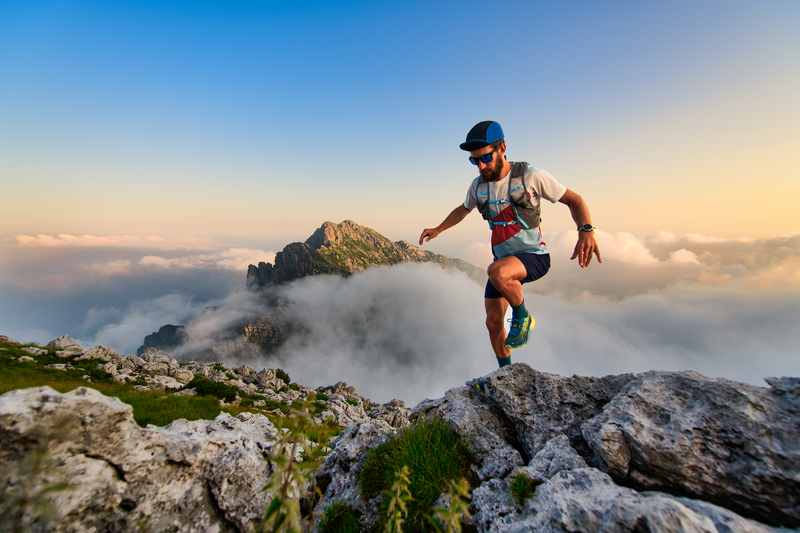
95% of researchers rate our articles as excellent or good
Learn more about the work of our research integrity team to safeguard the quality of each article we publish.
Find out more
ORIGINAL RESEARCH article
Front. Microbiol. , 20 October 2021
Sec. Systems Microbiology
Volume 12 - 2021 | https://doi.org/10.3389/fmicb.2021.711040
This article is part of the Research Topic Rumen Microbiome Dynamics and Their Implications in Health and Environment View all 16 articles
The objective of this study was to determine whether divergent feeding regimes during the first 41 weeks of the life of a calf are associated with long-term changes in the rumen microbiota and the associated fermentation end-products. Twenty-four calves (9 ± 5 days of age) were arranged in a 2 × 2 factorial design with two divergent treatments across three dietary phases. In phase 1 (P01), calves were offered a low-milk volume/concentrate starter diet with early weaning (CO) or high-milk volume/pasture diet and late weaning (FO). In phase 2 (P02), calves from both groups were randomly allocated to either high-quality (HQ) or low-quality (LQ) pasture grazing groups. In phase 3 (P03), calves were randomly allocated to one of two grazing groups and offered the same pasture-only diet. During each dietary phase, methane (CH4) and hydrogen (H2) emissions and dry matter intake (DMI) were measured in respiration chambers, and rumen samples for the evaluation of microbiota and short-chain fatty acid (SCFA) characterizations were collected. In P01, CO calves had a higher solid feed intake but a lower CH4 yield (yCH4) and acetate:propionate ratio (A:P) compared with FO calves. The ruminal bacterial community had lower proportions of cellulolytic bacteria in CO than FO calves. The archaeal community was dominated by Methanobrevibacter boviskoreani in CO calves and by Mbb. gottschalkii in FO calves. These differences, however, did not persist into P02. Calves offered HQ pastures had greater DMI and lower A:P ratio than calves offered LQ pastures, but yCH4 was similar between groups. The cellulolytic bacteria had lower proportions in HQ than LQ calves. In all groups, the archaeal community was dominated by Mbb. gottschalkii. No treatment interactions were observed in P02. In P03, all calves had similar DMI, CH4 and H2 emissions, SCFA proportions, and microbial compositions, and no interactions with previous treatments were observed. These results indicate that the rumen microbiota and associated fermentation end-products are driven by the diet consumed at the time of sampling and that previous dietary interventions do not lead to a detectable long-term microbial imprint or changes in rumen function.
The rumen is a fermentation chamber occupied by a diverse, interactive, and dynamic microbiota comprised of many species of bacteria, archaea, protozoa, and fungi (Hobson and Stewart, 1997). These microorganisms convert ingested feed into short-chain fatty acids (SCFA) and microbial biomass, which are the main source of energy and amino acids for ruminants (Puniya et al., 2015; Huws et al., 2018; Gruninger et al., 2019). Other fermentation end-products, including hydrogen (H2) and carbon dioxide (CO2), formic acid, and methyl groups, are utilized by methanogens to produce methane (CH4) (Moss et al., 2000; Liu and Whitman, 2008; Janssen, 2010). Methane production is both a loss of dietary gross energy (Bergman, 1990) and a greenhouse gas (Johnson and Johnson, 1995). Thus, manipulation of the ruminal ecosystem has been used in attempts to improve the efficiency of feed conversion and decrease environmental impacts (Mizrahi et al., 2021). However, manipulations in adult ruminants have shown limited and only short-term effects after treatment cessation (Weimer, 1998). The microbiota in the rumen of mature ruminants is characterized by a high degree of redundancy and resilience, which provides stability to the rumen environment and maintains the digestive function of the host across a range of feeding and management conditions (Weimer, 2015). These properties thus represent a barrier to manipulating rumen fermentation by selectively targeting groups of microorganisms. However, some studies in small ruminants suggest that in early life the rumen microbial community may be more plastic and, therefore, easier to manipulate (Yáñez-Ruiz et al., 2010; Abecia et al., 2014; De Barbieri et al., 2015).
During early postnatal life, the rumen microbiota of the young ruminant is very responsive to dietary interventions (Yáñez-Ruiz et al., 2010; Abecia et al., 2014; O’Hara et al., 2020). While sterile in utero (Malmuthuge and Griebel, 2018; Husso et al., 2021), the rumen of newborn animals undergoes a rapid microbial colonization during and after birth from maternal (Taschuk and Griebel, 2012; Yeoman et al., 2018) and environmental sources (Dehority and Orpin, 1997; Curtis and Sloan, 2004). After the initial colonization, microbial groups critical to the degradation of feed have been observed in the undeveloped rumen, as early as the third day of age (Fonty et al., 1987; Minato et al., 1992; Guzman et al., 2015; Wang et al., 2017). The rumen microbiota rapidly shifts toward obligate anaerobic microbes as young ruminants start to transition from milk to solid diets (Walters et al., 2011; Rey et al., 2014). In post-weaned calves, the consumption of solid diets is associated with a progressive shift in ruminal microbial composition toward a more diverse microbiota (Rey et al., 2014; Dias et al., 2017; Dill-McFarland et al., 2017). The ruminal microbiota in young ruminants acquires an adult-like composition as the solid feed intake increases between the weaning transition and 1 year of age (Dill-McFarland et al., 2019), with recent studies indicating that an increased solid feed intake can result in adult-like fermentation profiles (Cristobal-Carballo et al., 2019). As a result, dietary interventions, aimed at altering ruminal microbial composition and fermentation profiles, may be most effective during the weaning transition of young ruminants. However, there is little information available on the effect of early life nutrition of calves during the transition to weaning and immediately afterward on the rumen prokaryotic community and fermentation profiles. The aim of this study was to determine whether contrasting feeding regimes pre- and post-weaning could imprint the rumen microbial community and produce associated changes in rumen fermentation.
Animal procedures were reviewed and approved by the Grasslands Animal Ethics Committee (AE 13297) and complied with the institutional Codes of Ethical Conduct for the Use of Animals in Research, Testing and Teaching, as prescribed in the New Zealand Animal Welfare Act of 1999 and its amendments.
Twenty-four calves were randomly selected and balanced across dietary treatments from a parent production study using 200 Hereford–Friesian-cross female calves (Burggraaf et al., 2020). Treatments in the large production study were balanced for live weight and arrival date of the calves. The study was carried out in a 2 × 2 factorial design with different dietary treatments across three dietary phases. In phase 1 (P01, 0–14 weeks), calves were reared using either a low-milk volume and concentrate starter diet with early weaning (CO) or high-milk volume and pasture diet with later weaning (FO). In phase 2, post-weaning (P02, 14–19 weeks), each group of calves was evenly divided and randomly allocated to either a high-quality (HQ) or low-quality pasture (LQ) diet. The outcome was the generation of four groups in P02, where the main effects of pre-weaning rearing system and post-weaning diet quality and the interactions were compared. In phase 3 (P03, 30 to 41 weeks), all calves were randomly allocated to two groups that equally represented all four treatment groups and managed under commercial grazing conditions on the same farm. For this study, measurements and sampling were undertaken in week 9 (P01), week 19 (P02), and week 41 (P03).
Calves from P01 were managed in two pre-weaning rearing systems: FO calves were housed during week 1 and then moved to paddocks of ryegrass/white clover pasture from weeks 2 to 12. These calves were fed whole milk powder (WMP; Table 1; NZ Agbiz, Auckland, New Zealand) at 8.0 L/calf/day (1,000 g of WMP; 125 g/L of water), divided in two feeds for 5 weeks. In this group, the intake of WMP was increased from 5.0 to 8.0 L/calf/day during the first 2 weeks. Calves were fed 8.0 L once per day from weeks 5 to 9, then 4 L once a day for 2 weeks, then were gradually weaned over the following week. Calves from the CO group were housed on arrival and fed WMP at 4.0 L/calf/day (500 g WMP/day; mixed as per the FO group), divided in two feeds for 5 weeks and then once a day for 2 weeks, before abruptly weaning off milk at the end of week 7. This group was offered ad libitum starter concentrate (Table 1; Denver Stock Feeds, Palmerston North, New Zealand) from weeks 1 to 7, then calves were transferred to paddocks of ryegrass/white clover pasture with starter concentrate reduced to 1.5 kg/calf/day for 2 weeks and finally to 1 kg/calf/day for two more weeks until weaning off concentrate starter at week 12.
Table 1. Chemical composition (% of dry matter) of the whole milk powder (WMP), concentrate and pasturesa fed to calves in phase 1 (P01), pastures of highb (HQ) and low qualityc (LQ) in phase 2 (P02) and pasturesd fed to all calves in phase 3 (P03).
In P02, calves in the HQ and LQ groups were grazed on pastures of either high quality (HQ; irrigated pasture) or low quality (LQ; non-irrigated pasture). Calves grouped in LQ were also fed grass silage to meet dry matter intake (DMI) requirements. The botanical composition of high-quality pastures was 31.0% of ryegrass, 35.5% of white clover, 7.3% of herbs, 3.4% other grasses, and 22.4% of dead material (DM basis), while the botanical composition of non-irrigated low-quality pastures was of 16.3% of ryegrass, 4.0% of white clover, 1.3% of herbs, 16.0% other grasses, and 61.4% of dead material (DM basis) (Burggraaf et al., 2020). In P03, from approximately 7 months of age, the calves were randomly allocated to one of two groups which were balanced for all four previous treatments and grazed commercially on ryegrass/white clover pasture. Fresh water was available ad libitum at all times.
Enteric emissions [methane (CH4) and hydrogen (H2)] and animal performance [DMI and live weight (LW)] measurements were performed from the 24 selected calves, at weeks 9 (P01), 19 (P02), and 41 (P03). Before enteric emission measurements, calves were adapted to confinement conditions in covered yards as follows: in a group pen all together for the first 5 days and then in individual crates from days 5 to 7. Enteric emission measurements were carried out in open circuit respiratory chambers (Pinares-Patiño et al., 2012) over a 48-h period. The air flow through the chambers was set at 700, 1,000, and 1,200 L/min during the three measurement phases, respectively, to account for the increasing gas emissions as the solid feed intake of the calves increased. Calves entered the chambers in the morning (0900 h) when feed was offered: WMP (for FO calves in P01) and fresh solid feed (starter concentrate and/or pasture, depending on the phase and group). For LQ calves in P02, these animals received only low-quality grass (Table 1) during confinement and in respiration chambers; no grass silage was offered to these animals. Enteric emission measurements were paused for ∼45 min every morning to offer fresh feed and clean the chambers. During the adaptation and enteric emission measurement phases, pasture was cut daily, transported to the animal facility, and offered ad libitum. Water was available ad libitum.
