- 1AgroBioSciences Program, Mohammed VI Polytechnic University (UM6P), Ben Guerir, Morocco
- 2Laboratoire Commun de Microbiologie (LCM) IRD/ISRA/UCAD, Centre de Recherche de Bel Air, Dakar, Senegal
- 3Centre d’Excellence Africain en Agriculture pour la Sécurité Alimentaire et Nutritionnelle (CEA-AGRISAN), UCAD, Dakar, Senegal
- 4Département de Biologie Végétale, Faculté des Sciences et Techniques, Université Cheikh Anta Diop (UCAD), Dakar, Senegal
- 5Department of Environmental Science, School of Earth and Environmental Sciences, BBA University, Lucknow, India
- 6Department of Plant Protection, College of Agriculture Engineering Sciences, University of Baghdad, Baghdad, Iraq
- 7School of Biology Science, Universiti Sains Malaysia, Penang, Malaysia
- 8Laboratoire Campus de Biotechnologies Végétales (LCBV), Département de Biologie Végétale, Faculté des Sciences et Techniques, UCAD, Dakar, Senegal
- 9Laboratory of Microbial Biotechnologies, Agrosciences and Environment, Faculty of Sciences Semlalia, Cadi Ayyad University, Marrakesh, Morocco
Plant growth promoting microbes (PGPMs) play major roles in diverse ecosystems, including atmospheric nitrogen fixation, water uptake, solubilization, and transport of minerals from the soil to the plant. Different PGPMs are proposed as biofertilizers, biostimulants, and/or biocontrol agents to improve plant growth and productivity and thereby to contribute to agricultural sustainability and food security. However, little information exists regarding the use of PGPMs in micropropagation such as the in vitro plant tissue culture. This review presents an overview of the importance of PGPMs and their potential application in plant micropropagation. Our analysis, based on published articles, reveals that the process of in vitro classical tissue culture techniques, under strictly aseptic conditions, deserves to be reviewed to allow vitroplants to benefit from the positive effect of PGPMs. Furthermore, exploiting the potential benefits of PGPMs will lead to lessen the cost production of vitroplants during micropropagation process and will make the technique of plant tissue culture more efficient. The last part of the review will indicate where research is needed in the future.
Introduction
Plant tissue culture consists of producing, under aseptic conditions, a whole plant from an explant or even a single plant cell. This component of plant biotechnology relies on the phenomenon of cell totipotency, which is the ability of any single cells to produce all the differentiated cells characteristic of organs, and to regenerate into an entire plant (Trigiano and Gray, 2016). Micropropagation exploits this fundamental property of plant cells for the rapid mass multiplication of elite’s genotypes on large scales in a comparatively short period of time. Nowadays, micropropagation plays a considerable role in agriculture, horticulture, and industry through the production of healthy seedlings throughout the year, and the reduction of the vegetal cycle (Suman, 2017). It is also a core technology for conservation of plant genetic resources, crop improvement, and propagation of new varieties from somaclonal variation, and genetic manipulation. However, the technique requires the use of chemical disinfectants, variable concentrations of appropriate phytohormones, and sometimes antibiotics, antifungals, antivirals almost at each stage of growth and development process (Liang et al., 2019). Some of these plant growth regulators (PGRs) are very costly, and therefore, limit or restrain the expansion of this technology and its agricultural profitability.
To implement this technology and reduce the cost-intensive process, plant growth promoting microbes (PGPMs) can be used as a sustainable solution (Verma et al., 2019). Indeed, many PGPMs can synthesize phytohormones and various other organic compounds which can improve plant growth and productivity. Different microorganisms, including bacteria, archaea, and fungi, living in the plant rhizosphere and feeding on sloughed-off plant cells and the proteins and sugars released by roots (Hütsch et al., 2002; Al-Ani, 2019a,b), have been used as PGPMs in agriculture. Unfortunately, PGPMs are not sufficiently used in in vitro plant tissue culture, and only few studies have reported inoculation with PGPMs in micropropagation (Srivastava et al., 2002; Souza et al., 2015; Lopes et al., 2019). Because the presence of microorganisms in the in vitro environment was almost universally perceived as negative for in vitro plant culture (Orlikowska et al., 2017), most of the research dealing with micropropagation and microbes focused on the detection and elimination of contaminants. However, many PGPMs can help in rooting, shoot elongation, and they can be useful in the success of acclimatization phase. Indeed, they can protect against biotic and abiotic stress that occurs in vitro propagation mainly at the hardening and acclimatization phase; two crucial steps for the success of micropropagation. PGPMs are key components for achieving sustainable agriculture, and, therefore, fostering the use of PGPMs in micropropagation is challenging.
This review aims to highlight the potential role of PGPMs in in vitro plant tissue culture, with special emphasis on micropropagation. The possible contributions of PGPMs in the advancement of agricultural crop production and the current constraints of their use will be emphasized and discussed.
Plant Tissue Culture Technology
Micropropagation is an in vitro culture technique which allows the mass multiplication of a plant material from a plant segment named explant. The explant may consist of any part of the plant such as an immature embryo, a seed, a portion of leaves, roots, or shoots, an anther, a pollen grain, an ovule, a meristem, or an apex. Micropropagation of plants also means the process of using explants and allowing them to undergo growth of undifferentiated or differentiated cells (Bidabadi and Jain, 2020). The explant is grown in a culture container filled with an artificial nutrient culture medium under sterile conditions. In addition to mass multiplication of elite plants, plant tissue culture technology also provides the means to multiply and regenerate novel plants from genetically engineered cells. This technology improves cultures by producing somaclonal and gametoclonal variants (Suman, 2017). The process of micropropagation can be divided into six stages (Figure 1):
Stage 0: Plant stock immobilization and pre-treatments, selection of the explant.
Stage I: Culture establishment.
Stage II: Elongation and multiplication.
Stage III: Rooting.
Stage IV: Weaning, hardening, and acclimatization.
Stage V: Transfer under natural conditions (to the field).
The first four stages of the micropropagation process generally take place in a highly protected environment without the possibility of interaction with microbes normally found in nature (Orlikowska et al., 2017). Therefore, the regenerated plants are vulnerable when transferred directly to field conditions. So, it is important to consider the interaction with beneficial microbes such as symbiotic and non-symbiotic fungi and bacteria. In recent years, some scientific works have attempted to re-establish this link with beneficial microorganisms in the process of in vitro multiplication. These microorganisms can positively impact the growth of explants and ensure better survival by sustaining the transplantation shock into greenhouse or glasshouse and in field conditions (Weyens et al., 2009; Srinivasan et al., 2014).
Plant Growth Promoting Microbes and Their Multi-Functional Traits
Several soil microorganisms belonging to very different taxa have been identified as efficient PGPMs. Rhizospheric PGPMs are soil borne, living on root surfaces or colonizing the internal tissues of plants (named PGP endophytes) where they play different functions such as mineral solubilizing (Zn, P, and K), iron chelation, nitrogen fixation, production of phytohormones, and biocontrol ability against plant pathogens. Based on their activities, they are classified into three main groups corresponding to three growth promotion mechanisms (Figure 2):
i. Biofertilizers, they increase the availability of nutrients and their utilization by plants.
ii. Biostimulants or phytostimulants, produce beneficial substances such as PGRs, which are not nutrients, pesticides, or soil improvers.
iii. Biocontrol agents, they control pathogens development through the production of antimicrobial metabolites or competition for space and nutrients.
Some of PGPMs can display two to three plant growth promoting mechanisms. Through their multi-functional roles, PGPMs influence all aspects of plant life including seed germination, nutrition, growth, and response to biotic and/or abiotic stresses (Weyens et al., 2009; Subramaniam et al., 2020a,b; Sunita et al., 2020). PGPMs may enhance plant growth and protection by direct and/or indirect modes of action. The direct mechanisms enhance plant growth either by providing nutrients or by producing growth regulators, while indirect mechanisms help the plant to grow healthily under abiotic stresses or protect the plant against infections, parasites, or certain predators (biotic stresses; Goswami et al., 2016; Arora et al., 2020).
There are two main groups of PGPMs: plant growth promoting fungi (PGPF) and plant growth promoting bacteria (PGPB). Plants establish a variety of interactions with soil fungi. Diverse taxa, belonging to arbuscular mycorrhizal fungi (e.g., Gigaspora, Funneliformis, and Rhizophagus), orchid mycorrhizal fungi (Russula, Rhizoctonia, and Tulasnella species), ericoid mycorrhizal fungi (Harpophora oryzae and Colletotrichum tofieldiae), ectomycorrhial fungi (e.g., Laccaria, Pisolithus, and Scleroderma), Trichoderma spp., Piriformospora, and other root endophytes fungi such as Fusarium spp., Penicillium spp., Aspergillus spp., etc., have been recognized as PGPF. Arbuscular mycorrhizal fungi (AMF) establish symbioses with over 90% of all plant species and influence host plants at various growth stages (Begum et al., 2019). While orchid mycorrhiza (ORM) and ericoid mycorrhiza (ERM) occur in specific plant lineages, i.e., Orchidaceae and Ericaceae subfamilies, respectively, (Martin et al., 2016; Perotto et al., 2018). Ectomycorrhizal fungi (EMF) are associated with 10% of plant families and are the dominant group in temperate and boreal forests, where they play a major role in the biology and ecology of forest trees (Smith and Read, 2008). PGPF infect plants without causing symptoms and express different lifestyles (mutualistic, latent pathogen, and latent saprophyte) depending on host genotype, age, and physiology. However, a small proportion of fungi are latent pathogens (Promputtha et al., 2007; Zabalgogeazcoa, 2008; Yuan et al., 2010).
On the other hand, the PGPB group represent 2–5% of rhizospheric bacteria (Antoun and Prévost, 2005; Jha et al., 2010; Arora, 2015). They belong to the four bacterial groups including free-living bacteria, associative, endophytic bacteria, and nodule-forming bacteria (symbiotic). Like PGPF, they can act as biofertilizers, biostimulants, and/or biocontrol agents. The most widely exploited groups of PGPB belongs to genera of Pseudomonas, Bacillus, Azospirillum, Azotobacter, Rhizobium, Bradyrhizobium, Frankia, Burkholderia, Thiobacillus, Serratia, and Streptomyces (Adesemoye et al., 2008; Sivasakthivelan and Saranraj, 2013; Verma et al., 2019; Subramaniam et al., 2020a,b). The different interactions between the roots and surrounding soil PGPMs are summarized in Figure 3.
