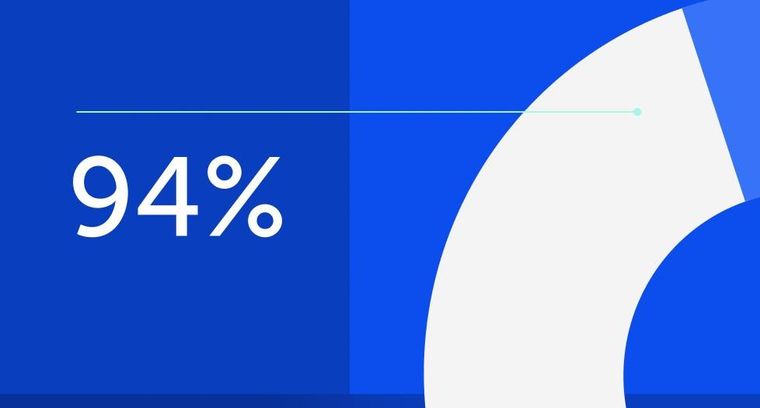
94% of researchers rate our articles as excellent or good
Learn more about the work of our research integrity team to safeguard the quality of each article we publish.
Find out more
ORIGINAL RESEARCH article
Front. Microbiol., 02 February 2021
Sec. Microbial Physiology and Metabolism
Volume 12 - 2021 | https://doi.org/10.3389/fmicb.2021.636421
Studies of biofilm formation by bacteria are crucial for understanding bacterial resistance and for development of novel antibacterial strategies. We have developed a new bioluminescence biofilm assay for Listeria innocua, which is considered a non-pathogenic surrogate for Listeria monocytogenes. L. innocua was transformed with a plasmid for inducible expression of NanoLuc luciferase (Nluc). Concentration-dependent bioluminescence signals were obtained over a concentration range of more than three log units. This biofilm assay enables absolute quantification of bacterial cells, with the necessary validation. For biofilm detection and quantification, this “Nluc bioluminescence” method has sensitivity of 1.0 × 104 and 3.0 × 104 colony forming units (CFU)/mL, respectively, with a dynamic range of 1.0 × 104 to 5.0 × 107 CFU/mL. These are accompanied by good precision (coefficient of variation, <8%) and acceptable accuracy (relative error for most samples, <15%). This novel method was applied to assess temporal biofilm formation of L. innocua as a function of concentration of inoculant, in comparison with conventional plating and CFU counting, the crystal violet assay, and the resazurin fluorescence assay. Good correlation (r = 0.9684) of this Nluc bioluminescence assay was obtained with CFU counting. The limitations of this Nluc bioluminescence assay include genetic engineering of bacteria and relatively high cost, while the advantages include direct detection, absolute cell quantification, broad dynamic range, low time requirement, and high sensitivity. Nluc-based detection of L. innocua should therefore be considered as a viable alternative or a complement to existing methods.
Biofilms are the predominant form of bacterial lifestyle that provide bacteria with resistance to adverse environmental conditions, including protection against antimicrobials and disinfectants (de la Fuente-Nunez et al., 2013). To combat the increasing occurrence of resistance to antimicrobials in bacteria, detailed understanding of biofilm formation is crucial. Studies of the processes involved in the different stages of biofilm formation will provide new concepts for development of novel antibacterial strategies.
Listeria sensu stricto are ubiquitous Gram-positive bacteria found in soil, water, and vegetation that can colonize mammalian hosts (Haase et al., 2014). Two species, L. monocytogenes and L. ivanovii, are considered pathogenic, whereas others live as saprophytes only. Infection with L. monocytogenes is associated with high hospitalization and mortality rates compared to other foodborne infectious diseases (EFSA and ECDC, 2019). Due to the L. monocytogenes pathogenicity and persistence, the use of a non-pathogenic surrogate is preferred for inactivation tests, and especially for in-field studies. L. innocua has been proposed as a surrogate organism for L. monocytogenes, because they have genetic and physiological similarities, but L. innocua lacks the virulence factors of L. monocytogenes (Fairchild and Foegeding, 1993; Friedly et al., 2008; Silva-Angulo et al., 2015; Costa et al., 2018) and allows work in Biosafety Level 1 laboratory. L. monocytogenes strains differ in their ability to form biofilms (Barbosa et al., 2013; Lee et al., 2019), and an interval of 105 to 108 colony forming units (CFU)/cm2 has been reported (Reis-Teixeira et al., 2017). High-biofilm-forming strains produce a dense, three-dimensional biofilm, whereas low-biofilm-forming strains produce a thin, patchy biofilm (Borucki et al., 2003). Increased biofilm formation was observed in serotypes 1/2a and 1/2c (Borucki et al., 2003), or serotypes 3a and 4a (Capita et al., 2019). The biofilm production correlates with environmental conditions, such as temperature and acidic conditions (Borucki et al., 2003; Nilsson et al., 2011; Barbosa et al., 2013). Similar biofilm forming ability was reported for L. monocytogenes and L. innocua (Costa et al., 2018); however, due to variability between strains this cannot be generalized.