Samples of concentrate and WMP were analyzed for chemical composition by wet chemistry (Hill Laboratories Ltd., Hamilton, New Zealand). Pasture samples of ryegrass/white clover-mixed sward were analyzed by near-infrared reflectance spectroscopy (NIRS; FeedTECH, Palmerston North, New Zealand). The chemical composition of the offered diets during the different rearing phases is shown in Table 1. During the gas measurement phases, DMI was calculated from the difference between the allowance and the residual feed. Milk DMI was not included in the calculation of the total DMI to estimate methane or hydrogen yield because most of the milk DM bypasses the rumen (Wise and Anderson, 1939) and, therefore, has little effect on rumen fermentation (Lane et al., 2000). Live weights were recorded a day before methane emission measurements during the morning and before feeding. Total daily production (g/day) of CH4 and H2 (pCH4 and pH2, respectively) were calculated from the enteric emission measurements (Pinares and Waghorn, 2014). Daily enteric emissions and animal performance parameters were used to calculate CH4 and H2 yield (yCH4 and yH2, respectively; g per kg DMI).
Rumen samples were collected in the morning via an oral stomach tube (Henderson et al., 2013) after enteric emission measurements and before feeding new milk and/or solid feed. Oral stomach tubing was performed using a stainless steel pipe (25 mm outside diameter, wall thickness 1.2 mm) measuring 520 mm in length with a “T” handle 350 mm from one end. The stainless steel pipe was used to guide the lavage tube over the back of the tongue to ensure it entered the rumen. The lavage tube (19 mm outside diameter) enabled contents to be aspirated using a 400-ml syringe from the center of the dorsal rumen. A modification of the technique was used at 9 and 19 weeks, where the stainless steel pipe was not used due to the size of the animals. Each sample of 10 to 30 ml of rumen fluid was subsampled for SCFA analysis and DNA extraction. For DNA analyses, 900 μl of each rumen fluid sample was snap frozen in a cool rack and immediately stored at −20°C until analysis. For SCFA analysis, 1.8 ml of each rumen sample was prepared as per Guyader et al. (2016). Gas chromatography was then used to analyze SCFA composition, as per Attwood et al. (1998), using a gas chromatograph (Model 6869, Hewlett-Packard, Montreal, QC, Canada) equipped with an auto-sampler, fitted with a Zebron ZB-FFAP 30.0 m × 0.53 mm I.D. × 1 μm film column (Phenomenex, Torrance, CA, United States) and a flame ionization detector set at 265°C.
DNA was extracted from 200 μl of thawed and vortexed rumen fluid samples using the phenol–chloroform, bead beating with filtration kit for purification II (PCQI) (Rius et al., 2012; Henderson et al., 2013). Primers used for PCR amplification of bacterial and archaeal 16S rRNA genes are listed in Supplementary Table S1. Amplification reactions used for PCR targeting the regions of bacterial (30 cycles) and archaeal (35 cycles) 16S rRNA genes were prepared in triplicate as described by Kittelmann et al. (2013). PCR products were pooled, and the correct size products (∼500 bp) were verified by agarose gel electrophoresis and quantified by fluorescence using the Quant-iT dsDNA BR assay kit (Invitrogen, Carlsbad, CA, United States). Bacteria and archaea PCR reactions included a negative control for each separate amplification run. Negative control reactions containing no template DNA were performed alongside each PCR amplification and were included in subsequent analyses to confirm no amplification of product. Agarose gel electrophoresis was performed using 2 μl of PCR product on a 1% (w/v) agarose gel containing SYBR Safe. Each amplicon (150 ng) from the same target gene and region (i.e., all bacteria and archaea amplicons) was pooled. Pooled samples were concentrated (vacuum dried) and the final PCR product concentration was determined using Quant-iT dsDNA HS assay kit (Invitrogen, Carlsbad, CA, United States). Pools were purified using the NucleoMag NGS kit (Macherey-Nagel, Dueren, Germany). The final purification of amplicons was done using the QIAquick PCR Purification kit (Qiagen, Valencia, CA, USA) and the DNA concentration quantified using Quant-iT dsDNA HS assay kit (Invitrogen, Carlsbad, CA, United States). Both pools were diluted to 6.0 × 109 copies per μl and combined at a “bacteria to archaea” ratio of 5:1 (Kittelmann et al., 2013). Pooled libraries were checked for quality control (QC) using Labchip GX Touch HT instrument (PerkinElmer, Waltham, MA, United States). Amplicons were sequenced using the Illumina MiSeq system according to the protocol of the manufacturer (Illumina, San Diego, CA, United States) at Massey Genome Service, Massey University, Palmerston North, New Zealand. The pooled library was run on one Illumina MiSeq (500 cycle V 2 kit). A control library for the run, Illumina-prepared PhiX, was loaded onto the Illumina MiSeq run at 20% volumes. Sequence reads were provided in fastq format. The sequences obtained were deposited in the European Nucleotide Archive under the accession number PRJEB37783.
Sequencing reads were quality-filtered using the DynamicTrim function of SolexaQA (Cox et al., 2010). Reads were then processed and analyzed using the QIIME software package 1.8 (Caporaso et al., 2010). Sequencing reads were grouped into operational taxonomic units (OTUs) sharing over 97% and 99% similarity for bacteria and archaea, respectively, by using the UCLUST algorithm (Edgar, 2010). Sequences were assigned to phylogenetic kingdoms using the BLAST (version 2.4.0) algorithm (Altschul et al., 1990). Bacterial 16S rRNA genes were assigned using SILVA 123 (Henderson et al., 2019) and archaeal 16S rRNA genes using RIM-DB (Seedorf et al., 2014). QIIME-generated OTU tables were used for downstream statistical analysis.
Data were checked for normality using Q–Q plots alongside the Shapiro–Wilk’s W test. After normality assessment, univariate analyses were performed using a linear mixed effect (LME) model via the restricted maximum likelihood (REML) framework as implemented in the NLME package in R (Pinheiro et al., 2015; R Core Team, 2016). The resulting LME models were analyzed using analysis of variance (ANOVA). Predicted means from the model, together with estimates of the standard error of the mean and pairwise comparisons (Tukey’s or Benjamini–Hochberg test), were obtained and back transformed (where applicable) using the PREDICTMEANS package of R (Luo et al., 2014). Statistical significance was declared at a P-value ≤0.05.
Dietary effects were evaluated on animal performance, enteric emissions, and rumen fermentation data. In P01, dietary treatment (FO or CO) was used as a fixed effect and animal as a random effect. Data from P02 and P03 were analyzed using dietary treatments from P01 (FO and CO) and P02 (HQ and LQ) as fixed effects and animal as random effect. Live weight analysis for each feeding phase was adjusted using the initial LW as covariate in the model. The resulting LME models were analyzed using one-way ANOVA for P01 and a 2 × 2 factorial ANOVA for P02 and P03. Treatment effects were assessed and predicted means from the model, together with estimates of the standard errors of the means, were obtained and compared using Tukey’s test.
A total of 364 bacterial OTUs and 17 archaea OTUs (Supplementary Tables S2, S3) were analyzed after using a minimum average cutoff of 70 reads per sample. The alpha diversity of the bacterial and archaeal community of calves under contrasting dietary management conditions was analyzed separately using Shannon index in the Vegan package of R (Oksanen et al., 2017). Dietary treatment effects for the Shannon index of the microbial (bacterial and archaeal) community during P01, P02, and P03 were fitted in an LME model and analyzed using ANOVA as described for DMI, rumen fermentation, and gas emissions data. Predicted means from the models, together with estimates of the standard error of the mean (SEM), were obtained, and pairwise comparisons were done using Tukey’s test. Beta diversity of the bacteria and archaea community in each group of calves was analyzed using a partial least squares discriminant analysis (PLSDA) using the mixOmics package of R (Lê Cao et al., 2016). Groups of calves in the PLSDA analysis were assigned combining phase and treatments as follows: phase 1 (P01) corresponded to groups FO and CO, and phases 2 (P02) and 3 (P03) were the groups formed by the combination of dietary treatments from P01 and P02, resulting in FOHQ, FOLQ, COHQ, and COLQ. Additionally, a PLSDA was conducted for abundant microbes. Abundant microbes were defined as bacteria genera and archaea species with a relative abundance ≥0.70% and ≥1.00%, respectively. The aim was to identify whether the abundant microbiota showed a similar cluster separation pattern to that observed in the whole microbiota. Association scores for bacteria and archaea were visualized using clustered image maps (CIM) representing the first two dimensions (Henderson et al., 2015).
Univariate analyses were used to determine the effect of dietary treatments on the abundant microbial community. The abundant microbial community was defined from the OTU data table as those taxa with an overall relative abundance across phases ≥1.0 and ≥0.7% at bacteria phylum and genus level, respectively, and ≥1.0% at species level for archaea. After checking for normality, bacteria (phyla and genera) and archaea (species) community data were transformed using natural logarithm. The analysis of the abundant microbial community in each feeding phase was assessed as described for animal performance, enteric emissions, and rumen fermentation data. Predicted means from the models, together with estimates of the confident intervals (CI) with upper limit (UL) and lower limit (LL), were obtained and back transformed, and pairwise comparisons were done using the Benjamini–Hochberg test.
The effects of dietary treatments on animal performance, enteric emissions, and rumen fermentation are presented in Table 2. In P01 (week 9), CO calves had a 136% greater (P < 0.01) DMI than FO calves (total DMI, including milk intake, CO = 2.10 kg vs. FO = 1.84 kg; P < 0.01). Live weight was 8% lower (P = 0.02) in CO than in FO calves. Daily pCH4 was 25% higher in CO than FO calves but this was not significant (P = 0.06). Calves in the CO group had 47% lower (P < 0.01) yCH4 than FO calves. Hydrogen production and yield were not affected (P ≥ 0.33) by the dietary regime. Total SCFA concentrations in the rumen were 45% higher (P < 0.01) in CO than FO calves. Compared with FO calves, the proportion of acetate in CO calves was lower (P < 0.01), while the proportions of propionate and valerate were greater (P < 0.01). The proportions of butyrate and caproate were similar (P ≥ 0.11) in both groups, while both isobutyrate and isovalerate were lower (P < 0.01) in CO than FO calves.
Table 2. Effect of dietary treatmentsa on dry matter intake (DMI)b, live weight (LW)c, enteric emissionsd, and fermentation profilese in calves during three measurement phasesf.
In P02 (week 19), CO calves were 10% lighter (P < 0.01) than FO calves. Isovalerate proportions were higher in CO than FO calves (1.00 vs. 0.87; P = 0.03). No other effects from P01 dietary regimes (i.e., CO and FO) and no interactions between the dietary regimes in P01 and P02 (i.e., HQ and LQ) were observed (P ≥ 0.13). Dry matter intake was 41% greater in HQ than LQ calves. Live weight in HQ calves was 8% lower (P = 0.03) than in LQ calves. The pCH4 and pH2 were 31% (P < 0.01) and 133% (P = 0.03) greater in HQ than LQ calves, respectively. No differences between yCH4 and yH2 were observed between these groups (P ≥ 0.15). Total SCFA concentrations in HQ calves were 36% greater (P < 0.01) than in LQ calves. In HQ calves, acetate proportions were lower (P < 0.01), while propionate and valerate proportions were greater (P < 0.01) than in LQ calves. The proportions of butyrate and caproate were 20% lower and 8% greater in HQ than in LQ calves, but these were not significant (P = 0.07). Both isobutyrate and isovalerate proportions were greater (P < 0.01) in HQ than LQ calves.
