- 1CNC—Center for Neuroscience and Cell Biology of Coimbra, Coimbra, Portugal
- 2FMUC—Faculty of Medicine, University of Coimbra, Coimbra, Portugal
- 3Mountain Research Center (CIMO), Polytechnic Institute of Bragança, Bragança, Portugal
- 4Laboratory of Separation and Reaction Engineering - Laboratory of Catalysis and Materials (LSRE-LCM), Polytechnic Institute of Bragança, Bragança, Portugal
- 5Centre for Mechanical Engineering, Materials and Processes, Department of Mechanical Engineering, University of Coimbra, Coimbra, Portugal
- 6Department of Molecular Microbiology and Immunology, Johns Hopkins Bloomberg School of Public Health, Baltimore, MD, United States
The genus Alternaria includes several of fungi that are darkly pigmented by DHN-melanin. These are pathogenic to plants but are also associated with human respiratory allergic diseases and with serious infections in immunocompromised individuals. The present work focuses on the alterations of the composition and structure of the hyphal cell wall of Alternaria alternata occuring under the catabolism of L-tyrosine and L-phenylalanine when cultured in minimal salt medium (MM). Under these growing conditions, we observed the released of a brown pigment into the culture medium. FTIR analysis demonstrates that the produced pigment is chemically identical to the pigment released when the fungus is grown in MM with homogentisate acid (HGA), the intermediate of pyomelanin, confirming that this pigment is pyomelanin. In contrast to other fungi that also synthesize pyomelanin under tyrosine metabolism, A. alternata inhibits DHN-melanin cell wall accumulation when pyomelanin is produced, and this is associated with reduced chitin cell wall content. When A. alternata is grown in MM containing L-phenylalanine, a L-tyrosine percursor, pyomelanin is synthesized but only at trace concentrations and A. alternata mycelia display an albino-like phenotype since DHN-melanin accumulation is inhibited. CmrA, the transcription regulator for the genes coding for the DHN-melanin pathway, is involved in the down-regulation of DHN-melanin synthesis when pyomelanin is being synthetized, since the CMRA gene and genes of the enzymes involved in DHN-melanin synthesis pathway showed a decreased expression. Other amino acids do not trigger pyomelanin synthesis and DHN-melanin accumulation in the cell wall is not affected. Transmission and scanning electron microscopy show that the cell wall structure and surface decorations are altered in L-tyrosine- and L-phenylalanine-grown fungi, depending on the pigment produced. In summary, growth in presence of L-tyrosine and L-phenylalanine leads to pigmentation and cell wall changes, which could be relevant to infection conditions where these amino acids are expected to be available.
Introduction
Alternaria alternata is the most common species of the genus Alternaria and it is the major environmental allergen associated with asthma and allergic rhinitis (Knutsen et al., 2012; Denning et al., 2014; Fukutomi and Taniguchi, 2015), also described as an opportunistic agent of infection in immunocompromised patients. Alternaria spp. are dematiaceous fungi characterized by the accumulation of melanin in the cell wall of conidia and hyphae. Melanins are a group of related pigments that share common physical and chemical characteristics (Eisenman and Casadevall, 2012). They are formed by the oxidative polymerization of phenolic or indolic compounds. In fungi, melanins may be synthesized from endogenous substrates via a 1,8-dihydroxynaphthalene (DHN) intermediate or alternatively, from L-3,4-dihydroxyphenylalanine (L-DOPA) (Eisenman and Casadevall, 2012). Other melanins called alkaptomelanin are derived from L-tyrosine (Yabuuchi and Ohyama, 1972). This pigment is referred as alkaptomelanin when is produced by humans with alkaptonuria, a rare disease due to mutations in the homogentisate dioxygenase gene but is usually called pyomelanin when synthesized by microbes. Pyomelanin is a water-soluble brown pigment first identified in Pseudomonas aeruginosa (Yabuuchi and Ohyama, 1972).
A. alternata accumulates DHN-melanin in hyphal and conidial cell walls (Carzaniga et al., 2002). This pigment is synthesized by the 1,8-dihydroxynaphthalene (DHN) pathway with 1,8-DHN as intermediate (Kimura and Tsuge, 1993; Tseng et al., 2011; Eisenman and Casadevall, 2012). Three classes of melanin mutants have been reported in A. alternata: two albino mutants (Δcmra, Δalm), a lightbrown (Δbrm1) and a brown mutant (Δbrm2) (Kimura and Tsuge, 1993; Fetzner et al., 2014). The color phenotype of these mutants depends on the gene deleted, since the pathway intermediates lead to the shunt products like flaviolin and/or 2-hydroxyjuglone, that are colored (Lee et al., 2003). The melanin-deficient strains are more sensitive to UV light (Kawamura et al., 1999). The transcription factor CmrA, the regulator for this melanin route was first discovered in Colletotrichum lagenarium and Magnaporthe grisea in a screen for insertional mutants with lowered pathogenicity (Tsuji et al., 2000) and was then characterized in Alternaria brassicicola and A. alternata, where it controls the expression of at least three structural genes for melanin biosynthesis (Cho et al., 2012; Fetzner et al., 2014).
Pyomelanin biosynthesis occurs by oxidative polymerization of homogentisate (HGA), an intermediate of the catabolism of L-tyrosine or L-phenylalanine (Lehninger, 1975; Figure 1A). The degradation of L-tyrosine to acetoacetate and fumarate requires the activity of the enzymes 4-hydroxyphenylpyruvic acid dioxygenase (4-HPPD) and homogentisic acid oxidase (HGA-oxidase). When HGA production exceeds HGA-oxidase activity, HGA is excreted from the cell (Yabuuchi and Ohyama, 1972; Ruzafa et al., 1994; Kotob et al., 1995). Auto-oxidation followed by self-polymerization of HGA leads to pyomelanin. Additionally, deletion of the gene that encodes for 4-HPPD or inhibition of this enzyme by specific inhibitor such as sulcotrione, impair the synthesis of pyomelanin (Ruzafa et al., 1995; Turick et al., 2010). This water-soluble pigment binds to the surface of hyphae (Heinekamp et al., 2013), and protects the fungus against reactive oxygen species (ROS) (Schmaler-Ripcke et al., 2009) and cell wall stress (Valiante et al., 2009). This pigment was associated with virulence in both Pseudomonas aeruginosa and Cryptococcus neoformans, since pyomelanin reduced the phagocytosis rate (Frases et al., 2007; Rodríguez-Rojas et al., 2009). However, in Aspergillus fumigatus, the mutants deficient for pyomelanin biosynthesis do not differ in virulence when tested in a murine infection model for invasive pulmonary aspergillosis Keller et al., 2011; Heinekamp et al., 2013), even though the transcription of the L-tyrosine degradation gene cluster is increased under cell wall stress (Jain et al., 2011) and when exposed to immature dendritic cells or human neutrophils (Morton et al., 2011).
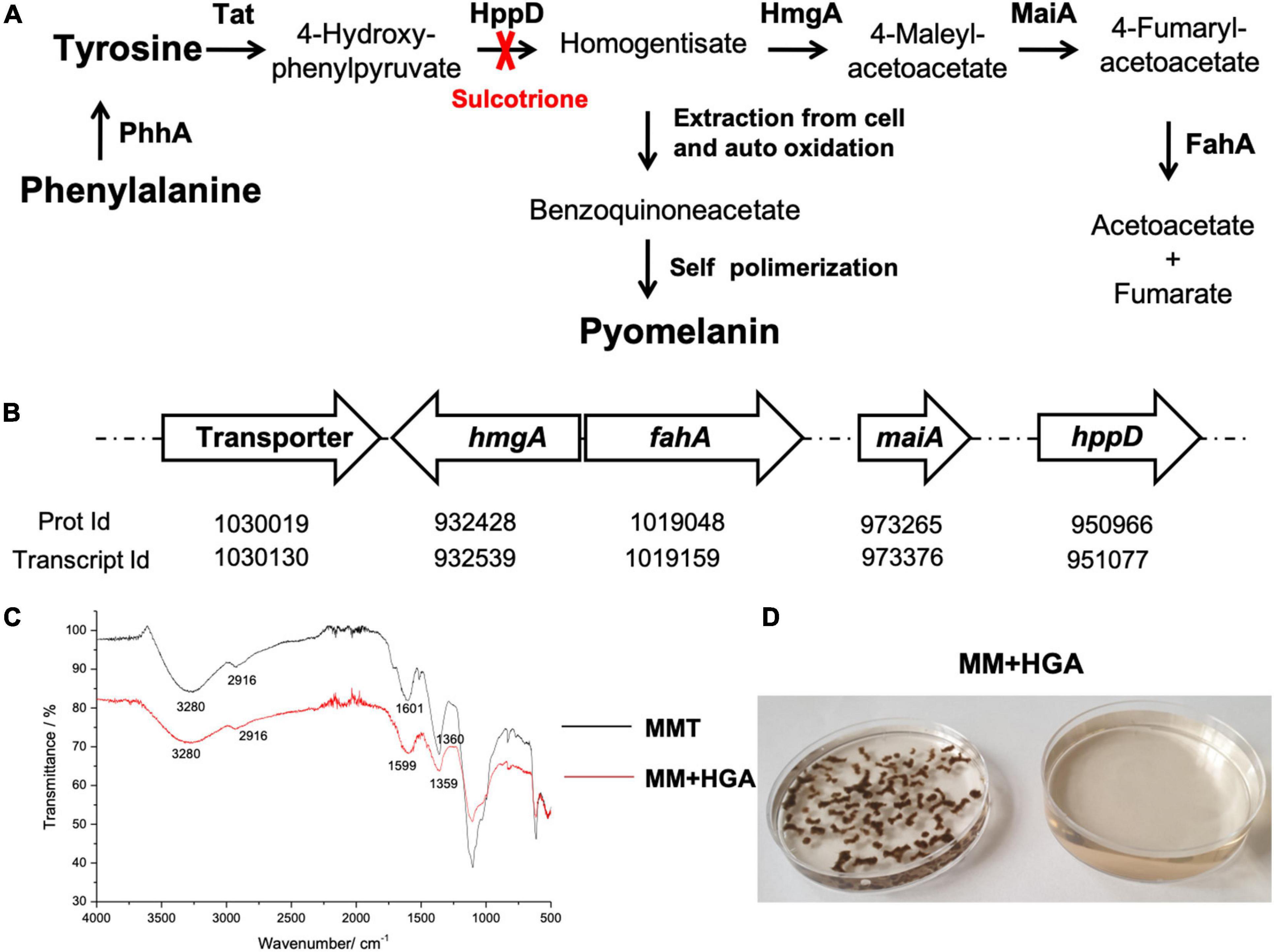
Figure 1. Pyomelanin synthesis in A. alternata by L-Tyrosine degradation to HGA. (A) L-Tyrosine degradation pathway and pyomelanin synthetic pathway. The enzymes indicated in bold as follows: PhhA, phenylalanine hydroxylase; Tat, L-tyrosine aminotransferase; HppD, 4-hydroxyphenylpyruvate dioxygenase; HmgA, homogentisate dioxygenase; MaiA, maleylacetoacetate isomerase; FahA, fumarylacetoacetate hydrolase. (B) Arrangement of the genes putatively involved in these pathways in A. alternata. The pyomelanin pigment derives from L-tyrosine through formation of 4-hydroxyphenylpyruvate and HGA which conversion is inhibited by sulcotrione. HGA is catabolized into acetoacetate and fumarate and depending on the HmgA activity, HGA may accumulates and can be secreted from the cell (Yabuuchi and Ohyama, 1972). Then, after auto-oxidation into benzoquinoneacetate and self-polymerization, forms a brown pigment, pyomelanin (Rodríguez et al., 2000). The genes encoding the enzymes involved in these pathways were found in A. alternata SRC1lrK2f v1.0 genome, at JGI: http://genome.jgi.doe.gov (The Genome Portal of the Department of Energy Joint Genome Institute). The transporter (Prot Id 985975) may be involved in the transport of HGA out of the cell. (C) FTIR spectra of the pigments obtained from culture medium of A. alternata grown in MMT (black) and in MM + HGA (red). (D) Culture medium and mycelia of A. alternata grown in MM + 5.5 mM HGA.
