- 1Laboratory of Emerging Pathogens, Division of Emerging and Transfusion Transmitted Diseases, Office of Blood Research and Review, Center for Biologics Evaluation and Research, Food and Drug Administration, Silver Spring, MD, United States
- 2Department of Bioscience and Biotechnology, Banasthali Vidyapith, Banasthali, India
- 3National Center for Biotechnology Information, National Library of Medicine, National Institutes of Health, Bethesda, MD, United States
- 4Department of Epidemiology of Microbial Diseases, Yale School of Public Health and Yale School of Medicine, New Haven, CT, United States
More than 100 Babesia spp. tick-borne parasites are known to infect mammalian and avian hosts. Babesia belong to Order Piroplasmid ranked in the Phylum Apicomplexa. Recent phylogenetic studies have revealed that of the three genera that constitute Piroplasmida, Babesia and Theileria are polyphyletic while Cytauxzoon is nested within a clade of Theileria. Several Babesia spp. and sub-types have been found to cause human disease. Babesia microti, the most common species that infects humans, is endemic in the Northeastern and upper Midwestern United States and is sporadically reported elsewhere in the world. Most infections are transmitted by Ixodid (hard-bodied) ticks, although they occasionally can be spread through blood transfusion and rarely via perinatal transmission and organ transplantation. Babesiosis most often presents as a mild to moderate disease, however infection severity ranges from asymptomatic to lethal. Diagnosis is usually confirmed by blood smear or polymerase chain reaction (PCR). Treatment consists of atovaquone and azithromycin or clindamycin and quinine and usually is effective but may be problematic in immunocompromised hosts. There is no human Babesia vaccine. B. microti genomics studies have only recently been initiated, however they already have yielded important new insights regarding the pathogen, population structure, and pathogenesis. Continued genomic research holds great promise for improving the diagnosis, management, and prevention of human babesiosis, and in particular, the identification of lineage-specific families of cell-surface proteins with potential roles in cytoadherence, immune evasion and pathogenesis.
Introduction
Human babesiosis is an emerging tick-borne disease caused by several Babesia species found in the temperate regions of the world. Babesia and related Theileria organisms are intraerythrocytic parasites that belong to the same phylum (Apicomplexa) as Plasmodia with which they share several microbiologic and pathogenic features. Among the many Babesia species that can infect humans, Babesia microti is the most prevalent and the primary cause of human babesiosis. Disease due to this pathogen is endemic in the Northeastern and northern Midwestern United States and reported sporadically in other temperate regions of the world (Figure 1) (Homer et al., 2000; Matsui et al., 2000; Hunfeld et al., 2008; Vannier and Krause, 2012; Zhou et al., 2014; Liu et al., 2017). B. microti is primarily transmitted by Ixodes scapularis ticks but also through blood transfusion, organ transplantation, and perinatally (Fox et al., 2006; Herwaldt et al., 2011; Brennan et al., 2016). Other Babesia spp. that are known to infect humans include Babesia crassa-like agent, Babesia divergens, B. divergens-like agent, Babesia duncani, B. microti-like agent, Babesia motasi, and Babesia venatorum. A comprehensive list of Babesia spp. that are reported to cause human babesiosis, their areas of transmission, reservoir hosts and tick-vectors are provided in Table 1.
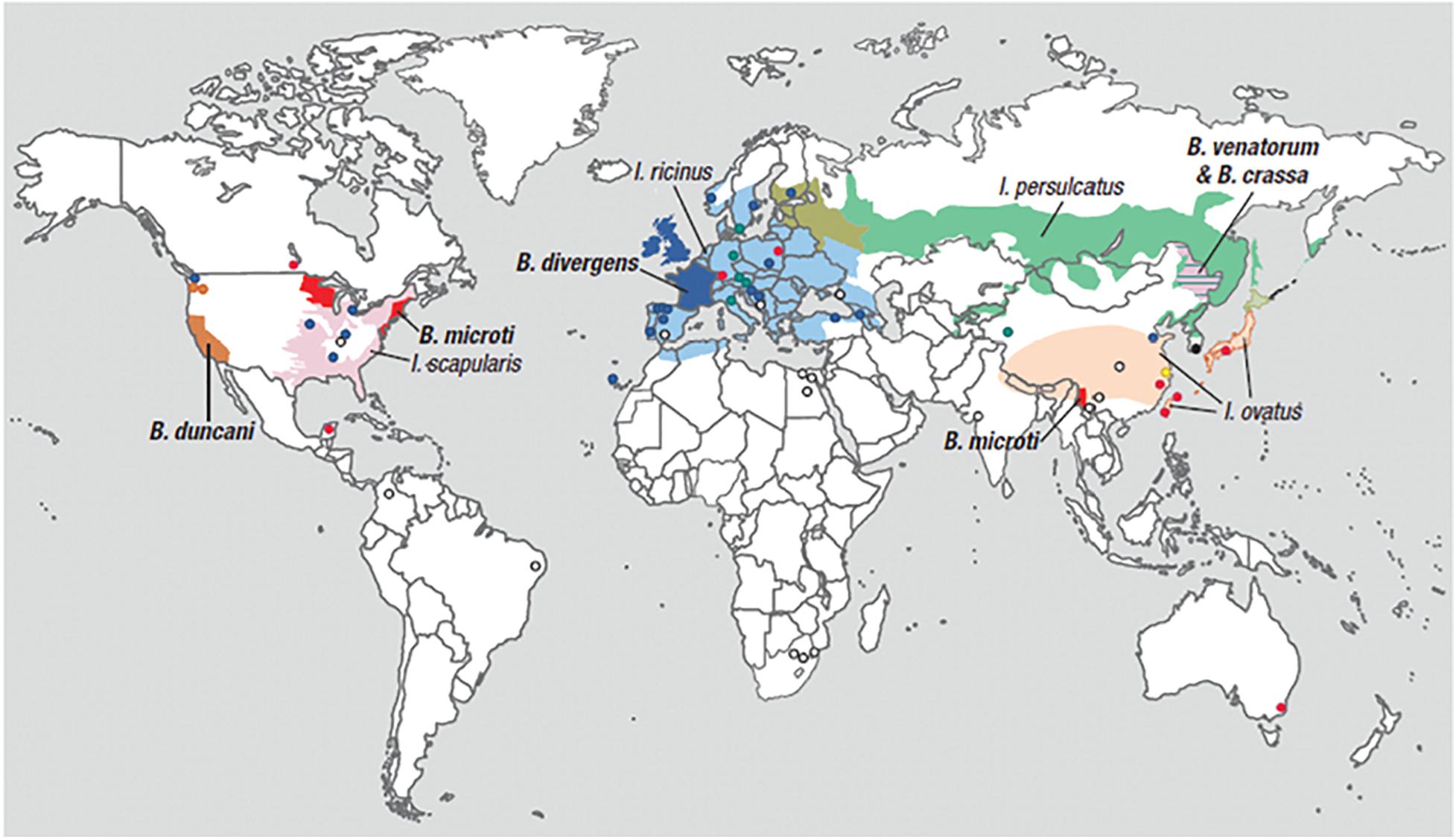
Figure 1. Geographic range of Ixodes-tick vectors and location of human babesiosis reports. The lighter colors represent the geographical range of several Ixodes tick species known or suspected to transmit Babesia parasites to humans but where human babesiosis is rare (<5 cases), unreported or absent. Ixodes ricinus and Ixodes persulcatus are sympatric in southern Finland, Estonia, Latvia, and northwestern Russia, while I. persulcatus and Ixodes ovatus are both enzootic in Hokkaido in northern Japan. The darker colors represent regions where human babesiosis is endemic or sporadic (defined by ≥5 cases). Circles indicate single cases, except in three locations (Mexico, Montenegro, and eastern Poland) where all patients at these locations were reported from the same hospital. Colors indicate Babesia species: red for B. microti, orange for B. duncani, blue for B. divergens, green for B. venatorum, pink for B. crassa like agent, black for KO-1, yellow for Babesia spp. XXB/HangZhou, and white for undetermined Babesia spp. The map does not show asymptomatic cases and travel-associated cases [adapted from Vannier and Krause (2012) and (Ryan et al., 2019) from The New England Journal of Medicine, Edouard Vannier, PhD., and Peter J. Krause, M. D., Human Babesiosis, 366, 2399. Copyright (2012) Massachusetts Medical Society. Reprinted with permission.
Most Babesia spp. are small (1–5 μm in length) and are pear-shaped, oval, or round with blue cytoplasm and red chromatin dots (DNA) when viewed on Giemsa-stained blood smears (Healy and Ruebush, 1980). Ring forms are the most common configurations noted on blood smears and are similar to those of Plasmodium falciparum. Babesia differ from P. falciparum by the absence of hemozoin pigment deposit in the ring form, the lack of schizonts and banana-shaped gametocytes, and the presence of tetrads (Maltese cross) (Figure 2) (Vannier and Krause, 2012). The latter are seldom noted but, if present, are pathognomonic of Babesia.
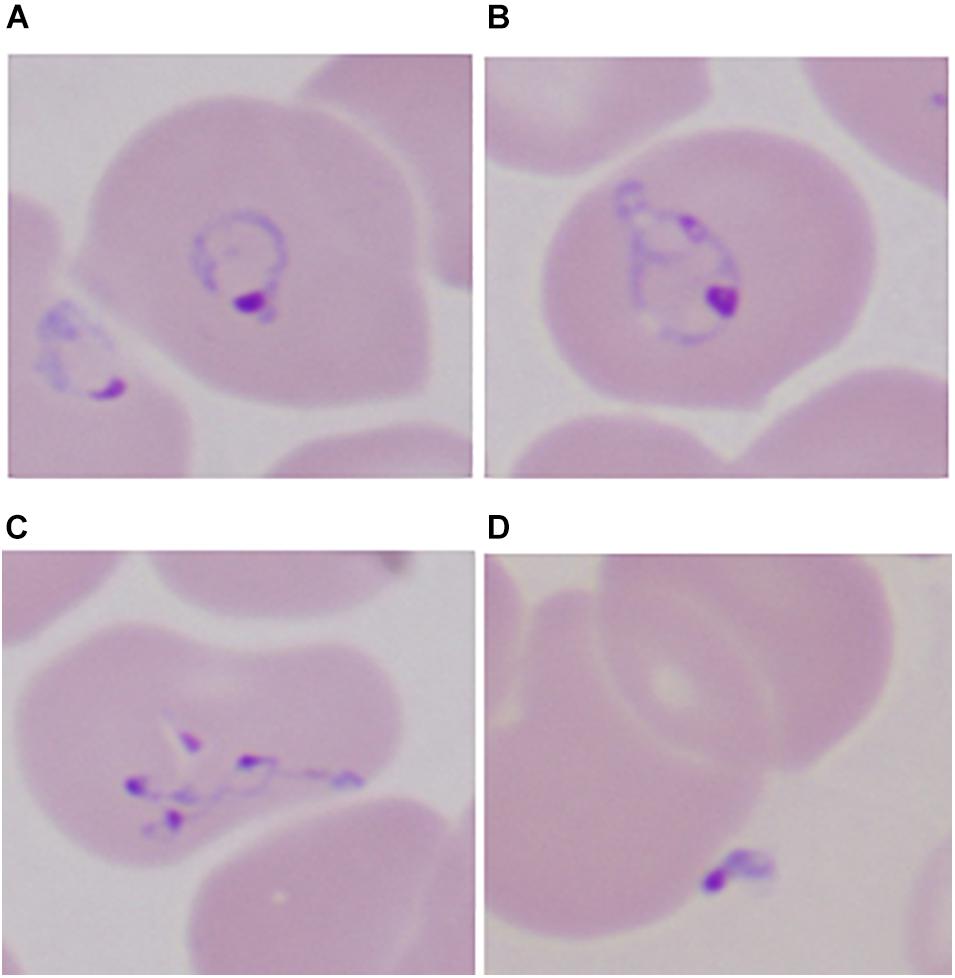
Figure 2. Developmental stages of intraerythrocytic Babesia microti parasite by Giemsa-stained thin blood microscopy. (A) Ring form with cytoplasm stained blue; (B) mature trophozoite with two small nuclei; (C) a tetrad (Maltese cross); and (D) an extracellular form. 1000× magnification. Adapted from Vannier and Krause (2012) (From The New England Journal of Medicine, Edouard Vannier, PhD., and Peter J. Krause, M. D., Human Babesiosis, 366, 2399. Copyright (2012) Massachusetts Medical Society. Reprinted with permission).
