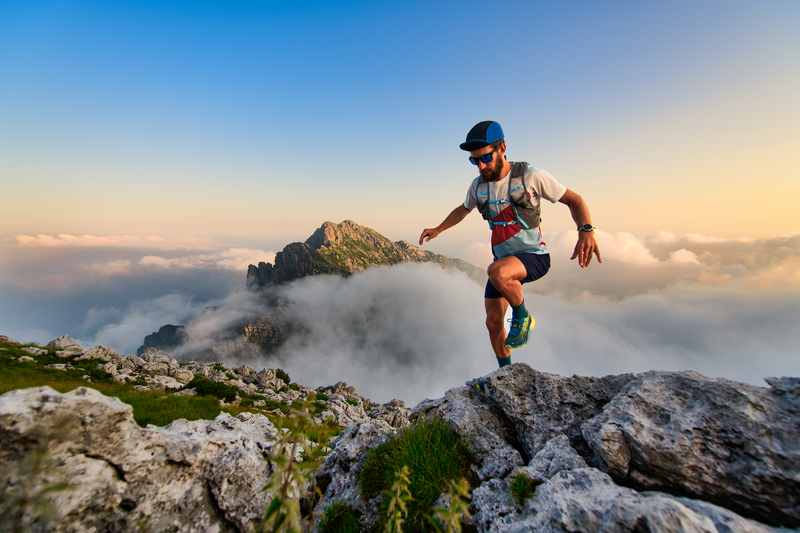
95% of researchers rate our articles as excellent or good
Learn more about the work of our research integrity team to safeguard the quality of each article we publish.
Find out more
MINI REVIEW article
Front. Microbiol. , 10 March 2021
Sec. Microbiotechnology
Volume 12 - 2021 | https://doi.org/10.3389/fmicb.2021.636486
This article is part of the Research Topic Bioconversion and Biorefinery of C1 Compounds View all 16 articles
Conventional chemical methods to transform methane and carbon dioxide into useful chemicals are plagued by the requirement for extreme operating conditions and expensive catalysts. Exploitation of microorganisms as biocatalysts is an attractive alternative to sequester these C1 compounds and convert them into value-added chemicals through their inherent metabolic pathways. Microbial biocatalysts are advantageous over chemical processes as they require mild-operating conditions and do not release any toxic by-products. Methanotrophs are potential cell-factories for synthesizing a wide range of high-value products via utilizing methane as the sole source of carbon and energy, and hence, serve as excellent candidate for methane sequestration. Besides, methanotrophs are capable of capturing carbon dioxide and enzymatically hydrogenating it into methanol, and hence qualify to be suitable candidates for carbon dioxide sequestration. However, large-scale production of value-added products from methanotrophs still presents an overwhelming challenge, due to gas-liquid mass transfer limitations, low solubility of gases in liquid medium and low titer of products. This requires design and engineering of efficient reactors for scale-up of the process. The present review offers an overview of the metabolic architecture of methanotrophs and the range of product portfolio they can offer. Special emphasis is given on methanol biosynthesis as a potential biofuel molecule, through utilization of methane and alternate pathway of carbon dioxide sequestration. In view of the gas-liquid mass transfer and low solubility of gases, the key rate-limiting step in gas fermentation, emphasis is given toward reactor design consideration essential to achieve better process performance.
Emission of greenhouse gases has been increasing globally at an alarming rate. Global anthropogenic emissions of CO2 and CH4 have almost hit 43.1 billion tons and 9390 mmtCO2e, respectively (Global Carbon Project Budget-20191; Global Methane Initiative Report-2018)2. The overall global atmospheric concentrations of CO2 and CH4 have increased by 12.07 and 5.9%, respectively, in the last two decades (NOAA-ESRL, 2020)3. Moreover, the global warming potential of CH4 is 28–36 times higher than CO2 (US-EPA)4. There have been efforts to sequester CO2 or CH4 and catalytically convert them into various value-added products, through hydrogenation and oxidation, respectively. However, chemical processes for the conversion of these C1 compounds are plagued by requirement for expensive catalysts, high temperature (∼450°C), high pressure (∼30 MPa) and release of toxic by-products like carbon monoxide; making the overall technology expensive and non-sustainable.
The use of microorganisms as biocatalysts for the sequestration of CH4 and CO2 is an attractive alternative as they require milder operating conditions and do not release any toxic by-products. Naturally occurring methane-oxidizing microorganisms are known as methanotrophs. Owing to their ability to utilize methane as the source of carbon and energy, methanotrophs serve as excellent candidates for methane sequestration. Some methanotrophs possess an added advantage of sequestering CO2 as substrate for enzymatic hydrogenation into methanol, and thereby qualify as suitable candidates for CO2 sequestration. Besides their ability to harness noxious C1 compounds, methanotrophs can also serve as potential cell-factories for a wide-range of high-value products, e.g., methanol, ectoine/hydroxyectoine, poly-β-hydroxybutyrate (PHB), single cell protein, extracellular polysaccharide, lipids etc. (Xin et al., 2007; Rostkowski et al., 2013; Cantera et al., 2018; Rasouli et al., 2018; Tsapekos et al., 2020). Despite their enormous potential, large-scale production of these high-value products is constrained by various limitations in solubility of gases in liquid medium and gas-liquid mass transfer, eventually resulting in -insignificant product titer. The present review provides an overview of methanotrophs, metabolic pathways to utilize CH4/CO2 and the high-value products synthesized by them. This review also sheds light on the biological production of methanol, a key product targeted as solvent and biofuel, through oxidation of CH4 and alternatively via reduction of CO2. In view of overcoming the rate-limiting factors in gas fermentation, toward efficient capture and conversion of harmful C1 compounds into high-value products at industrial-scale, special emphasis has been given on reactor design and configuration.
Methanotrophs are Gram-negative proteobacteria, noted for their ability to utilize methane as the sole source of carbon and energy. Methanotrophic bacterium was discovered by Söhngen in 1906 (Hanson and Hanson, 1996). Whittenbury et al. (1970) carried out comprehensive isolation and characterization of methanotrophs and introduced the Type I, Type II, and Type X classification system. Methanotrophs utilize methane via a metabolic cascade comprising of four enzymes, namely, methane monooxygenase (MMO), methanol dehydrogenase (MDH), formaldehyde dehydrogenase (FADH), and formate dehydrogenase (FDH) (Xin et al., 2004a, 2007). Based on the type of MMO produced, the methanotrophs are divided into three categories namely, (a) Type I (produce particulate MMO), (b) Type II (produce both particulate as well as soluble MMO) and (c) Type X (comprises of specific features of both Type I and Type II methanotrophs).