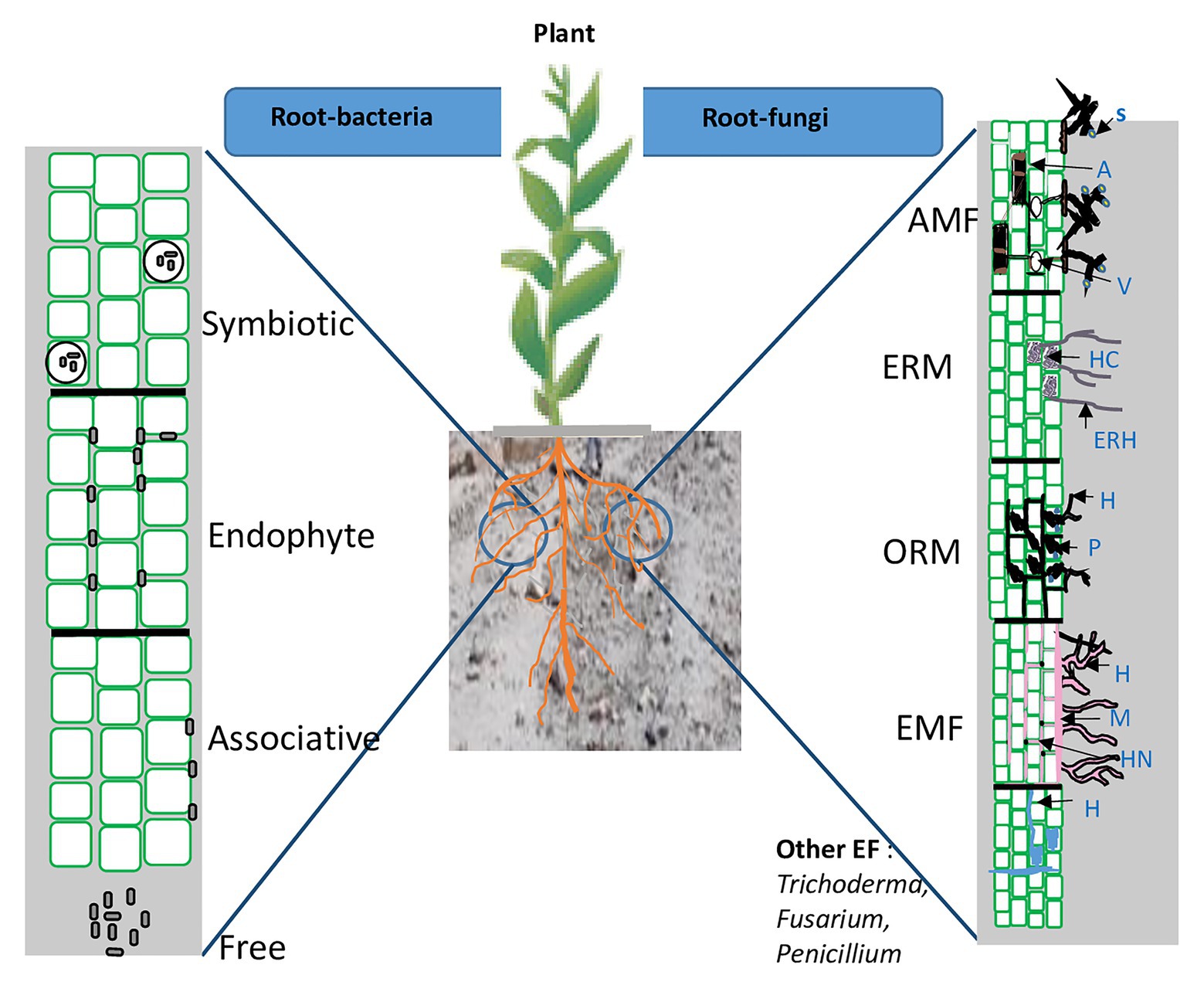
Figure 3. Schematic representation of root and rhizosphere colonization by beneficial microorganisms. AMF, arbuscular mycorrhizal fungi; ERM, ericoid mycorrhizal fungi; OMF, orchid mycorrhizal fungi; EMF, ectomycorrhizal fungi; EF, endophyte fungi; A, arbuscules; ERH, extraradical hyphae; V, vesicles; S, spore; HC, hyphal coils; P, peloton; HN, Hartig net; and M, mantle.
Plant Growth Regulators Required in Micropropagation Process
Plant growth regulators are organic compounds synthetized within plants in response to specific stimuli and occur in extremely low concentrations. These chemical messengers or signal molecules play critical roles in regulating and controlling growth, development, reproduction, and senescence of the plant. In other hand, they control all aspects of plant development, from embryogenesis (Méndez-Hernández et al., 2019), regulation of organ size, defense against pathogens (Shigenaga and Argueso, 2016), stress tolerance (Ku et al., 2018; Ullah et al., 2018), and reproductive development (Pierre-Jerome et al., 2018). PGRs allow the plant to adapt to changing environments, by mediating growth, development, and nutrient allocation (Dias, 2019). Based on their origin, PGRs are divided into three groups: synthesized by plants, microbial origin, and synthetic compounds (Blázquez et al., 2020). Nowadays, the term of “phytoregulators” is used for both synthetic and natural organic PGRs. Five main classes of PGRs can be distinguished based on their chemical structures and effects: (i) auxins, (ii) cytokinins, (iii) gibberellins, (iv) abscisic acid, and (v) ethylene. Beside these classical plant hormones, other PRGs such as polyamines, analogs of diphenyl urea, salicylic acid, jasmonates, sterols, brassinosteroids, strigolactones oligosaccharins, phosphoinositosides, systemins, and florigen were discovered more recently. Among all PGRs, auxin and cytokinin classes are usually considered to be the most important phytohormones in plant growth regulation because they regulate many metabolic processes (Pour et al., 2019). PGPMs have the potential to produce these two hormones. Table 1 summarizes the different auxin and cytokinin compounds commonly used in plant micropropagation.
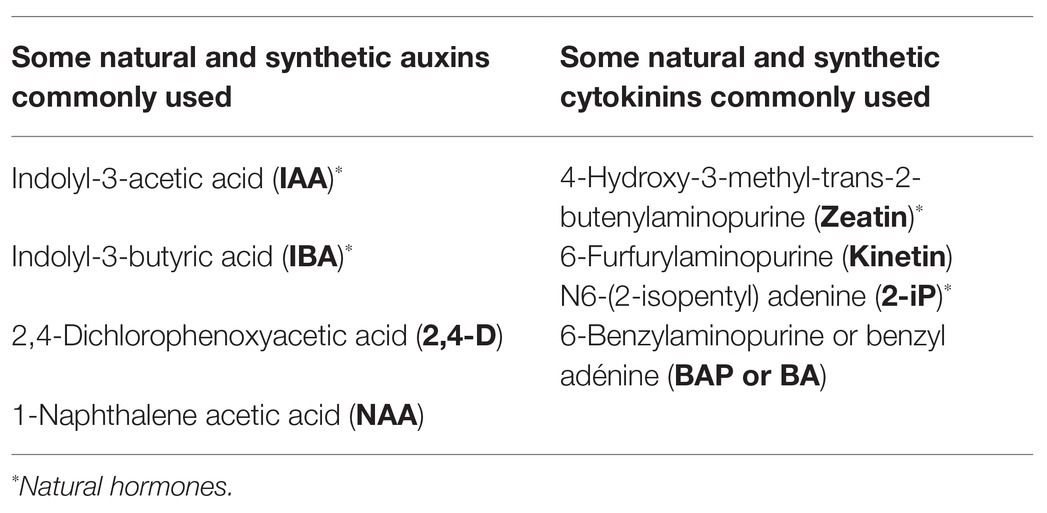
Table 1. Natural and synthetic auxin and cytokinin hormones commonly used in the micropropagation process of plants.
Plant Growth Promoting Microbes in Plant Tissue Culture
Traditionally, plant tissue culture systems are brought up in aseptic conditions. Thus, during the establishment of in vitro cultures, the explant is surface sterilized to eliminate all microorganisms. Since the role of PGPMs in plant growth and protection has been established, more attention has been paid to beneficial effects of these microorganisms in in vitro plant tissue cultures. In this respect, the use of competent PGPMs in micropropagation under in vitro and ex vitro conditions was analyzed and called “biotization” (Nowak, 1998). Microplant biotization is a biotechnological practice aimed at reducing chemical input in plant production (Kanani et al., 2020). The biotization can be done at all stages of in vitro propagation. In stage II and III of micropropagation by micro-cutting, PGPMs act generally as bio-stimulants by promoting elongation and increasing rooting, respectively, while in stage IV, they act as biocontrol agents and help to deal with biotic and abiotic stress factors (Figure 2). It is at this stage of acclimatization that biotization of microplants seems to be most important (Orlikowska et al., 2017). In addition to their three-main growth promoting mechanisms, certain PGPMs such as Rhizobium, Frankia, Bradyrhizobium, and mycorrhizal fungi have been recognized to be able to improve the physical properties of the soil by making it more conducive (Azcón-Aguilar and Barea, 2015; Egamberdieva et al., 2019).
Beneficial Effects of Plant Growth Promoting Fungi in in vitro Plant Culture
Plants from micropropagation are adversely affected by water stress, because of low absorption capacity of their roots. Inoculation with AMF in vitro is an important tool to deal with this problem (Rai, 2001). Through biosynthesis of phytohormones or PGRs, AMF impact on post-transplant performance of in vitro grown plants by increasing nutrients availability and inducing resistance to pathogens (Rai, 2001; Akin-Idowu et al., 2009). According to Chanclud et al. (2016), Kemler et al. (2017), and Streletskii et al. (2019), fungi produce phytohormones such as auxins, cytokinins (CKs), abscisic acid (ABA), gibberellic acids (GAs), ethylene (ET), salicylic acid (SA), and jasmonic acid (JA). These hormones control plant development and activate signaling pathways during biotic and/or abiotic stresses. Meixner et al. (2005) showed that, plants inoculated with AMF had a higher level of auxins than non-inoculated plants. It has been shown that a large diversity of fungal species can produce CKs for hyphal development and nutrient uptake during mycorrhizal symbiosis. Auxin and cytokinin act as messengers to regulate various cellular processes in plants such as bud activity, branching, cell cycle, synchronization of fruit setting and dropping (Müller and Leyser, 2011), plant defense responses (Naseem and Dandekar, 2012), grain size, and biomass production (Osugi and Sakakibara, 2015). A balance of both auxins and cytokinins leads to the development of callus, i.e., a mass of undifferentiated cells.
