- 1Institute of Marine Sciences, National Research Council, Rome, Italy
- 2Marine Biogeochemistry, GEOMAR Helmholtz Centre for Ocean Research, Kiel, Germany
The ability of marine diazotrophs to fix dinitrogen gas (N2) is one of the most influential yet enigmatic processes in the ocean. With their activity diazotrophs support biological production by fixing about 100–200 Tg N/year and turning otherwise unavailable dinitrogen into bioavailable nitrogen (N), an essential limiting nutrient. Despite their important role, the factors that control the distribution of diazotrophs and their ability to fix N2 are not fully elucidated. We discuss insights that can be gained from the emerging picture of a wide geographical distribution of marine diazotrophs and provide a critical assessment of environmental (bottom-up) versus trophic (top-down) controls. We expand a simplified theoretical framework to understand how top-down control affects competition for resources that determine ecological niches. Selective mortality, mediated by grazing or viral-lysis, on non-fixing phytoplankton is identified as a critical process that can broaden the ability of diazotrophs to compete for resources in top-down controlled systems and explain an expanded ecological niche for diazotrophs. Our simplified analysis predicts a larger importance of top-down control on competition patterns as resource levels increase. As grazing controls the faster growing phytoplankton, coexistence of the slower growing diazotrophs can be established. However, these predictions require corroboration by experimental and field data, together with the identification of specific traits of organisms and associated trade-offs related to selective top-down control. Elucidation of these factors could greatly improve our predictive capability for patterns and rates of marine N2 fixation. The susceptibility of this key biogeochemical process to future changes may not only be determined by changes in environmental conditions but also via changes in the ecological interactions.
Introduction
Biological N2 fixation has evolved early in Earth’s history (Falkowski, 1997), when the ocean was void of oxygen (O2) and fixed N but rich in dissolved iron (Fe2+). The appearance of this process marked the beginning of the modern ocean about 2.5 billion years ago (Canfield et al., 2010). It is thus not surprising that the nitrogenase enzyme complex, which catalyzes the energy-demanding reduction of the inert N2 to NH4+ (Postgate, 1982), functions only under strictly anaerobic conditions and has an elevated Fe2+ requirement (Kustka et al., 2003). As aerobic N2 fixers evolved and ocean chemistry changed, diazotrophs developed numerous strategies to protect nitrogenase from O2, including elevated respiration rates and temporal or spatial separation of oxygenic photosynthesis from N2 fixation (Berman-Frank, 2001).
The ability to fix N2 is associated with additional energetic costs that are generally understood to yield lower growth rates of N2 fixing phytoplankton as compared to their non-N2 fixing phytoplankton competitors. Key costs are the breaking of the triple bond and reduction of N2 to NH4+, and include the indirect costs required to maintain a functioning nitrogenase complex, e.g., an anaerobic intracellular environment (Großkopf and La Roche, 2012). While diazotrophs are generally considered facultative (Zehr and Capone, 2020), nitrate utilization seems to be beneficial only at rather high concentrations (above 7 μmol L–1, Holl and Montoya, 2005; Pahlow et al., 2013). We restrict our analysis here to conditions where diazotrophs fix N2. The slow-growth assumption has long guided our understanding of the competitive ability of diazotrophs (Redfield et al., 1963). It has been the basic tenet of resource competition theory (RCT) to explain coexistence patterns in idealized systems (Tilman, 1980), to understand N-inventory regulatory mechanisms on long time scales (Tyrrell, 1999; Gruber, 2004), and to simulate diazotroph activity in state-of-the-art global biogeochemical models (Ward et al., 2013; Dutkiewicz et al., 2014; Landolfi et al., 2015, 2017, 2018; Wang et al., 2019; Pahlow et al., 2020). Elevated growth rates have been reported recently for several diazotrophic species (Turk-Kubo et al., 2018), further questioning our conceptual understanding of diazotrophy. Nevertheless, the competitive advantage of N2 fixation in slowly growing autotrophic diazotrophs with elevated Fe-requirements should be restricted to N-limited and/or Fe-replete regions, leading to the traditional resource or “bottom-up” control paradigm of their ecological niche. This bottom-up control of autotrophic diazotrophs can be visualized using the simplified RCT graphical approach with nitrogen (N) and phosphorus (P) as limiting resources, in the absence of grazing pressure (Figure 1A).
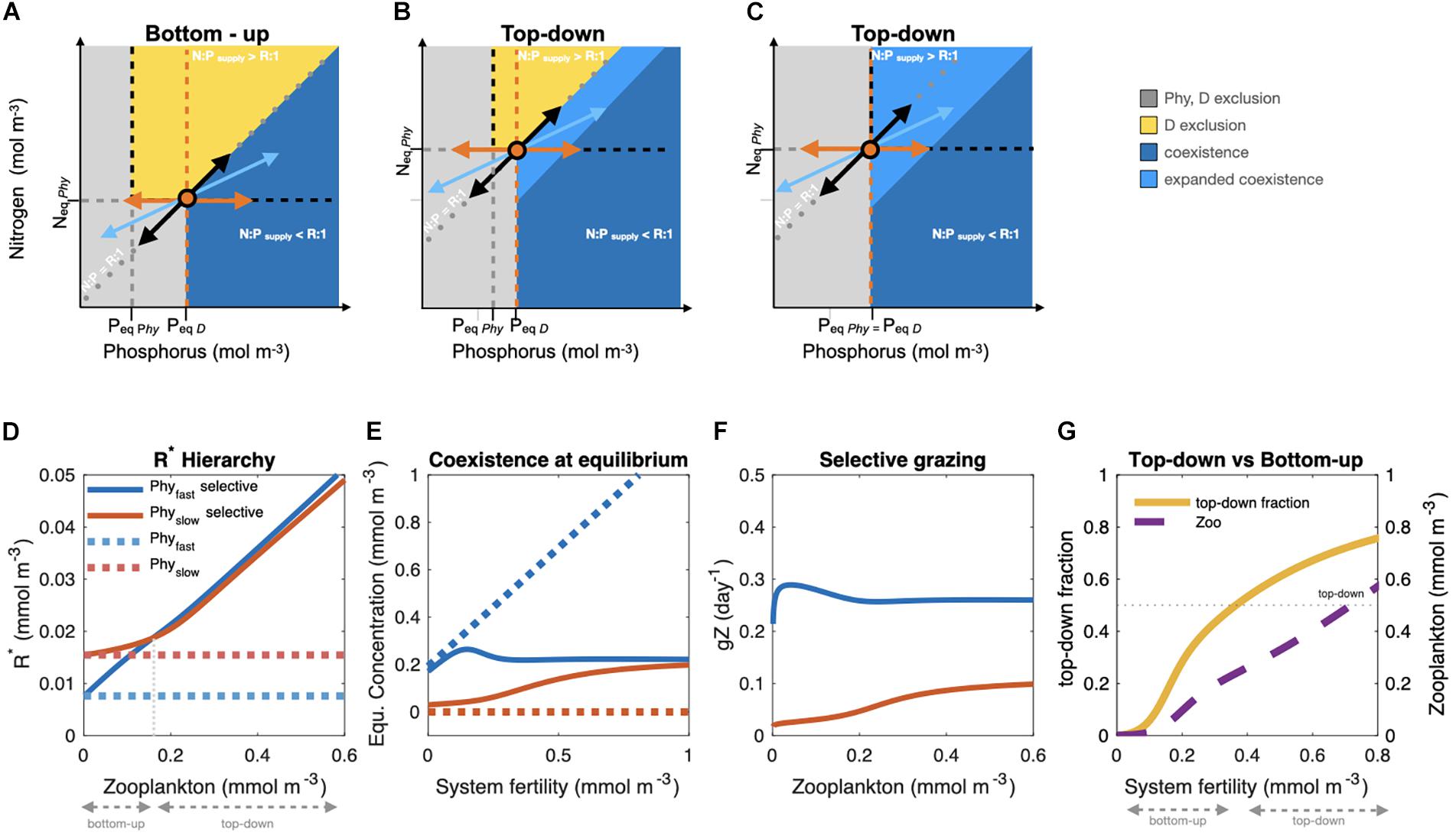
Figure 1. (A) Traditional RCT graphical approach (considering equal mortality and nutrient requirements independent from one another). In an idealized system, phytoplankton (Phy, black) and diazotrophs (D, orange) competing for nitrogen, N, and phosphorus, P, draw down nutrient concentrations to their individual equilibrium nutrient requirements (R*), here Neq and Peq, reaching steady state (growth = mortality) on the zero net growth isoclines (ZNGI, black, and orange dashed lines). For nutrient supply below Neq, Peq both algae cannot grow (gray region, mortality > growth). Phy consume nutrients (black arrow pointing toward axis origin) in their cellular proportions of R. D consume P only (orange arrow pointing left) and supply extra N. At steady state, total N and P consumption (blue vectors pointing toward low N and P) must be balanced by the nutrient supply (blue vectors pointing toward high N and P). Stable coexistence of Phy and D occurs at the interception of the ZNGIs where the growth of Phy is limited by N and that of D is limited by P. This occurs when N:Psupply < R, i.e., when the resource limiting the slower-growing phytoplankton is supplied in excess. Note that the region of coexistence extends all the way to the x axis (0 N supply) because the N added by D can be utilized by Phy. (B) R* depends on physiological characteristics and grazing pressure (gZ; Eq. 1). Accounting for selective grazing on Phy (gZPhy > gZD) can increase the nutrient requirements Neq and Peq of the faster-growing competitor, thereby expanding the region of coexistence. (C) Should PeqPhy = PeqD Phy becomes limited by P also for N:Psupply > R, allowing D to coexist in high N supply regions (see Supplementary Material for analytical derivation). (D) R* hierarchy: RCT