- 1Laboratory of Veterinary Pharmacokinetics and Pharmacodynamics, College of Veterinary Medicine, Kyungpook National University, Daegu, South Korea
- 2Development and Reproductive Toxicology Research Group, Korea Institute of Toxicology, Daejeon, South Korea
Salmonella enterica serovar Typhimurium, an intracellular pathogen, evades the host immune response mechanisms to cause gastroenteritis in animals and humans. After invading the host cells, the bacteria proliferate in Salmonella-containing vacuole (SCV) and escapes from antimicrobial therapy. Moreover, Salmonella Typhimurium develops resistance to various antimicrobials including, fluoroquinolones. Treating intracellular bacteria and combating drug resistance is essential to limit the infection rate. One way of overcoming these challenges is through combination therapy. In this study, Pyrogallol (PG), a polyphenol, is combined with marbofloxacin (MAR) to investigate its effect on Salmonella Typhimurium invasion and intracellular survival inhibition. The Minimum inhibitory concentration (MIC) and minimum bactericidal concentration (MBC) of PG against Salmonella Typhimurium were 128 and 256 μg/mL, respectively. The lowest fractional inhibitory concentration (FIC) index for a combination of PG and MAR was 0.5. The gentamycin protection assay revealed that PG (30 μg/mL) alone and in combination with sub-MIC of MAR inhibited 72.75 and 76.18% of the invading bacteria in Caco-2 cells, respectively. Besides, the intracellular survival of Salmonella Typhimurium was reduced by 7.69 and 74.36% in treatment with PG alone and combined with sub-MIC of MAR, respectively, which was visualized by the confocal microscopy. PG has also shown to increase the intracellular accumulation of fluoroquinolone by 15.2 and 34.9% at 30 and 100 μg/mL concentration, respectively. Quantitative real-time PCR demonstrated PG suppressed the genetic expression of hilA, invF, sipB, and acrA by 14.6, 15.4, 13.6, and 36%, respectively. However, the downregulation of hilA, invF, sipB, and acrA increased to 80, 74.6, 78, and 70.1%, in combination with sub-MIC of MAR, respectively. Similarly, PG combined with MAR inhibited the expression of sdiA, srgE, and rck genes by 78.6, 62.8, and 61.8%, respectively. In conclusion, PG has shown antimicrobial activity against Salmonella Typhimurium alone and in combination with MAR. It also inhibited invasion and intracellular survival of the bacteria through downregulation of quorum sensing, invading virulence, and efflux pump genes. Hence, PG could be a potential antimicrobial candidate which could limit the intracellular survival and replication of Salmonella Typhimurium.
Introduction
Salmonella enterica subsp. enterica serovar Typhimurium, an intracellular Gram-negative bacterium, is a non-typhoidal Salmonella serotype known to cause diarrhea and intestinal inflammation in humans and animals (Laupland et al., 2010). Upon ingestion, salmonella adhere to epithelial lining of the ilium and colon to cause gastrointestinal infection (Ribet and Cossart, 2015). In the small intestine, the bacteria invade specialized epithelial cells called M cells. It triggers membrane ruffling and actin rearrangement which leads to bacterial internalization upon injecting its effector proteins (Patel and Galán, 2005). The T3SS-1 genes plays a significant role to invade the intestinal epithelial cells and mediate bacterial entry and their persistence in the host cells (Thiennimitr et al., 2012). Successful adherence and invasion of the host cell are essential to cause infections. Salmonella Typhimurium invades epithelial cells either through cytoskeletal rearrangement known as the “Trigger” mechanism or receptor-mediated entry “Zipper” mechanism (Velge et al., 2012). Internalization is facilitated by several Salmonella secreted effector proteins (Agbor and Mccormick, 2011). After internalization Salmonella resides in the Salmonella-containing vacuoles to shield from host innate immune responses (Haraga et al., 2008). Hence, inhibition of bacterial adhesion, invasion, and intracellular survival is critical in controlling infections. However, effective delivery of antimicrobial agents within the host cells is the main challenge (Kamaruzzaman et al., 2017). Hence, identifying natural compounds with a higher penetration capacity has significant importance (Liu et al., 2020).
Besides, Salmonella Typhimurium developed resistance against fluoroquinolones, including marbofloxacin (MAR), which is a widely used antibacterial agent against intracellular bacteria in veterinary medicine (de Jong et al., 2012). Antimicrobial resistance has become a common trend worldwide, and most of the bacteria have been developing resistance to the commonly utilized antibacterial agents (Varma et al., 2005). To worsen the situation, the introduction of newly invented antimicrobials into the market is declining. Hence, designing alternative methods to overcome these challenges is critical. One means of tackling the alarmingly overgrowing drug resistance is through a combination therapy of currently available antimicrobials, either with other existing antimicrobials or using natural compounds (Fischbach, 2011).
Polyphenols are phytochemicals found richly in natural, edible plants. They are known for their antioxidant properties, antibacterial activity, and prevention of non-communicable diseases like cancer, cardiovascular, and neurodegenerative diseases (Manach et al., 2004; Taguri et al., 2006). Furthermore, polyphenols inhibit angiogenesis, and aging, maintains blood pressure and sugar level, and have anti-inflammatory properties, regulate enzyme function, and stimulate cell receptors (Middleton et al., 2000; Pandey and Rizvi, 2009; Moyle et al., 2015). Recently, polyphenols like methyl gallate showed to prevent microbial adhesion, invasion, and intracellular survival of microbial agents through inhibition of biofilm formation and quorum sensing signals (Hossain et al., 2017; Birhanu et al., 2018).
Pyrogallol (benzene-1,2,3-triol, PG) is a hydroxylated polyphenol compound that contains three hydroxyl groups in the ortho position of a benzene ring. It is also present in various fruits and vegetables. PG is mostly used in pharmaceutical and pesticide manufacturing companies for medicinal purposes as a topical antipsoriatic (Ozturk Sarikaya, 2015). PG exhibits both prooxidant and antioxidant activity. The earlier role of PG involves generating reactive oxygen species and critical for its antimicrobial activity (Baruah et al., 2015; Mendes et al., 2015). Besides, PG acts through enzymatic inhibition of oxidized compounds (Mason and Bruce, 1987).
Previously PG showed to inhibit Helicobacter pylori urease (Xiao et al., 2010) and Vibrio harveyi quorum sensing (Ni et al., 2008). Its acethlycholinesterase inhibitory activity shows its importance in treating Alzheimer’s disease (Ozturk Sarikaya, 2015). Moreover, PG prevents the HeLa cells cytotoxicity induced by V. vulnificus and inhibits bacterial growth (Lim et al., 2016). Furthermore, it showed a synergistic activity when combined with norfloxacin and gentamicin against Staphylococcus aureus (Lima et al., 2016).