In addition, fungi especially AMF play important role in water uptake and availability (Püschel et al., 2020), thereby increasing the rate of photosynthesis and osmotic adjustment under environmental stresses (Soumare et al., 2015a). AMF also increase the uptake of micronutrients such as P, Zn, Cu, Fe, etc. AMF contribution is especially important during the acclimatization phase because the adventitious and weak root system, without root hair, of vitroplants do not allow optimal absorption of nutrients from the soil during the early stage of the weaning step. AMF can help to overcome this problem, through their arbuscules and hyphae which transfer nutrients, especially phosphate from the soil to the plant (Karandashov and Bucher, 2005; Chen et al., 2018). Beneficial endophytic fungi promote plant growth by improving uptake of phosphorus, potassium, and zinc and/or production of phytohormones such as cytokinins, indole acetic acids, and gibberrellic acids (Rana et al., 2019). The lower survival rate and poor establishment of vitroplants in field conditions may be due to the fact that the transferred vitroplants did not find their natural microsymbiont partner. In this respect, Varma and Schuepp (1994) have shown that hydrangea vitroplants inoculated with the AMF, Glomus intraradices (current name: Rhizophagus intraradices) were strongly mycorrhized at the acclimatization stage and, therefore, the survival rate was 100% and no apparent “transient transfer shock” was visualized. Dı́ez et al. (2000) showed that in vitro mycorrhization with Pisolithus tinctorius and Scleroderma polyrhizum strains increased the formation of secondary roots and the survival after acclimatization of cork oak vitroplants raised from somatic embryos. Similarly, Sahay and Varma (2000) reported a 90% post-transplantation survival rate of micropropaged tobacco and brinjal plants treated with the endophytic fungus, Piriformospora indica. This biopriming has also been reported to increase resistance against pathogen attacks (Harish et al., 2008). Reports of some successful biotization with endophytic fungi are enlisted in Table 2. Nevertheless, certain endophytic fungi can be plant pathogens and limit the micropropagation process. It is the case with Fusarium equiseti which was suspected to cause bamboo blight and culm rot disease (Tyagi et al., 2018).
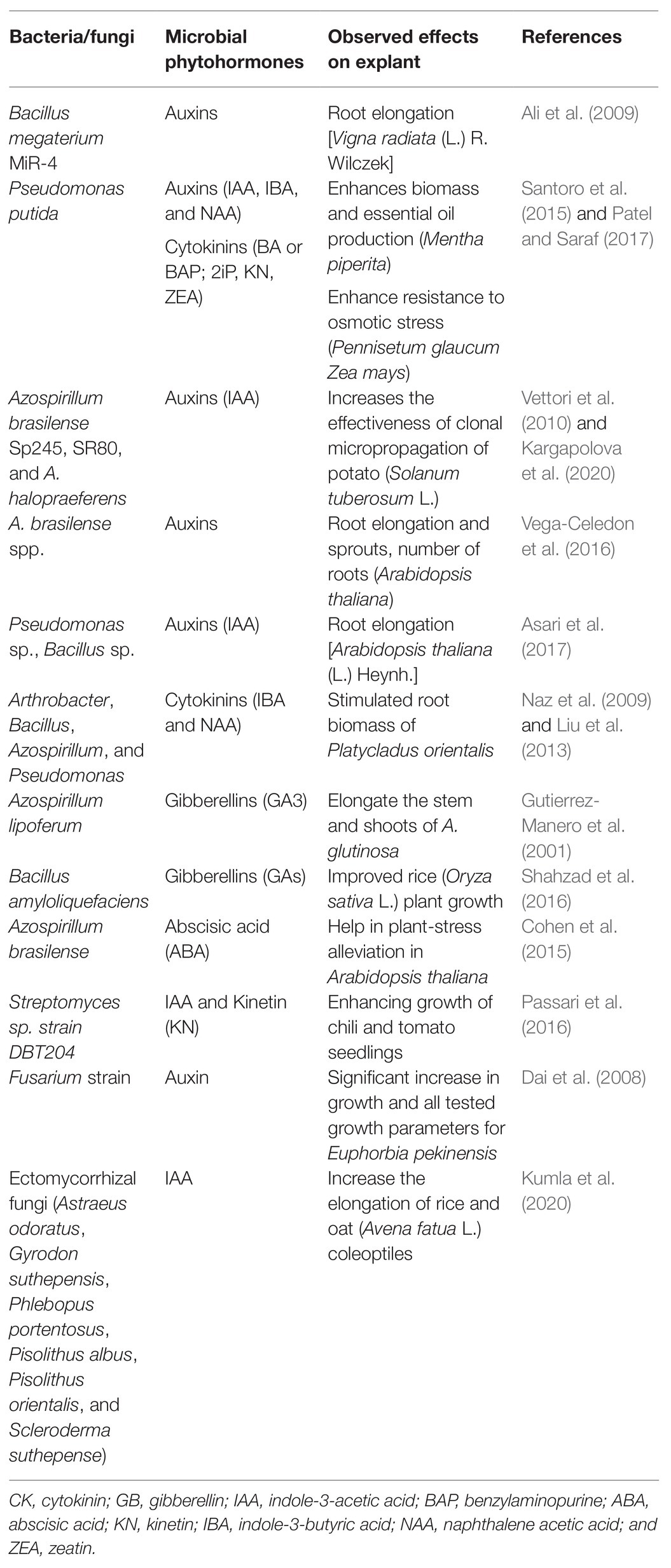
Table 2. Growth regulators produced by microorganisms, and their effect on plant development and morphology.
Although, the mycorrhization technique is important for the growth and development of the micropropagated plantlets, some problems need to be solved to optimize the technology efficiency. The main problem to be solved is how to produce pure fungal inoculum without contaminants for micropropagation. Currently, the disinfection and germination of spores in the agar medium are difficult. On the other hand, Murashige and Skoog (1962) medium (MS) systematically used in micropropagation does not seem to be favorable for the germination and growth of spores (Rana et al., 2019). This suggests that the methodology of propagation needs to be adapted by modifying the nutrient medium to overcome the problem. To overcome the obligatory biotrophy of AMF, the production of axenic inoculum from Ri T-DNA transformed carrot roots, under elevated CO2, raised great hope (Srinivasan et al., 2014). This method allowed significant production of extensive hyphal growth on modified Strullu Romand (MSR) medium and 8,500–9,000 spores per petri dish (Srinivasan et al., 2014). The possible utilization of sonication, gradient flotation, and enzymatic methods to separate intraradical spores and vesicles from roots and thereby to obtain a high-quality inoculum has been pointed out by Biermann and Linderman (1983). However, these processes seem to be time consuming, costly, and tedious.
Beneficial Effects of Plant Growth Promoting Bacteria in in vitro Plant Culture
The first in vitro bacterization was reported by Digat et al. (1987). These researchers showed a positive impact of Pseudomonas putida and Pseudomonas fluorescens strains on the rooting and acclimatization of Primula microshoots. A few years later, Elmeskaoui et al. (1995) have shown that biotized plant tissue cultures benefit from microbial presence through an improvement in photosynthetic efficiency and biomass production. Generally, PGPB improve growth by releasing PGRs required for vitropropagation (Quambusch and Winkelmann, 2018). Auxins and cytokinins biosynthesis are widespread among rhizobacteria, and different biosynthesis pathways have been identified (Kado, 1984; Amara et al., 2015). For instance, it is assumed that many bacteria can produce cytokinins in pure culture and more than 80% of soil bacteria in the rhizosphere can produce auxins especially indole-3-acetic acid (IAA) which is the major auxin active form in plants (Patten and Glick, 1996; Souza et al., 2015). PGRs from microorganisms play a compensatory role, especially when micropropagated plants are under sub-optimal environment with insufficient endogenous production. For different strains belonging to Bacillus, Pseudomonas, Rhizobium, Bradyrhizobium, Enterobacter, Methylobacterium, Microbacterium, Rhodococcus, and Acinetobacter, these PGRs have been quantified, characterized, and tested in plant tissue culture (Spaepen and Vanderleyden, 2011). Vereecke et al. (2000) identified 11 different cytokinins in the supernatant of the culture medium of Rhodococcus fascians. Their application in vivo and in vitro on the plant leads to galls, stem fasciation, and brooms. The study from Erturk et al. (2010) has demonstrated that different PGPB strains belonging to genus Bacillus, Paenibacillus, and Comamonas promoted root formation in kiwifruit cuttings in mass clonal propagation through IAA production. More recently, Lim et al. (2016) reported that the diazotroph Herbaspirillum seropedicae induced the proliferation and differentiation of calli and embryogenic calli of oil palm through nitrogen fixation and IAA production. Similar findings were previously reported by Rodríguez-Romero et al. (2008) on micropropagated banana plants with P. fluorescens, with a consistent increase of plant development. Kargapolova et al. (2020) have shown the efficacy of the inoculation with Ochrobactrum cytisi on potato microplants. A 50% increase of mitotic index of root meristem cells and 34% increase of shoot length were reported under ex vitro conditions. On the other hand, some plant growth promoting rhizobacteria (PGPR) can induce the production of phytohormones by the plant. Analyzing plant molecular responses to Burkholderia phytofirmans colonization, Poupin et al. (2013) showed that genes involved in auxin and gibberellin pathways were induced in Arabidopsis thaliana. Moreover, bacterial phytohormones such as gibberellins can interact with other hormones to support elongation (Bottini et al., 2004). Other phytoregulators such as abscisic acid and salicylic acid are produced by PGPB, but they are less studied. The phytohormones regulate both growth and senescence by modulating ethylene levels in the plant tissue (Khan et al., 2008; Iqbal et al., 2017). The later plays an essential role in the plant defense mechanisms against infections and external aggressions. Decreasing ethylene levels allows the plant to be more resistant to different environmental stresses (Glick, 2005). The findings of Nowak (1998) revealed that Origanum vulgare (L) plantlets inoculated with Pseudomonas spp., produced more phenolic compounds and chlorophyll than non-inoculated plantlets. Phenolic compounds are involved in plant pigmentation, growth, reproduction, and resistance to pathogens (Lattanzio et al., 2006). The growth of pathogens is suppressed by producing toxins, antibiotics, HCN, and/or hydrolytic enzymes such as proteases, chitinases, and lipases. These compounds degrade the cell wall, virulent, or pathogenic factors (Compant et al., 2005). It has been shown that inoculation with PGPB that produced aminocyclopropane carboxylate (ACC) deaminase enhanced stress tolerance and plant growth through a reduction of ethylene production (Ruzzi and Aroca, 2015; Gupta and Pandey, 2019). Souza et al. (2013) reported that ethylene acts as stress phytohormone which adversely affects the growth of the roots under abiotic and biotic stress. Similar results were previously reported on Camelina sativa by Heydarian et al. (2016). These authors have shown that PGPB can enhance growth and salt tolerance in camelina by the production of ACC deaminase. In vitro co-culture of explants with PGPB induces developmental and metabolic changes, which enhance their tolerance to abiotic and biotic stresses. In this regard, Sgroy et al. (2009) have demonstrated that Bacillus, Lysinibacillus, Pseudomonas, Achromobacter, and Brevibacterium associated with the halophyte Prosopis strombulifera act as stress homeostasis-regulating bacteria through IAA, zeatin, and GA production. These phytohormones increase roots length of P. strombulifera, allowing them to explore the soil in depth and absorb more water. In hydroponic media, De Garcia Salamone et al. (2005) compared Phaseolus vulgaris plants treated with auxin and cytokinin to those inoculated with Rhizobium. They noticed that the results in terms of growth were similar and the PGRs were detected in the medium of inoculated plants but not in the medium of non-inoculated roots. Bacteria can also produce volatile metabolites which can induce organogenesis (Gopinath et al., 2015), improve the efficiency of photosynthesis (Xie et al., 2009), and provide protection against abiotic stressors (Orlikowska et al., 2017). PGP bacteria and fungi living in the rhizosphere induce systemic resistance (ISR) and enhance defense against a broad range of pathogens and insects. Some PGPB (e.g., Pseudomonas and Bacillus) as well as some PGPF (e.g., Trichoderma) can sensitize the plant immune system for enhanced defense without directly activating costly defenses (Pieterse et al., 2014; Al-Ani and Mohammed, 2020).