Alternaria spp. secrete a wide variety of proteases during spore germination and growth (Boitano et al., 2011; Snelgrove et al., 2014) that can potentially degrade host proteins. Consequently, the amino acids L-tyrosine and L-phenylalanine are likely to be available to fungal cells during human and animal infection. Furthermore, normal human plasma L-tyrosine levels range from 21 to 107 μM (Gressner, 2007; Schmaler-Ripcke et al., 2009). The higher concentrations are located in neural tissues as L-tyrosine readily crosses the blood brain barrier can be used as a precursor of the neurotransmitters norepinephrine and dopamine (Fernstrom and Fernstrom, 2007). With regards to L-phenylalanine levels, reference blood phenylalanine concentrations are between 21 and 137 μmol/L in healthy children up to the age of 18 years and in adults, 35–85 μmol/L (Cleary et al., 2013). However, individuals with phenylketonuria, a disorder caused by an impaired conversion of L-phenylalanine to L-tyrosine (due to a deficiency in activity of a renal enzyme L-phenylalanine hydroxylase), may have in an increased in plasma L-phenylalanine concentrations, with values above 1.0 mM (Mitchell, 2013).
In this context, it is likely that L-tyrosine and L-phenylalanine are available during infection or during human lung colonization, leading to the transcription of the L-tyrosine degradation genes from A. alternata and catabolism of these amino acids. In the present work, we describe the production of pyomelanin in A. alternata during L-tyrosine catabolism and report the consequent inhibition of DHN-melanin synthesis together with changes in chitin content and cell wall structure.
Materials and Methods
Organisms and Media
A. alternata was kindly provided by R. M. B. Ferreira (Instituto Superior de Agronomia, Universidade de Lisboa, Lisbon, Portugal) and was stored at –80°C. Cultures were grown on PDA (Potato Dextrose Agar; Difco), minimal medium [MM; 1 L contained 20 mL of salts solution (NaNO3 300 g/L, KCl 26 g/L, MgSO4.7H2O 24.65 g/L), 9 g of glucose, 12 mL of phosphate buffer 1 M pH 6.8 (KH2PO4 68 g/L, K2HPO4 87.1 g/L), 1 mL of Clive Roberts trace elements solution (FeSO4.7H2O 1 g/L, ZnSO4.7H2O 8.8 g/L, CuSO4.5H2O 400 mg/L, MnSO4.H2O 150 mg/L, Na2B4O7.10H2O 100 mg/L, (NH4)6Mo7O24.4H2O 50 mg/L and 15 g/L of agar for solid media1)]. MM liquid medium was sterilized by filtration and solid medium by autoclaving at 121°C for 20 min. For pyomelanin production, the MM medium was added with 5.5 mM of L-tyrosine (Sigma, T-8566) (referred as MMT) or 5.5 mM of L-phenylalanine (Sigma, P-5482) (referred as MMP) and heated under agitation until solubilized and then sterilized by filtration. Solutions with 5.5 mM L-valine (Sigma, V-0513) and 5.5 mM L-glutamine (Sigma, G-3126) were also used as control in MM.
Preparation of Inocula and Growth Conditions
The procedures used to prepare inocula were as previously described (Anjos et al., 2012). Briefly, spores of A. alternata were collected from 2-weeks-old plate cultures grown at 28°C with alternating 16-h light 8-h dark cycle under a BLB blacklight blue lamp (15 W). The mycelium was submerged in liquid MM and scraped with an inoculation loop, and this suspension was used as the inoculum. Liquid A. alternata cultures were cultivated on MM at 28°C with constant orbital shaking at 130 rpm with alternating 16-h light 8-h dark cycle (Fernandes et al., 2014).
A. alternata Growth Curve, Quantification of the Pigment Released and Accumulated From Cultures
Several Erlenmeyer flasks with 50 mL of MM, MM supplemented with 5.5 mM of L-tyrosine (MMT) or MM supplemented with 5.5 mM of L-phenylalanine (MMP) were inoculated with 1 × 106 A. alternata conidia and incubated on a rotary shaker at 120 rpm at 28°C under 16 h-alternating light enriched with UV and 8 h dark. Every 24 h, for 10 days, Erlenmeyer flasks content were analyzed for pigment released and fungal growth determined by dry weight. The mycelial mats were harvested by filtration in a steel filter. The pigment was released and analyzed by absorbance measurements at 405 nm in a Spectra Max Plus384 spectrophotometer (Molecular Devices, LLC) (Schmaler-Ripcke et al., 2009). To estimate fungal biomass growth, the material was freeze-dried and weighed. The pigment bound to the fungal cell wall was quantified and expressed per mg of dry weight. For quantification, the samples were suspended in distilled H2O, sonicated (Sonics and Materials, Inc.) and extracted with NaOH 1 M as described before (Fernandes et al., 2015) and pigments were quantified spectrophotometrically at 405 nm.
Effect of Sulcotrione, Pyroquilon, and Glyphosate on Pigment Release From A. alternata
To evaluate the effect of sulcotrione, pyroquilon and glyphosate on pigment synthesis by A. alternata, we inoculated MM, MMT and MMP liquid medium with, and without, 100 μg/mL of sulcotrione, pyroquilon and glyphosate. The pigments released in the supernatant were quantified at 405 nm after 3 days of incubation and the mycelium was harvested.
Additionally, to understand whether A. alternata DHN-melanin pathway is activated under L-tyrosine metabolism, we analyzed the supernatants of A. alternata grown in MM and MMT, with and without 100 μg/mL pyroquilon, to follow the production of flaviolin, a shunt product released into the medium when DHN-melanin pathway is inhibited by pyroquilon. The supernatants of A. alternata grown in MM and MMT, and grown under these conditions with pyroquilon were filtered through a 0.2 μm sterile syringe cellulose acetate filter (VWR, United States) and concentrated to 100 mg/mL for chromatographic analysis performed in a Dionex Ultimate 3000 UPLC (Thermo Scientific, San Jose, CA, United States) system equipped with a diode array detector coupled to a electrospray ionization mass detector (LC-DAD-ESI/MSn), a quaternary pump, an auto-sampler (kept at 5°C), a degasser and an automated thermostatic column compartment.
Chromatographic separation was achieved with a Waters Spherisorb S3 ODS-2 C18 (3 μm, 4.6 mm × 150 mm, Waters, Milford, MA, United States) column thermostatic at 35°C.
The solvents used were: (A) 0.1% formic acid in water, (B) acetonitrile. The elution gradient established was isocratic 15% B (5 min), 15 B–20% B (5 min), 20–25% B (10 min), 25–35% B (10 min), 35–50% B (10 min), and re-equilibration of the column, using a flow rate of 0.5 mL/min. Double online detection was carried out in the DAD using 280, 370, 480, and 520 nm as preferred wavelengths and in a mass spectrometer (MS) connected to HPLC system via the DAD cell outlet.
MS detection was performed in negative mode, using a Linear Ion Trap LTQ XL mass spectrometer (Thermo Finnigan, San Jose, CA, United States) equipped with an ESI source. Nitrogen served as the sheath gas (50 psi); the system was operated with a spray voltage of 5 kV, a source temperature of 325°C, a capillary voltage of –20 V. The tube lens offset was kept at a voltage of –66 V. The full scan covered the mass range from m/z 100 to 1,500. The collision energy used was 35 (arbitrary units). Data acquisition was carried out with Xcalibur® data system (Thermo Finnigan, San Jose, CA, United States).
Characterization of the Pigments Released by Ultraviolet-Visible and Fourier Transform Infrared Spectroscopy (FTIR)
A volume of 200 mL of MM with 5.5 mM of L-tyrosine or 5.5 mM HGA (D1050, TCI) was inoculated with 5 × 106 conidia of A. alternata and incubated for 4 days on a rotary shaker at 120 rpm at 28°C under 16 h-alternating light enriched with UV and 8 h dark. The mycelial mats were harvested by filtration in a steel filter and the supernatant medium collected. The supernatant was filtered through a 0.2 μm cellulose acetate filter membrane, dialyzed overnight at room temperature against distilled H2O and lyophilized.
The infrared spectra of the pigments were recorded on a ThermoNicolet IR300 Fourier transform infrared spectrometer, equipped with a deuterated triglycine sulfate (DTGS) detector and a KBr beam splitter. Thermo Scientific Nicolet Smart Orbit diamond ATR accessory was also used with a resolution of 1 cm–1.
Quantification of Expression of the Transcription Factor CMRA and of the Genes PKSA, BRM1, and BRM2 of the DHN-Melanin Synthesis
Total RNA isolation was performed with the Bioline ISOLATE II RNA Mini Kit II BIO-52072 (Meridian Bioscience) following manufacturer’s instructions. Reverse transcription (RT) of 3 μg of total RNA was performed with the 1st Strand cDNA synthesis kit for RT-PCR (Roche) according to the manufacturer’s instructions. The relative quantification of the expression of CMRA and of the polyketide synthase gene (PKSA), scytalone dehydratase encoding gene (BRM1) and 1,3,8-trihydroxynaphthalene (THN) reductase gene (BRM2) was performed with the ß-tubulin rRNA gene as the reference. Real-time RT-quantitative PCRs (RT-qPCRs) were performed in a LightCycler 2.0 (Roche Diagnostics) with a EvaGreen supermix (BioRad). The primers used for gene quantification were based on Fetzner et al., 2014: CmrF: GAAATGTCACCTGCGCAAAC; CmrR: GTCTTGGGCTGCGATAATG; PksF: GATTGCCATC GTCGGTATG; PksR: GGCTCATCGATGAAGCAAC, Brm1F: CTACGAGTGGGCAGACAG; Brm1R: GTACCGCCGATGAA GTGCTG; Brm2F: CCGTGGTATCGGAAAGGC; Brm2R: GA AGTGGGCAACAACGTCAT; BtubF: GTTGAGAACTCAGAC GAGAC; BtubR: CATGTTGACGGCCAACTTC. The expression level of each gene was normalized to the value of the reference (ß-tubulin rRNA gene) according to the 2–ΔΔCT method (Livak and Schmittgen, 2001).
Quantification of Chitin and β-Glucan
A. alternata was grown in liquid MM, MMT, and MMP with or without 100 μg/mL pyroquilon at 28°C, at 120 rpm for 3 days under alternating 16-h light 8-h dark cycle. Quantification of cell wall chitin content was based on the measurement of the glucosamine released by acid hydrolysis of purified cell walls (Fernandes et al., 2014). Cells were disrupted by sonication and washed five times with 1 M NaCl and extracted in SDS-MerOH buffer [50 mM Tris, 2% sodium dodecyl sulphate (SDS), 0.3 M ß-mercaptoethanol, 1 mM EDTA; pH 8.0] at 100°C for 10 min, then washed in dH2O. Cell wall pellets were resuspended in sterile dH2O, freeze dried, and the dry weight of recovered cell walls was measured (Munro et al., 2007). Chitin contents were determined by measuring the glucosamine released by acid hydrolysis of purified cell walls (Kapteyn et al., 2000).
Quantification of glucan contents was performed with the aniline blue assay. The mycelium was washed and then sonicated in 1 M NaOH, and incubated at 52°C for 30 min. β-1,3-glucan concentration was determined by aniline blue fluorescence (Kahn et al., 2006).
Transmission Electron Microscopy (TEM)
The hyphal cell wall structure was evaluated by TEM. Liquid A. alternata cultures were cultivated on MM, MMT, MMP and on these media added with 75 μg/mL of pyroquilon, at 28°C with constant orbital shaking at 180 rpm for 3 days with the day-night cycles described above.
To evaluate the amount and the distribution of melanin accumulated in the cell wall of A. alternata grown in MM, MMT, MMP, we obtained melanin ghosts by chemical and enzymatic removal of the other cellular structures as previously described (Dadachova et al., 2007; Fernandes et al., 2015).
The samples were fixed with 2.5% glutaraldehyde in 0.1 M sodium cacodylate buffer (pH 7.2) for 2 h. Post fixation was performed using 1% osmium tetroxide for 1 h. After they were rinsed with the buffer, the samples were dehydrated in a graded ethanol series (30–100%), impregnated, and embedded in Epoxy resin (Fluka Analytical). Ultrathin sections (80 nm) were mounted on copper grids (300 mesh). Observations were carried out on an FEI-Tecnai G2 Spirit Bio Twin transmission electron microscope at 100 kV.