Babesiosis usually causes mild to moderate illness but asymptomatic infection may occur, and severe life-threatening disease occurs in immunocompromised hosts, such as those with asplenia, cancer, HIV/AIDS, the extremes of age (neonates and those over 50 years), and people taking immunosuppressive drugs (Falagas and Klempner, 1996; Hatcher et al., 2001; Froberg et al., 2004; Krause et al., 2008; Vannier and Krause, 2012; Menis et al., 2015; Dumic et al., 2020). Complications include acute respiratory distress syndrome (ARDS), severe anemia, congestive heart failure, disseminated intravascular coagulopathy (DIC), liver and renal failure, and shock (Hatcher et al., 2001; Krause et al., 2007; Dumic et al., 2020). We have a limited understanding of the pathogenesis of babesiosis but excessive proinflammatory cytokine production, cytoadherence of infected red blood cells, and severe anemia are posited as important pathologic mechanisms (Clark and Jacobson, 1998; Hemmer et al., 2000; Allred and Al-Khedery, 2004; Krause et al., 2007). Diagnosis is suspected on epidemiologic and clinical grounds and confirmed by blood smear and/or polymerase chain reaction (PCR; Krause et al., 2021). Treatment is generally effective with the use of atovaquone and azithromycin or clindamycin and quinine but may be problematic in highly immunocompromised hosts where acute fulminant disease may be fatal or prolonged antibiotic therapy may lead to antibiotic resistance (Krause et al., 2000, 2021; Wormser et al., 2010; Lemieux et al., 2016; Simon et al., 2017).
Genomics is the branch of molecular biology that focuses on the structure, function, evolution, and mapping of genomes. It aims at the characterization and quantification of all the genes and their interactions that affect the function of the organism. B. microti genomic studies have only recently been initiated but already have delivered important new insights regarding pathogen genomic structure, population structure, evolution, and pathogenesis; as well as biomarkers of detection, drug resistance markers, targets for novel therapeutics, and vaccines (Figure 3).
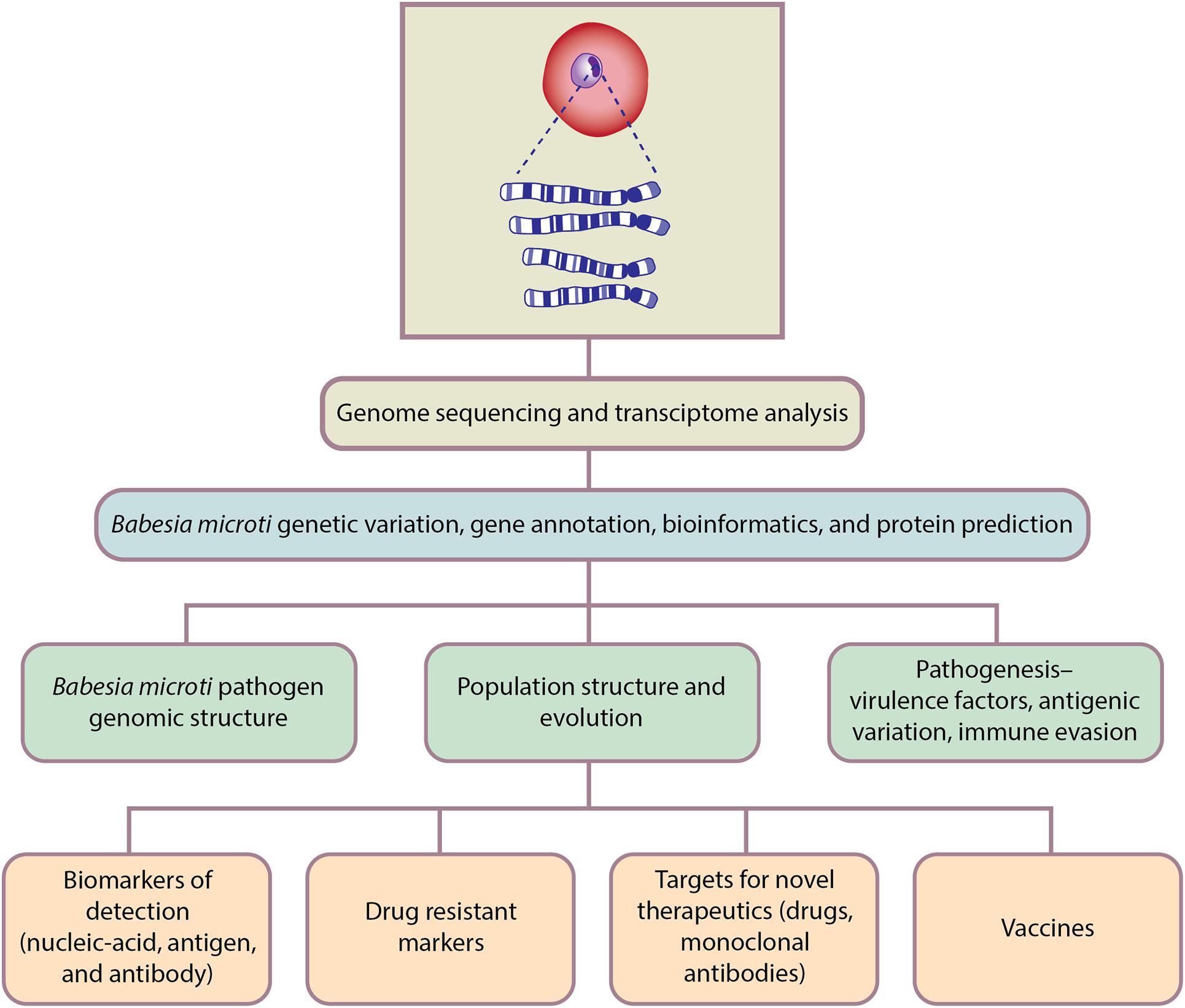
Figure 3. Genomics-based approaches for Babesia microti detection, molecular surveillance, understanding pathogenesis and developing therapeutics.
Babesia microti Pathogen Genomics
Babesia microti Genome Organization
The B. microti genome sequence was first published in 2012 (Cornillot et al., 2012). The B. microti genome is comprised of 4 chromosomes that contains approximately 6.5 megabase pairs (Mbp) that encode around 3,500 polypeptides, which is the smallest of all Apicomplexan genomes (Cornillot et al., 2012). A reannotation analysis of the B. microti genome sequence has identified 3,615 genes which encode for 3,567 proteins (Silva et al., 2016). A salient feature of the B. microti genome is the preponderance of unusually small introns that range in size between 18 and 21 base pairs, some of which are reported to be in frame, which is rare in eukaryotes (Silva et al., 2016). In contrast to the concentrated form of the mitochondrial genome in Plasmodium parasites, B. microti possesses a linear monomeric mitochondrial genome which contains a pair of unique repeats and a flip-flop inversion system. The flip-flop inversions may be involved in the switch on or off expression of the B. microti mitochondrial genes and gene fragments in their life cycle (Hikosaka et al., 2012).
In apicomplexan parasites, colocalization of virulence genes has been shown to influence spatial genome organization and is linked to virulence and survival (Bunnik et al., 2019). In P. falciparum, members belonging to multi-gene families that are responsible for virulence, antigenic variation, and immune evasion are colocalized in the genome (Reid, 2015; Bunnik et al., 2018, 2019). Studies of the comparative 3D genome organization of apicomplexan parasites has revealed that B. microti has a classical Rabl organization, which is similar to yeast and lacks a clear localization of virulent factor genes as seen with the P. falciparum parasite (Lenz and Le Roch, 2019). Thus, the B. microti genome organization is not strongly linked to the regulation of virulence genes.
Babesia microti Phylogeny and Taxonomy
The apicomplexan clade of Hematozoa, which includes parasites infecting vertebrate hemocytes, is further divided into two major clades – the well-studied Haemosporidia, such as Plasmodium, and the Piroplasmida. Piroplasmida includes the traditionally defined genera such as Babesia and Theileria. Phylogenetic analyses based on the nucleus-encoded genes revealed that the recently described parasites/symbionts of the ascidian tunicates, Cardiosporidium (infecting Ciona) and Nephromyces (infecting Molgula) have emerged as a sister-group of all Hematozoa (Munoz-Gomez et al., 2019). These parasites are monoxenous (i.e., complete their life cycle in a single host species), unlike the crown group Hematozoa which have a definitive invertebrate host and a secondary vertebrate host (Munoz-Gomez et al., 2019). This suggests that the transition from monoxenous blood-parasitism in ascidians, a sister group of the vertebrates, to the dixenous parasitism typical of Hematozoa happened early in their evolution with the emergence of invertebrate blood-feeders that could serve as vectors. While piroplasms retain many of the features of this ancestral vertebrate blood parasite, species classified in the genus Babesia exhibit specialized modes of transmission, such as transovarial transmission from females to their offspring, as well as transstadial transmission between different stages in the life cycle of the tick vector. These features appear to have emerged as adaptations to their arachnid vectors.