Aerobic methanotrophs were later on classified broadly into two major groups of proteobacteria based on 16S rRNA gene sequencing, namely, gamma-proteobacteria (Group I) and alpha-proteobacteria (Group II), in place of the earlier 3-type classification (Ge et al., 2014). Gamma-proteobacteria (Group I) comprise of previously classified Type I and Type X methanotrophs and Alpha-proteobacteria (Group II) comprise of formerly known Type II methanotrophs (Fei et al., 2014). The sub-division alpha-proteobacteria consists of four genera namely, Methylocella, Methylocapsa, Methylocystis, and Methylosinus. The sub-division gamma-proteobacteria consists of 12 genera namely, Methylothermus, Methylosoma, Methylosphaera, Methylosarcina, Methylomonas, Methylohalobius, Methylomicrobium, Methylococcus, Methylocaldum, Methylobacter, Clonothrix, and Crenothrix (Ge et al., 2014). Gamma-proteobacteria (Type I and Type X) and alpha-proteobacteria (Type II) use ribulose monophosphate (RuMP) cycle and serine cycle, respectively, for utilization of C1-carbon sources, such as formaldehyde/formate. Although, Type X species (Methylocaldum and Methylococcus), also utilize formaldehyde through RuMP cycle, yet they differ from Type I species as they express small quantities of enzymes of the serine cycle, ribulose-bisphosphate carboxylase, present in the Calvin-Benson-Bassham (CBB) cycle (Hanson and Hanson, 1996; Park and Kim, 2019). Type X species reportedly possess genes encoding enzymes for CO2-fixation through CBB cycle (Baxter et al., 2002). Novel methanotrophs have also been found in the phylum Verrumicrobia, which has been reported to fix CO2 by utilizing CBB cycle (Rasigraf et al., 2014).
Gamma-proteobacterial and alpha-proteobacterial methanotrophs can also be distinguished based on few other characteristics. In terms of arrangement of their intracytoplasmic membranes (ICMs), gamma-proteobacterial species contain bundles of ICMs, whereas, ICMs in alpha-proteobacterial species are aligned around the cell’s periphery (Kalyuzhnaya et al., 2019). As per cellular phospholipid fatty acid (PLFA) composition, Type I, Type II, and Type X methanotrophs, respectively, possess 14− 16−, 18−, and 16-carbon long PLFA (Ge et al., 2014). On the basis of storage carbon, most species of gamma-proteobacteria are known for glycogen accumulation and a few species for PHB accumulation; while, alpha-proteobacterial species are predicted to mainly accumulate PHB, since they excrete acetone, succinate, acetate, etc. (which are probable derivatives of PHB) from methane fermentation (Kalyuzhnaya, 2016).
Typical growth rates for gamma-proteobacterial and alpha-proteobacterial methanotrophs ranges within 0.009–0.4 and 0.005–0.16 h–1, respectively (Kalyuzhnaya, 2016). Methanotrophs are resistant to desiccation. They have been reported for bioremediation, bioleaching, and epoxidation. Conjugation (Nguyen et al., 2020) and electroporation-based (Yan et al., 2016) gene-transfer techniques have been developed for methanotrophs. However, the yield and productivity from methanotrophic cell factories are relatively low as compared to other hosts such as E. coli (Lee et al., 2016). Moreover, their obligate methanotrophic characteristics and slow growth rates limits the application of genetic engineering techniques. On the contrary, their obligate methanotrophic nature makes them a cheap industrial platform which can sequester waste greenhouse gas (methane), relative to other platforms which consume expensive substrates like glucose, xylose etc.
Few studies have explored genome-scale metabolic models (GSMM) for methanotrophs for extensively modeling the entire array of genes, metabolites and biochemical reactions in silico. Gupta et al. (2019) built a GSMM for Methylococcus capsulatus (Bath) comprising of 865 metabolites, 899 reactions and 535 genes. The model could predict the pathways and amino acids necessary for growth, and identified essential metabolic genes and lethal genes in the bacterium. Lieven et al. (2018) using GSMM predicted that methane oxidation by pMMO can be stimulated either through uphill electron transfer or direct-coupling, at decreased efficiency. GSMM helps the authors to build a centralized knowledge-base for the model organism, understand its metabolic physiology and simulate its metabolic behavior under diverse conditions.
All aerobic methanotrophs oxidize CH4 to CO2 through a common enzymatic cascade. The oxidation process produces methanol, formaldehyde and formate as reaction intermediates (Figure 1). This is accomplished through sequential catalysis by the enzymes, MMO, MDH, FADH, and FDH. A fraction of the formate/formaldehyde, produced as an intermediate, gets incorporated into the biomass as a source of carbon through the serine cycle or RuMP cycle. RuMP cycle involves assimilation of formaldehyde and its further conversion into hexulose-6-phosphate. Successive conversions produce ribulose-5-phosphate, thus closing the cycle. Formaldehyde gets transformed into formate through H4MPT pathway. Serine cycle involves assimilation of formate. Formate is transferred into serine cycle through H4F pathway. Successive intermediate reactions convert serine into glycine, closing the cycle. Assimilation of CO2 in Type X and Verrumicrobial methanotrophs via CBB cycle begins with the formation of 3-phospho-glycerate, which eventually gets converted into ribulose-1,5-bisphosphate, completing the cycle (Park and Kim, 2019).
Figure 1. Metabolism of CH4 and CO2 in methanotrophs (modified from Xin et al., 2007; Park and Kim, 2019). MMO, methane monooxygenase; MDH, methanol dehydrogenase; FADH, formaldehyde dehydrogenase; FDH, formate dehydrogenase.
Methane is oxidized to methanol by MMO, which requires two units of reducing equivalents (NADH) to break the double bond in oxygen. One of these oxygen atoms oxidizes methane into methanol, whereas the other gets hydrogenated to produce water. The oxidation of methanol to formaldehyde releases PQQH2 and the oxidation of formaldehyde to CO2 via formate releases NADH. NADH thus generated is reutilized by MMO for the oxidation of methane to methanol (Xin et al., 2007).