Scanning Electron Microscopy (SEM)
A. alternata was grown as described for TEM, washed twice in PBS and the mycelia were placed over silicon (Si) substrates and then chemically fixed with 5% glutaraldehyde solution for 15 min. The dehydration process, as previously described (Carvalho et al., 2016), consisted in immersing the set mycelia in solution with increasing concentrations of ethanol in the ethanol:water ratio: 0:100, 20:80, 50:50, and 100:0. In each solution the samples were kept at room temperature for 15 min. After the final step the ethanol was aspirated and the alcohol residue removed by evaporation overnight. Before SEM observation the Si/cells were sputter coated with a 10 nm thick gold layer, to turn the biological material electron conductive. The observations were made using a Philips X30 equipment.
Statistical Analysis
Analysis of variance was performed by One-way ANOVA followed by Tukey multiple comparison test, using Prism (version 5) software (GraphPad Software, Inc., La Jolla, CA). Mann-Whitney test was used for qPCR quantification (Prism). Data are presented as the means ± standard errors of the means (SEMs) and differences were considered significant at P-values of < 0.05.
Results
Production of Melanin Pigments in A. alternata
When grown in MM, A. alternata synthetized a black pigment that was not released into the medium. In MM supplemented with 5.5 mM of L-tyrosine (MMT), A. alternata produced a brown pigment bound to the hypha that was also released into the medium (Figure 2A). However, in MM supplemented with 5.5 mM of L-phenylalanine (MMP), a L-tyrosine precursor, A. alternata mycelium was pale and released a low amount of pigment into the medium (Figure 2A). As a control of the effect of amino acid catabolism on the melanisation, we used L-valine and L-glutamine in the same conditions as L-tyrosine and L-phenylalanine, but we did not observe any difference in relation to the control (MM) (results not shown). We also grew A. alternata in MM supplemented with phenylacetate to evaluate if the phenyl group might inhibit the DHN-melanin synthesis. In the presence of 0.2 and 0.02 g/L of phenylacetate, A. alternata mycelium mat was as black as with MM.
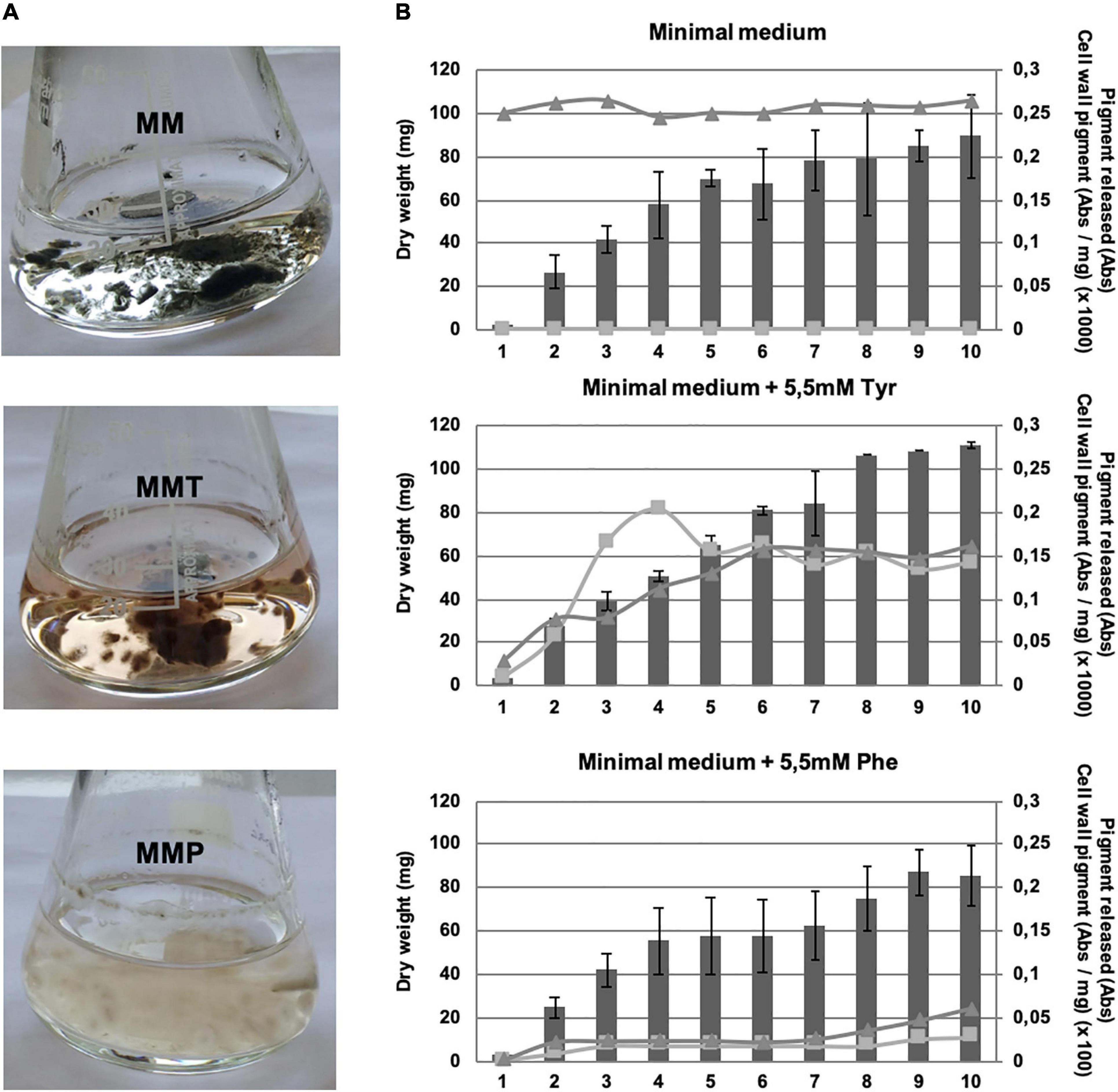
Figure 2. A. alternata melanin synthesis during mycelial growth. (A) Pigment formation of A. alternata grown in MM, in MM supplemented with 5.5 mM of L-tyrosine (MMT) and in MM supplemented with 5.5 mM of L-phenylalanine (MMP) after 3 days of growth. (B) Mycelial growth and quantification of the melanin content in A. alternata cells and released in liquid cultures over 10 days. Bars, mycelium dry weight; squares, pigment released into the medium, given as the absorbance at 405 nm (Abs); triangles, cell wall pigment content, measured by determination of the absorbance at 405 nm, as described in section “Materials and Methods”, and given as the absorbance (Abs) per milligram (dry weight) of mycelia (×1,000 for the scale of MM and MMT growth and × 100 for the MMP growth scale).
We also quantified the growth and the pigment accumulated in the cell wall hyphae and released into the medium along 10 days (Figure 2B). The growth was not enhanced or inhibited by the presence of L-tyrosine or L-phenylalanine. In MM, the mycelium was black from the very beginning of germination and in both MMT and MMP, the melanisation increased over time. Curiously, with MMT the pigment released by A. alternata reached a peak at day 4 and then decreased; this is possibly due to binding to the hyphal cell wall (Figure 2B, middle panel).
A. alternata Pigment Identification
Since A. alternata is known to synthesize DHN-melanin (Kimura and Tsuge, 1993), we grew A. alternata in MM with 50 μg/mL of pyroquilon, a DHN-melanin synthesis inhibitor. Under this condition, A. alternata mycelial mat changed from black to a reddish color and released a typically reddish pigment, a shunt product of the DHN-melanin inhibition (Figures 3A–D). When A. alternata was grown in MMT or in MMT + pyroquilon and in MMP or in MMP + pyroquilon this reddish pigment was not observed. To determine if the presence of pyomelanin masked the release of the reddish pigment, under growth in MMT or in MMT + pyroquilon we performed a chromatographic analysis of the supernatant. Only the culture medium resulting from the growth in MM + pyroquilon led to the detectable shunt intermediate flaviolin (Figures 3A–D).
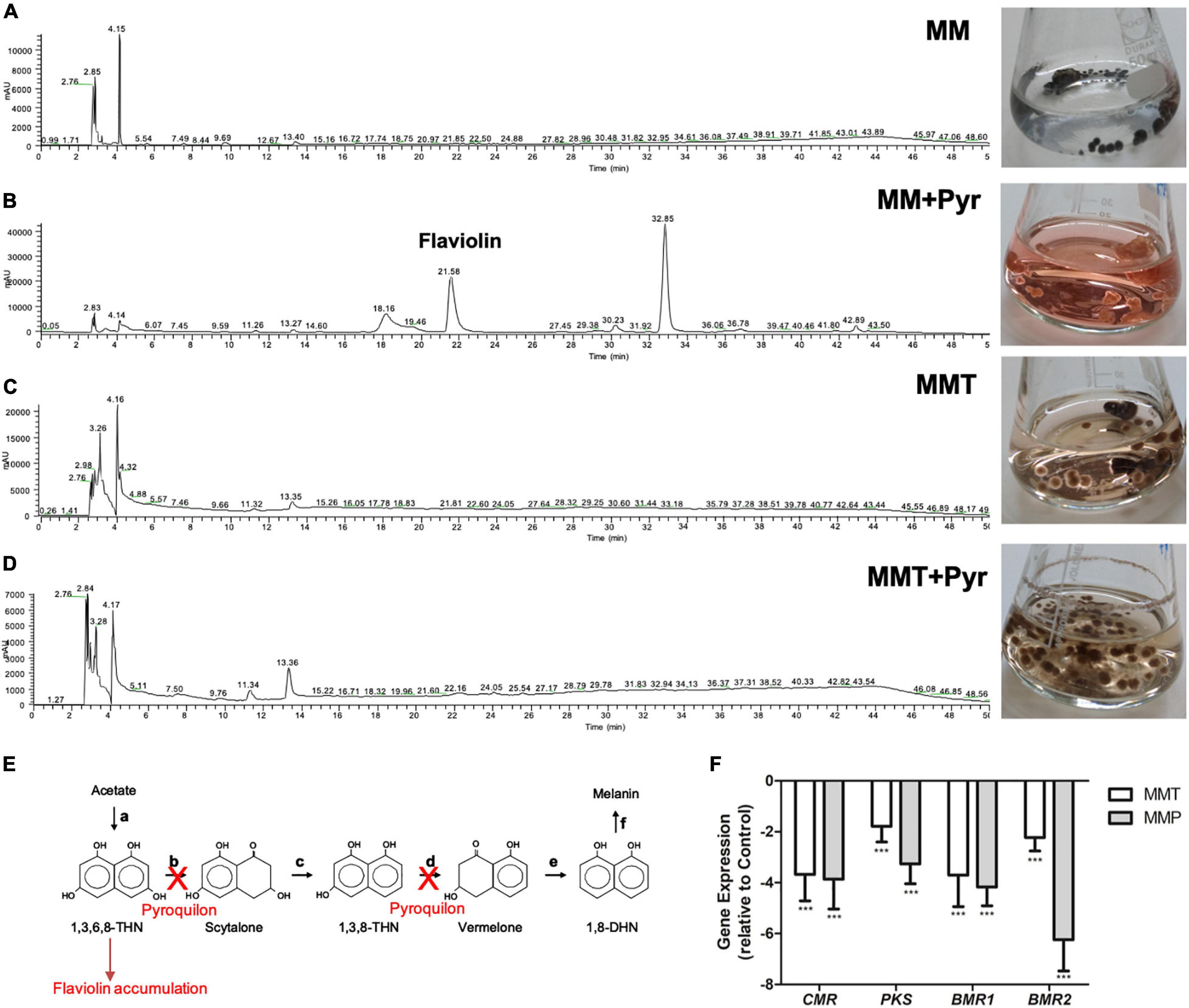
Figure 3. DHN-melanin pathway inhibition in A. alternata under tyrosine metabolism. (A–D) Chromatographic separation of the components of the supernatants of A. alternata grown in (A) MM, (B) MM + pyroquilon, (C) MMT and (D) MMT + pyroquilon and respective growth in the Erlenmeyers. In MM + pyroquilon, the DHN-melanin pathway is inhibition by pyroquilon leads to the released of the carmine shunt product flaviolin. (E) Biosynthetic pathway leading to the formation of DHN-melanin from acetate and identification of the enzymes involved [(a), polyketide synthase; (b), T4HN reductase; (c), scytalone dehydratase; (d), T3HN reductase; (e), vermelone dehydratase; (f), several candidate enzymes for this step] in fungi. 1,3,6,8-THN = 1,3,6,8-tetrahydroxynaphthalene; 1,3,8-THN = 1,3,8-trihydroxynaphthalene; 1,8-DHN = 1,8-dihydroxynaphthalene. Pyroquilon inhibits the T4HN reductase and the T3HN reductase which leads to the accumulation of flaviolin (Wheeler and Klich, 1995). (F) Analysis of the expression of CMRA, PKS, BRM1, BRM2 in A. alternata mycelium cultured in MM, MMT and MMP. Strains were grown for 3 days before mycelium was harvested and RNA isolated. RT-PCR was performed as described in section “Materials and Methods” with the ß-tubulin gene as standard and in comparison to the control MM. Results are the mean ± standard errors of the means (SEMs) of triplicates of three independent experiments (Mann-Whitney, Prism): ***P < 0.001.
