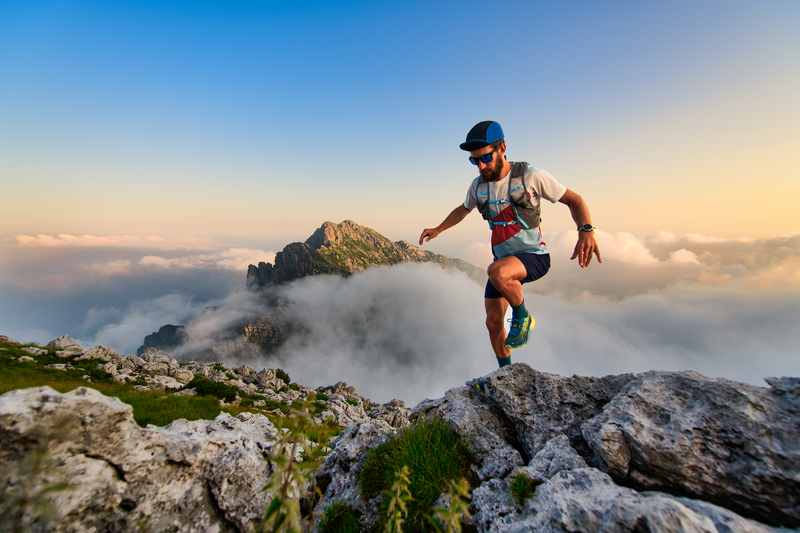
95% of researchers rate our articles as excellent or good
Learn more about the work of our research integrity team to safeguard the quality of each article we publish.
Find out more
REVIEW article
Front. Microbiol. , 20 January 2022
Sec. Infectious Agents and Disease
Volume 12 - 2021 | https://doi.org/10.3389/fmicb.2021.651081
This article is part of the Research Topic The Deadly Secrets of C. Difficile - Insights into Host-Pathogen Interaction, Volume II View all 12 articles
In this review, we focus on gut microbiota profiles in infants and adults colonized (CDC) or infected (CDI) with Clostridioides difficile. After a short update on CDI epidemiology and pathology, we present the gut dysbiosis profiles associated with CDI in adults and infants, as well as the role of dysbiosis in C. difficile spores germination and multiplication. Both molecular and culturomic studies agree on a significant decrease of gut microbiota diversity and resilience in CDI, depletion of Firmicutes, Bacteroidetes, and Actinobacteria phyla and a high abundance of Proteobacteria, associated with low butyrogenic and high lactic acid-bacteria levels. In symptomatic cases, microbiota deviations are associated with high levels of inflammatory markers, such as calprotectin. In infants, colonization with Bifidobacteria that trigger a local anti-inflammatory response and abundance of Ruminococcus, together with lack of receptors for clostridial toxins and immunological factors (e.g., C. difficile toxins neutralizing antibodies) might explain the lack of clinical symptoms. Gut dysbiosis amelioration through administration of “biotics” or non-toxigenic C. difficile preparations and fecal microbiota transplantation proved to be very useful for the management of CDI.
Clostridioides (formerly Clostridium) difficile is a Gram-positive, obligate anaerobe, spore-forming bacteria, harboring a plethora of surface and secreted proteins responsible for the colonic colonization and subsequent inflammation characteristic for C. difficile infection (CDI), among which the most important are the clostridial toxins: toxin A (TcdA) and toxin B (TcdB), and in some bacterial strains, the binary toxin CDT (Smits et al., 2016). Clinical symptoms range from mild diarrhea to fulminant colitis, known as pseudomembranous colitis, with its complications – toxic megacolon and large bowel perforation (McDonald et al., 2018; Dieterle et al., 2019). C. difficile is the number one causative agent of nosocomial post-antibiotic colitis, associated with high morbidity and mortality (Ghose, 2013). In the last decade, both frequency and severity of CDI have increased, largely due to the emergence of a hypervirulent strain called NAP1 (North American pulsed-field gel electrophoresis type 1 strain) (Depestel and Aronoff, 2013). Moreover, in the last two decades, there has been a significant increase in the incidence of CDI in previously considered low-risk population categories, including community-associated-Clostridioides difficile infections (CA-CDI), with more than 30% of cases not showing typical CDI risk factors, such as antibiotic treatment or recent hospitalization (Wilcox et al., 2008; Hensgens et al., 2011). Although CDI is the preserve of the elderly population, it can also affect other age segments. In a retrospective survey performed by U.S. National Hospital Discharge Surveys from 2001 to 2010 revealed that CDI incidence was highest among elderly adults (11.6 CDI discharges/1,000 total discharges), followed by adults (3.5 CDI discharges/1,000 total discharges) and pediatrics (<12 years) (1.2 CDI discharges/1,000 total discharges). The mortality rates attributable to CDI in the elderly were significantly higher (8.8%) compared to adults (3.1%) and pediatrics (1.4%) (Pechal et al., 2016). Although there is not much information available about the epidemiology of infection in infants, however, the carriage rate of non-toxigenic C. difficile is very high in newborns, suggesting the commensal status of this bacterium in this population segment (Sammons et al., 2013; Borali and De Giacomo, 2016). For this reason, C. difficile is not considered an enteric pathogen in infants and children affected by bloody diarrhea and younger than 12 months (Cama et al., 2019). However, asymptomatic infants could be also infected by toxigenic adult infectious strains both after hospitalization and even in the community, thus, constituting a reservoir for toxigenic strains (Rousseau et al., 2011; Ferraris et al., 2020).
This review aims to present some particular aspects of gut microbiota in CDI infants and adults, taking into account that the pathophysiology of this disease suggests that the clinical manifestations occur in cases of an imbalance of the intestinal microbiota, known as dysbiosis. In this purpose, studies performing culture-dependent (C. difficile cultivation) and independent (16S rRNA and metagenomics) have been analyzed.
Some of the underlying causes of intestinal dysbiosis are antibiotic treatments (Kukla et al., 2020), advanced age (over 65 years), hospitalization (particularly in patients sharing the hospital room with an infected patient, in intensive care units, during prolonged hospitalization), nursing home stay, severe associated diseases, immunological suppression, gastric acidity suppression by proton pump inhibitors or histamine2-receptor antagonists and prolonged use of elemental diet in the context of enteral nutrition, inflammatory bowel diseases, gastrointestinal surgery (in particular colectomy, small-bowel resection, and gastric resection were associated with the highest risk while patients undergoing cholecystectomy and appendectomy had the lowest risk), all of these circumstances being associated with characteristic changes in the configuration of the gut microbiota and with an increased CDI risk (O’Keefe, 2010; Fashner et al., 2011; Nitzan et al., 2013; Rodriguez et al., 2016; Sartelli et al., 2019; Avni et al., 2020). A strong argument regarding the impact of dysbiosis on CDI risk is offered by animal studies proving that correcting dysbiosis by administration of different substances such as phytophenolic compounds or carvacrol has been shown to decrease susceptibility to CDI. The gut dysbiosis of 6-week-old C57BL/6 mice was induced by the oral administration of an antibiotic cocktail in water simultaneously with the intra-peritoneal injection of clindamycin. The mice were infected with 105 CFU/ml of hypervirulent C. difficile ATCC 1870 spores. Carvacrol supplementation significantly reduced the incidence of diarrhea and improved mice’s clinical and diarrhea scores. Microbiome analysis revealed that carvacrol increased the abundance of Bacteroidetes and Firmicutes. An increased abundance of Lactobacillaceae and Lachnospiraceae was noticed among the beneficial taxa in carvacrol treated mice. Also, carvacrol decreased the proportion of pro-inflammatory microbiota, such as Proteobacteria (i.e., Enterobacteriaceae) and Verrucomicrobia, without significantly affecting the gut microbiome diversity compared to the control (Mooyottu et al., 2017).
Five clinical features (potential risk factors) predict dysbiosis in CDI patients: antibiotic use within the previous 3 weeks, immunosuppression, multimorbidity, recent/multiple hospitalization, and prior CDI (Battaglioli et al., 2018). In individuals whose normal intestinal microbiota has been disrupted, ingested C. difficile spores germinate in the presence of bile salts in the small intestine and colonize the colon epithelial cells, releasing the inflammatory enterotoxins, which are primarily and largely responsible for the colonic inflammation in C. difficile diseases, inducing cytoskeletal changes, disruption of tight junctions, and induction of inflammatory cytokine production (Shen, 2012; Winston and Theriot, 2016). The C. difficile spores are released by the patient facilitating CDI transmission to susceptible hosts (Czepiel et al., 2019).
The intestinal environment represents a complex network of bacterial cells and metabolic products and/or other unknown substances derived from their own structures or metabolisms, which are in close and continuous interaction, both with each other, as well as with the human intestinal cells and the host’s immune system (Lazar et al., 2018, 2019).
The characterization of the baseline healthy microbiota and differences that are associated with various diseases has been possible with the contribution of large-scale projects, such as Meta-HIT and the Human Microbiome Project (HMP), using different omics technologies (Qin et al., 2010; Human Microbiome Project, 2012).
During early development, the gut microbiota undergoes subsequent changes until a stable adult state is reached. The adult microbiota has three basic characteristics: diversity (a high microbiota diversity defined by high species richness and high functional diversity being generally associated with the health condition), resilience (the property of the gut microbiota to resist to an impact and to recover and to baseline after the disturbance cessation; the capacity of a microbial community to reach a stable state in response to chemical, physical or biological perturbations of different intensities is achieved through genetically diverse resident clonal populations and population-level dynamics) and long-term stability of high taxonomic level components (Levine and d’Antonio, 1999; Lozupone et al., 2012; McBurney et al., 2019; Priya and Blekhman, 2019; Dogra et al., 2020).
Regarding the diversity, human microbiota displays a remarkable heterogeneity within and between individuals, the results of the culture-independent studies leading to the generally accepted idea that we rather share a functional core microbiome, than a core microbiota (Lozupone et al., 2012). The >1,000 estimated species-level phylotypes are belonging to few microbial phyla, which are Firmicutes, Bacteroidetes, Actinobacteria, Proteobacteria, Fusobacteria, and Verrucomicrobia; among these, the two Firmicutes and Bacteroidetes phyla are representing 90% of gut microbiota (Magne et al., 2020). Firmicutes comprises more than 200 different genera, such as Lactobacillus, Bacillus, Clostridium, Enterococcus, and Ruminococcus (Kachrimanidou and Tsintarakis, 2020). Clostridium genera represent 95% of the Firmicutes, while Bacteroidetes include as predominant genera Bacteroidetes and Prevotella (Rinninella et al., 2019). Actinobacteria are proportionally less abundant and mainly represented by Bifidobacterium species (Rinninella et al., 2019). From the mentioned components, Ruminococcus and Bifidobacterium have been reported to exhibit protective roles against CDI (Seekatz and Young, 2014; Kho and Lal, 2018).
