- 1Food Microbiology and Mushroom Research Division, CSIR-Food Research Institute, Accra, Ghana
- 2Department of Nutrition and Food Science, University of Ghana, Accra, Ghana
- 3Food and Health Institute Strategic Programme, Quadram Institute Bioscience, Norwich Research Park, Norwich, United Kingdom
- 4Gut Microbes and Health Institute Strategic Programme, Quadram Institute Bioscience, Norwich Research Park, Norwich, United Kingdom
- 5Analytical Sciences Unit, Quadram Institute Bioscience, Norwich Research Park, Norwich, United Kingdom
- 6Department of Food Science, Section for Food Microbiology and Fermentation, University of Copenhagen, Copenhagen, Denmark
- 7Quadram Institute Bioscience, Norwich Research Park, Norwich, United Kingdom
- 8Department of Agro-Processing Technology and Food Bio-Sciences, CSIR College of Science and Technology, Accra, Ghana
Hausa koko is an indigenous porridge processed from millet in Ghana. The process involves fermentation stages, giving the characteristic organoleptic properties of the product that is produced largely at a small-scale household level and sold as a street food. Like many other indigenous foods, quality control is problematic and depends on the skills of the processor. In order to improve the quality of the product and standardize the process for large-scale production, we need a deeper understanding of the microbial processes. The aim of this study is to investigate the microbial community involved in the production of this traditional millet porridge and the metabolites produced during processing. High-throughput amplicon sequencing was used to identify the bacterial (16S rRNA V4 hypervariable region) and fungal [Intergenic Transcribed Spacer (ITS)] communities associated with the fermentation, while nuclear magnetic resonance (NMR) was used for metabolite profiling. The bacterial community diversity was reduced during the fermentation processes with an increase and predominance of lactobacilli. Other dominant bacteria in the fermentation included Pediococcus, Weissella, Lactococcus, Streptococcus, Leuconostoc, and Acetobacter. The species Limosilactobacillus fermentum and Ligilactobacillus salivarius accounted for some of the diversities within and between fermentation time points and processors. The fungal community was dominated by the genus Saccharomyces. Other genera such as Pichia, Candida, Kluyveromyces, Nakaseomyces, Torulaspora, and Cyberlindnera were also classified. The species Saccharomyces cerevisiae, Stachybotrys sansevieriae, Malassezia restricta, Cyberlindnera fabianii, and Kluyveromyces marxianus accounted for some of the diversities within some fermentation time points. The species S. sansevieria and M. restricta may have been reported for the first time in cereal fermentation. This is the most diverse microbial community reported in Hausa koko. In this study, we could identify and quantify 33 key different metabolites produced by the interactions of the microbial communities with the millet, composed of organic compounds, sugars, amino acids and intermediary compounds, and other key fermentation compounds. An increase in the concentration of organic acids in parallel with the reduction of sugars occurred during the fermentation process while an initial increase of amino acids followed by a decrease in later fermentation steps was observed.
Introduction
Porridges produced from fermented cereals such as maize, millet, and sorghum are essential diets in many parts of Africa, where they are used mostly as staple, weaning, or complementary foods, providing necessary nutrients (Omemu et al., 2007; Omemu and Omeike, 2010; Owusu-Kwarteng et al., 2010b). In Ghana, fermented thin cereal porridges are called koko and are usually eaten at breakfast. Fermented stiff cereal porridges are eaten as main meals mostly at lunch or dinner and include kenkey, banku, and tuo zaafi. One of the most popular of the Ghanaian thin porridges is Hausa koko, a spicy, smooth, and free-flowing fermented millet porridge that is mostly produced and sold as street food (Mensah et al., 2002; Haleegoah et al., 2016).
Hausa koko is produced by traditional food processors as a micro or cottage industry in mainly home-based operations using the two main indigenous methods shown in Figure 1. Lei and Jakobsen (2004) described and studied Process A while Process B is more commonly used and is the subject of the present investigation. The processes in Hausa koko production involve initial steeping of millet grains for 12–24 h, during which fermentation starts. The steeped grains are then washed and milled together with different spices in a plate mill. The resulting flour is mixed with water to prepare a slurry, which is then sieved using a cheese cloth. The slurry is allowed to ferment spontaneously for 8–12 h and becomes sour. During the fermentation of the slurry, it settles into a sediment and a supernatant with a foam sitting on top. The foam is scooped off and discarded, while the supernatant may be consumed in its raw state as koko sour water for medicinal purposes, especially for treatment of diarrhea. Indeed, Lei et al. (2006) have confirmed that koko sour water has natural probiotic properties. To prepare Hausa koko, most processors boil four volumes of water, and while stirring the fermented slurry (the supernatant and sediment together representing one volume), slowly pour in the hot boiled water to obtain the smooth gel-like porridge. A few processors, however, add water to the supernatant and boil the diluted supernatant. The hot diluted supernatant is then poured slowly into the sediment while stirring continuously to obtain Hausa koko. Hausa koko is sweetened with sugar before consumption and many consumers may add milk to the thin porridge or even roasted groundnuts. Traditionally, Hausa koko may be eaten with koose, a fried cowpea doughnut or masa, a fried sour millet, maize, or rice doughnut; however, Hausa koko is often eaten with bread.
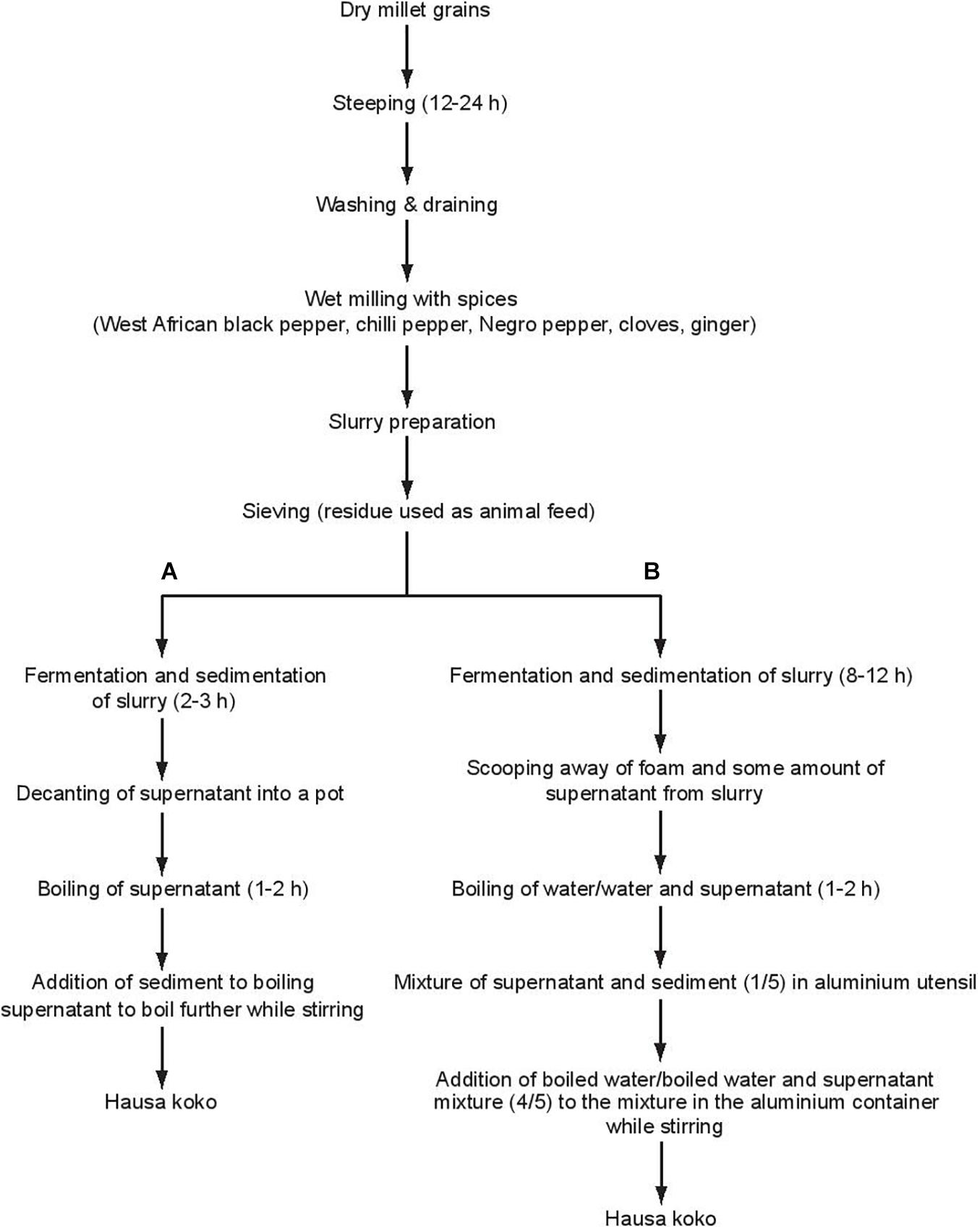
Figure 1. Flow diagram of Hausa koko production process. (A) Process described by Lei and Jakobson (2004). (B) Process described in the current work.
Only a few studies have been carried out to study the microbiology of Hausa koko processing, notably the work by Lei and Jakobsen (2004). They reported the spontaneous fermentation to be dominated by Weissella confusa and Lactobacillus (now Limosilactobacillus) fermentum, showing a pronounced taxonomic biodiversity at sub-species level between stages within the production as well as between production sites. Other species reported in koko sour water by Lei and Jakobsen (2004) were Lactobacillus (now Ligilactobacillus) salivarius, Pediococcus pentosaceus, Pediococcus acidilactici, and Lactobacillus (now Lactiplantibacillus) paraplantarum, and live LAB content of koko sour water has been estimated as c. 108 cells per ml.
Consumption of Hausa koko gives some of the benefits of fermentation. Fermentation of cereals is reported to reduce the levels of some anti-nutrients including tannins, polyphenols, and phytates, some of which can inhibit amylolysis and proteolysis and sequester proteins and valuable minerals in complexes; microbial enzymatic activity can disrupt these complexes and improve the bioavailability of minerals such as iron, calcium, zinc, and phosphorus (Sharma and Kapoor, 1996; Sindhu and Khetarpaul, 2001; Blandino et al., 2003). Fermentation also enhances the nutritional and sensory qualities as well as shelf life of the products, among many other benefits (Holzapfel, 2002; Ibnouf, 2012; Arena et al., 2014). Cereal fermented foods are also reported to provide health benefits such as blood-lowering effects, inhibition of allergies, antimicrobial effects, and control of diarrhea through probiotic mechanisms (Lei et al., 2006; Vasiljevic and Shah, 2008; Das et al., 2012; Zannini et al., 2012; Wang et al., 2014; Ray et al., 2016).
In the production of indigenous African foods, fermentations are carried out mostly spontaneously, i.e., without the use of a traditional inoculum or a starter culture. However, the products attain a low pH. In spite of this, the microbiological quality of Hausa koko and other porridges seem unpredictable. According to Yeleliere et al. (2017) indigenous Ghanaian cereal porridges can be contaminated with pathogens including Bacillus cereus, Staphylococcus aureus, and Enterobacteriaceae, which are significant in terms of food safety.
