- 1Key Lab of Urban Environment and Health, Institute of Urban Environment, Chinese Academy of Sciences (CAS), Xiamen, China
- 2Center for Excellence in Regional Atmospheric Environment, Institute of Urban Environment, Chinese Academy of Sciences (CAS), Xiamen, China
- 3School of Resources and Environmental Engineering, Anhui University, Hefei, China
Anaerobic ammonium oxidation coupled to nitrite reduction (termed as Anammox) was demonstrated as an efficient pathway to remove nitrogen from a wastewater treatment system. Recently, anaerobic ammonium oxidation was also identified to be linked to iron(III) reduction (termed Feammox) with dinitrogen, nitrite, or nitrate as end-product, reporting to enhance nitrogen removal from the wastewater treatment system. However, little is known about the role of Anammox bacteria in the Feammox process. Here, slurry from wastewater reactor amended with ferrihydrite was employed to investigate activity of Anammox bacteria in the Feammox process using the 15N isotopic tracing technique combined with 16S rRNA gene amplicon sequencing. A significantly positive relationship between rates of 15N2 production and iron(III) reduction indicated the occurrence of Feammox during incubation. Relative abundances of Anammox bacteria including Brocadia, Kuenenia, Jettenia, and unclassified Brocadiaceae were detected with low relative abundances, whereas Geobacteraceae dominated in the treatment throughout the incubation. 15N2 production rates significantly positively correlated with relative abundances of Geobacter, unclassified Geobacteraceae, and Anammox bacteria, revealing their contribution to nitrogen generation via Feammox. Overall, these findings suggested Anammox bacteria or cooperation between Anammox bacteria and iron(III) reducers serves a potential role in Feammox process.
Introduction
Feammox [anaerobic ammonium oxidation coupled to iron(III) reduction] is a pathway of nitrogen cycling identified recently and makes a contribution to nitrogen loss in various environments, such as terrestrial (e.g., wetland, tropical rainforest, and paddy soils) and aquatic ecosystem (e.g., freshwater and marine) in addition to denitrification, co-denitrification, and anaerobic ammonium oxidation (Clement et al., 2005; Yang et al., 2012; Ding et al., 2014, 2017, 2019; Huang and Jaffé, 2014; Zhou et al., 2016). Previous studies showed that iron(III)-reducing bacteria such as Anaeromyxobacter, Pseudomonas, Geobacter, Desulfosporosinus, Dechloromonas, and Geothrix always dominated in the Feammox “pool” (Zhou et al., 2016; Li et al., 2019); however, these iron(III) reducers prefer to utilize organic carbon for iron(III) reduction. Only a minor part of iron(III) reduction (0.4–6.1%) by these bacteria is estimated to be associated with Feammox in the natural or artificial environments (Yang et al., 2012; Ding et al., 2014, 2017, 2019; Li et al., 2015; Zhou et al., 2016). As a replacement, 15NH4+ is generally employed to trace the occurrence of Feammox; however, it is hard to directly identify the Feammox microbes through DNA or RNA stable isotope probing because the Feammox process results in conversion of 15NH4+ to 15N2/15NO3–/15NO2– but not assimilation of 15NH4+ into DNA and RNA. As a result, “Feammox microbes” are difficult to be directly captured from the incubation.
Sawayama (2006) has reported that the possibility of Feammox happened in a wastewater treatment system, which is recently expected to become another important way to remove nitrogen from the sludge of wastewater in addition to Anammox. Anammox is another anaerobic ammonium oxidation pathway that used nitrite as an electron acceptor with forming dinitrogen (Rikmann et al., 2014). Furthermore, the addition of iron(III) oxides is indicated to increase the efficiency of nitrogen removal in the system (Chen et al., 2014; Wang et al., 2016; Li H. et al., 2018; Yin et al., 2019). These discoveries further suggest the occurrence of Feammox in the Anammox-based nitrogen removal system of a wastewater treatment reactor. However, the Feammox-involving microorganisms in the wastewater treatment system is still under-characterized. Anammox bacteria, including members of the Planctomycetales such as Brocadia, Kuenenia, Anammoxoglobus, Jettenia, Anammoximicrobium moscowii, and Scalindua (Rikmann et al., 2014), possesses a versatile metabolism involved in the utilization of diverse electron donors (e.g., NH4+ and propionate) and acceptors (e.g., NO2–, NO3–, and SO42–) (Strous et al., 2002; Cervantes et al., 2009; Rikmann et al., 2014; Rios-Del Toro and Cervantes, 2016; Rios-Del Toro et al., 2018). Inspired by these recent findings, we hypothesized that Fe(III) can play as terminal electron acceptors in anaerobic ammonium oxidation mediated by Anammox bacteria in the sludge from a wastewater treatment reactor.
In order to verify our hypothesis, sludge from a wastewater treatment reactor, which has been demonstrated to employ the Anammox process to remove nitrogen (Zhang et al., 2012), was used in this study. Through the 15NH4+-based isotopic tracing technique with 16S rRNA gene Illumina sequencing, we amended the sludge with ferrihydrite to investigate whether (1) Feammox occurred in the Anammox enrichment and (2) the Anammox organisms were involved in Feammox process.
Materials and Methods
Experimental Procedures
The sludge was obtained from a wastewater treatment reactor (Zhang et al., 2012). The dominant electron donor and acceptor were NH4+ and NO2– in the wastewater treatment reactor, respectively, which has been established as a stable, completely autotrophic nitrogen removal process over nitrite (Canon). Theoretically, the Canon is a process combining partial nitrification with Anammox within a single reactor, which has been shown to be a cost-efficient autotrophic process for nitrogen removal, as it has no need for a carbon source and has low requirement for oxygen (Zhang et al., 2012). The characteristic of the sludge is detailed in Supplementary Table 1. The Feammox experiment was initiated by inoculating 10% (v/v) sludge into 20 ml basal medium and incubated at 30°C in the dark. The basal medium (pH 6.8–7.2) consists of MgCl2⋅6H2O (0.4 g L–1), CaCl2⋅H2O (0.1 g L–1), 14NH4Cl (0.027 g L–1), KH2PO4 (0.6 g L–1), 1 ml L–1 vitamin solution (Lovley and Phillips, 1988), 1 ml L–1 trace element solution (Lovley and Phillips, 1988), 30 mmol L–1 bicarbonate buffer, and 4 mmol L–1 Fe(III). The headspace of the serum vials was flushed with ultrapure helium. Ferrihydrite was synthesized as previously described (Kappler et al., 2014) and used as the Fe(III) source. The basal medium and ferrihydrite were autoclaved (120°C for 20 min) before use, and the vitamin solution and trace element solution were filtrated with a 0.22-μm filter from the stock solutions. In order to enrich the Feammox-associated microbial population, the cultures were anaerobically transferred (10%, v/v) to fresh medium for three generations once the Fe(III) was used up.
For the labeled experiment, 14NH4Cl was replaced by 15NH4Cl (15N, 99.14%; Cambridge Isotope Laboratories, Andover, MA, United States) to prepare the fresh medium. In brief, aliquots (2 ml) of the Feammox enrichment were centrifuged and washed three times with sterile deionized water before inoculating into the 20 ml of fresh 15NH4Cl-labeled medium. Three treatments were set up: (1) NH4+: Feammox enrichment was inoculated in the 20 ml of 15NH4Cl-added basal medium amended without ferrihydrite; (2) Fe(III): Feammox enrichment was inoculated in the 20 ml of ferrihydrite-containing basal medium amended without 15NH4Cl; and (3) Fe(III) + NH4+: Feammox enrichment was inoculated in the 20 ml of basal medium amended with both ferrihydrite and 15NH4Cl. The final concentrations of 15NH4Cl and ferrihydrite were 0.5 and 4 mmol L–1 in the treatments, respectively. The number of serum vials for each treatment was 4, 4, and 24, respectively. All the treatments were incubated at 30°C in a dark under anaerobic condition.