predicts that in competition for the same resource, the species with the lowest R* (mmol m– 3) will dominate. Fast growing species (blue dashed line) dominate slowly growing ones (red dashed line) in the absence of grazing (gZ = 0), or selective grazing (gZfast = gZslow). The inversion of the R* hierarchy occurs with selective grazing on the fastest growing organism (gZfast > gZslow, solid lines). (E) Coexistence at equilibrium as a function of ecosystem fertility (nutrient initial conditions) for the fast (blue) and slow (red) phytoplankton in the absence of grazing or selective grazing (dashed lines) and with selective grazing (gZfast > gZslow, solid lines). (F) Grazing rates (giZ, day– 1) on the fast and slow phytoplankton as a function of zooplankton biomass (Z, mmol m– 3). (G) Top-down versus bottom-up control on R* as a function of system fertility. Top-down fraction (tdf) calculated as: , where for each ith species for giZ = 0, i.e., for pure bottom-up control, and n is the number of species. Plots (D–G) are based on equilibrium solutions of a 100-member ensemble of a 0D ecosystem model [nutrients (N), fast-growing (Phyfast) and slow-growing phytoplankton (Physlow), and zooplankton (Z)] with different initial nutrient levels. Phyfast and Physlow differ only in their maximum growth rate (μmax, 1.3 and 0.65 day–1, respectively) and palatability (∅i,0.7 and 0.3, respectively). All other ecosystem model parameters are equal (half saturation constant for nutrient uptake k = 0.5 mmol m–3; linear mortality m = 0.03 day–1; maximum grazing rate gmax = 0.4day–1; and prey half saturation constant kz = 0.5 mmol m–3). The grazing rate per unit biomass gi (m3 mmol–1day–1) is of the form: .
Novel emerging data suggest that diazotrophs are distributed far more widely than the bottom-up control by N, Fe, and P predicts, spanning a wide range of ocean environments from surface to deep sea (Benavides et al., 2018) and hydrothermal vents (Mehta and Baross, 2006), from warm tropical to cold polar regions (Moisander et al., 2017; Shiozaki et al., 2017; Harding et al., 2018; Mulholland et al., 2019), from permanently oligotrophic waters of the subtropical gyres to high-NO3– oceanic and coastal waters (Knapp, 2012; Turk-Kubo et al., 2018) to aphotic oxygen minimum zones (Löscher et al., 2014) and hypoxic basins (Hamersley et al., 2011; Farnelid et al., 2013). These oceanic environments are populated by genetically and morphologically diverse diazotrophs reflecting a large ecological diversity, from unicellular (Zehr et al., 1998) free-living (UCYN-B) and symbiont species (UCYN-A, UCYN-C) and diazotroph-diatom associations, DDA (Foster et al., 2011) to multicellular species with heterocysts (Nodularia and Aphanizomenon) and colonial forms (Trichodesmium), including also non-cyanobacterial diazotrophs (Riemann et al., 2010; Moisander et al., 2017). Can recent observations be reconciled with theoretical predictions?
Laboratory experiments (Knapp, 2012) as well as RCT analyses and results from numerical models of different complexity (Pahlow et al., 2013; Landolfi et al., 2015; Inomura et al., 2018) collectively suggest that autotrophic diazotrophs can still coexist with non-N2 fixing phytoplankton where fixed forms of N (e.g., NO3–, NH4+, and NH3) are available, which may be due to a combination of co-limitation by N and P (and possibly Fe or other micro-nutrients), greater N requirements for nutrient acquisition (Klausmeier et al., 2004) and the high competitive ability of diazotrophs for P (Pahlow et al., 2013). In models, with explicit representation of physiologically costly nutrient acquisition strategies, coexistence among organisms that compete for limited resources occurs for a wide range of nutrient-supply conditions (Pahlow et al., 2013, 2020; Landolfi et al., 2015; Inomura et al., 2018; Chien et al., 2020). Insight from these recent works highlights the key role of competitive interactions in setting the ecological niche of diazotrophs.
There is a growing understanding of how environmental factors affecting diazotroph physiology, with regard to temperature optima (Moisander et al., 2010), Fe requirements (Saito et al., 2011), NO tolerance (Knapp, 2012; Inomura et al., 2018), O2 inhibition (Stal, 2009), and competition for P (Pahlow et al., 2013; Landolfi et al., 2015), shape competition patters. There is still little knowledge, however, of how ecological interactions, such as selective mortality by zooplankton grazing and/or viral-lysis, modulate the ability of diazotrophs to compete for resources in relation to their main competitors and thereby influence their ecological niche.
Top-Down Control Can Expand the Ecological Niche of Diazotrophs
Top-down control by grazers (Prowe et al., 2012a; Vallina et al., 2014) and mortality by viral infection (Suttle, 2007; Weitz et al., 2015) are thought to exert a major control on plankton diversity and coexistence, driving adaptation and evolution. For example, grazing can drive changes in cell size and morphology (Fenchel, 1980; Branco et al., 2020) as well as defense mechanisms (Lürling, 2021). Selective mortality, via grazing or viral-lysis, leads to increased diversity and coexistence (Thingstad, 2000), contributing, together with the spatial and temporal heterogeneity of the environment, to maintain plankton diversity (Hutchinson, 1961) on seasonal to centennial time scales (Barton et al., 2010; Tsakalakis et al., 2018; Dutkiewicz et al., 2020).
To better understand how selective mortality affects phytoplankton competition for resources and coexistence patterns, we use a simple model with one nutrient (N), a fast-growing and a slowly growing phytoplankton (Phyfast and Physlow) and one zooplankton (Z), and interpret our results within the RCT theoretical framework. The RCT predicts the outcome of competition and co-existence of autotrophic phytoplankton based on the R∗ hierarchy. R∗ is the resource concentration required by each phytoplankton type to reach equilibrium growth (growth = mortality). At equilibrium, each phytoplankton type draws down the ambient nutrient concentrations to its own equilibrium requirements R∗. It follows that different phytoplankton types can coexist only if each has at least one resource for which its R∗ is less than that of all others. The R∗ hierarchy depends on the combinations of physiological characteristics (maximal growth rate, μmax, and half saturation constants, k) and mortality terms [specific mortality m and grazing gZ, where g is the grazing rate per unit biomass (m3 mmol–1 day–1) and Z (mmol m–3) is zooplankton biomass] of each phytoplankton type, as expressed by Eq. 1 (see appendix for derivation).