Biofilm assays include direct and indirect methods, whereby the latter require detachment of the microorganisms from the surface prior to their counting (Grudlewska-Buda et al., 2020). Several methods for the evaluation of listerial biofilms have been developed, which include the classical culture-based plate counting method, biomass staining methods (e.g., crystal violet and safranin red), DNA staining methods (e.g., Syto 9), use of chromogenic or fluorogenic metabolic substrates for detection of live bacteria (e.g., tetrazolium salts, resazurin), and also qPCR and digital droplet PCR for quantification of bacterial DNA (Stiefel et al., 2016; Klančnik et al., 2017; Ripolles-Avila et al., 2019; Grudlewska-Buda et al., 2020). New methods for biofilm monitoring are still being introduced; however, both current and new methods have limitations, such as limited accuracy and precision, high detection limits, high cost, long duration, and high workload. Different methods are complementary and assess different pieces of information of the biofilm (e.g., microscopy and plate count) and their outcomes should be merged to obtain a clearer picture of the biofilm. In Listeria biofilm research, new methods that enable reproducible detection and quantification of low numbers of bacteria are needed, due to the low biofilm biomass.
Direct detection and quantification of bacteria can be facilitated through genetic engineering and expression of reporter proteins, such as fluorescent proteins and luciferases (Plavec and Berlec, 2019). These enable visualization of the bacteria, and are compatible with fluorescence and bioluminescence colony counting and microplate assays, as well as epifluorescence and confocal microscopy, and flow cytometry. However, the genetic engineering of fluorescent or bioluminescent Listeria is not straightforward, and only a few examples have been reported. The pNF8 Escherichia coli/Listeria shuttle plasmid that has a strong listerial promoter has been transferred from E. coli to L. monocytogenes via conjugation to express green fluorescent protein (Fortinea et al., 2000). The same plasmid was transformed into both L. monocytogenes and L. innocua by optimized electroporation conditions (Ma et al., 2011). A derivative of the pNZ8148 lactococcal plasmid with a strong promoter from lactobacilli was used to express the anaerobic fluorescent protein evoglow-Pp1 in L. innocua (Landete et al., 2017).
Fluorescence detection is often used for microscopy; however, it is less appropriate for high-throughput screening due to its lower sensitivity. With low numbers of bacteria, only a weak cumulative fluorescence signal is generated. A stronger signal can be obtained using bioluminescence, whereby the light is produced by luciferase conversion of an appropriate substrate, which provides amplification of the signal. Bioluminescence has already been applied to the monitoring of Listeria biofilms and to in vivo imaging of Listeria infection (Hibma et al., 1996; Hardy et al., 2004; Bron et al., 2006; Riedel et al., 2007). In the most effective example, the Lux operon from Photorhabdus luminescens was integrated into the L. monocytogenes genome (Bron et al., 2006; Riedel et al., 2007). The downside of this approach was the use of the whole operon (>5,000 bp), and the relatively low dynamic range of 2 log units. Also, none of the reports have provided precise quantification of Listeria on the basis of bioluminescence.
Recently, a new luciferase from Oplophorus gracilirostris was reported to have superior properties, which is known as NanoLuc (Nluc). Its advantages include its small size (19 kDa), high stability, and high bioluminescence efficiency (150-fold that of other luciferases). Several different applications have already been developed, which include studies of protein–protein interactions, gene regulation, cell signaling, and protein stability (England et al., 2016). However, to the best of our knowledge, the present study is the first example of the use of Nluc for monitoring and quantification of bacterial biofilms.
In the present study, the aim was to produce a non-pathogenic surrogate of L. monocytogenes, as L. innocua with the expression of Nluc, and to use this surrogate to set up a L. innocua quantification assay. This was then applied to monitor biofilm formation in microtiter plates, and included a comparison with other routinely used and established methods for biofilm determination. New information about these biofilms was also obtained. At present, the assay was established in L. innocua ŽM39, surrogate for L. monocytogenes ŽM58. To test the assay in other strains, recombinant variants will have to be engineered in further studies.
Listeria innocua ŽM39 (heat-treated chicken meat; Biosafety Level 1) and L. monocytogenes ŽM58 (Klančnik et al., 2015; Biosafety Level 2) were grown at 37°C in tryptic soy broth with shaking, or in the same medium solidified with 1.5% agar in accordance with institutional regulations (ŽM: strain designation in the collection of the Laboratory for Food Microbiology at the Biotechnical Faculty, University of Ljubljana, Slovenia). E. coli DH5α was grown at 37°C in lysogeny broth with aeration. Following transformation, erythromycin was added to the growth medium of L. innocua (10 μg/mL) and E. coli (200 μg/mL) to maintain the selection pressure. Nisin (25 ng/mL) was added to tryptic soy broth to induce Nluc expression in L. innocua.
The Nluc protein (Hall et al., 2012) was back-translated and codon-optimized in the nluc gene (GenBank accession number MW139745), which was synthesized as gBlock (Integrated DNA Technologies, Leuven, Belgium; Supplementary Figure S1). Restriction recognition sites were added by PCR amplification, with the primers Nluc-Nco-F (5′-TCCATGGTATTTACCCTTGAAGATTTTG-3′) and Nluc-Xba-R (5′-TTCTAGATTAAGCCAAGATTCTTTCGCATAATC-3′). The PCR amplicon was cloned into the pMSP3545 plasmid (Bryan et al., 2000; Berlec et al., 2006) using the NcoI and XbaI restriction enzymes (Fast Digest, Thermo Scientific, Vilnius, Lithuania), to provide the plasmid pMSP:Nluc (Supplementary Figure S1), and transformed into E. coli. Plasmid DNA was isolated using NucleoSpin Plasmid kit (Macherey-Nagel, Düren, Germany), and sequenced (Eurofins Genomics, Konstanz, Germany). L. innocua was transformed by electroporation as previously reported (Ma et al., 2011).