Traditionally, piroplasms have been classified based on morphological/life-history features such as presence (Theileria and Cytauxzoon) or absence (Babesia) of schizogony; as well as size- “small” species (e.g., B. microti and B. duncani) versus “large” species (e.g., Babesia bigemina). However, a series of molecular phylogenetic studies over the past decade (reviewed in Jalovecka et al., 2019) using nuclear and apicoplast sequences have shown that the traditional morphological taxonomy of Babesia and allied genera does not reflect their true evolutionary relationships. Notably, these studies have shown that the genera of Babesia and Theileria are not monophyletic and that the genus Cytauxzoon is nested with the lineages that have been assigned to the former two genera (Lack et al., 2012). Instead, these studies suggest that the Babesia-Theileria-Cytauxzoon complex can be divided into 10 monophyletic clades that encompass 14 reference species included in the genus Babesia, 9 species included in Theileria and 2 species included in Cytauxzoon. Piroplasms infect a wide range of eutherian mammals, marsupials (e.g., Theileria spp. Clade IV), monotremes (Theileria ornythorhynchi, Clade II) and birds (e.g., Babesia ugwidiensis, Clade V) (Schnittger et al., 2012). While reptilian-parasitic piroplasms, such as Sauroplasma and Serpentoplasma have been reported (Adl et al., 2019), their relationship to the above complex remains unclear. Nevertheless, the widespread presence of the Babesia-Theileria-Cytauxzoon complex in warm-blooded vertebrates suggests that they have likely emerged early in the history of these vertebrates and have repeatedly jumped hosts (Jalovecka et al., 2019).
Consistent with this possibility, human parasitism among piroplasms has emerged on multiple occasions, most likely through accidental extension of the parasite host range due to interactions with ticks feeding on infected domesticates or wildlife. Of these, the B. microti-like group, defines “clade I,” which is the earliest branching clade of the Babesia-Theileria-Cytauxzoon complex. This clade includes four distinct lineages within it: Clade Ia parasites that infect rodents, monkeys and humans (e.g., B. microti; the primary agent of human babesiosis); Clade Ib parasites that infect canids and mustelids (e.g., Babesia vulpes); Clade Ic parasites that infect rodents (e.g., Babesia rodhaini); and Clade Id parasites that infect cats (e.g., Babesia felis). Like other piroplasms, clade I Babesia show transstadial transmission but lack transovarial transmission typical of the “classic Babesia” of clade X (Babesia species “sensu stricto”). In addition to the Clade I B. microti-like group, clades of other human-infecting piroplasms include members of Clade III (the “Western group”) with representatives like B. duncani, Clade IX comprised of Theileria sensu stricto (e.g., T. annulata, T. parva, and T. orientalis), and Clade X consisting of Babesia sensu stricto (e.g., B. divergens and B. venatorum). With over 90 species infecting a variety of wild and domestic mammalian hosts (Schnittger et al., 2012), several piroplasms have a significant worldwide veterinary economic impact. These include: (1) the bovine-infecting species, e.g., B. bigemina, B. bovis, and B. divergens; (2) the equid-infecting species, e.g., Theileria equii and Babesia caballi; (3) sheep/goat-parasites, e.g., Babesia ovis and B. crassa; and (4) carnivoran parasites, e.g., B. felis and B. canis (for review see Schnittger et al., 2012).
Given the distinct features of the B. microti and B. microti-like group from the Babesia sensu stricto group, it has been argued that in a future taxonomic revision the B. microti and B. microti-like group should be elevated to a separate genus along with the other distinct clades identified in recent studies (Rudzinska, 1976; Rudzinska et al., 1976; Goethert and Telford, 2003; Cornillot et al., 2012; Lack et al., 2012; Schnittger et al., 2012; Jalovecka et al., 2019). Additional studies are needed that include the creation of a large database of whole genome sequencing of Babesia spp. This will provide a complete examination of the genetic diversity of Babesia spp. that have such a significant worldwide public health impact.
Genetic Variability in Babesia microti Populations
Genomic studies over the past decade have refined our understanding of the epidemiological characteristics of B. microti. In one study, genome sequence analyses of 42 B. microti samples from around the world show extensive genetic diversity (Lemieux et al., 2016). B. microti isolates from within the continental United States show a relatively stable genetic structure but these isolates possess significant genetic diversity compared with samples from geographically distant Alaska, Russia, and Japan. In the continental United States, two distinct genetic B. microti sub-populations were noted in the Northeast and Midwest regions. The authors predicted that these two parasite lineages entered the continent at different time points separated by more than 700 years (Lemieux et al., 2016). In the same study, parasite variants containing amino acid substitutions in the rp14, a subunit of riboendonuclease, were associated with relapsing disease. Additionally, genetic mutations in the atovaquone-binding regions of cytochrome b and the azithromycin-binding region of ribosomal protein subunit L4 were also identified. Based on the available whole genome wide sequence data, a 25 single nucleotide polymorphism (SNP) barcode was subsequently developed that supported the previous findings and identified two distinct B. microti lineages in the northeastern and midwestern United States (Baniecki et al., 2019).
The genetic diversity and population structure of B. microti parasites in the endemic regions of the northeastern United States has been characterized by Carpi et al. (2016). They employed the multiplex capture platform for characterizing genome-wide diversity and genetic relatedness in 25 B. microti isolates obtained from tick-vectors and humans. Their results showed that in the northeastern United States, B. microti was strongly structured into three highly differentiated genetic clusters. Interestingly, analyses of the apicoplast genome indicated that in the Northeast, the current genetic diversity in B microti dates back 46,000 years with evidence of population expansion in the past 1,000 years (Carpi et al., 2016). In another study, a total of 228 B. microti patient isolates from the New England area were genotyped by using variable number tandem repeat markers. Their results also showed the presence of three distinct B. microti population structures with each dominated by a single ancestral type (Goethert et al., 2018). The authors concluded that B. microti parasites prevalent in the northeastern United States have expanded from a common ancestral origin(s) on the mainland and not from Nantucket or other islands off the New England mainland where babesiosis was first reported to be endemic.
In summary, the genetic variability of B. microti in the United States is limited and different from B. microti in other parts of the world. B. microti can be divided into northeastern and midwestern groups with further division of three genetic clusters in the Northeast. It remains to be determined whether these closely related but different genetic groups are associated with different clinical severity.
Babesia microti Antigenic Polymorphism and Immunodominant Antigens
The publicly available whole B. microti genome sequence database is still limited and the majority of information comes from parasite isolates collected in endemic areas in United States. In one study, genomics and gene expression profiling experiments were performed to identify polymorphic genes involved in host–parasite interactions. Analyses based on seven B. microti isolates from the northeastern United States revealed that antigenic polymorphism is generally restricted to a small number of highly expressed genes which belonged to the parasite surface proteome and secretome (Silva et al., 2016). Among the 3568 protein coding genes, 205 genes carried a total of 257 amino acid altering mutations, 27 of which contained nearly all mutations (Silva et al., 2016). It is possible that these surface expressed antigens are major immune targets and subjected to antigen variation that allows the parasite to escape host immunological surveillance and establish long term chronic infection.
In the past two decades, several groups have made efforts to identify and characterize immunodominant B. microti antigens to study their potential role as mediators of immunity and pathogenesis and for diagnostic and vaccine use. The majority of currently available antigens have been identified by screening B. microti genomic expression libraries against sera from infected mice or humans (Lodes et al., 2000; Homer et al., 2003; Xu et al., 2018; Zhou et al., 2018; Verma et al., 2020). The most prominent among these proteins are the diverse polymorphic multigene antigens termed the BMN (B. microti MN1 strain seroreactive antigen) family of antigens. Sequence analyses revealed patient-to-patient variation in the number and location of the sequence repeats within the BMN1-6 homolog. Furthermore, parasite isolates from nearby geographical locations were genetically similar compared to those from geographically distant locations (Lodes et al., 2000).
In two recent reports, immuno-screening of protein arrays based on B. microti antigen expressed in Escherchia coli has led to identification of several immuno-reactive antigens, some of which were previously uncharacterized (Xu et al., 2018; Zhou et al., 2018). In another study, Elton et al. (2019) employed a mammalian expression system to identify 54 genes that are predicted to encode surface and secreted B. microti blood stage antigens. The recombinant antigens produced were conformationally dependent and were used to measure the kinetics of antibody responses during early and late phase B. microti infection in BALB/c mice. Antibody responses against several antigens were noted during the entire course of infection. While some mice were able to mount antibody responses against all immunoreactive antigens, other mice generated antibody responses against only a subset of antigens. These results are surprising because the mice used in the study were major histocompatibility (MHC) compatible. Some immunoreactive antigens continued to elicit antibody responses beyond day 125 following initial infection. Interestingly, a broadly similar profile of antibody reactivity was observed against the same antigen panel in serum samples obtained from mice that were infected with a genetically distant strain of B. microti. A heterogenic antibody response against a subset of these antigens also was observed in sera from babesiosis patients, although the sample size was too small to draw any firm conclusions.
Several studies have applied computational and bioinformatics analyses to predict B. microti proteins that could be involved in host–parasite interactions, immune evasion, and potential targets of antigenic polymorphism. Using this approach, Silva et al. predicted 420 proteins that putatively belonged to the B. microti secretome (Silva et al., 2016). Next, a protein array was constructed consisting of 174 proteins that are predicted to trigger immune responses in hosts during infection. Immuno-screening using sera from B. microti-infected mice identified 30 highly antigenic proteins. Fourteen of 30 proteins, including the two most antigenic proteins, were a part of the secretome (Silva et al., 2016). In a subsequent study using a 17-plex protein array based on glycosylphosphatidylinositol (GPI)-anchored proteins, BmGP12 was identified as a potential biomarker for detection of past or current B. microti infections in laboratory and field captured mice, as well as in babesiosis patients and healthy residents living in New England (Cornillot et al., 2016).
In a more recent study of B. microti immunodominant antigens, genome-wide immune-screening led to the discovery of 56 novel B. microti antigens, many previously uncharacterized (Verma et al., 2020). Five immunodominant B. microti proteins that were identified in the study were cell-surface proteins possessing antigenic extracellular domains characteristic of adherence proteins that interact with host proteins. For instance, one of these is a previously uncharacterized protein with four copies of the epidermal growth factor (EGF) domains BmEGF1 (BmR1_03g00690). While EGF domain repeat proteins are widely known in Apicomplexa (Anantharaman et al., 2007), this protein is not closely related to any of them and its EGF domains share specific features in the pattern of conserved cysteines with certain proteins from Giardia intestinalis and animal secreted proteins (Verma et al., 2020). Consistent with this data, confocal microscopy studies showed that two of these antigens were expressed on the surface of infected erythrocytes, raising the possibility of their role as cytoadherence antigens. A combination of these two novel antigens and one previously described antigen provided 96% sensitivity and 100% specificity in detecting B. microti specific antibodies in babesiosis patients using an enzyme-linked immunosorbent assay (ELISA) platform (Verma et al., 2020).