However, to our understanding, there are no reports on the effect of PG on bacterial intracellular survival and its antiinvasive mechanism. Hence, in this study, we evaluated the pharmacodynamics of PG and its inhibitory activity against Salmonella Typhimurium invasion and intracellular survival alone and in combination with MAR. Moreover, we have studied the mechanisms of bacterial invasion and intracellular survival inhibition.
Materials and Methods
Bacterial and Cell Culture Conditions
Two field strains of Salmonella enterica serovar Typhimurium, (KU325552 (S-2) and KU325565 (S-15), isolated from swine clinical infections as described earlier (Lee et al., 2017), in which one is susceptible while the other was intermediately resistant to MAR (Fluka, Germany) and ATCC 14028 strain were used. All the bacteria were cultured in Luria-Bertani (LB) broth or agar (Difco, BD, MD, United States) unless otherwise stated, at 37oC for various time lengths according to the purpose of the experiment. Two cell lines, RAW 264.7 and Caco-2 cells were used for the bacterial invasion and intracellular survival assays. RAW 264.7 cells were grown in RPMI 1640 medium (Sigma, United Kingdom) supplemented with 10% fetal bovine serum (FBS, Gibco, United States) and 1% penicillin-streptomycin (P/S) whereas, the Caco-2 cell was grown in minimum essential medium (MEM, Gibco, United States) supplemented with 1% nonessential amino acids (Sigma-Aldrich, MO, United States), 20% FBS, and 1% P/S and both cell lines were incubated at 37°C in a humidified atmosphere with 5% CO2.
Cell Viability Assay
The cell viability assay was performed using 3-(4,5-dimethyl-2-thiazolyl)-2,5-diphenyl-2H-tetrazolium bromide (MTT) assay. Confluent cells (105 cells/mL) were cultured onto a 96-well plate and incubated for 24 h at 37oC with 5% CO2. The medium was substituted with a new medium containing twofold dilutions of PG (Sigma, China) before incubated overnight at 37oC with 5% CO2. Finally, 0.45% of MTT reagent in cell culture media was substituted and incubated for 4 h before adding 100 μL of 100× DMSO. The plate was read at 570 nm after 5 min using Versamax (molecular devices, United States). The rate of cell viability was calculated using the following formula:
Minimum Inhibitory Concentration and Minimum Bactericidal Concentration Determination
The minimum inhibitory concentration (MIC) of MAR and PG was done using the micro-broth dilution method as described by the Clinical Laboratory and Standard Institute (CLSI) (CLSI, 2017). A two-fold dilution of MAR and PG in Muller Hinton broth II (MHBII, Difco, BD, United States) and RPMI medium were added to 105 CFU/mL of the three strains of S. Typhimurium. The bacteria were cultured for 24 h at 37oC aerobically and the results were read using a plate reader at 600 nm. To determine the minimum bactericidal concentration (MBC), 20 μL of the suspension from the microplate starting from the MIC onwards were taken and plated to LB agar. The plates were incubated at 37oC for 48 h to determine the possible slowly growing bacteria. The test was conducted three times in duplicate.
Fractional Inhibitory Concentration Determination
The fractional inhibitory concentration (FIC) of the combination of MAR with PG was conducted using a checkerboard method (Leber, 2016). A final concentration of 105 CFU/mL of the bacteria was added to the various fractional concentration of MAR and PG. The dilution was made in a 96-well plate and incubated aerobically overnight at 37oC. Finally, the results were read both visually and on a plate reader at 600 nm.
Time-Kill Assay
A time-kill assay was done as previously described with minor modifications (Sendi et al., 2015). An overnight grown Salmonella Typhimurium was inoculated into a 5 mL LB broth and incubated for 4 h at 37oC to obtain the logarithmic growing phase of bacteria. The bacteria were added to MHBII containing various concentrations of MAR and PG to make a final bacterial concentration of 105 CFU/mL. The bacteria were cultured at 37oC and sampled at 0, 0.5, 1, 2, 4, 8, 12, and 24 h and cultured on LB agar plates for 48 h after serially diluted. The results were read and recorded after a bacterial count.
Bacterial Invasion and Intracellular Inhibition Assay
For bacterial invasion inhibition experiments, the gentamicin protection assay was performed as previously described with minor modifications (Wu et al., 2014). Briefly, PG (30 μg/mL) alone or with sub-MIC of MAR (0.015 μg/mL for susceptible and 0.25 μg/mL for MAR resistant bacteria) was added to the fully confluent Caco-2 cells and RAW 264.7 cells (105 cells/mL) before incubating for 30 min. Salmonella Typhimurium (107 CFU/mL) was added and incubated for another 45 min after centrifuged at 500 g for 5 min. Gentamicin (100 μg/mL) was added and incubated for 30 min before cells were lysed by 0.1% Triton® x-100 (Sigma, United States) for 10 min. Cells were washed with PBS at least three-times in each step. The solution was serially diluted and cultured on a LB agar plate for a bacterial count after overnight incubation.
For intracellular killing experiments, RAW 264.7 cells (105 cells/mL) were grown on 24 well plates. The cells were infected with Salmonella Typhimurium (107 CFU/mL) and incubated for 1 h after centrifugation at 500 g for 5 min. Then the cells were washed, and PG (30 μg/mL) alone or combined with the sub-MIC of MAR were added and incubated for 1 h at 37°C and 5% CO2. Finally, the cells were treated with gentamicin (100 μg/mL) for 1 h and lysed in 0.1% Triton® x-100 before serially diluted and incubated on an agar plate overnight at 37oC for a bacterial count.
Confocal Microscopy
Caco-2 cells (105 cells/mL) were cultured on 12 mm glass coverslips in 24-well plates as described Johnson and Criss (2013). The cells were prepared and treated as mentioned above for the bacterial intracellular inhibition assay. The cells were rinsed gently in 0.1 M 3-(N-morpholino) propanesulfonic acid (MOPS), pH 7.2, containing 1 mM MgCl2 (MOPS/MgCl2). The rinsing solution from the cells were aspirated, and 0.5 mL Live/Dead Staining Solution containing 5 μM SYTO9, 30 μM propidium iodide (IP) (Invitrogen, United States), and 0.1% saponin in MOPS/MgCl2 were added. The cells incubated for 15 min at room temperature in the dark and rinsed with MOPS/MgCl2. The coverslips were inverted face down onto glass slides, and images were acquired using a fluorescence microscope (Carl Zeiss, LSM700, IL, United States) with excitation of 488 and 555 nm and emission range of 515–555 nm and 560–600 nm for SYTO9 and propidium iodide, respectively. The thicknesses of the optical sections of the confocal microscopy were 12.2 and 13.2 μm for PI and SYTO-9 fluorescence, respectively. Non-treated cells were used as a negative control. Comparison was made upon counting of green fluorescence.