Application in Agriculture, Horticulture, and Forestry
The development of biotization is a promising avenue which is gaining increasing amounts of attention from researchers in agriculture, horticulture, and forestry.
Plant tissue culture is considered as one of the important breeding methodologies and an efficient way of clonal propagation allowing to increase production of important crops (El-Sherif, 2018) such as groundnut or peanut (Arachis hypogaea L.), sorghum (Sorghum bicolor), carrot (Daucus carota L.), potato (Solanum tuberosum), maize (Zea mays), wheat (Triticum aestivum L.), and rice (Oryza sativa). Rice is one of the most important crops which currently feeds more than 50% of the world’s population (Ricepedia, 2020). Therefore, various protocols have been developed for rice plant tissue culture, but high mortality of micropropagated plants during or following the transfer from laboratory to land is still a limiting factor. In order to increase growth and reduce mortality rate in plantlets at the acclimatization stage, introduction of beneficial microorganisms (bacteria and/or fungi) was suggested (Srivastava et al., 2002). Indeed, some studies reported that endophytic fungi such as Phialemonium dimorphosphorum, Gaeumannomyces graminis, and Gaeumannomyces amomi significantly increase rice plant height, root length, and root system development (Kandar et al., 2018). Similary, Diédhiou et al. (2016) reported that the inoculation of rice plants with AMF can significantly increase plant biomass and grain yield of certain varieties under field conditions. Furthermore, Bernaola and Stout (2020) reported that AMF colonization influences the resistance of rice plants to herbivore feeding or pathogen infection. Biotization of micropropagated rice plants results in enhanced growth and higher survival rate during laboratory to land transfer (Chandra et al., 2010). Senthilkumar et al. (2008) reported that the biotized rice plants performed better, for root and shoot length, biomass, and grain yield over the uninoculated control. Other successfully biotization experiments were reported with Azotobacter chroococcum, which increased the shoot weight and number of roots in wheat (Andressen et al., 2009), Azospirillum barasilense which enhanced acclimatization of micropropagated fruit rootstocks (Vettori et al., 2010), and Pseudomonas aureofaciens which leaded to a better growth of potato and strawberry at acclimatization, when microshoots were inoculated before rooting (Zakharchenko et al., 2011). A pseudomonas strain has been also reported to promote root growth of watermelon (Nowak, 1998). Recently, our results showed that Streptomyces griseorubens and Norcardiopsis alba increased maize rooting, root hairs, and growth under phosphorus deficiency (Soumare et al., 2020a). Figure 4 summarizes some benefits of biotization compared to classical micropropagation.
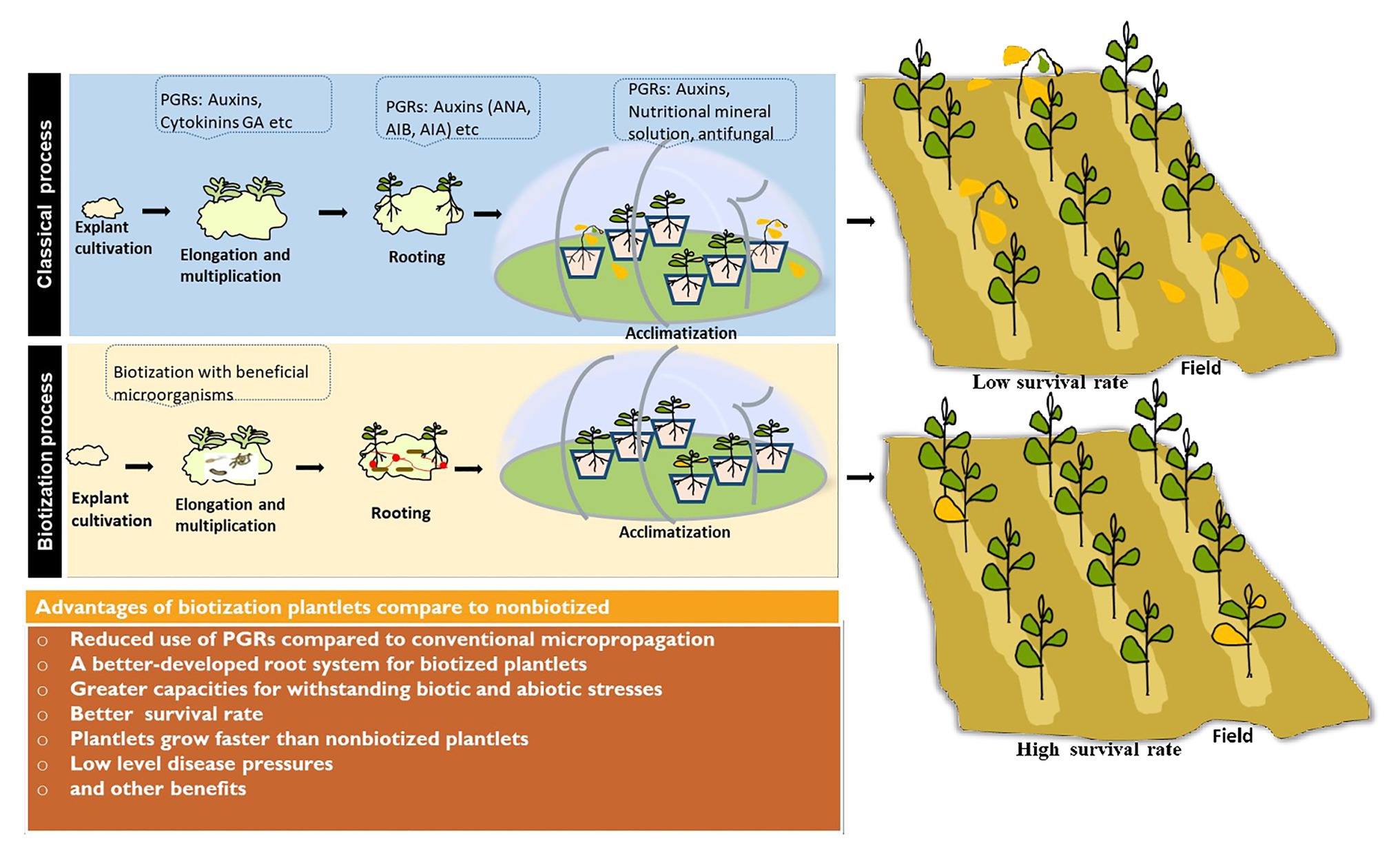
Figure 4. Schematic summary of some benefits of biotization process compared to classical micropropagation process.
Among ornamental plants, orchids dominate among the commercially micropropagated, and attract more attention. Orchidaceae is one of the largest family of flowering plants and horticulturally important species due to their ornamental and commercial values. In addition, orchids are used as traditional medicines (Rahamtulla et al., 2020). In vitro culture is a useful method to propagate endemic or endangered orchid species for conservation purposes because seeds of most of these plants are difficult to germinate. In nature, the germination of orchid seeds is induced by specific mycorrhizal fungi (symbiotic germination) which promote the embryo growth and supply it with required nutrients (Liu et al., 2010; Herrera et al., 2020). An effective system for the in vitro propagation of orchids must integrate the associated symbiotic fungi through biotization approach. This will allow good micropropagation and acclimatization with no apparent “transient transfer shock.” In addition, the technique of biotization could be a realistic way to produce low-cost micropropagated plantlets (Bezerra et al., 2020). Some bacteria have also exhibited good potential for application in orchid cultivation. In this sense, Bezerra et al. (2020) reported positive impact of the application of rooting-derived microorganisms, especially bacterial isolate in the in vitro culture and plantlet acclimatization of Oncidium varicosum. In vitro bacterization of photinia (Photinia × fraseri Dress), another ornamental plant has yielded interesting results. Using the PGPB Azospirillum brasilense and Azotobacter chroococcum during rhizogenesis, Larraburu et al. (2007) have reported that they induced earlier rooting of photinia shoots, and significant increase of root fresh and dry weight and root surface area. These results are in agreement with previous findings from Digat et al. (1987) which showed a positive impact of P. putida and P. fluorescens strains on the rooting and acclimatization of microshoots of Primula, a genus including many important commercial ornamental species.
It is widely admitted that deforestation and forest degradation take place at a faster rate than they are being regenerated naturally or replanted artificially. The potential benefits of in vitro plant regeneration in afforestation and reforestation programs have long been recognized (Di-Gaudio et al., 2020). Biotization in micropropagation technology may add advantage in terms of cost production of tissue-cultured plantlets. In nature, all species of forest trees depend upon a symbiotic association of their roots with ecto and/or endomycorrhizal fungi (Brundrett, 2009). Moreover, it has been shown that trees often fail to establish at new sites if their mycorrhizal fungal symbionts are absent (Lakhanpal, 2000). Therefore, to maximize the benefits of micropropagation, it is necessary to allow the vitroplants to form effective mycorrhiza, especially for species with high mycorrhizal dependency. In vitro mycorrhization of micropropagated plants before acclimatization increases survival and resistance to water stress and ensures a better mineral nutrition of the plant by enhancing the functionality of the root system (Siddiqui and Pichtel, 2008). Numerous findings validate the use of in vitro mycorrhization techniques for several plants such as Castanea sativa (Martins, 2008), Helianthemum spp. (Morte et al., 1994), Cistus spp. (Quatrini et al., 2003), Quercus suber L. (Dı́ez et al., 2000), and Eucalyptus (Freire et al., 2018; Di-Gaudio et al., 2020). Among these species, Eucalyptus genus is the most used species in forest plantation and in reafforestation programs (Soumare et al., 2017). Eucalyptus species are widely used for rapid production of solid wood and cellulose pulp (Soumare et al., 2015b, 2017). Unfortunately, micropropagated plants are adversely affected by water stress and require a long period of transition to become adapted to ex vitro conditions. Reddy and Satyanarayana (1998) showed that establishment of mycorrhiza in micropropagated plantlets of Eucalyptus tereticornis enables them to survive in ex vitro conditions more readily and improves their growth and acclimatization. Similarly, Nowak (1998) showed that in vitro mycorrhization of micropropagated plants has increased plant survival and shoot biomass during ex vitro weaning as well as to shorten the acclimatization phase. With very little modification, biotization approach can be widely applicable to other useful forest tree species used in reforestation programs, especially those with high mycorrhizal dependency (Diédhiou et al., 2004, 2005; Bâ et al., 2010). Niemi and Scagel (2007) have developed an in vitro micropropagation method to induce adventitious root formation in hypocotyl cuttings of Scots pine (Pinus sylvestris) by inoculating them with two ectomycorrhizal fungi, Pisolithus tinctorius and Paxillus involutus. In addition, the formation of their coat mantle on the external surface of the root, create a barrier against the soil borne phytopathogens.