The gut microbiota community shows resilience to different perturbations, such as those induced by different diets, antibiotic administration, invasion by new species (called colonization resistance) (Folke et al., 2004). Under the impact of a certain disturbances, such as antibiotic administration, microbiota enters an unstable state that progresses to a new stable state. When the latter is highly similar to the pre-disturbance state, this indicates a complete recovery. However, sometimes the post-disturbance stable state is distinct and this unfortunately can be both abnormal and resilient, as a response to the perturbation persistence (e.g., poor diet, antibiotic treatments etc) (Dupont, 2011). An example of gut microbiota resilience is the success of bacteriotherapy or microbiota transplantation in treating recurrent CDI. In this case, the gut microbiota switches from an initial dysbiosis state, favoring the CDI (e.g., increased abundance of Veillonella and Streptococcus) to a baseline state, in which the taxa from the healthy donor (e.g., Bacteroidetes) persisted 1 month after transplantation (Gough et al., 2011). A high diversity (high species richness, α-diversity) and host immune effectors, e.g., nucleotide-binding oligomerization domain (Nod2), an intracellular innate immune sensor involved in the anti-infectious host defense, were linked to gut microbiota resilience (Tap et al., 2015; Raymond et al., 2016; Goethel et al., 2019). A low microbiota diversity was correlated with recurrent CDI, but with unknown effects on microbiota resilience (Chang et al., 2008; Khoruts et al., 2010). However, a comparable phylum-level diversity was observed in individuals with initial CDI and healthy controls, while in case of recurrent CDI the phylum-level diversity switched to different highly divergent profiles very different from the healthy state other (Chang et al., 2008).
The stability of gut microbiota is affected by different factors, including genetic factors, early-life events, travel, dietary changes, weight loss or gain, diarrheal disease, antibiotics, immunosuppressants, premeditated interventions to influence the microbiota by administration of prebiotics, probiotics, postbiotics and symbiotics, as well as fecal transplantation (Faith et al., 2013; Britton and Young, 2014).
In this section, we will present some of the culture-dependent and independent studies that have been performed to identify the dysbiosis profiles (Table 1) and specific microbial derived biomarkers in patients prone to CDI. A metagenomic and culturomic analysis of gut microbiota dysbiosis during CDI has shown a significant depletion of Bacteroidetes in C. difficile patients compared with the control group (Amrane et al., 2019). Diversity was significantly higher in the control group. Proteobacteria were more common in the CDI group. Firmicutes and Actinobacteria were less common in the CDI group (Lozupone et al., 2012). Firmicutes are involved in butyrate, and other short chain fatty acids (SCFA) production, these molecules playing a role in gut homeostasis and inhibition of C. difficile germination (Kachrimanidou and Tsintarakis, 2020) and Bacteroidetes are involved in carbohydrates digestion, producing substrates for colonocytes (den Besten et al., 2013). Depletion of these two major phyla of gut microbiota was detected in the C. difficile group. The bacterial families conferring resistance to CDI are Bacteroidaceae, Bifidobacteriaceae, and Lachnospiraceae (Antharam et al., 2013). Studies in animals with CDI, revealed a high abundance of Proteobacteria (especially Enterobacteriaceae) and a numerical decrease of Lachnospiraceae (Firmicutes) in diseased animals (Reeves et al., 2011). Lachnospiraceae strains have been shown to be able to partially restore colonization resistance, the mice inoculated with such strains showing decreased C. difficile colonization, lower levels of cytotoxins and lower clinical signs of severe infection (Reeves et al., 2012). An increased relative abundance of Enterococcus, Lactobacillus, Escherichia coli, Enterobacter, Bacteroides, Parabacteroides, Akkermansia muciniphila, and decreased Faecalibacterium, Roseburia, Blautia, Prevotella, Megamonas, Streptococcus, and Bacteroides levels were evidenced in the gut microbiota of CDI patients (Rea et al., 2012). The bacteria found only in the control group, which may have a role against C. difficile, were Bacteroides ovatus, Bacteroides vulgatus and Oscillibacter massiliensis (Lozupone et al., 2012). Only three bacteria with a potential role against C. difficile were detected both by culturomics and metagenomics, namely Bifidobacterium adolescentis, Bifidobacterium longum and Bacteroides ovatus (Lozupone et al., 2012). Overrepresentation of Akkermansia may be a predictive marker for the development of nosocomial diarrhea, with a worsened CDI prognosis (Hernandez et al., 2018; Vakili et al., 2020).
Table 1. Gut microbiota dysbiosis associated with Clostridium difficile infection in adults and infants (proposed microbiota-derived biomarkers for CDI dysbiosis are presented in bold).
The gut dysbiosis also results in biochemical and immunological disruptions like decreased short chain fatty acids (SCFAs) levels, the abundance of primary bile acids, high availability of carbohydrates, suppression of immunological mechanisms and absence of competitors, all resulting in increased colonization capacity, favoring germination and growth of C. difficile (Ridlon et al., 2014; Carding et al., 2015; Goh and Klaenhammer, 2015; Valdes et al., 2018; Hills et al., 2019; Toor et al., 2019). Nosocomial diarrheal syndromes, including CDI, were associated not only with decreased bacterial diversity, Firmicutes paucity, low numbers of Ruminococcaceae, Lachnospiraceae but also with low levels of butyrogenic (e.g., Roseburia, Faecalibacterium, Subdoligranulum, Anaerostipes, and Pseudobutyrivibrio) and acetogenic (e.g., Blautia and Dorea) genera and with high levels of lactic acid bacteria (e.g., Enterococcus sp.) (Antharam et al., 2013). A decrease in butyrate-producing bacteria and an increase in lactic acid-producing bacteria were associated with increased CDI risk (Vakili et al., 2020). The role of SCFAs depletion in facilitating C. difficile infection is not yet elucidated, yet however, SCFAs could act by reducing the luminal pH (unfavorable for C. difficile) and stimulating the defensive barrier by production of mucin and antimicrobial peptides (defensins and cathelicidins, secreted by specialized cells, Paneth cells and leukocytes in the intestinal crypts) (Guilloteau et al., 2010; Solomon, 2013; Gupta et al., 2016). Among SCFAs, butyrate seems to have no effect on C. difficile colonization and toxin production, but it can protect the intestinal epithelium from the damage caused by C. difficile toxins by stabilizing hypoxia-inducible factor-1 (HIF-1) and increasing tight junctions, and thus decreasing intestinal epithelial permeability, inhibiting intestinal inflammation and bacterial translocation. The addition of butyrate to the drinking water of mice, administration of a pro-drug of butyrate, tributyrin, or of an inulin-rich diet (inulin can be fermented by gut commensal bacteria, which generate short-chain fatty acids, mainly acetate, propionate, and butyrate) resulted in the protection of mice against CDI (Fachi et al., 2019; Song et al., 2020).
C. difficile spore germination is regulated by the detection of bile salt and amino acid cogerminants by pseudoproteases CspC and CspA, respectively (Weingarden et al., 2014; Lawler et al., 2020). Although some cholate derivatives and the amino acid glycine could act as co-germinant factors, deoxycholate prevents vegetative growth (Sorg and Sonenshein, 2008), while chenodeoxycholate inhibits taurocholate-mediated germination (Sorg and Sonenshein, 2010). Commensal members of Clostridiales present in the gut contribute to the creation of an inappropriate environment for C. difficile germination and colonization by modulating the production of cogerminants (Perez-Cobas et al., 2015). For example, Clostridium scindens, a bile acid 7α-dehydroxylating intestinal strain, is associated with resistance to C. difficile infection, and, upon administration, it enhances resistance to infection in association with a secondary bile acid (Buffie et al., 2015). Depleting specific gut microbes responsible for converting primary bile acids into secondary antimicrobial bile acids could be associated with increased risk of CDI (Theriot et al., 2016; Winston and Theriot, 2016).
Clostridium species are among the best-described users of free amino acids as energy sources. Amino acids regulate in vitro toxin production and support colonization of C. difficile in antibiotic-treated mice. Dysbiotic microbial communities showed significantly decreased expression of multiple genes related to amino acid uptake and metabolism, resulting in increased concentrations of 12 amino acids, with proline showing significant differences when compared to healthy mice microbiota. The ability to utilize proline provides a competitive advantage to C. difficile in germ-free mice transplanted with healthy-like and dysbiotic human stool consortia. Fecal microbiota transplant reduced free proline and decreased CDI susceptibility in dysbiotic mice (Mooyottu et al., 2017).
Recent studies have found increased indole levels (tryptophan metabolite involved in microbial growth, virulence induction, acid resistance, biofilm development) in the intestinal lumen of CDI patients, suggesting that C. difficile, which cannot produce this metabolite itself, would stimulate the production of indole by other bacteria to stop the growth and the development of indole-sensitive strains, including protective gut microbiota representatives, thus ensuring an intestinal environment conducive to its survival (Darkoh et al., 2019).
A recent study evaluated the relationship between the composition of the intestinal microbiota and level of fecal calprotectin in C. difficile asymptomatic and symptomatic patients. The asymptomatic patients have shown a modified microbiota, comparatively with the non-colonized patients, harboring significantly lower levels of Ruminococcaceae, Bilophila, Blautia, Faecalibacterium, Ruminococcus, and Sutterella, and higher levels of Enterobacteriaceae. In symptomatic patients the main devations of gut microbiota were represented by higher levels of Bacteroides and lower levels of Blautia, Phascolarctobacterium, Prevotella, and Succinivibrio. These gut microbiota changes in symptomatic patients were accompanied by significantly higher levels of fecal calprotectin, comparatively with asymptomatic patients and controls. These data suggest that association of microbiota and inflammatory markers could be used to differentiate C. difficile colonization (CDC) from CDI (Han et al., 2020).
