- 1College of Environmental and Resource Sciences, Zhejiang University, Hangzhou, China
- 2Key Laboratory of Organic Pollution Process and Control, Zhejiang University, Hangzhou, China
Iron sulfide (FeS) nanoparticles have great potential in environmental remediation. Using the representative species Dehalococcoides mccartyi strain 195 (Dhc 195), the effect of FeS on trichloroethene (TCE) dechlorination was studied with hydrogen and acetate as the electron donor and carbon source, respectively. With the addition of 0.2 mM Fe2+ and S2–, the dechlorination rate of TCE was enhanced from 25.46 ± 1.15 to 37.84 ± 1.89 μmol⋅L–1⋅day–1 by the in situ formed FeS nanoparticles, as revealed through X-ray diffraction. Comparing the tceA gene copy numbers between with FeS and without FeS, real-time polymerase chain reaction (PCR) indicated that the abundance of the tceA gene increased from (2.83 ± 0.13) × 107 to (4.27 ± 0.21) × 108 copies/ml on day 12. The transcriptional activity of key genes involved in the electron transport chain was upregulated after the addition of FeS, including those responsible for the iron–sulfur cluster assembly protein gene (DET1632) and transmembrane transport of iron (DET1503, DET0685), cobalamin (DET0685, DET1139), and molybdenum (DET1161) genes. Meanwhile, the reverse transcription of tceA was increased approximately five times on the 12th day. These upregulations together suggested that the electron transport of D. mccartyi strain 195 was enhanced by FeS for apparent TCE dechlorination. Overall, the present study provided an eco-friendly and effective method to achieve high remediation efficiency for organohalide-polluted groundwater and soil.
Introduction
Trichloroethene (TCE) has been extensively used as a solvent in pesticides, dry cleaning, and anesthesia medicine (Vamvakas et al., 1998; Chowdhury and Viraraghavan, 2009). It has become one of the most detected organohalides in subsurface water because of improper disposal and leakage. Classified as a carcinogen (group I), TCE poses a threat to human respiratory organs and even human life (Stewart, 2001; Guha et al., 2012). Microbial reduction is a promising technique for TCE remediation. In particular, Dehalococcoides members can completely dechlorinate TCE to the benign product ethene (Maymo’-Gatell et al., 1997; Antoniou et al., 2019; Zhao and He, 2019; Wang et al., 2020).
The respiratory dechlorination of TCE can yield energy for the growth of Dehalococcoides, in which electrons are transferred from hydrogen to organohalides via the membrane-associated electron transport chain (ETC) (Magnuson et al., 2000; Löffler et al., 2013). Because of the lack of genes for the synthesis of quinones and cytochromes, Dehalococcoides uses the complex iron–sulfur molybdoenzyme (CISM) complex to transfer electrons from hydrogenase to reductive dehalogenase (RDase) (Löffler et al., 2013; Kublik et al., 2016). The major components of ETC include hydrogenases, CISM, and RDases (Seshadri et al., 2005; Pinske et al., 2011; Hartwig et al., 2017). The dominant hydrogenase Hup decomposes hydrogen to electrons and transfers these electrons to CISM using the iron–sulfur cluster subunit (Seshadri et al., 2005; Türkowsky et al., 2018). The CISM complex was formerly annotated as formate dehydrogenase containing multiple iron–sulfur clusters for electron transport (Wang et al., 2018). These iron–sulfur clusters are cubic crystal structures [4Fe-4S] and may have a low redox potential. Compared with quinone proteins, the lower redox potential of iron–sulfur clusters could avoid endothermic reactions, such as the reverse transfer of electrons (Löffler et al., 2013; Kublik et al., 2016). However, the accurate molecular components of the CISM complex and its exact functions are still unclear. The terminal electron sink of ETC is RDase, which also contains iron–sulfur clusters to accept electrons from the CISM complex (Schipp et al., 2013; Schubert et al., 2018). RDase is encoded by the tceA gene and catalyzes TCE dechlorination to vinyl chloride (VC) (Mansfeldt et al., 2014). From the ETC components, iron–sulfur clusters are crucial for organohalide reduction throughout the ETC, and close proximity ensures rapid intraprotein or interprotein electron transfer (Costentin et al., 2005; Payne et al., 2015).
Iron sulfide (FeS) has also been studied extensively for the abiotic degradation of chlorinated ethenes (Jeong and Hayes, 2007; He et al., 2015). With a high concentration of FeS (>10 g/L), the degradation products of TCE by FeS vary, including acetylene, cis-dichloroethene (cis-DCE), 1,1-DCE, and VC, under weakly alkaline conditions (Liang et al., 2007; He et al., 2010). However, the dechlorination rate of chlorinated ethenes by FeS was slow compared with biotic dechlorination. Thus, the interaction between FeS and microbes was studied. On the one hand, FeS acts as a naturally occurring electrical wire, bridges spatially discrete environments, and mediates long-distance extracellular electron transfer (Kondo et al., 2015); on the other hand, FeS can enter the periplasm of sulfate-reducing bacteria to accelerate electron transport (Deng et al., 2020). Moreover, the composition of FeS is the same as that of the iron–sulfur clusters. Thus, we hypothesized that FeS nanoparticles could enhance electron transport in Dehalococcoides.
Using the representative species Dehalococcoides mccartyi strain 195 (Dhc 195), we investigated the effects of FeS on both TCE dechlorination and the growth of Dhc 195. The underlying mechanism was explored by X-ray diffraction and transcriptomic analysis. Overall, this study provided an eco-friendly and effective way to improve the bioremediation of organohalides.
Materials and Methods
Materials
TCE (99.5%), cis-DCE (98%), and VC (99%) were purchased from J&K Chemicals (Shanghai, China). FeCl2⋅4H2O and Na2S were purchased from Aladdin (Shanghai, China). All other chemicals were of analytical reagent or guaranteed reagent grade.
Culture and Growth Conditions
Dhc 195 was donated by Jun Yan from the Institute of Applied Ecology, Chinese Academy of Sciences. Dhc 195 was cultivated in a defined mineral salt medium with 10 mM acetate as the carbon source and 2.5 mM H2 as the electron donor. The mineral salt medium was described by Löffler et al. (2005). Briefly, 10 ml of salt solution (specific components not shown), 1 ml of Se/W solution, 1 ml of trace element solution, 0.25 ml of 0.1% (w/v) resazurin stock solution, and 2.292 g of N-[Tris(hydroxymethyl) methyl]-2-aminoethanesulfonic acid were added to 1 L of double-distilled water. The medium was boiled and flushed with N2 to remove oxygen. Meanwhile, 0.242 g of L-cysteine, 0.0771 g of DL-dithiothreitol, and 2.52 g of NaHCO3 were added as reducing agents and buffer, respectively. Then, the medium was transferred into glass serum bottles sealed with butyl rubber stoppers and aluminum crimps. The medium was autoclaved and stored at 30°C. Before each experiment, 1 ml of ATCC vitamin supplement (ATCC MD-VS, United States) and 5 mL of the inoculum culture were transferred into one bottle, and the next steps were the same as described by Li et al. (2019). All cultures were incubated at 30°C in the dark.