In the past few decades, several successful attempts have been made to upgrade the technologies used in the production of some indigenous African foods, including fermented products. Many of these are now produced by small- and medium-scale enterprise (SME) as convenience foods for both local and foreign markets targeting Africans. Attempts have also been made to use starter cultures at the SME level to gain a greater control over the process, but this is yet to be widely adopted by industry. The use of starter cultures, however, is seen as a prerequisite for standardizing the sensory quality of the products and improving their safety. In Ghana, the use of starter culture to produce kenkey, a cooked fermented maize dumpling, was demonstrated at an upgraded traditional food processing facility, but could not be sustained at the facility by the plant owner (Halm et al., 1996; Amoa-Awua et al., 2007). With regard to Hausa koko, an in-depth understanding of the microbial community involved in its production is required, if a suitable starter culture is to be developed for the production of Hausa koko by the Ghanaian food industry as a high-quality convenience food.
Porridges made from fermented cereals are also reported to have micronutrient deficiencies, hence require some fortification (Owusu-Kwarteng et al., 2010a; Tano-Debrah et al., 2019). In view of this, a food supplement known as KOKO plus was used to improve the nutritional profile of koko produced from fermented maize dough, while Hausa koko from pearl millet was fortified with soybean for complementary feeding for infants and children (Owusu-Kwarteng et al., 2010a; Tano-Debrah et al., 2019). Unfortunately, there is no information available on the nutritional profile and other metabolites produced during the processing of millet into Hausa koko.
This study was carried out to determine the diversity of microorganisms involved in the processing of millet into Hausa koko following 15 small-scale processors located in six regions of Ghana, using high-throughput sequencing technology. It also sought to determine the metabolites produced during the process.
Materials and Methods
Sampling
Samples of millet-based Hausa koko taken during the main steps involved in its production were collected from 15 small-scale processing sites. The sites were located in six regions of Ghana, distributed in three different geographical belts – northern, middle, and southern belts. The sites were distributed as follows: Northern region (Northern belt) – Tamale Central (TAC), Tamale Kalariga (TAK), and Tamale Dabokpa (TAD); Bono East Region (Middle belt) – Techiman Diasempa (TED), Techiman Abourso (TEA), Techiman Pomaakrom (TEP), and Techiman Kenten (TEK); Bono Region (Middle belt) – Sunyani (SUN); Central Region (Southern belt) – Mankessim (MAN) and Winneba (WIN); Eastern Region (Southern Belt) – Dodowa (DOD); Greater Accra Region (Southern belt) – Accra Ashaiman-Tulaku (AAT), Accra Madina Zongo (AMZ), Accra Ashaley Botwe (AAB), and Accra Ashaiman-Fafraha (AAF). The following samples were collected during processing at sites: dry millet (D), 12-h fermented millet (12 h), 24-h fermented millet (24 h), milled millet with spices (M), supernatant of slurry (Su), sediment of slurry (Sd), and Hausa koko (K). About 500 g of each sample was aseptically collected in duplicates into sterile bags and containers and transported to the CSIR-Food Research Institute (FRI) Microbiology laboratory under cold storage, where they were preserved at -20°C. The samples were later transported under cold conditions (frozen with iced packs) to the Quadram Institute Bioscience (QIB, Norwich, United Kingdom), where they were also preserved at −20°C and processed for further analysis.
Total Microbial DNA Extraction From Fermented Samples
Microbial DNA from the fermented samples was extracted according to Diaz et al. (2019), with minor modifications. First, 20 g of each sample was homogenized with 10 ml of ice-cold sterile ultrapure H2O by vortexing and centrifuged (Eppendorf 5810R, Germany) for 1 min at 800 × g at 4°C to remove the solid particles of the sample. This process was repeated twice, and the resulting supernatants were pooled together and centrifuged at 3,000 × g at 4°C for 20 min to harvest the cells. The pellets were washed three times with 1 ml of phosphate buffered saline (PBS) and centrifuged at 14,000 × g for 2 min. Microbial DNA was extracted from the pellets using the FastDNA spin kit for soil (MP Biomedicals, United States). All steps were performed following the manufacturer’s instructions, with cell lysis performed mechanically using a FastPrep-24 instrument (MP Biomedicals, United Kingdom) for 60 s at a speed of 6.5 m/s. The process was repeated three times with samples kept on ice for 5 min between each homogenization. DNA was eluted in 50 μl of DES (DNase/Pyrogen free water) pre-warmed at 55°C and quantified using the Qubit 3.0 fluorometer (Invitrogen, Malaysia) using the Qubit dsDNA Broad Range (BR) Assay kit or the Qubit dsDNA High Sensitivity (HS) Assay kit (Invitrogen), depending on the DNA concentration of the sample.
16S rRNA Amplicon Sequencing
Bacterial diversity was analyzed by 16S rRNA high-throughput amplicon sequencing. Amplification and sequencing were performed by Novogene (HK) Company Limited (Hong Kong) as follows. The V4 hypervariable region of the 16S rRNA gene was amplified by PCR using specific primers 515F and 806R (Caporaso et al., 2011) and the Phusion High-Fidelity PCR master mix (New England Biolabs, United States), following the manufacturer’s instructions. The amplicons were used to generate libraries using the Illumina NEBNext Ultra II DNA Library Prep Kit (New England Biolabs, United Kingdom) and then sequenced using paired-end Illumina sequencing (2 × 250 bp) on the HiSeq 2500 platform (Illumina, United States).
ITS Amplicon Sequencing
Fungal diversity was analyzed by high-throughput amplicon sequencing of the internal transcribed spacer (ITS). For this analysis, a subset of samples obtained from five processors were included (MAN, SUN, TAD, TEK, and WIN). Amplification and sequencing were performed at QIB (Norwich, United Kingdom). The ITS region was amplified by using primers ITS1F (5′-CTTGGTCATTTAGAGGAAGTAA-3′) (Jackson et al., 1999) and ITS2 (5′-GCTGCGTTCTTCATCGATGC-3′) (White et al., 1990) and the KAPA2G Robust HotStart PCR Kit (Sigma). Amplification was performed at 95°C for 5 min, 30 cycles of 95°C for 30 s, 55°C for 30 s, and 72°C for 30 s followed by a final 72°C for 5 min. PCR products were purified using KAPA Pure Beads (Roche) and used to generate a library using the KAPA2G Robust HotStart PCR Kit and the Nextera XT Index Kit v2 index primers (Illumina) following the manufacturer’s instructions. The libraries were sequenced on an Illumina MiSeq instrument using MiSeq® Reagent Kit v3 (Illumina) following the Illumina denaturation and loading recommendations.
Metabolite Analysis and Quantification
Metabolites from samples at selected stages (D, 12 h, 24 h, M, Su, and K) were analyzed by 1H-nuclear magnetic resonance spectroscopy (NMR). The solid samples were ground into fine flour with a laboratory mortar and pestle, and 1 g of the product was mixed with 4 ml of ultra-pure H2O by vortexing for 30 s. Liquid and semi-liquid samples were homogenized by vortexing, and 5 ml was used for further analysis. All samples were centrifuged at 2,000 × g for 5 min at 4°C and 400 μl of the supernatant was collected and mixed with 400 μl of NMR buffer (4.2 g NaH2PO4.H2O, 3.3 g K2HPO4, 17.2 mg of Na3PO4, and 20 mg NaN3 in 100 μl of 100 mM EDTA in H2O). Six hundred microliters of the mixture was transferred into 5-mm NMR borosilicate glass NMR tubes (Wilmad, Vineland, NJ, United States) and run on a 600-MHz AVANCETM spectrometer (Bruker, Billerica, MA, United States) with cryoprobe. The recorded spectra were transformed with a 0.3-Hz line broadening, and manually phased, baseline-corrected, and referenced by setting the TSP methyl signal to 0 ppm using the TopSpin software. Metabolites were identified and quantified by computer-assisted manual fitting with Chenomx NMR suite v 8.12 (Chenomx, Edmonton, AB, Canada), using Chenomx 600 MHz HMDB Compounds library.
Bioinformatic and Statistical Analysis
Bacterial and fungal diversity were analyzed using the Quantitative Insights Into Microbial Ecology 2 (QIIME2) 2020.8 software (Bolyen et al., 2019). The demultiplexed paired-end reads were filtered to remove substitution and chimera errors and merged using DADA2. Bacterial taxonomic assignment was performed at 97% similarity using a Naive Bayes classifier trained on the Silva version 138.1 99% operational taxonomic unit (OTU) database, where the sequences have been trimmed to only include 250 bases from the V4 region bound by the 515 F/806 R primer pair. For fungal characterization, primer and adapter sequences were removed from the reads. Analysis was carried out with the forward reads only to avoid bias caused by amplicons of larger length (i.e., larger than ca. 500 bp) that could not be merged (Taylor et al., 2016). The demultiplexed single-end reads were filtered to remove substitution and chimera errors and merged using DADA2 and taxonomic assignment was performed at 97% using a Naïve Bayes classifier trained on the UNITE Community (2019) fungal classifier version 8_99. Bacterial and fungal alpha diversity was analyzed using observed OTUs, Shannon, Pielou’s evenness, and Faith’s phylogenetic diversity indexes. Rarefaction curves were computed using observed OTUs. Significant differences in alpha diversity were calculated using the alpha-group-significance script in QIIME2, which performs the Kruskal–Wallis test. Jaccard, unweighted, and weighted UniFrac distances were used to generate beta diversity PCoA biplots, which were visualized using the Emperor tool. Differences in beta diversity between groups were analyzed using PERMANOVA including pairwise test (Anderson, 2017). Significant differences in the bacterial community structure among the groups were evaluated by Analysis of Composition of Microbiomes (ANCOM) (Mandal et al., 2015). For metabolites, statistical analysis using two-way ANOVA with Tukey’s multiple comparisons test was applied using GraphPad Prism version 8.4.3. A p-value ≤ 0.05 was considered statistically significant.
Results
Bacterial Diversity
DNA sequencing of the V4 amplicons by the Illumina HiSeq platform resulted in 8,361,221 paired-end sequence reads with an average of 89,905.60 ± 5932.20 sequences per sample. Of these, 18.5% were discarded due to poor quality, reads not merging, or after being identified as chimeras; as a result of these steps, 6,813,680 high-quality sequences were retained and analyzed, with an average of 73,265.37 ± 6301.12 sequences per sample. Distribution of reads per sample can be found in Supplementary Table 1. Background DNA was removed by filtering out sequences assigned to chloroplast and mitochondrial taxonomic groups. Data were rarefied to 49,824 sequences per sample to avoid bias. As shown in Figure 2A, the rarefaction curves for the observed OTUs were enough to capture the diversities within all the samples.