Fluoroquinolone Accumulation in Intact Bacteria
The accumulation of fluoroquinolone in intact bacteria was determined as previously described (Vergalli et al., 2020) using ciprofloxacin due to its increased yield of fluorescent signal intensity. Salmonella Typhimurium was grown at 37°C in LB to mid-exponential phase. The bacterial suspension was centrifuged at 6,000 × g for 15 min and concentrated tenfold in 50 mM sodium phosphate buffer at pH 7 supplemented with 5 mM MgCl2 (NaPi–MgCl2 buffer) to obtain a density of 6 × 109 CFU/mL. In glass culture tubes, 2.4 ml of the bacterial suspension was incubated for 5 min at 37°C with ciprofloxacin at 10 μg/mL, or 100 μg/mL (final volume 3 ml), in the absence or in the presence of PG and a positive control, the efflux inhibitor PAβN (Phenylalanine-arginine β-naphthylamide) used at 40 μg/mL. Bacterial suspensions incubated without antibiotics was used as negative controls. Suspensions (800 μl) were then transferred to 1,100 μL of NaPi–MgCl2 buffer and centrifuged at 9,000 × g for 5 min at 4°C and the pelleted bacteria was collected. After centrifugation, pellets corresponding to 800 μl of bacterial suspensions were lysed with 500 μl of 0.1M Glycin-HCl pH 3 overnight at room temperature. After a centrifugation for 15 min at 9,000 × g at 4°C, 400 μl of lysates were mixed with 600 μl of Glycin-HCl buffer, and emission spectra were measured with a spectrofluorimeter (F-2500 Fluorescence Spectrophotometer, Hitachi, Japan). Excitation/emission range wavelengths (nm) used for detection of ciprofloxacin fluorescence signal with spectrofluorimetry was 275/435–450.
Total RNA Extraction and Virulence Gene Expression
For virulence gene expression, Salmonella Typhimurium was cultured in LB broth containing 3M of NaCl and incubated with different concentrations of PG and sub-MIC of MAR at 37oC for 13 h. The total RNA was extracted using Trizol® (Ambion®, life technologies, United States) reagent. The RNA purity and concentration were determined using Nanodrop measurement. RT-PCR premix (pioneer, Korea) was used to synthesize bacterial cDNA by adding random hexamers. Quantification of RNA was performed on CFX96 TouchTM real-time PCR detection systems (Biorad, United States) using IQTM SYBR® Green supermix for real-time PCR (Biorad, Singapore). The qRT-PCR condition was set for 95oC for 3 min and 40 cycles of 95oC for 10 s, 58oC for 15 s, and 72oC for 30 s for hilA, invF, sipB, and acrA genes. The primers used in the study are listed in Table 1.
Quorum Sensing Gene Inhibition
To determine the effect of PG on the genetic expression of quorum sensing genes of Salmonella Typhimurium (Li et al., 2014), N-Acyl homoserine lactone (AHL, 1 μmol/mL) was added to the LB broth containing bacteria and incubated with 30, 100 μg/mL of PG and in combination with sub-MIC of MAR for 8 h. Furanone at 10 μg/mL (Sigma, St. Louis, MO, United States) was used as a positive control for quorum sensing inhibition. Whereas bacteria exposed to AHL without treatment with PG and MAR was used as a negative control. The suspension was centrifuged, and the pellet was processed for RNA extraction using Trizol reagent as described above. The qRT-PCR was set at a denaturation temperature of 95oC for 30 s, followed by 40 cycles of 95oC for 5 s, 60oC for 30 s, and dissociation of 95oC for 15 s and 60oC for 30 s.
Prediction of Ligand-Protein Docking
The prediction of the binding and affinity of PG to the virulence and quorum sensing proteins of Salmonella Typhimurium was performed using iGEMDOCK (Version 2.1; NCTU, Hsinchu City, Taiwan) a graphical environment for recognizing pharmacological interactions and virtual screening (Yang and Chen, 2004). The chemical structure depiction for PG was retrieved from the PubChem database (Kim et al., 2019). Whereas the sequences of the bacterial virulence proteins were retrieved from the NCBI database. The protein structure was retrieved from PDB database (Berman et al., 2000; Burley et al., 2019) and predicted by RaptorX (Wang et al., 2017a,b, 2018; Xu, 2019; Xu and Wang, 2019). In addition, for the interactive visualization and analysis of molecular structures UCSF Chimera (Chimera, version 1.15, RBVI, San Francisco, CA, United States) was used (Pettersen et al., 2004).
Statistical Analysis
Graphpad prism 7 (GraphPad Software, La Jolla, CA, United States) was used to analyze the data and one-way analysis of variance (ANOVA) followed by Tukey’s multiple comparison test was used to compare between treatment groups and compute the p-value. P-value was considered significant if P < 0.05.
Results
To determine the effect of PG on mammalian cell growth, cell viability was investigated using the MTT reagent. PG at a concentration of 2,048 μg/mL did not show any significant cytotoxic activity on the viability of both Caco-2 and RAW 264.7 cells. The cell viability was more than 100% for concentrations less than 256 μg/mL (Figure 1).