Traditionally, RCT considers the simplest case of similar mortality terms for all phytoplankton types, assuming constant specific mortality m and negligible effects of grazing (gZ = 0; Tilman, 1980). Under these simplified assumptions, the phytoplankton with the largest maximal growth rate (μmax; or lowest nutrient half saturation constant k) will have the lowest resource requirement R∗ at equilibrium (Figure 1D, dashed blue line), and will be the superior competitor, out-competing the slower-growing competitor (Figures 1D,E, dashed red line; Tilman, 1980). Recognizing that mortality terms may differ among phytoplankton and thereby relaxing the original RCT assumption of negligible grazing effects, we find that differential mortality, in our example mediated by selective top-down processes, but which could also include viral-mediated processes, can allow coexistence for a wider range of conditions. If zooplankton graze equally on two phytoplankton types (i.e., gZfast = gZslow) the more slowly growing one will be competitively excluded as in the simplified gZfast = 0 case (Figure 1E, dashed lines). However, selective grazing on fast-growing phytoplankton (i.e., gZfast > gZslow, Figure 1F), can change the hierarchy of R∗ (Figure 1D, solid lines). This allows the inferior competitor, otherwise out-competed in the absence of selective grazing, to survive and coexist (Figure 1E, compare red lines) in top-down controlled systems (Prowe et al., 2012b; analytical derivation in Supplementary Material). The relative importance of top-down control on R∗ is not fixed but varies, increasing with resource levels, or “fertility” of the system, as a larger zooplankton biomass can be supported (Figure 1G). With selective grazing, the effect of top-down control on R∗ increases with system “fertility.” It should be noted that physiologically costly defense strategies (e.g., morphological and size changes, toxicity, etc.) have associated trade-offs, implying a reduction of the maximum growth rate that would affect R∗ (Eq. 1) and the resulting R∗ hierarchy. The extended RCT framework illustrates that selective top-down control can prevent the fastest-growing organism from exploiting all of the limiting resource, expanding the niche of slower-growing species in top-down controlled regions, effectively providing for novel coexistence regimes compared to bottom-up control and non-selective mortality. Although simple, the RCT principles can help interpret phytoplankton biogeographies emerging in complex global ecological-biogeochemical models that include multiple limiting nutrients, complex grazing functions and loss terms, and circulation and mixing (Dutkiewicz et al., 2009; Ward et al., 2012, 2014).
In the specific case of autotrophic diazotrophs (D) competing with faster-growing non-fixing phytoplankton (Phy), we now extend the traditional bottom-up paradigm within the RCT graphical framework (Figure 1A) to include selective grazing on Phy (Figures 1B,C). This effectively expands the diazotrophs’ region of co-existence by increasing the minimum nutrient requirements, Neq and Peq, of Phy (Figures 1B,C). Also, when accounting for interdependent N and P requirements (Sterner and Elser, 2002) as in chain models (e.g., Pahlow et al., 2013), selective grazing on Phy enlarges the niche of diazotrophs relative to bottom-up control only (Supplementary Figure 2). While competition for resources (P and Fe) has been central for explaining the diazotrophs’ spatiotemporal distribution in models (Landolfi et al., 2013, 2015, 2017; Ward et al., 2013; Dutkiewicz et al., 2014), the role of top-down control in modulating the space of diazotroph-non-diazotroph coexistence remains insufficiently explored (Wang et al., 2019). Our expanded resource-competition analysis suggests that physiological characteristics determine competitive outcomes in bottom-up controlled (nutrient scarce) environments, but as environmental resource levels increase, competition patterns become modulated by selective mortality. This can expand the ecological niche of autotrophic diazotrophs. A more comprehensive mechanistic understanding of the links between phytoplankton traits, environmental factors and ecological interactions (competition, predation, defense strategies, and mortality) is required.
Do We Know How Diazotrophs Die?
Zooplankton grazing is considered the predominant phytoplankton mortality in the ocean (Landry and Calbet, 2004), whereas virus-mediated mortality contributes less than 10% on average (Brussaard, 2004). At low latitudes, virus-induced mortality appears to be more prevalent than at higher latitudes (Mojica et al., 2016). Under environmental stress and/or viral attack, an autocatalytic programmed cell death (PCD) has been observed in many phytoplankton species, including diazotrophs (Bidle, 2016). However, the fate of diazotroph biomass across the food web is poorly understood (Mulholland, 2007) and little is known about diazotroph mortality due to grazing, viral-lysis, or PCD and their relative importance. Trichodesmium is generally regarded as having low palatability for grazers (Capone, 1997), yet whether this is because of poor nutritional quality, chemical defense (toxin production), or morphological characteristics, remains unclear. Virus-mediated mortality (Hewson et al., 2004) and PCD (Berman-Frank et al., 2004, 2007) have been described as significant loss processes for this diazotroph. However, the major loss mechanisms of other diazotrophs are poorly known. Which diazotrophs (unicellular, colonial, and symbiont) are hosts for viruses, which are grazed by which size classes of grazers, micro- (<200 μm) or meso-zooplankton (0.2–20 mm), by which strategy (passive or active feeding), and at what rates is mostly unresolved. Potential traits, associated with defense strategies (morphological, physiological, and behavioral) are hardly identified and their physiological costs (trade-offs) remain mostly uncharacterized. In the following we provide a tentative synthesis of the literature reporting grazing on diazotrophs as well as a list of potential traits that could affect selective feeding (Table 1). The lack of knowledge on grazer identity, grazing rates, and traits associated with top-down processes currently limits our deterministic power in numerical models.
Evidence of Defense Traits and Selective Top-Down Control Against Diazotrophs?
Selective top-down control can depend on prey abundance (Jürgens and DeMott, 1995; Boenigk et al., 2002), size and morphology (Armstrong, 1994), and nutritional quality (Schultz and Kiørboe, 2009), but can also be due to the host specificity of viruses. Defense strategies can be induced to reduce grazing and/or viral attack. For example, defense against grazing can include morphological and physiological traits (such as resting stages, motility), although the associated trade-offs, e.g., enhanced metabolic costs are not identified yet (Pančić and Kiørboe, 2018) and overall effects on the community level are unclear. Grazing experiments on diazotrophs are very limited (Table 1). The few observations suggest a high variability of taxon-specific grazing interactions (e.g., Wannicke et al., 2013; Deng et al., 2020) with mixed evidence of zooplankton selectivity for/against diazotrophs, based on size and morphology, extracellular environment (e.g., DOM release), nutritional quality and toxicity.
Size and Morphology
Size and morphology are regarded among the most important factors affecting prey selectivity (Armstrong, 1994). Zooplankton specific ingestion rates generally decrease with size of the predator (Kiørboe and Hirst, 2014), reflecting an increase in prey handling time with large sizes and complex morphologies (Wirtz, 2012). Size and morphological changes can be induced by grazing pressure and are achieved, e.g., via the formation of colonies and aggregates in phytoplankton, likely at the cost of reduced growth rates (Van Donk et al., 2011; Lürling, 2021).
In diazotrophs size, morphology, aggregation, and colony formation may thus also affect susceptibility to grazing pressure and potentially growth rates. The size spectrum of diazotrophs covers a wide range from 1 μm diameter (UCYN-A) up to Trichodesmium filaments of about 550 μm (LaRoche and Breitbarth, 2005; Goebel et al., 2008). Unicellular diazotrophs are part of the diet of heterotrophic and mixotrophic protists (microzooplankton; Turk-Kubo et al., 2018; Deng et al., 2020; Dugenne et al., 2020; Table 1). While large aggregates, symbionts of large organisms (>40 μm), and colonies may be protected against grazing, selective grazing on UCYN-A (Scavotto et al., 2015; Turk-Kubo et al., 2018) and UCYN-B (Scavotto et al., 2015; Conroy et al., 2017; Wilson et al., 2017; Dugenne et al., 2020) and Trichodesmium (Wilson and Steinberg, 2010), as part of symbionts and/or aggregates has been inferred. This suggests that aggregation and/or symbiosis may not be a deterrent for mesozooplankton grazers (Wilson and Steinberg, 2010; Hunt et al., 2016; Bonnet et al., 2018). However, given that the presence of nifH genes in the gut content may not unambiguously indicate direct ingestion of the diazotroph or its aggregate, conclusive evidence for direct ingestion of aggregates is scarce. In filamentous freshwater cyanobacteria, aggregation and size changes induced by the presence of grazers have been described (Cerbin et al., 2013). Triggers and costs of Trichodesmium colony formation and aggregation into parallel (tufts) or radial (puffs) trichomes, with presumedly different exposures to grazing, remain speculative.
The production of dissolved organic matter has been suggested to affect the size distribution, sinking speed, and accessibility to potential consumers, acting both as grazing deterrent (Liu and Buskey, 2000) or by making small cells available to larger consumers via aggregation (Passow, 2002). In Trichodesmium, extracellular C release has been documented and ascribed to buoyancy regulation (Villareal and Carpenter, 2003). During PCD extracellular carbohydrate release can promote the formation of sticky, fast-sinking aggregates (Bar-Zeev et al., 2013). Extracellular carbohydrates release has been observed also in Crocosphaera when forming aggregates (Sohm et al., 2011). The relevance of this process for reducing/increasing zooplankton grazing rates on diazotrophs is an open question that requires further elucidation.