To understand the reason of DHN-melanin lack of accumulation in A. alternata cell wall under the metabolism of L-tyrosine and L-phenylalanine, we determined the level of gene expression of the CMR gene, the transcription factor of the genes coding for the melanin pathway, and of the genes encoding the proteins involved in DHN-melanin synthesis (Figure 3E). We observed a statistical significative decrease in the expression of all these genes in A. alternata grown in MMT and MMP in relation to growth in MM (Figure 3F).
The pathway for pyomelanin synthesis from L-tyrosine catabolism was previously elucidated (Figure 1A; Boylan et al., 1987; Schmaler-Ripcke et al., 2009; Rodríguez-Rojas et al., 2009). The open reading frames encoding the enzymes putatively involved in L-tyrosine degradation and in the HGA formation were identified in A. alternata genome by a BLAST search at the JGI database2 (Figure 1B). The catabolism of L-tyrosine begins with a α-ketoglutarate dependent transamination through the L-tyrosine transaminase to para-hydroxyphenylpyruvate. Then, HppD (Protein Id: 950966) catalyzes the formation of HGA that yield to 4-maleylacetoacetate by the action of a HmgA homogentisate dioxygenase (Protein Id: 932428) and then to 4-fumarylacetoacetate and acetoacetate + fumarate in two reactions catalyzed by the maleylacetoacetate isomerase (Protein Id: 973265) and the fumarylacetoacetate hydrolase (Protein Id: 1019048), respectively. Depending on the activity of these enzymes, HGA may accumulate on the cell and can be excreted and auto oxidizes to benzoquinoneacetate that self-polymerizes into pyomelanin. Interestingly, an open reading frame encoding a putative transporter (Protein Id: 1030019) is present in this operon. The protein encoded by this gene may be involved in the transportation of HGA out of the cell (Figure 1B). The presence of these genes in A. alternata genome confirmed that A. alternata is provided with the enzymes needed for the synthesis of pyomelanin upon the L-tyrosine catabolism (Figures 1A,B). The pyomelanin synthesis inhibitor sulcotrione (100 μg/mL) reduced pigment released by A. alternata grown in MMT and prevented formation of pigment when cultivated in MMP. FTIR spectroscopy was used to analyze the pigment released by A. alternata grown in MMT and in MM + HGA (Figure 1C). The spectra are equivalent, confirming the similarity of the pigments produced under these two different conditions. The spectra exhibit a broad absorption at 3,280 cm–1, due to OH groups, the stretching vibrations for aliphatic CH bonding appear at 2,916 cm–1 and the carboxylate stretching vibrations (COO–) are detectable at 1,600 (1,601 for MMT and 1,599 for MM + HGA) and 1,360 for MMT (1,359 for MM + HGA) cm–1. It is established that pyomelanin pigment results from the polymerization of HGA (Rodríguez et al., 2000). Since we obtained a high level of identity between the FTIR spectra of the pigments synthesized from A. alternata cultured with tyrosine or HGA, this indicates that A. alternata produces pyomelanin when cultivated with L-tyrosine. As we can see in Figure 1D, the mycelia and the culture medium are brown as when A. alternata is grown in MMT (Figure 3C). The amount of melanin extracted from the medium of A. alternata grown in MMP was too low for FTIR spectral analysis.
Ultrastructural Changes in A. alternata Hypha Grown in MM, MMT, and MMP Medium
Transmission electron microscopy (TEM) and scanning electron microscopy (SEM) were used to appraise the ultrastructural changes in A. alternata hyphae grown in MM, MMT, or MMP medium and under these conditions plus pyroquilon. Using TEM, we observed that when grown in MM medium, under DHN-melanin synthesis conditions, A. alternata cell wall showed an internal electron-dense material layer (Figure 4, arrow), consistent with DHN-melanin being imbedded in the cell wall. When grown in MM + pyroquilon, this internal electron-dense material vanished (Figure 4). Yet, when A. alternata was grown in MMT (with or without pyroquilon), the electron-dense material is not internally distributed but is located at the cell wall surface (Figure 4). We also observed that the cell wall surface is rougher due to the presence of a superficial large electron-dense layer of pyomelanin (Figure 4). This electron-dense layer, supposedly of pyomelanin, is also present to a lower extent in A. alternata grown in MMP both in the presence of absence of pyroquilon (Figure 4).
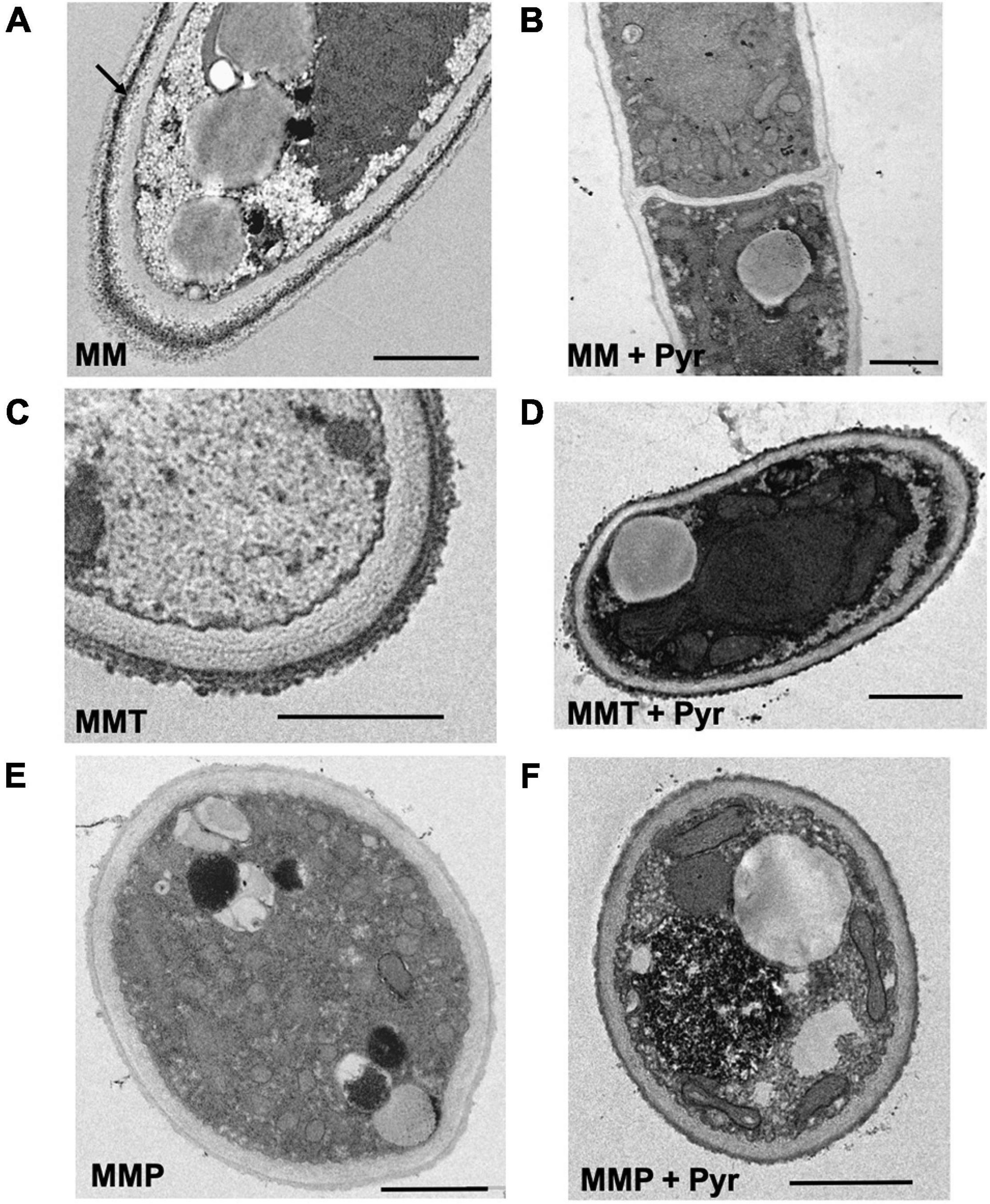
Figure 4. Transmission electron microscopic observations of the hyphae and hyphal cell wall morphology of A. alternata grown under different conditions: (A) MM, (B) MM with the presence of 50 μg/mL of pyroquilon, (C) MMT, (D) MMT supplemented with 50 μg/mL of pyroquilon, (E) MMP and (F) MMP + 50 μg/mL of pyroquilon, after 3 days of growth. Arrow in (A) show an internal melanin layer. Bars, 1,000 nm.
From TEM images we quantified the cell wall thickness and observed differences in the cell wall thickness depending on the growth media (Figure 5). We observed that the conditions leading to higher pigment cell wall contents (MM and MMT) presented significantly thicker cell walls than when A. alternata was grown in MMP or MM supplemented with pyroquilon (Figure 5). When A. alternata was grown in MMT or MMP, and under these conditions plus pyroquilon, there was no significant reduction in cell wall thickness, indicating the absence of DHN-melanin from the cell walls does not lead to increased cell wall thickness. Otherwise, when MM or MMP were supplemented with pyroquilon the cell wall decreased in relation to control (MM). Also, MMP supplementation with pyroquilon does not change the cell wall thickness when compared with MMP alone (Figure 5).
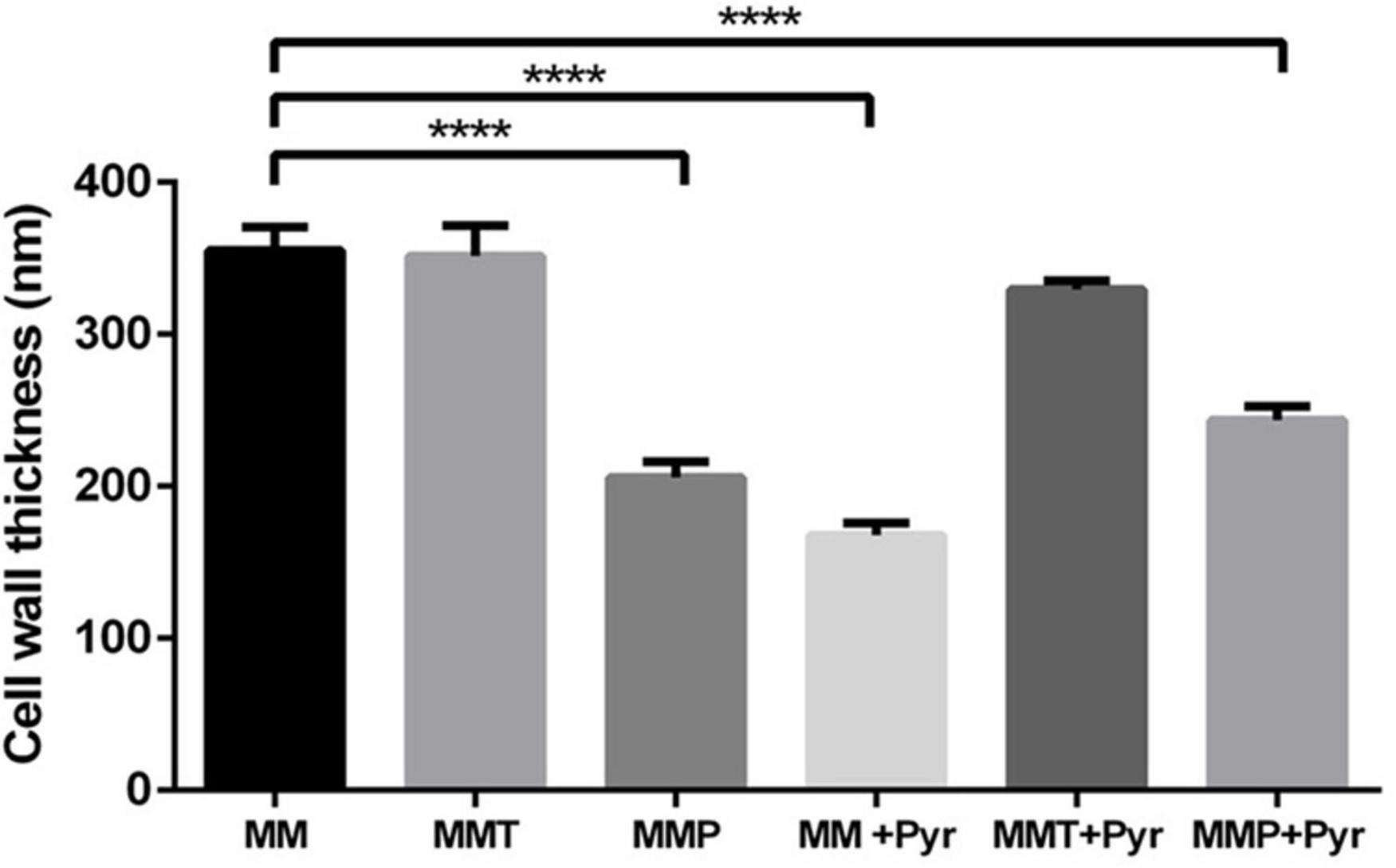
Figure 5. Measurement of the A. alternata cell wall when grown in MM, MMT, MMP and under these conditions with pyroquilon. A. alternata was cultivated in liquid cultures for 3 days with alternating 16-h light and 8-h dark periods. Results are the mean ± the standard error of the mean of triplicates of three independent experiments (Student’s t-test). ****P < 0.0001.