Two of these five immunodominant proteins that are the most reactive B. microti antigens (BmR1_03g04855 and BmR1_03g00785) belong to the BMN class of proteins (Lodes et al., 2000; Homer et al., 2003; Silva et al., 2016; Xu et al., 2018; Zhou et al., 2018; Verma et al., 2020). A comprehensive analysis of these proteins shows that BMN class proteins do not constitute a monophyletic group and should not be considered as a unified “BMN family.” Instead, the majority can be classified into multiple evolutionarily unrelated groups of BMN proteins.
The first major group of BMN proteins generally correlate with the following families: (i) BMN1–10, N1–10, BMN1–4, BMN1–3B, BMN1–8, and BMN1–11 proteins from the B. microti MN1 strain, (ii) the IRA protein from the B. microti Gray strain and, (iii) the Br-1 and Br-2 proteins from the B. rodhaini Japanese strain. These proteins correspond in large part to the antigens termed BMN1 by Silva et al. (2016). B. microti R1 was found to possess 10 members of this group, including BmR1_03g04855 (Table 3 in Verma et al., 2020). They are characterized by one to five copies per protein of a domain with a N-terminal 8 β-strand sandwich, followed by a C-terminal disulfide bond-supported structure. Divergent versions of this domain are found outside of the B. microti-like strain, in a group of secreted proteins expanded across Theileria. Hence, this domain was named piroplasm β-strand (PiβS) domain.
The second group of BMN proteins is comprised of BMN1–2, BMN1–3, BMN1–6, BMN1–7, BMN1–9, BMN1–13, BMN1–4, MN-10, N1–21, all from the B. microti MN1 strain; BmSA1 from the B. microti Gray strain; BmP32 from the B. microti Munich strain; MSA1 and MSA2 from B. rodhaini Australia strain; and Br-1, p25, and p26 from the B. rodhaini Japanese strain. The Br-1, p25, and p26 proteins correspond in large part to the antigens termed BMN2 by Silva et al., 2016. Nine proteins in the second group of BMN proteins are present in the B. microti R1 strain (Table 3 in Verma et al., 2020). These are distinguished by a novel B. microti-like group-specific extracellular domain with 9 α-helices and a C-terminal hydrophobic GPI-anchor. Accordingly, it was named the Babesia α-helical cell surface (BAHCS) domain. In contrast to the PiβS domain, the BAHCS domain is always found in a single copy in a protein; however, on occasions both these domains might occur in the same protein (e.g., Br-1 from B. rodhaini and its cognate BMN1–4 from the B. microti MN1 strain). Both the PiβS and BAHCS domains shows a similar evolutionary tendency for independent lineage-specific expansions in B. rodhaini and B. microti along with notable inter-strain radiations in the later species (Verma et al., 2020). Beyond these, smaller BMN groups are formed by the paralogous BMN1–17 and BMN1–20 proteins and the Maltese cross form-related antigen (BMN1–15/N1–15) and BmR1_02g04285 (BmMCFRP1) that are unrelated to these groups (Verma et al., 2020).
The biological relevance of the antigenically polymorphic BMN family antigens and other immunodominant family antigens, as well as other adherence antigens in B. microti parasites, remains to be determined. Their rapid evolution in phylogenetically close lineages and independent lineage-specific expansions between them are the hallmark of proteins in an arms race with the host. This is reminiscent of other apicomplexan surface proteins such as the rifin-like and the var/DBL1 superfamilies in P. falciparum, and the vir/yir superfamilies in Plasmodium vivax/P. yoelii (Anantharaman et al., 2007). Future studies are needed to determine whether any of these antigens expressed on the surface of B. microti-infected red blood cells contribute to an immune escape mechanism that leads to persistent infections in animals and humans.
While data on B. microti proteins that are derived from genomic studies are now expanding, our knowledge of the parasite proteome based on direct protein profiling is very limited. By applying a combination of nanotechnology and mass spectroscopy (MS), Magni et al. (2019) have generated a proteome profile of intraerythrocytic B. microti parasites during acute phase infection in hamsters. They have identified ∼500 proteins with assigned functions, such as transport, carbohydrate and energy metabolism, signaling transport, mobility and invasion, and immune response. This proteome database could be exploited for novel diagnostic and vaccine targets and better understanding of parasite biology, host immunity, and pathogenesis. In a subsequent study, the nanotechnology-MS methods were applied to identify B. microti proteins in blood, serum and urine during infection in hamsters (Magni et al., 2020). A distinct but less abundant protein profile was observed in urine compared to blood and plasma. These studies highlight the potential value of the nanoparticle-MS methods to detect B. microti proteins in complex analytes such as blood and plasma.
Overall, data from whole genome, parasite genotyping, and expression profiling studies are helping to develop genetic tools for surveillance of acute and asymptomatic B. microti infections and for monitoring transmission in new endemic areas. Such studies are also expanding our knowledge of B. microti antigens that perform important functions, such as inducing protective immune responses and mediating host pathogenesis.
Babesia microti Pathogenesis Genomics
The pathogenesis of Babesia spp. infections in humans is multifactorial, complex, and incompletely understood. In particular, molecular processes that underlie pathogenic mechanisms in human babesiosis have not been well researched. Pathogenesis studies are complicated by variation in pathogenic mechanisms for different Babesia spp. and variation in the immunologic status and pathophysiological response of hosts. Three clinical patterns of human babesiosis have been described: acute symptomatic disease which may be mild, moderate or severe, acute asymptomatic infection, and persistent infection (Vannier et al., 2008; Vannier and Krause, 2012). Acute asymptomatic infection is the most common clinical presentation. Asymptomatic infection occurs in about 20% of adults and is characterized by low parasitemia. Persistent infection follows acute symptomatic or asymptomatic infection and may last as long as 2 years (Krause et al., 1998, 2007; Raffalli and Wormser, 2016).
Acute Disease
Several mechanisms may account for the severity of acute B. microti infection: an aberrant immune response that include excessive pro-inflammatory cytokine release, erythrocyte cytoadherence, persistent infection, and hemolytic anemia.
Aberrant Immune Response, Cytokine Storm
It is well recognized that immune responses that help protect the host against invading pathogens can also contribute to severe disease (Yokota, 2003; Huang et al., 2005). Over production of several pro-inflammatory cytokines during an array of infections is often associated with acute inflammation and tissue damage in the microenvironment of the lungs and other organs (Rubenfeld et al., 2005). The phenomenon of hyperimmune immune responsiveness leading to excessive cytokine production has been termed “cytokine storm” and is attributed to the most severe pathogenic consequences of infections such as SARS-CoV-2 infection (Fajgenbaum and June, 2020). Key cytokines involved in cytokine storm are TNF-α, IFN-γ, IL-1, IL-6, and IL-18. The major immune cell types involved are neutrophils, macrophages and NK cells.
Cytokine storm has been implicated in severe disease complications in babesiosis (Clark and Jacobson, 1998; Hemmer et al., 2000; Krause et al., 2007). Excessive TNF-α and IL-1 production by macrophages, Th1 lymphocytes, and other cells in response to high parasitemia, decreases capillary integrity and can cause multi-organ dysfunction, including ARDS (Hemmer et al., 2000). When the effect is more widespread with loss of intravascular fluid, hypotension and shock may result. Increased nitric oxide production following TNF-α and IL-1 release can help eradicate microbial pathogens but also cause tissue damage (Aguilar-Delfin et al., 2001; Vannier et al., 2015; Djokic et al., 2018). Elevated blood concentrations of TNF-α have been associated with the expression of adherence molecules ICAM-1 and VCAM-1 in vascular epithelium and with cerebral malaria in children (World Health Organization (WHO)., 2000; Krause et al., 2007).
The genomic underpinnings of cytokine storm have just begun to be elucidated. The dynamic of cytokine production leading to cytokine storm is complex and balanced by a number of factors, including proinflammatory cytokines and their cognate soluble receptor or inhibitors and the production of anti-inflammatory cytokines such as IL-10 (Tisoncik et al., 2012). Previous genomic studies have demonstrated the upregulation of proinflammatory cytokines in animal models during influenza infection (Kash et al., 2006; Kobasa et al., 2007; Cilloniz et al., 2009). The signature cytokine genes showing strong upregulation include IL-6, IL-8, CCL2, CCL-5, CXCL10, and CXCL9 (Baskin et al., 2009; Zhou et al., 2020). More recently, genomic databases generated from transcriptome analyses of cytokines, chemokines and immune cells during acute infections, such as those caused by influenza virus, dengue viruses, SARS-CoV-2, and other pathogens have begun to provide new insights regarding events leading to cytokine storm (Afroz et al., 2016; Hancock et al., 2018; Zhou et al., 2020).
Other mechanisms that influence cytokine-mediated regulation of severe disease include the association of TNF-α promoter polymorphisms (G-238A and G-308A) with susceptibility to diseases as diverse as systemic lupus erythematous and P. falciparum malaria (Mahto et al., 2019), as well as epigenetic regulation of cytokine storm in COVID-19 patients (Sawalha et al., 2020). Carefully designed transcriptome and cytokine profiling studies in severe babesiosis patients and in chronic asymptomatic Babesia infections would help to identify the molecular factors that lead to severe disease with fatal consequences in susceptible individuals. Such studies may help to develop immunotherapies that could ameliorate the most severe B. microti complications, such as hemolytic anemia, ARDS and kidney damage in babesiosis patients.
Erythrocyte Cytoadherence
Residence within red blood cells offers some protection for Babesia against host immune factors but Babesia infected erythrocytes are recognized and removed in the spleen. Cytoadherence of Babesia-infected erythrocytes to vascular endothelium is thought to allow the pathogen to complete its life cycle, leave the erythrocyte briefly, and infect another red blood cell without ever traversing the spleen. Excessive Babesia-induced erythrocyte adherence may contribute to babesiosis complications. Babesia-induced red cell cytoadherence has been associated with B. bovis and B. duncani induced pathology (Clark et al., 2006a; Usmani-Brown et al., 2013; Allred, 2019). B. bovis strains with increased cytoadherence activity in vitro have increased virulence in cattle (O’Connor et al., 1999). When parasitemia is high in B. bovis-infected cattle, a large number of infected red blood cells may adhere to small capillaries in the brain, causing vascular obstruction, anoxia and death of neurons. This is associated with the same stroke syndrome that is seen with cerebral malaria. Cytoadherence also has been associated with lung injury in B. duncani infected hamsters and mice (Dao and Eberhard, 1996; Hemmer et al., 1999, 2000; Krause et al., 2007), as well as renal injury in B. bovis-infected cattle (Patarroyo et al., 1982; Everitt et al., 1986). It remains unclear whether B. microti induces red blood cell cytoadherence. Vascular stasis and blockage has been demonstrated in the retina of a human patient infected with B. microti but another single case study failed to demonstrate evidence of B. microti-infected red blood cell cytoadherence to vascular endothelium in the brain of a comatose patient (Ortiz and Eagle, 1982; Clark et al., 2006b; Ortiz et al., 2020).