In P03 (week 41), CO calves were 6% lighter than FO calves, but this was not significant (P = 0.08), while HQ calves were 23% heavier (P < 0.01) than LQ calves. No direct effect of previous dietary treatments (P ≥ 0.12) or their interactions (P ≥ 0.14) was observed on animal performance, enteric emissions, and rumen fermentation.
Negative control reactions containing no template for bacteria and archaea resulted in no 16S rRNA amplicons after PCR; therefore, no subsequent analysis was undertaken. After merging, filtering, and trimming, Illumina sequencing generated a total of 8,087,270 bacterial and archaeal 16S rRNA sequences from the 72 samples. The average number of sequences of bacteria and archaea was 97,286 ± 29,785 SD and 15,037 ± 2,875 SD as per sample, respectively, while the number of OTUs was 1,509 and 41 for bacteria and archaea, respectively.
Figure 1 shows the Shannon index of the bacterial and archaeal community in each of the dietary treatment groups of calves during each sampling phase. In P01, the bacteria diversity in CO calves was lower (P = 0.01) than in FO calves. However, the Shannon index for bacteria in P02 and P03 did not show dietary effects from P01 and P02 (P ≥ 0.69) or dietary interaction effects (P ≥ 0.70; Figure 1A). The archaea diversity did not show dietary treatment effect (P ≥ 0.29) in P01, or carryover effects (P ≥ 0.17) from P01, dietary treatment effects during P02, or their interaction effects during P02 and P03 (Figure 1B).
Figure 1. Effects of dietary treatments in the Shannon diversity indices of the microbial communities in the rumen of calves during the three measurement phases. The alpha diversity of dietary treatment concentrate (CO) vs. pasture (FO) during phase 1 (P01; blue) and the combination of dietary treatments from P01 and high-quality (HQ) vs. low-quality (LQ) pastures from phase 2 (P02), resulting in FOHQ, FOLQ, COHQ, and COLQ, evaluated during P02 (red) and phase 3 (P03; green) are shown for (A) bacteria Shannon index and (B) archaea Shannon index. Boxplots represent the 25th and 75th percentiles, the whiskers extend to the most extreme data points, lines within boxes are the medians, and dots represent outliers.
Figure 2 shows the beta diversity analysis of the bacteria and archaea for the community and abundant microbes, respectively, during each feeding phase. The PLSDA for the bacteria community (364 bacteria genus; Figure 2A) and abundant bacteria (25 genus; Figure 2B) in CO calves differed from pasture-fed calves in P01–P03. Within pasture-fed calves, the beta diversity for the bacteria community differed between calves in P01–P02 and those in P03; however, for the abundant bacteria, differences were only observed between pasture-fed calves in P01 and those in P03. The PLSDA of the archaea community (17 species; Figure 2C) and abundant archaea (7 species; Figure 2D) showed that concentrate-fed calves had different archaea diversity than those pasture-fed calves. Beta diversity within pasture-fed calves, for the archaea community and for the abundant archaea, showed that calves from the HQ groups (FOHQ and COHQ) in P02 differed from the other groups of calves in P01–P03.
Figure 2. Partial least square discriminant analysis (PLSDA) of the bacteria community at the genus level and archaea community at the species level from calves fed different treatments and treatment combinations in three sampling phases. Dietary treatments corresponded to: phase 1 (P01), concentrate (CO) vs. pasture (FO); and phase 2 (P02) high-quality (HQ) vs. low-quality (LQ) pastures. The treatment groups analyzed by phase were as follows: phase 1 (P01; blue) corresponded to groups from FO and CO; phase 2 (P02; red) and phase 3 (P03; green) were the groups formed by the combination of dietary treatments from P01 and P02, resulting in FOHQ, FOLQ, COHQ, and COLQ. (A) PLSDA of the bacteria community—364 bacterial genera, (B) PLSDA of the abundant (>0.7%) bacteria—25 abundant genera, (C) PLSDA of the archaea community—17 archaeal species, and (D) PLSDA of the abundant (>1.0%) archaea—7 abundant species.
The most prominent difference in P01 was a decrease (P < 0.01) in the proportion of Fibrobacteres and Tenericutes in CO compared with FO calves. No other differences were observed for bacteria phyla composition between treatments (Table 3). At the genus level, members of the Firmicutes phylum had greater (P < 0.01) Lachnospiraceae NK3A20 group, Roseburia, Erysipelotrichaceae UCG-002, and Succiniclasticum proportions, but lower (P < 0.01) Ruminiclostridium 9, Ruminococcaceae NK4A214 group, Ruminococcus 1, and Kandleria proportions in CO compared with FO calves. On the other hand, members of the Bacteroidetes phylum showed greater (P < 0.01) Prevotella 7 proportions but lower (P < 0.01) Prevotella 1; Bacteroidales BS11, RF16, and S24-7; Prevotellaceae UCG-003; and Rikenellaceae RC9 gut group proportions in CO compared with FO calves (Table 4).
Table 3. Effect of dietary treatmentsa on the abundant bacteria phylumb during the measurement phases: 1 (P01), 2 (P02), and 3 (P03)c.
Table 4. Effect of dietary treatmentsa on the abundant bacteria genusb during the three measurement phasesc.
During P02, the bacterial community in HQ when compared with LQ calves had greater Firmicutes proportions (P < 0.01) but lower Fibrobacteres proportions (P < 0.01). At the genus level, members of the Firmicutes phylum in HQ calves had greater (P ≤ 0.02) proportions of Butyrivibrio 2, Pseudobutyrivibrio, Roseburia, Ruminiclostridium 9, and Ruminococcaceae NK4A214, but lower (P ≤ 0.02) Christensenellaceae R-7, Ruminococcus 1, Saccharofermentans, and Succiniclasticum compared with LQ calves. Conversely, members of the Bacteroidetes phylum in HQ calves had lower (P ≤ 0.03) Bacteroidales BS11 and S24-7, Prevotellaceae UCG-003, and Rikenellaceae RC9 gut proportions than LQ calves. No effects (P > 0.10) from dietary treatments in P01 or the interaction (P = 0.07) between P01 and P02 were observed in P02 for the most abundant bacteria phyla and genera (Tables 3, 4).
In P03, no direct effect of previous dietary treatments or their interactions was observed on the abundant bacteria at the phylum and genus levels (Tables 3, 4). Low abundant bacterial genera showed similar patterns to abundant bacteria (Supplementary Table S2).
Table 5 shows the effects that dietary treatments during the three feeding phases have on the main archaea species in calves. During P01, the methanogenic community in CO calves was dominated by Methanobrevibacter (Mbb.) boviskoreani, while in FO calves, this was dominated by Mbb. gottschalkii. The abundant archaeal community in CO calves had greater (P < 0.01) proportions of Mbb. boviskoreanii, Methanosphaera (Mph.) A4, and Mph. Group 5, respectively, but lower (P < 0.01) proportions of Mbb. gottschalkii, Mbb. ruminantium, and Mph. ISO3_F5 when compared with FO calves (Supplementary Figure S1).
Table 5. Effect of dietary treatmentsa on the abundant archaea speciesb during the three measurement phasesc.
In P02, the archaea community was dominated by Mbb. gottschalkii in both treatment groups. Compared with LQ calves, the abundant archaea community composition in HQ calves had greater (P ≤ 0.04) proportions of Mph. sp. A4, Mph. Group 5, and Mph. ISO3_F5, but lower (P < 0.01) proportions of Methanomassiliicoccales (Mmc.) Group 10 sp. During P02, the archaea community did not show carryover effects from P01 treatments (P ≥ 0.24) or interactions between P01 and P02 treatments (P ≥ 0.32) in the abundant archaeal species.
In P03, animals that previously grazed the HQ swards showed greater (P < 0.01) Mbb. ruminantium proportions when compared with LQ calves. No main effects of P01 diets (P ≥ 0.08) on the relative proportions of the abundant archaea were observed in P03.
Ruminal microorganisms are required for the degradation of plant components (Puniya et al., 2015; Huws et al., 2018; Gruninger et al., 2019). The establishment of these microbes in the rumen has been shown to be a dynamic progression from birth to adulthood (Jami et al., 2013; Rey et al., 2014). Recent studies have suggested that early interventions in life might imprint the microbial community, with such interventions having a persistent effect throughout the adult life of the animal (Yáñez-Ruiz et al., 2015). In this study, we have shown that feeding contrasting diets in early life (1 to 30 weeks) affects rumen fermentation patterns and rumen microbiota composition at the time of sampling; however, a permanent microbial or rumen fermentation imprint was not achieved.
In pre-weaned ruminants, the intake of solid feed is affected by milk management (e.g., amount of milk, age at weaning, and weaning method) and both access to and the type of solid feed offered (Khan et al., 2011; Abbas et al., 2017). The above was observed in the present study in P01, where pre-weaning milk management and type of solid diet access resulted in differences in solid DMI. Despite the increased DMI in CO calves, the greater daily milk allowance and duration of milk feeding in FO calves resulted in heavier pre-weaning LW, as reported in the wider cohort of animals from the parent study (Burggraaf et al., 2020) and prior studies (Muir et al., 2002; Khan et al., 2011). Differences in solid feed intake between groups corresponded to differences in CH4 production between CO and FO calves, where greater DMI was associated with greater CH4 production (Jonker et al., 2016; Bird-Gardiner et al., 2017). However, CH4 production per kilogram of DMI (yCH4) was lower in CO calves with higher energy content in grain-based diets than in FO calves with a forage diets (Table 1), as previously stated by Johnson and Johnson (1995). Differences in dietary nutrient composition and its digestibility include changes in ruminal pH and in cellulolytic activity and fiber degradation, level of starch by-pass to the intestine, and percentage of SCFA which all may influence methanogenesis (Benchaar et al., 2001; Jentsch et al., 2007). Beauchemin and McGinn (2005) indicated that feeding high-concentrate diets (47–58% of starch) decreases methane yield and lowers the acetate:propionate ratio. In the rumen, the fermentation of diets rich in structural carbohydrates produces greater proportions of acetate with the release of hydrogen, whereas the intake of diets rich in starch contents results in greater propionate proportions without hydrogen production (Ungerfeld, 2020). The propionate pathway competes for hydrogen with hydrogenotrophic methanogens (Janssen, 2010). Therefore, the high availability of starch in the diet of CO calves resulted in a reduction of methane yield in part due to an increased propionate production (Sauvant et al., 2011; Williams et al., 2019). Moreover, the rumen pH of grass-fed ruminants ranges between 6.0 and 7.0 under normal physiological conditions (Grünberg and Constable, 2009). However, the consumption of diets rich in grains results in greater concentration of SCFA and production of lactic acid that can build up in the rumen and reduce the ruminal pH below 6.0 (Dijkstra et al., 2012). Ruminal pH was not measured in the current study, but it can be speculated that CO calves with higher SCFA concentrations and less fiber contents in the diet had a lower ruminal pH than the grazing groups (Hook et al., 2011). Reduction in ruminal pH may affect methanogenic microbes and further decrease CH4 yield (Van Kessel and Russell, 1996).