The bioluminescence was measured using a plate reader (Infinite M-1000; Tecan, Salzburg, Austria) (settings: luminescence mode; integration time, 1 s; settle time, 150 ms) in white flat-bottomed 96-well plates (Corning, NY, United States). Nano-Glo Luciferase assay reagent (Promega, Madison, United States) was prepared according to the manufacturer instructions, and brought to room temperature. Bacterial samples (50 μL) were mixed with 50 μL reagent, and incubated for 10 min at room temperature before measurement.
Different concentrations of bacteria were prepared from standardized inocula based on optical density measurements. This range was used for construction of the calibration curve [six serial fivefold dilutions from 5.0 × 107 CFU/mL bacterial dispersion in phosphate-buffered saline (PBS), all in duplicate]. The L. innocua concentrations in the samples were determined using linear regression of the calibration curve. The limit of detection (LOD) and limit of quantification (LOQ) were determined as the means of six measurements of the blank increased by 3 standard deviations (LOD) and by 10 standard deviations (LOQ), and calculated using the calibration curve. The accuracy was determined using three known concentrations of L. innocua (i.e., 5.0 × 106, 1.0 × 106, 1.0 × 105 CFU/mL), each as two biological and three technical repeats. The relative error was calculated for each measurement. The precision was determined using the same three known concentrations of L. innocua, each as six technical repeats. The coefficient of variation was calculated for each measurement. The accuracy and precision were determined twice, on two separate days, to estimate the repeatability of the assay.
Overnight L. innocua culture was diluted 1:50 in fresh TSB medium supplemented with erythromycin and nisin. After 8 h of incubation at 37°C with shaking, the bacteria were harvested by centrifugation (10 min at 24°C and 3,000 g) and the OD600 was adjusted in fresh PBS to the value of 0.55, corresponding to 5 × 107 CFU/ml (as verified by inoculation on TSA). Different concentrations of L. innocua (1.0 × 107, 1.0 × 105, 1.0 × 103 CFU/mL) were obtained by dilution, transferred in 100 μL to white flat-bottomed 96-well plates (Corning, NY, United States) and incubated at 30°C for 4, 8, 24, 48, and 72 h without shaking. At each time, the supernatant was removed and the biofilm was gently washed three times with 100 μL PBS.
For bioluminescence detection, 50 μL PBS was added to the biofilm-containing wells. Freshly prepared bacterial dispersions for the calibration curve were also added to the plates. Then 50 μL Nano-Glo Luciferase assay reagent was added to each well, and the bioluminescence was measured as described above.
For CFU counting, 100 μL PBS was added to the biofilm-containing wells. The plates were parafilm-protected and sonicated for 5 min to detach bound cells. Bacterial suspensions (100 μL) were then transferred to deep-wells containing 900 μL PBS and were serially diluted and plated as previously described (Klančnik et al., 2017). After drying the inoculum, the agar plates were incubated for up to 48 h at 37°C and the grown colonies were counted.
For the crystal violet assay (Kurinčič et al., 2016), the plates were dried after washing at 60°C for 15 min. Crystal violet (0.5%, Merck, Darmstadt. Germany) was added to each well (100 μL) and incubated for 15 min at room temperature. After staining, the plates were washed and dried as described above. The bound crystal violet was dissolved in 100 μL of 33% acetic acid (Merck, Darmstadt. Germany), and the absorbance was measured at 584 nm (VarioskanLux, Thermo Fisher Scientific, Yokohama, Japan).
For the resazurin fluorescence assay for biofilm detection, 100 μL PBS, 10 μL resazurin reagent (prepared from 10 mM resazurin sodium salt; Sigma-Aldrich, Saint Quentin−Fallavier, France), and 0.8 mM menadione (Sigma-Aldrich, Saint Quentin−Fallavier, France) (Kovač et al., 2015) were added, mixed, and incubated for 1 h at 37°C. The fluorescence was measured at 560 nm for excitation and 590 nm for emission (VarioskanLux, Thermo Fisher Scientific, Yokohama, Japan). The minimal reliable signal for the crystal violet and resazurin fluorescence assays were calculated by increasing the background signal by three times the standard deviation of the background (Stiefel et al., 2016).
Statistical analyses were performed using the GraphPad Prism 5 (GraphPad Software, San Diego, United States). Correlations were determined using Pearson’s coefficients.
Heterologous expression of Nluc in L. innocua was confirmed by comparison of the bioluminescence of L. innocua containing the nluc gene (plasmid pMSP:Nluc) and the control L. innocua containing the empty plasmid. Significantly higher and concentration-dependent bioluminescence intensity was obtained with the Nluc-expressing L. innocua (Figure 1), which suggested that this bioluminescence can be used for specific determination of the Nluc-expressing L. innocua, and for determination of L. innocua concentrations.