Nutritional Quality
The elemental composition of phytoplankton is characterized by high plasticity compared to that of grazers (Sterner and Elser, 2002). The ingestion of nutrient deficient phytoplankton (high C:N, C:P ratios) can result in compensatory or selective feeding in zooplankton. Compensatory feeding may lead to increased ingestion of prey (to fulfill the nutrient requirement Ng et al., 2017), whereas selective feeding reduces the ingestion of prey (due to lower growth rates of grazers; Mitra and Flynn, 2006). Selection by nutritional quality may require metabolically costly and sophisticated pre-ingestion sensory abilities, as observed in marine copepods (Meunier et al., 2016). Low fatty acid content has led freshwater cyanobacteria to be considered as being of low nutritional value (Müller-Navarra et al., 2000). The range of molar C:N ratios in diazotrophs (Trichodesmium C:N = 4.7–8.6, LaRoche and Breitbarth, 2005; White et al., 2006; Crocosphaera C:N = 5.9–11.4, Tuit et al., 2004), are within the range of other phytoplankton (C:N = 5.23–9.44, Garcia et al., 2018). However, high nutritional quality in UCYN-B (Crocosphaera) at night (day – night C:N range = 10–6.9), driven by a light-dark N2-fixation pattern, has been suggested to promote night-time selective grazing by microplanktonic protists (Deng et al., 2020).
Toxicity
Toxin production can be induced by the presence of grazers, and lead to several adverse effects on zooplankton feeding on toxic species as a sole diet, such as reduced survival, egg production or hatching success, or induce selection against toxic species in a mixed diet (Schultz and Kiørboe, 2009; Ger et al., 2019), fostering proliferation of toxic blooms (Turner, 2014). Anabaena (Dolichospermum), Nodularia, Aphanizomenon, and Trichodesmium are among the toxin producing diazotrophs (Huisman et al., 2018). Early studies found that aging Trichodesmium (Hawser et al., 1992; Guo and Tester, 1994) and Nodularia spumigena (Sellner et al., 1994, 1996; Engstrom, 2000; Lundgren et al., 2012) were toxic to calanoid copepods. Hence, Trichodesmium has been considered as being of low palatability for most zooplankton (O’Neil and Roman, 1992), although the harpacticoid copepod Macrosetella gracilis, relies on Trichodesmium as a food source (O’Neil, 1998). Neutral or positive effects have been found also for zooplankton feeding on Nodularia spumigena (Hogfors et al., 2014). Some copepods show compensatory feeding (high grazing rates) on Nodularia (Kozlowsky-Suzuki et al., 2003). Adaptive strategies to overcome toxicity (Dupuy et al., 2016) may explain the mixed evidence of diazotroph toxicity for zooplankton.
Model Parameterizations and Implications for Coexistence
In models a major control on coexistence of different phytoplankton functional types is exerted by the functional response that describes how predator’s ingestion depends on prey concentration (Prowe, 2012; Vallina et al., 2014). A multitude of functional responses exists that cover diverse predator-prey interactions driven by encounter, escape, selection, handling, ingestion, and digestion processes, governed by prey and predator traits and trade-offs. Three major types of functional responses (I-linear, II-hyperbolic, and III-sigmoidal) are often assumed to describe the feeding rate as function of prey concentration (Holling, 1965). However, these do not fully reproduce grazing responses observed in controlled laboratory experiments and field data (Pahlow and Prowe, 2010). The sigmoidal form (type III) stipulates proportional feeding on the most abundant prey compared to its relative contribution to total food, and may equalize R∗ values (Prowe, 2012), and is therefore often used to promote coexistence (Bates et al., 2016) and prevent extinction when diazotroph biomass is low (Landolfi et al., 2013; Ward et al., 2013). Experimental evidence for the link between feeding behavior and functional response has been found, e.g., for different copepods (Kiørboe et al., 2018). Experimental studies to derive the functional responses of zooplankton feeding strategies on diazotrophs are lacking (Table 1). Recently, a type II response has been obtained by automated imaging of grazing dynamics (Dugenne et al., 2020). In models, selective grazing can result from the combination of density dependent functional responses and phytoplankton type/size specific grazing preferences/palatability choices. Current models generally employ reduced grazing pressure for diazotrophs (selective grazing on non-fixing phytoplankton) resulting from lower palatability, size-selectivity (Dutkiewicz et al., 2020), and/or strong density dependence (e.g., type III, Landolfi et al., 2013). Diazotroph distribution and N2 fixation rates are very sensitive to grazing formulations. Similarly to what RCT predicts, strong selective grazing on non-fixing phytoplankton allows a greater expansion of the diazotrophs’ niche in top-down controlled nutrient rich regions, whereas weak selective grazing constrains the diazotrophs’ niche to bottom-up controlled regions in models with greater complexity in terms of multiple limiting nutrients, trophic interactions, and circulation and mixing (Supplementary Figure 3; Chien et al., 2020). Higher grazing preference for diazotrophs, relative to non-fixing phytoplankton, can result from optimized parameters in global biogeochemical models (Wang et al., 2019; Chien et al., 2020). This suggests that numerous interactions may arise in more complex models, calling for more explicit discussion and further scrutiny of the treatment of grazing formulations.
Conclusion and Future Perspectives
The remarkable variety of growth strategies that allows diazotrophs to flourish in waters ranging from warm oligotrophic regions to cold, nutrient-rich, and highly productive systems prompts the question: What are the underlying advantages of fixing N2, given the associated additional energetic costs? This remarkable and widely distributed ability demands a better understanding of interdependencies between physiology and ecological dynamics (competition and predation) that set the broad ecological niches of diazotrophs. While our comprehension of physiological constraints on diazotrophs and their environmental (bottom-up) sensitivity is growing, the role of selective mortality mediated by top-down processes and/or viral-lysis in shaping their ecological niche is less clear. Relaxing the RCT’s equal mortality assumption, we identify selective mortality of faster-growing competitors as a key process for expanding the niche of autotrophic diazotrophs in nutrient rich regions. However, to date, observational evidence is limited and insufficient to support the occurrence of selective grazing against diazotrophs. Identifying traits and trade-offs associated with selective top-down control (changes in size and morphology, nutritional quality, toxicity, and DOM release) and linking them in a multi-trait perspective remains a fundamental challenge for elucidating the mechanisms that allow the ecological complexity needed for insightful model applications. Whether future environmental and ecological changes will introduce benefits or disadvantages for N2 fixers will depend on how these changes affect the competitive ability of diazotrophs in relation to their main competitors and predators on seasonal to centennial timescales. This suggests that we need to move beyond correlative relationships and instead establish mechanistic links between physiologically costly traits and their function in ecological dynamics. Understanding and resolving these links is key to making ecological complexity and its impact on, and interaction with, marine nitrogen fixation emerge in biogeochemical models and allowing for more reliable predictions of the future ocean.
Data Availability Statement
The original contributions presented in the study are included in the article/Supplementary Material, further inquiries can be directed to the corresponding author/s.
Author Contributions
This is a contribution from the “N2-fixation pathfinder group” at the GEOMAR Biogeochemical Modelling group. AL has written the original draft and provided the resource competition analysis. All the authors have discussed and commented-on various stages of the manuscript.
Funding
This is a contribution to the Collaborative Research Centre SFB 754, funded by the Deutsche Forschungsgemeinschat (DFG).
Conflict of Interest
The authors declare that the research was conducted in the absence of any commercial or financial relationships that could be construed as a potential conflict of interest.
Publisher’s Note
All claims expressed in this article are solely those of the authors and do not necessarily represent those of their affiliated organizations, or those of the publisher, the editors and the reviewers. Any product that may be evaluated in this article, or claim that may be made by its manufacturer, is not guaranteed or endorsed by the publisher.
Acknowledgments
We acknowledge discussions with colleagues from the “1st and 2nd Workshop on Marine N2 fixation” and support by Euromarine.
Supplementary Material
The Supplementary Material for this article can be found online at: https://www.frontiersin.org/articles/10.3389/fmicb.2021.690200/full#supplementary-material
References
Armstrong, R. A. (1994). Grazing limitation and nutrient limitation in marine ecosystems: steady state solutions of an ecosystem model with multiple food chains. Limnol. Oceanogr. 39, 597–608. doi: 10.4319/lo.1994.39.3.0597
Barton, A. D., Dutkiewicz, S., Flierl, G., Bragg, J., and Follows, M. J. (2010). Patterns of diversity in marine phytoplankton. Science 327, 1509–1511.