Chemical Analysis
Ferrous iron and total iron were determined as described previously (Kappler et al., 2005). Briefly, Fe(II) was determined by anaerobically transferring 100 μl of culture suspension with a syringe into 900 μl of 40 mmol L–1 sulfamic acid and incubating for 1 h at room temperature. Total Fe was extracted using a mixture of 20 mmol L–1 hydroxylamine hydrochloride and 20 mmol L–1 sulfamic acid (v:v = 1:1) (Klueglein et al., 2015). A 100-μl extract was then added with 1 ml ferrozine solution (1 g ferrozine in 50 mmol L–1 HEPES buffer, pH 7) to generate the ferrous complex, which was quantified at 562 nm UV/Vis spectrometer. Change in Fe(II) concentrations between two given time points (23 days) were used to calculate iron(III) reduction rates.
For the 15N-N2 analysis, vials were shaken vigorously to equilibrate the dissolved phase with gaseous phase, and 1 ml of gas samples was collected from the headspace using gas-tight syringes and then injected into 12-ml glass vials (Exetainer; Labco, Lampeter, United Kingdom). The gas samples were taken on days 1, 4, 8, 10, 12, 14, 18, and 22, respectively. 30N2 and 29N2 concentrations were calculated by multiplying the moles of total N2 in the headspace by the 30N2 and 29N2 mole fractions (Zhou et al., 2016). The total N2 and N2O concentration in the headspace was measured using a robotized system coupled to a gas chromatograph (Agilent Technologies, Santa Clara, CA, United States) as previously described (Zhou et al., 2016). The mole fractions of 30N2 and 29N2 were determined by isotope ratio mass spectrometry (IRMS; Thermo Finnigan Delta V Advantage, Bremen, Germany) coupled with Gasbench II, respectively (Zhou et al., 2016). After gas collection, the remaining cultures were immediately centrifuged at 14,000 × g for 15 min and the pellets were used for DNA extraction. The resulting supernatant was filtered through 0.22-μm filters and then subjected to measurement of NH4+, NO2–, and NO3– concentrations by ion chromatography (Dionex ICS-3000 system; Diones, Sunnyvale, CA, United States). All the liquid was sampled in the anaerobic glovebox (Shel Lab Bactron IV; Shel Lab, Cornelius, OR, United States) to avoid chemical oxidation. pH was analyzed using a dual-channel pH-ion-conductivity-dissolved oxygenmeter (X60; Thermo Fisher Scientific, Carlsbad, CA, United States) in the anaerobic glovebox.
DNA Extraction and Illumina Sequencing
DNA was extracted using FastDNA Spin Kit (MP Biomedical, Illkirch-Graffenstaden, France) according to the manufacturer’s protocol and stored at −20°C for the molecular analyses. Since the biomass in the treatments amended with only Fe(III) or NH4+ was extremely low, there was enough DNA extracted from these treatments.
To investigate the bacterial community structures and compositions, the V4–V5 region of bacterial was amplified using the DNA extracted from the samples in the treatment of Fe(III) + NH4+ as template. The amplicons were purified, quantified, pooled, and then sequenced on an Illumina Miseq PE 250 platform (Novogene, Beijing, China) (Zhou et al., 2016). The forward primer was 515F (5′-GTGCCAGCMGCCGCGG-3′), and the reverse primer consisted of a 6-bp barcode and 907R (5′-CCGTCAATTCMTTTRAGTTT-3′) (Ren et al., 2014). Quantitative Insights into Microbial Ecology toolkit-version 1.9.0 (QIIME) was used to process and analyze sequences as previously described (Su et al., 2015). After removal of low-quality or ambiguous reads, operational taxonomic units (OTU) were determined at 97% similarity level using UCLUST clustering in accordance with the online instruction of QIIME for open-reference OTU pick, definition, and determination (Wang et al., 2007). The representative sequences of each OTU were assigned to taxonomy using an RDP classifier (Version 11).1
Quantitative PCR
The abundance of relevant genes and microbial organisms, including bacterial 16S rRNA gene, Geobacteraceae spp., Acidimicrobiaceae spp. (the reported potential microbe responsible for Feammox) (Huang and Jaffé, 2014), hzsB (hydrazine synthase), nirS (nitrite reductase), and nosZ (nitrous oxide reductase) was analyzed with a real-time PCR Detection System (Roche 480; Roche, Indianapolis, IN, United States). The primer sets and thermal cycles were detailed in Supplementary Table 2. The 20-μl qPCR reaction contained 10 μl 2 × TransStart® Top Green qPCR SuperMix (AQ131; Transgen Biotech, Beijing, China), 0.25 μM each primer, 0.8 μl bovine serum albumin (BSA, 20 mg ml–1), and 2 μl of fivefold diluted DNA as a template. The standard curve was obtained using 10-fold serial dilutions of plasmid DNA with target-genes. Three non-template controls were carried out for each quantitative assay. A melting curve for each reaction showed that only one special peak was detected. Only the reactions with efficiencies between 90 and 110%, and standard curves with correlation coefficient above 0.99 were employed in this study.
Statistical Analyses
Analysis of variance (ANOVA) and Pearson correlation analysis were conducted by SPSS 18.0 (SPSS Inc., Chicago, IL, United States) and Origin 9.0 (OriginLab, Northampton, MA, United States). Statistical significance was performed using Duncan’s multiple range test and denoted at p < 0.05. The differences of the bacterial communities were analyzed by non-metric multidimensional scaling (NMDS) based on weighted UniFrac dissimilarity among samples, which was represented by the ordination axes (Tunney et al., 2013).
Data Accessibility
The 16S rRNA gene sequences have been deposited in GenBank with accession number SRP116169.
Results
Iron(III) Reduction and Changes of N Species in the Enrichment
In the Fe(III) + NH4+ treatment, Fe(II) increased up to 1.83 ± 0.010 mmol L–1 after 22-day anaerobic incubation (Figure 1A). In comparison, iron(III) reduction was not detected in the treatments only amended with Fe(III) or NH4+ after the 22-day incubation (Figure 1A).
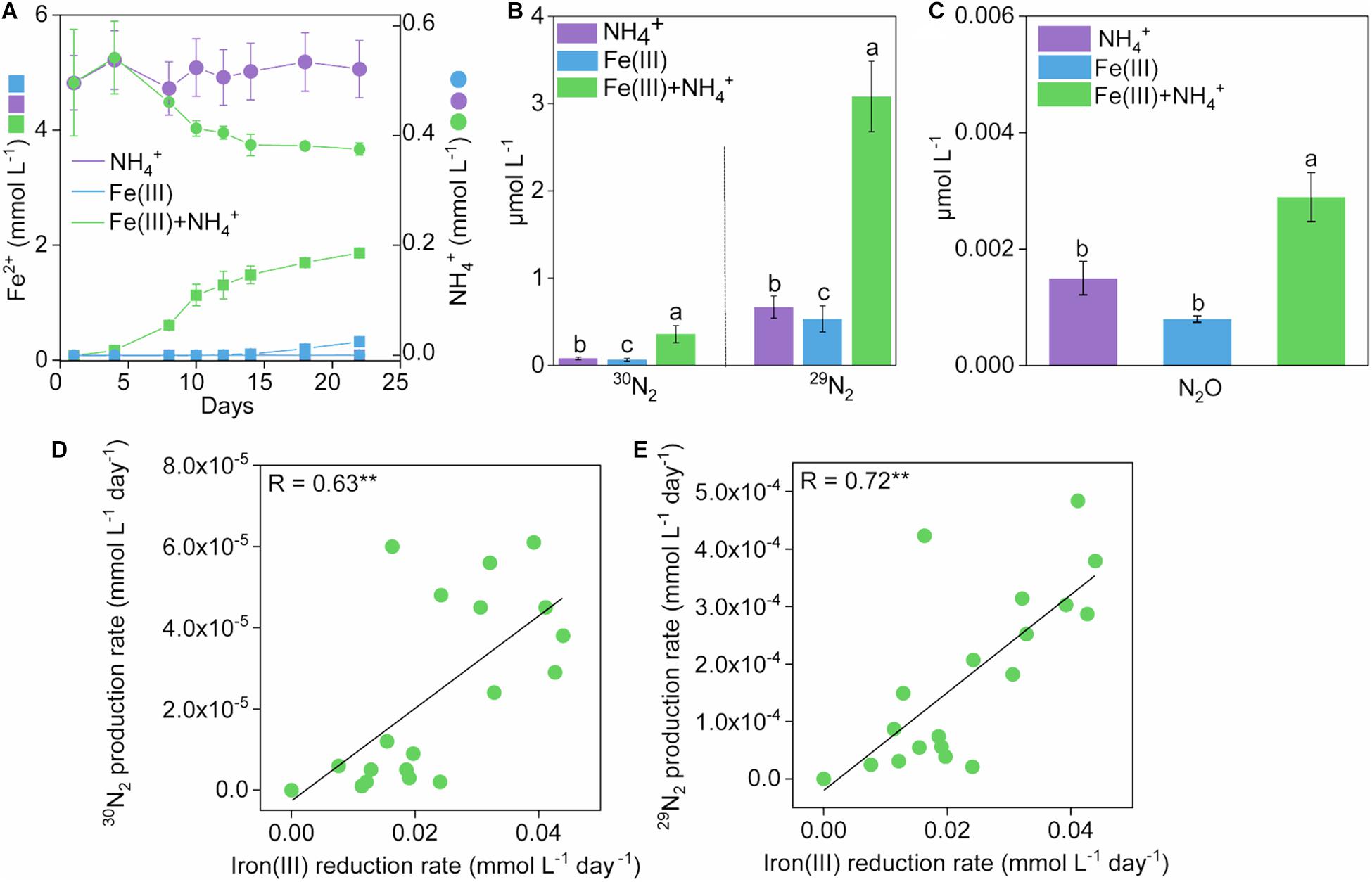
Figure 1. Development of Fe(II), NH4+ and N2 production in treatments during 22-day incubation. Kinetics of Fe(II) (A), NH4+ (B), and N2 (C) production in treatments of NH4+, Fe(III), and Fe(III) + NH4+ during 22-day incubation. Error bars represent standard deviations of three replications. The different lowercase letters above the error bar denote statistically significant (p < 0.05) differences among different treatments. (D,E) The correlation between rates of iron(III) reduction and 15N2 production in treatments of Fe(III) + NH4+ during 22-day incubation. “*” and “**” represent a statistically significant relationship between rates of iron(III) reduction and 15N2 production, which is denoted at p < 0.05 and p < 0.01, respectively.