Figure 1. Bioluminescence intensity (logLI) as a function of concentration (logC) of L. innocua expressing Nluc (i.e., containing plasmid pMSP:Nluc; red) or control L. innocua (i.e., containing empty plasmid pMSP3545; black).
An assay for determination of L. innocua concentrations was established and validated as previously reported for biological assays (Berlec and Štrukelj, 2014). The parameters determined were linear range interval, limit of detection, limit of quantification, accuracy, and precision, with repeatability determined by performing the assay on multiple days. A linear relationship between the logarithm of bacterial concentration and the logarithm of bioluminescence intensity was established in the L. innocua concentration range from 1.6 × 104 to 5.0 × 107 CFU/mL, thereby representing more than 3,000-fold dynamic range in a single assay without the need for dilution of the samples. The calibration curve consisted of six bacterial concentrations obtained with fivefold serial dilutions of the Nluc-expressing L. innocua suspension at 5.0 × 107 CFU/mL. The calibration curve was repeatable, with R2 > 0.99 routinely achieved in separate assays. Two representative examples of the calibration curve and R2 are shown in Supplementary Figure S2. The LOD and LOQ were determined on two separate days and are given in Table 1.
Table 1. Limits of detection (LOD) and quantification (LOQ) of the Nluc bioluminescence assay were calculated, using calibration curves, from the average of six measurements of the blank increased by 3 standard deviations (for LOD) and by 10 standard deviations (for LOQ).
The accuracy of the Nluc bioluminescence assay was determined for three different concentrations of L. innocua (i.e., 5.00 × 106, 1.00 × 106, 1.00 × 105 CFU/mL) in two independent biological repeats. Each of the measurements was performed as three technical repeats. To determine the repeatability, the accuracy was determined on two separate days (Table 2). The relative error of the majority of the samples was <15%. Two samples had a relative error from 15 to 30%, and on the second day, the relative errors of the samples with the lowest concentration were over 30% (31.5 and 33.3%).
To determine the precision, the same three concentrations of L. innocua (i.e., 5.00 × 106, 1.00 × 106, 1.00 × 105 CFU/mL) were assayed as six technical repeats. To address repeatability, the precision was also determined on two separate days. The coefficients of variation of all of the samples were well below 8%, which indicated the high precision and good repeatability of this Nluc bioluminescence assay (Table 3).
L. innocua biofilm formation in polystyrene microtiter plates was initiated with different concentrations of inoculum and monitored over 72 h using the Nluc bioluminescence assay, plating and CFU counting, crystal violet staining, and resazurin fluorescence (Figure 2).
Figure 2. Monitoring of biofilm formation on polystyrene plates over 72 h using the Nluc bioluminescence assay (A), CFU counting assay (B), crystal violet staining (C), and resazurin fluorescence (D). Bars of the same color indicate biological repeats, each performed as three technical repeats. Different concentrations of L. innocua were inoculated to trigger biofilm formation (1.0 × 107 CFU/mL, red; 1.0 × 105 CFU/mL, yellow; 1.0 × 103 CFU/mL, gray). Error bars: standard deviation; horizontal dashed blue lines, limit of detection (Nluc) or minimal reliable signal (crystal violet, resazurin). FI, fluorescence intensity; n.d., no CFU on agar plates (i.e., <1 log CFU/mL).
The dynamics of biofilm formation determined with the Nluc bioluminescence assay depended on the concentration of inoculum (Figure 2A). The biofilm formation initiated with the lowest concentration of inoculum required more time to reach the highest concentration of biofilm cells (72 h for 1.0 × 103 CFU/mL inoculum vs. 4 h for 5.0 × 107 CFU/mL inoculant); however, the final concentrations of biofilm cells tended to be higher for biofilms initiated with lower concentrations of inoculum (3.88 × 107 CFU/mL for 1.0 × 103 CFU/mL inoculum vs. 1.25 × 107 CFU/mL for 1.0 × 107 CFU/mL inoculum). The concentrations of the bacterial cells at each time point were calculated from freshly prepared calibration curves, and all of the concentrations determined were > LOD.
The quantification of the L. innocua cells (i.e., CFU) during biofilm formation was also determined with the plate counting method adopting the same experimental set-up (Figure 2B). Similar biofilm formation properties were also observed for control L. innocua containing empty plasmid pMSP3545 (Supplementary Figure S3), as well as for L. monocytogenes (Supplementary Figure S4). Good correlation for the L. innocua concentrations was obtained between the Nluc bioluminescence assay and the CFU counting assay by comparing the same time and the same inoculum concentration (Pearson coefficient r = 0.9684; Figure 3A). However, the Nluc bioluminescence assay indicated higher L. innocua concentrations by up to 2 log units, particularly during the first 24 h, which might be attributable to the indirect nature of the plate counting method and possibly to the detection of not only live, but also dead and viable-but-non-cultivable cells in the bioluminescence assay, which are not detected with plate counting.
Figure 3. Correlations of L. innocua concentrations in biofilms determined with Nluc bioluminescence assay and the CFU counting assay (A), the crystal violet (CV) staining assay (B), and the resazurin fluorescence intensity (FI) (C). The Pearson’s coefficients (r) are also shown.