Batch Experiments
Batch experiments were performed in 100 mL serum bottles with 50 mL of anaerobic medium (pH ≈ 7.2). Solid-state FeCl2⋅4H2O and Na2S⋅9H2O were purchased from Aladdin (United States). Eighty milligrams of FeCl2⋅4H2O and 96 mg of Na2S⋅9H2O were dissolved in 20 ml of anaerobic water and added to serum bottles with injectors in an anaerobic glove box. Then, Fe2+ and S2– formed black nanoparticles immediately. In all experiments, the sealed bottles were rotated at 200 rpm and 30°C in the dark. All the experimental results are presented as the average values from triplicates (error bars are shown in the associated figures).
Chemical Analysis
Characterization of Chloroethenes
Chloroethenes and ethene were detected by a gas chromatography-flame ionization detector (Agilent Technologies GC system, model 6890N, Agilent Technologies Inc., United States) equipped with a packed column (30 m long, 0.32 mm i.d., 0.5 mM thickness, cross-linked polydimethysiloxane film, J&W Scientific, United States). The detection method was described by Wen et al. (2015, 2020) using 100-μL headspace samples. The oven was kept at 60°C for 2 min, heated gradually to 120°C (20°C/min), and finally kept at 120°C for 2 min. The concentration of chloroethenes was calculated based on gas–liquid equilibrium using Henry’s law constants. The Henry’s law constants of TCE, cis-DCE, VC, and ethene were 0.419, 0.167, 1.137, and 8.71, respectively.
Characterization of Iron Sulfide
The morphology of the formed iron–sulfur nanoparticles was characterized using scanning electron microscopy equipped with energy dispersive X-ray spectrometry (Zeiss Gemini 300, Germany). The crystal structure of iron–sulfur nanoparticles was characterized by X-ray diffraction (Bruker D8 Advance diffractometer, Germany) at the Analysis Center of Agrobiology and Environmental Sciences, Zhejiang University.
Biological Analysis
Characterization of Cellular Morphology
The cellular morphology of Dhc 195 cells was characterized using transmission electron microscopy (JEM-1200EX, Japan). Liquid samples (50 mL) were centrifuged to collect cells, further immobilized by 2.5% glutaraldehyde and stored at 4°C for 12 h. After discarding glutaraldehyde, cells were washed three times with phosphate buffer (pH = 7.0). Osmic acid (1%) was used to immobilize cells again for 1.5 h, followed by three washes with phosphate buffer (pH = 7.0). Then, different concentrations of ethanol solution (30, 50, 70, 80, 90, 95, and 100%) were used for dehydration. Finally, acetone and embedding agent were used to treat cells.
DNA and RNA Extractions
Liquid samples (1.5 ml) obtained without concentrating the cells were centrifuged (21,000 × g, 10 min at 4°C) to collect cells. Chromosomal DNA was extracted using a DNeasy PowerSoil Pro Kit (QIAGEN, Germany) according to the manufacturer’s instructions. RNA was extracted using an E.Z.N. A Soil RNA Mini Kit (OMEGA, United States). Complementary DNA (cDNA) was synthesized using FastKing gDNA Dispelling RT SuperMix (TianGen Biotech, Beijing, China). The cDNA synthesis reaction was performed with an incubation time of 15 min at 42°C followed by 3 min at 95°C in a DNA Engine Peltier Thermal Cycler (Bio-Rad, CA, United States) (Doğan-Subaşı et al., 2014).
Real-Time Polymerase Chain Reaction
The dominant hydrogenase gene hup, formate dehydrogenase gene fdh, and RDase gene tceA were quantified. For hup and fdh, amplification was performed using a Step One Plus Real-Time Polymerase Chain Reaction (PCR) System (Applied Biosystems, United States) with SYBR green-based detection agents (Morris et al., 2006). The reaction solution contained 7.5 μL of SYBR Green mix (DBI Bestar, Ludwigshafen, Germany), 0.5 μL of Rox, 0.5 μL of each primer, 2 μL of DNA template, and 4 μL of double-distilled water. The program was run for 10 min at 95°C for Taq activation, followed by 40 cycles of 15 s at 95°C, 60 s at 55°C and a melting curve stage from 55 to 95°C. For the tceA gene, the method was the same as that described by Ritalahti et al. (2006). The method limit of quantitation was 103 copies/mL. The sequences of primers and probes were synthesized by Sangon Biotech, as shown in Supplementary Table 1. The standard curves and amplification efficiency are shown in Supplementary Table 2.
Differential Gene Expression Assay
For the transcriptomic analysis, cultures were collected for RNA extraction when approximately 75% of TCE was dechlorinated. To collect sufficient material, 24 bottles of Dhc 195 without FeS and 24 bottles of Dhc 195 with FeS (0.2 mM) were inoculated and grown in triplicate. For each setup, cells were collected from eight bottles by centrifugation (21,000 × g, 10 min at 4°C). Then, RNA of two different setups was extracted using an E.Z.N.A. Soil RNA Mini Kit (OMEGA, United States). RNA quality was determined by a 2100 Bioanalyzer (Agilent) and quantified using an ND-2000 (NanoDrop Technologies). Only high-quality RNA samples (OD260/280 = 1.8∼2.0, OD260/230 ≥ 2.0, RIN ≥ 6.5, 28S:18S ≥ 1.0) were used to construct the sequencing library. A Ribo-Zero Magnetic Kit (epicenter) was used to remove ribosomal RNA, and then all messenger RNAs were broken into short fragments (200 bp) by adding fragmentation buffer. After that, a SuperScript double-stranded cDNA synthesis kit (Invitrogen, CA, United States) with random hexamer primers (Illumina) was used to synthesize double-stranded cDNA. Using Phusion DNA polymerase, PCR amplicons after 15 PCR cycles were sequenced with the Illumina HiSeq × TEN (2 × 150 bp read length). The data generated from the Illumina platform were used for bioinformatics analyses as previously described (Barry et al., 2005; Gushgari-Doyle and Alvarez-Cohen, 2020). Statistical significance of differentially expressed transcripts was defined as fold-change ≥ 2 and p-value < 0.05. Genes with differential expression were categorized by the Gene Ontology annotation module.
Results and Discussion
Enhancement of Trichloroethene-Dechlorination Activity by Iron Sulfide
The initial concentration of S2– (0.2 mM, without adding Fe2+) in this study was consistent with the conventional concentration in Dehalococcoides medium without toxicity (Löffler et al., 2005; Im et al., 2019). After adding Fe2+ (from 0.5 to 1.5 mM) to the cultures (Supplementary Figure 1), the dechlorination period of TCE by Dhc 195 was shortened by one-third. To determine the enhanced activity caused by Fe2+ or iron–sulfur products, the TCE dechlorination by Dhc 195 was evaluated with Fe2+ or S2– only (Figures 1a,b). Interestingly, the dechlorination rate of TCE was enhanced from 25.46 ± 1.15 to 37.84 ± 1.89 μmol⋅L–1⋅day–1, only with the coexistence of Fe2+ and S2– (both 0.2 mM), suggesting that the iron–sulfur product improved TCE dechlorination.