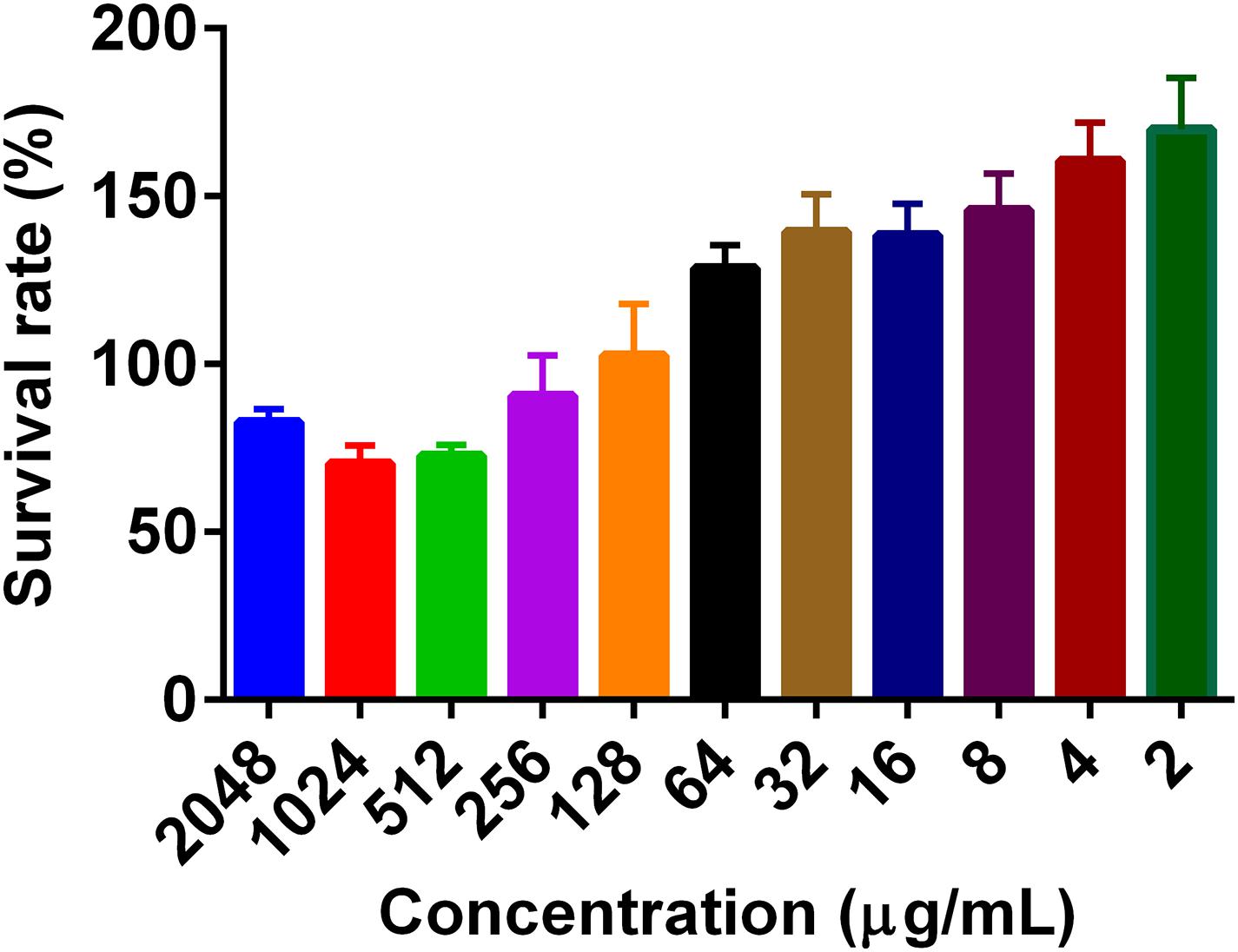
Figure 1. The effect of pyrogallol on the cell viability. The cells were treated with different concentrations of PG and incubated overnight, and the cell viability was determined using MTT reagent. Data is presented as Mean ± SEM.
The MIC and MBC of PG for all three strains were 128 and 256 μg/mL, respectively. The MIC of MAR against Salmonella Typhimurium ATTC 14028 and field isolate strain 2 (S-2) was 0.031 μg/mL, whereas it was 0.5 μg/mL for field isolate strain 15 (S-15). The MBC of MAR against ATTC 14028 and S-2 was 0.125 but 2 μg/mL for S-15. The log IC50 for MAR was −1.793, −0.5955, and −1.478, whereas it was 1.82, 1.459, and 1.511 for PG against ATCC 14028, S-15, and S-2 strains, respectively. The lowest FIC index results obtained for MAR in combination with PG in all the three strains were 0.5 (Table 2). Thus, for the consecutive experiments, we selected a concentration of 30 μg/mL of PG, which showed no antibacterial activity.
The time-kill assay was performed to detect the combined effect of sub_MIC of MAR with different concentrations of PG in a dynamic model. The test was conducted for 24 h. The sub-MIC of MAR in combination with 128 μg/mL of PG reduce Salmonella Typhimurium (ATCC 14028) CFU/mL by 2-log within 8 h. Whereas, the 2-log reduction for the S-2 strain was achieved after 4 h of incubation at all tested concentrations of PG except the 64 μg/mL. The 2-log reduction for the S-15 strain was observed after 12 h of incubation by the same concentration of PG and MAR (Figure 2). In strain ATCC 14028 and S-15, the combination of PG (256 μg/mL) and sub-MIC of MAR showed early killing of the bacteria in comparison with PG (256 μg/mL) used alone. However, PG (64 and 128 μg/mL) alone did not show to inhibit the growth of Salmonella Typhimurium strains tested.
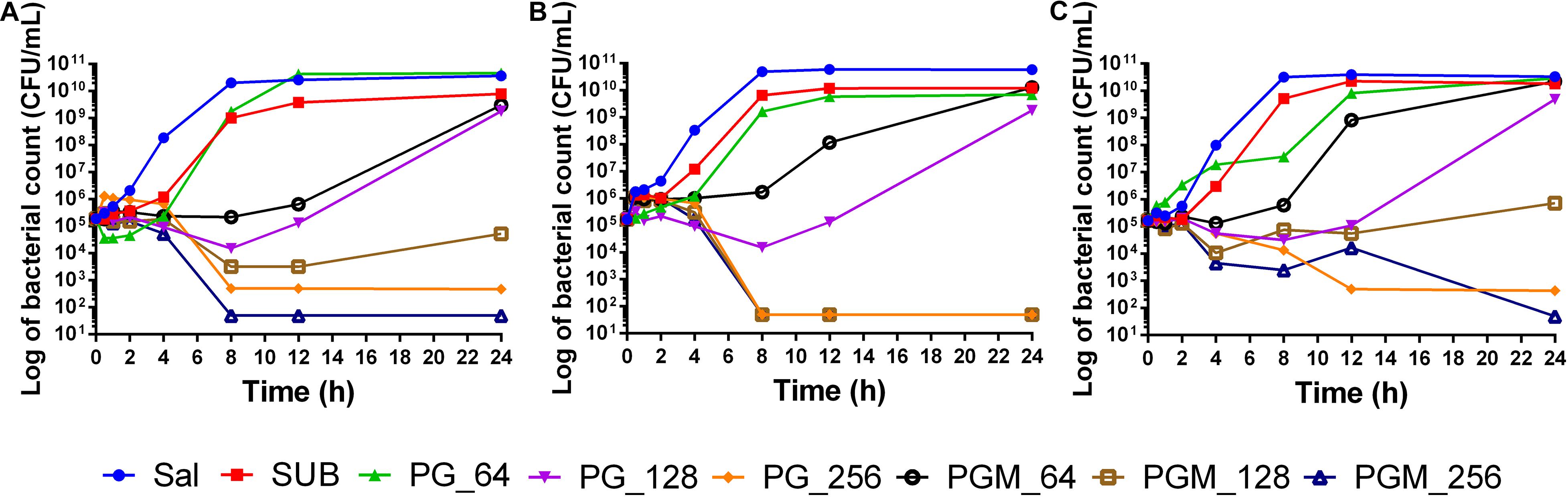
Figure 2. Time-Kill assay of pyrogallol alone and in combination with sub-MIC of MAR against different strains of Salmonella Typhimurium. (A) ATCC 14028 (B) susceptible field isolate (S-2), and (C) intermediately resistant field isolate (S-15). Sal: non-treated Salmonella Typhimurium control; SUB: treated with sub-MIC of marbofloxacin alone; PG-64: treated with only pyrogallol (64 μg/mL); PG-128: treated with only pyrogallol (128 μg/mL); PG-256: treated with only pyrogallol (256 μg/mL); PGM_64: treated with a combination of pyrogallol 64 μg/mL and sub-MIC of marbofloxacin; PGM_128: treated with a combination of pyrogallol 128 μg/mL and sub-MIC of marbofloxacin; PGM_256: treated with a combination of pyrogallol 256 μg/mL and sub-MIC of marbofloxacin.