Biofilm formation was also determined using crystal violet, which stains the whole biomass of the biofilm (i.e., bacteria and extracellular matrix). The temporal profile of the biofilm formation here was similar to that obtained with the other methods (Figure 2C). At 4 and 8 h, only one of the measured absorbances was above the minimal reliable signal, which indicated the lower sensitivity of this method in comparison to the Nluc bioluminescence and CFU counting assays. Also, the larger standard errors indicated the lower repeatability of the method.
Temporal biofilm formation determined using the resazurin assay was again similar to that for the other methods (Figure 2D). Similar to crystal violet staining, the resazurin fluorescence assay had lower sensitivity (4, 8, and even 24 h, several values below the minimal reliable signal). This resazurin fluorescence assay provides absolute quantification of bacterial concentration when calibrated against CFU count; however, the assay has a lower dynamic range compared to the Nluc bioluminescence assay when the same concentrations are used for calibration curve, with only 1 log unit increase in the signal (Supplementary Figure S5). The calibration curve was therefore not used for absolute quantification of L. innocua.
The correlations between the Nluc bioluminescence L. innocua concentrations and crystal violet staining and resazurin fluorescence were lower than for CFU counting (Figure 3). However, these correlations are not directly comparable, as for crystal violet staining and resazurin fluorescence the L. innocua concentrations were not determined.
Listeria are ubiquitous bacteria, of which L. monocytogenes is an important foodborne bacterium that can persist in the environment as biofilms (EFSA and ECDC, 2019). As these are pathogenic bacteria, L. innocua has been used as a surrogate in a number of studies (Fairchild and Foegeding, 1993; Friedly et al., 2008; Milillo et al., 2012; Silva-Angulo et al., 2015; Costa et al., 2018). Due to increasing research into bacterial biofilms and the way they are formed and maintained, methods based on microtiter plates are widely used, as these allow large numbers of simultaneous reactions to be carried out. However, the existing methods have some shortcomings, which indicates the need for the development of new methods for such studies. Therefore, this new method based on detection of Nluc bioluminescence of transformed L. innocua was developed.
NanoLuc luciferase was effectively expressed in L. innocua using the nisin-inducible expression system (Mierau and Kleerebezem, 2005), with a concentration-dependent bioluminescence signal obtained over a broad L. innocua concentration range of more than three log units. Validation of the method for quantification of Nluc-expressing L. innocua was performed. This method has high sensitivity (1.0 × 104 CFU/mL for detection, 3.0 × 104 CFU/mL for quantification), broad dynamic range (1.0 × 104–5.0 × 107 CFU/mL), good day-to-day repeatability and good precision (coefficient of variation, < 8%). The accuracy is also acceptable (relative error for most samples, <15%), with the exception of the lower concentrations, where the error can be up to 30%.
This Nluc bioluminescence assay was then used to monitor L. innocua adhesion and biofilm formation on a polystyrene surface over the course of 72 h. These data were also compared with data obtained with the other commonly used methods for biofilm detection in microtiter plates; i.e., conventional plating and CFU counting, the crystal violet staining assay, and the resazurin fluorescence assay. The kinetics of biofilm formation corresponded to previous studies on L. monocytogenes (Chavant et al., 2007; Saá Ibusquiza et al., 2012), which shows comparable biofilm formation to L. innocua (Costa et al., 2018), and was also observed in the present study using CFU count. However, to the best of our knowledge, the process of temporal biofilm formation in L. innocua has not been described yet. Under the conditions of the present study, the L. innocua biofilms formed in 24 h, with cell adhesion already seen in the first 8 h. The trend for biofilm formation over time was comparable between the methods used here. The data for the Nluc bioluminescence assay and plating (CFU counting) correlated well, with higher L. innocua concentrations determined using the bioluminescence assay. This can be attributed to the not cultivable cells also detected in the Nluc bioluminescence assay. Conversely, the correlations of the Nluc bioluminescence assay with crystal violet staining and resazurin fluorescence assays were a little lower. In addition to the influence of time, the effect of inoculum concentration on biofilm formation was observed, with particular focus in the first 24 h. At the lower inoculum concentrations, the process of biofilm formation was slower.
Advantages and disadvantages can be seen for all of these assays, which are summarized in Table 4. The methods differ in terms of the necessary research equipment and chemicals, the time needed to perform the assay, the sensitivity and suitability for observing dynamic processes, and the biofilm components that are detected. The Nluc bioluminescence assay detects all phenotypic subpopulations within the growing biofilm, cultivable, viable-but-not cultivable forms and also damaged but not lysed cells. This may be advantageous in evaluation of total biofilm population that includes slowly growing tolerant and persistent cells which are crucial for biofilm persistence (Matereke and Okoh, 2020). Crystal violet stain binds to negative charged molecules in the biofilm biomass and thus detects live and dead cells, as well as the exopolymeric matrix (Costa et al., 2018). The greatest problem with the crystal violet assay is its variability, and therefore deviations in the data, which are due to the operator that performs the assay (Lourenco et al., 2012). This was also evident in the present study, where detectable dye binding was only seen after 24 h. Better reproducibility of these data can be achieved with methods for the determination of live cells. As transition of Listeria cells to a viable-but-non-cultivable state is also possible under stress conditions (Highmore et al., 2018), the drawback of the CFU counting assay was that it determines only cultivable live cells. In contrast, all live cells are determined using the resazurin fluorescence assay, which is based on detection of metabolically active cells (Van den Driessche et al., 2014). However, this resazurin method was less sensitive, as at least 106 CFU/mL were required to detect any change in fluorescence. These three methods here are among the most commonly used to determine the formation of bacterial biofilms and for screening for anti-biofilm agents using microtiter plates. Due to the disadvantages and advantages that each of these methods have, the data obtained here suggest the need for application of complementary assays in biofilm studies.