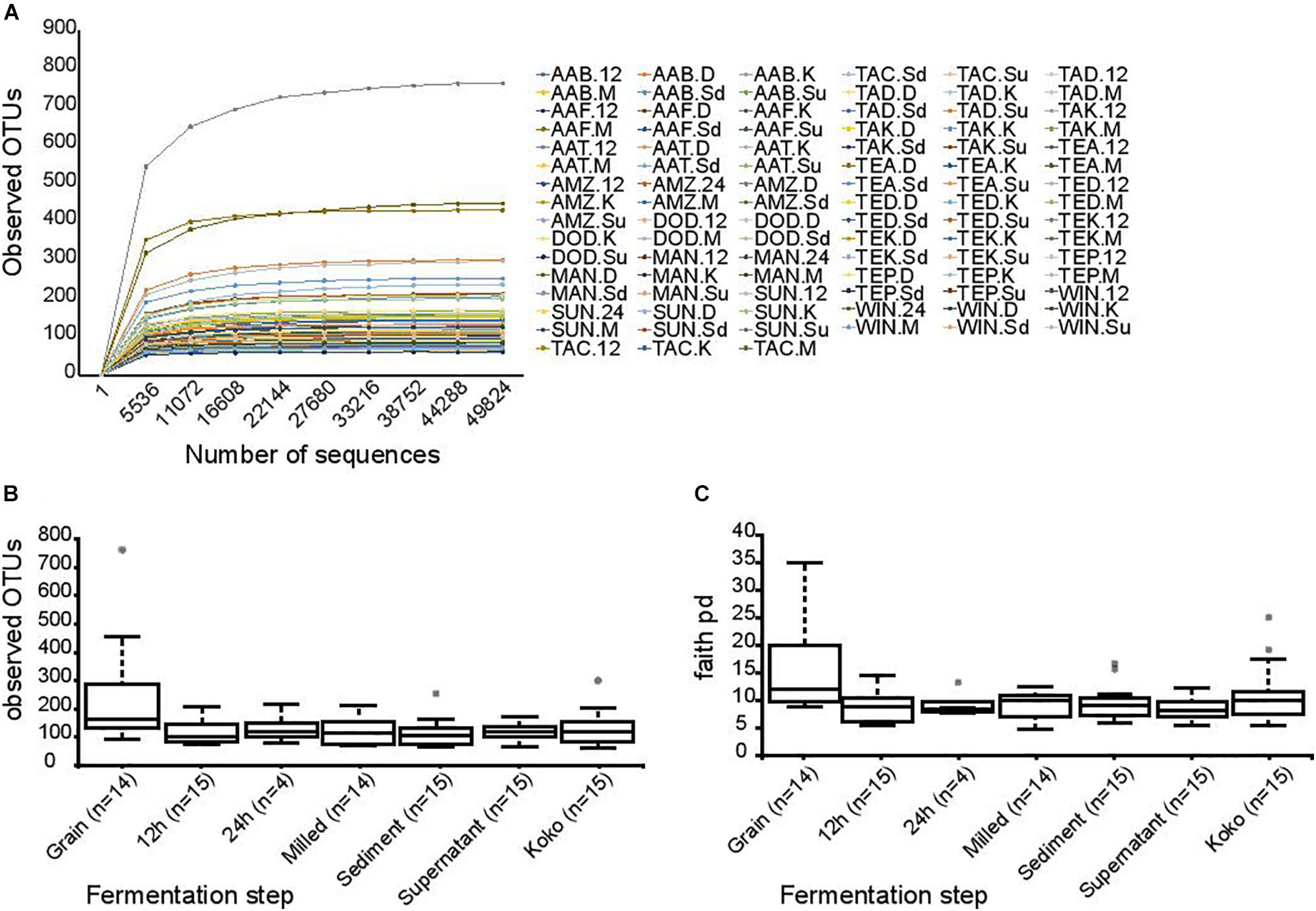
Figure 2. Bacterial alpha diversity indexes. (A) Rarefaction plots of observed OTUs depending on the number of sequences. Each curve represents a sample. Observed OTUs (B) and Faith’s phylogeny (C) diversity box plots for each fermentation step. Dots represent outliers.
Alpha diversity indexes (observed OTUs, Shannon, Faith’s phylogenetic diversity, and Pielou’s evenness) were compared based on the fermentation stages. Significant differences were detected between the observed OTUs at the various time points (p-value = 0.026), a reduction in the observed OTUs was detected between the grain samples and the 12-h fermentation, and the lower diversity was maintained during the production stages (p-values: vs. 12 h = 0.003; vs. milled = 0.004; vs. supernatant = 0.004; vs. sediment = 0.001; vs. koko = 0.012) (Figure 2B). Significant differences in the alpha diversity were also found between grain samples and other fermentation stages when using Faith’s phylogeny diversity index (Figure 2C) (p-value = 0.030 for all groups, p-values of grain vs.: 12 h = 0.005; 24 h = 0.033; milled = 0.021; supernatant = 0.002; sediment = 0.005; koko = 0.036). No statistical differences were observed when evenness and Shannon indexes were used.
Changes in the bacterial populations through the fermentation process and between different regions were analyzed by principal coordinate analysis (PCoA) (Figure 3) based on Jaccard (Figures 3A,C) and unweighted Unifrac (Figures 3B,D) distances. Differences between groups were mainly caused by OTUs within the genera Weissella, Pediococcus, and Acetobacter as well as the genus Lactobacillus and the species L. fermentum and L. salivarius. For Jaccard distances, statistical differences were found between samples based on the region in which they were produced (p-value = 0.001). Pairwise comparison showed statistically significant differences between all regions except between Central and Bono or Eastern regions. For unweighted Unifrac distances, statistical differences were observed between samples based on the region of production (p-value = 0.001). Samples produced in Northern and Greater Accra regions were the only ones showing significant differences with all other regions (p-values Northern region vs.: Bono East = 0.001; Bono = 0.002; Central = 0.001; Eastern = 0.001; Greater Accra = 0.001; p-values Central vs.: Bono East = 0.001; Bono = 0.002; Central = 0.001; Eastern = 0.001; p-values Greater Accra region vs.: Bono East = 0.001; Bono = 0.001; Central = 0.001; Eastern = 0.002; Northern = 0.001). Statistically significant differences in unweighted Unifrac distances were also found between the different fermentation steps (p-value = 0.024). Particularly, grain samples were different from the rest of time points (p-value of grain samples vs.: 12 h = 0.001; milled = 0.005; supernatant = 0.002; sediment = 0.002; koko = 0.023), except for 24 h, although this could be caused by the smaller sample size of the 24-h group.
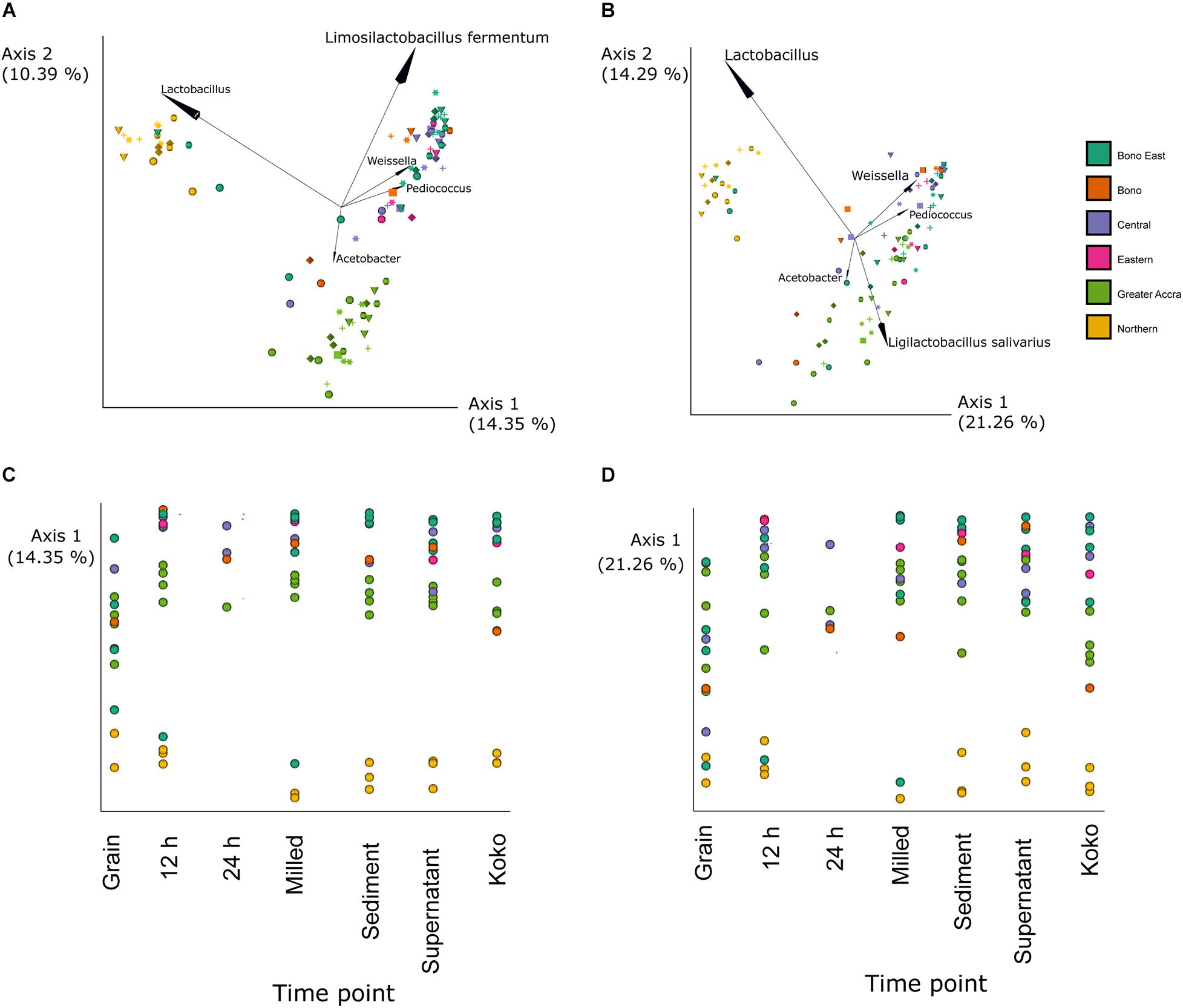
Figure 3. Bacterial beta diversity analysis. Principal coordinate analysis (PCoA) showing Jaccard (A,C) and unweighted UniFrac (B,D) distances. Samples produced in different regions are represented by different colors as indicated in the legend. In panels (A,B), fermentation time points are represented by different shapes: grain (circle), milled (triangle), 12 h (hexagon), 24 h (square), sediment (cross), supernatant (star), and Hausa koko (diamond). The arrows indicate the five taxonomic groups (at genera or species level) that contribute most to the differences. In panels (C,D), fermentation time points are represented along the x-axis. The percentage of variation explained by the plotted principal coordinates is indicated in the y-axis.
Over 400 different bacteria were profiled in this study. The samples from the different processors across the six regions showed that OTUs at the genus level in grain samples were mainly dominated by Sphingomonas, Clostridium, Staphylococcus, Pseudomonas, Bacteroides, Chryseobacterium, Enterobacteriaceae, and Escherichia-Shigella. Fermentation time points were, however, dominated by the genera Lactobacillus and Acetobacter. Pediococcus, Weissella, Pantoea, Leuconostoc, Gluconobacter, Streptococcus, and Lactococcus were also notable components. ANCOM at the genus level showed significant differences in the relative abundance depending on the processing step: the genus Pantoea (W = 842) decreased during the first fermentation step, and then was maintained at low levels, while the genus Limosilactobacillus increased during the fermentation process (W = 781) (Figure 4).