Future Prospects for Plant Growth Promoting Microbes in Plant Tissue Culture
The role of PGPMs in tissues cultures has not been studied sufficiently enough. Our knowledge in PGPM behavior at the root, leaf, or whole plant level and their function in the natural environment is still limited (Sunita et al., 2020). In the future, much research is needed to select efficient, multifunctional, stress tolerant PGRs-producing microbes and having ecological plasticity for their use in plant tissue culture. Indeed, the wide diversity of possible uses of beneficial microbes in plant tissue culture open new doors to identify appropriate candidates from PGPMs to be used at the different stages of micropropagation, with particular attention to mixed-strain consortiums rather than mono-strain inoculums to take advantage of functional complementarity (Soumare et al., 2020b). On the other hand, a great deal of effort should be devoted toward bioformulation of these microbes for suitable application in plant tissue culture. Currently, there is some constraints for the delivery of PGPMs, especially during explant cultivation, elongation and multiplication, and rooting. To implement their application of PGPMs in plant tissue culture, researchers should develop strategies to improve microbial inoculants and inoculation technologies. In this respect, the application of bionanotechnologies could provide new avenues for the development of carrier based microbial inoculants. The use of nanoformulations may enhance the stability of biofertilizers (Malusá et al., 2012; Arora et al., 2020) with respect to heat, desiccation, and UV inactivation. Currently, very few studies are interested in bionanotechnology inputs in in vitro tissue culture. Research would be based on what is done in the pharmaceutical industry in order to develop tailor bioformulations using PGPMs especially for the biotization purpose in plant tissue culture. The other challenge is improving the quality of microbial inoculants for vitroplants as well as developing adequate inoculation protocols. In this sense, the utilization of genetically modified inoculants may offer opportunities in order to achieve a specific purpose in the agricultural and/or food sector. Recent advances in biotechnological tools, such as functional genomics, signaling in rhizosphere, etc., could be useful in engineering of micro-organisms to confer improved benefits to plant especially in plant tissue culture field.
Conclusion
Plant tissue culture technique constitutes an important tool in modern agriculture, horticulture, and forestry. However, the process is expensive due to the requirement of chemicals and the high rate of mortality during the acclimatization phase. PGPMs have the innate potential to produce PGRs and can be considered as potential biofactories. The development and use of inoculants based on PGPMs will help to lessen the cost production of vitroplants by partially or totally replacing some commercial synthetic products with microbial phytohormones and by increasing the survival rate of vitroplants. However, the use of microbes deserves careful monitoring of endophytic communities, especially for plants used as raw food because some pathogenic strains for humans can be stably maintained in cultivated tissues and ex vitro plants. In addition, much remains to be learned from PGPMS in order to identify appropriate candidates and to develop bioformulations for suitable application in plant tissue culture. Along this same line, works on the responses of crops and other useful plants to inoculation with symbiotic and non-symbiotic PGPMs will help to identify which plants are suitable candidates for the microplant biotization.
Author Contributions
All authors listed have made a substantial, direct and intellectual contribution to the review and approved it for publication. AS and AD had the initial idea of the article, performed the literature search, and drafted the work. NA, LA-A, MN, SF, MH, YO, LK, and MS critically revised the paper.
Conflict of Interest
The authors declare that the research was conducted in the absence of any commercial or financial relationships that could be construed as a potential conflict of interest.
Acknowledgments
We thank the Agribiotech Business Unit, OCP, Casablanca for funding.
References
Adesemoye, A. O., Obini, M., and Ugoji, E. O. (2008). Comparison of plant growth-promotion with Pseudomonas aeruginosa and Bacillus subtilis in three vegetables. Braz. J. Microbiol. 39, 423–426. doi: 10.1590/S1517-83822008000300003
Akin-Idowu, P. E., Ibitoye, D. O., and Ademoyegun, O. T. (2009). Tissue culture as a plant production technique for horticultural crops. Afr. J. Biotechnol. 8, 3782–3788. doi: 10.4314/ajb.v8i16.62060
Al-Ani, L. K. T. (2019a). “Secondary metabolites of nonpathogenic Fusarium spp.; scope in agriculture” in Secondary metabolites of plant growth promoting rhizomicroorganisms: Discovery and applications. eds. H. B. Singh, C. Keswani, M. S. Reddy, E. S. Royano, and C. García-Estrada (Singapore: Springer), 59–76.
Al-Ani, L. K. T. (2019b). “Bioactive secondary metabolites of Trichoderma spp. for efficient management of phytopathogens” in Secondary metabolites of plant growth promoting rhizomicroorganisms: Discovery and applications. eds. H. B. Singh, C. Keswani, M. S. Reddy, E. S. Royano, and C. García-Estrada (Singapore: Springer), 125–143.
Al-Ani, L. K. T., and Mohammed, A. M. (2020). “Versatility of Trichoderma in plant disease management” in Molecular aspects of plant beneficial microbes in agriculture. eds. V. Sharma, R. Salwan, and L. K. T. Al-Ani (Cambridge: Elsevier Science), 159–168.
Ali, B., Sabri, A. N., Ljung, K., and Hasnain, S. (2009). Quantification of indole-3-acetic acid from plant associated Bacillus spp. and their phytostimulatory effect on Vigna radiata L. World J. Microbiol. Biotechnol. 25:519. doi: 10.1007/s11274-008-9918-9
Amara, U., Khalid, R., and Hayat, R. (2015). “Soil bacteria and phytohormones for sustainable crop production” in Bacterial metabolites in sustainable agroecosystem. Sustainable development and biodiversity. Vol. 12. ed. D. K. Maheshwari (Cham: Springer), 87–103.
Andressen, D., Manoochehri, I., Carletti, S., Llorente, B., Tacoronte, M., and Vielma, M. (2009). Optimization of the in vitro proliferation of jojoba (Simmondsia chinensis (link) Schn.) by using rotable central composite design and inoculation with rhizobacteria. Bioagro 21, 41–48.
Antoun, H., and Prévost, D. (2005). “Ecology of plant growth promoting rhizobacteria” in PGPR: Biocontrol and biofertilization. ed. Z. A. Siddiqui (Dordrecht: Springer), 1–38.
Arora, N. K., Fatima, T., Mishra, J., Verma, S., Verma, R., Verma, M., et al. (2020). Halo-tolerant plant growth promoting rhizobacteria for improving productivity and remediation of saline soils. J. Adv. Res. 26, 69–82. doi: 10.1016/j.jare.2020.07.003
Asari, S., Tarkowská, D., Rolčík, J., Novák, O., Palmero, D. V., Bejai, S., et al. (2017). Analysis of plant growth-promoting properties of Bacillus amyloliquefaciens UCMB5113 using Arabidopsis thaliana as host plant. Planta 245, 15–30. doi: 10.1007/s00425-016-2580-9
Azcón-Aguilar, C., and Barea, J. (2015). Nutrient cycling in the mycorrhizosphere. J. Soil Sci. Plant Nutr. 15, 372–396. doi: 10.4067/s0718-95162015005000035
Bâ, A. M., Diédhiou, A. G., Prin, Y., Galiana, A., and Duponnois, R. (2010). Management of ectomycorrhizal symbionts associated to useful exotic tree species to improve reforestation programme performances in tropical Africa. Ann. For. Sci. 67, 1–9. doi: 10.1051/forest/2009108
Begum, N., Qin, C., Ahanger, M. A., Raza, S., Khan, M. I., Ashraf, M., et al. (2019). Role of arbuscular mycorrhizal fungi in plant growth regulation: implications in abiotic stress tolerance. Front. Plant Sci. 10:1068. doi: 10.3389/fpls.2019.01068
Bernaola, L., and Stout, M. J. (2020). Effects of arbuscular mycorrhizal fungi on rice-herbivore interactions are soil-dependent. Sci. Rep. 9:14037. doi: 10.1038/s41598-019-50354
Bezerra, G. A., Gabriel, A. V. M. D., Mariano, E. D., and Cardoso, J. C. (2020). In vitro culture and greenhouse acclimatization of Oncidium varicosum (Orchidaceae) with microorganisms isolated from its roots. Ornam. Hortic. 25, 407–416. doi: 10.1590/2447-536x.v25i4.2046
Bidabadi, S. S., and Jain, S. M. (2020). Cellular, molecular, and physiological aspects of in vitro plant regeneration. Plan. Theory 9:702. doi: 10.3390/plants9060702
Biermann, B., and Linderman, R. G. (1983). Increased geranium growthusing pre-transplant inoculation with a mycorrhizal fungus. J. Am. Soc. Hortic. Sci. 108, 972–976.
Blázquez, M. A., Nelson, D. C., and Dolf, W. (2020). Evolution of plant hormone response pathways. Annu. Rev. Plant Biol. 71, 327–353. doi: 10.1146/annurev-arplant-050718-100309
Bottini, R., Cassan, F., and Piccoli, P. (2004). Gibberellin production by bacteria and its involvement in plant growth promotion and yield increase. Appl. Microbiol. Biotechnol. 65, 497–503. doi: 10.1007/s00253-004-1696-1
Brundrett, M. C. (2009). Mycorrhizal associations and other means of nutrition of vascular plants: understanding the global diversity of host plants by resolving conflicting information and developing reliable means of diagnosis. Plant Soil 320, 37–77. doi: 10.1007/s11104-008-9877-9
Chanclud, E., Kisiala, A., Emery, N. R. J., Chalvon, V., Ducasse, A., Romiti Michel, C., et al. (2016). Cytokinin production by the rice blast fungus is a pivotal requirement for full virulence. PLoS Pathog. 12:e1005457. doi: 10.1371/journal.ppat.1005457
Chandra, S., Bandopadhyay, R., Kumar, V., and Chandra, R. (2010). Acclimatization of tissue cultured plantlets: from laboratory to land. Biotechnol. Lett. 32, 1199–1205. doi: 10.1007/s10529-010-0290-0
Chen, M., Arato, M., Borghi, L., Nouri, E., and Reinhardt, D. (2018). Beneficial services of arbuscular mycorrhizal fungi: from ecology to application. Front. Plant Sci. 9:1270. doi: 10.3389/fpls.2018.01270
Cohen, A. C., Bottini, R., Pontin, M., Berli, F. J., Moreno, D., Boccanlandro, H., et al. (2015). Azospirillum brasilense ameliorates the response of Arabidopsis thaliana to drought mainly via enhancement of ABA levels. Physiol. Plant. 153, 79–90. doi: 10.1111/ppl.12221
Compant, S., Dufy, B., Nowak, J., Clement, C., and Barka, E. A. (2005). Use of plant growth-promoting bacteria for biocontrol of plant diseases: principles, mechanisms of action and future prospects. Appl. Environ. Microbiol. 71, 4951–4959. doi: 10.1128/AEM.71.9.4951-4959.2005
Dai, C. C., Yu, B. Y., and Li, X. (2008). Screening of endophytic fungi that promote the growth of Euphorbia pekinensis. Afr. J. Biotechnol. 7, 3505–3510.