MMO is the key enzyme responsible toward growth of methanotrophs since these microbes utilize methane as the primary source of carbon and energy. MMO is of two types, soluble MMO (sMMO) and particulate MMO (pMMO). sMMO is synthesized in the cytoplasm and is made up of two monomeric units. Each sMMO monomer consists of two iron atoms (Rosenzweig et al., 1995). pMMO is impregnated on the membrane, and is composed of three monomeric units with each unit comprising of either a di-copper or a mono-copper center (Culpepper and Rosenzweig, 2012). Therefore, concentrations of iron and copper ions primarily affect the MMO activity in culture media. High concentration of iron ions is known to stimulate sMMO expression (Chidambarampadmavathy et al., 2015). Additionally, sMMO is actively expressed at lower ratios of copper to biomass, and its expression is repressed at higher concentrations of copper in the medium (Park et al., 1991). On the contrary, pMMO has increased levels of expression at higher ratios of copper to biomass, and hence corroborates improved activity with increase in copper concentration in the medium (Park et al., 1992). Since pMMO is integrated into the membrane, it is supposed to rapidly oxidize methane compared to sMMO. However, authors have outlined that sMMO causes rapid oxidation of methane as compared to pMMO (Sirajuddin and Rosenzweig, 2015). The concentrations of these metal ions significantly influence MMO activity. Higher cellular MMO activity is associated with higher NADH generation (Zhang et al., 2008), which is a necessary cofactor for formation of various methanotrophic products. Few studies have reported the effect of Cu2+ and Fe2+ ions on the production of PHB and methanol by methanotrophs (Zhang et al., 2008; Patel et al., 2016b).
Some methanotrophs can also utilize CO2 as substrate and reduce it to methanol, in presence of excess CO2 (Xin et al., 2007). This happens in a two-stage process, where methane is utilized as carbon substrate during the first-stage to produce biomass, and CO2 is reduced by the generated biomass in the second-stage to produce methanol. CO2-reduction to methanol is the reverse reaction of methane-oxidation to CO2. It is a sequential catalysis carried out by FDH, FADH and MDH (Figure 1). Since MMO lacks the ability to catalyze this reverse reaction, the end-product of the CO2 reduction pathway is methanol (Xin et al., 2004a). The reduction of CO2 to methanol requires excess of reducing power to drive the reaction against the laws of energy. The reduction of CO2 to formaldehyde via formate requires NADH while the reduction of formaldehyde to methanol requires PQQH2. This exigency of reducing energy equivalent is compensated by the endogenous reductant source (PHB) of the cells, which is explained in “Biological Production of Methanol From Methane and Carbon Dioxide” section.
Wild type methanotrophs possess an inherent potential to synthesize a wide-range of products (Table 1). Molecules like ectoine (Methylomicrobium alcaliphilum, Methylobacter marinus, Methylomicrobium kenyense, Methylobacter modestohalophilus); hydroxyectoine (Methylomicrobium alcaliphilum); sucrose (Methylomicrobium alcaliphilum, Methylomicrobium buryatense, Methylobacter marinus); glutamate (Methylobacter alcaliphilus, Methylomicrobium buryatense, Methylobacter modestohalophilus); 5-oxoproline (Methylobacter alcaliphilus, Methylobacter modestohalophilus) are known as “compatible solutes.” These are secreted by halotolerant and halophilic methanotrophs in response to high salinity in the environment in order to balance the turgor pressure and minimize water-loss across the cell membrane (Khmelenina et al., 1999). They have applications in pharmaceutical industries as stabilizers for nucleic acids, DNA-protein complexes and enzymes. Methanotrophs are also able to produce extracellular polysaccharide (EPS) (Methylomicrobium alcaliphilum and methanotrophs enriched from soil/compost) as a defense mechanism against heat, desiccation, predation and other adverse conditions (Cantera et al., 2018). EPS finds application in textile, pharmaceutical and food industries owing to their adhesive and colloid-like properties.
Methanotrophs also synthesize poly-β-hydroxybutyrate (PHB) (Methylosinus trichosporium, Methylocystis parvus, Methylocystis hirsuta), a potential replacement of conventional plastic owing to its biocompatible and biodegradable characteristics (Rodríguez et al., 2020). Single cell proteins, a major methanotrophic-product (Methylococcus capsulatus, Methylomonas sp., Methylocystis sp.), can be used as a prospective substitute for traditional protein sources viz., fishmeal and soymeal (Valverde-Pérez et al., 2020). Lipid molecules derived from methanotrophs (Methylomicrobium buryatense, Methylococcus capsulatus) can be explored as futuristic biofuel (Dong et al., 2017).
Studies have reported engineered (heterologous gene expression) methanotrophic strains to synthesize products beyond their innate metabolic potential. Methylomicrobium buryatense (Henard et al., 2016) and Methylomicrobium alcaliphilum (Henard et al., 2018) have been genetically engineered to produce lactate, which finds application in the production of bioplastics (from lactic acid polymers), cheese and yogurt, dermatological drugs etc. Methylomonas sp. 16a has been engineered to produce astaxanthin (Tao et al., 2007; Rick and Kelly, 2012), a carotenoid pigment with huge commercial significance.
Methanotrophs synthesize a wide-range of products (Table 1) using cheap and wasteful carbon-based substrates (CH4 and/or CO2) unlike microorganisms utilizing cost-intensive carbon sources like glucose, fructose, xylose etc. These features render methanotroph-based gas fermentation processes economical and sustainable.
Methanol is a clean-burning fuel with high specific-energy ratio, flame-speed and octane number; and low combustion temperature and volatility. As has been already discussed, methanotrophs utilize methane, which is subsequently oxidized to methanol, formaldehyde, formate, and finally CO2 (Figure 1). To achieve direct production of methanol from methane, the subsequent biochemical pathway leading to methanol oxidation needs to be blocked. This is often accomplished by adding MDH inhibitors in the medium. Several studies have reported various irreversible MDH inhibitors for directly producing methanol from methane, viz., cyclopropanol, phosphate, EDTA, MgCl2, NaCl, and NH4Cl (Takeguchi et al., 1997; Yoo et al., 2015; Patel et al., 2016c,b). However, the activity of MMO is dependent on NADH availability, and inhibition of methanol oxidation by MDH inhibitors results in the depletion of NADH. Hence, to accomplish uninterrupted oxidation of methane to methanol, it is essential to produce NADH by feeding formate to the culture medium (Xin et al., 2004b). Formate is added as a source of reducing power, which assists in the regeneration of NADH- the co-factor for methanol synthesis. However, the use of MDH inhibitors and formate is cost-intensive. Moreover, the supplementation of MDH inhibitors blocks the metabolic pathway in the methanotrophic strain. This affects the viability and biochemical and physiological functions of the culture. Consequently, it reduces the quantity and quality of the biomass produced. These factors lead to low methanol titer and also exclude the possibility of applying biomass-recycling strategy to this process.