For the bacterial invasion inhibition assay, PG at a concentration of 30 μg/mL alone and in combination with sub-MIC of MAR was used. PG (30 μg/mL) inhibited 72.75 and 44.8% of Salmonella Typhimurium invasion in Caco-2 and Raw 264.7 cells, respectively. Whereas, for the combination of PG with sub-MIC of MAR, the inhibition percentage was increased to 76.19 and 59.3% in Caco-2 and Raw 264.7 cells, respectively. A significant difference (P < 0.05) was observed in the inhibition of Salmonella Typhimurium invasion in both cell lines by PG alone and combination with MAR. However, no significant difference was observed between Caco-2 and Raw 264.7 cells (Figures 3A,B).
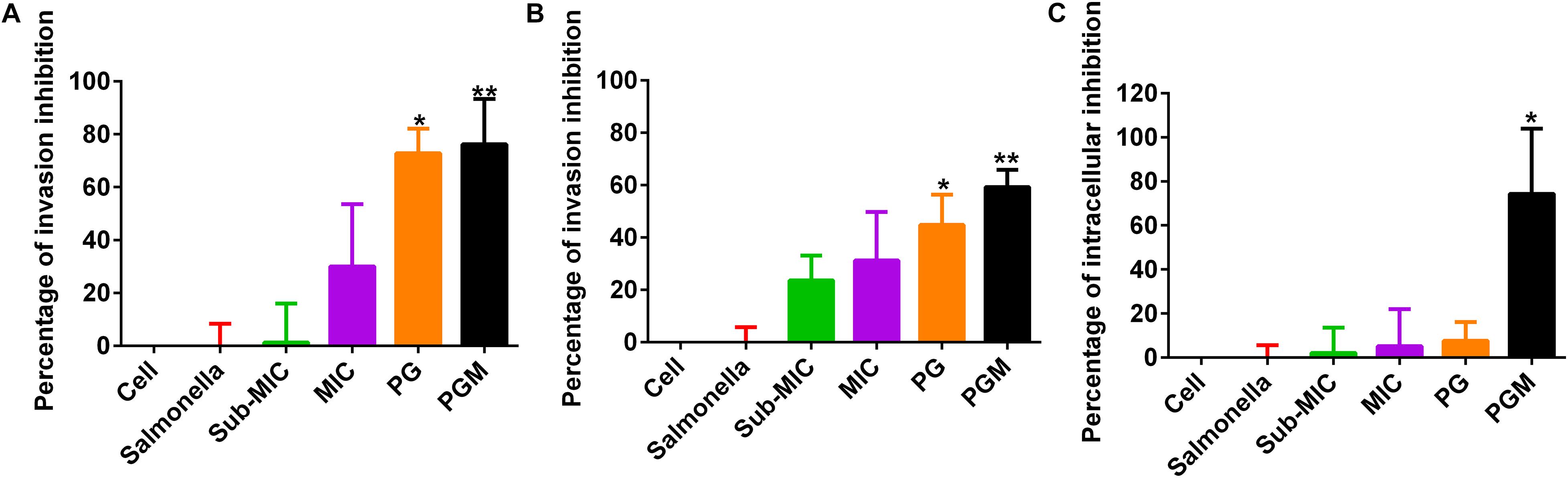
Figure 3. Percentage of inhibition of Salmonella Typhimurium invasion and intracellular survival by pyrogallol in Caco-2 and RAW 264.7 cells. (A) Inhibition of Salmonella Typhimurium invasion in Caco-2 cells; (B) inhibition of Salmonella Typhimurium invasion in RAW 264.7 cells and (C) Inhibition of intracellular survival of Salmonella Typhimurium by pyrogallol in RAW 246.7 cells. Cell – non-infected cells; Sal – infected with Salmonella Typhimurium but not treated; sub-MIC – infected with Salmonella Typhimurium and treated with sub-MIC of MAR alone; MIC – infected with Salmonella Typhimurium and treated with the MIC of MAR alone; PG – infected with Salmonella Typhimurium and treated with 30 μg/mL of PG; PGM – infected with Salmonella Typhimurium and treated with a combination of 30 μg/mL of PG and sub-MIC of MAR; *P < 0.05 and **P < 0.01.
Likewise, PG (30 μg/mL) inhibited 7.69% of intracellular Salmonella Typhimurium in RAW264.7 cells. Whereas in combination with sub-MIC of MAR, the suppression of the intracellular survival of Salmonella Typhimurium increased to 74.36%. A significant difference (P < 0.05) was indicated in cells treated with the combination of PG with sub-MIC of MAR (Figure 3C).
In compliance with the above findings, the confocal microscopy had also revealed the percentage of intracellular bacteria was significantly suppressed by the activity of PG alone and its combination with sub-MIC of MAR (Figure 4).