De Garcia Salamone, I. E., Hynes, R. K., and Nelson, L. M. (2005). “Role of cytokinins in plant growth promotion by rhizosphere bacteria” in PGPR: Biocontrol and biofertilization. ed. Z. A. Siddiqui (Dordrecht: Springer), 173–195.
Dias, J. P. T. (2019). Plant growth regulators in horticulture: practices and perspectives. Biotecnologia Vegetal 19, 3–14.
Diédhiou, A. G., Bâ, A. M., Sylla, S. N., Dreyfus, B., Neyra, M., and Ndoye, I. (2004). The early-stage ectomycorrhizal Thelephoroid fungal sp. is competitive and effective on Afzelia africana Sm. In nursery conditions in Senegal. Mycorrhiza 14, 313–322. doi: 10.1007/s00572-003-0276-z
Diédhiou, A. G., Guèye, O., Diabaté, M., Prin, Y., Duponnois, R., Dreyfus, B., et al. (2005). Contrasting responses to ectomycorrhizal inoculation in seedlings of six tropical African tree species. Mycorrhiza 16, 11–17. doi: 10.1007/s00572-005-0007-8
Diédhiou, A. G., Mbaye, F. K., Mbodj, D., Faye, M. N., Pignoly, S., Ndoye, I., et al. (2016). A field trials reveal ecotype-specific responses to mycorrhizal inoculation in rice. PLoS One 11:e0167014. doi: 10.1371/journal.pone.0167014
Dı́ez, J., Manjón, J. L., Kovács, G. M., Celestino, C., and Toribio, M. (2000). Mycorrhization of vitroplants raised from somatic embryos of cork oak (Quercus suber L.). Appl. Soil Ecol. 15, 119–123. doi: 10.1016/S0929-1393(00)00087-1
Digat, B., Brochard, P., Hermelin, V., and Tozet, M. (1987). Interest of bacterized synthetic substrates MILCAP® for in vitro culture. Acta Hortic. 212, 375–378.
Di-Gaudio, A. V., Tubert, E., Laino, L. E., Chaín, J. M., Pitta-Alvarez, S. I., Amodeo, G., et al. (2020). New and rapid micropropagation protocol for Eucalyptus grandis hill ex maiden. For. Syst. 29:eSC04. doi: 10.5424/fs/2020291-15965
Egamberdieva, D., Wirth, S., Bellingrath-Kimura, S. D., Mishra, J., and Arora, N. K. (2019). Salt-tolerant plant growth promoting rhizobacteria for enhancing crop productivity of saline soils. Front. Microbiol. 10:2791. doi: 10.3389/fmicb.2019.02791
Elmeskaoui, A., Damont, J., Poulin, M., Piche, Y., and Desjardins, Y. (1995). A tripartite culture system for endomycorrhizal inoculation of micropropagated strawberry plantlets in vitro. Mycorrhiza 5, 313–319. doi: 10.1007/BF00207403
El-Sherif, N. A. (2018). “Impact of plant tissue culture on agricultural sustainability” in Sustainability of agricultural environment in Egypt: Part II. The handbook of environmental chemistry. Vol. 77. eds. A. Negm and M. Abu-hashim (Cham: Springer), 93–107.
Erturk, Y., Ercisli, S., Haznedar, A., and Cakmakci, R. (2010). Effects of plant growth promoting rhizobacteria (PGPR) on rooting and root growth of kiwifruit (Actinidia deliciosa) stem cuttings. Biol. Res. 43, 91–98. doi: 10.4067/S0716-97602010000100011
Freire, C. G., Giachini, A. J., Gardin, J. P. P., Rodrigues, A. C., Vieira, R. L., Baratto, C. M., et al. (2018). First record of in vitro formation of ectomycorrhizae in Psidium cattleianum Sabine, a native Myrtaceae of the Brazilian Atlantic Forest. PLoS One 13:e0196984. doi: 10.1371/journal.pone.0196984
Glick, B. R. (2005). Modulation of plant ethylene levels by the bacterial enzyme ACC deaminase. FEMS Microbiol. Lett. 251, 1–7. doi: 10.1016/j.femsle.2005.07.030
Gopinath, S., Kumaran, K. S., and Sundararaman, M. (2015). A new initiative in micropropagation: airborne bacterial volatiles modulate organogenesis and antioxidant activity in tobacco (Nicotiana tabacum L.) callus. In Vitro Cell Dev. Biol. Plant 51, 514–523. doi: 10.1007/s11627-015-9717-6
Goswami, D., Thakker, J. N., and Dhandhukia, P. C. (2016). Portraying mechanics of plant growth promoting rhizobacteria (PGPR): a review. Cogent Food Agric. 2, 1–19. doi: 10.1080/23311932.2015.1127500
Gupta, S., and Pandey, S. (2019). ACC deaminase producing bacteria with multifarious plant growth promoting traits alleviates salinity stress in french bean (Phaseolus vulgaris) plants. Front. Microbiol. 10:1506. doi: 10.3389/fmicb.2019.01506
Gutierrez-Manero, F. J., Ramos-Solano, B., Probanza, A., Mehouachi, J., Tadeo, F. R., and Talon, M. (2001). The plant-growth-promoting rhizobacteria Bacillus pumilus and Bacillus licheniformis produce high amounts of physiologically active gibberellins. Physiol. Plant. 111, 206–211. doi: 10.1034/j.1399-3054.2001.1110211.x
Harish, S., Kavino, M., Kumar, N., saravanakumar, D., Soorianathasundaram, K., and Samiyappan, R. (2008). Biohardenig with plant growth promoting rhizosphere and endophytic bacteria induces systematic resistance against banana bunchy top virus. Appl. Soil Ecol. 39, 187–200. doi: 10.1016/j.apsoil.2007.12.006
Herrera, H., Sanhueza, T., Martiarena, R., Valadares, R., Fuentes, A., and Arriagada, C. (2020). Mycorrhizal fungi isolated from native terrestrial orchids from region of La Araucanía, southern Chile. Microorganisms 8:1120. doi: 10.3390/microorganisms8081120
Heydarian, Z., Yu, M., Gruber, M., Glick, B. R., Zhou, R., and Hegedus, D. D. (2016). Inoculation of soil with plant growth promoting bacteria producing 1-aminocyclopropane-1-carboxylate deaminase or expression of the corresponding acds gene in transgenic plants increases salinity tolerance in Camelina sativa. Front. Microbiol. 7:1966. doi: 10.3389/fmicb.2016.01966
Hütsch, B. W., Jürgen, A., and Merbach, W. (2002). Plant rhizodeposition: an important source for carbon turnover in soils. J. Plant Nutr. Soil Sci. 165, 397–407. doi: 10.1002/1522-2624(200208)165:4<397::AID-JPLN397>3.0.CO;2-C
Iqbal, N., Khan, N. A., Ferrante, A., Trivellini, A., Francini, A., and Khan, M. (2017). Ethylene role in plant growth, development and senescence: interaction with other phytohormones. Front. Plant Sci. 8:475. doi: 10.3389/fpls.2017.00475
Jha, C. K., Patel, D., Rajendran, N., and Saraf, M. (2010). Combinatorial assessment on dominance and informative diversity of PGPR from rhizosphere of Jatropha curcas L. J. Basic Microbiol. 50, 211–217. doi: 10.1002/jobm.200900272
Kado, C. I. (1984). “Phytohormone-mediated tumorigenesis by plant pathogenic bacteria” in Genes involved in microbe-plant interactions. eds. E. S. Dennis and B. Hohn (Vienna: Springer), 311–336.
Kanani, P., Modi, A., and Kumar, A. (2020). “Biotization of endophytes in micropropagation: a helpful enemy” in Microbial endophytes: Prospects for sustainable agriculture, food science, technology and nutrition. eds. A. Kumar and V. K. Singh (Woodhead Publishing), 357–379.
Kandar, M., Suhandono, S., and Aryantha, I. N. P. (2018). Growth promotion of rice plant by endophytic fungi. J. Pure Appl. Microbiol. 12, 1569–1577. doi: 10.22207/JPAM.12.3.62
Karandashov, V., and Bucher, M. (2005). Symbiotic phosphate transport in arbuscular mycorrhizas. Trends Plant Sci. 10, 22–29. doi: 10.1016/j.tplants.2004.12.003
Kargapolova, K. Y., Burygin, G. L., Tkachenko, O. V., Evseeva, N. V., Pukhalskiy, Y. V., and Belimov, A. A. (2020). Effectiveness of inoculation of in vitro-grown potato microplants with rhizosphere bacteria of the genus Azospirillum. Plant Cell Tissue Organ Cult. 141, 351–359. doi: 10.1007/s11240-020-01791-9
Kemler, M., Witfeld, F., Begerow, D., and Yurkov, A. (2017). “Phylloplane yeasts in temperate climates” in Yeasts in natural ecosystems: Diversity. eds. P. Buzzini, M. A. Lachance, and A. Yurkov (Cham: Springer).
Khan, N. A., Mir, M. R., Nazar, R., and Singh, S. (2008). The application of ethephon (an ethylene releaser) increases growth, photosynthesis and nitrogen accumulation in mustard (Brassica juncea L.) under high nitrogen levels. Plant Biol. 10, 534–538. doi: 10.1111/j.1438-8677.2008.00054.x
Ku, Y. S., Sintaha, M., Cheung, M. Y., and Lam, H. M. (2018). Plant hormone signaling crosstalks between biotic and abiotic stress responses. Int. J. Mol. Sci. 19:3206. doi: 10.3390/ijms19103206
Kumla, J., Suwannarach, N., Matsu, i. K., and Lumyong, S. (2020). Biosynthetic pathway of indole3-acetic acid in ectomycorrhizal fungi collected from northern Thailand. PLoS One 15:e0227478. doi: 10.1371/journal.pone.0227478
Lakhanpal, T. N. (2000). “Ectomycorrhiza-an overview” in Mycorrhizal biology. eds. K. G. Mukerji, B. P. Chamola, and J. Singh (New York: Kluwer Academic/Plenum), 336.
Larraburu, E. E., Carletti, S. M., Rodríguez Cáceres, E. A., and Llorente, B. E. (2007). Micropropagation of photinia employing rhizobacteria to promote root development. Plant Cell Rep. 26, 711–717. doi: 10.1007/s00299-006-0279-2
Lattanzio, V., Lattanzio, V. M. T., and Cardinali, A. (2006). “Role of phenolics in the resistance mechanisms of plants against fungal pathogens and insects” in Phytochemistry: Advances in research. ed. F. Imperato (Trivandrum, Kerala, India: Research Signpost).