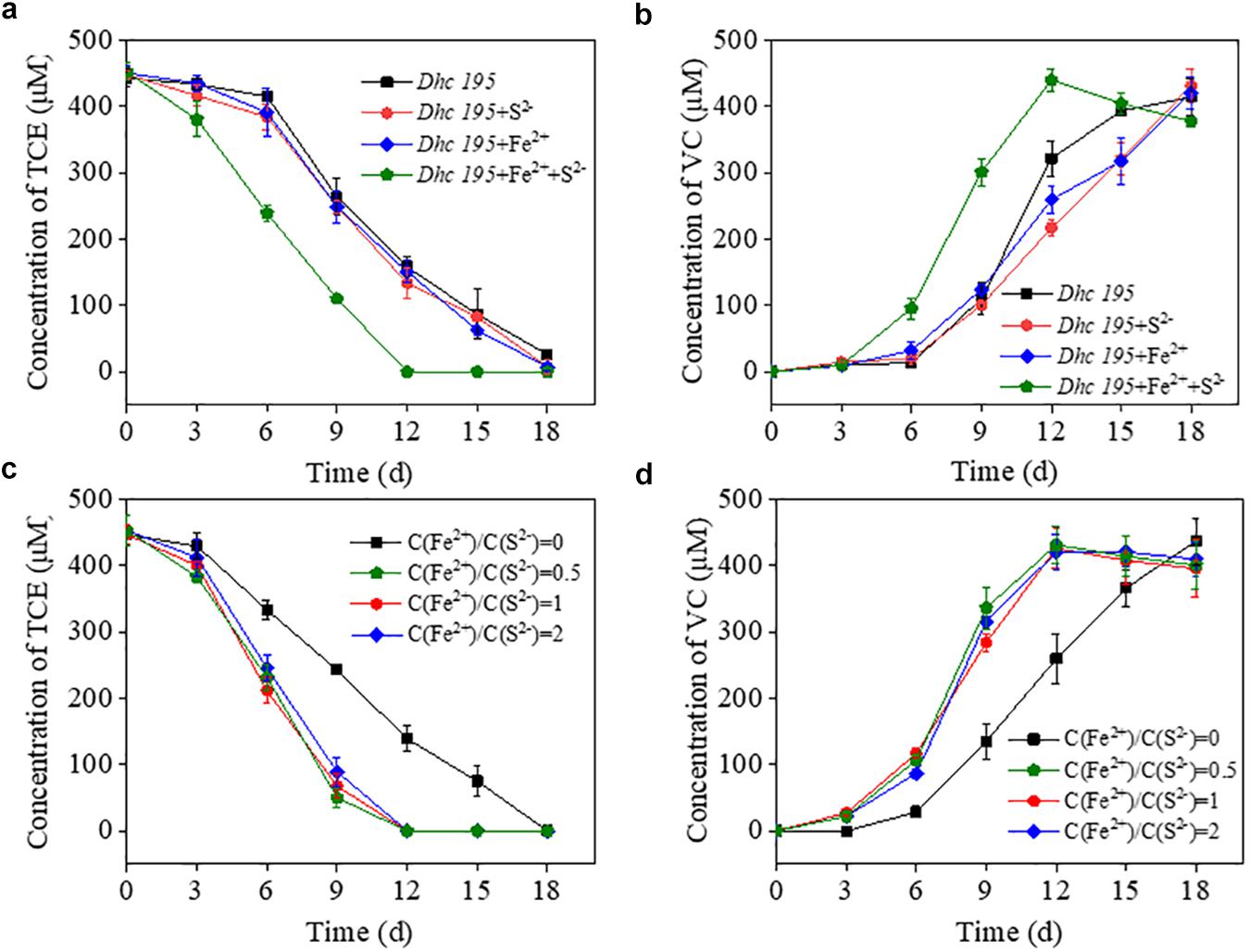
Figure 1. The effects of Fe2+ or S2– on the TCE dechlorination by Dhc 195 (a,b); The effects of different concentration ratios of Fe2+ and S2– on the TCE dechlorination by Dhc 195 (c,d).
Fe2+ and S2– might generate multiple forms of iron–sulfur compounds, such as FeS and FeS2 (Rickard and Luther, 2007; Xu et al., 2019), under different reactant ratios. Figures 1c,d show that the ratios of Fe2+/S2– (i.e., 0.5, 1, and 2) had negligible effects on TCE dechlorination rates, possibly attributed to the similar electrical conductivity of different iron–sulfur compounds. For example, both FeS and FeS2 could accelerate electron transport on the surface of nanoscale zero-valent iron (Xu et al., 2020). Multiple kinds of iron–sulfur clusters, such as [4Fe-4S] and [3Fe-4S], are also responsible for transferring electrons to metalloproteins (Zanello, 2018).
The exact components of iron–sulfur precipitates produced from Fe2+ and S2– (0.2 mM) were revealed by scanning electron microscopy equipped with energy dispersive X-ray spectrometry, showing the uniform distribution of iron and sulfur (Figures 2a–f). The mean size of the formed nanoparticles was smaller than 10 nm, labeled with white lines (Figures 2a,b). Iron–sulfur nanoparticles demonstrated a diffraction pattern identical to FeS (Figure 2g). The crystal structure of naturally formed FeS was similar to the iron–sulfur clusters in Dhc 195 (Kublik et al., 2016; Fincker and Spormann, 2017). When Fe2+ and S2– entered Dhc 195, they may form FeS and affect the activity of Dhc 195. Therefore, the optimum concentration of FeS was explored for TCE dechlorination with added concentrations ranging from 0 to 0.6 mM at a Fe2+/S2– ratio of 1. The dechlorination rate of TCE reached a maximum at 0.2 mM Fe2+ and S2–, whereas it was inhibited slightly at 0.6 mM (Supplementary Figure 2). Thus, the best concentration of FeS (0.2 mM) was selected for the downstream experiments.
Growth Promotion of Dehalococcoides mccartyi Strain 195 by Iron Sulfide
Without the addition of Fe2+ and S2–, Dhc 195 had a regular morphology with a clear cytomembrane, whereas with the addition of Fe2+ and S2–, many FeS nanoparticles surrounded/entered the cell, and the cytomembrane became misty (Figure 3). The activity of Dhc 195 cells should not be destroyed, as FeS enhanced TCE dechlorination but itself could not reduce TCE directly (Jacome et al., 2019). This was consistent with the previous observation, in which 2 mM in situ produced FeS did not destroy cells (Deng et al., 2020). Conversely, the growth of Dhc 195 was enhanced by the addition of Fe2+ and S2–. On day 12, the abundance of tceA was (2.83 ± 0.13) × 107 copies/ml without FeS, whereas it increased to (4.27 ± 0.21) × 108 copies/ml with FeS (Figure 4). A similar result was also observed in the cell yields from dechlorination, with 6.4 times more cells per molar produced Cl– than the FeS-absent treatment over 12 days (Supplementary Table 3). Dhc 195 required hydrogen, instead of Fe2+ or S2–, as the obligate electron donor for bioreductive dechlorination (Rosenthal et al., 2004; Mao et al., 2015). Therefore, the promoted cell growth of Dhc 195 by FeS is probably due to the regulation of physiological activity in Dhc 195 (Yan et al., 2013).