By SEM, we observed decorations coating A. alternata grown in MM (Figure 6A) and in MMT and MMT + pyroquilon (Figures 6B,E). These decorations were not present when A. alternata was grown in MM + pyroquilon (Figure 6D), MMP or MMP + pyroquilon (Figures 6C,F), indicating that these decorations are due to DHN-melanin and the surface layer (the most external observed when grown in MM; Figure 4A) and to a dense pyomelanin coating in the case of growth in MMT and MMT + pyroquilon.
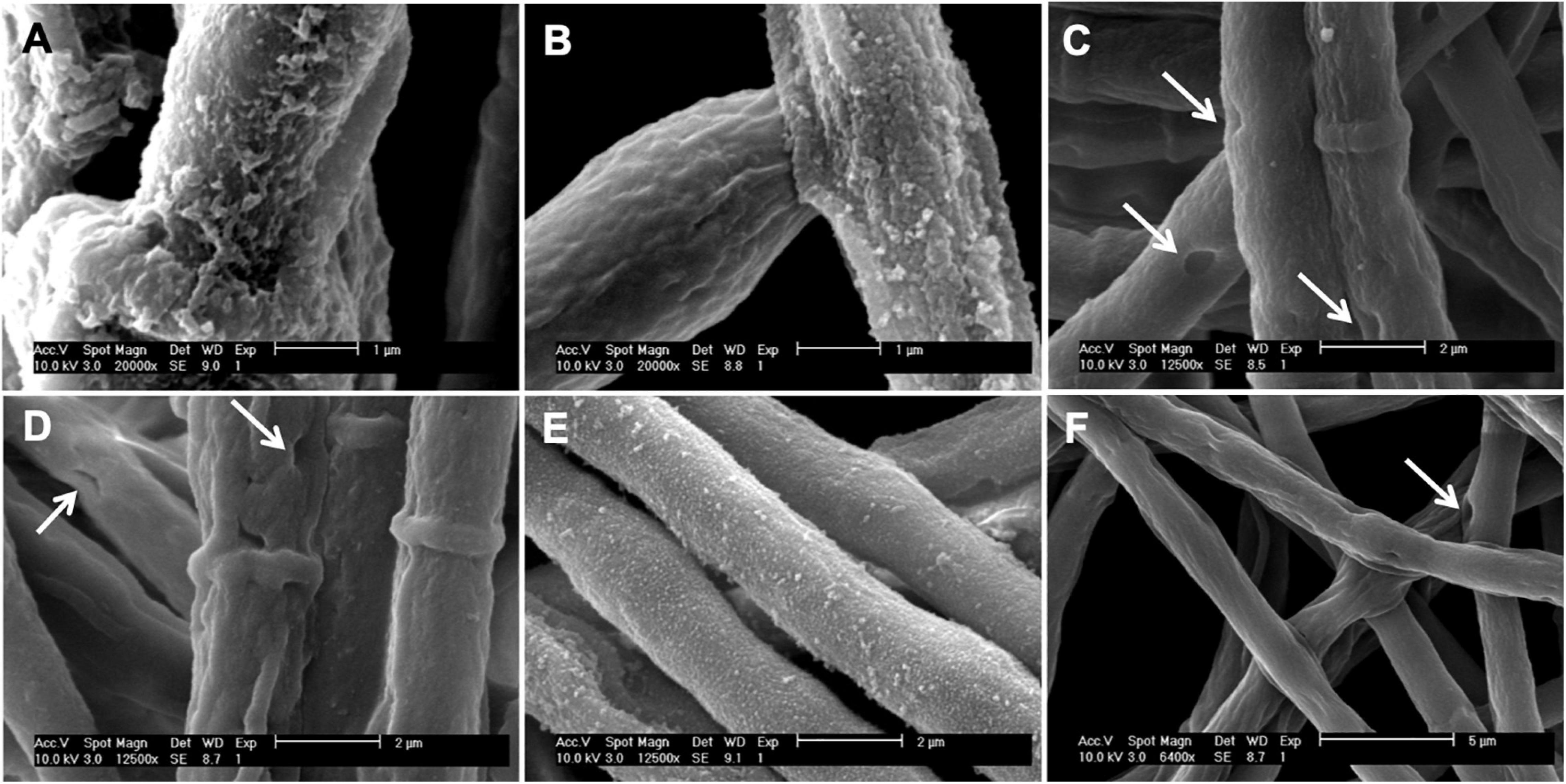
Figure 6. Scanning electron micrographs of the A. alternata hyphae grown in (A) MM, (B) MMT, (C) MMP, (D) MM + pyroquilon, (E) MMT + pyroquilon and (F) MMP + pyroquilon. Arrows indicate holes in the cell wall when the fungus is grown in MM + pyroquilon, MMP and MMP + pyroquilon.
When A. alternata was cultured in MMP, with or without pyroquilon, we observed numerous holes in the cell wall (Figure 6C). These were similar in appearance to those observed in MM + pyroquilon (Figure 6D) although in this condition were less frequent. We interpret these results as an indication of a damaged cell wall in the absence or reduced amounts of DHN-melanin.
Melanin particles of hollow spherical shape can be isolated from melanized cells by digestion in concentrated acid and are called “ghosts” because they retain the shape and dimensions of the parent cell due to the melanin structure, as previously performed in the yeast Cryptococcus neoformans (Rosas et al., 2000; Dadachova et al., 2007). Although A. alternata is a filamentous fungus, we performed this procedure and obtained melanin ghosts from fungus grown in MM, MMT and MMP. The resulting structures from A. alternata grown in MM did not fully retain hyphal shape but did retained the septa in a cell wall structure that was more spherical (Figure 7D). In contrast, when A. alternata was grown in MMT or MMP, no septa were observed, the cell walls collapsed and the remaining structures aggregated (Figures 7E,F). Growth in MM also resulted in the deposition of melanin granules along the cell wall according to a gradient, with the density of these particles increasing from the inner to the outer layers of the cell wall (Figure 7A). The L-tyrosine (MMT) or L-phenylalanine (MMP) metabolism led to alterations of this pattern, such that the density of melanin granules increased in the center of the electron-dense layer and then decreased along the inner and outer layers (Figures 7B,C, respectively).
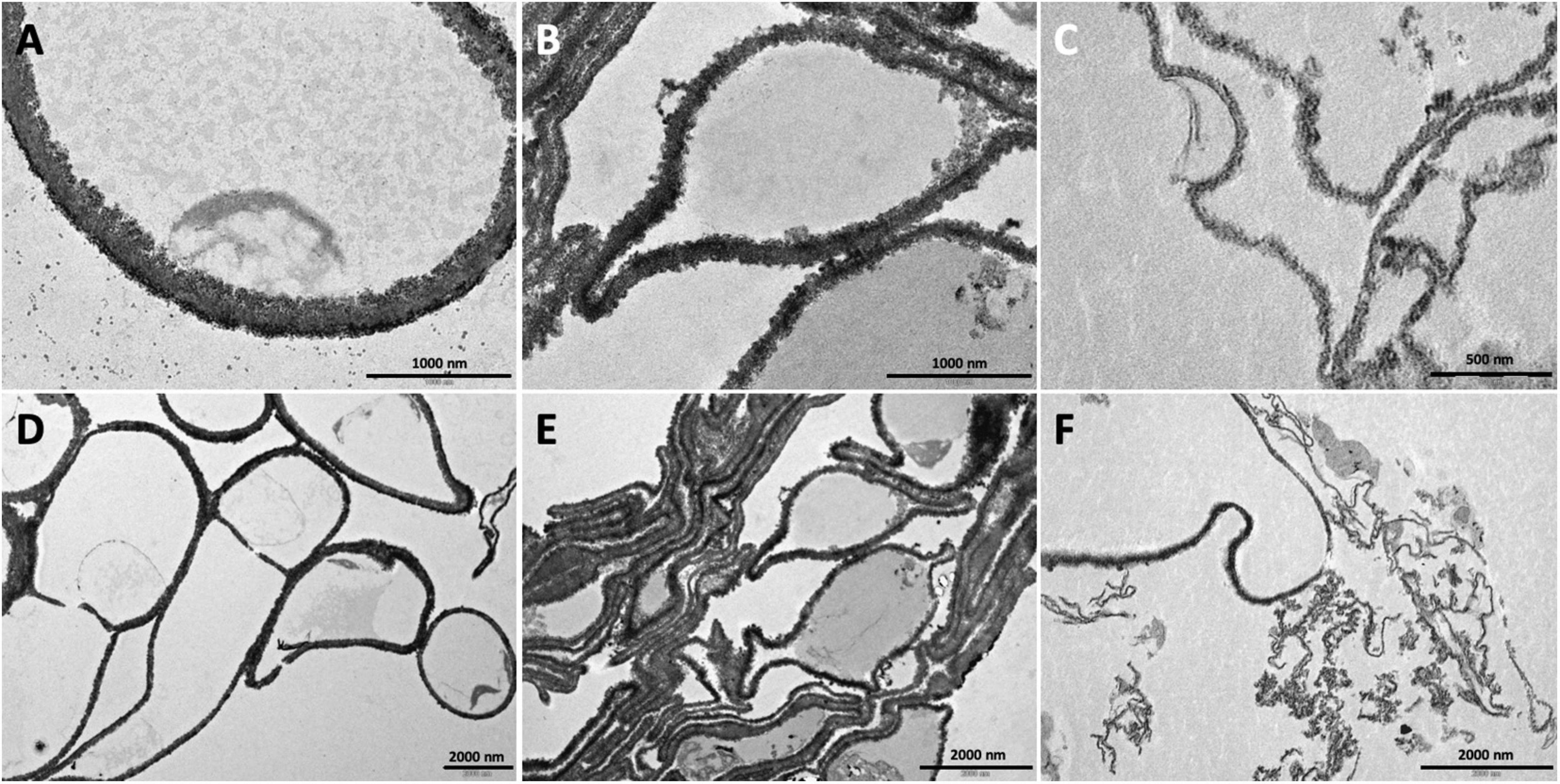
Figure 7. Transmission electron micrograph of the melanin ghosts prepared from A. alternata hyphae grown in MM (A,D), MMT (B,E) and MMP (C,F). Bars, 1,000 nm in (A–C) and 2,000 nm in (D–F).
Effects of L-Tyrosine and L-Phenylalanine Metabolism on Cell Wall Chitin and β-Glucan Content
Since growth in MMT and MMP impairs the cell wall DHN-melanin content (Figure 3), we aimed to appraise the modulation of other cell wall components. For that, the cell wall chitin and β-glucan contents were quantified in A. alternata mycelia obtained from MM, MMT-, and MMP-grown fungus. The absence of DHN-melanin synthesis (MM + Pyr) inhibited the chitin content to 62.5 ± 6.8% when A. alternata is grown in minimal media (MM; control conditions). The chitin content in the cell walls of fungi grown in MMT and MMP was similar, but corresponding to half of that quantified under control conditions (MM) (Figure 8). Adding pyroquilon to MMP and to MMT only decreased slightly the amount of chitin (not statistically significant). The glucan content followed the opposite pattern (Figure 8); in fact, MMP- and MMT-grown fungi had more glucan than in the MM-grown condition (130.2 ± 5.2 and 177.2 ± 2.9%, respectively) and adding pyroquilon did not significantly altered cell wall glucan content in any condition tested (Figure 8).