The genomic etiology of Babesia infected red blood cell cytoadherence has been described for B. bovis. A multigene family, ves1α, in B. bovis is responsible for the production of adherence variant erythrocyte surface antigen 1 or VESA1 (Allred et al., 2000). This family of variant proteins are found on the surface of B. bovis-infected erythrocytes. A similar multigene family (var genes) is found in P. falciparum that encodes for the production of P. falciparum erythrocyte membrane protein 1 or PfEMP1 (Jensen et al., 2020). These surface proteins mediate infected erythrocyte adherence to vascular endothelium, which make Babesia and Plasmodia less accessible to attack by host immune cells (Allred and Al-Khedery, 2006; Krause et al., 2007). In a recent study, several adherence proteins have been found on the surface of B. microti-infected red blood cells in laboratory mice (Verma et al., 2020). These findings support the possibility that B. microti-induced cytoadherence may contribute to disease complications.
Persistent Infection
Persistence of infection is critical for the survival of Babesia as they rely on transfer between rodent host and tick vector. Once infected, both the primary host (Peromyscus leucopus) and tick vector (I. scapularis) remain infected for life, increasing the chance of transfer of infection from one to the other. The same mechanism(s) that ensure persistence of infection in wildlife are likely to be operative in humans as they too experience persistent infection for months, even though they are dead end hosts (Spielman et al., 1981; Telford and Spielman, 1993; Krause et al., 1998; Moritz et al., 2016). At least three mechanisms are thought to contribute to persistence: intraerythrocytic location, cytoadherence, and regulation of parasite release from the erythrocyte.
The intraerythrocytic location of Babesia protect them against host immune factors. Cytoadherence of Babesia-infected erythrocytes to vascular endothelium avoids splenic destruction of Babesia. Variable parasite release from the erythrocyte also contributes to persistent Babesia infection. The work of Lobo and colleagues has shown that the intraerythrocytic life cycle of B. divergens is flexible and that egress from the erythrocyte can occur rather quickly after red blood cell invasion or later in infection. Once established within the red blood cell, early egress from erythrocytes would be favored when there is a strong need to expand the population, as occurs early in infection. Later egress following several cell divisions is more likely when infection is well established and the intravascular environment becomes hostile due to host immune activation and antimicrobial therapy (Lobo et al., 2019). It only takes a few infected erythrocytes to support persistence of infection in a host, which helps explain the recrudescence of infection that can occur in immunocompromised hosts despite low level parasitemia after prolonged (6 weeks or longer) antimicrobial therapy (Krause et al., 2008).
The clinical consequences of persistent infection include relapsing disease in immunocompromised individuals and transfer of Babesia to blood transfusion recipients following donation from an asymptomatic infected blood donor. A prospective follow-up study of 46 babesiosis patients demonstrated that B. microti parasitemia can persist for months with or without anti-B. microti therapy (Krause et al., 1998). Blood samples were obtained from patients during acute infection and every three to 6 months thereafter for amplification of B. microti DNA using PCR. About half (n = 22) of the patients were treated with clindamycin and quinine. Babesia DNA persisted asymptomatically in these patients for 2–13 months, depending in part on whether parasitemia duration was measured by the last recorded PCR positive sample or the first PCR negative sample. Asymptomatic infection persisted even longer in a group of patients who had mild B. microti infection and were not treated because of concern about side effects of clindamycin and quinine, the only effective therapy available at the time of the study. In these cases, parasitemia duration was 7–27 months. Only one of the 46 previously healthy patients had recrudescence of infection and that occurred 27 months after initial diagnosis (Krause et al., 1998). In contrast, recrudescence is more common in highly immunocompromised patients, especially those with defective antibody production (Krause et al., 2008). Prolonged Babesia disease has been described in immunocompromised hosts, with relapsing symptoms lasting up to 9 months and parasitemia continuing for more than 2 years (Krause et al., 2008; Raffalli and Wormser, 2016; Allred, 2019; Bloch et al., 2019). These patients are markedly immunocompromised with underlying diagnoses that include HIV/AIDS, malignancy, and asplenia. A retrospective case series of consecutively enrolled babesiosis patients who failed to respond to standard anti-Babesia antibiotic therapy also demonstrated that patients with these immunosuppressive conditions experienced persistent and relapsing babesiosis (Krause et al., 2008). Interestingly, 10 of the 14 patients in this series suffered from B cell lymphoma and had been treated with Rituximab, an anti-B cell monoclonal antibody. These data suggest that an impaired anti-Babesia antibody response, along with generalized immunosuppression, prevents clearance of B. microti infection. Long term antibiotic therapy of at least 6 weeks with at least 2 weeks of negative blood smears, rather than the standard 7–10 days, was required to resolve infection in these severely immunocompromised patients. Severe and persistent B. microti infection has been associated with advanced age in a mouse model and in humans (Vannier et al., 2004; Vannier and Krause, 2012).
Asymptomatic persistence of Babesia infection accounts for transmission of Babesia through blood donation. In the United States, B. microti is one of the most common pathogens transmitted by blood transfusion. Since 1979, there have been more than 250 cases of transfusion-transfusion transmitted babesiosis (Herwaldt et al., 2011; Moritz et al., 2016; Linden et al., 2018; Gray and Herwaldt, 2019). Furthermore, data from several studies, including the national babesiosis surveillance program that tracks clinical cases, indicate that both the clinical burden and foci of transmission of Babesia are expanding, which also means increased transfusion transmitted babesiosis risk to the U.S. blood supply (Menis et al., 2015; Linden et al., 2018; Gray and Herwaldt, 2019). Lack of knowledge of the mechanisms of persistence of parasitemia in asymptomatic carriers, and the intraerythrocytic characteristics of the parasite, present unique challenges in identifying Babesia infection in blood donors. While the minimum infectious dose to transmit Babesia in humans is not known, results from a mouse model suggest that as few as 10–100 infected RBC are sufficient to establish fulminant blood stage infection (Bakkour et al., 2018).
Limited epidemiological surveys in Babesia-endemic states using laboratory-based nucleic-acid-based tests (NAT) and antibody testing have provided important information about the percentage of asymptomatic infected donors, the relationship between seropositivity and parasitemia, and the seasonality of transmission in endemic areas (Leiby et al., 2005, 2014; Johnson et al., 2009). In more recent years, results from prospective studies in large cohorts of blood donors conducted under the Investigational New Drug protocols have shed light on the prevalence of B. microti infections in asymptomatic healthy adults in endemic areas and non-endemic states (Levin et al., 2016; Moritz et al., 2016; Tonnetti et al., 2020). Results showed one confirmed infected sample per 1331 donations in endemic states (67 positives/89,153 donations) (Moritz et al., 2014) and one confirmed positive per 2351 donations (211 positives/496,270 donations) (Tonnetti et al., 2020) in endemic states plus Florida using investigational NAT assays. Cumulative results from the surveillance programs (Herwaldt et al., 2011; Linden et al., 2018; Gray and Herwaldt, 2019) and donor testing studies (Johnson et al., 2009; Moritz et al., 2016) have revealed that while tick-borne infections are seasonal, transfusion transmitted cases occur year-round, although they too peak during the tick-transmission season. Transfusion transmitted babesiosis cases are also reported outside the bounds of recognized endemic states due to travel to endemic areas from non-endemic areas and import of blood products from endemic to non-endemic states (Cangelosi et al., 2008; Ngo and Civen, 2009).
Hemolytic Anemia and Hypercoagulability
After invasion of erythrocytes, B. microti multiply by binary fission, resulting in two to four daughter cells (merozoites). Rupture of erythrocytes that occurs with merozoite release is associated with fever, anemia, jaundice, hemoglobinemia, hemoglobinuria, tissue hypoxia, and renal insufficiency (Vannier and Krause, 2012; Vannier et al., 2015). Malaria merozoite egress from erythrocytes is synchronous while Babesia merozoite egress is non-synchronous. Synchronous release leads to paroxysms of fever interspersed with periods of apparent wellness, whereas non-synchronous release results in a more continuous pattern of fever. Because non-infectious hemolytic processes do not cause fever, additional factors associated with the lysis of the red cell are thought to result in febrile episodes. Hemolytic anemia with hypoxia has been implicated in exacerbation of congestive heart failure. Red cell membrane debris may lead to sequestration, vascular stasis, and functional impairment in the kidney and possibly other organs. Renal impairment is commonly noted, including renal failure in about 5% of babesiosis patients (Persing et al., 1995; Hatcher et al., 2001). Hemolytic anemia due to production of autoantibodies 2–4 weeks after the diagnosis of babesiosis has been described in a subset of asplenic patients who had no previous history of autoimmunity (Woolley et al., 2017). The genetic aspects of the severity of babesiosis-induced hemolytic anemia and hypercoagulability have not been investigated.
Conclusion
Genome sequencing of Babesia parasite isolates from diverse geographic locations has improved our understanding of genetic diversity of Babesia and provided genetic tools to monitor the areas of transmission and expansion where the disease had not previously existed. Genomic studies also have helped elucidate the pathogenesis of B. bovis and B. divergens infections that may have relevance for all pathogenic Babesia species. Such studies also have provided potential therapeutic and vaccine targets. Computational analyses and biological characterization of novel B. microti antigens has made possible the assignment of functions and elucidation of biological pathways that could provide new diagnostic tools and novel drug and vaccine targets. Availability of superior detection assays will allow improved diagnosis for acute babesiosis and help protect the blood supply against transfusion-transmitted babesiosis. Additional research is needed to improve our knowledge of parasite invasion, cell cycle and proliferation, and factors that mediate host immunity and pathogenesis. Finally, genomic studies should help in the development of novel treatment options. These include new drugs and/or biologics such as monoclonal antibodies that are urgently needed for treatment of those patients experiencing severe babesiosis in whom standard anti-Babesia antibiotics are not effective.
Author Contributions
SK and PK conceptualized the review, analyzed the data, and helped to write the manuscript. AP, SB, LA, and SM helped to write the manuscript and prepared the figures. All authors read and approved the final manuscript.
Conflict of Interest
The authors declare that the research was conducted in the absence of any commercial or financial relationships that could be construed as a potential conflict of interest.
Publisher’s Note
All claims expressed in this article are solely those of the authors and do not necessarily represent those of their affiliated organizations, or those of the publisher, the editors and the reviewers. Any product that may be evaluated in this article, or claim that may be made by its manufacturer, is not guaranteed or endorsed by the publisher.