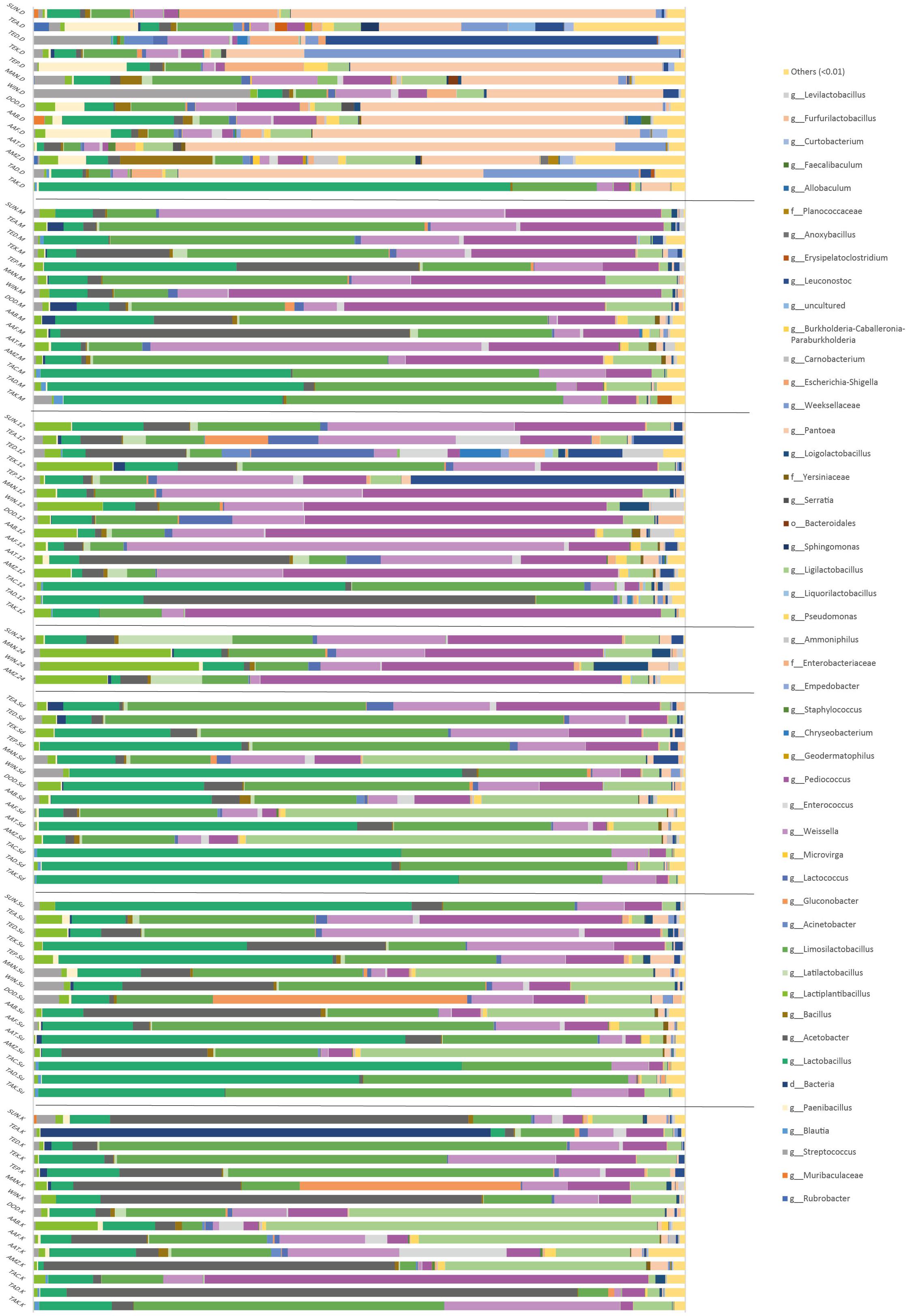
Figure 4. Relative abundance of the bacterial communities of the different processing steps during Hausa koko production. Each horizontal piled bar represents a single sample. Taxonomic groups at genera (g) level are identified where possible. Where genera could not be determined, order (o) or domain (d) are shown. Taxonomic groups with abundance <1% were included in the group “others.”
Fungal Diversity
Fungal diversity was profiled in a subset of samples obtained from five of the processing locations. DNA sequencing of the ITS amplicons by the Illumina MiSeq platform resulted in 4,792,254 single-end sequence reads with an average of 145,219.8 ± 56,955.31 sequences per sample. Of these, 34.36% were discarded due to poor quality or after being identified as chimeras; as a result of these steps, 1,646,961 high-quality sequences were retained and analyzed, with an average of 49,907.90 ± 44,668.62 sequences per sample. As observed in Supplementary Table 2, the distribution of reads per sample was very variable. Prior to alpha and beta diversity analysis, the data were rarefied to 4,640 sequences per sample. Although not all the observed OTUs were captured (Figure 5A), this sampling depth enabled us to analyze most of the samples (all except SUN-D, MAN-D, WIN-D, WIN-M, WIN-24, and MAN-K). For alpha diversity indexes (Figure 5B), significant differences (p-value = 0.025) in observed OTUs were detected between groups based on fermentation time points. Although grain samples seem to contain more observed OTUs, differences were not statistically significant (p = 0.051), probably due to the small number of samples that could be retained after removing samples with less than 4,640 reads per sample. Pairwise comparison showed significant differences between the following fermentation stages: 12 h vs. milled (p = 0.014); 12 h vs. sediment (p = 0.009); grains vs. sediment (p = 0.051); grain vs. supernatant (p = 0.051); milled vs. supernatant (p = 0.049); and supernatant vs. sediment (p = 0.016).
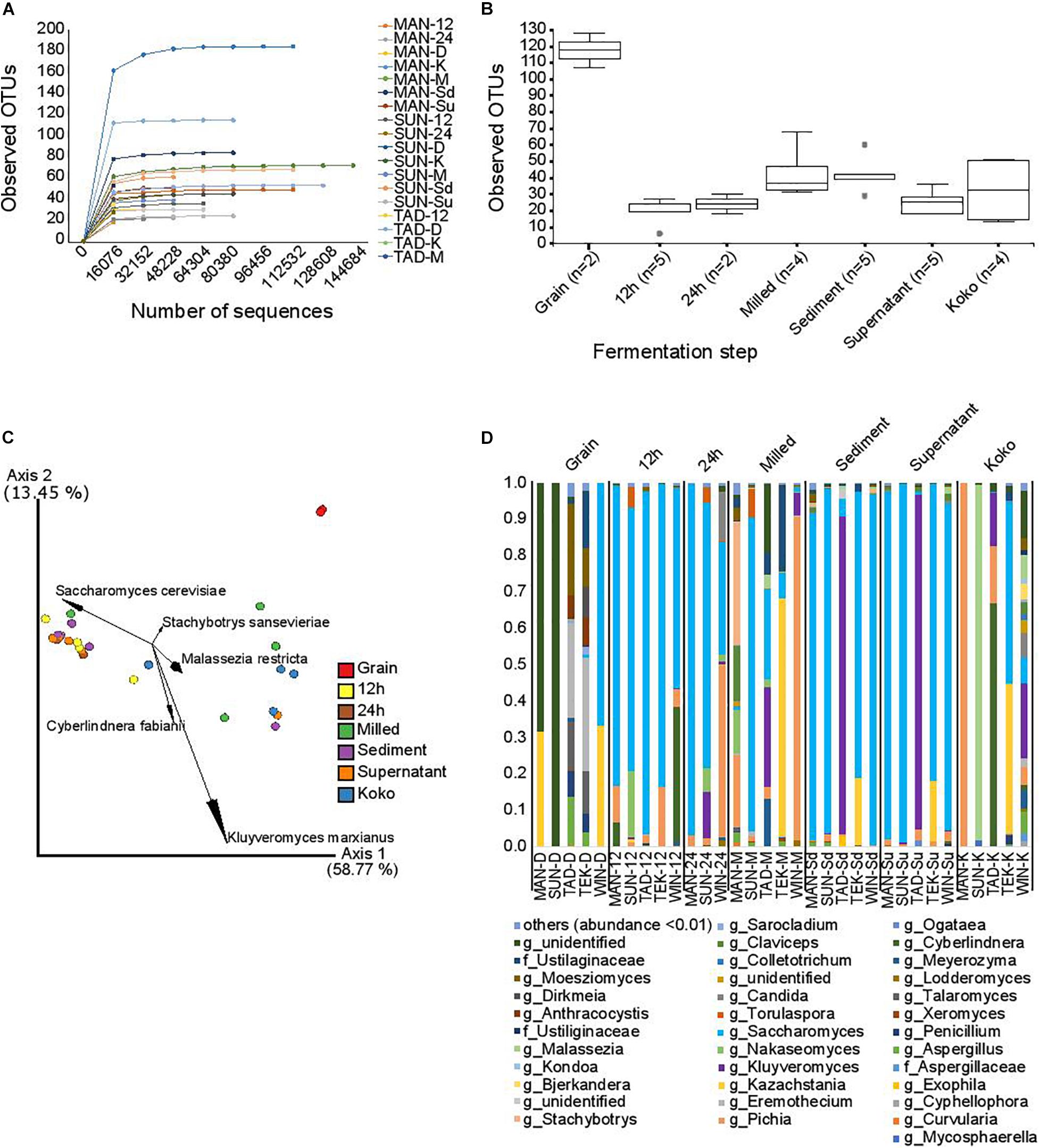
Figure 5. Fungal diversity. (A) Rarefaction plots of fungal observed OTUs depending on the sequencing depth. Each curve represents a sample. (B) Fungal alpha diversity based on the observed OTUs in the different fermentation steps. Dots represent outliers. (C) Fungal beta diversity represented by principal coordinate analysis based on weighted Unifrac distances. Fermentation time points are represented by different colors as indicated in the legend. The arrows indicate the five taxonomic groups that contribute most to the differences. (D) Relative abundance.
Analysis of the beta diversity indexes showed significant differences (p-value = 0.001) for weighted Unifrac distance based on fermentation time points (Figure 5C). Pairwise comparison showed significant differences between the following fermentation time points: grain vs. 12 h (p = 0.039), grain vs. supernatant (p = 0.042), and 12 h vs. koko (p = 0.01). Differences in weighted Unifrac distances were caused mainly by OTUs within the species Kluyveromyces marxianus, Saccharomyces cerevisiae, Cyberlindnera fabianii, Stachybotrys sansevieriae, and Malassezia restricta.
Analysis of Composition of Microbiomes at the genus level showed significant differences in the relative abundance depending on the processing step: the genera Saccharomyces (W = 123) and Pichia (W = 111) were more abundant in samples after the fermentation started than in the grain samples. Figure 5D shows the relative abundance across the fermentation steps.