During P02, results from the parent production trial (Burggraaf et al., 2020) showed no compensatory growth in CO calves reared on restricted milk, which correspond to observations in previous studies (Wardrop, 1966). Conversely, no growth checks were observed in FO calves, which indicated that an adequate rumen development was achieved, despite the high volume of milk fed, consistent with prior studies (Khan et al., 2011). Forage quality in this feeding phase was critical for lifetime performance of post-weaned calves, where calves fed HQ forages resulted in heavier LW when compared with calves fed LQ forages. This agrees with de Clifford et al. (2014), who showed that improved growth of calves is achieved when fed forages with higher metabolizable energy, metabolizable protein, and digestibility. The differences in methane production during P01 did not persist when these group of calves were allocated into different forage treatment diets in P02. During this P02, the intake of low-quality pastures with high fiber contents lowered DMI, resulting in low CH4 production (g/day). These observations corresponded to those reported in growing heifers, where DMI was reduced in forage diets with low-quality and high NDF content (Pino et al., 2018). The lack of difference in methane yield (g/kg of DM) between calves grazed in high- or low-quality pasture is likely a result of the effects that the diet had on daily methane output and DMI; this lack of a response of methane yield to pasture quality has been also shown in adult cattle (Jonker et al., 2016) and sheep (Muetzel and Clark, 2015). Conversely, the intake of grasses with high protein and low fiber content increased the total concentration of SCFA and decreased the acetate to propionate ratio, i.e., HQ calves, characteristic of the intake of grasses with high organic matter digestibility (Owens and Basalan, 2016; Pino et al., 2018). On the other hand, reduced proportions of protein degradation products, i.e., isobutyrate and isovalerate, in LQ calves reflected the low crude protein content in the pasture (Brinkhaus et al., 2017).
When the animals were on the same diet in P03, treatment differences in LW were sustained due to the lack of compensatory growth following the dietary intervention in P01 and P02. Results from the present study and the parent production study (Burggraaf et al., 2020) showed that extended nutritional restrictions imposed during the first 7 months of age limited the capacity of cattle to exhibit compensatory growth. This agrees with similar studies where severe pre- and post-weaning nutritional restrictions limit the capacity of cattle to exhibit compensatory growth and achieve equivalent weight for age in later life (Ryan, 1990; Hearnshaw, 1997; Shamay et al., 2005). No differences of DMI, CH4 emissions, and SCFA profiles were observed between the two groups. Similar results were observed in lambs fed high and low fiber diets in early life with no effect on rumen metabolites after 16 to 20 weeks of treatment cessation (Yáñez-Ruiz et al., 2010). Our results indicate that dietary composition at the time of measurement is the major driver for DMI, rumen fermentation pathways, and methane production independently of the previous feeding regimes, thus showing that at the metabolic level no imprint of pre- and post-weaning treatments had occurred.
The bacterial diversity in the rumen is host specific; however, variations in the composition of the ingested diet result in diversity changes of the prokaryotic domains harbored in the rumen (Henderson et al., 2015). In the present study, differences in bacterial diversity observed between treatments in the different dietary phases were consistent with those reported in young and adult ruminants, suggesting that the degradation of more structural diets is a complex process which requires a more diverse consortium of microbes working together (Kim et al., 2016; Belanche et al., 2019).
In young ruminants, the bacteria present in the rumen is largely represented by the phyla Bacteroidetes, Firmicutes, and Proteobacteria, whose changes in relative abundance have been associated with animal growth and diet (Li et al., 2012; Jami et al., 2013; Rey et al., 2014). Previously, reports in calves showed that the fiber content of the diet led to an increased Firmicutes:Bacteroidetes ratio (F:B ratio) (Kim et al., 2016), but no such difference was observed in P01 of the present experiment despite the differences in structural contents between diets. It cannot be elucidated whether restriction in forage intake by the allowance of high-milk volumes might affect the F:B ratio as observed in FO calves from the present study. However, in P02, the intake of low-quality pastures increased F:B ratio as previously stated. The intake of diets rich in fiber, e.g., FO in P01 and LQ in P02, resulted in increased proportion of cellulose-degrading microorganism such as Fibrobacteres (Ransom-Jones et al., 2012). The lack of any differences in P03 confirms that diet at the time is the major driver of the microbial community at the phylum level and that changes observed by differences in diet composition pre- and post-weaning do not lead to a permanent change of the rumen microbiota.
Prevotella is one of the most abundant ruminal bacterial groups and plays a key role in the degradation and utilization of a large variety of carbohydrates and proteins entering the rumen (Cotta, 1992; Kim et al., 2016; Solden et al., 2016). In the present study, Prevotella was the dominant genus in the rumen of calves independent of age and diet, as observed previously in young and adult ruminants (Rey et al., 2014; Henderson et al., 2015). However, our results indicate that the differences between Prevotella 7 and Prevotella 1 are driven by dietary composition. The dominance of Prevotella 7 was only observed in CO calves in accordance to sheep fed 95% concentrates (McLoughlin et al., 2020), while Prevotella 1 prevailed in FO and all the other groups of grazing calves similar to reports in sheep fed high forage diets (Xie et al., 2019). Prevotella 1 group includes the species P. ruminicola, P. brevis, and P. bryantii (Henderson et al., 2019) that produce mainly acetate and succinate (Avguštin et al., 1997), rather than propionate (Avguštin et al., 1997; Seshadri et al., 2018). Prevotella 1 species possess extensive repertoires of polysaccharide utilization loci and carbohydrate active enzymes targeting various plant polysaccharides (Accetto and Avguštin, 2019). Prevotella 7 includes species like P. albensis (Henderson et al., 2019) that mostly produce acetate, succinate, and propionate (Avguštin et al., 1997; Seshadri et al., 2018). Annotation of de novo assembled contigs from metagenomic data not only identified sequences encoding for α-amylase enzymes in uncharacterized strains of P. albensis but also revealed the potential to metabolize xylan as an alternative substrate (Bandarupalli and St-Pierre, 2020). The higher proportion of propionate in the rumen of concentrate-fed calves was at least partially due to the differences in these two dominant rumen bacterial genera.
The intake of concentrates in CO calves increased the relative abundance of bacteria from the genera Roseburia, Lachnospiraceae NK3A20 group, and Erysipelotrichaceae UCG-002, which have a high affinity for utilizing highly degradable mono- and polysaccharides (Stanton et al., 2009; Huo et al., 2014). Increases of these soluble carbohydrate-utilizing genera have been observed in the rumen contents of cattle and sheep fed greater ratios of dietary concentrates (McLoughlin et al., 2020). Roseburia and Lachnospiraceae NK3A20 are butyrate-producing microorganisms (Duncan et al., 2002). However, even though the principal fermentation product of these organisms is butyrate, no effect on the proportion or concentrations (CO = 11.3 mM vs. FO = 8.4 mM, SED = 1.62; P = 0.09) of this SCFA between the two groups was observed. This may be because Roseburia and Lachnospiraceae NK3A20 made up only 6.6% and 6.0% of the community, respectively. Likewise, the family Erysipelotrichaceae ferments a wide range of sugars to produce mainly lactic acid (Deusch et al., 2017). Studies in low methane-emitting sheep have shown that high proportions of Erysipelotrichaceae are associated with increases in lactic acid production, resulting in less hydrogen and methane formation (Kamke et al., 2016). In the present study, the relative abundance of Erysipelotrichaceae UCG-002 in the CO group may have favored the production of propionate by lactate-utilizing bacteria, i.e., Megasphaera (CO = 0.33% vs. FO = 0.00%, SED = 0.086; P < 0.01) (Kamke et al., 2016). Reductions in methane formation are attributed to the H2 utilization for propionate formation, which competes with the most common hydrogenotrophic methanogens (Liu and Whitman, 2008; Ungerfeld, 2020). Therefore, increases in the intake of starch favored the proportions of amylolytic microorganisms, whose metabolism increased the proportions of propionate during ruminal fermentation but reduced the production of hydrogen and ultimately its availability to be used by methanogens. However, although the amylolytic bacterial microbiota dominated during the pre-weaning rearing phase in concentrate-fed calves, it did not persist into the post-weaning phases of grazing calves.
Interestingly, Kandleria, which degrades different sugars, including D-galactose and lactose (lactate producer) (Kumar et al., 2018), was found in high proportions in calves from the FO group. The genus Kandleria has been isolated from the rumen of young calves fed on only milk diets (Salvetti et al., 2011). Therefore, the observed relative abundance of this genus in FO calves may be associated with the degradation of milk sugars leaking into the rumen. This can be confirmed by the low relative abundance of Kandleria in FO calves when transitioning into P02 and P03 despite the soluble sugars from fresh mixed sward of ryegrass and white clover.
Bacteria from the genus Ruminococcus and Fibrobacter are considered major cellulolytic degraders (Koike and Kobayashi, 2001; Ransom-Jones et al., 2012; Abdul Rahman et al., 2016). The proportion of Fibrobacter in P01 and P02 was higher in calves consuming diets with the highest fiber contents within each dietary phase. Such association of high relative abundance of Fibrobacter has been shown in heifers (Petri et al., 2013) and sheep (Belanche et al., 2019) fed forage diets. Similar effects have also been observed for the various genera in the Ruminococcaceae, but their proportional increases with the increase in fiber content of the diets was not as pronounced as for Fibrobacter, which may be due to the fact that Ruminococcaceae have a much wider spectrum of metabolizable substrates compared with Fibrobacter (Thurston et al., 1994).
Besides the known fiber degraders, some unclassified Bacteroidetes genera such as Rikenellaceae RC9, Bacteroidales, and Prevotellaceae UCG-003 and the Firmicutes genus Christensenellaceae R-7 were increased in calves fed diets rich in fiber, i.e., LQ calves. The genus Rikenellaceae RC9, with no as yet defined metabolic function, is one of the most prevalent microbes in the rumen microbiota (Henderson et al., 2015; De Mulder et al., 2017) and abundant in rich fibrous diets (Petri et al., 2013; Schären et al., 2017). We found similar results with increased proportions of Rikenellaceae in the high fiber treatments in P01 and P02. Correspondingly, hemicellulose and monomeric sugar (xylose, fucose, mannose, and rhamnose) degraders (Ormerod et al., 2016; Solden et al., 2017) from Bacteroidales, such as the genera BS11 gut group and S24-7 group, were increased in calves with high fiber intakes. In the present study, Christensenellaceae R-7 was the second most abundant bacteria genus in the rumen of grazing calves. These also appear to be related to fiber degradation as their levels were increased in the high fiber treatments in the first two phases. These findings agreed with reports in dairy cows, where increases of fiber in the diet resulted in an increase of this genus (Lima et al., 2015). Our data suggest that not only are the well-described families like Fibrobacteracea and Ruminococcaceae involved in plant fiber degradation but also members of the Rikenellaceae RC9, Bacteroidales BS11, Bacteroidales S24-7, and Christensenellaceae R-7. However, further studies of these genera are required to investigate their growth, ecology, and metabolism when ruminants are fed diets rich in fiber.