Taking these drawbacks into consideration, it is imperative to look for alternative substrates or metabolic pathways which can be exploited to produce methanol using methanotrophs. The limitations associated with biological production of methanol from methane can be overcome by utilizing CO2 as the substrate for some methanotrophic species (Xin et al., 2007; Patel et al., 2016a). This is done in a two-stage process, where methane is utilized as carbon substrate during the first-stage to produce biomass, and CO2 is reduced by the generated biomass in the second-stage to produce methanol (Xin et al., 2007). CO2, an end-product of general methanotrophic metabolism (first-stage), tends to shift the reaction equilibrium in the backward direction if fed in excess to the cells (second-stage). Methanotrophic biomass act as biocatalyst for the reduction of CO2 to methanol. The reduction of CO2 to methanol is the reverse reaction of the oxidation of methane to CO2 (Figure 1). However, MMO lacks the ability to catalyze the reverse reaction (reduction of methanol to methane). Therefore, the end-product of the CO2 reduction pathway is methanol, which gets secreted extracellularly into the medium (Xin et al., 2004a, 2007). The reverse reaction requires excess of reducing equivalents, in the form of NADH, to drive the reaction against the laws of energy. Cells in general produce intracellular NADH. However, supply of endogenous NADH is limited and hence may be fed exogenously to the medium for uninterrupted progression of the batch; but this again adds to the cost of production. However, methanotrophs have an added advantage as they can produce PHB (Ghoddosi et al., 2019). PHB is also a source of intracellular reducing equivalents, in addition to NADH. β-hydroxybutyrate dehydrogenase, a NAD+-linked enzyme, catalyzes the decomposition of PHB to acetoacetic acid, and this subsequently generates reducing equivalents. Hence, the endogenously stored PHB in methanotrophs, plays a crucial role in accomplishing the reduction of CO2 to methanol.
Xin et al. (2007) reported the production of 0.004 μmol methanol/mg (DCW) through reduction of CO2 using Methylosinus trichosporium as a biocatalyst. The study also reports that cells having 38.6% PHB content showed highest methanol titers. Patel et al. (2016a) reported the production of 0.33 mM methanol using Methylosinus sporium from 30% CO2 feed. These are few studies which demonstrate methanol production from CO2. Although, there are reports suggesting CO2 assimilation in Type X and Verrumicrobial methanotrophs toward biomass formation, however, there is no evidence in literature regarding these species converting CO2 into methanol.
Authors have mainly demonstrated methanol production in batch and repeated-batch modes. Duan et al. (2011) has reported the production of 1.1 g/l methanol from 50% methane under optimum conditions in batch mode, using M. trichosporium OB3b. Patel et al. (2020c) reported the production of 30.9 mmol/l methanol from 30% methane using free-cells of Methylocystis bryophila under repeated-batch mode. Methanol production was reported to improve upto 52.9 mmol/l through covalent immobilization of cells on coconut coir (Patel et al., 2020c). Different studies have also reported improved methanol production through cell encapsulation in alginate and silica-gel (Patel et al., 2018), immobilization in polyvinyl alcohol (Patel et al., 2020b) and chemically modified chitosan (Patel et al., 2020a). Few key studies on methanol production using methanotrophs are listed in Table 1. Despite of the strategies involved, the processes are limited by low methanol titer and productivity. Yu et al. (2009) reported that methanol is toxic to M. trichosporium at concentrations above 3.0 g/l. This report illustrates that methanotrophs are able to tolerate a very low concentration of methanol and hence, suffer from end-product toxicity, leading to lower methanol titer and productivity.
Common challenges associated with gas fermentation systems are gas-to-liquid mass transfer limitations and lower solubility of the gaseous substrates. Researchers have outlined the role of certain physico-chemical parameters like partial pressure, and extrinsic approaches like reactor configurations and mass transfer vectors to address these limitations.
Bioreactors help in achieving higher gas-to-liquid mass transfer rates as compared to other culture systems, facilitating easier and higher uptake of gases by the cells. Bubble column reactors have been predominantly used for cultivation systems where the objective is to replace mechanically driven stirring and to reduce the shear-stress on the cells. They are suitable for application when the requirement is to maintain directional flow and circulation, efficient mass transfer and heat transfer, especially while working with gas fermentation systems. Their application has mostly been reported for PHB production by methanotrophs (Ghoddosi et al., 2019; Rodríguez et al., 2020). Vertical tubular loop bioreactors have also been reported for gas fermentation. These reactors are characterized by defined direction of fluid circulation, often facilitated by a pump in gas-liquid based reactors and a propeller in fluidized bed reactors (Rahnama et al., 2012). Sheets et al. (2017) reported the production of methanol in a trickle-bed reactor (TBR) stuffed with ceramic beads. TBRs enhance air and methane mass transfer rates from the reactor headspace to the cells suspended in the medium. This study reported two-times greater oxygen mass transfer in the ceramic beads-stuffed reactor compared to the reactor without the bead-stuffing. The rate of methane oxidation was fourfold greater in the TBR as compared to shake-flask cultures. Volumetric mass-transfer coefficient (KLa) is a major metric for the performance evaluation of different reactor configurations. Airlift bioreactors have also been reported for high KLa values of upto 97.2 h–1 (Ghaz-Jahanian et al., 2018). Authors have reported the application of membrane biofilm reactors (MBR) for methanotroph-based bioremediation. MBRs overcome diffusional limitations by promoting high mass-transfer rates of sparingly soluble gaseous substrates to the biomass. MBRs have been reported for denitrification (Lee et al., 2019) and removal of perchlorate (Wu et al., 2019), chromate (Luo et al., 2019), vanadate (Wang et al., 2019), selenate (Shi et al., 2020), etc. from contaminated wastewater and groundwater using methanotrophs.
The problem of low solubility of gases in the liquid-phase in gas fermentation systems has been overcome by incorporating various modifications in the commonly used reactor configurations. Studies have reported the addition of mass transfer vector, an organic phase, which has high affinity for the gaseous substrate and eventually improves the gas hold-up in the liquid media and consequent increased availability of the gaseous substrate to the microbial cells (Rocha-Rios et al., 2010; Zúñiga et al., 2011). Ten percent silicone oil has been prevalently added as a “methane-vector” to the aqueous medium to demonstrate “two-phase partition bioreactors (TPPB).” Rocha-Rios et al. (2010) and Zúñiga et al. (2011) have reported upto 33–45 and 700% increase in methane-degradation, respectively, using TPPBs. Supplementary Table S1 summarizes different reactor configurations used for methanotrophic fermentations.