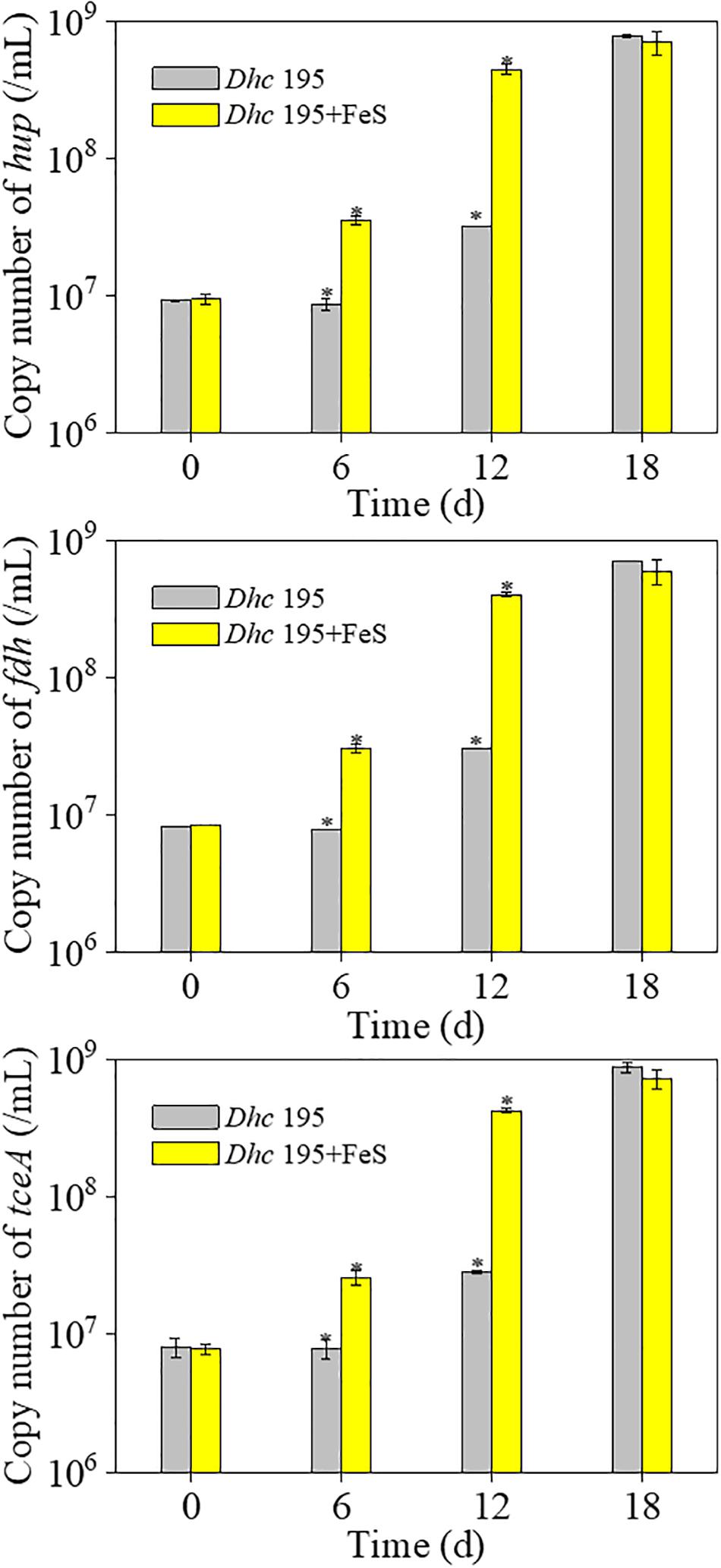
Figure 4. The copy number of three key genes of Dhe 195 with and without FeS (∗means significant difference, p-value < 0.05).
Differential Gene Expression of Dehalococcoides mccartyi Strain 195
Transcriptomic analysis was performed to identify gene expression after adding Fe2+ and S2–. The total expression pattern of cultures amended with and without FeS was relatively stable (Figure 5a). In Fe2+- and S2–-amended cultures, 90 genes were upregulated, and 63 genes were downregulated (≥2-fold change, p-value < 0.05) (Figure 5b and Supplementary Tables 4, 5). Among the upregulated genes, the main functions were associated with transmembrane transporter activity, enzyme regulator activity, molecular function regulator, and membrane components (Figure 6a). The addition of FeS enhanced the transcriptomic activity of genes responsible for the transmembrane transport of iron (DET1503, DET0685) and cobalamin (DET0685, DET1139, cobA), which is the active center of RDase (Yan et al., 2017). Meanwhile, modA (DET1161), involved in molybdenum transport and periplasmic molybdate binding, was upregulated 2.16 times; the gene (DET1632) encoding iron–sulfur cluster assembly protein was upregulated 2.11 times. Given the molybdenum and iron–sulfur clusters as the CISM center and the electron transporter, respectively (Mansfeldt et al., 2014; Kublik et al., 2016), these upregulations together suggested that electron transfer in the ETC was enhanced by the addition of FeS.
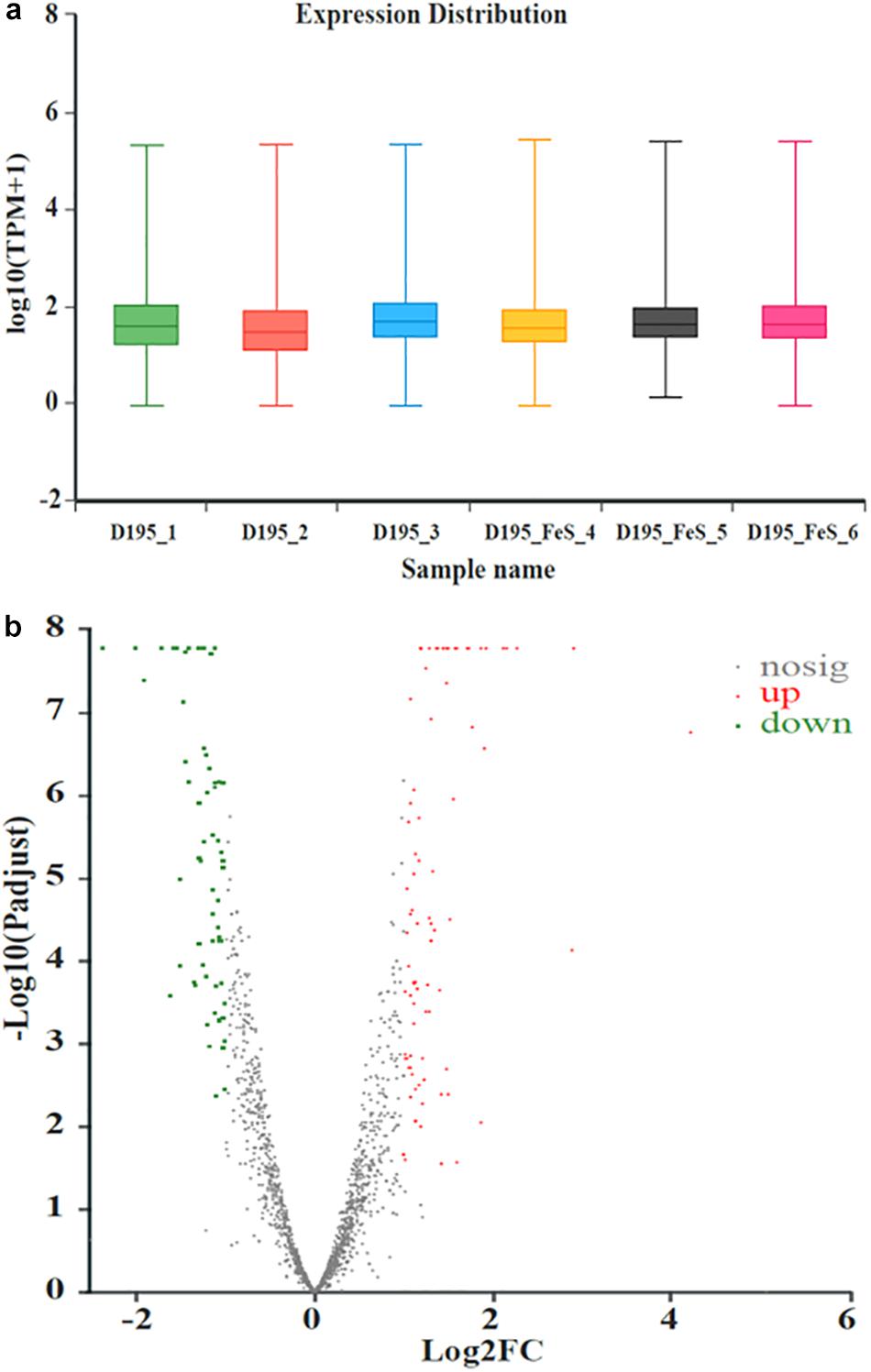
Figure 5. The expression distribution of transcripts for Dhc 195 (a); Microarray signal from Dhc 195 of two cultures (with and without FeS) (b) (≥2-fold difference, p-value < 0.05). The red dots represented the up-regulated genes and the green dots represented the down-regulated genes with the addition of FeS. All measurements were avenges from three biological replicates.