Significant (p < 0.05) accumulation of 30N2 was detected in the Fe(III) + NH4+ treatment (0.36 μmol L–1) compared to that in the treatment of Fe(III) (0.064 μmol L–1) or NH4+ (0.080 μmol L–1) during the incubation (Figure 1B and Supplementary Figure 1). The 29N2 production rates showed similar trends to that of 30N2 (Figure 1B and Supplementary Figure 1). Headspace N2O exhibited a higher concentration in the treatment of Fe(III) + NH4+ (2.89 × 10–3 μmol L–1) than that in the treatment of Fe(III) (8.00 × 10–4 μmol L–1) or NH4+ (9.01 × 10–4 μmol L–1) during the incubation (Figure 1C). An amount of 0.12 mmol L–1 NH4+ was consumed in the treatment of Fe(III) + NH4+ (Figure 1A). Almost no utilization of NH4+ was observed in the NH4+ treatment (Figure 1A).
The rates of 30N2 and 29N2 production were significantly (p < 0.001) correlated with iron(III) reduction rates in the Fe(III) + NH4+ treatment (Figures 1D,E).
Changes in Abundances of Bacteria and the N Cycling-Relevant Genes
16S rRNA gene copy number increased up to 2.36 × 1010 copies L–1 medium after incubation in the Fe(III) + NH4+ treatment (Figure 2A). Also, the abundances of hzsB, nirS, and nosZ rapidly elevated in the treatment of Fe(III) + NH4+ after 12 days (Figure 2 and Supplementary Figure 2). Especially, the gene copy numbers were higher for the genes nirS (1.26 × 107) and nosZ (2.62 × 107) than that for the gene hzsB (7.36 × 106 copies L–1 medium) in the treatment of Fe(III) + NH4+ (Figure 2A).
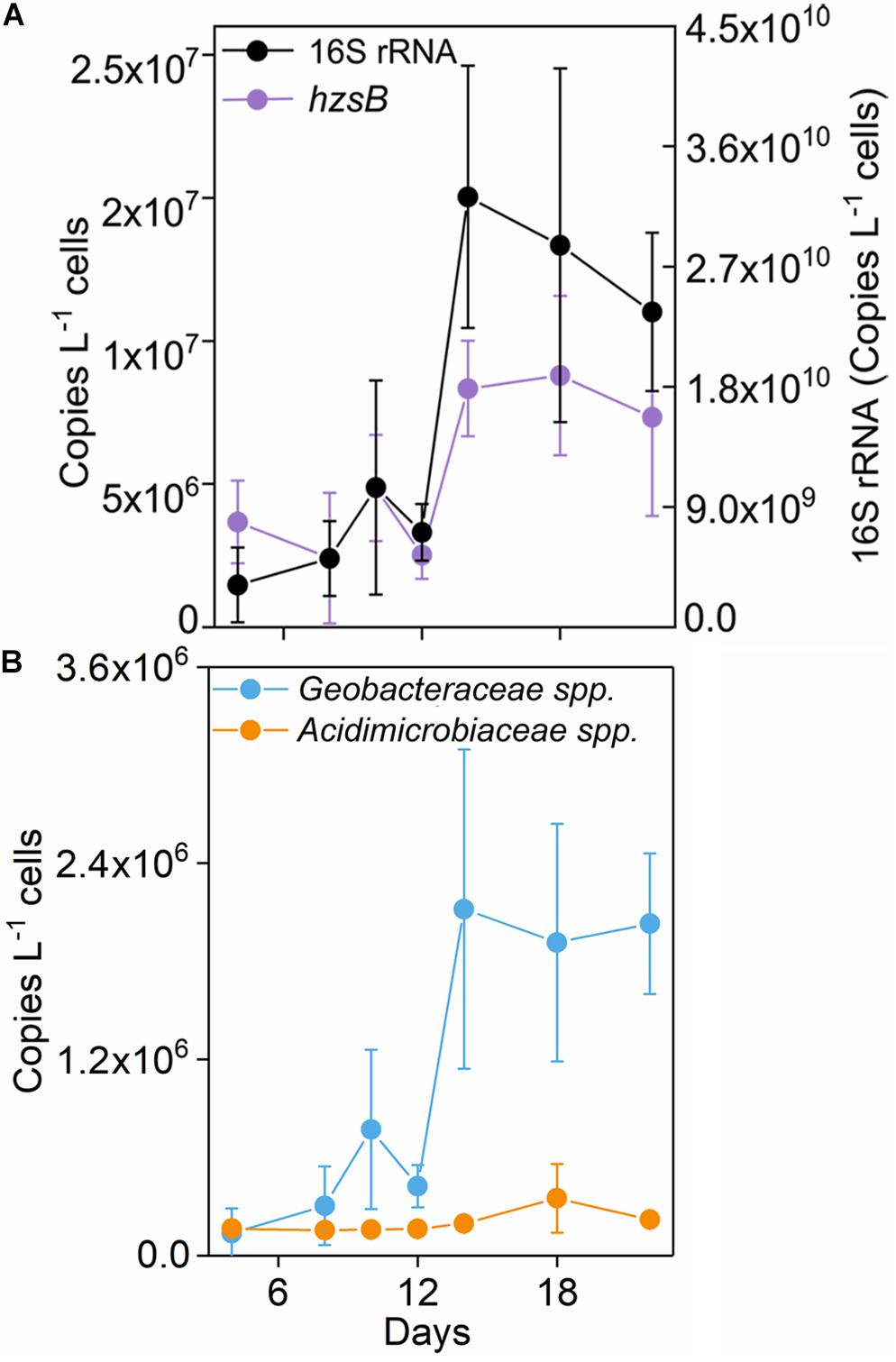
Figure 2. Copy numbers of 16S rRNA gene, and nosZ (A), Geobacteraceae spp., and Acidimicrobiaceae spp. (B) in the treatment of Fe(III) + NH4+ during 22-day incubation. Error bars represent standard deviations of three replication.