Partial pressure inside the reactor plays a crucial role in gas fermentation systems. The composition of the gas phase might vary depending upon the source, resulting in varying partial pressures. Timmers et al. (2015) reported that the growth of methanotrophs is faster at increased CH4 partial pressures, as this is associated with highly negative Gibb’s free energy change. While high partial pressures do result in increased solubility of the gases, they might interfere with the growth or product formation metabolism resulting from the interaction of the gaseous substrate with the key enzymes (Hurst and Lewis, 2010). In a study outlined by Soni et al. (1998), with pressure variation between 10 and 50 psi, Methylomonas albus showed a fourfold increase in methane uptake and 40% increase in biomass concentration at 20 psi, whereas higher pressure inhibited growth. Therefore, optimization of partial pressure is essential to maintain the balance between the solubility of gases and growth/product formation within the reactor.
Methanotrophs are excellent candidates for sequestration of harmful C1 compounds (CH4 and CO2). They are cellular factories for synthesis of a wide-range of value-added products. Amongst them, methanol has drawn significant attention owing to its potential applicability as a biofuel. Although, methanotrophs can synthesize methanol directly from methane, the associated limitations have driven the researchers to look for alternate pathways. Production of methanol from the methanotrophic reduction of CO2 appears to be a promising alternative in this regard. The current state-of-the-art technology for the production of methanotrophic high-value products, is constrained by low product titer and productivity. As far as methanol production is concerned, limitations associated with product-toxicity are very challenging. Integration of the process with continuous/intermittent methanol recovery system may assist in overcoming product toxicity and improve its titer and productivity. Regardless of several reactor configurations outlined by researchers to deal with gas fermentation associated challenges, the technology stands at its “nascent stage of development.” Hence, it becomes imperative to conduct detailed in-depth studies aiming at the development of process-engineering and design strategies for sustainable synthesis of methanotrophic high-value products on a commercial-scale. GSMM may be applied to evaluate different methanotrophic species in silico to predict their yields, rates of production and consumption, directed toward improved production of high-value products, without performing time- and labor-consuming experiments.
KS collected the data. KS and GG wrote the manuscript. DD did conceptualization, manuscript reviewing, and editing. All authors contributed to the article and approved the submitted version.
The authors declare that the research was conducted in the absence of any commercial or financial relationships that could be construed as a potential conflict of interest.
The Supplementary Material for this article can be found online at: https://www.frontiersin.org/articles/10.3389/fmicb.2021.636486/full#supplementary-material
Baxter, N. J., Hirt, R. P., Bodrossy, L., Kovacs, K. L., Embley, M. T., Prosser, J. I., et al. (2002). The ribulose-1, 5-bisphosphate carboxylase/oxygenase gene cluster of Methylococcus capsulatus (Bath). Archiv. Microbiol. 177, 279–289. doi: 10.1007/s00203-001-0387-x
Cantera, S., Lebrero, R., Rodríguez, E., García-Encina, P. A., and Muñoz, R. (2017). Continuous abatement of methane coupled with ectoine production by Methylomicrobium alcaliphilum 20Z in stirred tank reactors: a step further towards greenhouse gas biorefineries. J. Clean. Product. 152, 134–141. doi: 10.1016/j.jclepro.2017.03.123
Cantera, S., Sánchez-Andrea, I., Lebrero, R., García-Encina, P. A., Stams, A. J., and Muñoz, R. (2018). Multi-production of high added market value metabolites from diluted methane emissions via methanotrophic extremophiles. Bioresour. Technol. 267, 401–407. doi: 10.1016/j.biortech.2018.07.057
Chidambarampadmavathy, K., Obulisamy, P. K., and Heimann, K. (2015). Role of copper and iron in methane oxidation and bacterial biopolymer accumulation. Eng. Life Sci. 15, 387–399. doi: 10.1002/elsc.201400127
Culpepper, M. A., and Rosenzweig, A. C. (2012). Architecture and active site of particulate methane monooxygenase. Crit. Rev. Biochem. Mol. Biol. 47, 483–492. doi: 10.3109/10409238.2012.697865
Dong, T., Fei, Q., Genelot, M., Smith, H., Laurens, L. M., Watson, M. J., et al. (2017). A novel integrated biorefinery process for diesel fuel blendstock production using lipids from the methanotroph, Methylomicrobium buryatense. Energy Convers. Manag. 140, 62–70. doi: 10.1016/j.enconman.2017.02.075
Duan, C., Luo, M., and Xing, X. (2011). High-rate conversion of methane to methanol by Methylosinus trichosporium OB3b. Bioresour. Technol. 102, 7349–7353. doi: 10.1016/j.biortech.2011.04.096