Acknowledgments
The authors thank the FDA Intramural Research Program and the Gordon and Llura Gund Foundation for providing financial support. The views and opinions expressed here are those of authors and do not represent the official position of the FDA.
References
Adl, S. M., Bass, D., Lane, C. E., Lukes, J., Schoch, C. L., Smirnov, A., et al. (2019). Revisions to the Classification, Nomenclature, and Diversity of Eukaryotes. J. Eukaryot. Microbiol. 66, 4–119.
Afroz, S., Giddaluru, J., Abbas, M. M., and Khan, N. (2016). Transcriptome meta-analysis reveals a dysregulation in extra cellular matrix and cell junction associated gene signatures during Dengue virus infection. Sci. Rep. 6:33752.
Aguilar-Delfin, I., Homer, M. J., Wettstein, P. J., and Persing, D. H. (2001). Innate resistance to Babesia infection is influenced by genetic background and gender. Infect. Immun. 69, 7955–7958. doi: 10.1128/iai.69.12.7955-7958.2001
Allred, D. R. (2019). Variable and Variant Protein Multigene Families in Babesia bovis Persistence. Pathogens 8:76. doi: 10.3390/pathogens8020076
Allred, D. R., and Al-Khedery, B. (2004). Antigenic variation and cytoadhesion in Babesia bovis and Plasmodium falciparum: different logics achieve the same goal. Mol. Biochem. Parasitol. 134, 27–35. doi: 10.1016/j.molbiopara.2003.09.012
Allred, D. R., and Al-Khedery, B. (2006). Antigenic variation as an exploitable weakness of babesial parasites. Vet. Parasitol. 138, 50–60. doi: 10.1016/j.vetpar.2006.01.039
Allred, D. R., Carlton, J. M., Satcher, R. L., Long, J. A., Brown, W. C., Patterson, P. E., et al. (2000). The ves multigene family of B. bovis encodes components of rapid antigenic variation at the infected erythrocyte surface. Mol. Cell 5, 153–162. doi: 10.1016/s1097-2765(00)80411-6
Anantharaman, V., Iyer, L. M., Balaji, S., and Aravind, L. (2007). Adhesion molecules and other secreted host-interaction determinants in Apicomplexa: insights from comparative genomics. Int. Rev. Cytol. 262, 1–74. doi: 10.1016/s0074-7696(07)62001-4
Bakkour, S., Chafets, D. M., Wen, L., Muench, M. O., Telford, S. R. III., Erwin, J. L., et al. (2018). Minimal infectious dose and dynamics of Babesia microti parasitemia in a murine model. Transfusion 58, 2903–2910. doi: 10.1111/trf.14889
Baniecki, M. L., Moon, J., Sani, K., Lemieux, J. E., Schaffner, S. F., and Sabeti, P. C. (2019). Development of a SNP barcode to genotype Babesia microti infections. PLoS Negl. Trop. Dis. 13:e0007194. doi: 10.1371/journal.pntd.0007194
Baskin, C. R., Bielefeldt-Ohmann, H., Tumpey, T. M., Sabourin, P. J., Long, J. P., Garcia-Sastre, A., et al. (2009). Early and sustained innate immune response defines pathology and death in nonhuman primates infected by highly pathogenic influenza virus. Proc. Natl. Acad. Sci. U. S. A. 106, 3455–3460. doi: 10.1073/pnas.0813234106
Bloch, E. M., Kumar, S., and Krause, P. J. (2019). Persistence of Babesia microti Infection in Humans. Pathogens 8:102. doi: 10.3390/pathogens8030102
Brennan, M. B., Herwaldt, B. L., Kazmierczak, J. J., Weiss, J. W., Klein, C. L., Leith, C. P., et al. (2016). Transmission of Babesia microti Parasites by Solid Organ Transplantation. Emerg. Infect. Dis. 22, 1869–1876.
Bunnik, E. M., Cook, K. B., Varoquaux, N., Batugedara, G., Prudhomme, J., Cort, A., et al. (2018). Changes in genome organization of parasite-specific gene families during the Plasmodium transmission stages. Nat. Commun. 9:1910.
Bunnik, E. M., Venkat, A., Shao, J., Mcgovern, K. E., Batugedara, G., Worth, D., et al. (2019). Comparative 3D genome organization in apicomplexan parasites. Proc. Natl. Acad. Sci. U. S. A. 116, 3183–3192. doi: 10.1073/pnas.1810815116
Cangelosi, J. J., Sarvat, B., Sarria, J. C., Herwaldt, B. L., and Indrikovs, A. J. (2008). Transmission of Babesia microti by blood transfusion in Texas. Vox Sang. 95, 331–334.
Carpi, G., Walter, K. S., Mamoun, C. B., Krause, P. J., Kitchen, A., Lepore, T. J., et al. (2016). Babesia microti from humans and ticks hold a genomic signature of strong population structure in the United States. BMC Genomics 17:888. doi: 10.1186/s12864-016-3225-x
Cilloniz, C., Shinya, K., Peng, X., Korth, M. J., Proll, S. C., Aicher, L. D., et al. (2009). Lethal influenza virus infection in macaques is associated with early dysregulation of inflammatory related genes. PLoS Pathog. 5:e1000604. doi: 10.1371/journal.ppat.1000604
Clark, I. A., and Jacobson, L. S. (1998). Do babesiosis and malaria share a common disease process? Ann. Trop. Med. Parasitol. 92, 483–488. doi: 10.1080/00034989859456
Clark, I. A., Budd, A. C., Alleva, L. M., and Cowden, W. B. (2006a). Human malarial disease: a consequence of inflammatory cytokine release. Malar. J. 5:85.
Clark, I. A., Budd, A. C., Hsue, G., Haymore, B. R., Joyce, A. J., Thorner, R., et al. (2006b). Absence of erythrocyte sequestration in a case of babesiosis in a splenectomized human patient. Malar. J. 5:69.
Cornillot, E., Dassouli, A., Pachikara, N., Lawres, L., Renard, I., Francois, C., et al. (2016). A targeted immunomic approach identifies diagnostic antigens in the human pathogen Babesia microti. Transfusion 56, 2085–2099. doi: 10.1111/trf.13640
Cornillot, E., Hadj-Kaddour, K., Dassouli, A., Noel, B., Ranwez, V., Vacherie, B., et al. (2012). Sequencing of the smallest Apicomplexan genome from the human pathogen Babesia microti. Nucleic Acids Res. 40, 9102–9114. doi: 10.1093/nar/gks700
Dao, A. H., and Eberhard, M. L. (1996). Pathology of acute fatal babesiosis in hamsters experimentally infected with the WA-1 strain of Babesia. Lab. Invest. 74, 853–859.
Djokic, V., Akoolo, L., and Parveen, N. (2018). Babesia microti Infection Changes Host Spleen Architecture and Is Cleared by a Th1 Immune Response. Front. Microbiol. 9:85. doi: 10.3389/fmicb.2018.00085
Dumic, I., Madrid, C., Rueda Prada, L., Nordstrom, C. W., Taweesedt, P. T., and Ramanan, P. (2020). Splenic Complications of Babesia microti Infection in Humans: a Systematic Review. Can. J. Infect. Dis. Med. Microbiol. 2020:6934149.
Elton, C. M., Rodriguez, M., Ben Mamoun, C., Lobo, C. A., and Wright, G. J. (2019). A library of recombinant Babesia microti cell surface and secreted proteins for diagnostics discovery and reverse vaccinology. Int. J. Parasitol. 49, 115–125. doi: 10.1016/j.ijpara.2018.10.003
Everitt, J. I., Shadduck, J. A., Steinkamp, C., and Clabaugh, G. (1986). Experimental Babesia bovis infection in Holstein calves. Vet. Pathol. 23, 556–562. doi: 10.1177/030098588602300503
Falagas, M. E., and Klempner, M. S. (1996). Babesiosis in patients with AIDS: a chronic infection presenting as fever of unknown origin. Clin. Infect. Dis. 22, 809–812. doi: 10.1093/clinids/22.5.809
Fox, L. M., Wingerter, S., Ahmed, A., Arnold, A., Chou, J., Rhein, L., et al. (2006). Neonatal babesiosis: case report and review of the literature. Pediatr. Infect. Dis. J. 25, 169–173. doi: 10.1097/01.inf.0000195438.09628.b0
Froberg, M. K., Dannen, D., and Bakken, J. S. (2004). Babesiosis and HIV. Lancet 363:704. doi: 10.1016/s0140-6736(04)15645-6
Goethert, H. K., and Telford, S. R. III. (2003). What is Babesia microti? Parasitology 127, 301–309.
Goethert, H. K., Molloy, P., Berardi, V., Weeks, K., and Telford, S. R. III. (2018). Zoonotic Babesia microti in the northeastern U.S.: evidence for the expansion of a specific parasite lineage. PLoS One 13:e0193837. doi: 10.1371/journal.pone.0193837
Gray, E. B., and Herwaldt, B. L. (2019). Babesiosis Surveillance - United States, 2011-2015. MMWR Surveill. Summ. 68, 1–11. doi: 10.15585/mmwr.ss6806a1
Hancock, A. S., Stairiker, C. J., Boesteanu, A. C., Monzon-Casanova, E., Lukasiak, S., Mueller, Y. M., et al. (2018). Transcriptome Analysis of Infected and Bystander Type 2 Alveolar Epithelial Cells during Influenza A Virus Infection Reveals In Vivo Wnt Pathway Downregulation. J. Virol. 92, e01325–18.