The abundance of Geobacteraceae spp. was about 2.03 × 106 copies L–1 medium after 22-day incubation in the treatment of Fe(III) + NH4+ (Figure 2B). By contrast, Acidimicrobiaceae spp. kept constantly low abundance throughout the incubation (Figure 2B).
Shift of Bacterial Community Composition
In the initial inoculant, 42.94% of the total bacterial community were affiliated to the family of Ignavibacteriaceae, followed by unclassified Anaerolineae (23.68%) and unclassified Chlorobi (8.02%) (Figure 3). While in the treatment of Fe(III) + NH4+, Geobacteraceae was the family with the highest relative abundance, followed by Comamonadaceae, Pelobacteraceae, and Pseudomonadaceae (Figure 3). These four families occupied up to 96.13% of the total microbial community (Figure 3).
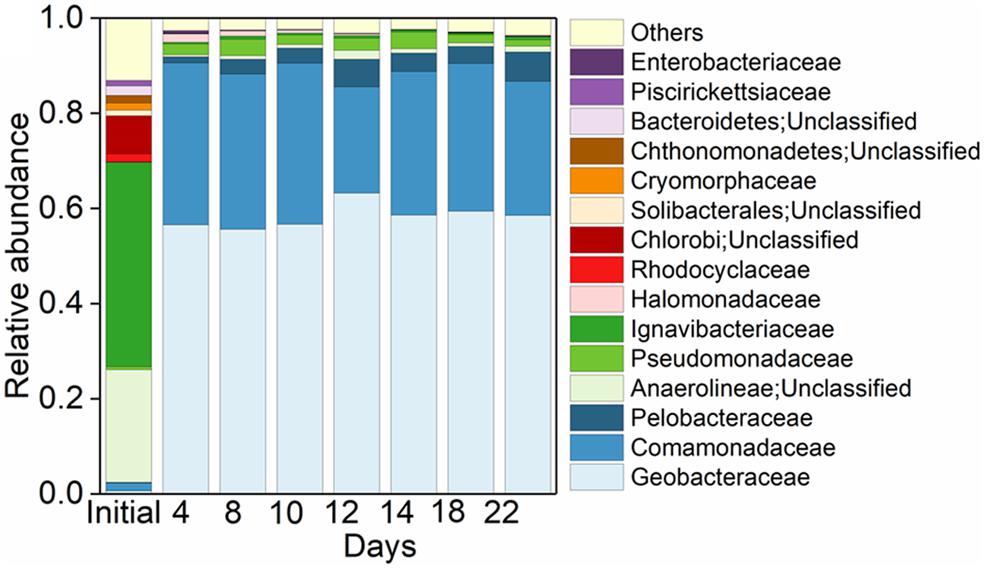
Figure 3. Pattern of microbial community in treatments. Top 15 taxa at family level in the initial Anammox inoculant and the Anammox inoculant-based treatment of Fe(III) + NH4+ during the 22-day incubation.
Shift in the Relative Abundances of Iron(III)-Reducers and Anammox Bacteria
The detected iron(III) reducers included Geobacter, Pseudomonas, Clostridium, Bacillus, Thiobacillus, unclassified Geobacteraceae, Desulfotomaculum, Desulfovibrio, Desulfobulbus, and Pelobacter in the treatment of Fe(III) + NH4+ (Figure 4A). In comparison with the initial inoculant, the genera of Geobacter, Pseudomonas, Clostridium, Desulfovibrio, Desulfotomaculum, and Pelobacter were significantly enriched, while the relative abundance of Bacillus and Thiobacillus decreased in the treatment of Fe(III) + NH4+ during the incubation (Figure 4A). Of all the iron(III) reducers, the change in relative abundances of Geobacter and unclassified Geobacteraceae were significantly (p < 0.05) correlated with the 30N2 and 29N2 production rates in the treatment of Fe(III) + NH4+ (Figure 4B).
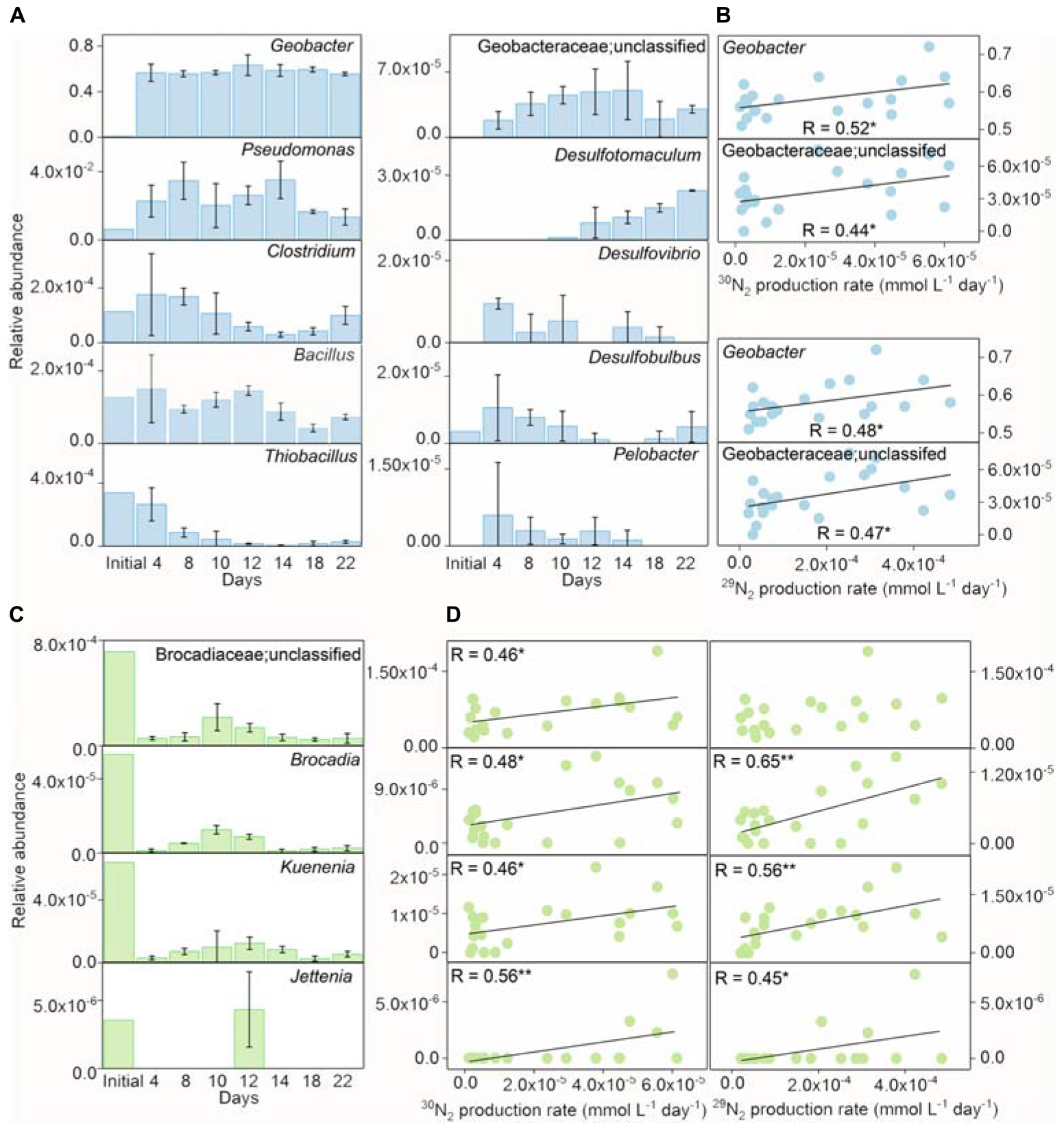
Figure 4. The relative abundances of iron(III) reducers and Anammox bacteria during incubation. The relative abundances of iron(III) reducers (A) and the correlation between the relative abundances of Geobacter/unclassified Geobacteraceae and 15N2 production rates (B) in the inoculant-based treatment of Fe(III) + NH4+ during 22-day incubation. Error bars represent standard deviations of three replications. The relative abundances of Brocadiaceae (unclassified), Brocadia, Kuenenia, and Jettenia (C), and the correlation between the relative abundance of Geobacter and 15N2 production rates (D) in the inoculant-based treatment of Fe(III) + NH4+. Error bars represent standard deviations of three replication. “*” and “**” represent a statistically significant relationship between relative abundances of iron(III) reducers, Anammox bacteria, and 15N2 production, which is denoted at p < 0.05 and p < 0.01, respectively.