Fei, Q., Guarnieri, M. T., Tao, L., Laurens, L. M., Dowe, N., and Pienkos, P. T. (2014). Bioconversion of natural gas to liquid fuel: opportunities and challenges. Biotechnol. Adv. 32, 596–614. doi: 10.1016/j.biotechadv.2014.03.011
García-Pérez, T., López, J. C., Passos, F., Lebrero, R., Revah, S., and Muñoz, R. (2018). Simultaneous methane abatement and PHB production by Methylocystis hirsuta in a novel gas-recycling bubble column bioreactor. Chemi. Eng. J. 334, 691–697. doi: 10.1016/j.cej.2017.10.106
Ge, X., Yang, L., Sheets, J. P., Yu, Z., and Li, Y. (2014). Biological conversion of methane to liquid fuels: status and opportunities. Biotechnol. Adv. 32, 1460–1475. doi: 10.1016/j.biotechadv.2014.09.004
Ghaz-Jahanian, M. A., Khoshfetrat, A. B., Rostami, M. H., and Parapari, M. H. (2018). An innovative bioprocess for methane conversion to methanol using an efficient methane transfer chamber coupled with an airlift bioreactor. Chem. Eng. Res. Design 134, 80–89. doi: 10.1016/j.cherd.2018.03.039
Ghoddosi, F., Golzar, H., Yazdian, F., Khosravi-Darani, K., and Vasheghani-Farahani, E. (2019). Effect of carbon sources for PHB production in bubble column bioreactor: emphasis on improvement of methane uptake. J. Environ. Chem. Eng. 7:102978. doi: 10.1016/j.jece.2019.102978
Gupta, A., Ahmad, A., Chothwe, D., Madhu, M. K., Srivastava, S., and Sharma, V. K. (2019). Genome-scale metabolic reconstruction and metabolic versatility of an obligate methanotroph Methylococcus capsulatus str. Bath. PeerJ 7:e6685. doi: 10.7717/peerj.6685
Henard, C. A., Franklin, T. G., Youhenna, B., But, S., Alexander, D., Kalyuzhnaya, M. G., et al. (2018). Biogas biocatalysis: methanotrophic bacterial cultivation, metabolite profiling, and bioconversion to lactic acid. Front. Microbiol. 9:2610. doi: 10.3389/fmicb.2018.02610
Henard, C. A., Smith, H., Dowe, N., Kalyuzhnaya, M. G., Pienkos, P. T., and Guarnieri, M. T. (2016). Bioconversion of methane to lactate by an obligate methanotrophic bacterium. Sci. Rep. 6:21585. doi: 10.1038/srep21585
Hurst, K. M., and Lewis, R. S. (2010). Carbon monoxide partial pressure effects on the metabolic process of syngas fermentation. Biochem. Eng. J. 48, 159–165. doi: 10.1016/j.bej.2009.09.004
Kalyuzhnaya, M. G. (2016). “Methane biocatalysis: selecting the right microbe,” in Biotechnology for Biofuel Production and Optimization, eds C. A. Eckert and C. T. Trinh (Amsterdam: Elsevier), 353–383. doi: 10.1016/B978-0-444-63475-7.00013-3
Kalyuzhnaya, M. G., Gomez, O. A., and Murrell, J. C. (2019). “The methane-oxidizing bacteria (methanotrophs),” in Taxonomy, Genomics and Ecophysiology of Hydrocarbon-Degrading Microbes. Handbook of Hydrocarbon and Lipid Microbiology, ed. T. McGenity (Cham: Springer), 245–278. doi: 10.1007/978-3-030-14796-9_10
Khmelenina, V. N., Kalyuzhnaya, M. G., Sakharovsky, V. G., Suzina, N. E., Trotsenko, Y. A., and Gottschalk, G. (1999). Osmoadaptation in halophilic and alkaliphilic methanotrophs. Archiv. Microbiol. 172, 321–329. doi: 10.1007/s002030050786
Khmelenina, V. N., Shchukin, V. N., Reshetnikov, A. S., Mustakhimov, I. I., Suzina, N. E., Eshinimaev, B. T., et al. (2010). Structural and functional features of methanotrophs from hypersaline and alkaline lakes. Microbiology 79, 472–482. doi: 10.1134/S0026261710040090
Lee, J., Alrashed, W., Engel, K., Yoo, K., Neufeld, J. D., and Lee, H. S. (2019). Methane-based denitrification kinetics and syntrophy in a membrane biofilm reactor at low methane pressure. Sci. Total Environ. 695:133818. doi: 10.1016/j.scitotenv.2019.133818
Lee, O. K., Hur, D. H., Nguyen, D. T. N., and Lee, E. Y. (2016). Metabolic engineering of methanotrophs and its application to production of chemicals and biofuels from methane. Biofuels Bioprod. Biorefin. 10, 848–863.
Lieven, C., Petersen, L. A., Jørgensen, S. B., Gernaey, K. V., Herrgard, M. J., and Sonnenschein, N. (2018). A genome-scale metabolic model for Methylococcus capsulatus (Bath) suggests reduced efficiency electron transfer to the particulate methane monooxygenase. Front. Microbiol. 9:2947. doi: 10.3389/fmicb.2018.02947
Luo, J. H., Wu, M., Liu, J., Qian, G., Yuan, Z., and Guo, J. (2019). Microbial chromate reduction coupled with anaerobic oxidation of methane in a membrane biofilm reactor. Environ. Intern. 130:104926. doi: 10.1016/j.envint.2019.104926
Mardina, P., Li, J., Patel, S. K., Kim, I. W., Lee, J. K., and Selvaraj, C. (2016). Potential of immobilized whole-cell Methylocella tundrae as a biocatalyst for methanol production from methane. J. Microbiol. Biotechnol. 26, 1234–1241. doi: 10.4014/jmb.1602.02074
Mustakhimov, I. I., Reshetnikov, A. S., But, S. Y., Rozova, O. N., Khmelenina, V. N., and Trotsenko, Y. A. (2019). Engineering of hydroxyectoine production based on the Methylomicrobium alcaliphilum. Appl. Biochem. Microbiol. 55, 626–630. doi: 10.1134/S0003683819130015
Nguyen, D. T. N., Lee, O. K., Lim, C., Lee, J., Na, J. G., and Lee, E. Y. (2020). Metabolic engineering of type II methanotroph, Methylosinus trichosporium OB3b, for production of 3-hydroxypropionic acid from methane via a malonyl-CoA reductase-dependent pathway. Metab. Eng. 59, 142–150. doi: 10.1016/j.ymben.2020.02.002