Hatcher, J. C., Greenberg, P. D., Antique, J., and Jimenez-Lucho, V. E. (2001). Severe babesiosis in Long Island: review of 34 cases and their complications. Clin. Infect. Dis. 32, 1117–1125. doi: 10.1086/319742
Healy, G. R., and Ruebush, T. K. II. (1980). Morphology of Babesia microti in human blood smears. Am. J. Clin. Pathol. 73, 107–109. doi: 10.1093/ajcp/73.1.107
Hemmer, R. M., Ferrick, D. A., and Conrad, P. A. (2000). Up-regulation of tumor necrosis factor-alpha and interferon-gamma expression in the spleen and lungs of mice infected with the human Babesia isolate WA1. Parasitol. Res. 86, 121–128. doi: 10.1007/s004360050021
Hemmer, R. M., Wozniak, E. J., Lowenstine, L. J., Plopper, C. G., Wong, V., and Conrad, P. A. (1999). Endothelial cell changes are associated with pulmonary edema and respiratory distress in mice infected with the WA1 human Babesia parasite. J. Parasitol. 85, 479–489. doi: 10.2307/3285783
Herwaldt, B. L., Caccio, S., Gherlinzoni, F., Aspock, H., Slemenda, S. B., Piccaluga, P., et al. (2003). Molecular characterization of a non-Babesia divergens organism causing zoonotic babesiosis in Europe. Emerg. Infect. Dis. 9, 942–948. doi: 10.3201/eid0908.020748
Herwaldt, B. L., Linden, J. V., Bosserman, E., Young, C., Olkowska, D., and Wilson, M. (2011). Transfusion-associated babesiosis in the United States: a description of cases. Ann. Intern. Med. 155, 509–519. doi: 10.7326/0003-4819-155-8-201110180-00362
Herwaldt, B. L., Persing, D. H., Précigout, E. A., Goff, W. L., Mathiesen, D. A., Taylor, P. W., et al. (1996). A fatal case of babesiosis in Missouri: identification of another piroplasm that infects humans. Ann. Intern. Med. 124, 643–650. doi: 10.7326/0003-4819-124-7-199604010-00004
Hikosaka, K., Tsuji, N., Watanabe, Y., Kishine, H., Horii, T., Igarashi, I., et al. (2012). Novel type of linear mitochondrial genomes with dual flip-flop inversion system in apicomplexan parasites, Babesia microti and Babesia rodhaini. BMC Genomics 13:622. doi: 10.1186/1471-2164-13-622
Homer, M. J., Aguilar-Delfin, I., Telford, S. R. III., Krause, P. J., and Persing, D. H. (2000). Babesiosis. Clin. Microbiol. Rev. 13, 451–469.
Homer, M. J., Lodes, M. J., Reynolds, L. D., Zhang, Y., Douglass, J. F., Mcneill, P. D., et al. (2003). Identification and characterization of putative secreted antigens from Babesia microti. J. Clin. Microbiol. 41, 723–729. doi: 10.1128/jcm.41.2.723-729.2003
Huang, K. J., Su, I. J., Theron, M., Wu, Y. C., Lai, S. K., Liu, C. C., et al. (2005). An interferon-gamma-related cytokine storm in SARS patients. J. Med. Virol. 75, 185–194. doi: 10.1002/jmv.20255
Hunfeld, K. P., Hildebrandt, A., and Gray, J. S. (2008). Babesiosis: recent insights into an ancient disease. Int. J. Parasitol. 38, 1219–1237. doi: 10.1016/j.ijpara.2008.03.001
Jalovecka, M., Sojka, D., Ascencio, M., and Schnittger, L. (2019). Babesia Life Cycle - When Phylogeny Meets Biology. Trends Parasitol. 35, 356–368. doi: 10.1016/j.pt.2019.01.007
Jensen, A. R., Adams, Y., and Hviid, L. (2020). Cerebral Plasmodium falciparum malaria: the role of PfEMP1 in its pathogenesis and immunity, and PfEMP1-based vaccines to prevent it. Immunol. Rev. 293, 230–252. doi: 10.1111/imr.12807
Jia, N., Zheng, Y. C., Jiang, J. F., Jiang, R. R., Jiang, B. G., Wei, R., et al. (2018). Human Babesiosis Caused by a Babesia crassa-Like Pathogen: a Case Series. Clin. Infect Dis. 67, 1110–1119. doi: 10.1093/cid/ciy212
Johnson, S. T., Cable, R. G., Tonnetti, L., Spencer, B., Rios, J., and Leiby, D. A. (2009). Seroprevalence of Babesia microti in blood donors from Babesia-endemic areas of the northeastern United States: 2000 through 2007. Transfusion 49, 2574–2582. doi: 10.1111/j.1537-2995.2009.02430.x
Kash, J. C., Tumpey, T. M., Proll, S. C., Carter, V., Perwitasari, O., Thomas, M. J., et al. (2006). Genomic analysis of increased host immune and cell death responses induced by 1918 influenza virus. Nature 443, 578–581. doi: 10.1038/nature05181
Kim, J. Y., Cho, S. H., Joo, H. N., Tsuji, M., Cho, S. R., Park, I. J., et al. (2007). First case of human babesiosis in Korea: detection and characterization of a novel type of Babesia sp. (KO1) similar to ovine babesia. J. Clin. Microbiol. 45, 2084–2087. doi: 10.1128/jcm.01334-06
Kobasa, D., Jones, S. M., Shinya, K., Kash, J. C., Copps, J., Ebihara, H., et al. (2007). Aberrant innate immune response in lethal infection of macaques with the 1918 influenza virus. Nature 445, 319–323. doi: 10.1038/nature05495
Krause, P. J., Auwaerter, P. G., Bannuru, R. R., Branda, J. A., Falck-Ytter, Y. T., Lantos, P. M., et al. (2021). Clinical Practice Guidelines by the Infectious Diseases Society of America (IDSA): 2020 Guideline on Diagnosis and Management of Babesiosis. Clin. Infect. Dis. 72, 185–189. doi: 10.1093/cid/ciab050
Krause, P. J., Daily, J., Telford, S. R., Vannier, E., Lantos, P., and Spielman, A. (2007). Shared features in the pathobiology of babesiosis and malaria. Trends Parasitol. 23, 605–610. doi: 10.1016/j.pt.2007.09.005
Krause, P. J., Gewurz, B. E., Hill, D., Marty, F. M., Vannier, E., Foppa, I. M., et al. (2008). Persistent and relapsing babesiosis in immunocompromised patients. Clin. Infect. Dis. 46, 370–376. doi: 10.1086/525852
Krause, P. J., Lepore, T., Sikand, V. K., Gadbaw, J. Jr., Burke, G., and Telford, S. R. III., et al. (2000). Atovaquone and azithromycin for the treatment of babesiosis. N. Engl. J. Med. 343, 1454–1458. doi: 10.1056/nejm200011163432004
Krause, P. J., Spielman, A., Telford, S. R. III., Sikand, V. K., Mckay, K., Christianson, D., et al. (1998). Persistent parasitemia after acute babesiosis. N. Engl. J. Med. 339, 160–165. doi: 10.1056/nejm199807163390304
Lack, J. B., Reichard, M. V., and Van Den Bussche, R. A. (2012). Phylogeny and evolution of the Piroplasmida as inferred from 18S rRNA sequences. Int. J. Parasitol. 42, 353–363. doi: 10.1016/j.ijpara.2012.02.005
Leiby, D. A., Chung, A. P., Gill, J. E., Houghton, R. L., Persing, D. H., Badon, S., et al. (2005). Demonstrable parasitemia among Connecticut blood donors with antibodies to Babesia microti. Transfusion 45, 1804–1810. doi: 10.1111/j.1537-2995.2005.00609.x
Leiby, D. A., Johnson, S. T., Won, K. Y., Nace, E. K., Slemenda, S. B., Pieniazek, N. J., et al. (2014). A longitudinal study of Babesia microti infection in seropositive blood donors. Transfusion 54, 2217–2225. doi: 10.1111/trf.12622
Lemieux, J. E., Tran, A. D., Freimark, L., Schaffner, S. F., Goethert, H., Andersen, K. G., et al. (2016). A global map of genetic diversity in Babesia microti reveals strong population structure and identifies variants associated with clinical relapse. Nat. Microbiol. 1:16079.
Lenz, T., and Le Roch, K. G. (2019). Three-Dimensional Genome Organization and Virulence in Apicomplexan Parasites. Epigenet. Insights 12:2516865719879436.
Levin, A. E., Williamson, P. C., Bloch, E. M., Clifford, J., Cyrus, S., Shaz, B. H., et al. (2016). Serologic screening of United States blood donors for Babesia microti using an investigational enzyme immunoassay. Transfusion 56, 1866–1874. doi: 10.1111/trf.13618
Linden, J. V., Prusinski, M. A., Crowder, L. A., Tonnetti, L., Stramer, S. L., Kessler, D. A., et al. (2018). Transfusion-transmitted and community-acquired babesiosis in New York, 2004 to 2015. Transfusion 58, 660–668. doi: 10.1111/trf.14476
Liu, J., Guan, G., Li, Y., Liu, A., Luo, J., and Yin, H. (2017). A Molecular Survey of Babesia Species and Detection of a New Babesia Species by DNA Related to B. venatorum from White Yaks in Tianzhu, China. Front. Microbiol. 8:419. doi: 10.3389/fmicb.2017.00419
Lobo, C. A., Cursino-Santos, J. R., Singh, M., and Rodriguez, M. (2019). Babesia divergens: a Drive to Survive. Pathogens 8:95. doi: 10.3390/pathogens8030095
Lodes, M. J., Houghton, R. L., Bruinsma, E. S., Mohamath, R., Reynolds, L. D., Benson, D. R., et al. (2000). Serological expression cloning of novel immunoreactive antigens of Babesia microti. Infect. Immun. 68, 2783–2790. doi: 10.1128/iai.68.5.2783-2790.2000
Magni, R., Luchini, A., Liotta, L., and Molesteina, R. E. (2019). Analysis of the Babesia microti proteome in infected red blood cells by a combination of naotechnology and mass spestorscopy. Int. J. Parasitol. 49, 139–144. doi: 10.1016/j.ijpara.2018.08.004
Magni, R., Luchini, A., Liotta, L., and Molestina, R. E. (2020). Proteomic analysis reveals pathogen-derived biomarkers of acute babesiosis in erythrocytes, plasma, and urine of infected hamsters. Parasitol. Res. 119, 2227–2235. doi: 10.1007/s00436-020-06712-5
Mahto, H., Tripathy, R., Meher, B. R., Prusty, B. K., Sharma, M., Deogharia, D., et al. (2019). TNF-alpha promoter polymorphisms (G-238A and G-308A) are associated with susceptibility to Systemic Lupus Erythematosus (SLE) and P. falciparum malaria: a study in malaria endemic area. Sci. Rep. 9:11752.
Matsui, T., Inoue, R., Kajimoto, K., Tamekane, A., Okamura, A., Katayama, Y., et al. (2000). [First documentation of transfusion-associated babesiosis in Japan]. Rinsho Ketsueki 41, 628–634.
Menis, M., Forshee, R. A., Kumar, S., Mckean, S., Warnock, R., Izurieta, H. S., et al. (2015). Babesiosis Occurrence among the Elderly in the United States, as Recorded in Large Medicare Databases during 2006-2013. PLoS One 10:e0140332. doi: 10.1371/journal.pone.0140332
Moritz, E. D., Winton, C. S., Johnson, S. T., Krysztof, D. E., Townsend, R. L., Foster, G. A., et al. (2014). Investigational screening for Babesia microti in a large repository of blood donor samples from nonendemic and endemic areas of the United States. Transfusion 54, 2226–2236. doi: 10.1111/trf.12693
Moritz, E. D., Winton, C. S., Tonnetti, L., Townsend, R. L., Berardi, V. P., Hewins, M. E., et al. (2016). Screening for Babesia microti in the U.S. Blood Supply. N. Engl. J. Med. 375, 2236–2245.