Table 4. Comparisons of the Nluc bioluminescence assay for biofilm evaluation with the assays using CFU counting, crystal violet staining, and resazurin fluorescence.
In this study, the Nluc bioluminescence assay enabled temporal monitoring of L. innocua biofilm formation in polystyrene plates. Thus, this represents a feasible alternative, or complement, to the existing methods. The limitation of this method includes the requirement for genetic engineering of the bacteria and the relatively high cost, although the cost remains lower than for molecular biology methods (e.g., qPCR), which were not included in this comparison. On the other hand, the Nluc bioluminescence assay has several important advantages over the other methods, which include direct detection mode, absolute cell quantification, broad dynamic range, low time requirement, and high sensitivity. In further studies, the assay will be introduced in other Listeria species and strains to confirm its general applicability.
The datasets presented in this study can be found in online repositories. The names of the repository/repositories and accession number(s) can be found below: https://www.ncbi.nlm.nih.gov/genbank/, MW139745.
AB, AK, and JS conceived and designed the research. NJ and MS conducted the experiments. AB and MS analyzed the data. AB wrote the manuscript. All authors read and approved the manuscript.
This study was funded by the Slovenian Research Agency (Grant Nos. P4-0127, J4-9327, and J4-1771).
The authors declare that the research was conducted in the absence of any commercial or financial relationships that could be construed as a potential conflict of interest.
We thank Natalija Vidic and Dominika Celar Šturm for excellent technical support and Dr. Christopher Berrie for the critical reading of the manuscript.
The Supplementary Material for this article can be found online at: https://www.frontiersin.org/articles/10.3389/fmicb.2021.636421/full#supplementary-material
Barbosa, J., Borges, S., Camilo, R., Magalhaes, R., Ferreira, V., Santos, I., et al. (2013). Biofilm formation among clinical and food isolates of Listeria monocytogenes. Int. J. Microbiol. 2013:524975. doi: 10.1155/2013/524975
Berlec, A., and Štrukelj, B. (2014). A high-throughput biliverdin assay using infrared fluorescence. J. Vet. Diagn. Invest. 26, 521–526. doi: 10.1177/1040638714535403
Berlec, A., Jevnikar, Z., Majhenič, A. C., Rogelj, I., and Štrukelj, B. (2006). Expression of the sweet-tasting plant protein brazzein in Escherichia coli and Lactococcus lactis: a path toward sweet lactic acid bacteria. Appl. Microbiol. Biotechnol. 73, 158–165. doi: 10.1007/s00253-006-0438-y
Borucki, M. K., Peppin, J. D., White, D., Loge, F., and Call, D. R. (2003). Variation in biofilm formation among strains of Listeria monocytogenes. Appl. Environ. Microbiol. 69, 7336–7342. doi: 10.1128/aem.69.12.7336-7342.2003
Bron, P. A., Monk, I. R., Corr, S. C., Hill, C., and Gahan, C. G. (2006). Novel luciferase reporter system for in vitro and organ-specific monitoring of differential gene expression in Listeria monocytogenes. Appl. Environ. Microbiol. 72, 2876–2884. doi: 10.1128/AEM.72.4.2876-2884.2006
Bryan, E. M., Bae, T., Kleerebezem, M., and Dunny, G. M. (2000). Improved vectors for nisin-controlled expression in gram-positive bacteria. Plasmid 44, 183–190. doi: 10.1006/plas.2000.1484
Capita, R., Felices-Mercado, A., Garcia-Fernandez, C., and Alonso-Calleja, C. (2019). Characterization of Listeria monocytogenes originating from the Spanish meat-processing chain. Foods 8:542. doi: 10.3390/foods8110542
Chavant, P., Gaillard-Martinie, B., Talon, R., Hebraud, M., and Bernardi, T. (2007). A new device for rapid evaluation of biofilm formation potential by bacteria. J. Microbiol. Methods 68, 605–612. doi: 10.1016/j.mimet.2006.11.010
Costa, A., Lourenco, A., Civera, T., and Brito, L. (2018). Listeria innocua and Listeria monocytogenes strains from dairy plants behave similarly in biofilm sanitizer testing. LWT 92, 477–483. doi: 10.1016/j.lwt.2018.02.073
de la Fuente-Nunez, C., Reffuveille, F., Fernandez, L., and Hancock, R. E. (2013). Bacterial biofilm development as a multicellular adaptation: antibiotic resistance and new therapeutic strategies. Curr. Opin. Microbiol. 16, 580–589. doi: 10.1016/j.mib.2013.06.013
EFSA, and ECDC (2019). The European Union one health 2018 zoonoses report. EFSA J. 17:5926. doi: 10.2903/J.Efsa.2019.5926