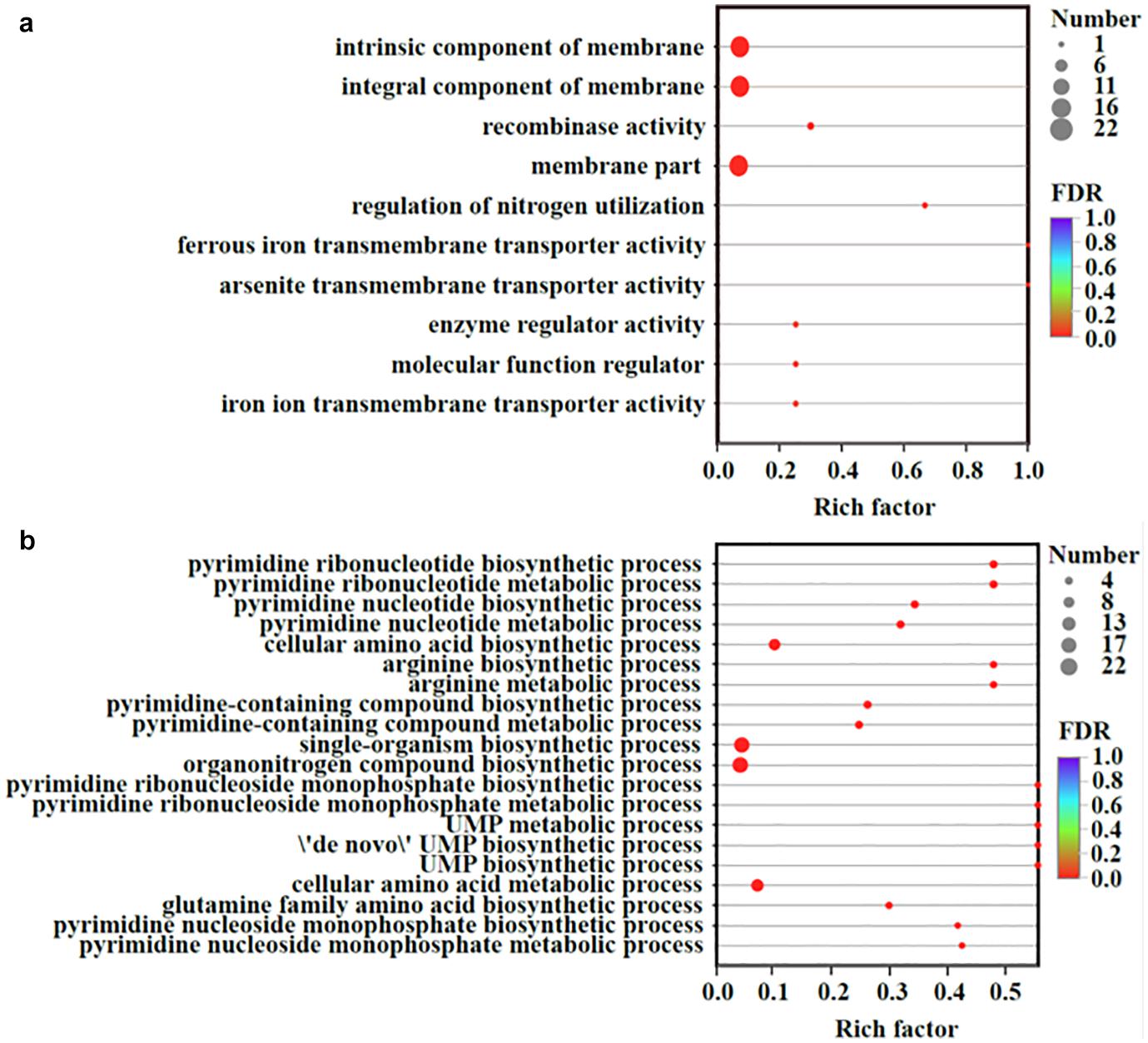
Figure 6. Up-regulated (a) and down-regulated (b) genes organized by the GO enrichment analysis. Vertical axis represented the GO term; Horizontal axis represented the ratios of Sample number/Background number. The size of dots indicated the number of genes/transcripts in this GO term. All measurements were averages from three biological replicates.
The transcriptional repressor gene LexA (DET0274) was also upregulated, resulting in the downregulation of some genes. Some genes (such as DET0657, DET0691, DET1198, and DET1481) were related to the biosynthesis of amino acids and pyrimidine-containing compounds (Figure 6b). They were involved in some processes, such as phosphorylation, secretion, and secondary processes. For example, the gene ccdA (DET0619), encoding a cytochrome c-type biogenesis protein, was downregulated, but it had little effect on the ETC of Dhc 195. Although the cobT (DET0657, DET0691) genes participating in cobalamin biosynthesis were downregulated, cobalamin (vitamin B12) was extrinsic and added directly to our experiments. The downregulation of cobT may have little effect on the activity of Dhc 195.
