in wastewater: origins, fate, and risks. Pravent. Gesundheitsforderung 9, 180–184. doi: 10.1007/s11553-014-0452-453
Manaia, C. M., Rocha, J., Scaccia, N., Marano, R., Radu, E., Biancullo, F., et al. (2018). Antibiotic resistance in wastewater treatment plants: tackling the black box. Environ. Int. 115, 312–324. doi: 10.1016/j.envint.2018.03.044
Marti, E., and Balcazar, J. L. (2013). “Antibiotic resistance in the aquatic environment,” in Analysis, Removal, Effects and Risk of Pharmaceuticals in the Water Cycle, eds M. Petrovic, S. Perez, and D. Barcelo (Chicago, ILL: Wilson & Wilson’s), 671–684.
Marti, E., Jofre, J., and Balcazar, J. L. (2013a). Prevalence of antibiotic resistance genes and bacterial community composition in a river influenced by a wastewater treatment plant. PLoS One 8:e78906. doi: 10.1371/journal.pone.0078906
Marti, R., Scott, A., Tien, Y. C., Murray, R., Sabourin, L., Zhang, Y., et al. (2013b). Impact of manure fertilization on the abundance of antibiotic-resistant bacteria and frequency of detection of antibiotic resistance genes in soil and on vegetables at harvest. Appl. Environ. Microbiol. 79, 5701–5709. doi: 10.1128/AEM.01682-1613
Marti, R., Tien, Y. C., Murray, R., Scott, A., Sabourin, L., and Topp, E. (2014). Safely coupling livestock and crop production systems: how rapidly do antibiotic resistance genes dissipate in soil following a commercial application of swine or dairy manure? Appl. Environ. Microbiol. 80, 3258–3265. doi: 10.1128/AEM.00231-214
Mazel, D. (2006). Integrons: agents of bacterial evolution. Nat. Rev. Microbiol. 4, 608–620. doi: 10.1038/nrmicro1462
Mccullagh, C., Robertson, J. M. C., Bahnemann, D. W., and Robertson, P. K. J. (2007). The application of TiO 2 photocatalysis for disinfection of water contaminated with pathogenic micro-organisms: a review. Res. Chem. Intermed. 33, 359–375. doi: 10.1163/156856707779238775
McKinney, C. W., and Pruden, A. (2012). Ultraviolet disinfection of antibiotic resistant bacteria and their antibiotic resistance genes in water and wastewater. Environ. Sci. Technol. 46, 13393–13400. doi: 10.1021/es303652q
McNamara, P. J., Koch, J. D., Liu, Z., and Zitomer, D. H. (2016). Pyrolysis of dried wastewater biosolids can be energy positive. Water Environ. Res. 88, 804–810. doi: 10.2175/106143016x14609975747441
Merkey, B. V., Lardon, L. A., Seoane, J. M., Kreft, J. U., and Smets, B. F. (2011). Growth dependence of conjugation explains limited plasmid invasion in biofilms: an individual-based modelling study. Environ. Microbiol. 13, 2435–2452. doi: 10.1111/j.1462-2920.2011.02535.x
Michael, S. G., Michael-Kordatou, I., Nahim-Granados, S., Polo-López, M. I., Rocha, J., Martínez-Piernas, A. B., et al. (2020). Investigating the impact of UV-C/H2O2 and sunlight/H2O2 on the removal of antibiotics, antibiotic resistance determinants and toxicity present in urban wastewater. Chem. Eng. J. 388:124383. doi: 10.1016/j.cej.2020.124383
Modi, S. R., Lee, H. H., Spina, C. S., and Collins, J. J. (2013). Antibiotic treatment expands the resistance reservoir and ecological network of the phage metagenome. Nature 499, 219–222. doi: 10.1038/nature12212.Antibiotic
Mokracka, J., Koczura, R., and Kaznowski, A. (2012). Multiresistant Enterobacteriaceae with class 1 and class 2 integrons in a municipal wastewater treatment plant. Water Res. 46, 3353–3363. doi: 10.1016/j.watres.2012.03.037
Moreira, N. F. F., Narciso-da-Rocha, C., Polo-López, M. I., Pastrana-Martínez, L. M., Faria, J. L., Manaia, C. M., et al. (2018). Solar treatment (H2O2, TiO2-P25 and GO-TiO2 photocatalysis, photo-Fenton) of organic micropollutants, human pathogen indicators, antibiotic resistant bacteria and related genes in urban wastewater. Water Res. 135, 195–206. doi: 10.1016/j.watres.2018.01.064
Munir, M., Wong, K., and Xagoraraki, I. (2011). Release of antibiotic resistant bacteria and genes in the effluent and biosolids of five wastewater utilities in Michigan. Water Res. 45, 681–693. doi: 10.1016/j.watres.2010.08.033
Munita, J. M., and Arias, C. A. (2016). Mechanisms of antibiotic resistance. HHS Public Access 4, 1–37.