Park, S., Hanna, L., Taylor, R. T., and Droege, M. W. (1991). Batch cultivation of Methylosinus trichosporium OB3b. I: production of soluble methane monooxygenase. Biotechnol. Bioeng. 38, 423–433. doi: 10.1002/bit.260380412
Park, S., Shah, N. N., Taylor, R. T., and Droege, M. W. (1992). Batch cultivation of Methylosinus trichosporium OB3b: II. production of particulate methane monooxygenase. Biotechnol. Bioeng. 40, 151–157. doi: 10.1002/bit.260400121
Park, S. Y., and Kim, C. G. (2019). Application and development of methanotrophs in environmental engineering. J. Mater. Cycles Waste Manag. 21, 415–422. doi: 10.1007/s10163-018-00826-w
Patel, S. K., Gupta, R. K., Kondaveeti, S., Otari, S. V., Kumar, A., Kalia, V. C., et al. (2020a). Conversion of biogas to methanol by methanotrophs immobilized on chemically modified chitosan. Bioresour. Technol. 315:123791. doi: 10.1016/j.biortech.2020.123791
Patel, S. K., Gupta, R. K., Kumar, V., Kondaveeti, S., Kumar, A., Das, D., et al. (2020b). Biomethanol production from methane by immobilized co-cultures of methanotrophs. Indian J. Microbiol. 60, 318–324. doi: 10.1007/s12088-020-00883-6
Patel, S. K., Kalia, V. C., Joo, J. B., Kang, Y. C., and Lee, J. K. (2020c). Biotransformation of methane into methanol by methanotrophs immobilized on coconut coir. Bioresour. Technol. 297:122433. doi: 10.1016/j.biortech.2019.122433
Patel, S. K., Kumar, V., Mardina, P., Li, J., Lestari, R., Kalia, V. C., et al. (2018). Methanol production from simulated biogas mixtures by co-immobilized Methylomonas methanica and Methylocella tundrae. Bioresour. Technol. 263, 25–32. doi: 10.1016/j.biortech.2018.04.096
Patel, S. K., Mardina, P., Kim, D., Kim, S. Y., Kalia, V. C., Kim, I. W., et al. (2016a). Improvement in methanol production by regulating the composition of synthetic gas mixture and raw biogas. Bioresour. Technol. 218, 202–208. doi: 10.1016/j.biortech.2016.06.065
Patel, S. K., Mardina, P., Kim, S. Y., Lee, J. K., and Kim, I. W. (2016b). Biological methanol production by a type II methanotroph Methylocystis bryophila. J. Microbiol. Biotechnol. 26, 717–724. doi: 10.4014/jmb.1601.01013
Patel, S. K., Selvaraj, C., Mardina, P., Jeong, J. H., Kalia, V. C., Kang, Y. C., et al. (2016c). Enhancement of methanol production from synthetic gas mixture by Methylosinus sporium through covalent immobilization. Appl. Energy 171, 383–391. doi: 10.1016/j.apenergy.2016.03.022
Rahnama, F., Vasheghani-Farahani, E., Yazdian, F., and Shojaosadati, S. A. (2012). PHB production by Methylocystis hirsuta from natural gas in a bubble column and a vertical loop bioreactor. Biochem. Eng. J. 65, 51–56. doi: 10.1016/j.bej.2012.03.014
Rasigraf, O., Kool, D. M., Jetten, M. S., Damsté, J. S. S., and Ettwig, K. F. (2014). Autotrophic carbon dioxide fixation via the calvin-benson-bassham cycle by the denitrifying methanotroph “Candidatus Methylomirabilis oxyfera”. Appl. Environ. Microbiol. 80, 2451–2460. doi: 10.1128/AEM.04199-13
Rasouli, Z., Valverde-Pérez, B., D’Este, M., De Francisci, D., and Angelidaki, I. (2018). Nutrient recovery from industrial wastewater as single cell protein by a co-culture of green microalgae and methanotrophs. Biochem. Eng. J. 134, 129–135. doi: 10.1016/j.bej.2018.03.010
Reshetnikov, A. S., Khmelenina, V. N., Mustakhimov, I. I., Kalyuzhnaya, M., Lidstrom, M., and Trotsenko, Y. A. (2011). Diversity and phylogeny of the ectoine biosynthesis genes in aerobic, moderately halophilic methylotrophic bacteria. Extremophiles 15:653. doi: 10.1007/s00792-011-0396-x
Rick, W. Y., and Kelly, K. (2012). “Construction of carotenoid biosynthetic pathways through chromosomal integration in methane-utilizing bacterium Methylomonas sp. strain 16a,” in Microbial Carotenoids from Bacteria and Microalgae, ed. J.-L. Barredo (Totowa, NJ: Humana Press), 185–195. doi: 10.1007/978-1-61779-879-5_10
Rocha-Rios, J., Bordel, S., Hernández, S., and Revah, S. (2009). Methane degradation in two-phase partition bioreactors. Chem. Eng. J. 152, 289–292. doi: 10.1016/j.cej.2009.04.028
Rocha-Rios, J., Muñoz, R., and Revah, S. (2010). Effect of silicone oil fraction and stirring rate on methane degradation in a stirred tank reactor. J. Chem. Technol. Biotechnol. 85, 314–319. doi: 10.1002/jctb.2339
Rocha-Rios, J., Quijano, G., Thalasso, F., Revah, S., and Muñoz, R. (2011). Methane biodegradation in a two-phase partition internal loop airlift reactor with gas recirculation. J. Chem. Technol. Biotechnol. 86, 353–360. doi: 10.1002/jctb.2523
Rodríguez, Y., Firmino, P. I. M., Pérez, V., Lebrero, R., and Muñoz, R. (2020). Biogas valorization via continuous polyhydroxybutyrate production by Methylocystis hirsuta in a bubble column bioreactor. Waste Manag. 113, 395–403. doi: 10.1016/j.wasman.2020.06.009
Rosenzweig, A. C., Nordlund, P., Takahara, P. M., Frederick, C. A., and Lippard, S. J. (1995). Geometry of the soluble methane monooxygenase catalytic diiron center in two oxidation states. Chem. Biol. 2, 409–418.