Munoz-Gomez, S. A., Durnin, K., Eme, L., Paight, C., Lane, C. E., Saffo, M. B., et al. (2019). Nephromyces Represents a Diverse and Novel Lineage of the Apicomplexa That Has Retained Apicoplasts. Genome Biol. Evol. 11, 2727–2740.
Ngo, V., and Civen, R. (2009). Babesiosis acquired through blood transfusion, California, USA. Emerg. Infect. Dis. 15, 785–787. doi: 10.3201/eid1505.081562
O’Connor, R. M., Long, J. A., and Allred, D. R. (1999). Cytoadherence of Babesia bovis-infected erythrocytes to bovine brain capillary endothelial cells provides an in vitro model for sequestration. Infect. Immun. 67, 3921–3928. doi: 10.1128/iai.67.8.3921-3928.1999
Ortiz, J. F., Millhouse, P. W., Morillo Cox, A., Campoverde, L., Kaur, A., Wirth, M., et al. (2020). Babesiosis: appreciating the Pathophysiology and Diverse Sequela of the Infection. Cureus 12:e11085.
Ortiz, J. M., and Eagle, R. C. Jr. (1982). Ocular findings in human babesiosis (Nantucket fever). Am. J. Ophthalmol. 93, 307–311. doi: 10.1016/0002-9394(82)90530-x
Patarroyo, J. H., Vargas, M. I., and Bicudo, P. L. (1982). Description of lesions in cattle in a natural outbreak of Babesia bovis infection in Brazil. Vet. Parasitol. 11, 301–308. doi: 10.1016/0304-4017(82)90098-x
Persing, D. H., Herwaldt, B. L., Glaser, C., Lane, R. S., Thomford, J. W., Mathiesen, D., et al. (1995). Infection with a babesia-like organism in northern California. N. Engl. J. Med. 332, 298–303. doi: 10.1056/nejm199502023320504
Quick, R. E., Herwaldt, B. L., Thomford, J. W., Garnett, M. E., Eberhard, M. L., Wilson, M., et al. (1993). Babesiosis in Washington State: a new species of Babesia? Ann. Intern. Med. 119, 284–290. doi: 10.7326/0003-4819-119-4-199308150-00006
Raffalli, J., and Wormser, G. P. (2016). Persistence of babesiosis for >2 years in a patient on rituximab for rheumatoid arthritis. Diagn. Microbiol. Infect. Dis. 85, 231–232. doi: 10.1016/j.diagmicrobio.2016.02.016
Reid, A. J. (2015). Large, rapidly evolving gene families are at the forefront of host-parasite interactions in Apicomplexa. Parasitology 142, S57–S70.
Rubenfeld, G. D., Caldwell, E., Peabody, E., Weaver, J., Martin, D. P., Neff, M., et al. (2005). Incidence and outcomes of acute lung injury. N. Engl. J. Med. 353, 1685–1693.
Rudzinska, M. A. (1976). Ultrastructure of intraerythrocytic Babesia microti with emphasis on the feeding mechanism. J. Protozool. 23, 224–233. doi: 10.1111/j.1550-7408.1976.tb03759.x
Rudzinska, M. A., Trager, W., Lewengrub, S. J., and Gubert, E. (1976). An electron microscopic study of Babesia microti invading erythrocytes. Cell Tissue Res. 169, 323–334.
Ryan, E., Hill, D., Solomon, T., Aronson, N., and Endy, T. (Eds). (2019). Hunter’s Tropical Medicine and Emerging Infectious Diseases, 10th edition. London: Elsevier.
Sawalha, A. H., Zhao, M., Coit, P., and Lu, Q. (2020). Epigenetic dysregulation of ACE2 and interferon-regulated genes might suggest increased COVID-19 susceptibility and severity in lupus patients. medRxiv [Preprint]. doi: 10.1101/2020.03.30.20047852
Schnittger, L., Rodriguez, A. E., Florin-Christensen, M., and Morrison, D. A. (2012). Babesia: a world emerging. Infect. Genet. Evol. 12, 1788–1809. doi: 10.1016/j.meegid.2012.07.004
Scholtens, R. G., Braff, E. H., Healey, G. A., and Gleason, N. (1968). A case of babesiosis in man in the United States. Am. J. Trop. Med. Hyg. 17, 810–813. doi: 10.4269/ajtmh.1968.17.810
Shih, C. M., Liu, L. P., Chung, W. C., Ong, S. J., and Wang, C. C. (1997). Human babesiosis in Taiwan: asymptomatic infection with a Babesia microti-like organism in a Taiwanese woman. J. Clin. Microbiol. 35, 450–454. doi: 10.1128/jcm.35.2.450-454.1997
Silva, J. C., Cornillot, E., Mccracken, C., Usmani-Brown, S., Dwivedi, A., Ifeonu, O. O., et al. (2016). Genome-wide diversity and gene expression profiling of Babesia microti isolates identify polymorphic genes that mediate host-pathogen interactions. Sci. Rep. 6:35284.
Simon, M. S., Westblade, L. F., Dziedziech, A., Visone, J. E., Furman, R. R., Jenkins, S. G., et al. (2017). Clinical and Molecular Evidence of Atovaquone and Azithromycin Resistance in Relapsed Babesia microti Infection Associated With Rituximab and Chronic Lymphocytic Leukemia. Clin. Infect. Dis. 65, 1222–1225. doi: 10.1093/cid/cix477
Skrabalo, Z., and Deanovic, Z. (1957). Piroplasmosis in man; report of a case. Doc. Med. Geogr. Trop. 9, 11–16.
Spielman, A., Etkind, P., Piesman, J., Ruebush, T. K. II., Juranek, D. D., and Jacobs, M. S. (1981). Reservoir hosts of human babesiosis on Nantucket Island. Am. J. Trop. Med. Hyg. 30, 560–565. doi: 10.4269/ajtmh.1981.30.560
Telford, S. R. III., and Spielman, A. (1993). Reservoir competence of white-footed mice for Babesia microti. J. Med. Entomol. 30, 223–227. doi: 10.1093/jmedent/30.1.223
Tisoncik, J. R., Korth, M. J., Simmons, C. P., Farrar, J., Martin, T. R., and Katze, M. G. (2012). Into the eye of the cytokine storm. Microbiol. Mol. Biol. Rev. 76, 16–32.
Tonnetti, L., Young, C., Kessler, D. A., Williamson, P. C., Reik, R., Proctor, M. C., et al. (2020). Transcription-mediated amplification blood donation screening for Babesia. Transfusion 60, 317–325. doi: 10.1111/trf.15630
Usmani-Brown, S., Halperin, J. J., and Krause, P. J. (2013). Neurological manifestations of human babesiosis. Handb. Clin. Neurol. 114, 199–203. doi: 10.1016/b978-0-444-53490-3.00014-5
Vannier, E. G., Diuk-Wasser, M. A., Ben Mamoun, C., and Krause, P. J. (2015). Babesiosis. Infect. Dis. Clin. North Am. 29, 357–370.
Vannier, E., Borggraefe, I., Telford, S. R. III., Menon, S., Brauns, T., Spielman, A., et al. (2004). Age-associated decline in resistance to Babesia microti is genetically determined. J. Infect. Dis. 189, 1721–1728. doi: 10.1086/382965
Vannier, E., Gewurz, B. E., and Krause, P. J. (2008). Human babesiosis. Infect. Dis. Clin. North Am. 22, 469–488.
Verma, N., Puri, A., Essuman, E., Skelton, R., Anantharaman, V., Zheng, H., et al. (2020). Antigen Discovery, Bioinformatics and Biological Characterization of Novel Immunodominant Babesia microti Antigens. Sci. Rep. 10:9598.
Woolley, A. E., Montgomery, M. W., Savage, W. J., Achebe, M. O., Dunford, K., Villeda, S., et al. (2017). Post-Babesiosis Warm Autoimmune Hemolytic Anemia. N. Engl. J. Med. 376, 939–946.
World Health Organization (WHO). (2000). Severe falciparum malaria. World Health Organization, Communicable Diseases Cluster. Trans. R. Soc. Trop. Med. Hyg. 94, S1–S90.
Wormser, G. P., Prasad, A., Neuhaus, E., Joshi, S., Nowakowski, J., Nelson, J., et al. (2010). Emergence of resistance to azithromycin-atovaquone in immunocompromised patients with Babesia microti infection. Clin. Infect. Dis. 50, 381–386. doi: 10.1086/649859
Xu, B., Liu, X. F., Cai, Y. C., Huang, J. L., Zhang, R. X., Chen, J. H., et al. (2018). Screening for biomarkers reflecting the progression of Babesia microti infection. Parasit. Vectors 11:379.
Yokota, S. (2003). [Influenza-associated encephalopathy–pathophysiology and disease mechanisms]. Nihon Rinsho 61, 1953–1958.
Zhou, X., Huang, J. L., Shen, H. M., Xu, B., Chen, J. H., and Zhou, X. N. (2018). Immunomics analysis of Babesia microti protein markers by high-throughput screening assay. Ticks Tick Borne Dis. 9, 1468–1474. doi: 10.1016/j.ttbdis.2018.07.004
Zhou, X., Xia, S., Huang, J. L., Tambo, E., Zhuge, H. X., and Zhou, X. N. (2014). Human babesiosis, an emerging tick-borne disease in the People’s Republic of China. Parasit. Vectors 7:509. doi: 10.1186/preaccept-1503099832120211
Keywords: Babesia microti, biomarkers, genomics, pathogen, population genetics, tick-borne diseases
Citation: Puri A, Bajpai S, Meredith S, Aravind L, Krause PJ and Kumar S (2021) Babesia microti: Pathogen Genomics, Genetic Variability, Immunodominant Antigens, and Pathogenesis. Front. Microbiol. 12:697669. doi: 10.3389/fmicb.2021.697669
Received: 20 April 2021; Accepted: 30 July 2021;
Published: 03 September 2021.
Edited by:
Marinda Catharina Oosthuizen, University of Pretoria, South AfricaReviewed by:
Leonhard Schnittger, Consejo Nacional de Investigaciones Científicas y Técnicas (CONICET), ArgentinaBernard Joseph Hudson, New South Wales Health Pathology, Australia
Copyright © 2021 Puri, Bajpai, Meredith, Aravind, Krause and Kumar. This is an open-access article distributed under the terms of the Creative Commons Attribution License (CC BY). The use, distribution or reproduction in other forums is permitted, provided the original author(s) and the copyright owner(s) are credited and that the original publication in this journal is cited, in accordance with accepted academic practice. No use, distribution or reproduction is permitted which does not comply with these terms.
*Correspondence: Peter J. Krause, cGV0ZXIua3JhdXNlQHlhbGUuZWR1