Murray, R., Tien, Y. C., Scott, A., and Topp, E. (2019). The impact of municipal sewage sludge stabilization processes on the abundance, field persistence, and transmission of antibiotic resistant bacteria and antibiotic resistance genes to vegetables at harvest. Sci. Total Environ. 651, 1680–1687. doi: 10.1016/j.scitotenv.2018.10.030
Neudorf, K. D., Huang, Y. N., Ragush, C. M., Yost, C. K., Jamieson, R. C., and Truelstrup Hansen, L. (2017). Antibiotic resistance genes in municipal wastewater treatment systems and receiving waters in Arctic Canada. Sci. Total Environ. 598, 1085–1094. doi: 10.1016/j.scitotenv.2017.04.151
Nielsen, P. H., and McMahon, K. D. (2014). “Microbiology and microbial ecology of the activated sludge process,” in Activated Sludge - 100 years and Counting, eds D. Jenkins and J. Wanner (London: IWA Publishing)
Nishino, K., and Yamaguchi, A. (2001). Analysis of a complete library of putative drug transporter genes in Escherichia coli. J. Bacteriol. 183, 5803–5812. doi: 10.1128/JB.183.20.5803-5812.2001
Nõlvak, H., Truu, M., Kanger, K., Tampere, M., Espenberg, M., Loit, E., et al. (2016). Inorganic and organic fertilizers impact the abundance and proportion of antibiotic resistance and integron-integrase genes in agricultural grassland soil. Sci. Total Environ. 562, 678–689. doi: 10.1016/j.scitotenv.2016.04.035
Novo, A., André, S., Viana, P., Nunes, O. C., and Manaia, C. M. (2013). Antibiotic resistance, antimicrobial residues and bacterial community composition in urban wastewater. Water Res. 47, 1875–1887. doi: 10.1016/j.watres.2013.01.010
One Health Initiative (2020). One health. Available online at: http://www.onehealthinitiative.com (accessed December 10, 2020).
Palominos, R., Freer, J., Mondaca, M. A., and Mansilla, H. D. (2008). Evidence for hole participation during the photocatalytic oxidation of the antibiotic flumequine. J. Photochem. Photobiol. A Chem. 193, 139–145. doi: 10.1016/j.jphotochem.2007.06.017
Paucar, N. E., Kim, I., Tanaka, H., and Sato, C. (2019). Ozone treatment process for the removal of pharmaceuticals and personal care products in wastewater. Ozone Sci. Eng. 41, 3–16. doi: 10.1080/01919512.2018.1482456
Pauwels, B., Fru Ngwa, F., Deconinck, S., and Verstraete, W. (2006). Effluent quality of a conventional activated sludge and a membrane bioreactor system treating hospital wastewater. Environ. Technol. 27, 395–402. doi: 10.1080/09593332708618651
Pazda, M., Kumirska, J., Stepnowski, P., and Mulkiewicz, E. (2019). Antibiotic resistance genes identified in wastewater treatment plant systems – a review. Sci. Total Environ. 697:134023. doi: 10.1016/j.scitotenv.2019.134023
Pei, R., Cha, J., Carlson, K. H., and Pruden, A. (2007). Response of antibiotic resistance genes (ARG) to biological treatment in dairy lagoon water. Environ. Sci. Technol. 41, 5108–5113. doi: 10.1021/es070051x
Polesel, F., Andersen, H. R., Trapp, S., and Plósz, B. G. (2016). Removal of antibiotics in biological wastewater treatment systems - a critical assessment using the activated sludge modeling framework for xenobiotics (ASM-X). Environ. Sci. Technol. 50, 10316–10334. doi: 10.1021/acs.est.6b01899
Qian, M., Yang, L., Chen, X., Li, K., Xue, W., Li, Y., et al. (2020). The treatment of veterinary antibiotics in swine wastewater by biodegradation and Fenton-like oxidation. Sci. Total Environ. 710:136299. doi: 10.1016/j.scitotenv.2019.136299