England, C. G., Ehlerding, E. B., and Cai, W. (2016). NanoLuc: a small luciferase is brightening up the field of bioluminescence. Bioconjug. Chem. 27, 1175–1187. doi: 10.1021/acs.bioconjchem.6b00112
Fairchild, T. M., and Foegeding, P. M. (1993). A proposed nonpathogenic biological indicator for thermal inactivation of Listeria monocytogenes. Appl. Environ. Microbiol. 59, 1247–1250. doi: 10.1128/AEM.59.4.1247-1250.1993
Fortinea, N., Trieu-Cuot, P., Gaillot, O., Pellegrini, E., Berche, P., and Gaillard, J. L. (2000). Optimization of green fluorescent protein expression vectors for in vitro and in vivo detection of Listeria monocytogenes. Res. Microbiol. 151, 353–360. doi: 10.1016/s0923-2508(00)00158-3
Friedly, E. C., Crandall, P. G., Ricke, S., O’Bryan, C. A., Martin, E. M., and Boyd, L. M. (2008). Identification of Listeria innocua surrogates for Listeria monocytogenes in hamburger patties. J. Food Sci. 73, M174–M178. doi: 10.1111/j.1750-3841.2008.00719.x
Grudlewska-Buda, K., Skowron, K., and Gospodarek-Komkowska, E. (2020). Comparison of the intensity of biofilm formation by Listeria monocytogenes using classical culture-based method and digital droplet PCR. AMB Express 10:75. doi: 10.1186/s13568-020-01007-5
Haase, J. K., Didelot, X., Lecuit, M., Korkeala, H., Group, L. M. M. S., and Achtman, M. (2014). The ubiquitous nature of Listeria monocytogenes clones: a large-scale multilocus sequence typing study. Environ. Microbiol. 16, 405–416. doi: 10.1111/1462-2920.12342
Hall, M. P., Unch, J., Binkowski, B. F., Valley, M. P., Butler, B. L., Wood, M. G., et al. (2012). Engineered luciferase reporter from a deep sea shrimp utilizing a novel imidazopyrazinone substrate. ACS Chem. Biol. 7, 1848–1857. doi: 10.1021/cb3002478
Hardy, J., Francis, K. P., DeBoer, M., Chu, P., Gibbs, K., and Contag, C. H. (2004). Extracellular replication of Listeria monocytogenes in the murine gall bladder. Science 303, 851–853. doi: 10.1126/science.1092712
Hibma, A. M., Jassim, S. A., and Griffiths, M. W. (1996). In vivo bioluminescence to detect the attachment of L-forms of Listeria monocytogenes to food and clinical contact surfaces. Int. J. Food Microbiol. 33, 157–167. doi: 10.1016/0168-1605(96)01123-3
Highmore, C. J., Warner, J. C., Rothwell, S. D., Wilks, S. A., and Keevil, C. W. (2018). Viable-but-nonculturable Listeria monocytogenes and Salmonella enterica serovar Thompson induced by chlorine stress remain infectious. mBio 9:e00540-18. doi: 10.1128/mBio.00540-18
Klančnik, A., Šikić Pogačar, M., Trošt, K., Tušek Žnidarič, M., Mozetič Vodopivec, B., and Smole Možina, S. (2017). Anti-Campylobacter activity of resveratrol and an extract from waste Pinot noir grape skins and seeds, and resistance of Camp. jejuni planktonic and biofilm cells, mediated via the CmeABC efflux pump. J. Appl. Microbiol. 122, 65–77. doi: 10.1111/jam.13315
Klančnik, A., Toplak, N., Kovač, M., Marquis, H., and Jeršek, B. (2015). Quantification of Listeria monocytogenes cells with digital PCR and their biofilm cells with real-time PCR. J. Microbiol. Methods 118, 37–41. doi: 10.1016/j.mimet.2015.08.012
Kovač, J., Šimunović, K., Wu, Z., Klančnik, A., Bučar, F., Zhang, Q., et al. (2015). Antibiotic resistance modulation and modes of action of (-)-alpha-pinene in Campylobacter jejuni. PLoS One 10:e0122871. doi: 10.1371/journal.pone.0122871
Kurinčič, M., Jeršek, B., Klančnik, A., Smole Možina, S., Fink, R., Dražić, G., et al. (2016). Effects of natural antimicrobials on bacterial cell hydrophobicity, adhesion, and zeta potential. Arh. Hig. Rada Toksikol. 67, 39–45. doi: 10.1515/aiht-2016-67-2720
Landete, J. M., Peiroten, A., Medina, M., and Arques, J. L. (2017). Short communication: labeling Listeria with anaerobic fluorescent protein for food safety studies. J. Dairy Sci. 100, 113–117. doi: 10.3168/jds.2016-11226
Lee, B. H., Cole, S., Badel-Berchoux, S., Guillier, L., Felix, B., Krezdorn, N., et al. (2019). Biofilm Formation of Listeria monocytogenes strains under food processing environments and pan-genome-wide association study. Front. Microbiol. 10:2698. doi: 10.3389/fmicb.2019.02698
Lourenco, A., Rego, F., Brito, L., and Frank, J. F. (2012). Evaluation of methods to assess the biofilm-forming ability of Listeria monocytogenes. J. Food Prot. 75, 1411–1417. doi: 10.4315/0362-028X.JFP-11-464
Ma, L., Zhang, G., and Doyle, M. P. (2011). Green fluorescent protein labeling of Listeria, Salmonella, and Escherichia coli O157:H7 for safety-related studies. PLoS One 6:e18083. doi: 10.1371/journal.pone.0018083