Knowledge regarding the gut microbiota in C. difficile colonized patients may elucidate the mechanisms that allow for colonization whilst protecting against infection (Crobach et al., 2020). To this end, a recent study by Crobach et al. (2020) analyzed the bacterial signatures associated with resistance and susceptibility to CDC and CDI. Both CDC and CDI were associated with decreased gut microbial diversity and differences in the relative abundance of taxa such as Lachnospiraceae, Ruminococcaceae, Fusicatenibacter, Bacteroides, Veillonella, and Eubacterium hallii (Crobach et al., 2020).
During childhood, the intestinal microbiota is subject to many factors that shape the microbiota on short- and long-term. Apart from the maternal-fetal transmission of certain bacterial components, the microbiota is influenced by the type and the time of birth, the place of birth (hospitals or home births), the type of feeding (breastfeeding or artificial feeding), administration of probiotic and prebiotic supplements, dietary factors, antibiotics and other drugs, sex, and other genetic differences and environmental factors, such as exposure to pets, number of family members, rural or urban environment, hygiene, geographical factors (Combellick et al., 2018; Mohammadkhah et al., 2018; Akagawa et al., 2019).
Taking into account the numerous factors that could influence the intestinal microbiota of the newborns, the healthy profile of this age group is considered to be represented by the types of gut bacteria and the abundances found in vaginally delivered, exclusively breastfed and not exposed to antibiotics neonates (Arboleya et al., 2015; Combellick et al., 2018; Mohammadkhah et al., 2018; Akagawa et al., 2019). It has been hypothesized that bacterial colonization of the digestive tract begins in utero, the healthy placenta bearing a low biomass microbiome, composed of non-pathogenic species belonging to the Tenericutes, Firmicutes, Bacteroidetes, Proteobacteria, and Fusobacteria phyla, while in the amniotic fluid predominate Proteobacteria. However, the results reported by different studies are contradictory and depend on the sampling method and the culture-based or molecular-based approaches. Recent metagenomic studies conclude that the placenta does not harbor a specific, consistent and functional microbiota (Gschwind et al., 2020). The meconium harbors a cultivable microbiota, initially dominated by Bacteroides-Prevotella. The digestive contamination of the fetus occurs most probably by swallowing the amniotic fluid, starting with the 10th week after conception (Chong et al., 2018). The transfer of Enterococcus faecium from pregnant female mice into meconium was also demonstrated experimentally (Jimenez et al., 2008). All these lead to the conclusion that changes in the maternal internal environment may affect both fetal and newborn development (Zhuang et al., 2019).
Neonates are uniquely susceptible to C. difficile colonization because of the neonatal intestine’s immaturity and intestinal microbiota instability (Lees et al., 2016). The main source of colonization seems to be the environmental exposure to C. difficile spores within the nursery or healthcare environment rather than the mother, the rates of C. difficile detection increasing with the length of stay in these units.
In infants <1 month of age, C. difficile has an average colonization rate of 37%, ranging between 0 and 61%. Between 1 and 6 months of age, the colonization rate is still high at 30% and drops to about 10% by the end of the first year of life (Kuiper et al., 2017). However, the colonization rates reported by different studies vary from 14 to 71% in children <12 months of age. This age group is most commonly colonized with non-toxigenic strains and they are asymptomatic (Khalaf et al., 2012). The asymptomatic carriage rate continues to drop until about 3 years of age, when it stabilizes to carriage rates of 0–3%, similar to those found in adults, together with a progressive raise in serum IgG antibody concentrations against toxins A and B between birth and 24 months of age (Antonara and Leber, 2016). Around 3 years of age, the intestinal microbiota of the child is stabilized, acquiring the characteristics of the adult microbiota, which might explain the increase of symptomatic CDI starting with this age.
Moreover, other studies are assuming that asymptomatic carriage of C. difficile is common in the young individuals of many other species, including dogs, pigs, and cattle (Deng and Swanson, 2015). In puppies, the association between lower bacterial community diversity and C. difficile colonization was statistically significant, and certain bacterial taxa were preferentially associated with C. difficile colonization (Berry et al., 2019). Similar associations have also been found in human studies. Unweaned puppies that were not colonized with C. difficile had higher relative abundance of taxa from the clostridia genera than unweaned puppies that were colonized with C. difficile (Berry et al., 2019).
Many studies have found higher colonization rates with C. difficile in formula-fed infants than in breastfed infants (Cooperstock et al., 1983; Jangi and Lamont, 2010). Also, the breastfed infants colonized by C. difficile had significantly lower colony counts than formula-fed infants, probably because the human colostrum contains neutralizing antibodies to toxins A and B (Rolfe and Song, 1995; Jangi and Lamont, 2010).
There are no studies comparing C. difficile carriage rate regarding the delivery mode, but it might have persistent effects on microbiota beyond infancy. The lack of “bacterial baptism” of vaginal birth or other confounding factors associated with cesarean delivery, as well as maternal obesity, antibiotic administration, gestational age and breastfeeding pattern, could influence the C. difficile carriage rate (Kyne et al., 2000; Liu et al., 2019). However, there were found a significantly higher number of Clostridia in the stool of children vaginally delivered (VD) than in those delivered by C-section (CS) (Reyman et al., 2019). No association was found with prematurity as a risk factor for C. difficile infection (Lees et al., 2016).
The question arises whether the newborns and adults, which are asymptomatic carriers of C. difficile, might have a particular gut microbiota composition that allows colonization to occur without any clinical manifestations. Lack of disease has also been related to immature or diminished receptor sites for toxin A along the intestinal epithelium (Keel and Songer, 2007). In exchange, CDI may be more likely to manifest in certain populations of infants harboring pathological intestinal conditions, such as those with Hirschsprung’s disease, all these demonstrating the link between C. difficile colonization and intestinal homeostasis (Sammons et al., 2013).
In conclusion, the high carriage rate of C. difficile colonization in neonates can be explained by the immaturity of the neonatal intestine and the presence of a less complex intestinal microbiota, as compared to adults. However, postnatal microbial species, together with the lack of receptors for clostridial toxins protect help babies from the deleterious effects of C. difficile toxins.
Considering that C. difficile only occasionally produces clinical manifestations in infants, one can state that a specific microbiota composition probably consolidating a specific environment in newborns helps protect babies from the deleterious effects of C. difficile toxins, which occur in dysbiotic adults.
The newborn microbiota is dominated by Gram-positive cocci, Enterobacteriaceae or Bifidobacteriaceae, with a sequential transition to a microbiota dominated by Bifidobacteriaceae. It is well known that Bifidobacteria upregulate IL-10 production by intestinal dendritic cells explaining the lack of clinical symptoms in infants colonized with C. difficile (Huurre et al., 2008). In CS neonates, decreased levels of T cells and CD4+ helper T cells were noticed, probably due to the failure of the immature infant immune system to activate an inflammatory response (Jangi and Lamont, 2010; Collins and Auchtung, 2017; Francino, 2018).
Prematurity might not be a risk factor for C. difficile infection (Lees et al., 2016), probably due to the fact that in general, the intestinal microbiota of the premature child is dominated by Proteobacteria, even if breastfed, and the species of Clostridium and Veillonella appear later. However, the microbiota of the premature infant is strongly influenced by pre- and postnatal antibiotic therapy (Staude et al., 2018). Hospitalization and antibiotic exposure induce indigenous microbiota imbalance (McFarland et al., 2016). Antibiotic treatment in neonate’s intensive care units (NICU) was associated with a lower C. difficile colonization rate, but colonization with C. difficile occurred rapidly after cessation of antibiotics. In children in the NICU, born prematurely, the colonization with Bifidobacteriaceae is delayed.
Breast milk protects against infections in infants due to the presence of immunological factors such as immunoglobulin A (IgA), including neutralizing antibodies to C. difficile toxins A and B (Jangi and Lamont, 2010). Ruminococcus (which is more commonly found in the gut of breastfed infants) is thought to inhibit the growth of Clostridia, thereby preventing colonization by C. difficile (Morelli, 2008).
Increased levels of immunoglobulin-producing cells in peripheral blood have been observed in CS infants, probably due to excessive exposure to antigens at the level of the vulnerable intestinal barrier. In addition, breastfeeding contributes to the maturation of the infant’s immune system and modulates microbiota development. The microbiota of breastfed children is less diverse but contains more Bifidobacterium spp., also explaining the protection against deleterious pro-inflammatory responses triggered by CDI (Derrien et al., 2019). However, Bifidobacteria significantly decrease in abundance upon cessation of breastfeeding.
In the clinical cases of neonate necrotizing enterocolitis (NEC), a decrease of bacterial diversity and of Bacteroidetes and Firmicutes phyla, as well as of Bifidobacteria, were observed, with the more frequent presence of potentially pathogenic organisms, such as Staphylococcus aureus, Enterococcus, Escherichia coli, Shigella spp., Citrobacter spp., Klebsiella spp. (Dobbler et al., 2017). Among the strictly anaerobic bacteria that have been associated with NEC, the majority belong to the Clostridium genus (e.g., C. butyricum, C. neonatale, C. perfringens, C. paraputrificum, and C. difficile have been associated with NEC in preterm neonates) (Zhou et al., 2015; Rozé et al., 2017; Schönherr-Hellec et al., 2018; Schönherr-Hellec and Aires, 2019). Moreover, a NEC-associated microbiota, such as C. perfringens has been identified in meconium samples (Heida et al., 2016).