Qiao, M., Ying, G. G., Singer, A. C., and Zhu, Y. G. (2018). Review of antibiotic resistance in China and its environment. Environ. Int. 110, 160–172. doi: 10.1016/j.envint.2017.10.016
Qin, K., Chen, Y., Li, J., Xue, C., Wei, L., Song, X., et al. (2020). Removal trends of sulfonamides and their ARGs during soil aquifer treatment and subsequent chlorination: effect of aerobic and anaerobic biodegradation. Environ. Sci. Water Res. Technol. 6, 2331–2340. doi: 10.1039/d0ew00270d
Rahube, T. O., Marti, R., Scott, A., Tien, Y. C., Murray, R., Sabourin, L., et al. (2014). Impact of fertilizing with raw or anaerobically digested sewage sludge on the abundance of antibiotic-resistant coliforms, antibiotic resistance genes, and pathogenic bacteria in soil and on vegetables at harvest. Appl. Environ. Microbiol. 80, 6898–6907. doi: 10.1128/AEM.02389-2314
Ren, S., Boo, C., Guo, N., Wang, S., Elimelech, M., and Wang, Y. (2018). Photocatalytic reactive ultrafiltration membrane for removal of antibiotic resistant bacteria and antibiotic resistance genes from wastewater effluent. Environ. Sci. Technol. 52, 8666–8673. doi: 10.1021/acs.est.8b01888
Riquelme Breazeal, M. V., Novak, J. T., Vikesland, P. J., and Pruden, A. (2013). Effect of wastewater colloids on membrane removal of antibiotic resistance genes. Water Res. 47, 130–140. doi: 10.1016/j.watres.2012.09.044
Rizzo, L., Manaia, C., Merlin, C., Schwartz, T., Dagot, C., Ploy, M. C., et al. (2013). Urban wastewater treatment plants as hotspots for antibiotic resistant bacteria and genes spread into the environment: a review. Sci. Total Environ. 447, 345–360. doi: 10.1016/j.scitotenv.2013.01.032
Rodriguez-Mozaz, S., Chamorro, S., Marti, E., Huerta, B., Gros, M., Sànchez-Melsió, A., et al. (2015). Occurrence of antibiotics and antibiotic resistance genes in hospital and urban wastewaters and their impact on the receiving river. Water Res. 69, 234–242. doi: 10.1016/j.watres.2014.11.021
Ross, J., and Topp, E. (2015). Abundance of antibiotic resistance genes in bacteriophage following soil fertilization with dairy manure or municipal biosolids, and evidence for potential transduction. Appl. Environ. Microbiol. 81, 7905–7913. doi: 10.1128/AEM.02363-2315
Rowe, W. P. M., Baker-Austin, C., Verner-Jeffreys, D. W., Ryan, J. J., Micallef, C., Maskell, D. J., et al. (2017). Overexpression of antibiotic resistance genes in hospital effluents over time. J. Antimicrob. Chemother. 72, 1617–1623. doi: 10.1093/jac/dkx017
Sabri, N. A., Schmitt, H., Van der Zaan, B., Gerritsen, H. W., Zuidema, T., Rijnaarts, H. H. M., et al. (2018). Prevalence of antibiotics and antibiotic resistance genes in a wastewater effluent-receiving river in the Netherlands. J. Environ. Chem. Eng. 8:102245. doi: 10.1016/j.jece.2018.03.004
Sahar, E., Ernst, M., Godehardt, M., Hein, A., Herr, J., Kazner, C., et al. (2011). Comparison of two treatments for the removal of organic micro-pollutants: conventional Activated Sludge (CAS) Followed by Ultrafiltration (UF) vs. Membrane Bioreactor (MBR). NATO Sci. Peace Secur. Ser. C Environ. Secur. 106, 147–161. doi: 10.1007/978-94-007-0280-6_13
Sandikly, N., Kassir, M., El Jamal, M., Takache, H., Arnoux, P., Mokh, S., et al. (2019). Comparison of the toxicity of waters containing initially sulfaquinoxaline after photocatalytic treatment by TiO2 and polyaniline/TiO2. Environ. Technol. (United Kingdom) 42, 419–428. doi: 10.1080/09593330.2019.1630485