Liang, C., Wu, R., Han, Y., Wan, T., and Cai, Y. (2019). Optimizing suitable antibiotics for bacterium control in micropropagation of cherry rootstock using a modified leaf disk diffusion method and E test. Plan. Theory 8:66. doi: 10.3390/plants8030066
Lim, S. L., Subramaniam, S., Zamzuri, I., and Amir, H. G. (2016). Biotization of in vitro calli and embryogenic calli of oil palm (Elaeis guineensis Jacq.) with diazotrophic bacteria Herbaspirillum seropedicae (Z78). Plant Cell Tissue Organ Cult. 127, 251–262. doi: 10.1007/s11240-016-1048-8
Liu, H., Luo, Y., and Liu, H. (2010). Studies of mycorrhizal fungi of Chinese orchids and their role in orchid conservation in China: a review. Bot. Rev. 76, 241–262. doi: 10.1007/s12229-010-9045-9
Liu, F., Xing, S., Ma, H., Du, Z., and Ma, B. (2013). Cytokinin-producing, plant growth-promoting rhizobacteria that confer resistance to drought stress in Platycladus orientalis container seedlings. Appl. Microbiol. Biotechnol. 97, 9155–9164. doi: 10.1007/s00253-013-5193-2
Lopes, E. A. P., da Silva, A. D. A., Mergulhão, A. C. d. E. S., da Silva, E. V. N., Santiago, A. D., and Figueiredo, M. d. V. B. (2019). Co-inoculation of growth promoting bacteria and Glomus clarum in micropropagated cassava plants. Rev. Caatinga 32, 152–166. doi: 10.1590/1983-21252019v32n116rc
Malusá, E., Sas-Paszt, L., and Ciesielska, J. (2012). Technologies for beneficial microorganisms inocula used as biofertilizers. Sci. World J. 2012, 1–12. doi: 10.1100/2012/491206
Martins, A. (2008). “In vitro mycorrhization of micropropagated plants: studies on Castanea sativa mill” in Mycorrhizae: Sustainable agriculture and forestry. eds. Z. A. Siddiqui, M. S. Akhtar, and K. Futai (Dordrecht: Springer), 321–336.
Martin, F., Kohler, A., Murat, C., Veneault-Fourrey, C., and Hibbett, D. S. (2016). Unearthing the roots of ectomycorrhizal symbioses. Nat. Rev. Microbiol. 14, 760–773. doi: 10.1038/nrmicro.2016.149
Meixner, C., Ludwig-Müller, J., Miersch, O., Gresshoff, P., Staehelin, C., and Vierheilig, H. (2005). Lack of mycorrhizal autoregulation and phytohormonal changes in the supernodulating soybean mutant nts1007. Planta 222, 709–715. doi: 10.1007/s00425-005-0003-4
Méndez-Hernández, H. A., Ledezma-Rodríguez, M., Avilez-Montalvo, R. N., Juárez-Gómez, Y. L., Skeete, A., Avilez-Montalvo, J., et al. (2019). Signaling overview of plant somatic embryogenesis. Front. Plant Sci. 10:77. doi: 10.3389/fpls.2019.00077
Morte, M. A., Cano, A., Honrubia, M., and Torres, P. (1994). In vitro mycorrhization of micropropagated Helianthemum almeriense plantlets with Terfezia claveryi (desert truffle). Agric. Food Sci. 3, 309–314. doi: 10.23986/afsci.72700
Müller, D., and Leyser, O. (2011). Auxin, cytokinin and the control of shoot branching. Ann. Bot. 107, 1203–1212. doi: 10.1093/aob/mcr069
Murashige, T., and Skoog, F. (1962). A revised medium for rapid growth and bioassays with tobacco tissue cultures. Physiol. Plant. 15:473497.
Naseem, M., and Dandekar, T. (2012). The role of auxin-cytokinin antagonism in plant pathogen interactions. PLoS Pathog. 8:e1003026. doi: 10.1371/journal.ppat.1003026
Naz, I., Bano, A., and Ul-Hassan, T. (2009). Isolation of phytohormones producing plant growth promoting rhizobacteria from weeds growing in Khewra salt range, Pakistan and their implication in providing salt tolerance to Glycine max L. Afr. J. Biotechnol. 8, 5762–5766. doi: 10.5897/AJB09.1176
Niemi, K., and Scagel, C. (2007). “Root induction of Pinus sylvestris L. hypocotyl cuttings using specific ectomycorrhizal fungi in vitro” in Protocols for micropropagation of woody trees and truits. eds. S. M. Jain and H. Häggman (Dordrecht: Springer).
Nowak, J. (1998). Benefits of in vitro “biotization” of plant tissue cultures with microbial inoculants. In Vitro Cell Dev. Biol. Plant 34, 122–130. doi: 10.1007/BF02822776
Orlikowska, T., Nowak, K., and Reed, B. (2017). Bacteria in the plant tissue culture environment. Plant Cell Tissue Organ Cult. 128, 487–508. doi: 10.1007/s11240-016-1144-9
Osugi, A., and Sakakibara, H. (2015). Q&A: how do plants respond to cytokinins and what is their importance? BMC Biol. 13:102. doi: 10.1186/s12915-015-0214-5
Passari, A. K., Chandra, P., Zothanpuia, Mishra, V. K., Leo, V. V., Gupta, V. K., et al. (2016). Detection of biosynthetic gene and phytohormone production by endophytic actinobacteria associated with Solanum lycopersicum and their plant-growth-promoting effect. Res. Microbiol. 167, 692–705. doi: 10.1016/j.resmic.2016.07.001
Patel, T., and Saraf, M. (2017). Biosynthesis of phytohormones from novel rhizobacterial isolates and their in vitro plant growth-promoting efficacy. J. Plant Interact. 12, 480–487. doi: 10.1080/17429145.2017.1392625
Patten, C. L., and Glick, B. R. (1996). Bacterial biosynthesis of indole-3-acetic acid. Can. J. Microbiol. 42, 207–220. doi: 10.1139/m96-032
Perotto, S., Daghino, S., and Martino, E. (2018). Ericoid mycorrhizal fungi and their genomes: another side to the mycorrhizal symbiosis? New Phytol. 220, 1141–1147. doi: 10.1111/nph.15218
Pierre-Jerome, E., Drapek, C., and Benfey, P. N. (2018). Regulation of division and differentiation of plant stem cells. Annu. Rev. Cell Dev. Biol. 34, 289–310. doi: 10.1146/annurev-cellbio-100617-062459
Pieterse, C. M. J., Zamioudis, C., Berendsen, R. L., Weller, D. M., Van Wees, S. C. M., and Bakker, P. A. (2014). Induced systemic resistance by beneficial microbes. Annu. Rev. Phytopathol. 52, 347–375. doi: 10.1146/annurev-phyto-082712-102340
Poupin, M. J., Timmermann, T., Vega, A., Zuñiga, A., and González, B. (2013). Effects of the plant growth-promoting bacterium Burkholderia phytofirmans PsJN throughout the life cycle of Arabidopsis thaliana. PLoS One 8:e69435. doi: 10.1371/journal.pone.0069435
Pour, M. M., Saberi-Riseh, R., Mohammadinejad, R., and Hossein, A. (2019). Nano-encapsulation of plant growth-promoting rhizobacteria and their metabolites using alginate-silica nanoparticles and carbon nanotube improves UCB1 pistachio micropropagation. J. Microbiol. Biotechnol. 29, 1096–1103. doi: 10.4014/jmb.1903.03022
Promputtha, I., Lumyong, S., Dhanasekaran, V., McKenzie, E. H. C., Hyde, K. D., and Jeewon, R. A. (2007). Phylogenetic evaluation of whether endophytes become saprotrophs at host senescence. Microb. Ecol. 53, 579–590. doi: 10.1007/s00248-006-9117-x
Püschel, D., Bitterlich, M., Rydlová, J., and Jansa, J. (2020). Facilitation of plant water uptake by an arbuscular mycorrhizal fungus: a Gordian knot of roots and hyphae. Mycorrhiza 30, 299–313. doi: 10.1007/s00572-020-00949-9
Quambusch, M., and Winkelmann, T. (2018). Bacterial endophytes in plant tissue culture: mode of action, detection, and control. Methods Mol. Biol. 1815, 69–88. doi: 10.1007/978-1-4939-8594-4_4
Quatrini, P., Gentile, M., Carimi, F., Pasquale, F. D., and Puglia, A. M. (2003). Effect of native arbuscular mycorrhizal fungi and Glomus mosseae on acclimatization and development of micropropagated Citrus limon (L.) Burm. J. Hortic. Sci. Biotechnol. 78, 39–45. doi: 10.1080/14620316.2003.11511584
Rahamtulla, M., Pradhan, U. C., Roy, A. K., Rampilla, V., and Khasim, S. M. (2020). “Ethnomedicinal aspects of some orchids from Darjeeling Himalaya, India” in Orchid biology: Recent trends and challenges. eds. S. Khasim, S. Hegde, M. González-Arnao, and K. Thammasiri (Singapore: Springer).
Rai, M. K. (2001). Current advances in mycorrhization in microporopagation. In Vitro Cell Dev. Biol. Plant 37, 158–167. doi: 10.1007/s11627-001-0028-8
Rana, K. L., Kour, D., Sheikh, I., Yadav, N., Yadav, A. N., Kumar, V., et al. (2019). “Biodiversity of endophytic fungi from diverse niches and their biotechnological applications” in Advances in endophytic fungal research. Fungal biology. ed. B. Singh (Cham: Springer), 105–144.
Reddy, M. S., and Satyanarayana, T. (1998). Inoculation of micropropagated plantlets of Eucalyptus tereticornis with ectomycorrhizal fungi. New For. 16, 273–279. doi: 10.1023/A:1006589310990
Ricepedia (2020). Rice productivity. Available at: http://ricepedia.org/rice-as-a-crop/rice-productivity (Accessed June 20, 2020).
Rodríguez-Romero, A. S., Badosa, E., Montesinos, E., and Jaizme-Vega, M. C. (2008). Growth promotion and biological control of root-knot nematodes in micropropagated banana during the nursery stage by treatment with specific bacterial strains. Ann. Appl. Biol. 152, 41–48. doi: 10.1111/j.1744-7348.2007.00189.x
Ruzzi, M., and Aroca, R. (2015). Plant growth-promoting rhizobacteria act as biostimulants in horticulture. Sci. Hortic. 196, 124–134. doi: 10.1016/j.scienta.2015.08.042
Sahay, N. S., and Varma, A. (2000). A biological approach towards increasing the rates of survival of micropropagated plants. Curr. Sci. 78, 126–129.