Bar-Zeev, E., Avishay, I., Bidle, K. D., and Berman-Frank, I. (2013). Programmed cell death in the marine cyanobacterium Trichodesmium mediates carbon and nitrogen export. ISME J. 7, 2340–2348. doi: 10.1038/ismej.2013.121
Bates, M. L., Cropp, R. A., Hawker, D. W., and Norbury, J. (2016). Which functional responses preclude extinction in ecological population-dynamic models? Ecol. Complex. 26, 57–67. doi: 10.1016/j.ecocom.2016.03.003
Benavides, M., Bonnet, S., Berman-Frank, I., and Riemann, L. (2018). Deep into oceanic N2 fixation. Front. Mar. Sci. 5:108. doi: 10.3389/fmars.2018.00108
Berman-Frank, I. (2001). Segregation of nitrogen fixation and oxygenic photosynthesis in the marine cyanobacterium Trichodesmium. Science 294, 1534–1537. doi: 10.1126/science.1064082
Berman-Frank, I., Bidle, K. D., Haramaty, L., and Falkowski, P. G. (2004). The demise of the marine cyanobacterium, Trichodesmium spp., via an autocatalyzed cell death pathway. Limnol. Oceanogr. 49, 997–1005. doi: 10.4319/lo.2004.49.4.0997
Berman-Frank, I., Rosenberg, G., Levitan, O., Haramaty, L., and Mari, X. (2007). Coupling between autocatalytic cell death and transparent exopolymeric particle production in the marine cyanobacterium Trichodesmium. Environ. Microbiol. 9, 1415–1422. doi: 10.1111/j.1462-2920.2007.01257.x
Bidle, K. D. (2016). Programmed cell death in unicellular phytoplankton. Curr. Biol. 26, R594–R607. doi: 10.1016/j.cub.2016.05.056
Boenigk, J., Matz, C., Jürgens, K., and Arndt, H. (2002). Food concentration-dependent regulation of food selectivity of interception-feeding bacterivorous nanoflagellates. Aquat. Microb. Ecol. 27, 195–202. doi: 10.3354/ame027195
Bonnet, S., Baklouti, M., Gimenez, A., Berthelot, H., and Berman-Frank, I. (2016). Biogeochemical and biological impacts of diazotroph blooms in a low-nutrient, low-chlorophyll ecosystem: synthesis from the VAHINE mesocosm experiment (New Caledonia). Biogeosciences 13, 4461–4479. doi: 10.5194/bg-13-4461-2016
Bonnet, S., Caffin, M., Berthelot, H., Grosso, O., Benavides, M., Helias-Nunige, S., et al. (2018). In-depth characterization of diazotroph activity across the western tropical South Pacific hotspot of N2 fixation (OUTPACE cruise). Biogeosciences 15, 4215–4232. doi: 10.5194/bg-15-4215-2018
Branco, P., Egas, M., Hall, S. R., and Huisman, J. (2020). Why do phytoplankton evolve large size in response to grazing? Am. Nat. 195, E20–E37. doi: 10.1086/706251
Brussaard, C. P. D. (2004). Viral control of phytoplankton populations-a review1. J. Eukaryot. Microbiol. 51, 125–138. doi: 10.1111/j.1550-7408.2004.tb00537.x
Canfield, D. E., Glazer, A. N., and Falkowski, P. G. (2010). The evolution and future of Earth’s nitrogen cycle. Science 330, 192–196. doi: 10.1126/science.1186120
Capone, D. G. (1997). Trichodesmium, a globally significant marine cyanobacterium. Science 276, 1221–1229. doi: 10.1126/science.276.5316.1221
Cerbin, L., Slawek, D., and Wejnerowski, M. (2013). View of Aphanizomenon gracile increases in width in the presence of Daphnia. A defence mechanism against grazing? J. Limnol. 72:505.
Chan, F., Marino, R., Howarth, R., and Pace, M. (2006). Ecological constraints on planktonic nitrogen fixation in saline estuaries: II. Grazing controls on cyanobacterial population dynamics. Mar. Ecol. Prog. Ser. 309, 41–53. doi: 10.3354/meps309041
Chien, C.-T., Pahlow, M., Schartau, M., and Oschlies, A. (2020). Optimality-based non-redfield plankton–ecosystem model (OPEM v1.1) in UVic-ESCM 2.9 – part 2: sensitivity analysis and model calibration. Geosci. Model Dev. 13, 4691–4712. doi: 10.5194/gmd-13-4691-2020
Conroy, B. J., Steinberg, D. K., Song, B., Kalmbach, A., Carpenter, E. J., and Foster, R. A. (2017). Mesozooplankton in graze on Cyanobacteria the Amazon River plume and Western tropical North Atlantic. Front. Microbiol. 8:1436. doi: 10.3389/fmicb.2017.01436
Deng, L., Cheung, S., and Liu, H. (2020). Protistal grazers increase grazing on unicellular cyanobacteria diazotroph at night. Front. Mar. Sci. 7:135. doi: 10.3389/fmars.2020.00135
Dugenne, M., Henderikx Freitas, F., Wilson, S. T., Karl, D. M., and White, A. E. (2020). Life and death of Crocosphaera sp. in the Pacific Ocean: fine scale predator–prey dynamics. Limnol. Oceanogr. 65, 2603–2617. doi: 10.1002/lno.11473
Dupuy, C., Pagano, M., Got, P., Domaizon, I., Chappuis, A., Marchessaux, G., et al. (2016). Trophic relationships between metazooplankton communities and their plankton food sources in the Iles Eparses (Western Indian Ocean). Mar. Environ. Res. 116, 18–31. doi: 10.1016/j.marenvres.2016.02.011
Dutkiewicz, S., Cermeno, P., Jahn, O., Follows, M. J., Hickman, A. E., Taniguchi, D. A. A., et al. (2020). Dimensions of marine phytoplankton diversity. Biogeosciences 17, 609–634. doi: 10.5194/bg-17-609-2020
Dutkiewicz, S., Follows, M. J., and Bragg, J. G. (2009). Modeling the coupling of ocean ecology and biogeochemistry: Coupling of Ecology and Biogeochemistry. Glob. Biogeochem. Cycles 23:3268. doi: 10.1029/2008GB003405
Dutkiewicz, S., Ward, B. A., Scott, J. R., and Follows, M. J. (2014). Understanding predicted shifts in diazotroph biogeography using resource competition theory. Biogeosciences 11, 5445–5461. doi: 10.5194/bg-11-5445-2014
Engstrom, J. (2000). Feeding interactions of the copepods Eurytemora affinis and Acartia bifilosa with the cyanobacteria Nodularia sp. J. Plankton Res. 22, 1403–1409. doi: 10.1093/plankt/22.7.1403
Falkowski, P. G. (1997). Evolution of the nitrogen cycle and its influence on the biological sequestration of CO2 in the ocean. Nature 387, 272–275. doi: 10.1038/387272a0
Farnelid, H., Bentzon-Tilia, M., Andersson, A. F., Bertilsson, S., Jost, G., Labrenz, M., et al. (2013). Active nitrogen-fixing heterotrophic bacteria at and below the chemocline of the central Baltic Sea. ISME J. 7, 1413–1423. doi: 10.1038/ismej.2013.26
Fenchel, T. (1980). Relation between particle size selection and clearance in suspension-feeding ciliates. Limnol. Oceanogr. 25, 733–738. doi: 10.4319/lo.1980.25.4.0733
Foster, R. A., Kuypers, M. M. M., Vagner, T., Paerl, R. W., Musat, N., and Zehr, J. P. (2011). Nitrogen fixation and transfer in open ocean diatom–cyanobacterial symbioses. ISME J. 5, 1484–1493. doi: 10.1038/ismej.2011.26
Garcia, N. S., Sexton, J., Riggins, T., Brown, J., Lomas, M. W., and Martiny, A. C. (2018). High variability in cellular stoichiometry of carbon, nitrogen, and phosphorus within classes of marine eukaryotic phytoplankton under sufficient nutrient conditions. Front. Microbiol. 9:543. doi: 10.3389/fmicb.2018.00543
Ger, K. A., Naus-Wiezer, S., De Meester, L., and Lürling, M. (2019). Zooplankton grazing selectivity regulates herbivory and dominance of toxic phytoplankton over multiple prey generations. Limnol. Oceanogr. 64, 1214–1227. doi: 10.1002/lno.11108
Goebel, N. L., Edwards, C. A., Carter, B. J., Achilles, K. M., and Zehr, J. P. (2008). Growth and carbon content of three different-sized diazotrophic cyanobacteria observed in the subtropical north pacific1. J. Phycol. 44, 1212–1220.
Großkopf, T., and La Roche, J. (2012). Direct and indirect costs of dinitrogen fixation in Crocosphaera watsonii WH8501 and possible implications for the nitrogen cycle. Front. Microbiol. 3:236. doi: 10.3389/fmicb.2012.00236
Gruber, N. (2004). “The dynamics of the marine nitrogen cycle and its influence on atmospheric CO2 variations,” in The Ocean Carbon Cycle and Climate, eds M. Follows and T. Oguz (Dordrecht: Springer Netherlands), 97–148.