Schlüter, A., Szczepanowski, R., Pühler, A., and Top, E. M. (2007). Genomics of IncP-1 antibiotic resistance plasmids isolated from wastewater treatment plants provides evidence for a widely accessible drug resistance gene pool. FEMS Microbiol. Rev. 31, 449–477. doi: 10.1111/j.1574-6976.2007.00074.x
Schwermer, C. U., Krzeminski, P., Wennberg, A. C., Vogelsang, C., and Uhl, W. (2018). Removal of antibiotic resistant E. coli in two Norwegian wastewater treatment plants and by nano- and ultra-filtration processes. Water Sci. Technol. 77, 1115–1126. doi: 10.2166/wst.2017.642
Seoane, J., Yankelevich, T., Dechesne, A., Merkey, B., Sternberg, C., and Smets, B. F. (2011). An individual-based approach to explain plasmid invasion in bacterial populations. FEMS Microbiol. Ecol. 75, 17–27. doi: 10.1111/j.1574-6941.2010.00994.x
Sharma, S. K., and Kennedy, M. D. (2017). Soil aquifer treatment for wastewater treatment and reuse. Int. Biodeterior. Biodegrad. 119, 671–677. doi: 10.1016/j.ibiod.2016.09.013
Sharma, V. K., Siskova, K. M., Zboril, R., and Gardea-Torresdey, J. L. (2014). Organic-coated silver nanoparticles in biological and environmental conditions: fate, stability and toxicity. Adv. Colloid Interface Sci. 204, 15–34. doi: 10.1016/j.cis.2013.12.002
Shingare, R. P., Thawale, P. R., Raghunathan, K., Mishra, A., and Kumar, S. (2019). Constructed wetland for wastewater reuse: role and efficiency in removing enteric pathogens. J. Environ. Manage. 246, 444–461. doi: 10.1016/j.jenvman.2019.05.157
Simon, M., Grossart, H. P., Schweitzer, B., and Ploug, H. (2002). Microbial ecology of organic aggregates in aquatic ecosystems. Aquat. Microb. Ecol. 28, 175–211. doi: 10.3354/ame028175
Solon, K., Volcke, E. I. P., Spérandio, M., and Van Loosdrecht, M. C. M. (2019). Resource recovery and wastewater treatment modelling. Environ. Sci. Water Res. Technol. 5, 631–642. doi: 10.1039/c8ew00765a
Song, X., Liu, R., Chen, L., and Kawagishi, T. (2017). Comparative experiment on treating digested piggery wastewater with a biofilm MBR and conventional MBR: simultaneous removal of nitrogen and antibiotics. Front. Environ. Sci. Eng. 11:915. doi: 10.1007/s11783-017-0919-915
Sousa, J. M., Macedo, G., Pedrosa, M., Becerra-Castro, C., Castro-Silva, S., Pereira, M. F. R., et al. (2017). Ozonation and UV254nm radiation for the removal of microorganisms and antibiotic resistance genes from urban wastewater. J. Hazard. Mater. 323, 434–441. doi: 10.1016/j.jhazmat.2016.03.096
Spicknall, I. H., Foxman, B., Marrs, C. F., and Eisenberg, J. N. S. (2013). A modeling framework for the evolution and spread of antibiotic resistance: literature review and model categorization. Am. J. Epidemiol. 178, 508–520. doi: 10.1093/aje/kwt017
Stensel, D. H., and Makinia, J. (2014). “Activated sludge process development,” in Activated Sludge - 100 Years and Counting, eds D. Jenkins and W. Jiri (London: IWA Publishing), doi: 10.2166/9781780404943
Stepanauskas, R., Glenn, T. C., Jagoe, C. H., Tuckfield, R. C., Lindell, A. H., and McArthur, J. V. (2005). Elevated microbial tolerance to metals and antibiotics in metal-contaminated industrial environments. Environ. Sci. Technol. 39, 3671–3678. doi: 10.1021/es048468f
Sullivan, B. A., Vance, C. C., Gentry, T. J., and Karthikeyan, R. (2017). Effects of chlorination and ultraviolet light on environmental tetracycline-resistant bacteria and tet(W) in water. J. Environ. Chem. Eng. 5, 777–784. doi: 10.1016/j.jece.2016.12.052