The ingestion of high-quality forage diets, i.e., HQ calves, with less NDF and ADF content favored the growth of bacteria from the Firmicutes genera such as Ruminococcaceae NK4A214, Butyrivibrio 2, Ruminiclostridium 9, and Pseudobutyrivibrio (Rainey, 1996; Ravachol et al., 2016). In post-weaned calves, Butyrivibrio and Pseudobutyrivibrio were found in greater proportion when transitioning into forages with lower contents of hemicellulose. Species belonging to the genus Butyrivibrio and Pseudobutyrivibrio are important degraders of plant polysaccharides, i.e., hemicelluloses (arabinoxylans) and pectin (Palevich et al., 2019b). However, some species of Butyrivibrio are unable to grow on structural plant components, and their role in the rumen appears to be as a utilizer of monosaccharides, disaccharides, and oligosaccharides made available by the degradative activities of other bacterial species (Palevich et al., 2017; Palevich et al., 2019a). Correspondingly, bacterial species from the genus Pseudobutyrivibrio are metabolically versatile and capable of growing on a range of simple mono- or oligosaccharides derived from complex plant polysaccharides such as pectins, mannans, starch, and hemicelluloses (Palevich et al., 2020). These findings may explain the increased relative abundance of Butyrivibrio and Pseudobutyrivibrio in high-quality forages.
Rumen archaea are much less diverse than rumen bacteria, which likely reflects the narrow range of substrates they use (Janssen, 2010; Seedorf et al., 2014). Variations in the dietary composition have been shown to alter the archaeal community (Henderson et al., 2015) due to changes in fermentation patterns that affect the proportion of their substrates and metabolic activity (Lana et al., 1998; Ungerfeld, 2020). In the current study, the archaeal microbiota of grazing calves, across all treatments, was dominated by Mbb. gottschalkii and Mbb. ruminantium, which was in agreement with observations from adult ruminants fed diets with high fiber contents (Henderson et al., 2015; Seedorf et al., 2015). Conversely, the group of calves fed high proportions of concentrate in the diet showed increases of Mbb. boviskoreani, an organism that has been previously found and isolated from cattle fed diets rich in concentrates (Lee et al., 2013; Snelling et al., 2019). Therefore, these results indicate that the fermentation of diets with high contents in structural carbohydrates favored the prevalence of hydrogenotrophic archaea such as Mbb. gottschalkii and Mbb. ruminantium. Additionally, as previously discussed, rumen pH was likely to be lower in CO calves, which may decrease the relative abundance of abundant archaea species such as Mbb. gottschalkii and Mbb. Ruminantium in these calves. The metabolic activity of these methanogen species, however, begins to be inhibited when the pH drops below their optimum pH of 7.0–7.2 for growing (Miller and Lin, 2002; Janssen, 2010). In contrast, Mbb. boviskoreani growth is supported at a ruminal pH of 5.5, whereas its optimum pH is between 6.0 and 7.0 (Lee et al., 2013). Another observation is the low relative abundance of Methanomassiliicoccus (Mmc.) Group 10 sp. and Methanosphaera (Mph.) ISO3_F5 in the CO group. Our results agreed with the low proportions of Mmc. reported in heifers fed diets rich in concentrate (Zhang et al., 2017). However, it is not clear whether the low abundance of Mmc. observed in concentrate-fed calves is due to a reduction in ruminal pH or a competition for substrates, e.g., methanol, with methylotrophic archaea such as Mph, as further discussed.
The intake of highly digestible diets, i.e., CO and HQ treatments, showed high proportions of the genera Methanosphaera (Mph). These methanogens reduce methanol (Fricke et al., 2006; Kelly et al., 2019). Methanol in the rumen is derived from the demethoxylation of dietary pectins and other methylated plant polysaccharides via pectin methylesterase activity (Dehority, 1969; Kelly et al., 2019). Additionally, methanol production is negatively affected by pasture maturity that has lower pectin degradation (Dehority et al., 1962). Clover and other non-grass pasture species usually contain higher proportion of pectins than grasses (Thomson, 1984; Hammond et al., 2011). In cows, the intake of diets rich in clover can favor the relative abundance of Mph. sp. Group 5 and Mph. sp. ISO3_F5 in the rumen (Bowen et al., 2018; Smith et al., 2020). In the present study, calves consuming high-quality sward with 35.5% of white clover (DM basis) may have produced more methanol, favoring the increase of Mph. sp. Group 5 and Mph. sp. ISO3_F5 when compared with the intake of non-irrigated low-quality pastures with 4.0% of white clover and more fibrous contents (Burggraaf et al., 2020). Conversely, Mph. sp. A4 was found in high proportions in CO diets similar to that observed in pre-weaned calves by Dias et al. (2017), who indicated that the pectins present in the starter concentrate may contribute to the formation of methanol and increases in the relative abundance of this archaea species. However, further studies are required to elucidate that the production of methanol, and a hypothetical reduction in ruminal pH, in concentrate-fed calves may correspond to the increasing proportion of Mph. sp. A4 in the rumen.
In the present study, calves consuming diets rich in fiber showed increases in Mmc. This order is a methylotrophic methanogen that utilizes compounds like methanol, methylamines, dimethylamine, and trimethylamine (Borrel et al., 2012; Poulsen et al., 2013). Plant-derived glycine, betaine, and choline are rapidly metabolized by ruminal bacteria using choline trimethylamine lyase (Kelly et al., 2019). Fiber-rich diets, where fermentation results in high ratios of acetate to propionate, are associated with a greater concentration of methylamine, dimethylamine, and trimethylamine compared with highly digestible diets such as corn silage (Deusch et al., 2017). In our study, the fiber content in the diets and the acetate to propionate ratios were higher in calves consuming greater fiber contents in the diet, which might have resulted in greater production of methylamines. Morgavi et al. (2015) showed that Mph. and Mmc. occupy similar trophic niches; however, the more versatile use of substrates by Mmc. explained their higher relative abundance in the rumen of lambs after receiving an inoculum of rumen fluid obtained from wethers fed a hay diet. Therefore, the intake of swards rich in fiber with low white clover content may produce high concentrations of methylamines in the rumen, offering a competitive advantage to low abundant methylotrophic methanogens from the order Methanomassiliicoccales over the genus Methanosphaera, whose growth is limited by the availability of methanol in the rumen (Kelly et al., 2019). Our results indicate that the apparent methanogen structure community, specifically the low abundant archaea, is affected by changes in the chemical composition of the diet consumed.
In conclusion, our results showed that the rumen microbial community in the growing calf is diet dependent, with early life differences having only negligible effects on the microbiota of the growing ruminant. Different dietary regimes, pre- and post-weaning, were unable to leave a microbial imprint in the rumen of calves when the animals were fed a common diet. These findings showed that interventions after feeding colostrum to calves did not leave a permanent effect in the early microbial colonization and function in the rumen. Further studies should target earlier microbial interventions, during microbial colonization of the rumen milieu, in an attempt to imprint the ruminal microbiota.
The data presented in the study are deposited in the European Nucleotide Archive, accession number PRJEB37783.
The animal study was reviewed and approved by Grasslands Animal Ethics Committee.
SMu and SMc designed the study and secured funding. OC-C, SMu, and SL generated the data. SG and OC-C completed the statistical analysis and all authors contributed to the interpretation. OC-C wrote the initial manuscript and all authors contributed to editing.
This study was funded by the Ministry of Primary Industries (FACCE # A21059 JPI Program), AgResearch Strategic Science Investment Fund, and New Zealand AID Program (MFAT), and CONACYT and INIFAP in Mexico for Ph.D. stipend support.
The authors declare that the research was conducted in the absence of any commercial or financial relationships that could be construed as a potential conflict of interest.
All claims expressed in this article are solely those of the authors and do not necessarily represent those of their affiliated organizations, or those of the publisher, the editors and the reviewers. Any product that may be evaluated in this article, or claim that may be made by its manufacturer, is not guaranteed or endorsed by the publisher.
The authors thank Vicki Burggraaf and Muhammad A. Khan (AgResearch), and Paul Muir and Beverly Thomson (On-Farm Research) for their support with coordinating the use of the subset of animals from the parent study for this research. The authors thank Sarah MacLean, Frederik Knol, Daniel Robinson, and Greg Skelton for their technical support for animal husbandry, sampling, and measurement procedures and Steve Lees, Richard Templeton, and the Aorangi farm staff for management of the subset of calves used in this study. The authors also gratefully acknowledge Sinead Leahy for the phylogenetic analysis of sequencing data.
The Supplementary Material for this article can be found online at: https://www.frontiersin.org/articles/10.3389/fmicb.2021.711040/full#supplementary-material
Abbas, W., Bhatti, S. A., Khan, M. S., Saeed, N., Warriach, H. M., Wynn, P., et al. (2017). Effect of weaning age and milk feeding volume on growth performance of Nili-Ravi buffalo calves. Ital. J. Anim. Sci. 16, 490–499. doi: 10.1080/1828051X.2017.1291282
Abdul Rahman, N., Parks, D. H., Vanwonterghem, I., Morrison, M., Tyson, G. W., and Hugenholtz, P. (2016). A phylogenomic analysis of the bacterial phylum Fibrobacteres. Front. Microbiol. 6:1469. doi: 10.3389/fmicb.2015.01469
Abecia, L., Ramos-Morales, E., Martínez-Fernandez, G., Arco, A., Martín-García, A. I., Newbold, C. J., et al. (2014). Feeding management in early life influences microbial colonisation and fermentation in the rumen of newborn goat kids. Anim. Produc. Sci. 54, 1449–1454. doi: 10.1071/AN14337
Accetto, T., and Avguštin, G. (2019). The diverse and extensive plant polysaccharide degradative apparatuses of the rumen and hindgut Prevotella species: a factor in their ubiquity? Syst. Appl. Microbiol. 42, 107–116. doi: 10.1016/j.syapm.2018.10.001
Alderman, G., and Cottrill, B. (1996). Energy and protein requirements of ruminants. Acribia, SA: CABI Publishing.