Guo, C., and Tester, P. A. (1994). Toxic effect of the bloom-forming Trichodesmium sp. (cyanophyta) to the copepod Acartia tonsa. Nat. Toxins 2, 222–227. doi: 10.1002/nt.2620020411
Hamersley, M., Turk, K., Leinweber, A., Gruber, N., Zehr, J., Gunderson, T., et al. (2011). Nitrogen fixation within the water column associated with two hypoxic basins in the Southern California Bight. Aquat. Microb. Ecol. 63, 193–205. doi: 10.3354/ame01494
Harding, K., Turk-Kubo, K. A., Sipler, R. E., Mills, M. M., Bronk, D. A., and Zehr, J. P. (2018). Symbiotic unicellular cyanobacteria fix nitrogen in the Arctic Ocean. Proc. Natl. Acad. Sci. U. S. A. 115, 13371–13375. doi: 10.1073/pnas.1813658115
Hawser, S. P., O’Neil, J. M., Roman, M. R., and Codd, G. A. (1992). Toxicity of blooms of the cyanobacterium Trichodesmium to zooplankton. J. Appl. Phycol. 4, 79–86. doi: 10.1007/BF00003963
Hewson, I., Govil, S., Capone, D., Carpenter, E., and Fuhrman, J. (2004). Evidence of Trichodesmium viral lysis and potential significance for biogeochemical cycling in the oligotrophic ocean. Aquat. Microb. Ecol. 36, 1–8. doi: 10.3354/ame036001
Hogfors, H., Motwani, N. H., Hajdu, S., El-Shehawy, R., Holmborn, T., Vehmaa, A., et al. (2014). Bloom-forming cyanobacteria support copepod reproduction and development in the Baltic Sea. PLoS One 9:e112692. doi: 10.1371/journal.pone.0112692
Holl, C. M., and Montoya, J. P. (2005). Interactions between nitrate uptake and nitrogen fixation in continuous cultures of the marine diazotroph trichodesmium (cyanobacteria)1: trichodesmium: nitrate and N2 fixation. J. Phycol. 41, 1178–1183. doi: 10.1111/j.1529-8817.2005.00146.x
Holling, C. S. (1965). The functional response of predators to prey density and its role in mimicry and population regulation. Mem. Entomol. Soc. Can. 97, 5–60. doi: 10.4039/entm9745fv
Huisman, J., Codd, G. A., Paerl, H. W., Ibelings, B. W., Verspagen, J. M. H., and Visser, P. M. (2018). Cyanobacterial blooms. Nat. Rev. Microbiol. 16, 471–483. doi: 10.1038/s41579-018-0040-1
Hunt, B. P. V., Bonnet, S., Berthelot, H., Conroy, B. J., Foster, R. A., and Pagano, M. (2016). Contribution and pathways of diazotroph-derived nitrogen to zooplankton during the VAHINE mesocosm experiment in the oligotrophic New Caledonia lagoon. Biogeosciences 13, 3131–3145. doi: 10.5194/bg-13-3131-2016
Inomura, K., Bragg, J., Riemann, L., and Follows, M. J. (2018). A quantitative model of nitrogen fixation in the presence of ammonium. PLoS One 13:e0208282. doi: 10.1371/journal.pone.0208282
Jürgens, K., and DeMott, W. R. (1995). Behavioral flexibility in prey selection by bacterivorous nanoflagellates. Limnol. Oceanogr. 40, 1503–1507. doi: 10.4319/lo.1995.40.8.1503
Kiørboe, T., and Hirst, A. G. (2014). Shifts in mass scaling of respiration, feeding, and growth rates across life-form transitions in marine pelagic organisms. Am. Nat. 183, E118–E130. doi: 10.1086/675241
Kiørboe, T., Saiz, E., Tiselius, P., and Andersen, K. H. (2018). Adaptive feeding behavior and functional responses in zooplankton: functional responses in zooplankton. Limnol. Oceanogr. 63, 308–321. doi: 10.1002/lno.10632
Klausmeier, C. A., Litchman, E., Daufresne, T., and Levin, S. A. (2004). Optimal nitrogen-to-phosphorus stoichiometry of phytoplankton. Nature 429, 171–174. doi: 10.1038/nature02454
Knapp, A. N. (2012). The sensitivity of marine N2 fixation to dissolved inorganic nitrogen. Front. Microbiol 3:374. doi: 10.3389/fmicb.2012.00374
Koski, M., Schmidt, K., Engström-Öst, J., Viitasalo, M., Jónasdóttir, S., Repka, S., et al. (2002). Calanoid copepods feed and produce eggs in the presence of toxic cyanobacteria Nodularia spumigena. Limnol. Oceanogr. 47, 878–885. doi: 10.4319/lo.2002.47.3.0878
Kozlowsky-Suzuki, B., Karjalainen, M., Lehtiniemi, M., Engström-Öst, J., Koski, M., and Carlsson, P. (2003). Feeding, reproduction and toxin accumulation by the copepods Acartia bifilosa and Eurytemora affinis in the presence of the toxic cyanobacterium Nodularia spumigena. Mar. Ecol. Prog. Ser. 249, 237–249. doi: 10.3354/meps249237
Kustka, A. B., Sañudo-Wilhelmy, S. A., Carpenter, E. J., Capone, D., Burns, J., and Sunda, W. G. (2003). Iron requirements for dinitrogen- and ammonium-supported growth in cultures of Trichodesmium (IMS 101): comparison with nitrogen fixation rates and iron: carbon ratios of field populations. Limnol. Oceanogr. 48, 1869–1884. doi: 10.4319/lo.2003.48.5.1869
Landolfi, A., Dietze, H., Koeve, W., and Oschlies, A. (2013). Overlooked runaway feedback in the marine nitrogen cycle: the vicious cycle. Biogeosciences 10, 1351–1363. doi: 10.5194/bg-10-1351-2013
Landolfi, A., Kähler, P., Koeve, W., and Oschlies, A. (2018). Global marine N2 fixation estimates: from observations to models. Front. Microbiol. 9:2112. doi: 10.3389/fmicb.2018.02112
Landolfi, A., Koeve, W., Dietze, H., Kähler, P., and Oschlies, A. (2015). A new perspective on environmental controls of marine nitrogen fixation. Geophys. Res. Lett. 42, 4482–4489. doi: 10.1002/2015GL063756
Landolfi, A., Somes, C. J., Koeve, W., Zamora, L. M., and Oschlies, A. (2017). Oceanic nitrogen cycling and N 2 O flux perturbations in the Anthropocene: N cycling in the anthropocene. Glob. Biogeochem. Cycles 31, 1236–1255. doi: 10.1002/2017GB005633
Landry, M. R., and Calbet, A. (2004). Microzooplankton production in the oceans. ICES J. Mar. Sci. 61, 501–507. doi: 10.1016/j.icesjms.2004.03.011
LaRoche, J., and Breitbarth, E. (2005). Importance of the diazotrophs as a source of new nitrogen in the ocean. J. Sea Res. 53, 67–91. doi: 10.1016/j.seares.2004.05.005
Liu, H., and Buskey, E. J. (2000). The exopolymer secretions (EPS) layer surrounding Aureoumbra lagunensis cells affects growth, grazing, and behavior of protozoa. Limnol. Oceanogr. 45, 1187–1191. doi: 10.4319/lo.2000.45.5.1187
Loick-Wilde, N., Dutz, J., Miltner, A., Gehre, M., Montoya, J. P., and Voss, M. (2012). Incorporation of nitrogen from N 2 fixation into amino acids of zooplankton. Limnol. Oceanogr. 57, 199–210. doi: 10.4319/lo.2012.57.1.0199
Löscher, C. R., Großkopf, T., Desai, F. D., Gill, D., Schunck, H., Croot, P. L., et al. (2014). Facets of diazotrophy in the oxygen minimum zone waters off Peru. ISME J. 8, 2180–2192. doi: 10.1038/ismej.2014.71
Lundgren, V., Granéli, E., and Pflugmacher, S. (2012). Influence of Acartia cf. bifilosa (Copepoda) on morphology and toxicity of Nodularia spumigena (Cyanophyceae). Harmful Algae 18, 35–46. doi: 10.1016/j.hal.2012.04.003
Lürling, M. (2021). Grazing resistance in phytoplankton. Hydrobiologia 848, 237–249. doi: 10.1007/s10750-020-04370-3
McClelland, J. W., Holl, C. M., and Montoya, J. P. (2003). Relating low δ15N values of zooplankton to N2-fixation in the tropical North Atlantic: insights provided by stable isotope ratios of amino acids. Deep Sea Res. Part 1 Oceanogr. Res. Pap. 50, 849–861. doi: 10.1016/S0967-0637(03)00073-6
Mehta, M. P., and Baross, J. A. (2006). Nitrogen fixation at 92 C by a hydrothermal vent archaeon. Science 314, 1783–1786. doi: 10.1126/science.1134772
Meunier, C. L., Boersma, M., Wiltshire, K. H., and Malzahn, A. M. (2016). Zooplankton eat what they need: copepod selective feeding and potential consequences for marine systems. Oikos 125, 50–58. doi: 10.1111/oik.02072