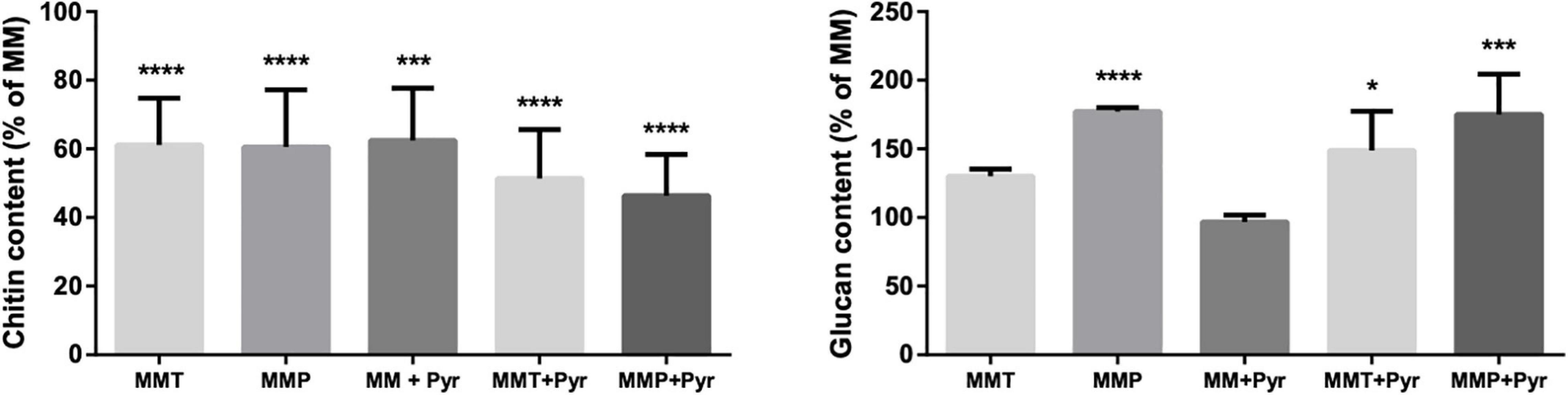
Figure 8. Cell wall components levels in A. alternata grown in the presence and absence of L-tyrosine or L-phenylalanine metabolism. Chitin and β-1,3-glucan contents of A. alternata are shown. A. alternata was cultivated in liquid cultures for 3 days with alternating 8-h light and 16-h dark periods. Results are the mean ± standard errors of the means (SEMs) of triplicates of three independent experiments (Analysis of variance was performed by One-way ANOVA and followed by Tukey multiple comparison test, Prism): *P < 0.05; ***P < 0.001; ****P < 0.0001.
Discussion
DHN-melanin is recognized by MelLec receptors in the human host (Stappers et al., 2018) and melanins are considered virulence factors since this pigment protects fungal cells against the attack by immune effector cells by diverse mechanisms, such as inhibiting cytokine production in the host or by scavenging free radicals (Chai et al., 2010; Eisenman and Casadevall, 2012; Heinekamp et al., 2013). Furthermore, melanin makes the fungal cell wall stronger, thus conferring protection against antifungals (Fernandes et al., 2015). The anchorage of melanin to the cell wall has been associated to the cell wall chitin content and structure, another component that is responsible for the cell wall strength (Lenardon et al., 2010). In fact, in both Candida albicans and Cryptococcus neoformans the supplementation with GlcNAc, which results in increased synthesis of cell wall chitin, impacts melanisation (Walker et al., 2010; Camacho et al., 2017). In this context, studies on the process of melanisation and on the inhibition of melanin synthesis in fungi are of particular importance for the elucidation of pathogenesis mechanisms and identification of new drug targets.
In the present work, we report for the first time that A. alternata produce another melanin-type pigment in addition to DHN-melanin, which was identified as pyomelanin, using Fourier transform infrared spectroscopy (FTIR). This pigment derives from L-tyrosine through formation of 4-hydroxyphenylpyruvate and HGA (Figure 3; Fernández-Cañón and Peñalva, 1995; Schmaler-Ripcke et al., 2009). Depending on the HmgA activity, HGA accumulates and is secreted from the cell (Yabuuchi and Ohyama, 1972) and then, after auto-oxidation and self-polymerization, forms a brown pigment, pyomelanin (Rodríguez et al., 2000). Here we establish that in A. alternata this pigment is produced from the beginning of culture growth and is released into the culture medium, and deposited on the hyphal surface as observed by SEM and TEM, leading to dark brown hypha. The addition of sulcotrione, a HppD inhibitor, to A. alternata culture medium with L-tyrosine reduced pyomelanin formation but did not abolished it totally, questioning the specificity of this inhibitor for the HppD from A. alternata. L-phenylalanine, a L-tyrosine precursor also induced the formation of pyomelanin, but to a lower extent than L-tyrosine, leading to an albino-like phenotype. This is probably due to the progressive conversion of L-phenylalanine into L-tyrosine. As L-tyrosine is formed, it is converted into hydroxyphenylpyruvate that is then converted into HGA. This compound is broken down into fumarate and acetoacetate (catabolism of L-tyrosine pathway) leading, consequently to low HGA accumulation and low pyomelanin formation (Figure 9). This effect seems independent of amino acid catabolism in general, since neither L-valine and L-glutamine changed the melanisation pattern.
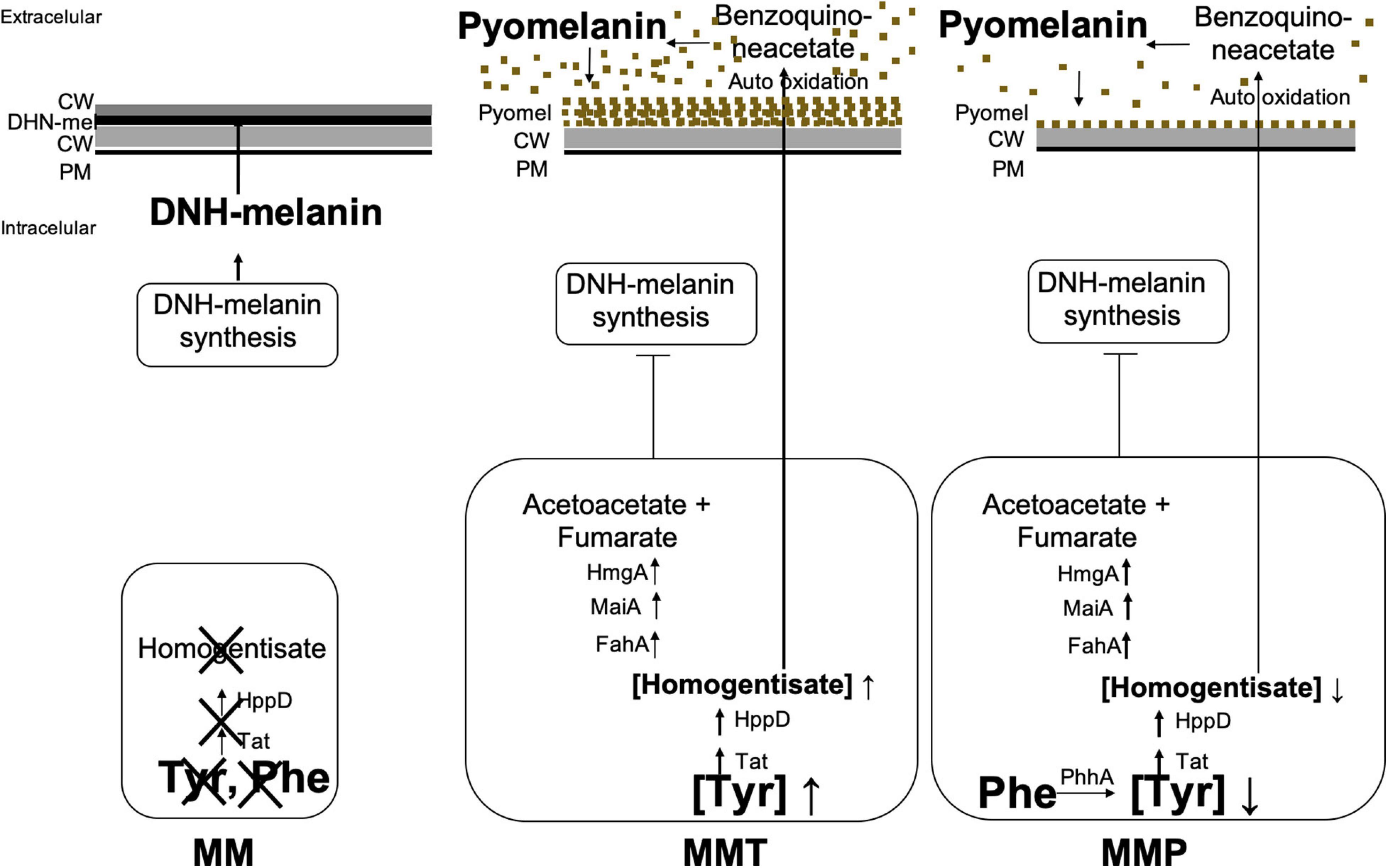
Figure 9. Regulation of pyomelanin synthesis in A. alternata. Here, we report that when A. alternata is grown in MM medium, no precursor of HGA is present, thus there is no formation of pyomelanin and the DHN-melanin pigment is formed. In MMT medium, L-tyrosine leads the production of large concentrations of HGA that accumulates in the cell and is released into the medium leading to the formation of pyomelanin. There is inhibition of DHN-melanin synthesis. The fungus turns brown. In MMP medium, L-phenylalanine, a L-tyrosine precursor is progressively converted into L-tyrosine by PhhA and also leads to the formation of HGA, but to a lower extent than with L-tyrosine supplemented into the medium. This is probably due to the progressive conversion of L-phenylalanine into L-tyrosine. As L-tyrosine is formed, it is converted into hydroxyphenylpyruvate that is then converted into HGA. This compound is degradated into fumarate and acetoacetate (catabolism of L-tyrosine pathway) leading, consequently to low HGA accumulation and efflux out of the cell and low pyomelanin formation. The DHN-melanin synthesis is inhibited leading to an albino-like phenotype.
Pyroquilon has the same mechanism of action as other DHN-melanin synthesis inhibitors like tricyclazole, since it inhibits the hydroxynaphtalene reductases, which leads to the accumulation of the shunt products flaviolin and 2-hydroxyjuglone (Wheeler and Klich, 1995). When A. alternata was grown in MM medium with pyroquilon, the hyphae were not black and we observed the release and accumulation into the medium of carmine red compounds. Earlier we described this effect of pyroquilon in A. infectoria, which was also reported in other fungi, providing evidence that the DHN-melanin biosynthesis is inhibited (Geis et al., 1984; Fernandes et al., 2015). However, when A. alternata was grown in minimal media supplemented with L-tyrosine (MMT) with pyroquilon or with L-phenylalanine (MMP) with pyroquilon, we did not observe the generation of these products, which lead us to conclude that DHN-melanin was not synthesized by A. alternata during L-tyrosine or L-phenylalanine metabolism. Moreover, under these conditions, we observed a significative decrease in the expression of the genes encoding enzymes of the DHN-melanin pathway. In fact, two genes, encoding a polyketide synthase (PKSA) and a 1,3,8-trihydroxynaphthalene reductase (BRM2), along with their transcription factor, CmrA, that compose a small gene cluster were down-regulated as well as a scytalone dehydratase encoding gene (BRM1), located elsewhere in the genome but that is also under the control of this transcription factor, CmrA (Fetzner et al., 2014).