The first step in C. difficile treatment is the de-escalation of antibiotic treatment. Depending on the degree of C. difficile risk induction, the antibiotics were divided into three groups: high (fluoroquinolones, 2nd and 3rd generation cephalosporins, clindamycin, ampicillin, broad-spectrum penicillins with inhibitors, except for ticarcillin with clavulanate, and piperacillin with tazobactam), moderate (macrolides, trimethoprim/sulfamethoxazole, other penicillins, and sulfonamides) and low risk (aminoglycosides, bacitracin, carbapenems, chloramphenicol, daptomycin, metronidazole, rifampicin, teicoplanin, tigecycline, tetracycline, and vancomycin) (Kukla et al., 2020). Current standard treatment for CDI involves treatment with antibiotics such as metronidazole, vancomycin, or fidaxomicin (Mills et al., 2018; Gnocchi et al., 2020). Vancomycin is the first-line antibiotic therapy for both first episode of infection and fulminant infections in adults (Esposito et al., 2015; Wang et al., 2020). Unfortunately, vancomycin is a strong disruptor of gut microbiota, while the rate of CDI recurrence after treatment cessation occurs in 20–30% of patients (Lessa et al., 2015; Winston and Theriot, 2016). On the other hand, metronidazole is used especially in the first episodes of mild acute CDI and less for severe disease because the concentrations in the colon become readily undetectable due to the fact that it is absorbed very quickly (Gnocchi et al., 2020). The rapid absorption from the gut is also reflected in a negligible effect on normal microbiota (Lessa et al., 2015; Chilton et al., 2018). Fidaxomicin can be used in C. difficile non-severe and also severe infections treatment due to the fact that it is poorly absorbed at the intestinal level, ensuring the persistence of killing concentration in the gut and has a narrow antimicrobial spectrum (e.g., Gram-positive and Gram-negative anaerobes and facultative aerobes) unaffecting the equilibrium of the normal intestinal microbiota (Tannock et al., 2010; Louie et al., 2011, 2012; Gnocchi et al., 2020). Moreover, in another study, vancomycin and metronidazole treatment, but not fidaxomicin were associated with the potentially pathogenic fungal operational taxonomic units’ emergence as well as with bacterial functions enriched for xenobiotic metabolism that could contribute to dysbiosis that could favor the occurrence, persistence and recurrence of CDI (Lamendella et al., 2018).
Adjunctive therapies are frequently used due to the important role of gut microbiota disturbances in C. difficile pathogenesis (Table 1 and Figure 1). Thus, specific manipulation of the microbiota to ameliorate dysbiotic changes and restore intestinal microbiota homeostasis could represent an essential part of the therapy (Table 2).
Figure 1. Comparison of C. difficile infection in neonates versus adults. The presence of postnatal microbial species (Bifidobacteria and Enterobacteriaceae) and immaturity of the immune system as well as the lack of receptors for clostridial toxins helps to protect babies from the deleterious effects of C. difficile toxins, which occur in dysbiotic adults. In individuals with disrupted microbiota, ingested C. difficile spores germinate in the presence of bile salts in the small intestine and target the colon epithelial cells, releasing the inflammatory toxins, which subsequently induce disruption of tight junctions, and production of inflammatory cytokines. Targeting the microbiota using various strategies-probiotics, prebiotics, synbiotics, postbiotics, non-toxigenic C. difficile (NTCD), fecal transplant (FMT)-has proven to be effective in alleviating CDI.
One way to modulate microbiota is using “biotics” with beneficial impact on resident microbiota that confer health benefits for the host, such as probiotics (e.g., Saccharomyces boulardii, Lactobacillus, Bifidobacterium, and probiotic mixtures), prebiotics, symbiotics or postbiotics (Surawicz et al., 2000; Na and Kelly, 2011; Maziade et al., 2015; Collins and Auchtung, 2017). According to International Scientific Association for Probiotics and Prebiotics (ISAPP)1, prebiotics are substrates different from fibers, that are selectively metabolized by host microorganisms. Synbiotics are complementary/synergistic mixtures comprising live microorganisms (probiotics) and prebiotics. In case of synergistics synbiotics the two components taken individually do not have to meet criteria for prebiotic or probiotic. Postbiotics are cellular fractions or structures prepared from inactivated microbes. The postbiotic preparations exclude filtrates or live cultures individual components, while inactivated probiotics are not considered automatically postbiotics, unless a health benefit is demonstrated.
Most data regarding the protective role of commensal bacteria against C. difficile infection were obtained by studying the effects of different probiotics. For example, Bifidobacterium breve (YH68), widely used in the field of food fermentation and biomedicine, has shown antibacterial activity against C. difficile, by inhibiting the growth, spore production, toxigenesis and virulence gene expression (Valdes-Varela et al., 2016; Yang and Yang, 2019), potentiating the effect of anti-C. difficile antibiotics in vitro (Yang and Yang, 2018) or preventing the occurrence of clinical manifestations in vivo (Yun et al., 2017). Probiotic use has been shown to decrease CDI incidence in high-risk populations by as much as 50%, especially when they are combined with prebiotics (Shen et al., 2017).
It was demonstrated that the administration of non-living bacteria or microbial components (e.g., proteins, lipids, or nucleic acids) has an immunostimulatory effect proving that the beneficial impact on the host health is due to the physical interaction of specific microbial components, but in order to be effective for a long period, these need continuous administration.
Many bacterial strains such as Bacillus clausii and Lactobacillus reuteri have been shown to secrete soluble compounds that directly inhibit C. difficile (Khalaf et al., 2012; Deng and Swanson, 2015; Antonara and Leber, 2016). Organisms that produce secondary bile acids, such as Clostridium scindens, enhance C. difficile colonization resistance (Winston and Theriot, 2016).
Fecal microbiota transplantation (FMT) is considered the most effective microbiota-targeted intervention for the treatment of antibiotic-refractory CDI (Arbel et al., 2017), but however, the long-term effects, including the risk of other diseases, are not known (Schaffler and Breitruck, 2018).
Another therapeutic approach is the administration of non-toxigenic C. difficile strains or a mixture of spore-forming commensals, which act by providing nutritional niche competition. Despite their efficiency in decreasing CDI recurrence, there is the risk of switching to the toxigenic phenotype (Brouwer et al., 2013; Khanna et al., 2016).
The available studies suggest that C. difficile colonization and infection are influenced by the presence, absence or abundance of certain bacteria in the human gut, which could generate favorable conditions for germination, proliferation and production of clostridial toxins which, on their turn, will alter the integrity of the intestinal mucosa. Therefore, the clinical manifestations and severity of CDI are linked to gut dysbiosis, that could have multiple causes, among which the administration of high-risk antibiotics. The presence of protective microbial species, together with the particularities of the immune system and lack of receptors for clostridial toxins could explain the fact that in children, despite the high carriage rate, the symptomatic and severe cases are rare. However, if there is a gut microbiota composition predisposing to C. difficile asymptomatic carriage or clinical infection still needs clarification. Also, the mechanisms involved in C. difficile crosstalk with the commensal microbiota and/or particular soluble compounds remain only partially explained. Adjunctive microbiota-targeting therapies based on probiotics, prebiotics, postbiotics, synbiotics, non-toxigenic bacteria or fecal microbiota transplantation proved to be very useful for the therapeutic management of CDI.
All authors contributed equally to this manuscript with the conception and design of the study, literature review and analysis, drafting and critical revision and editing, and final approval of the final version.
This research was funded by the Research Projects PN-III-P4-ID-PCCF-2016-0114, C1.2.PFE-CDI.2021-587, and CNFIS-FDI-2021-0405 awarded to M-CC.
The authors declare that the research was conducted in the absence of any commercial or financial relationships that could be construed as a potential conflict of interest.
All claims expressed in this article are solely those of the authors and do not necessarily represent those of their affiliated organizations, or those of the publisher, the editors and the reviewers. Any product that may be evaluated in this article, or claim that may be made by its manufacturer, is not guaranteed or endorsed by the publisher.
Abt, M. C., McKenney, P. T., and Pamer, E. G. (2016). Clostridium difficile colitis: pathogenesis and host defence. Nat. Rev. Microbiol. 14, 609–620. doi: 10.1038/nrmicro.2016.108
Akagawa, S., Tsuji, S., Onuma, C., Akagawa, Y., Yamaguchi, T., Yamagishi, M., et al. (2019). Effect of delivery mode and nutrition on gut microbiota in neonates. Ann. Nutr. Metab. 74, 132–139. doi: 10.1159/000496427
Aktories, K., Schwan, C., and Jank, T. (2017). Clostridium difficile toxin biology. Annu. Rev. Microbiol. 71, 281–307.
Amrane, S., Hocquart, M., Afouda, P., Kuete, E., Pham, T. P., Dione, N., et al. (2019). Metagenomic and culturomic analysis of gut microbiota dysbiosis during Clostridium difficile infection. Sci. Rep. 9:12807. doi: 10.1038/s41598-019-49189-8
Antharam, V. C., Li, E. C., Ishmael, A., Sharma, A., Mai, V., Rand, K. H., et al. (2013). Intestinal dysbiosis and depletion of butyrogenic bacteria in Clostridium difficile infection and nosocomial diarrhea. J. Clin. Microbiol. 51, 2884–2892. doi: 10.1128/JCM.00845-13
Antonara, S., and Leber, A. L. (2016). Diagnosis of Clostridium difficile infections in children. J. Clin. Microbiol. 54, 1425–1433.
Arbel, L. T., Hsu, E., and McNally, K. (2017). Cost-effectiveness of fecal microbiota transplantation in the treatment of recurrent Clostridium difficile infection: a literature review. Cureus 9:e1599.
Arboleya, S., Sanchez, B., Milani, C., Duranti, S., Solis, G., Fernandez, N., et al. (2015). Intestinal microbiota development in preterm neonates and effect of perinatal antibiotics. J. Pediatr. 166, 538–544. doi: 10.1016/j.jpeds.2014.09.041
Avni, T., Babitch, T., Ben-Zvi, H., Hijazi, R., Ayada, G., Atamna, A., et al. (2020). Clostridioides difficile infection in immunocompromised hospitalized patients is associated with a high recurrence rate. Int. J. infect. Dis. 90, 237–242. doi: 10.1016/j.ijid.2019.10.028
Barker, A. K., Duster, M., Valentine, S., Hess, T., Archbald-Pannone, L., Guerrant, R., et al. (2017). A randomized controlled trial of probiotics for Clostridium difficile infection in adults (PICO). J. Antimicrob. Chemother. 72, 3177–3180. doi: 10.1093/jac/dkx254
Battaglioli, E. J., Hale, V. L., Chen, J., Jeraldo, P., Ruiz-Mojica, C., Schmidt, B. A., et al. (2018). Clostridioides difficile uses amino acids associated with gut microbial dysbiosis in a subset of patients with diarrhea. Sci. Transl. Med. 10:eaam7019. doi: 10.1126/scitranslmed.aam7019
Berkell, M., Mysara, M., Xavier, B. B., van Werkhoven, C. H., Monsieurs, P., Lammens, C., et al. (2021). Microbiota-based markers predictive of development of Clostridioides difficile infection. Nat. Commun. 12:2241. doi: 10.1038/s41467-021-22302-0
Berry, A. S. F., Kelly, B. J., Barnhart, D., Kelly, D. J., Beiting, D. P., Baldassano, R. N., et al. (2019). Gut microbiota features associated with Clostridioides difficile colonization in puppies. PLoS One 14:e0215497. doi: 10.1371/journal.pone.0215497
Borali, E., and De Giacomo, C. (2016). Clostridium Difficile infection in children: a review. J. Pediatr. Gastroenterol. Nutr. 63, e130–e140.