Meyer-Harms, B., Reckermann, M., Voß, M., Siegmund, H., and von Bodungen, B. (1999). Food selection by calanoid copepods in the euphotic layer of the Gotland Sea (Baltic Proper) during mass occurrence of N2-fixing cyanobacteria. Mar. Ecol. Prog. Ser. 191, 243–250. doi: 10.3354/meps191243
Mitra, A., and Flynn, K. J. (2006). Accounting for variation in prey selectivity by zooplankton. Ecol. Model. 199, 82–92. doi: 10.1016/j.ecolmodel.2006.06.013
Moisander, P. H., Beinart, R. A., Hewson, I., White, A. E., Johnson, K. S., Carlson, C. A., et al. (2010). Unicellular cyanobacterial distributions broaden the oceanic N2 fixation domain. Science 327, 1512–1514. doi: 10.1126/science.1185468
Moisander, P. H., Benavides, M., Bonnet, S., Berman-Frank, I., White, A. E., and Riemann, L. (2017). Chasing after Non-cyanobacterial nitrogen fixation in marine pelagic environments. Front. Microbiol. 8:1736. doi: 10.3389/fmicb.2017.01736
Mojica, K. D. A., Huisman, J., Wilhelm, S. W., and Brussaard, C. P. D. (2016). Latitudinal variation in virus-induced mortality of phytoplankton across the North Atlantic Ocean. ISME J. 10, 500–513. doi: 10.1038/ismej.2015.130
Motwani, N. H., Duberg, J., Svedén, J. B., and Gorokhova, E. (2018). Grazing on cyanobacteria and transfer of diazotrophic nitrogen to zooplankton in the Baltic Sea: Cyanobacteria blooms support zooplankton growth. Limnol. Oceanogr. 63, 672–686. doi: 10.1002/lno.10659
Mulholland, M. R. (2007). The fate of nitrogen fixed by diazotrophs in the ocean. Biogeosciences 4, 37–51. doi: 10.5194/bg-4-37-2007
Mulholland, M. R., Bernhardt, P. W., Widner, B. N., Selden, C. R., Chappell, P. D., Clayton, S., et al. (2019). High rates of N 2 fixation in temperate, Western North Atlantic Coastal waters expand the realm of marine diazotrophy. Glob. Biogeochem. Cycles 33, 826–840. doi: 10.1029/2018GB006130
Müller-Navarra, D. C., Brett, M. T., Liston, A. M., and Goldman, C. R. (2000). A highly unsaturated fatty acid predicts carbon transfer between primary producers and consumers. Nature 403, 74–77. doi: 10.1038/47469
Ng, W. H. A., Liu, H., and Zhang, S. (2017). Diel variation of grazing of the dinoflagellate Lepidodinium sp. and ciliate Euplotes sp. on algal prey: the effect of prey cell properties. J. Plankton Res. 39, 450–462. doi: 10.1093/plankt/fbx020
O’Neil, J., and Roman, M. R. (1992). “Grazers and associated organisms of Trichodesmium,” in Marine Pelagic Cyanobacteria: Trichodesmium and other Diazotrophs, in Trichodesmium and other Diazotrophs, eds E. J. Carpenter, D. G. Capone, and J. G. Rueter (Netherlands: Springer).
O’Neil, J. M., and Roman, M. R. (1994). Ingestion of the cyanobacterium Trichodesmium spp. by pelagic harpacticoid copepods Macrosetella, Miracia and Oculosetella. Hydrobiologia 235–240. doi: 10.1007/BF00229946
O’Neil, J. M. (1998). The colonial cyanobacterium Trichodesmium as a physical and nutritional substrate for the harpacticoid copepod Macrosetella gracilis. J. Plankton Res. 20, 43–59.
Pahlow, M., Chien, C.-T., Arteaga, L. A., and Oschlies, A. (2020). Optimality-based non-redfield plankton–ecosystem model (OPEM v1.1) in UVic-ESCM 2.9 – Part 1: implementation and model behaviour. Geosci. Model Dev. 13, 4663–4690. doi: 10.5194/gmd-13-4663-2020
Pahlow, M., Dietze, H., and Oschlies, A. (2013). Optimality-based model of phytoplankton growth and diazotrophy. Mar. Ecol. Prog. Ser. 489, 1–16. doi: 10.3354/meps10449
Pahlow, M., and Prowe, A. (2010). Model of optimal current feeding in zooplankton. Mar. Ecol. Prog. Ser. 403, 129–144. doi: 10.3354/meps08466
Pančić, M., and Kiørboe, T. (2018). Phytoplankton defence mechanisms: traits and trade-offs. Biol. Rev. Camb. Philos. Soc. 93, 1269–1303.
Passow, U. (2002). Transparent exopolymer particles (TEP) in aquatic environments. Prog. Oceanogr. 55, 287–333.
Postgate, F. R. A. (1982). Biological nitrogen fixation: fundamentals. Philos. Trans. R. Soc. Lond. B 296, 375–385.
Prowe, A. E. F. (2012). Controls on the diversity–productivity relationship in a marine ecosystem model. Ecol. Model. 225, 167–176.
Prowe, A. E. F., Pahlow, M., Dutkiewicz, S., Follows, M., and Oschlies, A. (2012a). Top-down control of marine phytoplankton diversity in a global ecosystem model. Prog. Oceanogr. 101, 1–13. doi: 10.1016/j.pocean.2011.11.016
Prowe, A. E. F., Pahlow, M., and Oschlies, A. (2012b). Controls on the diversity–productivity relationship in a marine ecosystem model. Ecol. Model. 225, 167–176. doi: 10.1016/j.ecolmodel.2011.11.018
Redfield, A. C., Ketchum, B. H., and Richards, F. A. (1963). “The Influence of organisms on the composition of the sea water,” in The Sea, ed. M. N. Hill (New York, NY: Interscience Publishers), 26–77.
Riemann, L., Farnelid, H., and Steward, G. (2010). Nitrogenase genes in non-cyanobacterial plankton: prevalence, diversity and regulation in marine waters. Aquat. Microb. Ecol. 61, 235–247. doi: 10.3354/ame01431
Saito, M. A., Bertrand, E. M., Dutkiewicz, S., Bulygin, V. V., Moran, D. M., Monteiro, F. M., et al. (2011). Iron conservation by reduction of metalloenzyme inventories in the marine diazotroph Crocosphaera watsonii. Proc. Natl. Acad. Sci. U. S. A. 108, 2184–2189. doi: 10.1073/pnas.1006943108
Sandel, V., Kiko, R., Brandt, P., Dengler, M., Stemmann, L., Vandromme, P., et al. (2015). Nitrogen fuelling of the pelagic food web of the tropical Atlantic. PLoS One 10:e0131258. doi: 10.1371/journal.pone.0131258
Scavotto, R. E., Dziallas, C., Bentzon-Tilia, M., Riemann, L., and Moisander, P. H. (2015). Nitrogen-fixing bacteria associated with copepods in coastal waters of the North Atlantic Ocean: diazotroph community in association with copepods. Environ. Microbiol. 17, 3754–3765. doi: 10.1111/1462-2920.12777
Schultz, M., and Kiørboe, T. (2009). Active prey selection in two pelagic copepods feeding on potentially toxic and non-toxic dinoflagellates. J. Plankton Res. 31, 553–561. doi: 10.1093/plankt/fbp010
Sellner, K. G., Olson, M. M., and Kononen, K. (1994). “Copepod grazing in a summer cyanobacteria bloom in the Gulf of Finland,” in Ecology and Morphology of Copepods, eds F. D. Ferrari and B. P. Bradley (Dordrecht: Springer Netherlands), 249–254. doi: 10.1007/978-94-017-1347-4_33
Sellner, K. G., Olson, M. M., and Olli, K. (1996). Copepod interactions with toxic and non-toxic cyanobacteria from the Gulf of Finland. Phycologia 35, 177–182. doi: 10.2216/i0031-8884-35-6S-177.1
Shiozaki, T., Bombar, D., Riemann, L., Hashihama, F., Takeda, S., Yamaguchi, T., et al. (2017). Basin scale variability of active diazotrophs and nitrogen fixation in the North Pacific, from the tropics to the subarctic Bering Sea: BNF from the tropical to Subarctic Ocean. Glob. Biogeochem. Cycles 31, 996–1009. doi: 10.1002/2017GB005681
Sohm, J. A., Edwards, B. R., Wilson, B. G., and Webb, E. A. (2011). Constitutive extracellular polysaccharide (EPS) production by specific isolates of Crocosphaera watsonii. Front. Microbiol. 2:229. doi: 10.3389/fmicb.2011.00229
Sommer, F., Hansen, T., and Sommer, U. (2006). Transfer of diazotrophic nitrogen to mesozooplankton in Kiel Fjord, Western Baltic Sea: a mesocosm study. Mar. Ecol. Prog. Ser. 324, 105–112. doi: 10.3354/meps324105
Stal, L. J. (2009). Is the distribution of nitrogen-fixing cyanobacteria in the oceans related to temperature? Environ. Microbiol. 11, 1632–1645. doi: 10.1111/j.1758-2229.2009.00016.x
Sterner, R. W., and Elser, J. J. (2002). Ecological Stoichiometry: The Biology of Elements From Molecules to the Biosphere. Princeton, NJ: Princeton Univ. Press.