The grain samples were characterized by fungi of the genera Kazachstania, Aspergillus, Penicillium, Talaromyces, Eremothecium, Anthracocystis, Moesziomyces, Sarocladium, and Dirkmeia; a limited population of Saccharomyces; and a number of unidentified fungi. The 12-h and 24-h fermentation time points recorded a shift from this diverse population to a less diversified community, dominated by Saccharomyces and Pichia. Candida, Kluyveromyces, Nakaseomyces, Torulaspora, Stachybotrys, and Cyberlindnera were also present. The milled samples with spices also showed a diverse profile made up of OTUs including yeasts Saccharomyces, Pichia, Kazachstania, Kluyveromyces, and Torulaspora. The sediment and supernatant fermentation time points were mainly dominated by Saccharomyces. Other fungi such as Kluyveromyces, Pichia, Kazachstania, Candida, Stachybotrys, Moesziomyces, Claviceps, and Malassezia were also associated with this time point. Hausa koko samples were highly diversified with yeast communities dominated by Pichia, Malassezia, Cyberlindnera, Kluyveromyces, and Saccharomyces. The Hausa koko sample from Winneba particularly was highly diversified compared to the others. Other fungal genera identified included Aspergillus, Meyerozyma, Eremothecium, Candida, Claviceps, Bjerkandera, Kondoa, Malassezia, Moesziomyces, and some unidentified communities.
Metabolic Composition
The metabolites present in the samples that are consumed (Su and K) as well as the different time points (D, 12 h, 24 h, and M) during Hausa koko production by 15 producers within six regions were analyzed. A total of 33 metabolites were detected and quantified (Figure 6) along the fermentation stages with varying trends in similar patterns in all the samples, irrespective of the geographical location the sample was obtained from. Two-way ANOVA test showed significant differences between time points with regard to organic acids. Among them, post hoc analysis showed significant increase in the concentration of lactate between grains and all the fermentation steps except koko (p-value grain vs. 12 h, 24 h, milled, and supernatant was <0.0001). Although no significant differences were detected, the concentration of lactate in koko was slightly higher than that in the grains. Similar changes were observed for acetate: statistical increases in acetate concentration between grains and 12 h (p < 0.0001), 24 h (p = 0.035), and milled samples (p < 0.0001) were detected. Again, statistical differences were found for ethanol between grains and 12 h (p < 0.0127), 24 h (p = 0.0342), and M (p < 0.0001). There were no significant differences for the other organic acids produced at the different time points. The changes observed in organic acids were inverse to the changes observed in sugar levels: glucose, the most abundant sugar found in the samples, decreased significantly in the first step of the fermentation (p < 0.0001) and increased slightly in the milled sample, although with a high variation between samples, to then decrease again in the supernatant and koko samples (p < 0.0001). Significant differences also existed in the concentration of fructose between grains and all the fermentation time points (p < 0.0001).
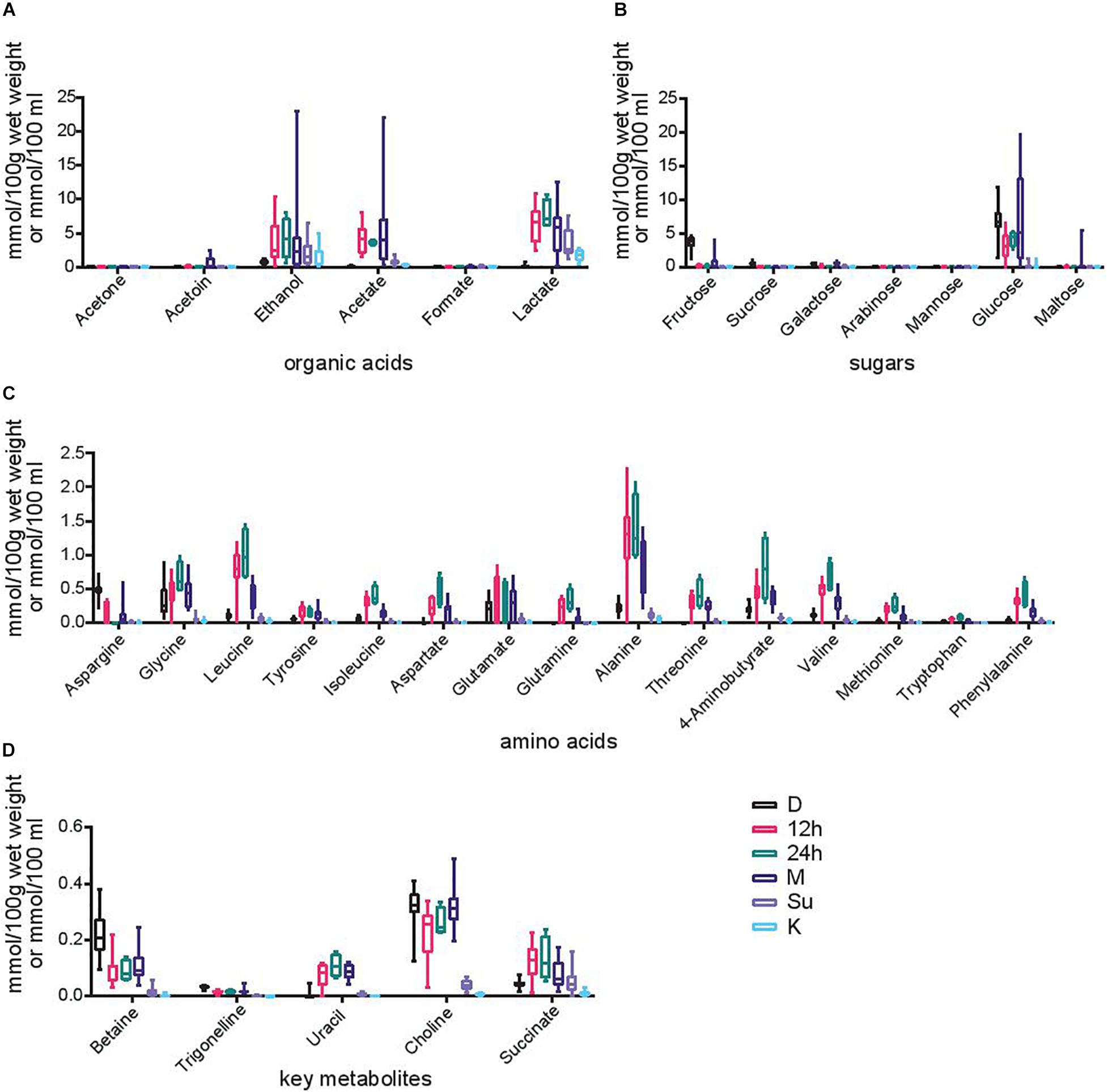
Figure 6. Metabolite profiles. Box plots of the concentration of various metabolites expressed in mmol per 100 g of wet weight for grains, 12 h, 24 h, and milled samples or mmol per 100 ml for supernatant and Hausa koko samples. Each box represents the 5th to 95th percentile. Each panel represents a different category of metabolites produced at different time points (dry, 12 h, 24 h, milled, supernatant, and koko) during Hausa koko production. (A) Organic acids, (B) sugars, (C) amino acids, and (D) key metabolites.
Another group of metabolites of importance identified at the various stages were amino acids (Figure 6C). The amino acid concentrations generally increased from the dry grains to the fermenting time points and reduced in the later stages of production, particularly in both supernatant and Hausa koko samples. Significant increase between grains and the 12- and 24-h fermentation points were detected for almost all of them (p < 0.0001 for leucine, isoleucine, aspartate, alanine, threonine, valine, and phenylalanine; p = 0.0007 for glutamate, p = 0.0002 for glutamine, and p = 0.0137 for methionine), although the concentration of glycine only increased significantly after 24 h (p = 0.0003) and asparagine decreased between these time points (p = 0.0001). Between the fermentation and milling of the samples, the concentration of some amino acids decreased significantly (p < 0.0001 for leucine, alanine, and valine; p = 0.0239 for glutamine), while for others, the decrease in concentration was observed in the supernatant or koko samples (p < 0.0001 for glycine, isoleucine, aspartate, alanine, threonine, and phenylalanine; p = 0.026 for tyrosine; p = 0.0005 for aspartate; p = 0.002 for glutamine; p = 0.02 for methionine).
Other metabolites not included in previous groups were detected at very low concentrations: betaine, trigonelline, uracil, choline, and succinate. Betaine and choline decreased after the first 12 and 24 h of fermentation (p < 0.0001) while uracil and succinate increased (p < 0.0001). The concentration of these compounds was maintained in the milled samples and dramatically decreased in supernatant and koko samples (p < 0.0001).
Discussion
Hausa koko is one of the most popular fermented foods consumed in Ghana. It is an affordable and nutritious porridge for all people. Understanding the microbial community dynamics and the metabolic changes during the production of this fermented porridge is needed to design starter cultures to perform safer, standardized, and scalable production batches. In this study, we used culture-independent high-throughput sequencing of the 16S rRNA gene to study the bacterial diversity of Hausa koko, showing a high species richness at the different time points from the different geographical sampling locations. Significant differences were observed between the different time points during the production process.
The grain samples, used as the raw material to produce the Hausa koko, recorded more OTUs and higher relative abundance of the genus Pantoea compared to the other time points. This genus of Gram-negative bacteria of the Enterobacteriaceae family is usually isolated from several sources and ecological niches including plants (Kini et al., 2018; Azizi et al., 2020) and they are most probably associated with the original plant material and the soil environment (Sarita and Singh, 2016). The grain samples were also dominated by other Gram-negative microbes including Pseudomonas, Enterobacteriaceae, and Escherichia-Shigella, some of which may be potential pathogens and are commonly associated with feces, soil, and intestinal tracts of humans and warm-blooded animals and may cause gastroenteritis, diarrhea, vomiting, and nausea (Gadaga et al., 2004, 2008).
As expected, the fermentation stages presented a major shift to mainly fermentation-related genera dominated by lactic acid bacteria (LAB), which were detected already after 12 h of fermentation. A significant increase in the relative abundance of the genus Limosilactobacillus (formerly included in the Lactobacillus genus) (Zheng et al., 2020) was observed and other genera became dominant, including Pediococcus, Weissella, Lactococcus, Streptococcus, Leuconostoc, and other genera within the lactobacilli group, such as Lactobacillus and Ligilactobacillus. Consequently, an increase in the concentration of organic acids produced by the fermenting microbes was observed and a subsequent decrease in the population of Pantoea spp. was noticed. Shifts in the acidity after 24 to 48 h of fermentation of maize dough, used in the preparation of three popular Ghanaian traditional foods, have been reported (Halm et al., 2004). These organic acids, lactic acid in particular, are key fermentation features, important for both organoleptic qualities and pathogen reduction (Oguntoyinbo and Narbad, 2015). Sour foods are popular in Ghana, and in all such sour foods, the role of LAB has been demonstrated (Amoa-Awua et al., 1996; Obilie et al., 2004; Obodai and Dodd, 2006; Atter et al., 2014; Annan et al., 2015). The relative abundance of LAB populations increased along the fermentation stages from 12 to 24 h, M, Su, and Sd. The population, however, was reduced in the final porridge, which may be attributed primarily to application of heat. Acetic acid bacteria, Acetobacter and Gluconobacter genera, were also present in varying relative abundances at the different time points in the Hausa koko production process. Although not significant, some of the Hausa koko samples contained a higher relative abundance of acetic acid bacteria. Acetic acid bacteria produce mainly acetic acid, some vitamin C, and cellulose during spontaneous fermentation of foods and beverages (De Roos and De Vuyst, 2018). Their presence has been reported in other African cereal fermented foods including kunu and burukutu (Oguntoyinbo, 2014; Ezekiel et al., 2019). They are responsible for oxidation of ethanol produced during fermentation to acetic acid (Gómez-Manzo et al., 2010; Ezekiel et al., 2019).