Altschul, S. F., Gish, W., Miller, W., Myers, E. W., and Lipman, D. J. (1990). Basic local alignment search tool. J. mol. Biol. 215, 403–410. doi: 10.1016/S0022-2836(05)80360-2
Attwood, G. T., Klieve, A. V., Ouwerkerk, D., and Patel, B. K. C. (1998). Ammonia-hyperproducing bacteria from New Zealand ruminants. Appl. Environ. Microbiol. 64, 1796–1804. doi: 10.1128/AEM.64.5.1796-1804.1998
Avguštin, G., Wallace, R. J., and Flint, H. J. (1997). Phenotypic diversity among ruminal isolates of Prevotella ruminicola: proposal of Prevotella brevis sp. nov., Prevotella bryantii sp. nov., and Prevotella albensis sp. nov. and redefinition of Prevotella ruminicola. Int. J. Syst. Evol. Microbiol. 47, 284–288. doi: 10.1099/00207713-47-2-284
Bandarupalli, V. V. K., and St-Pierre, B. (2020). Identification of a candidate starch utilizing strain of prevotella albensis from bovine rumen. Microorganisms 8:2005. doi: 10.3390/microorganisms8122005
Beauchemin, K., and McGinn, S. (2005). Methane emissions from feedlot cattle fed barley or corn diets. J. Anim. Sci. 83, 653–661. doi: 10.2527/2005.833653x
Belanche, A., Kingston-Smith, A. H., Griffith, G. W., and Newbold, C. J. (2019). A multi-kingdom study reveals the plasticity of the rumen microbiota in response to a shift from non-grazing to grazing diets in sheep. Front. Microbiol. 10:122. doi: 10.3389/fmicb.2019.00122
Benchaar, C., Pomar, C., and Chiquette, J. (2001). Evaluation of dietary strategies to reduce methane production in ruminants: a modelling approach. Can. J. Anim. Sci. 81, 563–574. doi: 10.4141/A00-119
Bergman, E. (1990). Energy contributions of volatile fatty acids from the gastrointestinal tract in various species. Physiol. Rev. 70, 567–590. doi: 10.1152/physrev.1990.70.2.567
Bird-Gardiner, T., Arthur, P., Barchia, I., Donoghue, K., and Herd, R. (2017). Phenotypic relationships among methane production traits assessed under ad libitum feeding of beef cattle. J. Anim. Sci. 95, 4391–4398. doi: 10.2527/jas2017.1477
Borrel, G., Harris, H. M., Tottey, W., Mihajlovski, A., Parisot, N., Peyretaillade, E., et al. (2012). Genome sequence of “Candidatus Methanomethylophilus alvus” Mx1201, a methanogenic archaeon from the human gut belonging to a seventh order of methanogens. J. Bacteriol. 194, 6944–6945. doi: 10.1128/JB.01867-12
Bowen, J. M., Mccabe, M. S., Lister, S. J., Cormican, P., and Dewhurst, R. J. (2018). Evaluation of microbial communities associated with the liquid and solid phases of the rumen of cattle offered a diet of perennial ryegrass or white clover. Front. Microbiol. 9:2389. doi: 10.3389/fmicb.2018.02389
Brinkhaus, A. G., Wyss, U., Arrigo, Y., Girard, M., Bee, G., Zeitz, J., et al. (2017). In vitro ruminal fermentation characteristics and utilisable CP supply of sainfoin and birdsfoot trefoil silages and their mixtures with other legumes. Animal 11, 580–590. doi: 10.1017/S1751731116001816
Burggraaf, V. T., Craigie, C. R., Muir, P. D., Khan, M. A., Thomson, B. C., Knol, F. W., et al. (2020). Effect of rearing diet and early post-weaning pasture quality on the life-time growth, meat quality, carcass traits and environmental impact of dairy-beef cattle. Livestock Sci. 2020:104031. doi: 10.1016/j.livsci.2020.104031
Caporaso, J. G., Kuczynski, J., Stombaugh, J., Bittinger, K., Bushman, F. D., Costello, E. K., et al. (2010). QIIME allows analysis of high-throughput community sequencing data. Nat. Methods 7, 335–336. doi: 10.1038/nmeth.f.303
Corson, D., Waghorn, G., Ulyatt, M., and Lee, J. (1999). “NIRS: forage analysis and livestock feeding,” in Proceedings of the Conference New Zealand Grassland Association, Hawkes Bay, 127–132. doi: 10.33584/jnzg.1999.61.2340
Cotta, M. A. (1992). Interaction of ruminal bacteria in the production and utilization of maltooligosaccharides from starch. Appl. Environ. Microbiol. 58, 48–54. doi: 10.1128/aem.58.1.48-54.1992
Cox, M. P., Peterson, D. A., and Biggs, P. J. (2010). SolexaQA: At-a-glance quality assessment of Illumina second-generation sequencing data. BMC Bioinform. 11:485. doi: 10.1186/1471-2105-11-485
Cristobal-Carballo, O., Khan, M. A., Knol, F. W., Lewis, S. J., Stevens, D. R., Laven, R. A., et al. (2019). Impact of weaning age on rumen development in artificially reared lambs. J. Anim. Sci. 97, 3498–3510. doi: 10.1093/jas/skz148
Curtis, T. P., and Sloan, W. T. (2004). Prokaryotic diversity and its limits: microbial community structure in nature and implications for microbial ecology. Curr. Opin. Microbiol. 7, 221–226. doi: 10.1016/j.mib.2004.04.010
De Barbieri, I., Hegarty, R. S., Silveira, C., Gulino, L. M., Oddy, V. H., Gilbert, R. A., et al. (2015). Programming rumen bacterial communities in newborn Merino lambs. Small Ruminant Res. 129, 48–59. doi: 10.1016/j.smallrumres.2015.05.015
de Clifford, R., Hickson, R., Martin, N., and Back, P. (2014). Growth rates of dairy heifers fed alternative feeds. Proc. NZ Soc. Anim. Produc. 74, 29–34.
De Mulder, T., Goossens, K., Peiren, N., Vandaele, L., Haegeman, A., De Tender, C., et al. (2017). Exploring the methanogen and bacterial communities of rumen environments: solid adherent, fluid and epimural. FEMS Microbiol. Ecol. 93:fiw251. doi: 10.1093/femsec/fiw251
Dehority, B. (1969). Pectin-fermenting bacteria isolated from the bovine rumen. J. Bacteriol. 99, 189–196. doi: 10.1128/jb.99.1.189-196.1969
Dehority, B., and Orpin, C. (1997). “Development of, and natural fluctuations in, rumen microbial populations,” in The rumen microbial ecosystem, eds P. N. Hobson and C. S. Stewart (Dordrecht: Springer), 196–245. doi: 10.1007/978-94-009-1453-7_5
Dehority, B. A., Johnson, R. R., and Conrad, H. R. (1962). Digestibility of forage hemicellulose and pectin by rumen bacteria in vitro and the effect of lignification thereon. J. Dairy Sci. 45, 508–512. doi: 10.3168/jds.S0022-0302(62)89436-3
Deusch, S., Camarinha-Silva, A., Conrad, J., Beifuss, U., Rodehutscord, M., and Seifert, J. (2017). A structural and functional elucidation of the rumen microbiome influenced by various diets and microenvironments. Front. Microbiol. 8:1605. doi: 10.3389/fmicb.2017.01605
Dias, J., Marcondes, M. I., Noronha, M. F., Resende, R. T., Machado, F. S., Mantovani, H. C., et al. (2017). Effect of pre-weaning diet on the ruminal archaeal, bacterial, and fungal communities of dairy calves. Front. Microbiol. 8:1553. doi: 10.3389/fmicb.2017.01553
Dijkstra, J., Ellis, J., Kebreab, E., Strathe, A., López, S., France, J., et al. (2012). Ruminal pH regulation and nutritional consequences of low pH. Anim. Feed Sci. Technol. 172, 22–33. doi: 10.1016/j.anifeedsci.2011.12.005
Dill-McFarland, K. A., Breaker, J. D., and Suen, G. (2017). Microbial succession in the gastrointestinal tract of dairy cows from 2 weeks to first lactation. Sci. Rep. 7:40864. doi: 10.1038/srep40864
Dill-McFarland, K. A., Weimer, P. J., Breaker, J. D., and Suen, G. (2019). Diet influences early microbiota development in dairy calves without long-term impacts on milk production. Appl. Environ. Microbiol. 85:e2141–18. doi: 10.1128/AEM.02141-18
Duncan, S. H., Hold, G. L., Barcenilla, A., Stewart, C. S., and Flint, H. J. (2002). Roseburia intestinalis sp. nov., a novel saccharolytic, butyrate-producing bacterium from human faeces. Int. J. Syst. Evol. Microbiol. 52, 1615–1620. doi: 10.1099/00207713-52-5-1615
Edgar, R. C. (2010). Search and clustering orders of magnitude faster than BLAST. Bioinformatics 26, 2460–2461. doi: 10.1093/bioinformatics/btq461
Fonty, G., Gouet, P., Jouany, J.-P., and Senaud, J. (1987). Establishment of the microflora and anaerobic fungi in the rumen of lambs. Microbiology 133, 1835–1843. doi: 10.1099/00221287-133-7-1835
Fricke, W. F., Seedorf, H., Henne, A., Krüer, M., Liesegang, H., Hedderich, R., et al. (2006). The genome sequence of Methanosphaera stadtmanae reveals why this human intestinal archaeon is restricted to methanol and H2 for methane formation and ATP synthesis. J. Bacteriol. 188, 642–658. doi: 10.1128/JB.188.2.642-658.2006
Grünberg, W., and Constable, P. D. (2009). “CHAPTER 6 - Function and Dysfunction of the Ruminant Forestomach,” in Food Animal Practice, Fifth Edn, eds D. E. Anderson and D. M. Rings (Saint Louis: W.B. Saunders), 12–19. doi: 10.1016/B978-141603591-6.10006-5
Gruninger, R., Ribeiro, G., Cameron, A., and Mcallister, T. (2019). Invited review: Application of meta-omics to understand the dynamic nature of the rumen microbiome and how it responds to diet in ruminants. Animal 13, 1843–1854. doi: 10.1017/S1751731119000752
Guyader, J., Tavendale, M., Martin, C., and Muetzel, S. (2016). Dose-response effect of nitrate on hydrogen distribution between rumen fermentation end products: An in vitro approach. Anim. Produc. Sci. 56, 224–230. doi: 10.1071/AN15526
Guzman, C. E., Bereza-Malcolm, L. T., De Groef, B., and Franks, A. E. (2015). Presence of selected methanogens, fibrolytic bacteria, and proteobacteria in the gastrointestinal tract of neonatal dairy calves from birth to 72 hours. PloS One 10:e0133048. doi: 10.1371/journal.pone.0133048
Hammond, K., Hoskin, S., Burke, J., Waghorn, G., Koolaard, J., and Muetzel, S. (2011). Effects of feeding fresh white clover (Trifolium repens) or perennial ryegrass (Lolium perenne) on enteric methane emissions from sheep. Anim. Feed Sci. Technol. 166, 398–404. doi: 10.1016/j.anifeedsci.2011.04.028
Hearnshaw, H. (1997). “Effect of pre-weaning nutrition on post-weaning growth carcass and meat quality traits,” in Proceedings of the Growth and Development Workshop, eds D. W. Hennessy, S. R. McLennan, and V. H. Oddy (Armidale, NSW: The Cattle and Beef CRC for Meat Quality, University of New England), 59–67.