Suttle, C. A. (2007). Marine viruses — major players in the global ecosystem. Nat. Rev. Microbiol. 5, 801–812. doi: 10.1038/nrmicro1750
Thingstad, T. F. (2000). Elements of a theory for the mechanisms controlling abundance, diversity, and biogeochemical role of lytic bacterial viruses in aquatic systems. Limnol. Oceanogr. 45, 1320–1328. doi: 10.4319/lo.2000.45.6.1320
Tilman, D. (1980). Resources: a graphical-mechanistic approach to competition and predation. Am. Nat. 116, 361–393. doi: 10.1086/283633
Tsakalakis, I., Pahlow, M., Oschlies, A., Blasius, B., and Ryabov, A. B. (2018). Diel light cycle as a key factor for modelling phytoplankton biogeography and diversity. Ecol. Model. 384, 241–248. doi: 10.1016/j.ecolmodel.2018.06.022
Tuit, C., Waterbury, J., and Ravizza, G. (2004). Diel variation of molybdenum and iron in marine diazotrophic cyanobacteria. Limnol. Oceanogr. 49, 978–990. doi: 10.4319/lo.2004.49.4.0978
Turk-Kubo, K. A., Connell, P., Caron, D., Hogan, M. E., Farnelid, H. M., and Zehr, J. P. (2018). In Situ diazotroph population dynamics under different resource ratios in the North Pacific subtropical gyre. Front. Microbiol. 9:1616. doi: 10.3389/fmicb.2018.01616
Tyrrell, T. (1999). The relative influences of nitrogen and phosphorus on oceanic primary production. Nature 400, 525–531. doi: 10.1038/22941
Vallina, S. M., Ward, B. A., Dutkiewicz, S., and Follows, M. J. (2014). Maximal feeding with active prey-switching: a kill-the-winner functional response and its effect on global diversity and biogeography. Prog. Oceanogr. 120, 93–109. doi: 10.1016/j.pocean.2013.08.001
Van Donk, E., Ianora, A., and Vos, M. (2011). Induced defences in marine and freshwater phytoplankton: a review. Hydrobiologia 668, 3–19.
Villareal, T. A., and Carpenter, E. J. (2003). Buoyancy Regulation and the Potential for Vertical Migration in the Oceanic Cyanobacterium Trichodesmium. Microb. Ecol. 45, 1–10. doi: 10.1007/s00248-002-1012-5
Wang, W.-L., Moore, J. K., Martiny, A. C., and Primeau, F. W. (2019). Convergent estimates of marine nitrogen fixation. Nature 566, 205–211. doi: 10.1038/s41586-019-0911-2
Wannicke, N., Korth, F., Liskow, I., and Voss, M. (2013). Incorporation of diazotrophic fixed N2 by mesozooplankton — case studies in the southern Baltic Sea. J. Mar. Syst. 11, 1–13. doi: 10.1016/j.jmarsys.2013.03.005
Ward, B. A., Dutkiewicz, S., and Follows, M. J. (2014). Modelling spatial and temporal patterns in size-structured marine plankton communities: top–down and bottom–up controls. J. Plankton Res. 36, 31–47. doi: 10.1093/plankt/fbt097
Ward, B. A., Dutkiewicz, S., Jahn, O., and Follows, M. J. (2012). A size-structured food-web model for the global ocean. Limnol. Oceanogr. 57, 1877–1891. doi: 10.4319/lo.2012.57.6.1877
Ward, B. A., Dutkiewicz, S., Moore, C. M., and Follows, M. J. (2013). Iron, phosphorus, and nitrogen supply ratios define the biogeography of nitrogen fixation. Limnol. Oceanogr. 58, 2059–2075. doi: 10.4319/lo.2013.58.6.2059
Weitz, J. S., Stock, C. A., Wilhelm, S. W., Bourouiba, L., Coleman, M. L., Buchan, A., et al. (2015). A multitrophic model to quantify the effects of marine viruses on microbial food webs and ecosystem processes. ISME J. 9, 1352–1364. doi: 10.1038/ismej.2014.220
White, A. E., Spitz, Y. H., Karl, D. M., and Letelier, R. M. (2006). Flexible elemental stoichiometry in Trichodesmium spp. and its ecological implications. Limnol. Oceanogr. 51, 1777–1790. doi: 10.4319/lo.2006.51.4.1777
Wilson, S., and Steinberg, D. (2010). Autotrophic picoplankton in mesozooplankton guts: evidence of aggregate feeding in the mesopelagic zone and export of small phytoplankton. Mar. Ecol. Prog. Ser. 412, 11–27. doi: 10.3354/meps08648
Wilson, S. T., Aylward, F. O., Ribalet, F., Barone, B., Casey, J. R., Connell, P. E., et al. (2017). Coordinated regulation of growth, activity and transcription in natural populations of the unicellular nitrogen-fixing cyanobacterium Crocosphaera. Nat. Microbiol. 2:17118. doi: 10.1038/nmicrobiol.2017.118
Wirtz, K. W. (2012). Intermittency in processing explains the diversity and shape of functional grazing responses. Oecologia 169, 879–894. doi: 10.1007/s00442-012-2257-4
Woodland, R. J., Holland, D. P., Beardall, J., Smith, J., Scicluna, T., and Cook, P. L. M. (2013). Assimilation of diazotrophic nitrogen into pelagic food webs. PLoS One 8:e67588. doi: 10.1371/journal.pone.0067588
Zehr, J. P., Mellon, M. T., and Zani, S. (1998). New nitrogen-fixing microorganisms detected in oligotrophic oceans by amplification of nitrogenase (nifH) genes. Appl. Environ. Microbiol. 64, 3444–3450. doi: 10.1128/AEM.64.9.3444-3450.1998
Keywords: N2 fixation, selective grazing, environmental controls, bottom-up control, top-down control, ecological niche, marine diazotrophs
Citation: Landolfi A, Prowe AEF, Pahlow M, Somes CJ, Chien C-T, Schartau M, Koeve W and Oschlies A[cpsbreak] (2021) Can Top-Down Controls Expand the Ecological Niche of Marine N2 Fixers? Front. Microbiol. 12:690200. doi: 10.3389/fmicb.2021.690200
Received: 02 April 2021; Accepted: 05 July 2021;
Published: 18 August 2021.
Edited by:
Mar Benavides, Institut de Recherche Pour le Développement (IRD), FranceReviewed by:
Kalle Olli, Estonian University of Life Sciences, EstoniaSilvia Pajares Moreno, National Autonomous University of Mexico, Mexico
Pearse James Buchanan, University of Liverpool, United Kingdom
Copyright © 2021 Landolfi, Prowe, Pahlow, Somes, Chien, Schartau, Koeve and Oschlies. This is an open-access article distributed under the terms of the Creative Commons Attribution License (CC BY). The use, distribution or reproduction in other forums is permitted, provided the original author(s) and the copyright owner(s) are credited and that the original publication in this journal is cited, in accordance with accepted academic practice. No use, distribution or reproduction is permitted which does not comply with these terms.
*Correspondence: Angela Landolfi, YW5nZWxhLmxhbmRvbGZpQGNuci5pdA==