Generally, significant differences did not occur between most of the samples within the same time point from the different regions or within different processing facilities of the same region, although some significant differences were detected in samples from the Northern and Greater Accra region, which could be attributed to the microbial communities of the millet substrates, as they may vary depending on the source. Additionally, the quality of water, spices, utensils, contact surfaces, and hands may influence these differences (Gadaga et al., 2008). The significant difference in the samples from the Northern region and the other five regions was due to OTUs within the genus Lactobacillus while the differences in the samples from the Greater Accra region seem to be attributed to OTUs within the Acetobacter genus. This study did not find any previous observations of regional differences in African cereal fermented foods. Therefore, further studies would be needed to determine what is causing those differences.
In this study, we selected a subset of five samples from three of the main regions, to characterize the fungal populations. Yeasts are the most common microorganisms apart from bacteria in spontaneous cereal fermentations (Achi and Ukwuru, 2015). Synergism occurs between bacteria and yeast in fermentation niches, with acidification of the medium by bacteria supporting yeast growth and subsequent release of amino acids and vitamins (Stadie et al., 2013). Factors such as the raw materials used, processing operations including duration and temperature of fermentation, hygienic practices, interactions between the microbes, and their successions, which are influenced by intrinsic and extrinsic growth factors, may influence the diversity of yeast in Hausa koko (Jespersen, 2003; Achi and Ukwuru, 2015; Johansen et al., 2019). The fungal community at the different stages of Hausa koko production was profiled using the ITS sequence data. Although most of the grain samples had to be removed in the diversity analysis due to the low number of reads obtained in the sample, the two samples that could be retained showed a highly diverse population of molds and yeast genera in the grains that are likely to be soil and grass inhabitants with very little Saccharomyces. In some of the grain samples, there was a high abundance of OTUs that could not be classified. The dominance of soil- and grass-associated fungi was drastically reduced in the fermentation time point samples at 12 h, 24 h, supernatants, and sediments. There was an increase in the relative abundance of the genera Saccharomyces and Pichia. Some of the samples were dominated by the genus Kluyveromyces. This trend may be attributed to acidification of the samples at these time points and microbial succession, which may have led to the inhibition of growth of some of these fungi while promoting the growth of others (Achi and Ukwuru, 2015). These time points were significantly different from the others due to OTUs classified as S. cerevisiae, which are predominant in indigenous African fermented cereal foods (Achi and Ukwuru, 2015; Johansen et al., 2019). The presence of S. sansevieriae and M. restricta in some of the milled samples have not been reported in indigenous African fermented foods yet. The genus Pichia is another frequently occurring yeast in fermented cereals and reported extensively. Pichia kudriavzevii was reported in another spontaneously fermented pearl millet product, fura, in West Africa (Pedersen et al., 2012), ogi (Omemu et al., 2007), and gowé (Greppi et al., 2013). K. marxianus is one of the promising yeast species isolated from fermented foods with beneficial characteristics and has been associated with spontaneous fermentation in West Africa (Karim et al., 2020; Motey et al., 2020). All the yeasts profiled at the different time points may contribute to production of aroma compounds from different carbon sources, mycotoxin degradation, increase in shelf life, safety, and nutritional value of Hausa koko (Forti et al., 2018; Johansen et al., 2019). Although significant reads from both bacteria and fungi were obtained from the K samples, cooking of the final Hausa koko porridge by addition of boiling water is expected to affect the number of live microbes in the final product. This could potentially reduce the level of most contaminants, potential pathogens, and aflatoxin-producing fungi that started the fermentation, making Hausa koko safer. It has been established that even though cooking of fermented cereal porridges actually reduces the antimicrobial effect of LAB and yeast on pathogens, significant inhibition of these pathogens still occurs (Mensah et al., 1991; Mensah, 1997).
The presence and interactions between the wide array of microbes at the various stages of Hausa koko production yielded different metabolites following the conversion of carbohydrates in accordance to the principles of fermentation (Hagman and Piškur, 2015). These metabolites were produced by the various fermenting microbes, predominantly LAB and yeast during this mixed fermentation process (Ohimain, 2016). Inconsistent trends in the sugar concentrations with a general increase in metabolic products of LAB and yeast was observed. There was a general reduction in the sugar concentrations along the processing time points. The milling breaks down the grains and makes the carbohydrates more accessible to the enzymes produced during fermentation. These enzymes then break down the complex carbohydrates into simple sugars that are utilized by the fermenting microbes for energy (mainly glycolysis). The spices may also have contributed to the increase in sugars. The high concentrations of sugars dominated by glucose in the milled samples make them ideal substrates and carbon source for use by fermenting microbes for growth (Charalampopoulos et al., 2002; Di Stefano et al., 2017). The production of organic acids such as lactate, acetate, and ethanol progressed steadily along the fermentation stages peaking in the milled millet samples. Dilution of the milled millet with water resulted in a decrease in their concentrations in the supernatants and Hausa koko samples. Other organic acids occurred in small concentrations. The presence of such organic metabolites is indicative of the involvement of heterofermentative LAB and yeast (Michodjèhoun-Mestres et al., 2005) and contributes to the flavor, taste, and sensorial properties of the final product (Sripriya et al., 1997; Onyango et al., 2000; Akpinar-Bayizit et al., 2010; Weldemichael et al., 2019). Amino acid concentrations in the grain samples were generally low but increased marginally in the 12-h fermented millet and milled samples (Mbithi-Mwikya et al., 2000; Adebiyi et al., 2017). This could be attributed to increased hydrolytic enzyme activities from the grains as well as breakdown of complex proteins to amino acids (Saleh et al., 2013). The concentration of some amino acids was reduced in the milled samples, but most of them decreased in the supernatant samples, which could be due to a combination of factors: the consumption by the microorganisms in order to multiply and the dilution with water in the supernatant and Hausa koko samples. Other important metabolites including betaine and trigonelline were profiled at the various time points of Hausa koko production. Whole grain cereals are excellent dietary sources for betaine and its precursor choline, which are associated with amino acid and lipid metabolism (Bruce et al., 2010; Ross et al., 2014). Trigonelline has been described as possessing anti-diabetic properties and decreasing blood cholesterol level, and is used for treating migraine, cancer, and other conditions (Basch et al., 2003; Bahmani et al., 2016).
The different metabolites profiled in the current study as a result of fermentation by the various microbes may contribute to the flavor and aroma of Hausa koko, similar to compounds developed during fermentation of other products (Salmerón et al., 2014; Weldemichael et al., 2019). These metabolites, including organic compounds, are antimicrobial substances involved in inhibiting the proliferation and survival of potential pathogens and other contaminants. Their presence contributes to the safety of the product and reduces incidence of diarrhea and other food borne diseases (Achi and Ukwuru, 2015; Adebo et al., 2017).
Conclusion
Significant differences were observed in bacterial diversity at the different time points of Hausa koko production across the six regions. The grain samples were more diverse with high abundance of the genus Pantoea. Lactobacilli, however, increased steadily as fermentation progressed during the production stages, peaking in the sediment samples but reduced in the final Hausa koko as a result of dilution with water and application of heat. There were few differences in diversity between regions. The Northern region samples were different from the other regions due to OTUs within the genus Lactobacillus. ITS sequence data also revealed a high population of fungal genera in the grains including known contaminants, and soil and grass inhabitants with many unidentified. In general, the most abundant yeast during Hausa koko fermentation was the genus Saccharomyces. This may be the first time S. sansevieriae and M. restricta are reported in indigenous African fermented foods. Hausa koko samples recorded OTUs of the genera Pichia, Malassezia, Cyberlindnera, Kluyveromyces, and Saccharomyces and were differentiated from other time points by C. fabianii and M. restricta. The metabolomics study using NMR unveiled the profile of metabolites that were produced during Hausa koko fermentation. From the profile obtained, it can be suggested that traditional fermentation of Hausa koko undergoes the typical shifts from the fermentation process but the concentration of most of the organic acids and amino acids is drastically reduced in the final product that is consumed.
Data Availability Statement
The sequencing datasets generated in this study can be found in the SRA database under the BioProject accession number PRJNA70037.
Author Contributions
AA collected the samples, performed the DNA and metabolite extractions, interpreted the data, and drafted the original manuscript. MD supervised the work, performed bioinformatic and statistical analysis, interpreted the data, and contributed to the manuscript writing. KT-D and AK contributed to supervision and review and editing. MM and AN contributed to conceptualization, funding acquisition, supervision, and review and editing of the manuscript. IC performed nuclear magnetic resonance spectroscopy. DB sequenced the yeast samples. DN reviewed and edited the manuscript. WA-A contributed to conceptualization, supervision, and review and editing. All authors contributed to the article and approved the submitted version.
Funding
This work was supported by the UK Biotechnology and Biological Sciences Research Council (BBSRC) via a Global Challenges Research Fund Data and Resources award and Institute Strategic Programmes for Food Innovation and Health (BB/R012512/1, and its constituent projects Theme 1 BBS/E/F/000PR10343 and Theme 3 BBS/E/F/000PR10346) and Gut Microbes and Health (BB/R012490/1, Theme 3 BBS/E/F/000PR10356). MD was the beneficiary of a Clarin COFUND outgoing grant (ACA17-16) co-funded by the 7th WP of the European Union, Marie Curie Actions, and the FICyT Foundation.
Conflict of Interest
The authors declare that the research was conducted in the absence of any commercial or financial relationships that could be construed as a potential conflict of interest.
Publisher’s Note
All claims expressed in this article are solely those of the authors and do not necessarily represent those of their affiliated organizations, or those of the publisher, the editors and the reviewers. Any product that may be evaluated in this article, or claim that may be made by its manufacturer, is not guaranteed or endorsed by the publisher.
Acknowledgments
The authors acknowledge Steve James and Andrea Telatin (Quadram Institute Bioscience) for their suggestions on the selection of methods for fungal diversity analysis.