In terms of genes associated with TCE dechlorination in the ETC, only three of 35 putative RDase genes were upregulated, whereas the dominant RDase gene tceA (DET 0079) showed no significant differential expression. The stable expression of RDase genes was also reported by Mao et al. (2015), although the yield of Dhc 195 increased approximately 16 times when cocultured with Syntrophomonas wolfei. The absolute transcription of three key genes, tceA, hup, and fdh, was further quantified at the optimum concentration of FeS (0.2 mM). With the addition of FeS, the transcript number of tceA was increased approximately five times on day 12, whereas hup and fdh were increased approximately three times (Supplementary Figure 3). The increased transcription of key genes confirmed the enhanced electron transport in the ETC for TCE dechlorination.
Transcription is an indicator of the physiological activity of Dehalococcoides (Lee et al., 2006; Rahm et al., 2006; Mao et al., 2017). Throughout the transcriptomic results discussed earlier, it was obvious that the addition of Fe2+ and S2– upregulated transmembrane transporter genes such as cobA and modA and iron–sulfur cluster assembly genes. The transcript numbers of key genes, including tceA, hup, and fdh, in the ETC were also increased. This physiological evidence indicated that FeS enhanced the dechlorination of TCE by promoting electron transport in the ETC.
Conclusion
Bioremediation is a promising method for the in situ treatment of polluted soil and groundwater because of its low cost and low secondary pollution (Wang et al., 2016; Zhao et al., 2020). However, the inefficient reduction of organohalides is still a challenge due to the strict growth conditions of Dehalococcoides. By adding Fe2+ and S2–, both the dechlorination rate of TCE and the growth of Dhc 195, as well as the abundance of key genes, were improved substantially. In particular, the transcriptomic activity of genes involved in the electron transport chain was enhanced, suggesting that FeS promoted the dechlorination of TCE by regulating the electron transport of Dhc 195. Collectively, this study provides an eco-friendly and effective method to increase the dechlorination efficiency of organochlorides. Fe2+ and S2– are ubiquitous in the natural environment and are produced by the reduction of Fe3+ and SO42– under the activity of iron- and sulfate-reducing bacteria (Trudinger et al., 1985; Flynn et al., 2014; Sheu et al., 2016; Lu et al., 2017; Picard et al., 2019). With the coexistence of organohalide-respiring bacteria, this method has wide application prospects for removing organohalide pollution in situ.
Data Availability Statement
The raw data supporting the conclusions of this article will be made available by the authors, without undue reservation.
Author Contributions
YL designed, analysis, interpretation, and wrote the manuscript. H-PZ revised the manuscript critically for important intellectual content. LZ participated in conception, design, and approval of the final version. All authors contributed to the article and approved the submitted version.
Funding
This work was supported by the National Key Research and Development Program of China (2017YFA0207002) and the National Natural Science Foundation of China (21836003 and 21621005).
Conflict of Interest
The authors declare that the research was conducted in the absence of any commercial or financial relationships that could be construed as a potential conflict of interest.
Supplementary Material
The Supplementary Material for this article can be found online at: https://www.frontiersin.org/articles/10.3389/fmicb.2021.665281/full#supplementary-material
References
Antoniou, K., Mamais, D., and Pantazidou, M. (2019). Reductive dechlorination of trichloroethene under different sulfate-reducing and electron donor conditions. J. Contam. Hydrol. 226:103519. doi: 10.1016/j.jconhyd.2019.103519
Barry, W. T., Nobel, A. B., and Wright, F. A. (2005). Significance analysis of functional categories in gene expression studies: a structured permutation approach. Bioinformatics 21, 1943–1949. doi: 10.1093/bioinformatics/bti260
Chowdhury, P., and Viraraghavan, T. (2009). Sonochemical degradation of chlorinated organic compounds, phenolic compounds and organic dyes - a review. Sci. Total Environ. 407, 2474–2492. doi: 10.1016/j.scitotenv.2008.12.031
Costentin, C., Robert, M., and Savéant, J. M. (2005). Does catalysis of reductive dechlorination of tetra- and trichloroethylenes by vitamin B12 and corrinoid-based dehalogenases follow an electron transfer mechanism? J. Am. Chem. Soc. 127, 12154–12155. doi: 10.1021/ja0520464
Deng, X., Dohmae, N., Kaksonen, A. H., and Okamoto, A. (2020). Biogenic iron sulfide nanoparticles to enable extracellular electron uptake in sulfate-reducing bacteria. Angew. Chem. Int. Ed. 59, 5995–5999. doi: 10.1002/anie.201915196
Doğan-Subaşı, E., Bastiaens, L., Leys, N., Boon, N., and Dejonghe, W. (2014). Quantitative and functional dynamics of Dehalococcoides spp. and its tceA and vcrA genes under TCE exposure. Biodegradation 25, 493–504. doi: 10.1007/s10532-013-9676-8
Fincker, M., and Spormann, A. M. (2017). Biochemistry of catabolic reductive dehalogenation. Annu. Rev. Biochem. 86, 357–386. doi: 10.1146/annurev-biochem-061516-044829
Flynn, T. M., O’Loughlin, E. J., Mishra, B., DiChristina, T. J., and Kemner, K. M. (2014). Sulfur-mediated electron shuttling during bacterial iron reduction. Science 344, 1039–1042. doi: 10.1126/science.1252066
Guha, N., Loomis, D., Grosse, Y., Lauby-Secretan, B., El Ghissassi, F., Bouvard, V., et al. (2012). Carcinogenicity of trichloroethylene, tetrachloroethylene, some other chlorinated solvents, and their metabolites. Lancet Oncol. 13, 1192–1193. doi: 10.1016/s1470-2045(12)70485-0
Gushgari-Doyle, S., and Alvarez-Cohen, L. (2020). Effects of arsenic on trichloroethene-dechlorination activities of Dehalococcoides mccartyi 195. Environ. Sci. Technol. 54, 1276–1285.