In addition to the synthesis of pyomelanin under L-tyrosine and L-phenylalanine metabolism, we also report modifications of A. alternata cell wall structure and surface decorations depending on the synthesis of the different types of melanin. In recent years, the importance of the cell wall surface in the interaction of filamentous fungi with the host has become a subject of great interest together with surface differences among fungal species (Aimanianda et al., 2009; Rambach et al., 2015; Gow et al., 2017). Most of the current knowledge on melanin interactions with host receptors was obtained with A. fumigatus, where the conidia are covered with a proteinaceous layer of hydrophobins and hyphae are covered with polysaccharides (Aimanianda et al., 2009; Rambach et al., 2015). The information regarding the cell wall organization of fungi belonging to the genus Alternaria is scarce. Here, we noted extensive surface alterations in A. alternata hypha using SEM and TEM when the fungus was grown under the tested conditions and in the presence of a DHN-melanin synthesis inhibitor. In MMT, A. alternata was coated with a superficial electron-dense layer, probably made up with pyomelanin, that did not vanish even in the presence of pyroquilon, indicating that this layer is not DHN-melanin. In MMP, in the presence or absence of pyroquilon, the fungal cell walls present a smooth surface with high number of holes. In fact, under low melanotic pigment conditions (MM + pyr, MMP and MMP + Pyr) the fungi exhibit holes in the cell wall structure. Based on this observation there is a high likelihood that DHN-melanin or pyomelanin play a role in A. alternata hyphal cell wall final assembly and robustness. Consistent with this notion, when A. alternata was grown in MM + pyroquilon, the hyphal cell walls were smoother, thinner, and lighter than when melanin was synthetized. We also prepared melanin ghosts from A. alternata hyphae previously grown in MM, MMT or MMP. In contrast to the ghosts produced from the fungi grown in MM, the melanin ghosts from the A. alternata cultured in MMT and MMP collapsed and aggregated, indicating that DHN-melanin was required for cell wall strength and proper architecture. Although there are strong indications that melanin is essential for cell wall organization and function (Fernandes et al., 2015; Gow et al., 2017), here we show how depending on the melanin synthetized, the cell wall is modulated. Moreover, we observed that the cell wall chitin decreased significantly under pyomelanin synthesis, when no DNH-melanin was synthetized, and that this decrease was compensated by increasing β-glucan levels, possibly as a salvage compensatory mechanism. Otherwise, under MM growth, the higher chitin contents associated to DHN-melanin biosynthesis can be important as a scaffold to cross-link DHN-melanin to the cell wall components, as demonstrated previously in other fungi that instead of DHN-melanin synthetize L-DOPA-melanin (Walker et al., 2010; Camacho et al., 2017). Consistent with this, for both Candida albicans and Cryptococcus neoformans, supplementation with GlcNAc increases the synthesis of the cell wall chitin, and affects melanisation (Walker et al., 2010; Camacho et al., 2017). Similarly, it has been demonstrated that the composition of the cell wall and its molecular arrangement are critical factors for the anchoring of melanin in C. neoformans and C. gattii (Banks et al., 2005; Chrissian et al., 2020). Putting together those observations with our results in this study suggests that the association between melanin and chitin synthesis may be a general principle in the fungal kingdom. Yet, during specific environmental conditions, such as L-tyrosine and L-phenylalanine metabolism, when DHN-melanin is not produced, chitin may not be as necessary to provide a cell wall structure acting as a scaffold for this pigment. In growth conditions that bolster pyomelanin synthesis, we anticipate that this soluble pigment does not require anchorage; it is released into the external medium and then polymerizes to the cell wall. Nevertheless, under these conditions, a lower chitin turns the fungal cell wall more fragile, as attested by SEM and TEM.
In summary, we report that changing growth conditions and the availability of the amino acids L-tyrosine and L-phenylalanine results in profound changes to A. alternata pigment production and cell wall architecture (summarized in Figure 9). Our major finds were that: (1) DHN-melanin production is inhibited during pyomelanin synthesis; (2) the absence of DHN-melanin accumulation is associated to a significant reduction in cell wall chitin content; and (3) that the cell wall architecture is affected by which type of melanin is being synthetized. Given that A. alternata is an opportunistic agent of animal/human infection that is almost certain to encounter, during infection, nutritional environments where the amino acids L-tyrosine and L-phenylalanine are available, there is a high likelihood that similar pigment responses occur in vivo. These changes could influence the cell wall structure, and thus impact the robustness and preparedness of the fungal cell when it faces host defenses, which in turn could affect the outcome of colonization/infection.
Data Availability Statement
The original contributions presented in the study are included in the article/supplementary material, further inquiries can be directed to the corresponding author/s.
Author Contributions
CF performed the design of the work, all the experimental procedures (except HPLC and electron microscopy acquisition), analysis, interpretation of data, and most of the writing and revision of the manuscript. MM contributed for the gene expression quantification. LB, MD, and IF performed HPLC analysis. AP carried out Scanning Electron Microscopy. AC participated in the writing and critically revised the work highlighting the scientific impact of the work. TG contributed to the conception and design of the work, funding, analysis and interpretation of data, and writing and revision of the manuscript. All authors contributed to the article and approved the submitted version.
Funding
This study was partly supported by the FEDER funds through the Operational Programme Competitiveness Factors-COMPETE and national funds by FCT-Foundation for Science and Technology under the strategic projects UIDB/00285/2020 and UID/NEU/04539/2013 the European Regional Development Fund (ERDF), through the Centro 2020 Regional Operational Programme under project CENTRO-01-0145-FEDER-000012 and project CENTRO-01-0145-FEDER-022095:ViraVector, and through the COMPETE 2020—Operational Programme for Competitiveness and Internationalisation and Portuguese national funds via FCT—Fundação para a Ciência e a Tecnologia, under project UIDB/04539/2020, and the European Regional Development Fund (ERDF), through the Centro 2020 Regional Operational Programme: project CENTRO-01-0145-FEDER-000012-HealthyAging 2020, the COMPETE 2020—Operational Programme for Competitiveness and Internationalisation, and the Portuguese national funds via FCT—Fundação para a Ciência e a Tecnologia, I.P.: project POCI-01-0145-FEDER-007440. IF thank the Foundation for Science and Technology (FCT, Portugal) and FEDER under Program PT2020 for financial support to CIMO (UID/AGR/00690/2013), LB (SFRH/BPD/107855/2015) and MD (SFRH/BD/84485/2012) grants. To POCI-01-0145-FEDER-006984 (LA LSRE-LCM), funded by ERDF, through POCI-COMPETE2020 and FCT. AC was supported in part by 5R01HL059842, 5R01AI033774, 5R37AI033142, and 5R01AI052733.
Conflict of Interest
The authors declare that the research was conducted in the absence of any commercial or financial relationships that could be construed as a potential conflict of interest.
Publisher’s Note
All claims expressed in this article are solely those of the authors and do not necessarily represent those of their affiliated organizations, or those of the publisher, the editors and the reviewers. Any product that may be evaluated in this article, or claim that may be made by its manufacturer, is not guaranteed or endorsed by the publisher.
Acknowledgments
We thank M. Ermelinda S. Eusébio from the Chemistry Department, FCTUC, for the use of the FTIR spectrometer.
Abbreviations
MM, minimal salt medium; MMT, minimal salt medium supplemented with L-tyrosine; MMP, minimal salt medium supplemented with L-phenylalanine.
Footnotes
References
Aimanianda, V., Bayry, J., Bozza, S., Kniemeyer, O., Perruccio, K., Elluru, S. R., et al. (2009). Surface hydrophobin prevents immune recognition of airborne fungal spores. Nature 460, 1117–1121. doi: 10.1038/nature08264
Anjos, J., Fernandes, C., Silva, B. M., Quintas, C., Abrunheiro, A., Gow, N. A., et al. (2012). β(1,3)-glucan synthase complex from Alternaria infectoria, a rare dematiaceous human pathogen. Med. Mycol. 50, 716–725. doi: 10.3109/13693786.2012.675525
Banks, I. R., Specht, C. A., Donlin, M. J., Gerik, K. J., Levitz, S. M., and Lodge, J. K. (2005). A chitin synthase and its regulator protein are critical for chitosan production and growth of the fungal pathogen Cryptococcus neoformans. Eukaryot. Cell 4, 1902–1912. doi: 10.1128/ec.4.11.1902-1912.2005
Boitano, S., Flynn, A. N., Sherwood, C. L., Schulz, S. M., Hoffman, J., Gruzinova, I., et al. (2011). Alternaria alternata serine proteases induce lung inflammation and airway epithelial cell activation via PAR2. Am. J. Physiol. Lung Cell Mol. Physiol. 300, 605–614.
Boylan, M. T., Mirabito, P. M., Willett, C. E., Zimmerman, C. R., and Timberlake, W. E. (1987). Isolation and physical characterization of three essential conidiation genes from Aspergillus nidulans. Mol. Cell Biol. 7, 3113–3118. doi: 10.1128/mcb.7.9.3113-3118.1987
Camacho, E., Chrissian, C., Cordero, R. J. B., Liporagi-Lopes, L., Stark, R. E., and Casadevall, A. (2017). N-acetylglucosamine affects Cryptococcus neoformans cell-wall composition and melanin architecture. Microbiology 163, 1540–1556. doi: 10.1099/mic.0.000552
Carvalho, D., Sousa, T., Morais, P. V., and Piedade, A. P. (2016). Polymer/metal nanocomposite coating with antimicrobial activity against hospital isolated pathogen. Appl. Surf. Sci. 379, 489–496. doi: 10.1016/j.apsusc.2016.04.109
Carzaniga, R., Fiocco, D., Bowyer, P., and O’Connell, R. J. (2002). Localization of melanin in conidia of Alternaria alternata using phage display antibodies. Mol. Plant Microbe Interact. 15, 216–224. doi: 10.1094/mpmi.2002.15.3.216
Chai, L. Y., Netea, M. G., Sugui, J., Vonk, A. G., van de Sande, W. W., Warris, A., et al. (2010). Aspergillus fumigatus conidial melanin modulates host cytokine response. Immunobiol. 215, 915–920. doi: 10.1016/j.imbio.2009.10.002
Cho, Y., Srivastava, A., Ohm, R. A., Lawrence, C. B., Wang, K. H., Igor, V., et al. (2012). Transcription Factor Amr1 induces melanin biosynthesis and suppresses virulence in Alternaria brassicicola. PLoS Pathog. 8:e1002974. doi: 10.1371/journal.ppat.1002974
Chrissian, C., Camacho, E., Fu, M. S., Prados-Rosales, R., Chatterjee, S., Cordero, R. J. B., et al. (2020). Melanin deposition in two Cryptococcus species depends on cell-wall composition and flexibility. J. Biol. Chem. 295, 1815–1828. doi: 10.1074/jbc.ra119.011949
Cleary, M., Trefz, F., Muntau, A. C., Feillet, F., van Spronsen, F. J., Burlina, A., et al. (2013). Fluctuations in phenylalanine concentrations in phenylketonuria: a review of possible relationships with outcomes. Mol. Genet. Metab. 110, 418–423. doi: 10.1016/j.ymgme.2013.09.001
Dadachova, E., Bryan, R. A., Huang, X., Moadel, T., Schweitzer, A. D., Aisen, P., et al. (2007). Ionizing radiation changes the electronic properties of melanin and enhances the growth of melanized fungi. PLoS One 2:e457. doi: 10.1074/jbc.RA119.011949
Denning, D. W., Pashley, C., Hartl, D., Wardlaw, A., Godet, C., Del Giacco, S., et al. (2014). Fungal allergy in asthma—state of the art and research needs. Clin. Transl. Allergy 4:14. doi: 10.1186/2045-7022-4-14
Eisenman, H. C., and Casadevall, A. (2012). Synthesis and assembly of fungal melanin. Appl. Microbiol. Biotechnol. 93, 931–940. doi: 10.1007/s00253-011-3777-2
Fernandes, C., Anjos, J., Walker, L. A., Silva, B. M., Cortes, L., Mota, M., et al. (2014). Modulation of Alternaria infectoria cell wall chitin and glucan synthesis by cell wall synthase inhibitors. Antimicrob. Agents Chemother. 58, 2894–2904. doi: 10.1128/aac.02647-13
Fernandes, C., Prados-Rosales, R., Silva, B., Nakouzi-Naranjo, A., Zuzarte, M., Chatterjee, S., et al. (2015). Activation of melanin synthesis in Alternaria infectoria by antifungal drugs. Antimicrob. Agents Chemother. 60, 1646–1655. doi: 10.1128/aac.02190-15
Fernández-Cañón, J. M., and Peñalva, M. A. (1995). Fungal metabolic model for human type I hereditary tyrosinaemia. Proc. Natl. Acad. Sci. U.S.A. 92, 9132–9136. doi: 10.1073/pnas.92.20.9132
Fernstrom, J. D., and Fernstrom, M. H. (2007). Tyrosine, phenylalanine, and catecholamine synthesis and function in the brain. J. Nutr. 137, 1539S–1547S.