Britton, R. A., and Young, V. B. (2014). Role of the intestinal microbiota in resistance to colonization by Clostridium difficile. Gastroenterology 146, 1547–1553.
Brouwer, M. S., Roberts, A. P., Hussain, H., Williams, R. J., Allan, E., and Mullany, P. (2013). Horizontal gene transfer converts non-toxigenic Clostridium difficile strains into toxin producers. Nat. Commun. 4:2601. doi: 10.1038/ncomms3601
Buffie, C. G., Bucci, V., Stein, R. R., McKenney, P. T., Ling, L., Gobourne, A., et al. (2015). Precision microbiome reconstitution restores bile acid mediated resistance to Clostridium difficile. Nature 517, 205–208. doi: 10.1038/nature13828
Cama, V., Pieragostini, L., Fontanelli, G., Velletri, M. R., and Mariarosa, C. (2019). Clostridium difficile severe infection in a newborn. Arch. Dis. Child. 104, A311.
Carding, S., Verbeke, K., Vipond, D. T., Corfe, B. M., and Owen, L. J. (2015). Dysbiosis of the gut microbiota in disease. Microb. Ecol. Health Dis. 26:26191.
Chang, J. Y., Antonopoulos, D. A., Kalra, A., Tonelli, A., Khalife, W. T., Schmidt, T. M., et al. (2008). Decreased diversity of the fecal microbiome in recurrent Clostridium difficile-associated diarrhea. J. Infect. Dis. 197, 435–438. doi: 10.1086/525047
Chilton, C. H., Pickering, D. S., and Freeman, J. (2018). Microbiologic factors affecting Clostridium difficile recurrence. Clin. Microbiol. Infect. 24, 476–482. doi: 10.1016/j.cmi.2017.11.017
Chong, C. Y. L., Bloomfield, F. H., and O’Sullivan, J. M. (2018). Factors affecting gastrointestinal microbiome development in neonates. Nutrients 10:274. doi: 10.3390/nu10030274
Collins, J., and Auchtung, J. M. (2017). Control of Clostridium difficile infection by defined microbial communities. Microbiol. Spectr. 5, 1–25.
Combellick, J. L., Shin, H., Shin, D., Cai, Y., Hagan, H., Lacher, C., et al. (2018). Differences in the fecal microbiota of neonates born at home or in the hospital. Sci. Rep. 8:15660.
Cooperstock, M., Riegle, L., Woodruff, C. W., and Onderdonk, A. (1983). Influence of age, sex, and diet on asymptomatic colonization of infants with Clostridium difficile. J. Clin. Microbiol. 17, 830–833. doi: 10.1128/jcm.17.5.830-833.1983
Crobach, M. J. T., Ducarmon, Q. R., Terveer, E. M., Harmanus, C., Sanders, I. M. J. G., Verduin, K. M., et al. (2020). The bacterial gut microbiota of adult patients infected, colonized or noncolonized by Clostridioides difficile. Microorganisms 8:677. doi: 10.3390/microorganisms8050677
Czepiel, J., Drozdz, M., Pituch, H., Kuijper, E. J., Perucki, W., Mielimonka, A., et al. (2019). Clostridium difficile infection: review. Eur. J. Clin. Microbiol. Infect. Dis. 38, 1211–1221.
Darkoh, C., Plants-Paris, K., Bishoff, D., and DuPont, H. L. (2019). Clostridium difficile modulates the gut microbiota by inducing the production of indole, an interkingdom signaling and antimicrobial molecule. mSystems 4, e00346–e00318. doi: 10.1128/mSystems.00346-18
den Besten, G., van Eunen, K., Groen, A. K., Venema, K., Reijngoud, D. J., and Bakker, B. M. (2013). The role of short-chain fatty acids in the interplay between diet, gut microbiota, and host energy metabolism. J. Lipid Res. 54, 2325–2340. doi: 10.1194/jlr.R036012
Deng, P., and Swanson, K. S. (2015). Gut microbiota of humans, dogs and cats: current knowledge and future opportunities and challenges. Br. J. Nutr. 113(Suppl.), S6–S17. doi: 10.1017/S0007114514002943
Depestel, D. D., and Aronoff, D. M. (2013). Epidemiology of Clostridium difficile infection. J. Pharm. Pract. 26, 464–475.
Derrien, M., Alvarez, A. S., and de Vos, W. M. (2019). The gut microbiota in the first decade of life. Trends Microbiol. 27, 997–1010. doi: 10.1016/j.tim.2019.08.001
Dieterle, M. G., Rao, K., and Young, V. B. (2019). Novel therapies and preventative strategies for primary and recurrent Clostridium difficile infections. Ann. N.Y. Acad. Sci. 1435, 110–138. doi: 10.1111/nyas.13958
Dobbler, P. T., Procianoy, R. S., Mai, V., Silveira, R. C., Corso, A. L., Rojas, B. S., et al. (2017). Low microbial diversity and abnormal microbial succession is associated with necrotizing enterocolitis in preterm infants. Front. Microbiol. 8:2243. doi: 10.3389/fmicb.2017.02243
Dogra, S. K., Dore, J., and Damak, S. (2020). Gut microbiota resilience: definition, link to health and strategies for intervention. Front. Microbiol. 11:572921. doi: 10.3389/fmicb.2020.572921
Dupont, H. L. (2011). Gastrointestinal infections and the development of irritable bowel syndrome. Curr. Opin. Infect. Dis. 24, 503–508. doi: 10.1097/QCO.0b013e32834a962d
Esposito, S., Umbrello, G., Castellazzi, L., and Principi, N. (2015). Treatment of Clostridium difficile infection in pediatric patients. Exp. Rev. Gastroenterol. Hepatol. 9, 747–755. doi: 10.1586/17474124.2015.1039988
Fachi, J. L., Felipe, J. S., Pral, L. P., da Silva, B. K., Correa, R. O., de Andrade, M. C. P., et al. (2019). Butyrate protects mice from Clostridium difficile-induced colitis through an HIF-1-dependent mechanism. Cell Rep. 27, 750–761.e7. doi: 10.1016/j.celrep.2019.03.054
Faith, J. J., Guruge, J. L., Charbonneau, M., Subramanian, S., Seedorf, H., Goodman, A. L., et al. (2013). The long-term stability of the human gut microbiota. Science 341:1237439.
Fashner, J., Garcia, M., Ribble, L., and Crowell, K. (2011). Clinical inquiry: what risk factors contribute to C difficile diarrhea? J. Family Pract. 60, 545–547.
Ferraris, L., Couturier, J., Eckert, C., Delannoy, J., Barbut, F., Butel, M. J., et al. (2020). Carriage and colonization of C. difficile in preterm neonates: a longitudinal prospective study. PLoS One 14:e0212568. doi: 10.1371/journal.pone.0212568
Folke, C., Carpenter, S., Walker, B., Scheffer, M., Elmqvist, T., Gunderson, L., et al. (2004). Regime shifts, resilience, and biodiversity in ecosystem management. Annu. Rev. Ecol. Evol. Syst. 35, 557–581.
Francino, M. P. (2018). Birth mode-related differences in gut microbiota colonization and immune system development. Ann. Nutr. Metab. 73(Suppl. 3), 12–16. doi: 10.1159/000490842
Franzosa, E. A., Sirota-Madi, A., Avila-Pacheco, J., Fornelos, N., Haiser, H. J., Reinker, S., et al. (2019). Gut microbiome structure and metabolic activity in inflammatory bowel disease. Nat. Microbiol. 4, 293–305.
Gerding, D. N., Meyer, T., Lee, C., Cohen, S. H., Murthy, U. K., Poirier, A., et al. (2015). Administration of spores of nontoxigenic Clostridium difficile strain M3 for prevention of recurrent C. difficile infection: a randomized clinical trial. JAMA 313, 1719–1727. doi: 10.1001/jama.2015.3725
Gevers, D., Kugathasan, S., Denson, L. A., Vazquez-Baeza, Y., Van Treuren, W., Ren, B., et al. (2014). The treatment-naive microbiome in new-onset Crohn’s disease. Cell Host Microbe 15, 382–392.
Ghose, C. (2013). Clostridium difficile infection in the twenty-first century. Emerg. Microbes Infect. 2:e62. doi: 10.1038/emi.2013.62
Gnocchi, M., Gagliardi, M., Gismondi, P., Gaiani, F., De’ Angelis, G. L., and Esposito, S. (2020). Updated management guidelines for Clostridioides difficile in paediatrics. Pathogens 9:291. doi: 10.3390/pathogens9040291
Goethel, A., Turpin, W., Rouquier, S., Zanello, G., Robertson, S. J., Streutker, C. J., et al. (2019). Nod2 influences microbial resilience and susceptibility to colitis following antibiotic exposure. Mucosal Immunol. 12, 720–732. doi: 10.1038/s41385-018-0128-y
Goh, Y. J., and Klaenhammer, T. R. (2015). Genetic mechanisms of prebiotic oligosaccharide metabolism in probiotic microbes. Annu. Rev. Food Sci. Technol. 6, 137–156. doi: 10.1146/annurev-food-022814-015706
Gough, E., Shaikh, H., and Manges, A. R. (2011). Systematic review of intestinal microbiota transplantation (Fecal Bacteriotherapy) for recurrent Clostridium difficile infection. Clin. Infect. Dis. 53, 994–1002. doi: 10.1093/cid/cir632
Gschwind, R., Fournier, T., Kennedy, S., Tsatsaris, V., Cordier, A. G., Barbut, F., et al. (2020). Evidence for contamination as the origin for bacteria found in human placenta rather than a microbiota. PLoS One 15:e0237232. doi: 10.1371/journal.pone.0237232
Guilloteau, P., Martin, L., Eeckhaut, V., Ducatelle, R., Zabielski, R., and Van Immerseel, F. (2010). From the gut to the peripheral tissues: the multiple effects of butyrate. Nutr. Res. Rev. 23, 366–384. doi: 10.1017/S0954422410000247
Gupta, P., Yakubov, S., Tin, K., Zea, D., Garankina, O., Ghitan, M., et al. (2016). Does alkaline colonic pH predispose to Clostridium difficile infection? Southern Med. J. 109, 91–96. doi: 10.14423/SMJ.0000000000000414
Hamilton, M. J., Weingarden, A. R., Unno, T., Khoruts, A., and Sadowsky, M. J. (2013). High-throughput DNA sequence analysis reveals stable engraftment of gut microbiota following transplantation of previously frozen fecal bacteria. Gut Microbes 4, 125–135.