The Anammox-relevant Planctomycetes remained with low relative abundances in the treatment of Fe(III) + NH4+ after 22-day incubation. Dynamics of relative abundances of Anammox-relevant taxa, including unclassified Brocadiaceae, Brocadia, Kuenenia, and Jettenia, displayed a similar pattern in the treatment of Fe(III) + NH4+. These relative abundances of Anammox bacteria reached a peak on days 10–12 and then decreased during the incubation (Figure 4C). The relative abundances of these Anammox bacteria significantly (p < 0.05) correlated with the rates of 30N2 and 29N2 production (Figure 4D).
Discussion
Feammox is a recently identified pathway of dinitrogen generation, expecting to be applied to remove nitrogen from a wastewater treatment system. In this study, we aimed to verify the occurrence of Feammox and investigate the Feammox-associated microbes in the sludge of a wastewater treatment system. Detection of 15N2 production from sludge amended with iron(III) indicated the existence of Feammox in the incubation. Anammox bacteria such as Brocadiaceae, Kuenenia, and Jettenia and iron(III) reducers including Geobacter and unclassified Geobacteraceae were found potentially involved in the Feammox process.
The Feammox incubation was established using the sludge as inoculant, which was subjected to three generations with freshly prepared medium under anaerobic condition. As a result, the major electron acceptor for anaerobic oxidation of 15N-NH4+ was ferrihydrite during the incubation. Previous reports suggested that Anammox can be linked to the microbial reduction of natural organic matters (Rios-Del Toro et al., 2018), likely originating from the breakdown of dead biomass, for example, carbohydrate residues including cellulose and lignin (Siemann et al., 2012). The transformation between oxidized and reduced state enables the quinone group-abundant organic matters serving as electron shuttles to mediate anaerobic oxidation of ammonium to N2 (Westereng et al., 2015; Rios-Del Toro et al., 2018). However, the standard Gibbs free energy released from this process (NH4+ + 1.5 quinone-NOMox → 0.5N2 + 1.5 quinoneH2-NOMred + 4H+) ranged from 5.8 kJ mol–1 to −124.6 kJ mol–1 (Rios-Del Toro et al., 2018), greatly lower than that produced from the Feammox process [3Fe(OH)3 + 5H+ + NH4+ → 3Fe2+ + 9H2O + 0.5N2, ΔrGm = −245 kJ mol–1; 6Fe(OH)3 + 10H+ + NH4+ → 6Fe2+ + 16H2O + NO2–, ΔrGm = −164 kJ mol–1; 8Fe(OH)3 + 14H+ + NH4+ → 8Fe2+ + 21H2O + NO3–, ΔrGm = −207 kJ mol–1] (Yang et al., 2012). Therefore, Anammox was probably coupled to ferrihydrite reduction during the incubation. The significant accumulation of 30N2 provided a solid evidence for the occurrence of Feammox in the treatment of Fe(III) + NH4+. Codenitrification is another potential source of 30N2 (Laughlin and Stevens, 2002). However, it can be ruled out in this study because other 15N-labeled nitrogen compounds (e.g., hydrazine and amino compounds) that could reduce 15NO2– and 15NO3– to N2 were not available in the culture. Under these conditions, direct N2 production from Feammox, or Feammox-produced NO2– or NO3– followed by denitrification or Anammox are the possible pathways for 30N2 generation, supporting the occurrence of Feammox in the treatment of Fe(III) + NH4+. The positive correlation (p < 0.0001; Figures 1D,E) between Fe(III) reduction and 30N2/29N2 production rates further verified the existence of Feammox in the treatment of Fe(III) + NH4+ during the anoxic incubation.
The amount of 15N2, N2O, and 15NOx– (3.63 μmol L–1; Supplementary Table 3) produced from the treatment of Fe(III) + NH4+ was far less than that of NH4+ depleted during the incubation, indicating that the majority of NH4+ was assimilated into microbial biomass (Tupas and Koike, 1990). The abundant genera such as Pseudomonas and Bacillus detected in this study, which are able to assimilate ammonia during their growth (Kim and Hollocher, 1982; Kanamori et al., 1989), may be the major NH4+ consumers in the treatment of Fe(III) + NH4+.
The molar ratio of reduced Fe (III) to the total NH4+ oxidation was about 15.41 in the Fe(III) + NH4+ treatment (Figure 1), which did not match the stoichiometry (ranging from 3 to 8) in the three Feammox equations (Yang et al., 2012; Ding et al., 2014; Zhou et al., 2016). This suggested that only a minor fraction of reduced Fe(III) was linked to the NH4+ oxidation in the Fe(III) + NH4+ treatment. According to the thermodynamic calculations, the amount of iron reduction associated with Feammox was 0.36–0.96 mmol L–1, accounting for 19.67–52.46% of total Fe(III) reduction in the treatment of Fe(III) + NH4+. Thus, a majority of the reduced Fe(III) was linked to the oxidation of other substrates mediated by microorganisms. Because exogenous organic matter was not added in the treatment of Fe(III) + NH4+, the substrates might be organic compounds sourced from the dead biomass or extracellular secretion from the microbes. Among all the iron(III)-reducing bacteria, the family of Geobacteraceae was abundant in the treatment of Fe(III) + NH4+ (Figure 3). The genus of Geobacter is able to reduce Fe(III) associated with organic substrate oxidation to support its growth (Lovley, 1991). The relative abundance of Geobacteraceae showed an increase on days 10 and 12, which was in agreement with the rapidly increasing copy number of Geobacter after 12-day incubation in the treatment of Fe(III) + NH4+ (Figures 2B, 3, 4A), indicating Geobacter was the dominant genus in Geobacteraceae. The positive correlation between the abundance of Geobacter and accumulation of 15N2 in the treatment of Fe(III) + NH4+ (Figure 4B) suggested that Geobacter may exert a potential role in Feammox.
The abundance of Anammox bacteria, including Brocadiaceae, Kuenenia, and Jettenia, showed significant correlation with 15N2 production in the treatment of Fe(III) + NH4+ (Figure 4D), suggesting Anammox bacteria were linked with Feammox during the incubation. Although the mechanism about the role of Anammox bacteria as Feammox players was still unknown, a variety of reports have shown their versatile metabolism. Firstly, Anammox bacteria are capable of anaerobically oxidizing ammonium via anammoxosome coupled with other electron acceptors such as sulfate in addition to nitrite (Liu et al., 2008; Rikmann et al., 2014). The ΔGo of sulfate-reducing anaerobic ammonium oxidation proceeded by Anammox species Anammoxoglobus sulfate (2NH4+ + SO42– → So + N2 + 4H2O ΔGo = −46 kJ mol–1; 8NH4+ + 3SO42– → HS– + 4N2 + 12H2O + 5H+ ΔGo = −22 kJ mol–1) is obviously lower than that of Feammox (Liu et al., 2008; Rikmann et al., 2014), suggesting that Anammox bacteria in the enrichment should favor Feammox. Secondly, genera of Brocadia and Kuenenia have iron(III)-reducing ability using organic matter (e.g., formate, acetate, and propionate) as electron donor (Graaf et al., 1996; Zhao et al., 2014); 80% of the ferric iron reductase in these Anammox bacteria locates in the membrane fraction and part of them termed as dissimilatory ferric iron reductases are the essential terminal reductase of the Fe(III) respiratory pathway in iron(III)-reducing bacteria (Schröder et al., 2003; Zhao et al., 2014). It provided a cue that the Anammox bacteria detected in our study, including Brocadiaceae, Kuenenia, and Jettenia, were capable of reducing iron(III) linked to oxidizing ammonium anaerobically at same time. Moreover, several publications demonstrated the potential role of Anammox bacteria in Feammox based on the increase in the N2 production after amendment with iron(III) oxides in the Anammox sludge (Chen et al., 2014; Wang et al., 2016; Li H. et al., 2018; Li X. et al., 2018; Yin et al., 2019). The Feammox bacteria Acidimicrobiaceae sp. were previously identified in acid soil with pH between 3.5 and 4.5 (Huang and Jaffé, 2014); however, the gene copy of the Acidimicrobiaceae spp. was extremely low (Figure 2B), and members of this family were not detected via Illumina sequencing in this study, which might indicate that this reported family made very little contribution to Feammox-linked N2 production under neutral condition. In addition, the aerobic ammonium-oxidizing bacteria Nitrosomonas spp. were found to be highly enriched in the ammonium-containing anoxic condition (Lek Noophan et al., 2009), whereas they were not detected through Illumina sequencing and amoA-based qPCR (data not shown) in the treatment of Fe(III) + NH4+ in this study. All of these disclosed that the Anammox bacteria Brocadiaceae, Kuenenia, and Jettenia had potential for Feammox-associated anaerobic ammonium oxidation (Figure 5A).