Henderson, G., Cox, F., Ganesh, S., Jonker, A., Young, W., Collaborators, G. R. C., et al. (2015). Rumen microbial community composition varies with diet and host, but a core microbiome is found across a wide geographical range. Sci. Rep. 5:14567. doi: 10.1038/srep14567
Henderson, G., Cox, F., Kittelmann, S., Miri, V. H., Zethof, M., Noel, S. J., et al. (2013). Effect of DNA extraction methods and sampling techniques on the apparent structure of cow and sheep rumen microbial communities. PLoS One 8:e74787. doi: 10.1371/journal.pone.0074787
Henderson, G., Yilmaz, P., Kumar, S., Forster, R. J., Kelly, W. J., Leahy, S. C., et al. (2019). Improved taxonomic assignment of rumen bacterial 16S rRNA sequences using a revised SILVA taxonomic framework. PeerJ 7:e6496. doi: 10.7717/peerj.6496
Hobson, P. N., and Stewart, C. S. (1997). The Rumen Microbial Ecosystem. Springer: Netherlands. doi: 10.1007/978-94-009-1453-7
Hook, S. E., Steele, M. A., Northwood, K. S., Wright, A.-D. G., and Mcbride, B. W. (2011). Impact of high-concentrate feeding and low ruminal pH on methanogens and protozoa in the rumen of dairy cows. Microb. Ecol. 62, 94–105. doi: 10.1007/s00248-011-9881-0
Huo, W., Zhu, W., and Mao, S. (2014). Impact of subacute ruminal acidosis on the diversity of liquid and solid-associated bacteria in the rumen of goats. World J. Microbiol. Biotechnol. 30, 669–680. doi: 10.1007/s11274-013-1489-8
Husso, A., Lietaer, L., Pessa-Morikawa, T., Grönthal, T., Govaere, J., Van Soom, A., et al. (2021). The composition of the microbiota in the full-term fetal gut and amniotic fluid: A bovine cesarean section study. Front. Microbiol. 12:626421. doi: 10.3389/fmicb.2021.626421
Huws, S. A., Creevey, C. J., Oyama, L. B., Mizrahi, I., Denman, S. E., Popova, M., et al. (2018). Addressing global ruminant agricultural challenges through understanding the rumen microbiome: past, present, and future. Front. Microbiol. 9:2161. doi: 10.3389/fmicb.2018.02161
Jami, E., Israel, A., Kotser, A., and Mizrahi, I. (2013). Exploring the bovine rumen bacterial community from birth to adulthood. ISME J. 7, 1069–1079. doi: 10.1038/ismej.2013.2
Janssen, P. H. (2010). Influence of hydrogen on rumen methane formation and fermentation balances through microbial growth kinetics and fermentation thermodynamics. Anim. Feed Sci. Technol. 160, 1–22. doi: 10.1016/j.anifeedsci.2010.07.002
Jentsch, W., Schweigel, M., Weissbach, F., Scholze, H., Pitroff, W., and Derno, M. (2007). Methane production in cattle calculated by the nutrient composition of the diet. Arch. Anim. Nutr. 61, 10–19. doi: 10.1080/17450390601106580
Johnson, K. A., and Johnson, D. E. (1995). Methane emissions from cattle. J. Anim. Sci. 73, 2483–2492. doi: 10.2527/1995.7382483x
Jonker, A., Muetzel, S., Molano, G., and Pacheco, D. (2016). Effect of fresh pasture forage quality, feeding level and supplementation on methane emissions from growing beef cattle. Anim. Produc. Sci. 56, 1714–1721. doi: 10.1071/AN15022
Kamke, J., Kittelmann, S., Soni, P., Li, Y., Tavendale, M., Ganesh, S., et al. (2016). Rumen metagenome and metatranscriptome analyses of low methane yield sheep reveals a Sharpea-enriched microbiome characterised by lactic acid formation and utilisation. Microbiome 4, 1–16. doi: 10.1186/s40168-016-0201-2
Kelly, W. J., Leahy, S. C., Kamke, J., Soni, P., Koike, S., Mackie, R., et al. (2019). Occurrence and expression of genes encoding methyl-compound production in rumen bacteria. Anim. Microb. 1:15. doi: 10.1186/s42523-019-0016-0
Khan, M., Weary, D., and Von Keyserlingk, M. (2011). Invited review: Effects of milk ration on solid feed intake, weaning, and performance in dairy heifers. J. Dairy Sci. 94, 1071–1081. doi: 10.3168/jds.2010-3733
Kim, Y.-H., Nagata, R., Ohtani, N., Ichijo, T., Ikuta, K., and Sato, S. (2016). Effects of dietary forage and calf starter diet on ruminal pH and bacteria in holstein calves during weaning transition. Front. Microbiol. 7:1575. doi: 10.3389/fmicb.2016.01575
Kittelmann, S., Seedorf, H., Walters, W. A., Clemente, J. C., Knight, R., Gordon, J. I., et al. (2013). Simultaneous amplicon sequencing to explore co-occurrence patterns of bacterial, archaeal and eukaryotic microorganisms in rumen microbial communities. PLoS One 8:e47879. doi: 10.1371/journal.pone.0047879
Koike, S., and Kobayashi, Y. (2001). Development and use of competitive PCR assays for the rumen cellulolytic bacteria: Fibrobacter succinogenes, Ruminococcus albus and Ruminococcus flavefaciens. FEMS Microbiol. Lett. 204, 361–366. doi: 10.1111/j.1574-6968.2001.tb10911.x
Kumar, S., Treloar, B. P., Teh, K. H., Mckenzie, C. M., Henderson, G., Attwood, G. T., et al. (2018). Sharpea and Kandleria are lactic acid producing rumen bacteria that do not change their fermentation products when co-cultured with a methanogen. Anaerobe 54, 31–38. doi: 10.1016/j.anaerobe.2018.07.008
Lana, R. P., Russell, J. B., and Van Amburgh, M. E. (1998). The role of pH in regulating ruminal methane and ammonia production. J. Anim. Sci. 76, 2190–2196. doi: 10.2527/1998.7682190x
Lane, M. A., Baldwin, R. L., and Jesse, B. W. (2000). Sheep rumen metabolic development in response to age and dietary treatments. J. Anim. Sci. 78, 1990–1996. doi: 10.2527/2000.7871990x
Lê Cao, K., Rohart, F., Gonzalez, I., and Dejean, S. (2016). mixOmics: Omics Data Integration Project. R package Version 6.1. 1.
Lee, J.-H., Kumar, S., Lee, G.-H., Chang, D.-H., Rhee, M.-S., Yoon, M.-H., et al. (2013). Methanobrevibacterboviskoreani sp. nov., isolated from the rumen of Korean native cattle. Int. J. Syst. Evol. Microbiol. 63, 4196–4201. doi: 10.1099/ijs.0.054056-0
Li, R. W., Connor, E. E., Li, C., Baldwin, V. I. R. L., and Sparks, M. E. (2012). Characterization of the rumen microbiota of pre-ruminant calves using metagenomic tools. Environ. Microbiol. 14, 129–139. doi: 10.1111/j.1462-2920.2011.02543.x
Lima, F. S., Oikonomou, G., Lima, S. F., Bicalho, M. L., Ganda, E. K., De Oliveira Filho, J. C., et al. (2015). Prepartum and postpartum rumen fluid microbiomes: characterization and correlation with production traits in dairy cows. Appl. Environ. Microbiol. 81, 1327–1337. doi: 10.1128/AEM.03138-14
Liu, Y., and Whitman, W. B. (2008). Metabolic, phylogenetic, and ecological diversity of the methanogenic archaea. Ann. N.Y. Acad. Sci. 1125, 171–189. doi: 10.1196/annals.1419.019
Malmuthuge, N., and Griebel, P. J. (2018). Fetal environment and fetal intestine are sterile during the third trimester of pregnancy. Vet. Immunol. Immunopathol. 204, 59–64. doi: 10.1016/j.vetimm.2018.09.005
McLoughlin, S., Spillane, C., Claffey, N., Smith, P. E., O’rourke, T., Diskin, M. G., et al. (2020). Rumen microbiome composition is altered in sheep divergent in feed efficiency. Front. Microbiol. 11:1981. doi: 10.3389/fmicb.2020.01981
Miller, T. L., and Lin, C. (2002). Description of Methanobrevibacter gottschalkii sp. nov., Methanobrevibacter thaueri sp. nov., Methanobrevibacter woesei sp. nov. and Methanobrevibacter wolinii sp. nov. Int. J. Syst. Evol. Microbiol. 52, 819–822.
Minato, H., Otsuka, M., Shirasaka, S., Itabashi, H., and Mitsumori, M. (1992). Colonization of microorganisms in the rumen of young calves. J. General Appl. Microbiol. 38, 447–456. doi: 10.2323/jgam.38.447
Mizrahi, I., Wallace, R. J., and Moraïs, S. (2021). The rumen microbiome: balancing food security and environmental impacts. Nat. Rev. Microbiol. 19, 553–566. doi: 10.1038/s41579-021-00543-6
Morgavi, D. P., Rathahao-Paris, E., Popova, M., Boccard, J., Nielsen, K. F., and Boudra, H. (2015). Rumen microbial communities influence metabolic phenotypes in lambs. Front. Microbiol. 6:1060. doi: 10.3389/fmicb.2015.01060
Moss, A. R., Jouany, J. P., and Newbold, J. (2000). Methane production by ruminants: its contribution to global warming. Ann. Zootech. 49, 231–253. doi: 10.1051/animres:2000119
Muetzel, S., and Clark, H. (2015). Methane emissions from sheep fed fresh pasture. NZ J. Agric. Res. 58, 472–489. doi: 10.1080/00288233.2015.1090460
Muir, P., Fugle, C., and Ormond, A. (2002). Calf rearing using a once-a-day milk feeding system: Current best practice. Proc. NZ Grassland Assoc. 64, 21–24. doi: 10.33584/jnzg.2002.64.2469
O’Hara, E., Kenny, D., Mcgovern, E., Byrne, C. J., Mccabe, M. S., Guan, L., et al. (2020). Investigating temporal microbial dynamics in the rumen of beef calves raised on two farms during early life. FEMS Microbiol. Ecol. 96:203.
Oksanen, F., Blanchet, G., Friendly, M., Kindt, R., Legendre, P., Mcglinn, D., et al. (2017). vegan: Community Ecology Package. R package version 2.4-4. Available Online at: https://CRAN.R-project.org/package=vegan.
Ormerod, K. L., Wood, D. L. A., Lachner, N., Gellatly, S. L., Daly, J. N., Parsons, J. D., et al. (2016). Genomic characterization of the uncultured Bacteroidales family S24-7 inhabiting the guts of homeothermic animals. Microbiome 4:36. doi: 10.1186/s40168-016-0181-2
Owens, F. N., and Basalan, M. (2016). Ruminal fermentation,” in Rumenology. Cham: Springer, 63–102. doi: 10.1007/978-3-319-30533-2_3
Palevich, N., Kelly, W. J., Ganesh, S., Rakonjac, J., and Attwood, G. T. (2019a). Butyrivibrio hungatei MB2003 competes effectively for soluble sugars released by Butyrivibrio proteoclasticus B316T during growth on xylan or pectin. Appl. Environ. Microbiol. 85:e2056–18. doi: 10.1128/AEM.02056-18
Palevich, N., Kelly, W. J., Leahy, S. C., Denman, S., Altermann, E., Rakonjac, J., et al. (2019b). Comparative genomics of rumen Butyrivibrio spp. uncovers a continuum of polysaccharide-degrading capabilities. Appl. Environ. Microbiol. 86:e1993–19. doi: 10.1128/AEM.01993-19
Palevich, N., Kelly, W. J., Leahy, S. C., Altermann, E., Rakonjac, J., and Attwood, G. T. (2017). The complete genome sequence of the rumen bacterium Butyrivibrio hungatei MB2003. Stand. Genom. Sci. 12, 1–14. doi: 10.1186/s40793-017-0285-8
Palevich, N., Maclean, P. H., Kelly, W. J., Leahy, S. C., Rakonjac, J., and Attwood, G. T. (2020). Complete genome sequence of the polysaccharide-degrading rumen bacterium pseudobutyrivibrio xylanivorans MA3014 reveals an incomplete glycolytic pathway. Genome Biol. Evol. 12, 1566–1572. doi: 10.1093/gbe/evaa165
Petri, R. M., Schwaiger, T., Penner, G. B., Beauchemin, K. A., Forster, R. J., Mckinnon, J. J., et al. (2013). Characterization of the core rumen microbiome in cattle during transition from forage to concentrate as well as during and after an acidotic challenge. PLoS One 8:e83424. doi: 10.1371/journal.pone.0083424
Pinares, C., and Waghorn, G. (2014). Technical manual on respiration chamber designs. Wellington, New Zealand: Ministry of Agriculture and Forestry.
Pinares-Patiño, C., Hunt, C., Martin, R., West, J., Lovejoy, P., and Waghorn, G. (2012). “New Zealand ruminant methane measurement centre, AgResearch, Palmerston North,” in Technical manual on respiration chamber design, eds C. S. Pinares-Patiño and G. C. Waghorn (Wellington, New Zealand: Ministry of Agriculture and Forestry), 11–30.
Pinheiro, J., Bates, D., Debroy, S., Sarkar, D., and Team, R. C. (2015). nlme: Linear and Nonlinear Mixed Efects Models. 3.1-121.
Pino, F., Mitchell, L. K., Jones, C. M., and Heinrichs, A. J. (2018). Comparison of diet digestibility, rumen fermentation, rumen rate of passage, and feed efficiency in dairy heifers fed ad-libitum versus precision diets with low and high quality forages. J. Appl. Anim. Res. 46, 1296–1306. doi: 10.1080/09712119.2018.1498788
Poulsen, M., Schwab, C., Jensen, B. B., Engberg, R., Spang, A., Canibe, N., et al. (2013). Methylotrophic methanogenic Thermoplasmata implicated in reduced methane emissions from bovine rumen. Nat. Commun. 4:1428.