Supplementary Material
The Supplementary Material for this article can be found online at: https://www.frontiersin.org/articles/10.3389/fmicb.2021.681983/full#supplementary-material
References
Achi, O. K., and Ukwuru, M. (2015). Cereal-based fermented foods of Africa as functional foods. Int. J. Microbiol. Appl. 2, 71–83. doi: 10.1007/978-3-319-54528-8_31-1
Adebiyi, J. A., Obadina, A. O., Adebo, O. A., and Kayitesi, E. (2017). Comparison of nutritional quality and sensory acceptability of biscuits obtained from native, fermented, and malted pearl millet (Pennisetum glaucum) flour. Food Chem. 232, 210–217. doi: 10.1016/j.foodchem.2017.04.020
Adebo, O. A., Njobeh, P. B., Adebiyi, J. A., Gbashi, S., and Kayitesi, E. (2017). “Food metabolomics: a new frontier in food analysis and its application to understanding fermented foods,” in Functional Food-Improve Health Through Adequate Food, ed. M. C. Hueda (Rijeka: INTECH). doi: 10.5772/intechopen.69171
Akpinar-Bayizit, A., Yilmaz-Ersan, L., and Ozcan, T. (2010). Determination of boza’s organic acid composition as it is affected by raw material and fermentation. Int. J. Food Prop. 13, 648–656. doi: 10.1080/10942911003604194
Amoa-Awua, W. K., Ngunjiri, P., Anlobe, J., Kpodo, K., and Halm, M. (2007). The effect of applying GMP and HACCP to traditional food processing at a semi-commercial kenkey production plant in Ghana. Food Control 18, 1449–1457. doi: 10.1016/j.foodcont.2006.10.009
Amoa-Awua, W. K. A., Appoh, F. E., and Jakobsen, M. (1996). Lactic acid fermentation of cassava dough into agbelima. Int. J. Food Microbiol. 31, 87–98. doi: 10.1016/0168-1605(96)00967-1
Anderson, M. J. (2017). “Permutational multivariate analysis of variance (PERMANOVA),” in Wiley StatsRef: Statistics Reference Online, eds N. Balakrishnan, T. Colton, B. Everitt, W. Piegorsch, F. Ruggeri, and J. L. Teugels (Chichester: John Wiley and Sons Ltd), 1–15. doi: 10.1002/9781118445112.stat07841
Annan, T., Obodai, M., Anyebuno, G., Tano-debrah, K., and Amoa-awua, K. (2015). Characterization of the dominant microorganisms responsible for the fermentation of dehulled maize grains into nsiho in Ghana. Afr. J. Biotechnol. 14, 1640–1648. doi: 10.5897/AJB2014.14134
Arena, M. P., Russo, P., Capozzi, V., López, P., Fiocco, D., and Spano, G. (2014). Probiotic abilities of riboflavin-overproducing Lactobacillus strains: a novel promising application of probiotics. Appl. Microbiol. Biotechnol. 98, 7569–7581. doi: 10.1007/s00253-014-5837-x
Atter, A., Obiri-Danso, K., and Amoa-Awua, W. K. (2014). Microbiological and chemical processes associated with the production of burukutu a traditional beer in Ghana. Int. Food Res. J. 21, 1769–1775.
Azizi, M. M. F., Ismail, S. I., Ina-Salwany, M. Y., Hata, E. M., and Zulperi, D. (2020). The emergence of Pantoea species as a future threat to global rice production. J. Plant Prot. Res. 60, 327–335. doi: 10.24425/jppr.2020.133958
Bahmani, M., Shirzad, H., Mirhosseini, M., Mesripour, A., and Rafieian-Kopaei, M. (2016). A review on ethnobotanical and therapeutic uses of fenugreek (Trigonella foenum-graceum L). Evid. Based Complementary Altern. Med. 21, 53–62. doi: 10.1177/2156587215583405
Basch, E., Ulbricht, C., Kuo, G., Szapary, P., and Smith, M. (2003). Therapeutic applications of fenugreek. Altern. Med. Rev. 81, 20–27.
Blandino, A., Al-Aseeri, M. E., Pandiella, S. S., Cantero, D., and Webb, C. (2003). Cereal-based fermented foods and beverages. Food Res. Int. 36, 527–543. doi: 10.1016/S0963-9969(03)00009-7
Bolyen, E., Rideout, J. R., Dillon, M. R., Bokulich, N. A., Abnet, C., Al-Ghalith, G. A., et al. (2019). Reproducible, interactive, scalable and extensible microbiome data science using QIIME 2. Nat. Biotechnol. 37, 852–857. doi: 10.1038/s41587-019-0209-9
Bruce, S. J., Guy, P. A., Rezzi, S., and Ross, A. B. (2010). Quantitative measurement of betaine and free choline in plasma, cereals and cereal products by isotope dilution LC-MS/MS. J. Agric. Food Chem. 58, 2055–2061. doi: 10.1021/jf903930k
Caporaso, J. G., Lauber, C. L., Walters, W. A., Berg-Lyons, D., Lozupone, C. A., Turnbaugh, P. J., et al. (2011). Global patterns of 16S rRNA diversity at a depth of millions of sequences per sample. Proc. Natl. Acad. Sci. U.S.A. 108, 4516–4522. doi: 10.1073/pnas.1000080107
Charalampopoulos, D., Wang, R., Pandiella, S. S., and Webb, C. (2002). Application of cereals and cereal components in functional foods: a review. Int. J. Food Microbiol. 79, 131–141. doi: 10.1016/S0168-1605(02)00187-3
Das, A., Raychaudhuri, U., and Chakraborty, R. (2012). Cereal based functional food of Indian subcontinent: a review. J. Food Sci. Technol. 49, 665–672. doi: 10.1007/s13197-011-0474-1
De Roos, J., and De Vuyst, L. (2018). Acetic acid bacteria in fermented foods and beverages. Curr. Opin. Biotechnol. 49, 115–119. doi: 10.1016/j.copbio.2017.08.007
Di Stefano, E., White, J., Seney, S., Hekmat, S., McDowell, T., Sumarah, M., et al. (2017). A novel millet-based probiotic fermented food for the developing world. Nutrients 9:529. doi: 10.3390/nu9050529
Diaz, M., Kellingray, L., Akinyemi, N., Adefiranye, O. O., Olaonipekun, A. B., Bayili, G. R., et al. (2019). Comparison of the microbial composition of African fermented foods using amplicon sequencing. Sci. Rep. 9:13863. doi: 10.1038/s41598-019-50190-4
Ezekiel, C. N., Ayeni, K. I., Ezeokoli, O. T., Sulyok, M., van Wyk, D. A., Oyedele, O. A., et al. (2019). High-throughput sequence analyses of bacterial communities and multi-mycotoxin profiling during processing of different formulations of Kunu, a traditional fermented beverage. Front. Microbiol. 9:3282. doi: 10.3389/fmicb.2018.03282
Forti, L., Cramarossa, M. R., Filippucci, S., Tasselli, G., Turchetti, B., and Buzzini, P. (2018). “Nonconventional Yeast-Promoted Biotransformation for the Production of Flavor Compounds,” in Natural and Artificial Flavoring Agents and Food Dyes, eds A. M. Grumezescu and A. M. Holbaned (Cambridge, MA: Academic Press), 165–187. doi: 10.1016/B978-0-12-811518-3.00006-5
Gadaga, T. H., Nyanga, L. K., and Mutukumira, A. N. (2004). The occurrence, growth and control of pathogens in African fermented foods. Afr. J. Food Agric. Nutr. Dev. 4, 406–413.
Gadaga, T. H., Samende, B. K., Musuna, C., and Chibanda, D. (2008). The microbiological quality of informally vended foods in Harare, Zimbabwe. Food Control 19, 829–832. doi: 10.1016/j.foodcont.2007.07.016
Gómez-Manzo, S., Chavez-Pacheco, J. L., Contreras-Zentella, M., Sosa-Torres, M. E., Arreguín-Espinosa, R., De La Mora, M. P., et al. (2010). Molecular and catalytic properties of the aldehyde dehydrogenase of Gluconacetobacter diazotrophicus, a quinoheme protein containing pyrroloquinoline quinone, cytochrome b, and cytochrome c. J. Bacteriol. 192, 5718–5724. doi: 10.1128/JB.00589-10
Greppi, A., Rantsiou, K., Padonou, W., Hounhouigan, J., Jespersen, L., Jakobsen, M., et al. (2013). Determination of yeast diversity in ogi, mawè, gowé and tchoukoutou by using culture-dependent and-independent methods. Int. J. Food Microbiol. 165, 84–88. doi: 10.1016/j.ijfoodmicro.2013.05.005
Hagman, A., and Piškur, J. (2015). A study on the fundamental mechanism and the evolutionary driving forces behind aerobic fermentation in yeast. PLoS One 10:e0116942. doi: 10.1371/journal.pone.0116942
Haleegoah, J. A. S., Ruivenkamp, G., Essegbey, G., Frempong, G., and Jongerden, J. (2016). Street-vended local foods transformation: case of hausa koko, waakye and ga kenkey in urban Ghana. Adv. Appl. Sociol. 6, 90–100. doi: 10.4236/aasoci.2016.63009
Halm, M., Amoa-Awua, W. K., and Jakobsen, M. (2004). “Kenkey: an African fermented maize product. Chap. 44,” in Handbook of Food and Beverage Fermentation Technology, eds Y. H. Hui, L. Meunier-Goddik, J. Josephsen, W. K. Nip, and P. S. Stanfield (New York, NY: CRC Press), 799–816. doi: 10.1201/9780203913550
Halm, M., Osei-Yaw, A., Kpodo, K., and Amoa-Awua, W. K. (1996). Experiences with the use of starter culture for the fermentation of maize. World J. Microbiol. Biotechnol. 19, 135–143.
Holzapfel, W. H. (2002). Appropriate starter culture technologies for small-scale fermentation in developing countries. Int. J. Food Microbiol. 75, 197–212. doi: 10.1016/S0168-1605(01)00707-3
Ibnouf, F. O. (2012). The value of women’s indigenous knowledge in food processing and preservation for achieving household food security in rural Sudan. J. Food Res. 1:238. doi: 10.5539/jfr.v1n1p238
Jackson, C. J., Barton, R. C., and Evans, E. G. V. (1999). Species identification and strain differentiation of dermatophyte fungi by analysis of ribosomal-DNA intergenic spacer regions. J. Clin. Microbiol. 37, 931–936. doi: 10.1128/JCM.37.4.931-936.1999
Jespersen, L. (2003). Occurrence and taxonomic characteristics of strains of Saccharomyces cerevisiae predominant in African indigenous fermented foods and beverages. FEMS Yeast Res. 3, 191–200. doi: 10.1016/S1567-1356(02)00185-X
Johansen, P. G., Owusu-Kwarteng, J., Parkouda, C., Padonou, S. W., and Jespersen, L. (2019). Occurrence and importance of yeasts in indigenous fermented food and beverages produced in sub-Saharan Africa. Front. Microbiol. 10:1789. doi: 10.3389/fmicb.2019.01789
Karim, A., Gerliani, N., and Aïder, M. (2020). Kluyveromyces marxianus: an emerging yeast cell factory for applications in food and biotechnology. Int. J. Food Microbiol. 333:108818. doi: 10.1016/j.ijfoodmicro.2020.108818
Kini, K., Agnimonhan, R., Dossa, R., Silué, D., and Koebnik, R. (2018). A diagnostic multiplex PCR scheme for identification of plant-associated bacteria of the genus Pantoea. bioRχiv [Preprint]. doi: 10.1101/456806
Lei, V., Friis, H., and Michaelsen, K. F. (2006). Spontaneously fermented millet product as a natural probiotic treatment for diarrhoea in young children: an intervention study in Northern Ghana. Int. J. Food Microbiol. 110, 246–253. doi: 10.1016/j.ijfoodmicro.2006.04.022
Lei, V., and Jakobsen, M. (2004). Microbiological characterization and probiotic potential of koko and koko sour water, African spontaneously fermented millet porridge and drink. J. Appl. Microbiol. 96, 384–397. doi: 10.1046/j.1365-2672.2004.02162.x
Mandal, S., Van Treuren, W., White, R. A., Eggesbø, M., Knight, R., and Peddada, S. D. (2015). Analysis of composition of microbiomes: a novel method for studying microbial composition. Microb. Ecol. Health Dis. 26:27663. doi: 10.3402/mehd.v26.27663
Mbithi-Mwikya, S., Ooghe, W., Van Camp, J., Ngundi, D., and Huyghebaert, A. (2000). Amino acid profiles after sprouting, autoclaving, and lactic acid fermentation of finger millet (Eleusine coracan) and kidney beans (Phaseolus vulgaris L.). J. Agric. Food Chem. 48, 3081–3085. doi: 10.1021/jf0002140
Mensah, P. (1997). Fermentation—the key to food safety assurance in Africa? Food Control 8, 271–278. doi: 10.1016/S0956-7135(97)00020-0
Mensah, P., Tomkins, A. M., Drasar, B. S., and Harrison, T. J. (1991). Antimicrobial effect of fermented Ghanaian maize dough. J. Appl. Microbiol. 70, 203–210. doi: 10.1111/j.1365-2672.1991.tb02925.x
Mensah, P., Yeboah-Manu, D., Owusu-Darko, K., and Ablordey, A. (2002). Street foods in Accra, Ghana: how safe are they? Bull. World Health Organ. 80, 546–554.