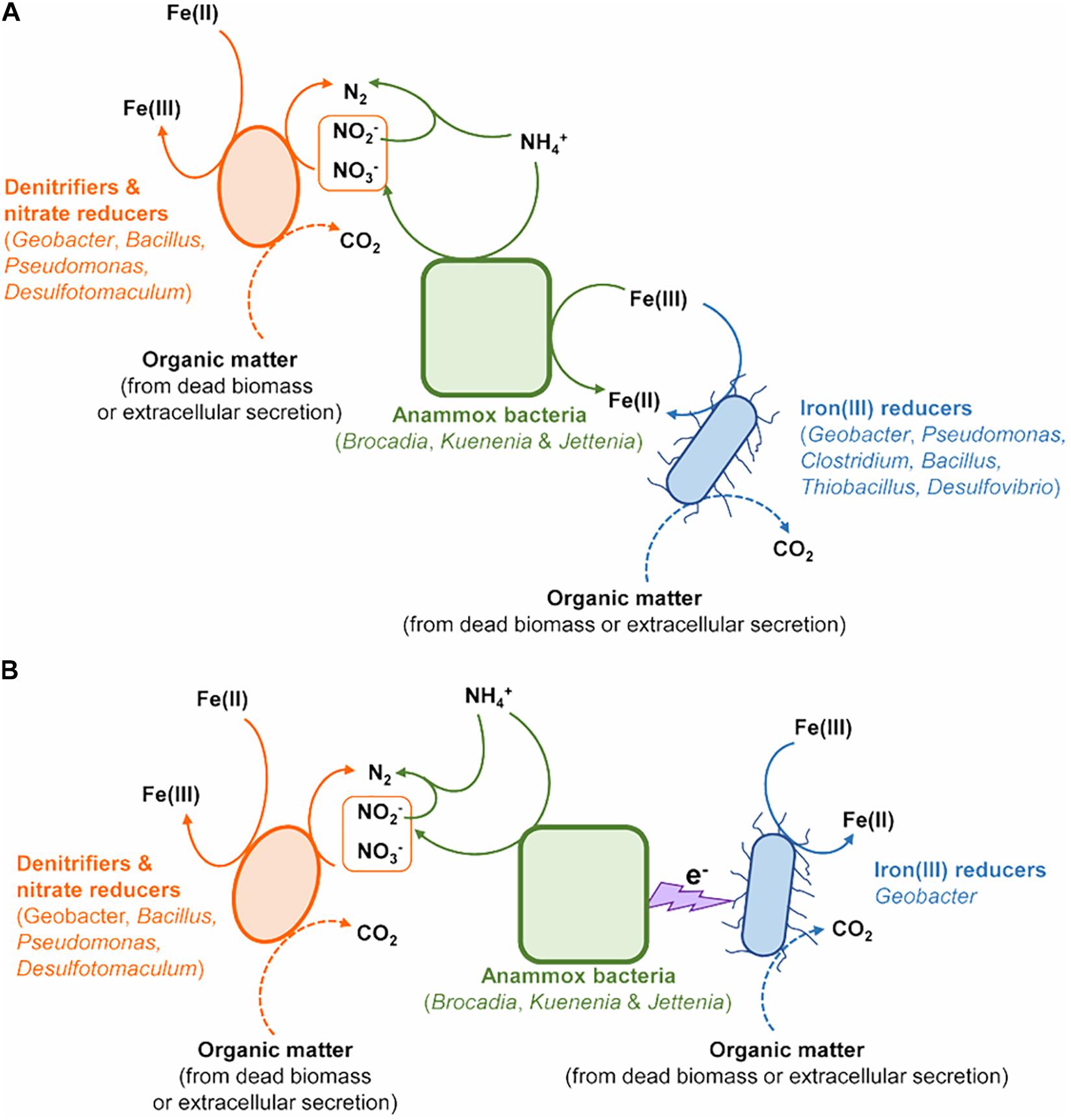
Figure 5. The potential models of microorganisms contributing to N removal via Feammox. (A) Anammox bacteria directly involved in the Feammox-associated N removal. (B) Interaction between Anammox bacteria and Geobacter linked to Feammox-associated N removal.
Cooperation between Anammox bacteria and iron(III)-reducers such as Geobacter could also complete the Feammox process (Figure 5B). The conductive pili of Geobacter provide the chance to extend electron transfer ability beyond the outer surface of their cells (Reguera et al., 2005). These pili offer the possibility for Geobacter to accept electrons from anaerobic ammonium oxidation by Anammox bacteria through periplasmic or outer membrane electron transfer protein (Reguera et al., 2005). The increase in the gene copy numbers of nirS, nosZ, hzsB, and relative abundances in nitrate reducers/denitrifiers (including Geobacter, Pseudomonas, Bacillus, Clostridium, and Desulfotomaculum) (Zhou et al., 2019) further indicated the contribution of denitrification, nitrate reduction, Anammox and nitrate reduction dependent iron(II) oxidation to N turnover in the Fe(III) + NH4+ treatment during the incubation (Figures 5A,B).
Potential Feammox rate was estimated with a value of 0.49 μg N kg–1 d–1 based on the 30N2 production rates, which was comparable to that reported in paddy soil (0.17–0.59 μg N kg–1 d–1), intertidal wetland (0.24–0.36 μg N kg–1 d–1), and tropical forest soil (about 0.32 mg N kg–1 d–1) (Clement et al., 2005; Yang et al., 2012; Ding et al., 2014; Li et al., 2019). However, the contribution of Feammox to N loss was much lower than that of Anammox enrichment from the sludge (4.1 × 106 μg N kg–1 d–1) (Chen et al., 2014), suggesting a substantially low N removal efficiency via Feammox. Furthermore, the minor contribution ratio of Feammox to N loss in this study was inconsistent with the previous reports in the Anammox sludge, which showed that Fe(III) addition increased the N removal up to 0.8 × 106 μg N kg–1 d–1 (Chen et al., 2014; Wang et al., 2016). The significantly lower relative abundance of Anammox bacteria in the treatment of Fe(III) + NH4+ (Figures 2A, 3, 4C) than the those reported in the previous wastewater treatment system (Chen et al., 2014; Wang et al., 2016) may be an important reason for low amount of N2 via Feammox. Firstly, limited capability of substrate utilization by Anammox bacteria led to slow growth rate and long doubling time (Jetten et al., 2005). As a result, these Anammox bacteria were overwhelmed by iron(III) reducers that could efficiently obtain energy from dissimilatory iron(III) reduction. Secondly, the relative abundances of the Brocadiaceae, Kuenenia, and Jettenia decreased in the treatment of Fe(III) + NH4+ (Figure 4C), which was likely due to the accumulation of NO2–/NO3– after 12-day incubation (Supplementary Table 3). Iron(II) coexistence with NO2– (Supplementary Figure 2), which might lead to the NOx– dependent Fe(II) oxidation, can severely inhibit the activity of Anammox bacteria (Zhao et al., 2014). Thirdly, dilution of Anammox bacteria via three generations of subculture may be another important reason for the low activity of Feammox-associated N2 production decreased during the incubation. Besides, Fe3O4 can form from rapid iron(III) reduction with the production of Fe(II) absorbed on the ferric oxides surface and then improve N removal during Feammox process (Kappler et al., 2014; Li H. et al., 2018; Li X. et al., 2018). Hence, it can be inferred that Fe(II) was accumulated with relatively low extent after subculturing in the treatment of Fe(III) + NH4+; therefore, the amount of Fe3O4 produced in the treatment was lower compared to the sludge reactor in continuous operation.