Rostkowski, K. H., Pfluger, A. R., and Criddle, C. S. (2013). Stoichiometry and kinetics of the PHB-producing Type II methanotrophs Methylosinus trichosporium OB3b and Methylocystis parvus OBBP. Bioresour. Technol. 132, 71–77. doi: 10.1016/j.biortech.2012.12.129
Schouten, S., Bowman, J. P., Rijpstra, W. I. C., and Sinninghe Damsté, J. S. (2000). Sterols in a psychrophilic methanotroph, Methylosphaera hansonii. FEMS Microbiol. Lett. 186, 193–195. doi: 10.1111/j.1574-6968.2000.tb09103.x
Sheets, J. P., Lawson, K., Ge, X., Wang, L., Yu, Z., and Li, Y. (2017). Development and evaluation of a trickle bed bioreactor for enhanced mass transfer and methanol production from biogas. Biochem. Eng. J. 122, 103–114. doi: 10.1016/j.bej.2017.03.006
Shi, L. D., Lv, P. L., Wang, M., Lai, C. Y., and Zhao, H. P. (2020). A mixed consortium of Methanotrophic archaea and bacteria boosts methane-dependent selenate reduction. Sci. Total Environ. 732:139310. doi: 10.1016/j.scitotenv.2020.139310
Sirajuddin, S., and Rosenzweig, A. C. (2015). Enzymatic oxidation of methane. Biochemistry 54, 2283–2294. doi: 10.1021/acs.biochem.5b00198
Soni, B. K., Conrad, J., Kelley, R. L., and Srivastava, V. J. (1998). “Effect of temperature and pressure on growth and methane utilization by several methanotrophic cultures,” in Biotechnology for Fuels and Chemicals, eds M. Finkelstein and B. H. Davison (Totowa, NJ: Humana Press), 729–738. doi: 10.1007/978-1-4612-1814-2_67
Stȩpniewska, Z., Goraj, W., Kuźniar, A., Pytlak, A., Ciepielski, J., and Fra̧czek, P. (2014). Biosynthesis of ectoine by the methanotrophic bacterial consortium isolated from Bogdanka coalmine (Poland). Appl. Biochem. Microbiol. 50, 594–600. doi: 10.1134/S0003683814110039
Takeguchi, M., Furuto, T., Sugimori, D., and Okura, I. (1997). Optimization of methanol biosynthesis by Methylosinus trichosporium OB3b: an approach to improve methanol accumulation. Appl. Biochem. Biotechnol. 68, 143–152. doi: 10.1007/BF02785987
Tao, L., Sedkova, N., Yao, H., Rick, W. Y., Sharpe, P. L., and Cheng, Q. (2007). Expression of bacterial hemoglobin genes to improve astaxanthin production in a methanotrophic bacterium Methylomonas sp. Appl. Microbiol. Biotechnol. 74, 625–633. doi: 10.1007/s00253-006-0708-8
Timmers, P. H., Gieteling, J., Widjaja-Greefkes, H. A., Plugge, C. M., Stams, A. J., Lens, P. N., et al. (2015). Growth of anaerobic methane-oxidizing archaea and sulfate-reducing bacteria in a high-pressure membrane capsule bioreactor. Appl. Environ. Microbiol. 81, 1286–1296. doi: 10.1128/AEM.03255-14
Tsapekos, P., Zhu, X., Pallis, E., and Angelidaki, I. (2020). Proteinaceous methanotrophs for feed additive using biowaste as carbon and nutrients source. Bioresour. Technol. 313:123646. doi: 10.1016/j.biortech.2020.123646
Valverde-Pérez, B., Xing, W., Zachariae, A. A., Skadborg, M. M., Kjeldgaard, A. F., Palomo, A., et al. (2020). Cultivation of methanotrophic bacteria in a novel bubble-free membrane bioreactor for microbial protein production. Bioresour. Technol. 310:123388. doi: 10.1016/j.biortech.2020.123388
Wang, Z., Shi, L. D., Lai, C. Y., and Zhao, H. P. (2019). Methane oxidation coupled to vanadate reduction in a membrane biofilm batch reactor under hypoxic condition. Biodegradation 30, 457–466. doi: 10.1007/s10532-019-09887-6
Whittenbury, R., Phillips, K. C., and Wilkinson, J. F. (1970). Enrichment, isolation and some properties of methane-utilizing bacteria. Microbiology 61, 205–218. doi: 10.1099/00221287-61-2-205
Wu, M., Luo, J. H., Hu, S., Yuan, Z., and Guo, J. (2019). Perchlorate bio-reduction in a methane-based membrane biofilm reactor in the presence and absence of oxygen. Water Res. 157, 572–578. doi: 10.1016/j.watres.2019.04.008
Xin, J. Y., Cui, J., Niu, J. Z., Hua, S. F., Xia, C., Li, S., et al. (2004a). Biosynthesis of methanol from CO2 and CH4 by methanotropic bacteria. Biotechnology 3, 67–71.
Xin, J. Y., Cui, J. R., Niu, J. Z., Hua, S. F., Xia, C. G., Li, S. B., et al. (2004b). Production of methanol from methane by methanotrophic bacteria. Biocatalys. Biotransform. 22, 225–229. doi: 10.1080/10242420412331283305
Xin, J. Y., Zhang, Y. X., Zhang, S., Xia, C. G., and Li, S. B. (2007). Methanol production from CO2 by resting cells of the methanotrophic bacterium Methylosinus trichosporium IMV 3011. J. Basic Microbiol. 47, 426–435. doi: 10.1002/jobm.200710313
Yan, X., Chu, F., Puri, A. W., Fu, Y., and Lidstrom, M. E. (2016). Electroporation-based genetic manipulation in type I methanotrophs. Appl. Environ. Microbiol. 82, 2062–2069. doi: 10.1128/AEM.03724-15
Yoo, Y. S., Han, J. S., Ahn, C. M., and Kim, C. G. (2015). Comparative enzyme inhibitive methanol production by Methylosinus sporium from simulated biogas. Environ. Technol. 36, 983–991. doi: 10.1080/09593330.2014.971059
Yu, Y., Ramsay, J. A., and Ramsay, B. A. (2009). Production of soluble methane monooxygenase during growth of Methylosinus trichosporium on methanol. J. Biotechnol. 139, 78–83. doi: 10.1016/j.jbiotec.2008.09.005
Zhang, Y., Xin, J., Chen, L., Song, H., and Xia, C. (2008). Biosynthesis of poly-3-hydroxybutyrate with a high molecular weight by methanotroph from methane and methanol. J. Nat. Gas Chem. 17, 103–109. doi: 10.1016/S1003-9953(08)60034-1
Keywords: methanotrophs, carbon dioxide, methane, greenhouse gas sequestration, methanol, high-value products, reactor configuration
Citation: Sahoo KK, Goswami G and Das D (2021) Biotransformation of Methane and Carbon Dioxide Into High-Value Products by Methanotrophs: Current State of Art and Future Prospects. Front. Microbiol. 12:636486. doi: 10.3389/fmicb.2021.636486
Received: 01 December 2020; Accepted: 22 February 2021;
Published: 10 March 2021.
Edited by:
Pramod P. Wangikar, Indian Institute of Technology Bombay, IndiaReviewed by:
Shireesh Srivastava, International Centre for Genetic Engineering and Biotechnology, IndiaCopyright © 2021 Sahoo, Goswami and Das. This is an open-access article distributed under the terms of the Creative Commons Attribution License (CC BY). The use, distribution or reproduction in other forums is permitted, provided the original author(s) and the copyright owner(s) are credited and that the original publication in this journal is cited, in accordance with accepted academic practice. No use, distribution or reproduction is permitted which does not comply with these terms.
*Correspondence: Debasish Das, ZGViYXNpc2hkQGlpdGcuYWMuaW4=
Disclaimer: All claims expressed in this article are solely those of the authors and do not necessarily represent those of their affiliated organizations, or those of the publisher, the editors and the reviewers. Any product that may be evaluated in this article or claim that may be made by its manufacturer is not guaranteed or endorsed by the publisher.
Research integrity at Frontiers
Learn more about the work of our research integrity team to safeguard the quality of each article we publish.