Han, S. H., Yi, J., Kim, J. H., and Moon, A. H. (2020). Investigation of intestinal microbiota and fecal calprotectin in non-toxigenic and toxigenic Clostridioides difficile colonization and infection. Microorganisms 8:882. doi: 10.3390/microorganisms8060882
Hanchi, H., Hammami, R., Gingras, H., Kourda, R., Bergeron, M. G., Ben Hamida, J., et al. (2017). Inhibition of MRSA and of Clostridium difficile by durancin 61A: synergy with bacteriocins and antibiotics. Future Microbiol. 12, 205–212. doi: 10.2217/fmb-2016-0113
Hatziioanou, D., Gherghisan-Filip, C., Saalbach, G., Horn, N., Wegmann, U., Duncan, S. H., et al. (2017). Discovery of a novel lantibiotic nisin O from Blautia obeum A2-162, isolated from the human gastrointestinal tract. Microbiology 163, 1292–1305. doi: 10.1099/mic.0.000515
Heida, F. H., van Zoonen, A. G. J. F., Hulscher, J. B. F., Te Kiefte, B. J. C., Wessels, R., Kooi, E. M. W., et al. (2016). A necrotizing enterocolitis-associated gut microbiota is present in the meconium: results of a prospective study. Clin. Infect. Dis. 62, 863–870. doi: 10.1093/cid/ciw016
Hensgens, M. P., Goorhuis, A., van Kinschot, C. M., Crobach, M. J., Harmanus, C., and Kuijper, E. J. (2011). Clostridium difficile infection in an endemic setting in the Netherlands. Eur. J. Clin. Microbiol. Infect. Dis. 30, 587–593. doi: 10.1007/s10096-010-1127-4
Hernandez, M., de Frutos, M., Rodriguez-Lazaro, D., Lopez-Urrutia, L., Quijada, N. M., and Eiros, J. M. (2018). Fecal microbiota of toxigenic Clostridioides difficile-associated Diarrhea. Front. Microbiol. 9:3331. doi: 10.3389/fmicb.2018.03331
Hills, R. D. Jr., Pontefract, B. A., Mishcon, H. R., Black, C. A., Sutton, S. C., and Theberge, C. R. (2019). Gut microbiome: profound implications for diet and disease. Nutrients 11:1613. doi: 10.3390/nu11071613
Hota, S. S., Sales, V., Tomlinson, G., Salpeter, M. J., McGeer, A., Coburn, B., et al. (2017). Oral vancomycin followed by fecal transplantation versus tapering oral vancomycin treatment for recurrent Clostridium difficile infection: an open-label, randomized controlled trial. Clin. Infect. Dis. 64, 265–271. doi: 10.1093/cid/ciw731
Human Microbiome Project, C. (2012). Structure, function and diversity of the healthy human microbiome. Nature 486, 207–214. doi: 10.1038/nature11234
Huurre, A., Kalliomaki, M., Rautava, S., Rinne, M., Salminen, S., and Isolauri, E. (2008). Mode of delivery - effects on gut microbiota and humoral immunity. Neonatology 93, 236–240. doi: 10.1159/000111102
Jangi, S., and Lamont, J. T. (2010). Asymptomatic colonization by Clostridium difficile in infants: implications for disease in later life. J. Pediatr. Gastroenterol. Nutr. 51:2. doi: 10.1097/MPG.0b013e3181d29767
Jimenez, E., Marin, M. L., Martin, R., Odriozola, J. M., Olivares, M., Xaus, J., et al. (2008). Is meconium from healthy newborns actually sterile? Res. Microbiol. 159, 187–193. doi: 10.1016/j.resmic.2007.12.007
Kachrimanidou, M., and Tsintarakis, E. (2020). Insights into the role of human gut microbiota in clostridioides difficile infection. Microorganisms 8:200. doi: 10.3390/microorganisms8020200
Keel, M. K., and Songer, J. G. (2007). The distribution and density of Clostridium difficile toxin receptors on the intestinal mucosa of neonatal pigs. Vet. Pathol. 44, 814–822. doi: 10.1354/vp.44-6-814
Kelly, C. R., Khoruts, A., Staley, C., Sadowsky, M. J., Abd, M., Alani, M., et al. (2016). Effect of fecal microbiota transplantation on recurrence in multiply recurrent Clostridium difficile infection: a randomized trial. Ann. Intern. Med. 165, 609–616. doi: 10.7326/M16-0271
Khalaf, N., Crews, J. D., DuPont, H. L., and Koo, H. L. (2012). Clostridium difficile: an emerging pathogen in children. Discov. Med. 14, 105–113.
Khanna, S., Pardi, D. S., Kelly, C. R., Kraft, C. S., Dhere, T., Henn, M. R., et al. (2016). A novel microbiome therapeutic increases gut microbial diversity and prevents recurrent Clostridium difficile infection. J. Infect. Dis. 214, 173–181. doi: 10.1093/infdis/jiv766
Kho, Z. Y., and Lal, S. K. (2018). The Human gut microbiome - a potential controller of wellness and disease. Front. Microbiol. 9:1835. doi: 10.3389/fmicb.2018.01835
Khoruts, A., Dicksved, J., Jansson, J. K., and Sadowsky, M. J. (2010). Changes in the composition of the human fecal microbiome after bacteriotherapy for recurrent Clostridium difficile-associated diarrhea. J. Clin. Gastroenterol. 44, 354–360. doi: 10.1097/MCG.0b013e3181c87e02
Kuiper, G. A., van Prehn, J., Ang, W., Kneepkens, F., van der Schoor, S., and de Meij, T. (2017). Clostridium difficile infections in young infants: case presentations and literature review. IDCases 10, 7–11. doi: 10.1016/j.idcr.2017.07.005
Kukla, M., Adrych, K., Dobrowolska, A., Mach, T., Regula, J., and Rydzewska, G. (2020). Guidelines for Clostridium difficile infection in adults. Prz. Gastroenterol. 15, 1–21. doi: 10.5114/pg.2020.93629
Kyne, L., Warny, M., Qamar, A., and Kelly, C. P. (2000). Asymptomatic carriage of Clostridium difficile and serum levels of IgG antibody against toxin A. N. Engl. J. Med. 342, 390–397. doi: 10.1056/NEJM200002103420604
Lamendella, R., Wright, J. R., Hackman, J., McLimans, C., Toole, D. R., Bernard Rubio, W., et al. (2018). Antibiotic treatments for Clostridium difficile infection are associated with distinct bacterial and fungal community structures. mSphere 3, e00572-17. doi: 10.1128/mSphere.00572-17
Lawler, A. J., Lambert, P. A., and Worthington, T. (2020). A revised understanding of Clostridioides difficile spore germination. Trends Microbiol. 28, 744–752. doi: 10.1016/j.tim.2020.03.004
Lazar, V., Ditu, L. M., Pircalabioru, G. G., Gheorghe, I., Curutiu, C., Holban, A. M., et al. (2018). Aspects of gut microbiota and immune system interactions in infectious diseases, immunopathology, and cancer. Front. Immunol. 9:1830. doi: 10.3389/fimmu.2018.01830
Lazar, V., Ditu, L. M., Pircalabioru, G. G., Picu, A., Petcu, L., Cucu, N., et al. (2019). Gut microbiota, host organism, and diet trialogue in diabetes and obesity. Front. Nutr. 6:21. doi: 10.3389/fnut.2019.00021
Lees, E. A., Miyajima, F., Pirmohamed, M., and Carrol, E. D. (2016). The role of Clostridium difficile in the paediatric and neonatal gut - a narrative review. Eur. J. Clin. Microbiol. Infect. Dis. 35, 1047–1057. doi: 10.1007/s10096-016-2639-3
Lessa, F. C., Mu, Y., Bamberg, W. M., Beldavs, Z. G., Dumyati, G. K., Dunn, J. R., et al. (2015). Burden of Clostridium difficile infection in the United States. N. Engl. J. Med. 372, 825–834.
Levine, J. M., and d’Antonio, C. M. (1999). Elton revisited: a review of evidence linking diversity and invasibility. Oikos 87, 15–26.
Liu, Y., Qin, S., Song, Y., Feng, Y., Lv, N., Xue, Y., et al. (2019). The perturbation of infant gut microbiota caused by cesarean delivery is partially restored by exclusive breastfeeding. Front. Microbiol. 10:598. doi: 10.3389/fmicb.2019.00598
Lo Presti, A., Zorzi, F., Del Chierico, F., Altomare, A., Cocca, S., Avola, A., et al. (2019). Fecal and mucosal microbiota profiling in irritable bowel syndrome and inflammatory bowel disease. Front. Microbiol. 10:1655. doi: 10.3389/fmicb.2019.01655
Louie, T. J., Cannon, K., Byrne, B., Emery, J., Ward, L., Eyben, M., et al. (2012). Fidaxomicin preserves the intestinal microbiome during and after treatment of Clostridium difficile infection (CDI) and reduces both toxin reexpression and recurrence of CDI. Clin. Infect. Dis. 55(Suppl. 2), S132–S142. doi: 10.1093/cid/cis338
Louie, T. J., Miller, M. A., Mullane, K. M., Weiss, K., Lentnek, A., Golan, Y., et al. (2011). Fidaxomicin versus vancomycin for Clostridium difficile infection. N. Engl. J. Med. 364, 422–431.