Santoro, M. V., Cappellari, L. R., Giordano, W., and Banchio, E. (2015). Plant growth-promoting effects of native Pseudomonas strains on Mentha piperita (peppermint): an in vitro study. Plant Biol. 17, 1218–1226. doi: 10.1111/plb.12351
Senthilkumar, M., Madhaiyan, M., Sundaram, S. P., Sangeetha, H., and Kannaiyan, S. (2008). Induction of endophytic colonization in rice (Oryza sativa L.) tissue culture plants by Azorhizobium caulinodans. Biotechnol. Lett. 30, 1477–1487. doi: 10.1007/s10529-008-9693-6
Sgroy, V., Cassán, F., Masciarelli, O., Del Papa, M. F., Lagares, A., and Luna, V. (2009). Isolation and characterization of endophytic plant growth-promoting (PGPB) or stress homeostasis-regulating (PSHB) bacteria associated to the halophyte Prosopis strombulifera. Appl. Microbiol. Biotechnol. 85, 371–381. doi: 10.1007/s00253-009-2116-3
Shahzad, R., Waqas, M., Khan, A. L., Asaf, S., Khan, M. A., Kang, S. M., et al. (2016). Seed-borne endophytic Bacillus amyloliquefaciens RWL-1 produces gibberellins and regulates endogenous phytohormones of Oryza sativa. Plant Physiol. Biochem. 106, 236–243. doi: 10.1016/j.plaphy.2016.05.006
Shigenaga, A. M., and Argueso, C. T. (2016). No hormone to rule them all: interactions of plant hormones during the responses of plants to pathogens. Semin. Cell Dev. Biol. 56, 174–189. doi: 10.1016/j.semcdb.2016.06.005
Siddiqui, Z. A., and Pichtel, J. (2008). “Mycorrhizae: an overview” in Mycorrhizae: Sustainable agriculture and forestry. eds. Z. A. Siddiqui, M. S. Akhtar, and K. Futai (Dordrecht: Springer), 1–35.
Sivasakthivelan, P., and Saranraj, P. (2013). Azospirillum and its formulations: a rewiew. Int. J. Microbiol. Res. 4, 275–287. doi: 10.5829/idosi.ijmr.2013.4.3.825
Smith, S. E., and Read, D. J. (2008). Mycorrhizal symbiosis. 3rd Edn. New York, London, Burlington, San Diego: Academic Press.
Soumare, A., Boubekri, K., Lyamlouli, K., Hafidi, M., Ouhdouch, Y., and Kouisni, L. (2020a). Efficacy of phosphate solubilizing Actinobacteria to improve rock phosphate agronomic effectiveness and plant growth promotion. Rhizosphere 17:100284. doi: 10.1016/j.rhisph.2020.100284
Soumare, A., Diedhiou, A. G., and Kane, A. (2017). Eucalyptus plantations in the Sahel: distribution, socio-economic importance and ecological concern. Int. J. Biol. Chem. Sci. 11, 3005–3017. doi: 10.4314/ijbcs.v11i6.36
Soumare, A., Diédhiou, A. G., Thuita, M., Hafidi, M., Ouhdouch, Y., Gopalakrishnan, S., et al. (2020b). Exploiting biological nitrogen fixation: a route towards a sustainable agriculture. Plants 9:1011. doi: 10.3390/plants9081011
Soumare, A., Diop, T., Manga, A., and Ndoye, I. (2015a). Role of arbuscular mycorrhizal fungi and nitrogen fixing bacteria on legume growth under various environmental stresses. Int. J. Biosci. 7, 31–46. doi: 10.12692/ijb/7.4.31-46
Soumare, A., Manga, A., Fall, S., Ndoye, I., Hafidi, M., and Duponnois, R. (2015b). Effect of Eucalyptus camaldulensis amendment on soil chemical properties, enzymatic activity, and Acacia species growth and roots symbioses. Agrofor. Syst. 89, 97–106. doi: 10.1007/s10457-014-9744-z
Souza, R. d., Ambrosini, A., and Passaglia, L. M. (2015). Plant growth-promoting bacteria as inoculants in agricultural soils. Genet. Mol. Biol. 38, 401–419. doi: 10.1590/S1415-475738420150053
Souza, R., Beneduzi, A., Ambrosini, A., Costa, P. B., Meyer, J., Vargas, L. K., et al. (2013). The effect of plant growth-promoting rhizobacteria on the growth of rice (Oryza sativa L.) cropped in southern Brazilian fields. Plant Soil 366, 585–603. doi: 10.1007/s11104-012-1430-1
Spaepen, S., and Vanderleyden, J. (2011). Auxin and plant-microbe interactions. Cold Spring Harb. Perspect. Biol. 3:a001438. doi: 10.1101/cshperspect.a001438
Srinivasan, M., Kumar, K., Kumutha, K., and Marimuthu, P. (2014). Establishing monoxenic culture of arbuscular mycorrhizal fungus Glomus intraradices through root organ culture. J. Appl. Nat. Sci. 6, 290–293. doi: 10.31018/jans.v6i1.417
Srivastava, P. S., Bharti, N., Pande, D., and Srivastava, S. (2002). “Role of mycorrhiza in in vitro micropropagation of plants” in Techniques in mycorrhizal studies. eds. K. G. Mukerji, C. Manoharachary, and B. P. Chamola (Dordrecht: Springer), 443–468.
Streletskii, R. A., Kachalkin, A. V., Glushakova, A. M., Yurkov, A. M., and Demin, V. V. (2019). Yeasts producing zeatin. PeerJ 7:e6474. doi: 10.7717/peerj.6474
Subramaniam, G., Srinivas, V., and Prasanna, S. L. (2020a). “Streptomyces” in Beneficial microbes in agro-ecology: Bacteria and fungi. eds. N. Amaresan, M. Senthil Kumar, K. Annapurna, K. Kumar, and A. Sankaranarayanan (Academic Press), 55–71.
Subramaniam, G., Thakur, V., Saxena, R. K., Vadlamudi, S., Purohit, S., Kumar, V., et al. (2020b). Complete genome sequence of sixteen plant growth promoting Streptomyces strains. Sci. Rep. 10:10294. doi: 10.1038/s41598-020-67153-9
Suman, S. (2017). Plant tissue culture: a promising tool of quality material production with special reference to micropropagation of banana. Biochem. Cell. Arch. 17, 1–26.
Sunita, K., Mishra, I., Mishra, J., Prakash, J., and Arora, N. K. (2020). Secondary metabolites from halotolerant plant growth promoting rhizobacteria for ameliorating salinity stress in plants. Front. Microbiol. 11:567768. doi: 10.3389/fmicb.2020.567768
Trigiano, R. N., and Gray, D. J. (2016). Plant tissue culture, development, and biotechnology. United States: CRC Press, 608.
Tyagi, B., Tewari, S., and Dubey, A. (2018). Biochemical characterization of fungus isolated during in vitro propagation of Bambusa balcooa. Pharmacogn. Mag. 13(Suppl. 4), S775–S779. doi: 10.4103/pm.pm_20_17
Ullah, A., Manghwar, H., Shaban, M., Khan, A. H., Akbar, A., Ali, U., et al. (2018). Phytohormones enhanced drought tolerance in plants: a coping strategy. Environ. Sci. Pollut. Res. Int. 25, 33103–33118. doi: 10.1007/s11356-018-3364-5
Varma, A., and Schuepp, H. (1994). Infectivity and effectiveness of Glomus intraradices in micropropagated plants. Mycorrhiza 5, 29–37. doi: 10.1007/BF00204017
Vega-Celedon, P., Canchignia, M. H., Gonzalez, M., and Seeger, M. (2016). Biosynthesis of indole-3-acetic acid and plant growth promoting by bacteria. Des. Cult. 37, 33–39.
Vereecke, D., Burssens, S., Simón-Mateo, C., Inzé, D., Van Montagu, M., Goethal, K., et al. (2000). The Rhodococcus fascians-plant interaction: morphological traits and biotechnological applications. Planta 210, 241–251. doi: 10.1007/PL00008131
Verma, M., Mishra, J., and Arora, N. K. (2019). “Plant growth-promoting rhizobacteria: diversity and applications” in Environmental biotechnology: For sustainable future. eds. R. C. Sobti, N. K. Arora, and R. Kothari (New Delhi: Springer), 129–173.
Vettori, L., Russo, A., Felici, C., Fiaschi, G., Morini, S., and Toffanin, A. (2010). Improving micropropagation: effect of Azospirillum brasilense Sp245 on acclimatization of rootstocks of fruit tree. J. Plant Interact. 5, 249–259. doi: 10.1080/17429145.2010.511280
Weyens, N., van der Lelie, D., Taghavi, S., Newman, L., and Vangronsveld, J. (2009). Exploiting plant—microbe partnerships to improve biomass production and remediation. Trends Biotechnol. 27, 591–598. doi: 10.1016/j.tibtech.2009.07.006
Xie, X., Zhang, H., and Pare, P. (2009). Sustained growth promotion in Arabidopsis with long-term exposure to the beneficial soil bacterium Bacillus subtilis (GB03). Plant Signal. Behav. 4, 948–953. doi: 10.4161/psb.4.10.9709
Yuan, Z. L., Zhang, C. L., and Lin, F. C. (2010). Role of diverse non-systemic fungal endophytes in plant performance and response to stress: progress and approaches. J. Plant Growth Regul. 29, 116–126. doi: 10.1007/s00344-009-9112-9
Zabalgogeazcoa, I. (2008). Fungal endophytes and their interaction with plant pathogens. Span. J. Agric. Res. 6, 138–146. doi: 10.5424/sjar/200806S1-382
Keywords: plant tissue culture, phytohormones, plant growth promoting microbe, agriculture, biotechnology, plant growth promoting bacteria, plant growth promoting fungi
Citation: Soumare A, Diédhiou AG, Arora NK, Al-Ani LKT, Ngom M, Fall S, Hafidi M, Ouhdouch Y, Kouisni L and Sy MO (2021) Potential Role and Utilization of Plant Growth Promoting Microbes in Plant Tissue Culture. Front. Microbiol. 12:649878. doi: 10.3389/fmicb.2021.649878
Edited by:
Jacob George Malone, John Innes Centre, United KingdomReviewed by:
Jay Shankar Singh, Babasaheb Bhimrao Ambedkar University, IndiaAjar Nath Yadav, Eternal University, India
Copyright © 2021 Soumare, Diédhiou, Arora, Al-Ani, Ngom, Fall, Hafidi, Ouhdouch, Kouisni and Sy. This is an open-access article distributed under the terms of the Creative Commons Attribution License (CC BY). The use, distribution or reproduction in other forums is permitted, provided the original author(s) and the copyright owner(s) are credited and that the original publication in this journal is cited, in accordance with accepted academic practice. No use, distribution or reproduction is permitted which does not comply with these terms.
*Correspondence: Abdoulaye Soumare, YWJkb3VsYXllLnNvdW1hcmVAdW02cC5tYQ==; Abdala G. Diédhiou, YWJkYWxhLmRpZWRoaW91QHVjYWQuZWR1LnNu