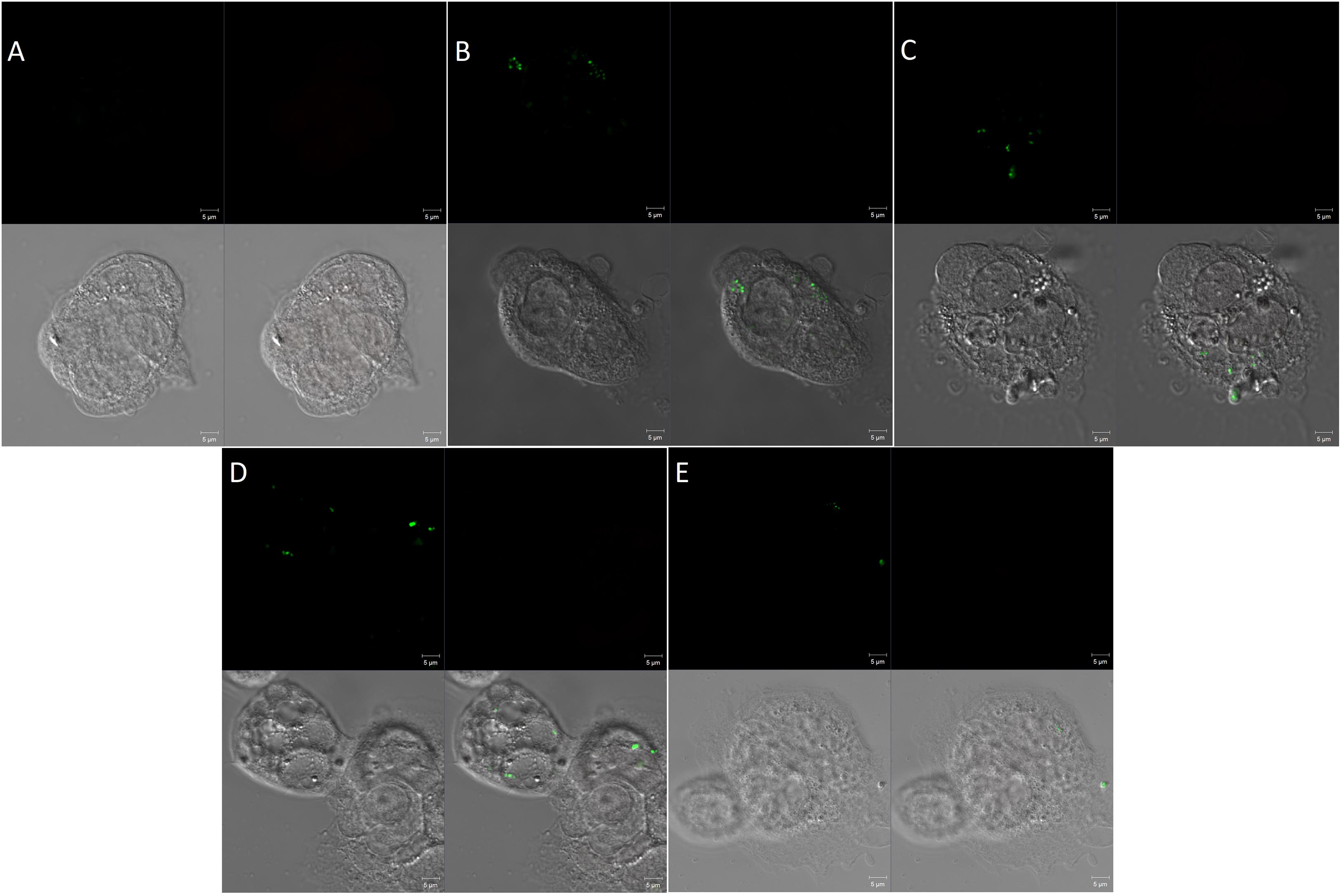
Figure 4. Confocal microscopy of invasion of Caco-2 cells by Salmonella Typhimurium (A) Uninfected cells, (B) cells infected with Salmonella Typhimurium but not treated, (C) Infected cells and treated with sub_MIC of MAR. (D) Cells infected and treated with pyrogallol (30 μg/mL) and (E) cells infected and treated with pyrogallol and sub-MIC of MAR. The top left panel is a SYTO-9 fluorescence; the top right panel is a propidium iodide fluorescence; the bottom left panel indicates cell structure without fluorescent; the bottom right panel shows a merged image. (Red, dead bacteria; Green, live bacteria; image: ×1,000 magnification).
The fluoroquinolone accumulation assay indicated that PG (30 μg/mL) increased the accumulation of ciprofloxacin by 15.2%. The accumulation was increased to 34.9% upon increasing the concentration of PG to 100 μg/mL which showed a significant difference in comparison with the negative control (Figure 5).
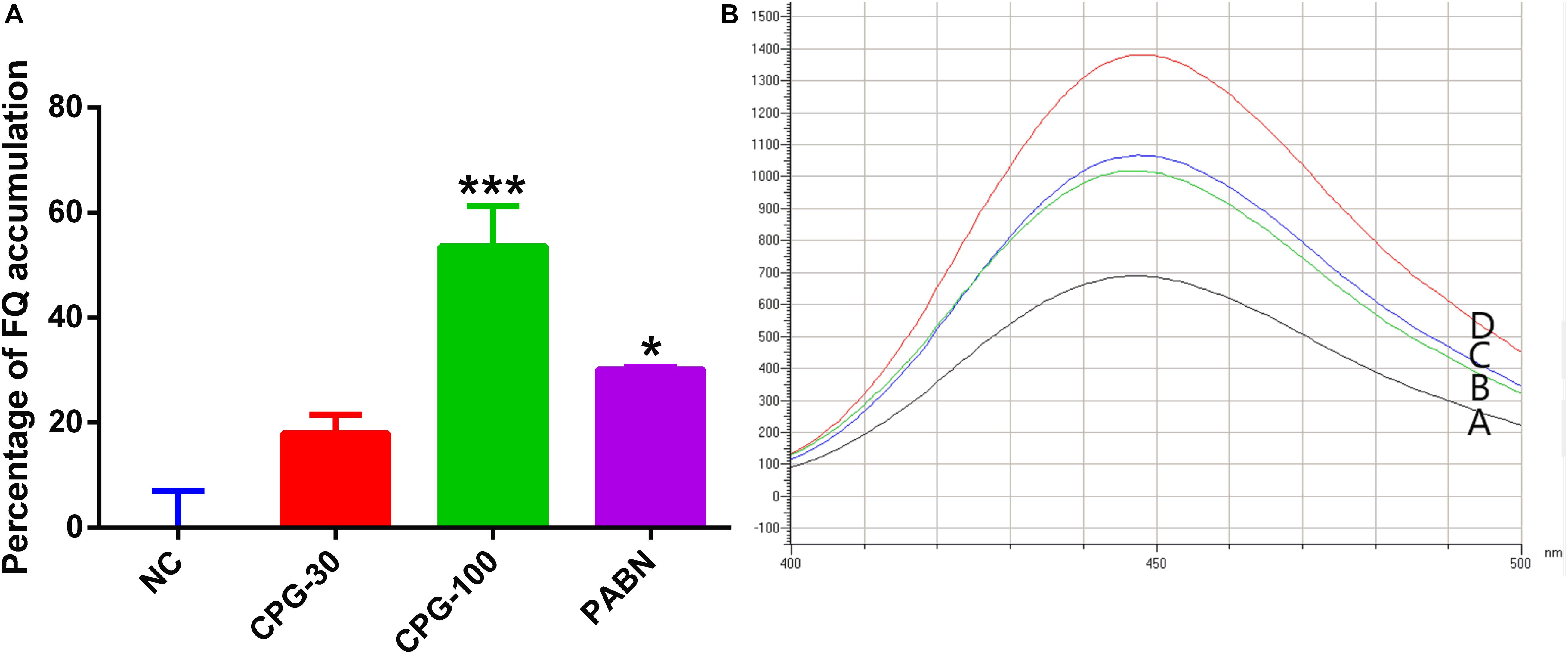
Figure 5. Accumulation of fluoroquinolone by pyrogallol. (A) The graph showed the increase in the accumulation of ciprofloxacin by various concentration of pyrogallol using PAβN as a positive control. (B) Fluorescence microscopy showed increased accumulation of fluoroquinolone by pyrogallol (A, Ciprofloxacin; B, Ciprofloxacin with PG 30 μg/mL; C, Ciprofloxacin with 100 μg/mL pyrogallol; D, Ciprofloxacin with 40 μg/mL of PAβN). NC – bacteria exposed to ciprofloxacin only; CPG-30 – bacteria exposed to ciprofloxacin and 30 μg/mL of pyrogallol; CPG-100 – bacteria exposed to ciprofloxacin and 100 μg/mL of pyrogallol; PAβN – bacteria exposed to ciprofloxacin and 40 μg/mL of PAβN. *p < 0.05.