Michodjèhoun-Mestres, L., Hounhouigan, J. D., Dossou, J., and Mestres, C. (2005). Physical, chemical and microbiological changes during natural fermentation of “gowé”, a sprouted or non-sprouted sorghum beverage form West-Africa. Afr. J. Biotechnol. 4, 487–496.
Motey, G. A., Johansen, P. G., Owusu-Kwarteng, J., Ofori, L. A., Obiri-Danso, K., Siegumfeldt, H., et al. (2020). Probiotic potential of Saccharomyces cerevisiae and Kluyveromyces marxianus isolated from West African spontaneously fermented cereal and milk products. Yeast 37, 403–412. doi: 10.1002/yea.3513
Obilie, E. M., Tano-Debrah, K., and Amoa-Awua, W. K. (2004). Souring and breakdown of cyanogenic glucosides during the processing of cassava into akyeke. Int. J. Food Microbiol. 93, 115–121. doi: 10.1016/j.ijfoodmicro.2003.11.006
Obodai, M., and Dodd, C. E. R. (2006). Characterization of dominant microbiota of a Ghanaian fermented milk product, nyarmie, by culture-and nonculture-based methods. J. Appl. Microbiol. 100, 1355–1363. doi: 10.1111/j.1365-2672.2006.02895.x
Oguntoyinbo, F. A. (2014). Safety challenges associated with traditional foods of West Africa. Food Rev. Int. 30, 338–358. doi: 10.1080/87559129.2014.940086
Oguntoyinbo, F. A., and Narbad, A. (2015). Multifunctional properties of Lactobacillus plantarum strains isolated from fermented cereal foods. J. Funct. Foods. 17, 621–631. doi: 10.1016/j.jff.2015.06.022
Ohimain, E. I. (2016). Methanol contamination in traditionally fermented alcoholic beverages: the microbial dimension. Springerplus 5:1607. doi: 10.1186/s40064-016-3303-1
Omemu, A. M., Bankole, M. O., Oyewole, O. B., and Akintokun, A. K. (2007). Yeasts and moulds associated with ogi–a cereal based weaning food during storage. Res. J. Microbiol. 2, 141–148. doi: 10.3923/jm.2007.141.148
Omemu, A. M., and Omeike, S. O. (2010). Microbiological hazard and critical control points identification during household preparation of cooked ogi used as weaning food. Int. Food Res. J. 17, 257–266.
Onyango, C., Okoth, M. W., and Mbugua, S. K. (2000). Effect of drying lactic fermented uji (an East African sour porridge) on some carboxylic acids. J. Sci. Food Agric. 80, 1854–1858. doi: 10.1002/1097-0010(200010)80
Owusu-Kwarteng, J., Akabanda, F., and Glover, R. L. K. (2010a). Effect of soybean fortification on fermentation characteristics and consumer acceptability of Hausa koko, a Ghanaian fermented porridge. J. Appl. Biosci. 28, 1712–1717.
Owusu-Kwarteng, J., Tano-Debrah, K., Glover, R. L. K., and Akabanda, F. (2010b). Process characteristics and microbiology of fura produced in Ghana. Nat. Sci. 8, 41–51.
Pedersen, L. L., Owusu-Kwarteng, J., Thorsen, L., and Jespersen, L. (2012). Biodiversity and probiotic potential of yeasts isolated from Fura, a West African spontaneously fermented cereal. Int. J. Food Microbiol. 159, 144–151. doi: 10.1016/j.ijfoodmicro.2012.08.016
Ray, M., Ghosh, K., Singh, S., and Mondal, K. C. (2016). Folk to functional: an explorative overview of rice-based fermented foods and beverages in India. J. Ethn. Foods. 3, 5–18. doi: 10.1016/j.jef.2016.02.002
Ross, A. B., Zangger, A., and Guiraud, S. P. (2014). Cereal foods are the major source of betaine in the Western diet–analysis of betaine and free choline in cereal foods and updated assessments of betaine intake. Food Chem. 145, 859–865. doi: 10.1016/j.foodchem.2013.08.122
Saleh, A. S., Zhang, Q., Chen, J., and Shen, Q. (2013). Millet grains: nutritional quality, processing, and potential health benefits. Compr. Rev. Food Sci. Food Saf. 12, 281–295. doi: 10.1111/1541-4337.12012
Salmerón, I., Rozada, R., Thomas, K., Ortega-Rivas, E., and Pandiella, S. S. (2014). Sensory characteristics and volatile composition of a cereal beverage fermented with Bifidobacterium breve NCIMB 702257. Food Sci. Technol. Int. 20, 205–213. doi: 10.1177/1082013213481466
Sarita, E. S., and Singh, E. (2016). Potential of millets: nutrients composition and health benefits. J. Sci. Innov. Res. 5, 46–50.
Sharma, A., and Kapoor, A. C. (1996). Levels of antinutritional factors in pearl millet as affected by processing treatments and various types of fermentation. Plant Foods Hum Nutr. 49, 241–252. doi: 10.1007/BF01093221
Sindhu, S. C., and Khetarpaul, N. (2001). Probiotic fermentation of indigenous food mixture: effect on antinutrients and digestibility of starch and protein. J. Food Compos. Anal. 14, 601–609. doi: 10.1006/jfca.2001.1022
Sripriya, G., Antony, U., and Chandra, T. S. (1997). Changes in carbohydrate, free amino acids, organic acids, phytate and HCl extractability of minerals during germination and fermentation of finger millet (Eleusine coracana). Food Chem. 58, 345–350. doi: 10.1016/S0308-8146(96)00206-3
Stadie, J., Gulitz, A., Ehrmann, M. A., and Vogel, R. F. (2013). Metabolic activity and symbiotic interactions of lactic acid bacteria and yeasts isolated from water kefir. Food Microbiol. 35, 92–98. doi: 10.1016/j.fm.2013.03.009
Tano-Debrah, K., Saalia, F. K., Ghosh, S., and Hara, M. (2019). Development and sensory shelf-life testing of KOKO plus: a food supplement for improving the nutritional profiles of traditional complementary foods. Food Nutr. Bull. 40, 340–356. doi: 10.1177/0379572119848290
Taylor, D. L., Walters, W. A., Lennon, N. J., Bochicchio, J., Krohn, A., Caporaso, J. G., et al. (2016). Accurate estimation of fungal diversity and abundance through improved lineage-specific primers optimized for Illumina amplicon sequencing. Appl. Environ. Microbiol. 82, 7217–7226. doi: 10.1128/AEM.02576-16
Vasiljevic, T., and Shah, N. P. (2008). Probiotics—from Metchnikoff to bioactives. Int. Dairy J. 18, 714–728. doi: 10.1016/j.idairyj.2008.03.004
Wang, C. Y., Wu, S. J., and Shyu, Y. T. (2014). Antioxidant properties of certain cereals as affected by food-grade bacteria fermentation. J. Biosci. Bioeng. 117, 449–456. doi: 10.1016/j.jbiosc.2013.10.002
Weldemichael, H., Stoll, D., Weinert, C., Berhe, T., Admassu, S., Alemu, M., et al. (2019). Characterization of the microbiota and volatile components of kocho, a traditional fermented food of Ethiopia. Heliyon 5:e01842. doi: 10.1016/j.heliyon.2019.e01842
White, T. J., Bruns, T., Lee, S. J. W. T., and Taylor, J. (1990). “Amplification and direct sequencing of fungal ribosomal RNA genes for phylogenetics,” in PCR protocols: A Guide to Methods and Applications, Vol 18, eds Gelfand MAIDH, J. J. Sninsky and T. J. White (New York, NY: Academic Press), 315–322 doi: 10.1016/b978-0-12-372180-8.50042-1
Yeleliere, E., Cobbina, S. J., and Abubakari, Z. I. (2017). Review of microbial food contamination and food hygiene in selected capital cities of Ghana. Cogent Food Agric. 3:1395102. doi: 10.1080/23311932.2017.1395102
Zannini, E., Pontonio, E., Waters, D. M., and Arendt, E. K. (2012). Applications of microbial fermentations for production of gluten-free products and perspectives. Appl. Microbiol. Biotechnol. 93, 473–485. doi: 10.1007/s00253-011-3707-3
Zheng, J., Wittouck, S., Salvetti, E., Franz, C. M., Harris, H. M., Mattarelli, P., et al. (2020). A taxonomic note on the genus Lactobacillus: description of 23 novel genera, emended description of the genus Lactobacillus Beijerinck 1901, and union of Lactobacillaceae and Leuconostocaceae. Int. J. Syst. Evol. Microbiol. 70, 2782–2858. doi: 10.1099/ijsem.0.004107
Keywords: fermented cereal, Hausa koko, Africa, metabolomics, bacteria, fungi, millet
Citation: Atter A, Diaz M, Tano-Debrah K, Kunadu AP-H, Mayer MJ, Colquhoun IJ, Nielsen DS, Baker D, Narbad A and Amoa-Awua W (2021) Microbial Diversity and Metabolite Profile of Fermenting Millet in the Production of Hausa koko, a Ghanaian Fermented Cereal Porridge. Front. Microbiol. 12:681983. doi: 10.3389/fmicb.2021.681983
Received: 17 March 2021; Accepted: 07 June 2021;
Published: 04 August 2021.
Edited by:
Rosane Freitas Schwan, Universidade Federal de Lavras, BrazilReviewed by:
Dimitrios Tsaltas, Cyprus University of Technology, CyprusKoshy Philip, University of Malaya, Malaysia
Copyright © 2021 Atter, Diaz, Tano-Debrah, Kunadu, Mayer, Colquhoun, Nielsen, Baker, Narbad and Amoa-Awua. This is an open-access article distributed under the terms of the Creative Commons Attribution License (CC BY). The use, distribution or reproduction in other forums is permitted, provided the original author(s) and the copyright owner(s) are credited and that the original publication in this journal is cited, in accordance with accepted academic practice. No use, distribution or reproduction is permitted which does not comply with these terms.
*Correspondence: Maria Diaz, TWFyaWEuZGlhekBxdWFkcmFtLmFjLnVr; Wisdom Amoa-Awua, d2lzLmFtb2FAZ21haWwuY29t
†These authors have contributed equally to this work