Svara, F., and Rankin, D. J. (2011). The evolution of plasmid-carried antibiotic resistance. BMC Evol. Biol. 11:26–31. doi: 10.1186/1471-2148-11-130
Szekeres, E., Baricz, A., Chiriac, C. M., Farkas, A., Opris, O., Soran, M. L., et al. (2017). Abundance of antibiotics, antibiotic resistance genes and bacterial community composition in wastewater effluents from different Romanian hospitals. Environ. Pollut. 225, 304–315. doi: 10.1016/j.envpol.2017.01.054
Taylor, S. C., Nadeau, K., Abbasi, M., Lachance, C., Nguyen, M., and Fenrich, J. (2019). The ultimate qPCR experiment: producing publication quality. Reproducible Data the First Time. Trends Biotechnol. 37, 761–774. doi: 10.1016/j.tibtech.2018.12.002
Tchobanoglous, G., Stensel, D. H., Tsuchihashi, R., and Burton, F. L. (2014). Wastewater Engineering Treatment and Resource Recovery. New York, NY: McGraw-Hill.
Thwaites, B. J., Short, M. D., Stuetz, R. M., Reeve, P. J., Alvarez Gaitan, J. P., Dinesh, N., et al. (2018). Comparing the performance of aerobic granular sludge versus conventional activated sludge for microbial log removal and effluent quality: implications for water reuse. Water Res. 145, 442–452. doi: 10.1016/j.watres.2018.08.038
Tremblay, C., and Rose, M. R. (1985). Population dynamics of gene transfer. Theor. Popul. Biol. 28, 359–381. doi: 10.1016/0040-5809(85)90035-90038
Tuckfield, R. C., and McArthur, J. V. (2008). Spatial analysis of antibiotic resistance along metal contaminated streams. Microb. Ecol. 55, 595–607. doi: 10.1007/s00248-007-9303-9305
United States Environmental Protection Agency (1994). A Plain English Guide to the EPA Part 503 Biosolids Rule. Washington, DC: United States Environmental Protection Agency.
United States Environmental Protection Agency (2003). Control of Pathogens and Vector Attraction in Sewage Sludge Under 40 CFR Part 503. Washington, DC: United States Environmental Protection Agency.
van Loosdrecht, M. C. M., and Brdjanovic, D. (2014). Anticipating the next century of wastewater treatment. Science 344, 1452–1453. doi: 10.1126/science.1255183
Van Loosdrecht, M. C. M., Lopez-Vazquez, C. M., Meijer, S. C. F., Hooijmans, C. M., and Brdjanovic, D. (2015). Twenty-five years of ASM1: past, present and future of wastewater treatment modelling. J. Hydroinformatics 17, 697–718. doi: 10.2166/hydro.2015.006
Vila, J., Martí, S., and Sánchez-Céspedes, J. (2007). Porins, efflux pumps and multidrug resistance in Acinetobacter baumannii. J. Antimicrob. Chemother. 59, 1210–1215. doi: 10.1093/jac/dkl509
von Wintersdorff, C. J. H., Penders, J., van Niekerk, J. M., Mills, N. D., Majumder, S., van Alphen, L. B., et al. (2016). Dissemination of antimicrobial resistance in microbial ecosystems through horizontal gene transfer. Front. Microbiol. 7:173. doi: 10.3389/fmicb.2016.00173
Wale, N., Sim, D. G., Jones, M. J., Salathe, R., Day, T., and Read, A. F. (2017). Resource limitation prevents the emergence of drug resistance by intensifying within-host competition. Proc. Natl. Acad. Sci. U S A. 114, 13774–13779. doi: 10.1073/pnas.1715874115
Walsh, C. (2003). Where will new antibiotics come from? Nat. Rev. Microbiol. 1, 65–70. doi: 10.1038/nrmicro727
Wang, L., Ben, W., Li, Y., Liu, C., and Qiang, Z. (2018). Behavior of tetracycline and macrolide antibiotics in activated sludge process and their subsequent removal during sludge reduction by ozone. Chemosphere 206, 184–191. doi: 10.1016/j.chemosphere.2018.04.180