Fetzner, R., Seither, K., Wenderoth, M., Herr, A., and Fischer, R. (2014). Alternaria alternata transcription factor CmrA controls melanization and spore development. Microbiol. 160, 1845–1854. doi: 10.1099/mic.0.079046-0
Frases, S., Salazar, A., Dadachova, E., and Casadevall, A. (2007). Cryptococcus neoformans can utilize the bacterial melanin precursor homogentisic acid for fungal melanogenesis. Appl. Environ. Microbiol. 73, 615–621. doi: 10.1128/aem.01947-06
Fukutomi, Y., and Taniguchi, M. (2015). Sensitization to fungal allergens: resolved and unresolved issues. Allergol. Int. 64, 321–331. doi: 10.1016/j.alit.2015.05.007
Geis, P. A., Wheeler, M. H., and Szaniszlo, P. J. (1984). Pentaketide metabolites of melanin synthesis in the dematiaceous fungus Wangiella dermatitidis. Arch. Microbiol. 137, 324–328. doi: 10.1007/bf00410729
Gow, N. A. R., Latgé, J. P., and Munro, C. A. (2017). The fungal cell wall: structure, biosynthesis, and function. Microbiol. Spectr. 5:3.
Heinekamp, T., Thywißen, A., Macheleidt, J., Keller, S., Valiante, V., and Brakhage, A. A. (2013). Aspergillus fumigatus melanins: interference with the host endocytosis pathway and impact on virulence. Front. Microbiol. 3:440. doi: 10.3389/fmicb.2012.00440
Jain, R., Valiante, V., Remme, N., Docimo, T., Heinekamp, T., Hertweck, C., et al. (2011). The MAP kinase MpkA controls cell wall integrity, oxidative stress response, gliotoxin production and iron adaptation in Aspergillus fumigatus. Mol. Microbiol. 82, 39–53. doi: 10.1111/j.1365-2958.2011.07778.x
Kahn, J. N., Hsu, M. J., Racine, F., Giacobbe, R., and Motyl, M. (2006). Caspofungin susceptibility in Aspergillus and non-Aspergillus molds: inhibition of glucan synthase and reduction of beta-D-1,3 glucan levels in culture. Antimicrob. Agents Chemother. 50, 2214–2216. doi: 10.1128/aac.01610-05
Kapteyn, J. C., Hoyer, L. L., Hecht, J. E., Muller, W. H., Andel, A., and Verkleij, A. J. (2000). The cell wall architecture of Candida albicans wild-type cells and cell wall-defective mutants. Mol. Microbiol. 35, 601–611. doi: 10.1046/j.1365-2958.2000.01729.x
Kawamura, C., Tsujimoto, T., and Tsuge, T. (1999). Targeted disruption of a melanin biosynthesis gene affects conidial development and UV tolerance in the Japanese pear pathotype of Alternaria alternata. Mol. Plant Microbe Interact. 12, 59–63. doi: 10.1094/mpmi.1999.12.1.59
Keller, S., Macheleidt, J., Scherlach, K., Schmaler-Ripcke, J., Jacobsen, I. D., Heinekamp, T., et al. (2011). Pyomelanin formation in Aspergillus fumigatus requires HmgX and the transcriptional activator HmgR but is dispensable for virulence. PLoS One 6:e26604. doi: 10.1371/journal.pone.0026604
Kimura, N., and Tsuge, T. (1993). Gene cluster involved in melanin biosynthesis of the filamentous fungus Alternaria alternata. J. Bacteriol. 175, 4427–4435. doi: 10.1128/jb.175.14.4427-4435.1993
Knutsen, A. P., Bush, R. K., Demain, J. G., Denning, D. W., Dixit, A., Fairs, A., et al. (2012). Fungi and allergic lower respiratory tract diseases. J. Allergy Clin. Immunol. 129, 280–291.
Kotob, S. I., Coon, S. L., Quintero, E. J., and Weiner, R. M. (1995). Homogentisic acid is the primary precursor of melanin synthesis in Vibrio cholerae, a Hyphomonas strain, and Shewanella colwelliana. Appl. Environ. Microbiol. 61, 1620–1622. doi: 10.1128/aem.61.4.1620-1622.1995
Lee, J.-K., Jung, H.-M., and Kim, S.-Y. (2003). 1,8-Dihydroxynaphthalene (DHN)-melanin biosynthesis inhibitors increase erythritol production in Torula corallina, and DHN-melanin inhibits erythrose reductase. Appl. Environ. Microbiol. 69, 3427–3434. doi: 10.1128/aem.69.6.3427-3434.2003
Lehninger, A. L. (ed.). (1975). “Oxidative degradation of amino acids,” in Biochemistry (New York, N.Y: Worth Publishers), 568–570.
Lenardon, M. D., Munro, C. A., and Gow, N. A. (2010). Chitin synthesis and fungal pathogenesis. Curr. Opin. Microbiol. 13, 416–423. doi: 10.1016/j.mib.2010.05.002
Livak, K. J., and Schmittgen, T. D. (2001). Analysis of relative gene expression data using real-time quantitative PCR and the 2(-Delta Delta C(T)) method. Methods 25, 402–408. doi: 10.1006/meth.2001.1262
Mitchell, J. J. (2013). Phenylalanine Hydroxylase Deficiency GeneReviews, eds R. A. Pagon, M. P. Adam, H. H. Ardinger, et al. (Seattle, WA: University of Washington), 1993–2016.
Morton, C. O., Varga, J. J., Hornbach, A., Mezger, M., Sennefelder, H., Kneitz, S., et al. (2011). The temporal dynamics of differential gene expression in Aspergillus fumigatus interacting with human immature dendritic cells in vitro. PLoS One. 6:e16016. doi: 10.1371/journal.pone.0016016
Munro, C. A., Selvaggini, S., de Bruijn, I., Walker, L., Lenardon, M. D., Gerssen, B., et al. (2007). The PKC, HOG and Ca2+ signalling pathways co-ordinately regulate chitin synthesis in Candida albicans. Mol. Microbiol. 63, 1399–1413. doi: 10.1111/j.1365-2958.2007.05588.x
Rambach, G., Blum, G., Latgé, J. P., Fontaine, T., Heinekamp, T., Hagleitner, M., et al. (2015). Identification of Aspergillus fumigatus surface components that mediate interaction of conidia and hyphae with human platelets. J. Infect. Dis. 212, 1140–1149. doi: 10.1093/infdis/jiv191
Rodríguez, J. M., Timm, D. E., Titus, G. P., Beltrán-Valero De Bernabé, D., Criado, O., Mueller, H. A., et al. (2000). Structural and functional analysis of mutations in alkaptonuria. Hum. Mol. Genet. 9, 2341–2350. doi: 10.1093/oxfordjournals.hmg.a018927
Rodríguez-Rojas, A., Mena, A., Martín, S., Borrell, N., Oliver, A., and Blázquez, J. (2009). Inactivation of the hmgA gene of Pseudomonas aeruginosa leads to pyomelanin hyperproduction, stress resistance and increased persistence in chronic lung infection. Microbiol. 155, 1050–1057. doi: 10.1099/mic.0.024745-0
Rosas, A. L., Nosanchuk, J. D., Gómez, B. L., Edens, W. A., Henson, J. M., and Casadevall, A. (2000). Isolation and serological analyses of fungal melanins. J. Immunol. Methods 244, 69–80. doi: 10.1016/s0022-1759(00)00255-6
Ruzafa, C., Sanchez-Amat, A., and Solano, F. (1995). Characterization of the melanogenic system in Vibrio cholerae ATCC 14035. Pigment Cell Res. 8, 147–152. doi: 10.1111/j.1600-0749.1995.tb00656.x
Ruzafa, C., Solano, F., and Sanchez-Amat, A. (1994). The protein encoded by the Shewanella colwelliana melA gene is p-hydroxyphenylpyruvate dioxygenase. FEMS Microbiol. Lett. 124, 179–184. doi: 10.1111/j.1574-6968.1994.tb07282.x
Schmaler-Ripcke, J., Sugareva, V., Gebhardt, P., Winkler, R., Kniemeyer, O., Heinekamp, T., et al. (2009). Production of pyomelanin, a second type of melanin, via the tyrosine degradation pathway in Aspergillus fumigatus. Appl. Environ. Microbiol. 75, 493–503. doi: 10.1128/aem.02077-08
Snelgrove, R. J., Gregory, L. G., Peiró, T., Akthar, S., Campbell, G. A., Walker, S. A., et al. (2014). Alternaria-derived serine protease activity drives IL-33-mediated asthma exacerbations. J. Allergy Clin. Immunol. 134, 583–592. doi: 10.1016/j.jaci.2014.02.002
Stappers, M., Clark, A. E., Aimanianda, V., Bidula, S., Reid, D. M., Asamaphan, P., et al. (2018). Recognition of DHN-melanin by a C-type lectin receptor is required for immunity to Aspergillus. Nature 555, 382–386.
Tseng, M. N., Chung, P. C., and Tzean, S. S. (2011). Enhancing the stress tolerance and virulence of an entomopathogen by metabolic engineering of dihydroxynaphthalene melanin biosynthesis genes. Appl. Environ. Microbiol. 77, 4508–4519. doi: 10.1128/aem.02033-10
Tsuji, G., Kenmochi, Y., Takano, Y., Sweigard, J., Farrall, L., Furusawa, I., et al. (2000). Novel fungal transcriptional activators, Cmr1p of Colletotrichum lagenarium and pig1p of Magnaporthe grisea, contain Cys2His2 zinc finger and Zn(II)2Cys6 binuclear cluster DNA-binding motifs and regulate transcription of melanin biosynthesis genes in a developmentally specific manner. Mol. Microbiol. 38, 940–954. doi: 10.1046/j.1365-2958.2000.02181.x
Turick, C. E., Knox, A. S., Becnel, J. M., Ekechukwu, A. A., and Milliken, C. E. (2010). “Properties and function of pyomelanin,” in Biopolymers, ed. M. Elnashar (London: InTech).
Valiante, V., Jain, R., Heinekamp, T., and Brakhage, A. A. (2009). The MpkA MAP kinase module regulates cell wall integrity signaling and pyomelanin formation in Aspergillus fumigatus. Fungal Genet. Biol. 46, 909–918. doi: 10.1016/j.fgb.2009.08.005
Walker, C. A., Gómez, B. L., Mora-Montes, H. M., Mackenzie, K. S., Munro, C. A., Brown, A. J. P., et al. (2010). Melanin externalization in Candida albicans depends on cell wall chitin structures. Eukaryot. Cell 9, 1329–1342. doi: 10.1128/ec.00051-10
Wheeler, M. H., and Klich, M. A. (1995). The effects of tricyclazole, pyroquilon, phthalide, and related fungicides on the production of conidial wall pigments by Penicillium and Aspergillus species. Pestic. Biochem. Phys. 52, 125–136. doi: 10.1006/pest.1995.1037
Keywords: pyomelanin, DHN-melanin, Alternaria alternata, chitin, L-tyrosine, L-phenylalanine, melanin
Citation: Fernandes C, Mota M, Barros L, Dias MI, Ferreira ICFR, Piedade AP, Casadevall A and Gonçalves T (2021) Pyomelanin Synthesis in Alternaria alternata Inhibits DHN-Melanin Synthesis and Decreases Cell Wall Chitin Content and Thickness. Front. Microbiol. 12:691433. doi: 10.3389/fmicb.2021.691433
Received: 06 April 2021; Accepted: 26 July 2021;
Published: 27 August 2021.
Edited by:
Agostinho Carvalho, University of Minho, PortugalReviewed by:
Lea Atanasova, University of Natural Resources and Life Sciences Vienna, AustriaThorsten Heinekamp, Leibniz Institute for Natural Product Research and Infection Biology, Germany
Hans de Cock, Utrecht University, Netherlands
Copyright © 2021 Fernandes, Mota, Barros, Dias, Ferreira, Piedade, Casadevall and Gonçalves. This is an open-access article distributed under the terms of the Creative Commons Attribution License (CC BY). The use, distribution or reproduction in other forums is permitted, provided the original author(s) and the copyright owner(s) are credited and that the original publication in this journal is cited, in accordance with accepted academic practice. No use, distribution or reproduction is permitted which does not comply with these terms.
*Correspondence: Teresa Gonçalves, dG1mb2dAY2kudWMucHQ=; dG1mb2dvbmNhbHZlc0BnbWFpbC5jb20=