Puniya, A. K., Singh, R., and Kamra, D. N. (2015). Rumen microbiology: from evolution to revolution. Cham: Springer. doi: 10.1007/978-81-322-2401-3
Rainey, F. (1996). Genus XV. Pseudobutyrivibrio van Gylswyk, Hippe and Rainey, 1996, 561”, in: Bergey’s manual of systematic bacteriology. New York: Springer.
Ransom-Jones, E., Jones, D. L., Mccarthy, A. J., and Mcdonald, J. E. (2012). The fibrobacteres: An important phylum of cellulose-degrading bacteria. Microb. Ecol. 63, 267–281. doi: 10.1007/s00248-011-9998-1
Ravachol, J., De Philip, P., Borne, R., Mansuelle, P., Maté, M. J., Perret, S., et al. (2016). Mechanisms involved in xyloglucan catabolism by the cellulosome-producing bacterium Ruminiclostridium cellulolyticum. Sci. Rep. 6:22770. doi: 10.1038/srep22770
R Core Team (2016). R: A Language and Environment for Statistical Computing. Vienna: R Foundation for Statistical Computing.
Rey, M., Enjalbert, F., Combes, S., Cauquil, L., Bouchez, O., and Monteils, V. (2014). Establishment of ruminal bacterial community in dairy calves from birth to weaning is sequential. J. Appl. Microbiol. 116, 245–257.
Rius, A. G., Kittelmann, S., Macdonald, K. A., Waghorn, G. C., Janssen, P. H., and Sikkema, E. (2012). Nitrogen metabolism and rumen microbial enumeration in lactating cows with divergent residual feed intake fed high-digestibility pasture. J. Dairy Sci. 95, 5024–5034. doi: 10.3168/jds.2012-5392
Ryan, W. (1990). Compensatory growth in cattle and sheep. Nutr. Abst. Rev. Ser. Livestock Feeds Feed. 60, 653–664.
Salvetti, E., Felis, G. E., Dellaglio, F., Castioni, A., Torriani, S., and Lawson, P. A. (2011). Reclassification of Lactobacillus catenaformis (Eggerth 1935) Moore and Holdeman 1970 and Lactobacillus vitulinus Sharpe et al. 1973 as Eggerthia catenaformis gen. nov., comb. nov. and Kandleria vitulina gen. nov., comb. nov., respectively. Int. J. Syst. Evol. Microbiol. 61, 2520–2524. doi: 10.1099/ijs.0.029231-0
Sauvant, D., Giger-Reverdin, S., Serment, A., and Broudiscou, L. (2011). Influences of diet and rumen fermentation on methane production by ruminants. INRA Productions Anim. 24, 433–446. doi: 10.20870/productions-animales.2011.24.5.3276
Schären, M., Drong, C., Kiri, K., Riede, S., Gardener, M., Meyer, U., et al. (2017). Differential effects of monensin and a blend of essential oils on rumen microbiota composition of transition dairy cows. J. Dairy Sci. 100, 2765–2783. doi: 10.3168/jds.2016-11994
Seedorf, H., Kittelmann, S., Henderson, G., and Janssen, P. H. (2014). RIM-DB: a taxonomic framework for community structure analysis of methanogenic archaea from the rumen and other intestinal environments. PeerJ 2:e494. doi: 10.7717/peerj.494
Seedorf, H., Kittelmann, S., and Janssen, P. H. (2015). Few highly abundant operational taxonomic units dominate within rumen methanogenic archaeal species in New Zealand sheep and cattle. Appl. Environ. Microbiol. 81, 986–995. doi: 10.1128/AEM.03018-14
Seshadri, R., Leahy, S. C., Attwood, G. T., Teh, K. H., Lambie, S. C., Cookson, A. L., et al. (2018). Cultivation and sequencing of rumen microbiome members from the Hungate1000 Collection. Nat. Biotechnol. 36:359. doi: 10.1038/nbt.4110
Shamay, A., Werner, D., Moallem, U., Barash, H., and Bruckental, I. (2005). Effect of nursing management and skeletal size at weaning on puberty, skeletal growth rate, and milk production during first lactation of dairy heifers. J. Dairy Sci. 88, 1460–1469. doi: 10.3168/jds.S0022-0302(05)72814-9
Smith, P. E., Enriquez-Hidalgo, D., Hennessy, D., Mccabe, M. S., Kenny, D. A., Kelly, A. K., et al. (2020). Sward type alters the relative abundance of members of the rumen microbial ecosystem in dairy cows. Sci. Rep. 10, 1–10. doi: 10.1038/s41598-020-66028-3
Snelling, T. J., Auffret, M. D., Duthie, C.-A., Stewart, R. D., Watson, M., Dewhurst, R. J., et al. (2019). Temporal stability of the rumen microbiota in beef cattle, and response to diet and supplements. Anim.Microbiome 1:16. doi: 10.1186/s42523-019-0018-y
Solden, L. M., Hoyt, D. W., Collins, W. B., Plank, J. E., Daly, R. A., Hildebrand, E., et al. (2016). New roles in hemicellulosic sugar fermentation for the uncultivated Bacteroidetes family BS11. ISME J. 11:691. doi: 10.1038/ismej.2016.150
Solden, L. M., Hoyt, D. W., Collins, W. B., Plank, J. E., Daly, R. A., Hildebrand, E., et al. (2017). New roles in hemicellulosic sugar fermentation for the uncultivated Bacteroidetes family BS11. ISME J. 11:691.
Stanton, T., Duncan, S., and Flint, H. (2009). Genus XVI. Roseburia Stanton and Savage 1983a, 626”, in: Bergey’s manual of systematic bacteriology. New York: Springer.
Taschuk, R., and Griebel, P. J. (2012). Commensal microbiome effects on mucosal immune system development in the ruminant gastrointestinal tract. Anim. Health res. Rev. 13, 129–141. doi: 10.1017/S1466252312000096
Thomson, D. (1984). “The nutritive value of white clover,” in Forage legumes, ed. D. J. Thomson (Dunston, UK: British Grassland Society Occasional Symposium), 78–92.
Thurston, V., Dawson, K. A., and Strobel, H. J. (1994). Pentose utilization by the ruminal bacterium Ruminococcus albus. Appl. Environ. Microbiol. 60, 1087–1092. doi: 10.1128/aem.60.4.1087-1092.1994
Ungerfeld, E. M. (2020). Metabolic hydrogen flows in rumen fermentation: principles and possibilities of interventions. Front. Microbiol. 11:589. doi: 10.3389/fmicb.2020.00589
Van Kessel, J. A. S., and Russell, J. B. (1996). The effect of pH on ruminal methanogenesis. FEMS Microbiol. Ecol. 20, 205–210. doi: 10.1111/j.1574-6941.1996.tb00319.x
Walters, W. A., Caporaso, J. G., Lauber, C. L., Berg-Lyons, D., Fierer, N., and Knight, R. (2011). PrimerProspector: de novo design and taxonomic analysis of barcoded polymerase chain reaction primers. Bioinformatics 27, 1159–1161. doi: 10.1093/bioinformatics/btr087
Wang, Z., Elekwachi, C., Jiao, J., Wang, M., Tang, S., Zhou, C., et al. (2017). Changes in metabolically active bacterial community during rumen development, and their alteration by rhubarb root powder revealed by 16S rRNA amplicon sequencing. Front. Microbiol. 8:159. doi: 10.3389/fmicb.2017.00159
Wardrop, I. (1966). The effects of the plane of nutrition in early post-natal life on the subsequent growth and development of cattle. Aus. J. Agric. Res. 17, 375–385. doi: 10.1071/AR9660375
Weimer, P. J. (1998). Manipulating ruminal fermentation: a microbial ecological perspective. J. Anim. Sci. 76, 3114–3122. doi: 10.2527/1998.76123114x
Weimer, P. J. (2015). Redundancy, resilience and host specificity of the ruminal microbiota: Implications for engineering improved ruminal fermentations. Front. Microbiol. 6:296. doi: 10.3389/fmicb.2015.00296
Williams, S. R. O., Hannah, M., Jacobs, J. L., Wales, W. J., and Moate, P. J. (2019). Volatile fatty acids in ruminal fluid can be used to predict methane yield of dairy cows. Animals 9:1006. doi: 10.3390/ani9121006
Wise, G. H., and Anderson, G. (1939). Factors affecting the passage of liquids into the rumen of the dairy Calf. I. method of administering liquids: drinking from open pail versus sucking through a rubber Nipple1. J. Dairy Sci. 22, 697–705. doi: 10.3168/jds.S0022-0302(39)92926-7
Xie, F., Zhang, L., Jin, W., Meng, Z., Cheng, Y., Wang, J., et al. (2019). Methane emission, rumen fermentation, and microbial community response to a nitrooxy compound in low-quality forage fed hu sheep. Curr. Microbiol. 76, 435–441. doi: 10.1007/s00284-019-01644-5
Yáñez-Ruiz, D. R., Abecia, L., and Newbold, C. J. (2015). Manipulating rumen microbiome and fermentation through interventions during early life: a review. Front. Microbiol. 6:1133. doi: 10.3389/fmicb.2015.01133
Yáñez-Ruiz, D. R., Macías, B., Pinloche, E., and Newbold, C. J. (2010). The persistence of bacterial and methanogenic archaeal communities residing in the rumen of young lambs. FEMS Microbiol. Ecol. 72, 272–278. doi: 10.1111/j.1574-6941.2010.00852.x
Yeoman, C. J., Ishaq, S. L., Bichi, E., Olivo, S. K., Lowe, J., and Aldridge, B. M. (2018). Biogeographical differences in the influence of maternal microbial sources on the early successional development of the bovine neonatal gastrointestinal tract. Sci. Rep. 8, 1–14. doi: 10.1038/s41598-018-21440-8
Keywords: calves, early-life, microbial community, fermentation, dietary transitions, enteric emissions
Citation: Cristobal-Carballo O, McCoard SA, Cookson AL, Laven RA, Ganesh S, Lewis SJ and Muetzel S (2021) Effect of Divergent Feeding Regimes During Early Life on the Rumen Microbiota in Calves. Front. Microbiol. 12:711040. doi: 10.3389/fmicb.2021.711040
Received: 17 May 2021; Accepted: 31 August 2021;
Published: 20 October 2021.
Edited by:
Diego P. Morgavi, INRAE Clermont-Auvergne-Rhône-Alpes, FranceReviewed by:
Jeyamalar Jeyanathan, Ghent University, BelgiumCopyright © 2021 Cristobal-Carballo, McCoard, Cookson, Laven, Ganesh, Lewis and Muetzel. This is an open-access article distributed under the terms of the Creative Commons Attribution License (CC BY). The use, distribution or reproduction in other forums is permitted, provided the original author(s) and the copyright owner(s) are credited and that the original publication in this journal is cited, in accordance with accepted academic practice. No use, distribution or reproduction is permitted which does not comply with these terms.
*Correspondence: Stefan Muetzel, c3RlZmFuLm11ZXR6ZWxAYWdyZXNlYXJjaC5jby5ueg==
Disclaimer: All claims expressed in this article are solely those of the authors and do not necessarily represent those of their affiliated organizations, or those of the publisher, the editors and the reviewers. Any product that may be evaluated in this article or claim that may be made by its manufacturer is not guaranteed or endorsed by the publisher.
Research integrity at Frontiers
Learn more about the work of our research integrity team to safeguard the quality of each article we publish.