Conclusion
This study demonstrated the occurrence of Feammox in Anammox inoculant-based enrichment. The relative abundances of Geobacter and Anammox bacteria such as Brocadiaceae, Kuenenia, and Jettenia were significantly correlated with 15N2 production rates, indicating their potential role in Feammox-involved N removal. We proposed that sole Anammox bacteria or cooperation between Anammox bacteria and Geobacter or unclassified Geobacteraceae could complete the Feammox process during the incubation. Our results suggested the potential role of Anammox bacteria in the nitrogen removal via the Feammox process.
Data Availability Statement
The datasets presented in this study can be found in online repositories. The names of the repository/repositories and accession number(s) can be found in the article/Supplementary Material.
Author Contributions
X-RY, HL, and G-WZ did the experiments, conceived and designed the project, and analyzed the data. J-QS gave assistance in lab work and laboratory analyses. X-RY wrote the manuscript. X-RY, HL, J-QS, and G-WZ revised the manuscript. All authors read and approved the final manuscript.
Funding
This work was supported by the National Natural Science Foundation of China (41771285 and 42021005).
Conflict of Interest
The authors declare that the research was conducted in the absence of any commercial or financial relationships that could be construed as a potential conflict of interest.
Publisher’s Note
All claims expressed in this article are solely those of the authors and do not necessarily represent those of their affiliated organizations, or those of the publisher, the editors and the reviewers. Any product that may be evaluated in this article, or claim that may be made by its manufacturer, is not guaranteed or endorsed by the publisher.
Acknowledgments
We thank Han Zhang (Institute of Urban Environment, Chinese Academy of Sciences) for kindly supporting the determination of δ15N-nitrate and δ15N-nitrite.
Supplementary Material
The Supplementary Material for this article can be found online at: https://www.frontiersin.org/articles/10.3389/fmicb.2021.717249/full#supplementary-material
Supplementary Figure 1 | The dynamics of 29N2 and 30N2 production in the treatment of Fe(III) + NH4+ during 22-day incubation.
Supplementary Figure 2 | The time course of abundances of nirS and nosZ, and concentrations of NO3– and NO2– in the treatment of Fe(III) + NH4+ during 22-day incubation.
Supplementary Table 1 | Characteristic of the initial inoculant slurry.
Supplementary Table 2 | Primers and qPCR processes used in this study.
Supplementary Table 3 | The concentrations of 15NOx– in the Fe(III) + NH4+ treatment after 22-day incubation.
Footnotes
References
Cervantes, F. J., Meza-Escalante, E. R., Texier, A. C., and Gómez, J. (2009). Kinetic limitations during the simultaneous removal of p-cresol and sulfide in a denitrifying process. J. Ind. Microbiol. Biotechnol. 36, 1417–1424. doi: 10.1007/s10295-009-0628-6
Chen, H., Yu, J. J., Jia, X. Y., and Jin, R. C. (2014). Enhancement of anammox performance by Cu(II), Ni(II) and Fe(III) supplementation. Chemosphere 117, 610–616. doi: 10.1016/j.chemosphere.2014.09.047
Clement, J., Shrestha, J., Ehrenfeld, J., and Jaffe, P. (2005). Ammonium oxidation coupled to dissimilatory reduction of iron under anaerobic conditions in wetland soils. Soil Biol. Biochem. 37, 2323–2328. doi: 10.1016/j.soilbio.2005.03.027
Ding, B., Chen, Z., Li, Z., Qin, Y., and Chen, S. (2019). Nitrogen loss through anaerobic ammonium oxidation coupled to Iron reduction from ecosystem habitats in the Taihu estuary region. Sci. Total Environ. 662, 600–606. doi: 10.1016/j.scitotenv.2019.01.231
Ding, B., Li, Z., and Qin, Y. (2017). Nitrogen loss from anaerobic ammonium oxidation coupled to Iron(III) reduction in a riparian zone. Environ. Pollut. 231(Pt 1), 379–386. doi: 10.1016/j.envpol.2017.08.027
Ding, L. J., An, X. L., Li, S., Zhang, G. L., and Zhu, Y. G. (2014). Nitrogen loss through anaerobic ammonium oxidation coupled to iron reduction from paddy soils in a chronosequence. Environ. Sci. Technol. 48, 10641–10647. doi: 10.1021/es503113s
Graaf, A. A., Bruijn, P., Robertson, L. A., Jetten, M. S. M., and Kuenen, J. G. (1996). Autotrophic growth of anaerobic ammonium-oxidizing microorganisms in a fluidized bed reactor. Microbiology 142, 2187–2196. doi: 10.1099/13500872-142-8-2187
Huang, S., and Jaffé, P. R. (2014). Characterization of incubation experiments and development of an enrichment culture capable of ammonium oxidation under iron reducing conditions. Biogeosciences 11, 12295–12321. doi: 10.5194/bgd-11-12295-2014
Jetten, M. S. M., Cirpus, I., Kartal, B., Niftrik, L., Pas-Schoonen, K. T., Sliekers, O., et al. (2005). 1994–2004: 10 years of research on the anaerobic oxidation of ammonium. Biochem. Soc. Trans. 33, 119–123. doi: 10.1042/BST0330119
Kanamori, K., Weiss, R. L., and Roberts, J. D. (1989). Ammonia assimilation pathways in nitrogen-fixing Clostridium kluyverii and Clostridium butyricum. J. Bacteriol. 171, 2148–2154.
Kappler, A., Schink, B., and Newman, D. K. (2005). Fe(III) mineral formation and cell encrustation by nitrate dependent Fe(II) oxidizers the nitrate dependent Fe(II) oxidizer strain BoFeN1. Geobiology 3, 235–245. doi: 10.1111/j.1472-4669.2006.00056.x
Kappler, A., Wuestner, M. L., Ruecker, A., Harter, J., Halama, M., and Behrens, S. (2014). Biochar as an electron shuttle between bacteria and Fe(III) minerals. Environ. Sci. Technol. Lett. 1, 339–344. doi: 10.1021/ez5002209
Kim, C. H., and Hollocher, T. C. (1982). 13N isotope studies on the pathway of ammonia assimilation in Bacillus megaterium and Escherichia coli. J. Bacteriol. 151, 358–366. doi: 10.1128/JB.151.1.358-366.1982
Klueglein, N., Picardal, F., Zedda, M., Zwiener, C., and Kappler, A. (2015). Oxidation of Fe(II)-EDTA by nitrite and by two nitrate-reducing Fe(II)-oxidizing Acidovorax strains. Geobiology 13, 198–207. doi: 10.1111/gbi.12125
Laughlin, R. J., and Stevens, R. J. (2002). Evidence for fungal dominance of denitrification and codenitrification in a grassland soil. Soil Sci. Soc. Am. J. 66, 1540–1548. doi: 10.2136/sssaj2002.1540
Lek Noophan, P., Sripiboon, S., Damrongsri, M., and Munakata-Marr, J. (2009). Anaerobic ammonium oxidation by Nitrosomonas spp. and anammox bacteria in a sequencing batch reactor. J. Environ. Manage. 90, 967–972. doi: 10.1016/j.jenvman.2008.03.003
Li, H., Chi, Z., and Yan, B. (2018). Insight into the impact of Fe3O4 nanoparticles on anammox process of subsurface-flow constructed wetlands under long-term exposure. Environ. Sci. Pollut. Res. Int. 25, 29584–29592. doi: 10.1007/s11356-018-2975-1
Li, H., Su, J. Q., Yang, X. R., Zhou, G. W., Lassen, S. B., and Zhu, Y. G. (2019). RNA stable isotope probing of potential Feammox population in paddy soil. Environ. Sci. Technol. 53, 4841–4849. doi: 10.1021/acs.est.8b05016
Li, X., Hou, L., Liu, M., Zheng, Y., Yin, G., Lin, X., et al. (2015). Evidence of nitrogen loss from anaerobic ammonium oxidation coupled with ferric iron reduction in an intertidal wetland. Environ. Sci. Technol. 49, 11560–11568. doi: 10.1021/acs.est.5b03419
Li, X., Huang, Y., Liu, H. W., Wu, C., Bi, W., Yuan, Y., et al. (2018). Simultaneous Fe(III) reduction and ammonia oxidation process in Anammox sludge. J. Environ. Sci. 64, 42–50. doi: 10.1016/j.jes.2017.01.002
Liu, S., Yang, F., Gong, Z., Meng, F., Chen, H., Xue, Y., et al. (2008). Application of anaerobic ammonium-oxidizing consortium to achieve completely autotrophic ammonium and sulfate removal. Bioresour. Technol. 99, 6817–6825. doi: 10.1016/j.biortech.2008.01.054
Lovley, D. R. (1991). Dissimilatory Fe(III) and Mn(IV) reduction. Mircrobiol. Rev. 55, 259–287. doi: 10.1016/S0065-2911(04)49005-5
Lovley, D. R., and Phillips, E. J. (1988). Novel mode of microbial energy metabolism: organic carbon oxidation coupled to dissimilatory reduction of iron or manganese. Appl. Environ. Microbiol. 54, 1472–1480.