Lozupone, C. A., Stombaugh, J. I., Gordon, J. I., Jansson, J. K., and Knight, R. (2012). Diversity, stability and resilience of the human gut microbiota. Nature 489, 220–230. doi: 10.1038/nature11550
Magne, F., Gotteland, M., Gauthier, L., Zazueta, A., Pesoa, S., Navarrete, P., et al. (2020). The firmicutes/bacteroidetes ratio: a relevant marker of gut dysbiosis in obese patients? Nutrients 12:1474. doi: 10.3390/nu12051474
Maziade, P. J., Pereira, P., and Goldstein, E. J. (2015). A decade of experience in primary prevention of Clostridium difficile infection at a community hospital using the probiotic combination Lactobacillus acidophilus CL1285, Lactobacillus casei LBC80R, and Lactobacillus rhamnosus CLR2 (Bio-K+). Clin. Infect. Dis. 60(Suppl. 2), S144–S147. doi: 10.1093/cid/civ178
McBurney, M. I., Davis, C., Fraser, C. M., Schneeman, B. O., Huttenhower, C., Verbeke, K., et al. (2019). Establishing what constitutes a healthy human gut microbiome: state of the science, regulatory considerations, and future directions. J. Nutr. 149, 1882–1895. doi: 10.1093/jn/nxz154
McDonald, L. C., Gerding, D. N., Johnson, S., Bakken, J. S., Carroll, K. C., Coffin, S. E., et al. (2018). Clinical practice guidelines for Clostridium difficile infection in adults and children: 2017 update by the Infectious Diseases Society of America (IDSA) and Society for Healthcare Epidemiology of America (SHEA). Clin. Infect. Dis. 66, e1–e48.
McFarland, L. V., Ozen, M., Dinleyici, E. C., and Goh, S. (2016). Comparison of pediatric and adult antibiotic-associated diarrhea and Clostridium difficile infections. World J. Gastroenterol. 22, 3078–3104. doi: 10.3748/wjg.v22.i11.3078
Milani, C., Ticinesi, A., Gerritsen, J., Nouvenne, A., Lugli, G. A., Mancabelli, L., et al. (2016). Gut microbiota composition and Clostridium difficile infection in hospitalized elderly individuals: a metagenomic study. Sci. Rep. 6:25945. doi: 10.1038/srep25945
Mills, J. P., Rao, K., and Young, V. B. (2018). Probiotics for prevention of Clostridium difficile infection. Curr. Opin. Gastroenterol. 34, 3–10. doi: 10.1097/mog.0000000000000410
Mohammadkhah, A. I., Simpson, E. B., Patterson, S. G., and Ferguson, J. F. (2018). Development of the gut microbiome in children, and lifetime implications for obesity and cardiometabolic disease. Children 5:160. doi: 10.3390/children5120160
Mooyottu, S., Flock, G., Upadhyay, A., Upadhyaya, I., Maas, K., and Venkitanarayanan, K. (2017). Protective effect of carvacrol against gut dysbiosis and Clostridium difficile associated disease in a mouse model. Front. Microbiol. 8:625. doi: 10.3389/fmicb.2017.00625
Morelli, L. (2008). Postnatal development of intestinal microflora as influenced by infant nutrition. J. Nutr. 138, 1791S–1795S. doi: 10.1093/jn/138.9.1791S
Na, X., and Kelly, C. (2011). Probiotics in Clostridium difficile Infection. J. Clin. Gastroenterol. 45(Suppl.), S154–S158.
Nitzan, O., Elias, M., Chazan, B., Raz, R., and Saliba, W. (2013). Clostridium difficile and inflammatory bowel disease: role in pathogenesis and implications in treatment. World J. Gastroenterol. 19, 7577–7585. doi: 10.3748/wjg.v19.i43.7577
O’Keefe, S. J. (2010). Tube feeding, the microbiota, and Clostridium difficile infection. World J. Gastroenterol. 16, 139–142.
Orenstein, R., Dubberke, E., Hardi, R., Ray, A., Mullane, K., Pardi, D. S., et al. (2016). Safety and durability of RBX2660 (Microbiota Suspension) for recurrent Clostridium difficile infection: results of the PUNCH CD Study. Clin. Infect. Dis. 62, 596–602. doi: 10.1093/cid/civ938
Ott, S. J., Waetzig, G. H., Rehman, A., Moltzau-Anderson, J., Bharti, R., Grasis, J. A., et al. (2017). Efficacy of sterile fecal filtrate transfer for treating patients with Clostridium difficile infection. Gastroenterology 152, 799–811.e7. doi: 10.1053/j.gastro.2016.11.010
Pechal, A., Lin, K., Allen, S., and Reveles, K. (2016). National age group trends in Clostridium difficile infection incidence and health outcomes in United States community hospitals. BMC Infect. Dis. 16:682. doi: 10.1186/s12879-016-2027-8
Perez-Cobas, A. E., Moya, A., Gosalbes, M. J., and Latorre, A. (2015). Colonization resistance of the gut microbiota against Clostridium difficile. Antibiotics 4, 337–357.
Priya, S., and Blekhman, R. (2019). Population dynamics of the human gut microbiome: change is the only constant. Genome Biol. 20:150. doi: 10.1186/s13059-019-1775-3
Qin, J., Li, R., Raes, J., Arumugam, M., Burgdorf, K. S., Manichanh, C., et al. (2010). A human gut microbial gene catalogue established by metagenomic sequencing. Nature 464, 59–65. doi: 10.1038/nature08821
Rajilic-Stojanovic, M., Shanahan, F., Guarner, F., and de Vos, W. M. (2013). Phylogenetic analysis of dysbiosis in ulcerative colitis during remission. Inflamm. Bowel Dis. 19, 481–488. doi: 10.1097/MIB.0b013e31827fec6d
Ratsep, M., Koljalg, S., Sepp, E., Smidt, I., Truusalu, K., Songisepp, E., et al. (2017). A combination of the probiotic and prebiotic product can prevent the germination of Clostridium difficile spores and infection. Anaerobe 47, 94–103. doi: 10.1016/j.anaerobe.2017.03.019
Raymond, F., Ouameur, A. A., Déraspe, M., Iqbal, N., Gingras, H., Dridi, B., et al. (2016). The initial state of the human gut microbiome determines its reshaping by antibiotics. ISME J. 10, 707–720. doi: 10.1038/ismej.2015.148
Rea, M. C., O’Sullivan, O., Shanahan, F., O’Toole, P. W., Stanton, C., Ross, R. P., et al. (2012). Clostridium difficile carriage in elderly subjects and associated changes in the intestinal microbiota. J. Clin. Microbiol. 50, 867–875. doi: 10.1128/JCM.05176-11
Reeves, A. E., Koenigsknecht, M. J., Bergin, I. L., and Young, V. B. (2012). Suppression of Clostridium difficile in the gastrointestinal tracts of germfree mice inoculated with a murine isolate from the family Lachnospiraceae. Infect. Immun. 80, 3786–3794. doi: 10.1128/IAI.00647-12
Reeves, A. E., Theriot, C. M., Bergin, I. L., Huffnagle, G. B., Schloss, P. D., and Young, V. B. (2011). The interplay between microbiome dynamics and pathogen dynamics in a murine model of Clostridium difficile Infection. Gut Microbes 2, 145–158. doi: 10.4161/gmic.2.3.16333
Reyman, M., van Houten, M. A., van Baarle, D., Bosch, A., Man, W. H., Chu, M., et al. (2019). Impact of delivery mode-associated gut microbiota dynamics on health in the first year of life. Nat. Commun. 10:4997.
Ridlon, J. M., Kang, D. J., Hylemon, P. B., and Bajaj, J. S. (2014). Bile acids and the gut microbiome. Curr. Opin. Gastroenterol. 30, 332–338.
Rinninella, E., Raoul, P., Cintoni, M., Franceschi, F., Miggiano, G. A. D., Gasbarrini, A., et al. (2019). What is the healthy gut microbiota composition? A changing ecosystem across age, environment, diet, and diseases. Microorganisms 7:14. doi: 10.3390/microorganisms7010014
Ripert, G., Racedo, S. M., Elie, A. M., Jacquot, C., Bressollier, P., and Urdaci, M. C. (2016). Secreted compounds of the probiotic Bacillus clausii strain O/C inhibit the cytotoxic effects induced by Clostridium difficile and Bacillus cereus toxins. Antimicrob. Agents Chemother. 60, 3445–3454. doi: 10.1128/AAC.02815-15
Rodriguez, C., Romero, E., Garrido-Sanchez, L., Alcain-Martinez, G., Andrade, R. J., Taminiau, B., et al. (2020). Microbiota insights in Clostridium difficile infection and inflammatory bowel disease. Gut Microbes 12:1725220.
Rodriguez, C., Taminiau, B., Korsak, N., Avesani, V., Van Broeck, J., Brach, P., et al. (2016). Longitudinal survey of Clostridium difficile presence and gut microbiota composition in a Belgian nursing home. BMC Microbiol. 16:229. doi: 10.1186/s12866-016-0848-7
Rolfe, R. D., and Song, W. (1995). Immunoglobulin and non-immunoglobulin components of human milk inhibit Clostridium difficile toxin A-receptor binding. J. Med. Microbiol. 42, 10–19. doi: 10.1099/00222615-42-1-10
Ross, C. L., Spinler, J. K., and Savidge, T. C. (2016). Structural and functional changes within the gut microbiota and susceptibility to Clostridium difficile infection. Anaerobe 41, 37–43. doi: 10.1016/j.anaerobe.2016.05.006
Rousseau, C., Lemée, L., Le Monnier, A., Poilane, I., Pons, J. L., and Collignon, A. (2011). Prevalence and diversity of Clostridium difficile strains in infants. J. Med. Microbiol. 60(Pt. 8), 1112–1118. doi: 10.1099/jmm.0.029736-0
Rozé, J. C., Ancel, P. Y., Lepage, P., Martin-Marchand, L., Al Nabhani, Z., Delannoy, J., et al. (2017). Nutrition EPIPAGE 2 study group; EPIFLORE study group. nutritional strategies and gut microbiota composition as risk factors for necrotizing enterocolitis in very-preterm infants. Am. J. Clin. Nutr. 106, 821–830. doi: 10.3945/ajcn.117.152967
Sammons, J. S., Toltzis, P., and Zaoutis, T. E. (2013). Clostridium difficile Infection in children. JAMA Pediatr. 167, 567–573.