Hartwig, S., Dragomirova, N., Kublik, A., Türkowsky, D., von Bergen, M., Lechner, U., et al. (2017). A H2-oxidizing, 1,2,3-trichlorobenzene-reducing multienzyme complex isolated from the obligately organohalide-respiring bacterium Dehalococcoides mccartyi strain CBDB1. Environ. Microbiol. Rep. 9, 618–625. doi: 10.1111/1758-2229.12560
He, Y. T., Wilson, J. T., Su, C., and Wilkin, R. T. (2010). Impact of iron sulfide transformation on trichloroethylene degradation. Geochim. Cosmochim. Ac. 74, 2025–2039. doi: 10.1016/j.gca.2010.01.013
He, Y. T., Wilson, J. T., Su, C., and Wilkin, R. T. (2015). Review of abiotic degradation of chlorinated solvents by reactive iron minerals in aquifers. Ground Water Monit. R. 35, 57–75. doi: 10.1111/gwmr.12111
Im, J., Mack, E. E., Seger, E. S., and Loffler, F. E. (2019). Biotic and abiotic dehalogenation of 1,1,2-trichloro-1,2,2-trifluoroethane (CFC-113): Implications for bacterial detoxification of chlorinated ethenes. Environ. Sci. Technol. 53, 11941–11948. doi: 10.1021/acs.est.9b04399
Jacome, L. A. P., Wang, P. H., Molenda, O., Li, Y. X., Islam, M. A., and Edwards, E. A. (2019). Sustained dechlorination of vinyl chloride to ethene in Dehalococcoides-enriched cultures grown without addition of exogenous vitamins and at low pH. Environ. Sci. Technol. 53, 11364–11374. doi: 10.1021/acs.est.9b02339
Jeong, H. Y., and Hayes, K. F. (2007). Reductive dechlorination of tetrachloroethylene and trichloroethylene by mackinawite (FeS) in the presence of metals: reaction rates. Environ. Sci. Technol. 41, 6390–6396. doi: 10.1021/es0706394
Kondo, K., Okamoto, A., Hashimoto, K., and Nakamura, R. (2015). Sulfur-mediated electron shuttling sustains microbial long-distance extracellular electron transfer with the aid of metallic iron sulfide. Langmuir 31, 7427–7434. doi: 10.1021/acs.langmuir.5b01033
Kublik, A., Deobald, D., Hartwig, S., Schiffmann, C. L., Andrades, A., von Bergen, M., et al. (2016). Identification of a multi-protein reductive dehalogenase complex in Dehalococcoides mccartyi strain CBDB1 suggests a protein-dependent respiratory electron transport chain obviating quinone involvement. Environ. Microbiol. 18, 3044–3056. doi: 10.1111/1462-2920.13200
Lee, P. K. H., Johnson, D. R., Holmes, V. F., He, J. Z., and Alvarez-Cohen, L. (2006). Reductive dehalogenase gene expression as a biomarker for physiological activity of Dehalococcoides spp. Appl. Environ. Microbiol. 72, 6161–6168. doi: 10.1128/aem.01070-06
Li, Y. R., Wen, L. L., Zhao, H. P., and Zhu, L. Z. (2019). Addition of Shewanella oneidensis MR-1 to the Dehalococcoides-containing culture enhances the trichloroethene dechlorination. Environ. Inter. 133:105245. doi: 10.1016/j.envint.2019.105245
Liang, X., Dong, Y., Kuder, T., Krumholz, L. R., Philp, R. P., and Butler, E. C. (2007). Distinguishing abiotic and biotic transformation of tetrachloroethylene and trichloroethylene by stable carbon isotope fractionation. Environ. Sci. Technol. 41, 7094–7100. doi: 10.1021/es070970n
Löffler, F. E., Sanford, R. A., and Ritalahti, K. M. (2005). Enrichment, cultivation, and detection of reductively dechlorinating bacteria. Environ. Microbiol. 397, 77–111. doi: 10.1016/s0076-6879(05)97005-5
Löffler, F. E., Yan, J., Ritalahti, K. M., Adrian, L., Edwards, E. A., Konstantinidis, K. T., et al. (2013). Dehalococcoides mccartyi gen. nov., sp nov., obligately organohalide-respiring anaerobic bacteria relevant to halogen cycling and bioremediation, belong to a novel bacterial class, Dehalococcoidia classis nov., order Dehalococcoidales ord. nov and family Dehalococcoidaceae fam. nov., within the phylum Chloroflexi. Int. J. Syst. Evol. Microbiol. 63, 625–635. doi: 10.1099/ijs.0.034926-0
Lu, Y. S., Xu, L., Shu, W. K., Zhou, J. Z., Chen, X. P., Xu, Y. F., et al. (2017). Microbial mediated iron redox cycling in Fe(hydr)oxides for nitrite removal. Bioresource Technol. 224, 34–40. doi: 10.1016/j.biortech.2016.10.025
Magnuson, J. K., Romine, M. F., Burris, D. R., and Kingsley, M. T. (2000). Trichloroethene reductive dehalogenase from Dehalococcoides ethenogenes: sequence of tceA and substrate range characterization. Appl. Environ. Microbiol. 66, 5141–5147. doi: 10.1128/aem.66.12.5141-5147.2000
Mansfeldt, C. B., Rowe, A. R., Heavner, G. L., Zinder, S. H., and Richardson, R. E. (2014). Meta-analyses of Dehalococcoides mccartyi strain 195 transcriptomic profiles identify a respiration rate-related gene expression transition point and interoperon recruitment of a key oxidoreductase subunit. Appl. Environ. Microbiol. 80, 6062–6072. doi: 10.1128/aem.02130-14
Mao, X. W., Oremland, R. S., Liu, T., Gushgari, S., Landers, A. A., Baesman, S. M., et al. (2017). Acetylene fuels TCE reductive dechlorination by defined Dehalococcoides/Pelobacter consortia. Environ. Sci. Technol. 51, 2366–2372.
Mao, X. W., Stenuit, B., Polasko, A., and Alvarez-Cohen, L. (2015). Efficient metabolic exchange and electron transfer within a syntrophic trichloroethene-degrading coculture of Dehalococcoide mccartyi 195 and Syntrophomonas wolfei. Appl. Environ. Microbiol. 81, 2015–2024. doi: 10.1128/aem.03464-14
Maymo’-Gatell, X., Chien, Y., Gossett, J. M., and Zinder, S. H. (1997). Isolation of a bacterium that reductively dechlorinates tetrachloroethene to ethene. Science 276, 1568–1571. doi: 10.1126/science.276.5318.1568
Morris, R. M., Sowell, S., Barofsky, D., Zinder, S., and Richardson, R. (2006). Transcription and mass-spectroscopic proteomic studies of electron transport oxidoreductases in Dehalococcoides ethenogenes. Environ. Microbiol. 8, 1499–1509. doi: 10.1111/j.1462-2920.2006.01090.x
Payne, K. A., Quezada, C. P., Fisher, K., Dunstan, M. S., Collins, F. A., Sjuts, H., et al. (2015). Reductive dehalogenase structure suggests a mechanism for B12-dependent dehalogenation. Nature 517, 513–516. doi: 10.1038/nature13901
Picard, A., Gartman, A., Cosmidis, J., Obst, M., Vidoudez, C., Clarke, D. R., et al. (2019). Authigenic metastable iron sulfide minerals preserve microbial organic carbon in anoxic environments. Chem. Geol. 530:119343. doi: 10.1016/j.chemgeo.2019.119343
Pinske, C., Kruger, S., Soboh, B., Ihling, C., Kuhns, M., Braussemann, M., et al. (2011). Efficient electron transfer from hydrogen to benzyl viologen by the [NiFe]-hydrogenases of Escherichia coli is dependent on the coexpression of the iron-sulfur cluster-containing small subunit. Arch. Microbiol. 193, 893–903. doi: 10.1007/s00203-011-0726-5
Rahm, B. G., Morris, R. M., and Richardson, R. E. (2006). Temporal expression of respiratory genes in an enrichment culture containing Dehalococcoides ethenogenes. Appl. Environ. Microbiol. 72, 5486–5491. doi: 10.1128/aem.00855-06
Rickard, D., and Luther, G. W. (2007). Chemistry of iron sulfides. Chem. Rev. 107, 514–562. doi: 10.1021/cr0503658
Ritalahti, K. M., Amos, B. K., Sung, Y., Wu, Q. Z., Koenigsberg, S. S., and Löffler, F. E. (2006). Quantitative PCR targeting 16S rRNA and reductive dehalogenase genes simultaneously monitors multiple Dehalococcoides strains. Appl. Environ. Microbiol. 72, 2765–2774. doi: 10.1128/aem.72.4.2765-2774.2006
Rosenthal, H., Adrian, L., and Steiof, M. (2004). Dechlorination of PCE in the presence of Fe0 enhanced by a mixed culture containing two Dehalococcoides strains. Chemosphere 55, 661–669. doi: 10.1016/j.chemosphere.2003.11.053
Schipp, C. J., Marco-Urrea, E., Kublik, A., Seifert, J., and Adrian, L. (2013). Organic cofactors in the metabolism of Dehalococcoides mccartyi strains. Phil. Trans. R. Soc. B 368:20120321. doi: 10.1098/rstb.2012.0321
Schubert, T., Adrian, L., Sawers, R. G., and Diekert, G. (2018). Organohalide respiratory chains: composition, topology and key enzymes. FEMS Microbiol. Ecol. 94:fiy035.