Wanger, A., Chavez, V., Huang, R. S. P., Wahed, A., Actor, J. K., and Dasgupta, A. (2017). “Chapter 7 - antibiotics, antimicrobial resistance, antibiotic susceptibility testing, and therapeutic drug monitoring for selected drugs,” in Microbiology and Molecular Diagnosis in Pathology eds A. Wanger, V. Chavez, R. S. P. Huang, A. Wahed, J. K. Actor, and A. Dasgupta (Elsevier), 119–153, doi: 10.1016/B978-0-12-805351-5.00007-7
Wen, Q., Yang, L., Duan, R., and Chen, Z. (2016). Monitoring and evaluation of antibiotic resistance genes in four municipal wastewater treatment plants in Harbin. Northeast China. Environ. Pollut. 212, 34–40. doi: 10.1016/j.envpol.2016.01.043
Wright, G. D. (2005). Bacterial resistance to antibiotics: enzymatic degradation and modification. Adv. Drug Deliv. Rev. 57, 1451–1470. doi: 10.1016/j.addr.2005.04.002
Wright, G. D. (2010). Q&A: antibiotic resistance: where does it come from and what can we do about it? BMC Biol. 8:123. doi: 10.1186/1741-7007-8-123
Wright, G. D. (2011). Molecular mechanisms of antibiotic resistance. Chem. Commun. 47, 4055–4061. doi: 10.1039/c0cc05111j
Xu, J., Xu, Y., Wang, H., Guo, C., Qiu, H., He, Y., et al. (2015). Occurrence of antibiotics and antibiotic resistance genes in a sewage treatment plant and its effluent-receiving river. Chemosphere 119, 1379–1385. doi: 10.1016/j.chemosphere.2014.02.040
Yang, Y., Li, B., Zou, S., Fang, H. H. P., and Zhang, T. (2014). Fate of antibiotic resistance genes in sewage treatment plant revealed by metagenomic approach. Water Res. 62, 97–106. doi: 10.1016/j.watres.2014.05.019
Yazdankhah, S., Rudi, K., and Bernhoft, A. (2014). Zinc and copper in animal feed – development of resistance and co-resistance to antimicrobial agents in bacteria of animal origin. Microb. Ecol. Heal. Dis. 25:25862. doi: 10.3402/mehd.v25.25862
Yi, X., Tran, N. H., Yin, T., He, Y., and Gin, K. Y. H. (2017). Removal of selected PPCPs, EDCs, and antibiotic resistance genes in landfill leachate by a full-scale constructed wetlands system. Water Res. 121, 46–60. doi: 10.1016/j.watres.2017.05.008
You, R., Margenat, A., Lanzas, C. S., Cañameras, N., Carazo, N., Navarro-Martín, L., et al. (2020). Dose effect of Zn and Cu in sludge-amended soils on vegetable uptake of trace elements, antibiotics, and antibiotic resistance genes: human health implications. Environ. Res. 191:109879. doi: 10.1016/j.envres.2020.109879
Yuan, Q., Bin, Huang, Y. M., Wu, W., Bin, Zuo, P., et al. (2019). Redistribution of intracellular and extracellular free & adsorbed antibiotic resistance genes through a wastewater treatment plant by an enhanced extracellular DNA extraction method with magnetic beads. Environ. Int. 131:104986. doi: 10.1016/j.envint.2019.104986
Zhang, J., Zhang, L., Loh, K. C., Dai, Y., and Tong, Y. W. (2017). Enhanced anaerobic digestion of food waste by adding activated carbon: fate of bacterial pathogens and antibiotic resistance genes. Biochem. Eng. J. 128, 19–25. doi: 10.1016/j.bej.2017.09.004
Zhang, S., Song, H. L., Yang, X. L., Li, H., and Wang, Y. W. (2018). A system composed of a biofilm electrode reactor and a microbial fuel cell-constructed wetland exhibited efficient sulfamethoxazole removal but induced sul genes. Bioresour. Technol. 256, 224–231. doi: 10.1016/j.biortech.2018.02.023