The genetic expression level of different virulent invasion genes of Salmonella Typhimurium was evaluated using qRT-PCR. The result indicated that bacteria treated with PG (30 μg/mL) have a reduction of gene expression of hilA, invF, sipB, and acrA genes by 14.6, 15.4, 13.6, and 36%, respectively. The suppression of the gene expression level was dose dependent. At a higher concentration of PG (100 μg/mL), the inhibition was increased significantly to 59.3, 78.1, 46.7, and 63.8% for hilA, invF, sipB, and acrA genes, respectively. Whereas the combination of PG with sub-MIC of MAR showed a significant difference in suppressing the expression of hilA, invF, sipB, and acrA genes by 80, 74.6, 78, and 70.1%, respectively (Figures 6A–D).
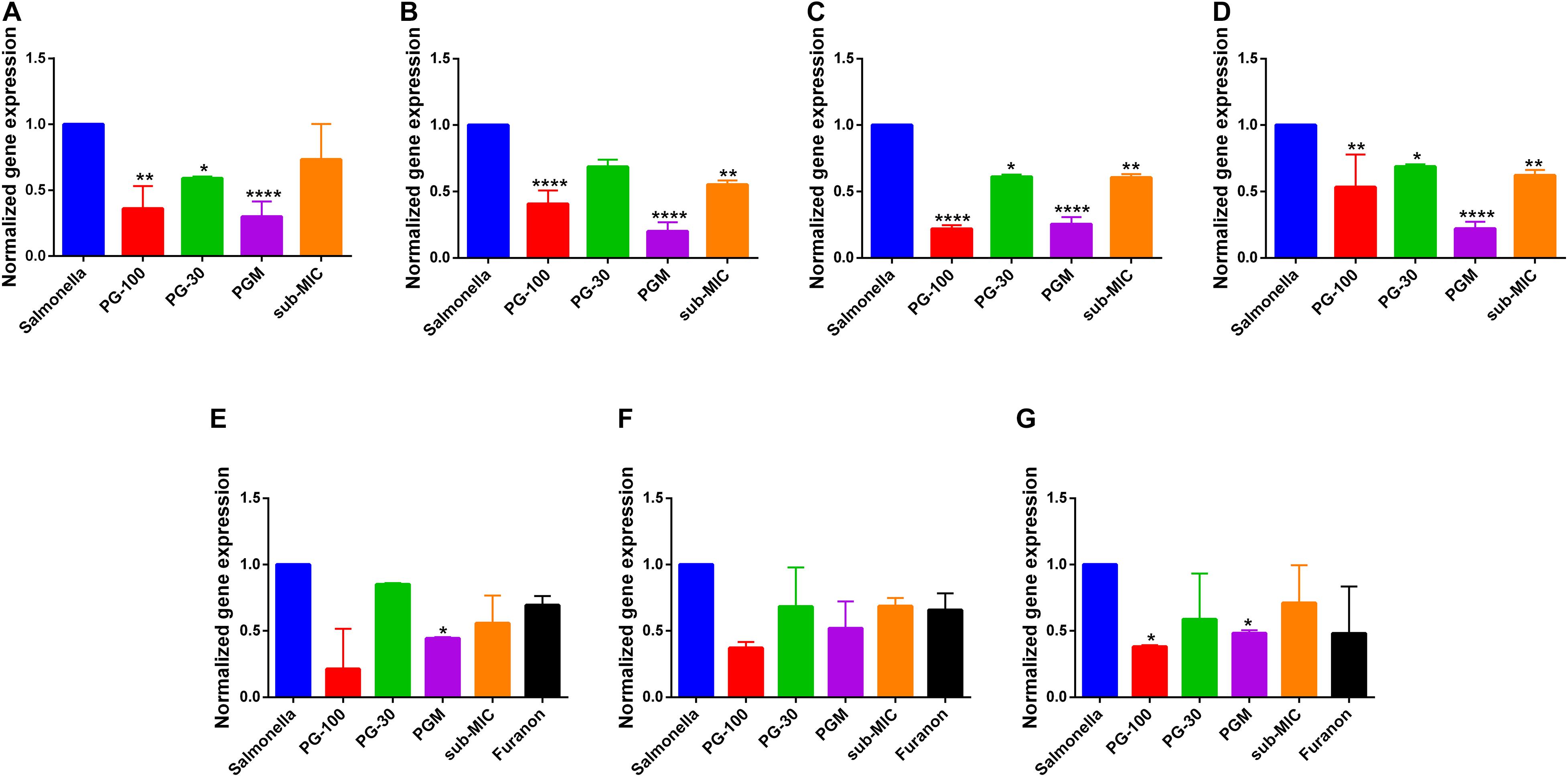
Figure 6. Effect of pyrogallol on the expression of Salmonella invasion and quorum sensing virulence genes. (A) acrA (B) hilA (C) invF, (D) sipB, (E) sdiA (F) srgE, and (G) rck gene. Salmonella – untreated culture; PG-100 – treated with 100 μg/mL of pyrogallol; PG-30 – treated with 30 μg/mL of pyrogallol; PGM – treated with a combination of 30 μg/mL of pyrogallol and sub-MIC of marbofloxacin; sub-MIC – treated with sub-MIC of marbofloxacin alone. For the quorum sensing gene expression assay all groups are treated with N-Acyl homoserine lactone (AHL, 1 μmol/mL). *P < 0.05; **p < 0.01; ****P < 0.001.
The effect of PG on the quorum sensing genes of Salmonella Typhimurium was evaluated using AHL as an inducer of quorum sensing signaling. The genetic expression levels of sdiA, srgE, and rck genes were reduced by 14.9, 31.6, and 41.2% upon treatment with PG (30 μg/mL), respectively. However, the inhibition was increased to 78.6, 62.8, and 61.8% for a higher concentration of PG (100 μg/mL), respectively. Whereas the combination of PG (30 μg/mL) with the sub-MIC of MAR inhibited the expression level of sdiA, srgE, and rck genes by 55.5, 48.1, and 46.7%, respectively (Figures 6E–G).
The binding energy affinity of PG to the suppressed proteins of acrA, hilA, invF, sdiA, sipB, and rck, proteins of Salmonella Typhimurium is presented in Table 3. The binding of PG with the amino acid residues showed a hydrogen bond linkage for of acrA, hilA, invF, sdiA, and rck, proteins (Figure 7).