Sartelli, M., Di Bella, S., McFarland, L. V., Khanna, S., Furuya-Kanamori, L., Abuzeid, N., et al. (2019). 2019 update of the WSES guidelines for management of Clostridioides (Clostridium) difficile infection in surgical patients. World J. Emerg. Surg.14:8. doi: 10.1186/s13017-019-0228-3
Schaffler, H., and Breitruck, A. (2018). Clostridium difficile - from colonization to infection. Front. Microbiol. 9:646. doi: 10.3389/fmicb.2018.00646
Schönherr-Hellec, S., and Aires, J. (2019). Clostridia and necrotizing enterocolitis in preterm neonates. Anaerobe 58, 6–12. doi: 10.1016/j.anaerobe.2019.04.005
Schönherr-Hellec, S., Klein, G. L., Delannoy, J., Ferraris, L., Rozé, J. C., Butel, M. J., et al. (2018). Clostridial strain-specific characteristics associated with necrotizing enterocolitis. Appl. Environ. Microbiol. 84, e02428-17. doi: 10.1128/AEM.02428-17
Seekatz, A. M., and Young, V. B. (2014). Clostridium difficile and the microbiota. J. Clin. Investig. 124, 4182–4189.
Shen, A. (2012). Clostridium difficile toxins: mediators of inflammation. J. Innate Immun. 4, 149–158. doi: 10.1159/000332946
Shen, N. T., Maw, A., Tmanova, L. L., Pino, A., Ancy, K., Crawford, C. V., et al. (2017). Timely use of probiotics in hospitalized adults prevents Clostridium difficile infection: a systematic review with meta-regression analysis. Gastroenterology 152, 1889–1900.e9. doi: 10.1053/j.gastro.2017.02.003
Smits, W. K., Lyras, D., Lacy, D. B., Wilcox, M. H., and Kuijper, E. J. (2016). Clostridium difficile infection. Nat. Rev. Dis. Primers 2:16020.
Solomon, K. (2013). The host immune response to Clostridium difficile infection. Ther. Adv. Infect. Dis. 1, 19–35. doi: 10.1177/2049936112472173
Song, J., Li, Q., Everaert, N., Liu, R., Zheng, M., Zhao, G., et al. (2020). Dietary inulin supplementation modulates short-chain fatty acid levels and cecum microbiota composition and function in chickens infected With Salmonella. Front. Microbiol. 11:584380. doi: 10.3389/fmicb.2020.584380
Sorg, J. A., and Sonenshein, A. L. (2008). Bile salts and glycine as cogerminants for Clostridium difficile spores. J. Bacteriol. 190, 2505–2512. doi: 10.1128/JB.01765-07
Sorg, J. A., and Sonenshein, A. L. (2010). Inhibiting the initiation of Clostridium difficile spore germination using analogs of chenodeoxycholic acid, a bile acid. J. Bacteriol. 192, 4983–4990. doi: 10.1128/JB.00610-10
Spinler, J. K., Auchtung, J., Brown, A., Boonma, P., Oezguen, N., Ross, C. L., et al. (2017). Next-generation probiotics targeting Clostridium difficile through precursor-directed antimicrobial biosynthesis. Infect. Immun. 85:e00303-17. doi: 10.1128/IAI.00303-17
Staude, B., Oehmke, F., Lauer, T., Behnke, J., Gopel, W., Schloter, M., et al. (2018). The microbiome and preterm birth: a change in paradigm with profound implications for pathophysiologic concepts and novel therapeutic strategies. BioMed. Res. Int. 2018, 7218187. doi: 10.1155/2018/7218187
Surawicz, C. M., McFarland, L. V., Greenberg, R. N., Rubin, M., Fekety, R., Mulligan, M. E., et al. (2000). The search for a better treatment for recurrent Clostridium difficile disease: use of high-dose vancomycin combined with Saccharomyces boulardii. Clin. Infect. Dis. 31, 1012–1017. doi: 10.1086/318130
Tannock, G. W., Munro, K., Taylor, C., Lawley, B., Young, W., Byrne, B., et al. (2010). A new macrocyclic antibiotic, fidaxomicin (OPT-80), causes less alteration to the bowel microbiota of Clostridium difficile-infected patients than does vancomycin. Microbiology 156(Pt. 11), 3354–3359. doi: 10.1099/mic.0.042010-0
Tap, J., Furet, J. P., Bensaada, M., Philippe, C., Roth, H., Rabot, S., et al. (2015). Gut microbiota richness promotes its stability upon increased dietary fibre intake in healthy adults. Environ. Microbiol. 17, 4954–4964. doi: 10.1111/1462-2920.13006
Theriot, C. M., Bowman, A. A., and Young, V. B. (2016). Antibiotic-induced alterations of the gut microbiota alter secondary bile acid production and allow for Clostridium difficile spore germination and outgrowth in the large intestine. mSphere 1:e00045-15. doi: 10.1128/mSphere.00045-15
Toor, D., Wsson, M. K., Kumar, P., Karthikeyan, G., Kaushik, N. K., Goel, C., et al. (2019). Dysbiosis disrupts gut immune homeostasis and promotes gastric diseases. Int. J. Mol. Sci. 20:2432. doi: 10.3390/ijms20102432
Vakili, B., Fateh, A., Asadzadeh Aghdaei, H., Sotoodehnejadnematalahi, F., and Siadat, S. D. (2020). Characterization of gut microbiota in hospitalized patients with Clostridioides difficile infection. Curr. Microbiol. 77, 1673–1680. doi: 10.1007/s00284-020-01980-x
Valdes, A. M., Walter, J., Segal, E., and Spector, T. D. (2018). Role of the gut microbiota in nutrition and health. BMJ 361:k2179.
Valdes-Varela, L., Hernandez-Barranco, A. M., Ruas-Madiedo, P., and Gueimonde, M. (2016). Effect of bifidobacterium upon Clostridium difficile growth and toxicity when co-cultured in different prebiotic substrates. Front. Microbiol. 7:738. doi: 10.3389/fmicb.2016.00738
Wang, Y., Schluger, A., Li, J., Gomez-Simmonds, A., Salmasian, H., and Freedberg, D. E. (2020). Does addition of intravenous metronidazole to oral vancomycin improve outcomes in Clostridioides difficile infection? Clin. Infect. Dis. 71, 2414–2420. doi: 10.1093/cid/ciz1115
Weingarden, A. R., Chen, C., Bobr, A., Yao, D., Lu, Y., Nelson, V. M., et al. (2014). Microbiota transplantation restores normal fecal bile acid composition in recurrent Clostridium difficile infection. Am. J. Physiol. Gastrointest. Liver Physiol. 306, G310–G319. doi: 10.1152/ajpgi.00282.2013
Wilcox, M. H., Mooney, L., Bendall, R., Settle, C. D., and Fawley, W. N. (2008). A case-control study of community-associated Clostridium difficile infection. J. Antimicrob. Chemother. 62, 388–396.
Wilson, B. C., Vatanen, T., Cutfield, W. S., and O’Sullivan, J. M. (2019). The super-donor phenomenon in fecal microbiota transplantation. Front. Cell. Infect. Microbiol. 9:2. doi: 10.3389/fcimb.2019.00002
Winston, J. A., and Theriot, C. M. (2016). Impact of microbial derived secondary bile acids on colonization resistance against Clostridium difficile in the gastrointestinal tract. Anaerobe 41, 44–50. doi: 10.1016/j.anaerobe.2016.05.003
Yang, J., and Yang, H. (2018). Effect of bifidobacterium breve in combination with different antibiotics on Clostridium difficile. Front. Microbiol. 9:2953. doi: 10.3389/fmicb.2018.02953
Yang, J., and Yang, H. (2019). Antibacterial activity of bifidobacterium breve against Clostridioides difficile. Front. Cell. Infect. Microbiol. 9:288. doi: 10.3389/fcimb.2019.00288
Yun, B., Song, M., Park, D. J., and Oh, S. (2017). Beneficial effect of Bifidobacterium longum ATCC 15707 on survival rate of Clostridium difficile infection in mice. Korean J. Food Sci. Anim. Resourc. 37, 368–375. doi: 10.5851/kosfa.2017.37.3.368
Zhang, L., Dong, D., Jiang, C., Li, Z., Wang, X., and Peng, Y. (2015). Insight into alteration of gut microbiota in Clostridium difficile infection and asymptomatic C. difficile colonization. Anaerobe 34, 1–7. doi: 10.1016/j.anaerobe.2015.03.008
Zhou, Y., Shan, G., Sodergren, E., Weinstock, G., Walker, W. A., and Gregory, K. E. (2015). Longitudinal analysis of the premature infant intestinal microbiome prior to necrotizing enterocolitis: a case-control study. PLoS One 10:e0118632. doi: 10.1371/journal.pone.0118632
Keywords: Clostridium difficile infection, gut microbiota, dysbiosis, biotics, fecal microbiota transplantation
Citation: Vasilescu I-M, Chifiriuc M-C, Pircalabioru GG, Filip R, Bolocan A, Lazăr V, Diţu L-M and Bleotu C (2022) Gut Dysbiosis and Clostridioides difficile Infection in Neonates and Adults. Front. Microbiol. 12:651081. doi: 10.3389/fmicb.2021.651081
Received: 09 April 2021; Accepted: 15 December 2021;
Published: 20 January 2022.
Edited by:
Axel Cloeckaert, Institut National de Recherche pour l’Agriculture, l’Alimentation et l’Environnement (INRAE), FranceReviewed by:
Felix Broecker, Idorsia Pharmaceuticals Ltd., SwitzerlandCopyright © 2022 Vasilescu, Chifiriuc, Pircalabioru, Filip, Bolocan, Lazăr, Diţu and Bleotu. This is an open-access article distributed under the terms of the Creative Commons Attribution License (CC BY). The use, distribution or reproduction in other forums is permitted, provided the original author(s) and the copyright owner(s) are credited and that the original publication in this journal is cited, in accordance with accepted academic practice. No use, distribution or reproduction is permitted which does not comply with these terms.
*Correspondence: Mariana-Carmen Chifiriuc, Y2FybWVuLmNoaWZpcml1Y0BnbWFpbC5jb20=
†These authors have contributed equally to this work
Disclaimer: All claims expressed in this article are solely those of the authors and do not necessarily represent those of their affiliated organizations, or those of the publisher, the editors and the reviewers. Any product that may be evaluated in this article or claim that may be made by its manufacturer is not guaranteed or endorsed by the publisher.
Research integrity at Frontiers
Learn more about the work of our research integrity team to safeguard the quality of each article we publish.