Zhang, T., Zhang, X. X., and Ye, L. (2011). Plasmid metagenome reveals high levels of antibiotic resistance genes and mobile genetic elements in activated sludge. PLoS One 6:e26041. doi: 10.1371/journal.pone.0026041
Zhang, X. X., Zhang, T., and Fang, H. H. P. (2009). Antibiotic resistance genes in water environment. Appl. Microbiol. Biotechnol. 82, 397–414. doi: 10.1007/s00253-008-1829-z
Zhang, Y., Geng, J., Ma, H., Ren, H., Xu, K., and Ding, L. (2016a). Characterization of microbial community and antibiotic resistance genes in activated sludge under tetracycline and sulfamethoxazole selection pressure. Sci. Total Environ. 571, 479–486. doi: 10.1016/j.scitotenv.2016.07.014
Zhang, Y., Zhuang, Y., Geng, J., Ren, H., Xu, K., and Ding, L. (2016b). Reduction of antibiotic resistance genes in municipal wastewater effluent by advanced oxidation processes. Sci. Total Environ. 550, 184–191. doi: 10.1016/j.scitotenv.2016.01.078
Zhang, Y., Zhuang, Y., Geng, J., Ren, H., Zhang, Y., Ding, L., et al. (2015). Inactivation of antibiotic resistance genes in municipal wastewater effluent by chlorination and sequential UV/chlorination disinfection. Sci. Total Environ. 51, 125–132. doi: 10.1016/j.scitotenv.2015.01.028
Zheng, J., Su, C., Zhou, J., Xu, L., Qian, Y., and Chen, H. (2017). Effects and mechanisms of ultraviolet, chlorination, and ozone disinfection on antibiotic resistance genes in secondary effluents of municipal wastewater treatment plants. Chem. Eng. J. 317, 309–316. doi: 10.1016/j.cej.2017.02.076
Zhu, T., Su, Z., Lai, W., Zhang, Y., and Liu, Y. (2021). Insights into the fate and removal of antibiotics and antibiotic resistance genes using biological wastewater treatment technology. Sci. Total Environ. 776:145906. doi: 10.1016/j.scitotenv.2021.145906
Zhu, Y., Wang, Y., Zhou, S., Jiang, X., Ma, X., and Liu, C. (2018). Robust performance of a membrane bioreactor for removing antibiotic resistance genes exposed to antibiotics: role of membrane foulants. Water Res. 130, 139–150. doi: 10.1016/j.watres.2017.11.067
Keywords: antibiotics, antibiotic resistance genes, antibiotic resistant bacteria, spread mechanisms, wastewater treatment plants
Citation: Uluseker C, Kaster KM, Thorsen K, Basiry D, Shobana S, Jain M, Kumar G, Kommedal R and Pala-Ozkok I (2021) A Review on Occurrence and Spread of Antibiotic Resistance in Wastewaters and in Wastewater Treatment Plants: Mechanisms and Perspectives. Front. Microbiol. 12:717809. doi: 10.3389/fmicb.2021.717809
Received: 31 May 2021; Accepted: 15 September 2021;
Published: 11 October 2021.
Edited by:
Elisabet Marti, Agroscope, SwitzerlandReviewed by:
Pin Gao, Donghua University, ChinaRachelle E. Beattie, University of North Carolina at Chapel Hill, United States
Copyright © 2021 Uluseker, Kaster, Thorsen, Basiry, Shobana, Jain, Kumar, Kommedal and Pala-Ozkok. This is an open-access article distributed under the terms of the Creative Commons Attribution License (CC BY). The use, distribution or reproduction in other forums is permitted, provided the original author(s) and the copyright owner(s) are credited and that the original publication in this journal is cited, in accordance with accepted academic practice. No use, distribution or reproduction is permitted which does not comply with these terms.
*Correspondence: Ilke Pala-Ozkok, aWxrZS5wYWxhb3prb2tAdWlzLm5v
†These authors have contributed equally to this work