Reguera, G., McCarthy, K. D., Mehta, T., Nicoll, J. S., Tuominen, M. T., and Lovley, D. R. (2005). Extracellular electron transfer via microbial nanowires. Nature 435, 1098–1101. doi: 10.1038/nature03661
Ren, G. D., Zhang, H. Y., Lin, X. G., Zhu, J. G., and Jia, Z. J. (2014). Response of phyllosphere bacterial communities to elevated CO2 during rice growing season. Appl. Microbiol. Biotechnol. 98, 9459–9471. doi: 10.1007/s00253-014-5915-0
Rikmann, E., Zekker, I., Tomingas, M., Vabamae, P., Kroon, K., Saluste, A., et al. (2014). Comparison of sulfate-reducing and conventional Anammox upflow anaerobic sludge blanket reactors. J. Biosci. Bioeng. 118, 426–433. doi: 10.1016/j.jbiosc.2014.03.012
Rios-Del Toro, E. E., and Cervantes, F. J. (2016). Coupling between anammox and autotrophic denitrification for simultaneous removal of ammonium and sulfide by enriched marine sediments. Biodegradation 27, 107–118. doi: 10.1007/s10532-016-9759-4
Rios-Del Toro, E. E., Valenzuela, E. I., Ramírez, J. E., López-Lozano, N. E., and Cervantes, F. J. (2018). Anaerobic ammonium oxidation linked to microbial reduction of natural organic matter in marine sediments. Environ. Sci. Technol. Lett. 5, 571–577. doi: 10.1021/acs.estlett.8b00330
Sawayama, S. (2006). Possibility of anoxic ferric ammonium oxidation. J. Biosci. Bioeng. 101, 70–72. doi: 10.1263/jbb.101.70
Schröder, I., Johnson, E., and de Vries, S. (2003). Microbial ferric iron reductases. FEMS Microbiol. Rev. 27, 427–447. doi: 10.1016/s0168-6445(03)00043-3
Siemann, S., Caron, F., Riopel, R., and Borraro, V. (2012). Laboratory study on the impact of pH and salinity on the fluorescence signal of natural organic matter (NOM) relevant to groundwaters from a Canadian shield sampling site. Water Qual. Res. J. Can. 47, 131–139. doi: 10.2166/wqrjc.2012.028
Strous, M., Kuenen, J. G., Furerst, J. A., Wagner, M., and Jetten, M. S. M. (2002). The Anammox case e a new experimental manifesto for microbiological ecophysiology. Antonie Van Leeuwenhoek 81, 693–702. doi: 10.1023/A:1020590413079
Su, J. Q., Wei, B., Ou-Yang, W. Y., Huang, F. Y., Zhao, Y., Xu, H. J., et al. (2015). Antibiotic resistome and its association with bacterial communities during sewage sludge composting. Environ. Sci. Technol. 49, 7356–7363. doi: 10.1021/acs.est.5b01012
Tunney, M. M., Einarsson, G. G., Wei, L., Drain, M., Klem, E. R., Cardwell, C., et al. (2013). Lung microbiota and bacterial abundance in patients with bronchiectasis when clinically stable and during exacerbation. Am. J. Respir. Cell Mol. Biol. 187, 1118–1126. doi: 10.1164/rccm.201210-1937OC
Tupas, L., and Koike, I. (1990). Amino acid and ammonium utilization by heterotrophic marine bacteria grown in enriched seawater. Limnol. Oceanogr. 35, 1145–1155. doi: 10.4319/lo.1990.35.5.1145
Wang, Q., Garrity, G. M., Tiedje, J. M., and Cole, J. R. (2007). Naive Bayesian classifier for rapid assignment of rRNA sequences into the new bacterial taxonomy. Appl. Environ. Microbiol. 73, 5261–5267. doi: 10.1128/AEM.00062-07
Wang, X., Shu, D., and Yue, H. (2016). Taxonomical and functional microbial community dynamics in an Anammox-ASBR system under different Fe (III) supplementation. Appl. Microbiol. Biotechnol. 100, 10147–10163. doi: 10.1007/s00253-016-7865-1
Westereng, B., Cannella, D., Wittrup Agger, J., Jorgensen, H., Larsen Andersen, M., Eijsink, V. G., et al. (2015). Enzymatic cellulose oxidation is linked to lignin by long-range electron transfer. Sci. Rep. 5:18561. doi: 10.1038/srep18561
Yang, W. H., Weber, K. A., and Silver, W. L. (2012). Nitrogen loss from soil through anaerobic ammonium oxidation coupled to iron reduction. Nat. Geosci. 5, 538–541. doi: 10.1038/ngeo1530
Yin, S., Li, J., Dong, H., and Qiang, Z. (2019). Enhanced nitrogen removal through marine anammox bacteria (MAB) treating nitrogen-rich saline wastewater with Fe(III) addition: nitrogen shock loading and community structure. Bioresour. Technol. 287:121405. doi: 10.1016/j.biortech.2019.121405
Zhang, Z. J., Li, Y., Chen, S., Wang, S., and Bao, X. (2012). Simultaneous nitrogen and carbon removal from swine digester liquor by the Canon process and denitrification. Bioresour. Technol. 114, 84–89. doi: 10.1016/j.biortech.2012.03.006
Zhao, R., Zhang, H., Li, Y., Jiang, T., and Yang, F. (2014). Research of iron reduction and the iron reductase localization of anammox bacteria. Curr. Microbiol. 69, 880–887. doi: 10.1007/s00284-014-0668-7
Zhou, G. W., Yang, X. R., Li, H., Marshall, C. W., Zheng, B. X., Yan, Y., et al. (2016). Electron shuttles enhance anaerobic ammonium oxidation coupled to iron(III) reduction. Environ. Sci. Technol. 50, 9298–9307. doi: 10.1021/acs.est.6b02077
Keywords: Feammox, Anammox bacteria, ammonium oxidation, iron(III) reduction, 15N2 production
Citation: Yang X-R, Li H, Su J-Q and Zhou G-W (2021) Anammox Bacteria Are Potentially Involved in Anaerobic Ammonium Oxidation Coupled to Iron(III) Reduction in the Wastewater Treatment System. Front. Microbiol. 12:717249. doi: 10.3389/fmicb.2021.717249
Received: 30 May 2021; Accepted: 04 August 2021;
Published: 10 September 2021.
Edited by:
Lei Yan, Heilongjiang Bayi Agricultural University, ChinaReviewed by:
Nan Li, Tianjin University, ChinaKevin Thomas Finneran, Clemson University, United States
Copyright © 2021 Yang, Li, Su and Zhou. This is an open-access article distributed under the terms of the Creative Commons Attribution License (CC BY). The use, distribution or reproduction in other forums is permitted, provided the original author(s) and the copyright owner(s) are credited and that the original publication in this journal is cited, in accordance with accepted academic practice. No use, distribution or reproduction is permitted which does not comply with these terms.
*Correspondence: Guo-Wei Zhou, Z3d6aG91QGFodS5lZHUuY24=