Matereke, L. T., and Okoh, A. I. (2020). Listeria monocytogenes virulence, antimicrobial resistance and environmental persistence: a review. Pathogens 9:528. doi: 10.3390/pathogens9070528
Mierau, I., and Kleerebezem, M. (2005). 10 years of the nisin-controlled gene expression system (NICE) in Lactococcus lactis. Appl. Microbiol. Biotechnol. 68, 705–717. doi: 10.1007/s00253-005-0107-6
Milillo, S. R., Friedly, E. C., Saldivar, J. C., Muthaiyan, A., O’Bryan, C., Crandall, P. G., et al. (2012). A review of the ecology, genomics, and stress response of Listeria innocua and Listeria monocytogenes. Crit. Rev. Food Sci. Nutr. 52, 712–725. doi: 10.1080/10408398.2010.507909
Nilsson, R. E., Ross, T., and Bowman, J. P. (2011). Variability in biofilm production by Listeria monocytogenes correlated to strain origin and growth conditions. Int. J. Food Microbiol. 150, 14–24. doi: 10.1016/j.ijfoodmicro.2011.07.012
Plavec, T. V., and Berlec, A. (2019). Engineering of lactic acid bacteria for delivery of therapeutic proteins and peptides. Appl. Microbiol. Biotechnol. 103, 2053–2066. doi: 10.1007/s00253-019-09628-y
Reis-Teixeira, F. B. D., Alves, V. F., and de Martinis, E. C. P. (2017). Growth, viability and architecture of biofilms of Listeria monocytogenes formed on abiotic surfaces. Braz. J. Microbiol. 48, 587–591. doi: 10.1016/j.bjm.2017.01.004
Riedel, C. U., Monk, I. R., Casey, P. G., Morrissey, D., O’Sullivan, G. C., Tangney, M., et al. (2007). Improved luciferase tagging system for Listeria monocytogenes allows real-time monitoring in vivo and in vitro. Appl. Environ. Microbiol. 73, 3091–3094. doi: 10.1128/AEM.02940-06
Ripolles-Avila, C., Cervantes-Huaman, B. H., Hascoet, A. S., Yuste, J., and Rodriguez-Jerez, J. J. (2019). Quantification of mature Listeria monocytogenes biofilm cells formed by an in vitro model: a comparison of different methods. Int. J. Food Microbiol. 289, 209–214. doi: 10.1016/j.ijfoodmicro.2018.10.020
Saá Ibusquiza, P., Herrera, J. J. R., Vázquez-Sánchez, D., and Cabo, M. L. (2012). Adherence kinetics, resistance to benzalkonium chloride and microscopic analysis of mixed biofilms formed by Listeria monocytogenes and Pseudomonas putida. Food Control 25, 202–210. doi: 10.1016/j.foodcont.2011.10.002
Silva-Angulo, A. B., Zanini, S. F., Rosenthal, A., Rodrigo, D., Klein, G., and Martinez, A. (2015). Comparative study of the effects of citral on the growth and injury of Listeria innocua and Listeria monocytogenes cells. PLoS One 10:e0114026. doi: 10.1371/journal.pone.0114026
Stiefel, P., Rosenberg, U., Schneider, J., Mauerhofer, S., Maniura-Weber, K., and Ren, Q. (2016). Is biofilm removal properly assessed? Comparison of different quantification methods in a 96-well plate system. Appl. Microbiol. Biotechnol. 100, 4135–4145. doi: 10.1007/s00253-016-7396-9
Keywords: Listeria innocua, biofilm assay, luciferase, bioluminescence, NanoLuc
Citation: Berlec A, Janež N, Sterniša M, Klančnik A and Sabotič J (2021) Expression of NanoLuc Luciferase in Listeria innocua for Development of Biofilm Assay. Front. Microbiol. 12:636421. doi: 10.3389/fmicb.2021.636421
Received: 01 December 2020; Accepted: 13 January 2021;
Published: 02 February 2021.
Edited by:
Anne Mai-Prochnow, The University of Sydney, AustraliaReviewed by:
Elaine C. P. De Martinis, University of São Paulo, BrazilCopyright © 2021 Berlec, Janež, Sterniša, Klančnik and Sabotič. This is an open-access article distributed under the terms of the Creative Commons Attribution License (CC BY). The use, distribution or reproduction in other forums is permitted, provided the original author(s) and the copyright owner(s) are credited and that the original publication in this journal is cited, in accordance with accepted academic practice. No use, distribution or reproduction is permitted which does not comply with these terms.
*Correspondence: Aleš Berlec, YWxlcy5iZXJsZWNAaWpzLnNp
Disclaimer: All claims expressed in this article are solely those of the authors and do not necessarily represent those of their affiliated organizations, or those of the publisher, the editors and the reviewers. Any product that may be evaluated in this article or claim that may be made by its manufacturer is not guaranteed or endorsed by the publisher.
Research integrity at Frontiers
Learn more about the work of our research integrity team to safeguard the quality of each article we publish.