Seshadri, R., Adrian, L., Fouts, D. E., Eisen, J. A., Phillippy, A. M., Methe, B. A., et al. (2005). Genome sequence of the PCE-dechlorinating bacterium Dehalococcoides ethenogenes. Science 307, 105–108. doi: 10.1126/science.1102226
Sheu, Y. T., Lien, P. J., Chen, K. F., Ou, J. H., and Kao, C. M. (2016). Application of NZVI-contained emulsified substrate to bioremediate PCE-contaminated groundwater - a pilot-scale study. Chem. Eng. J. 304, 714–727. doi: 10.1016/j.cej.2016.06.126
Stewart, B. W. (2001). Trichloroethylene and cancer: a carcinogen on trial. Med. J. Australia 174, 244–247. doi: 10.5694/j.1326-5377.2001.tb143249.x
Trudinger, P. A., Chambers, L. A., and Smith, J. W. (1985). Low-temperature sulfate reduction - biological versus. Can. J. Earth Sci. 22, 1910–1918. doi: 10.1139/e85-207
Türkowsky, D., Jehmlich, N., Diekert, G., Adrian, L., von Bergen, M., and Goris, T. (2018). An integrative overview of genomic, transcriptomic and proteomic analyses in organohalide respiration research. FEMS Microbiol. Ecol. 94: fiy013.
Vamvakas, S., Bruning, T., Thomasson, B., Lammert, M., Baumuller, A., Bolt, H. M., et al. (1998). Renal cell cancer correlated with occupational exposure to trichloroethene. J. Cancer Res. Clin. 124, 374–382. doi: 10.1007/s004320050186
Wang, B. Y., Krajmalnik-Brown, R., Zhou, C., Luo, Y. H., Rittmann, B. E., and Tang, Y. N. (2020). Modeling trichloroethene reduction, methanogenesis, and homoacetogenesis in a H2-based biofilm. J. Environ. Eng. 146:04019115. doi: 10.1061/(asce)ee.1943-7870.0001642
Wang, S. Q., Chen, S. Y., Wang, Y., Low, A., Lu, Q. H., and Qiu, R. L. (2016). Integration of organohalide-respiring bacteria and nanoscale zero-valent iron (Bio-nZVI-RD): A perfect marriage for the remediation of organohalide pollutants? Biotechnol. Adv. 34, 1384–1395. doi: 10.1016/j.biotechadv.2016.10.004
Wang, S. Q., Qiu, L., Liu, X. W., Xu, G. F., Siegert, M., Lu, Q. H., et al. (2018). Electron transport chains in organohalide-respiring bacteria and bioremediation implications. Biotechnol. Adv. 36, 1194–1206. doi: 10.1016/j.biotechadv.2018.03.018
Wen, L. L., Li, Y. R., Zhu, L. Z., and Zhao, H. P. (2020). Influence of non-dechlorinating microbes on trichloroethene reduction based on vitamin B12 synthesis in anaerobic cultures. Environ. Pollut. 259:113947. doi: 10.1016/j.envpol.2020.113947
Wen, L. L., Zhang, Y., Pan, Y. W., Wu, W. Q., Meng, S. H., Zhou, C., et al. (2015). The roles of methanogens and acetogens in dechlorination of trichloroethene using different electron donors. Environ. Sci. Pollut. Res. 22, 19039–19047. doi: 10.1007/s11356-015-5117-z
Xu, J., Avellan, A., Li, H., Liu, X. T., Noël, V., Lou, Z. M., et al. (2020). Sulfur loading and speciation control the hydrophobicity, electron transfer, reactivity, and selectivity of sulfidized nanoscale zerovalent iron. Adv. Mater. 32;1906910. doi: 10.1002/adma.201906910
Xu, J., Cao, Z., Zhou, H., Lou, Z. M., Wang, Y., Xu, X. H., et al. (2019). Sulfur dose and sulfidation time affect reactivity and selectivity of post-sulfidized nanoscale zerovalent iron. Environ. Sci. Technol. 53, 13344–13352. doi: 10.1021/acs.est.9b04210
Yan, J., Bi, M., Bourdon, A. K., Farmer, A. T., Wang, P. H., Molenda, O., et al. (2017). Purinyl-cobamide is a native prosthetic group of reductive dehalogenases. Nat. Chem. Biol. 14, 1–12.
Yan, J., Im, J., Yang, Y., and Loffler, F. E. (2013). Guided cobalamin biosynthesis supports Dehalococcoides mccartyi reductive dechlorination activity. Phil. Trans. R. Soc. B 368:20120320. doi: 10.1098/rstb.2012.0320
Zanello, P. (2018). Structure and electrochemistry of proteins harboring iron-sulfur clusters of different nuclearities. Part II. [4Fe-4S] and [3Fe-4S] iron-sulfur proteins. J. Theor. Biol. 202, 250–263. doi: 10.1016/j.jsb.2018.01.010
Zhao, S. Y., and He, J. Z. (2019). Reductive dechlorination of high concentrations of chloroethenes by a Dehalococcoides mccartyi strain 11G. FEMS Microbiol. Ecol. 905:fiy209.
Keywords: Dehalococcoides mccartyi strain 195, FeS, trichloroethene, electron transport, iron-sulfur cluster
Citation: Li YR, Zhao HP and Zhu LZ (2021) Iron Sulfide Enhanced the Dechlorination of Trichloroethene by Dehalococcoides mccartyi Strain 195. Front. Microbiol. 12:665281. doi: 10.3389/fmicb.2021.665281
Received: 07 February 2021; Accepted: 06 April 2021;
Published: 01 June 2021.
Edited by:
Shanquan Wang, Sun Yat-sen University, ChinaReviewed by:
Zhiling Li, Harbin Institute of Technology, ChinaYi Yang, Institute of Applied Ecology (CAS), China
Copyright © 2021 Li, Zhao and Zhu. This is an open-access article distributed under the terms of the Creative Commons Attribution License (CC BY). The use, distribution or reproduction in other forums is permitted, provided the original author(s) and the copyright owner(s) are credited and that the original publication in this journal is cited, in accordance with accepted academic practice. No use, distribution or reproduction is permitted which does not comply with these terms.
*Correspondence: Lizhong Zhu, emx6QHpqdS5lZHUuY24=