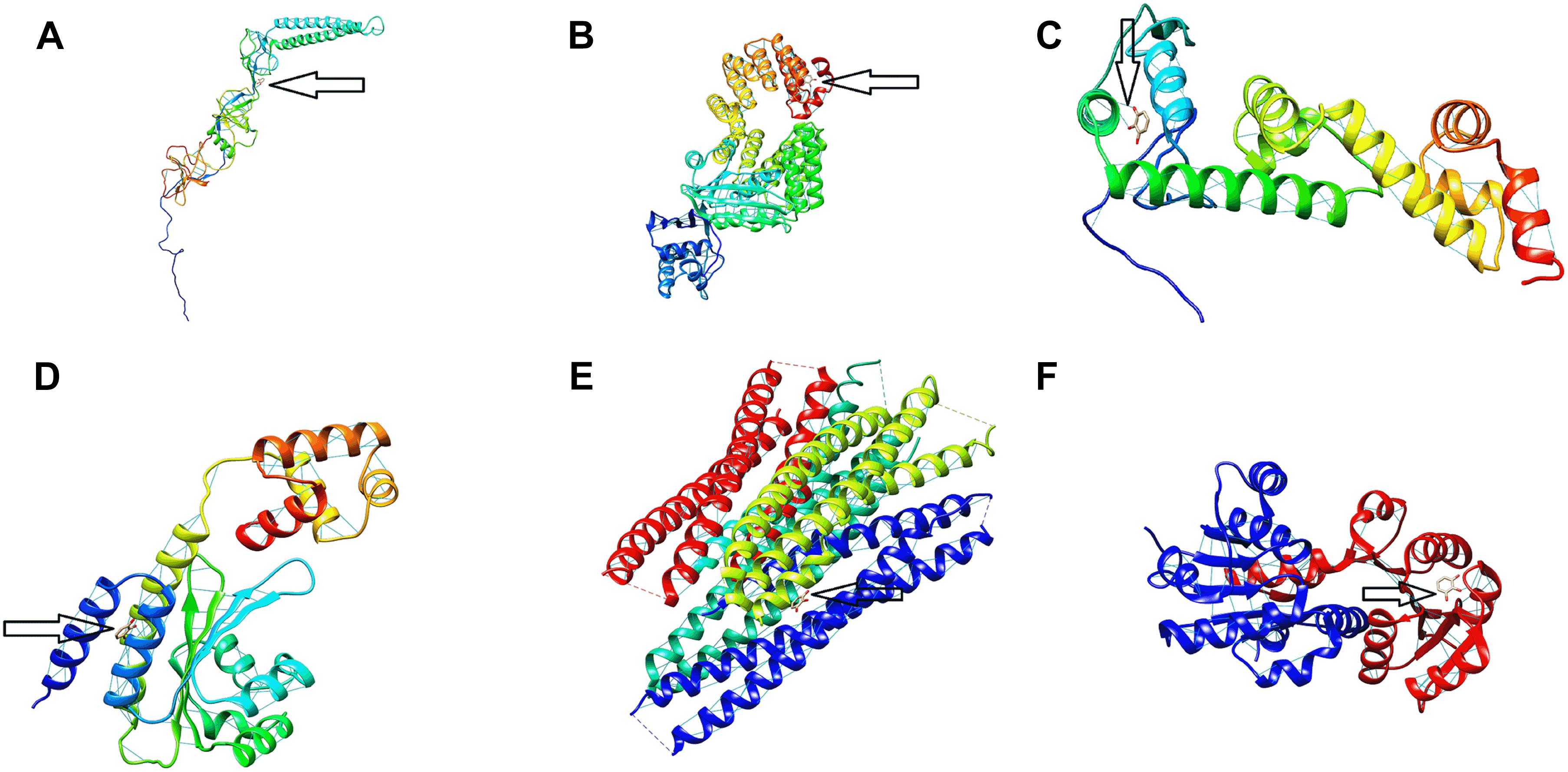
Figure 7. Protein binding site of pyrogallol on (A) acrA, (B) hilA (C) invF, (D) sdiA, (E) sipB, and (F) rck proteins of Salmonella Typhimurium. The arrow indicates the binding site of pyrogallol with the respective proteins and the hydrogen-bond formed between the protein and ligand.
Discussion
Treatment of intracellular bacteria is a major health challenge globally. Besides, these bacteria developed strategies to evade the host defense mechanism, invade the cells and reside intracellularly. Moreover, regardless of the possibility of developing new antimicrobials, microbial agents acquire resistance to various drugs (Hrvatin, 2017). Even though quinolones are referred to as the therapeutic options against intracellular pathogens, multiple bacteria are developing resistance against these conserved groups of antimicrobial agents (Van Bambeke et al., 2005). Similarly, Salmonella Typhimurium developed resistance against fluoroquinolones (de Jong et al., 2012). Hence, searching for an active compound that targets bacterial invasion and intracellular survival has paramount significance. In consequence, combination therapies are emerging as an alternative for the treatment of intracellular residing and multidrug-resistant microbes (Worthington and Melander, 2013).
Polyphenols are characterized by the presence of multiple hydroxyl groups in their benzene ring and are known for their antioxidant properties and modulation of enzymatic and cell receptors (Middleton et al., 2000). PG, a polyphenol, possesses antioxidant, antimicrobial activity, quorum sensing inhibition, and suppression of cytotoxicity properties (Ni et al., 2008; Baruah et al., 2015; Mendes et al., 2015). Therefore, in this study, we discovered the effect of PG alone or in combination with MAR in the invasion and intracellular survival of Salmonella Typhimurium isolated from pigs.
The determination of cytotoxicity is critical in detecting the potential toxic effect of a given compound in mammalian cells. In our findings, PG did not show any cytotoxic activity against both Caco-2 and Raw 264.7 cells even at a more significant concentration. In agreement with this, PG also did not show any cytotoxicity on the growth of HeLa cells (Lim et al., 2016).
The anti-infective activity of polyphenols has been described in various bacterial and fungal agents (Yanagawa et al., 2003; Friedman et al., 2006). They have indicated strong inhibitory activity against both Gram-negative and positive bacteria. In this study, we have indicated the antibacterial activity of PG against two field strains of Salmonella Typhimurium isolated from a clinical infection of pigs and ATCC control strains. In all the cases, PG exhibited similar antibacterial activity against Salmonella Typhimurium isolates at the same concentration. In the same way, PG has shown antibacterial activity against Pseudomonas putida, P. pyocyanea, Corynebacterium xerosis, Vibrio parahaemolyticus, V. vulnificus, and Staphylococcus aureus (Kocaçalişkan et al., 2006; Lim et al., 2016; Lima et al., 2016; Tinh et al., 2016). This indicated the likelihood of PG in the making of a novel antibacterial agent against infectious agents (Lim et al., 2016).
Moreover, in combination with the sub-MIC of MAR, which remains a widely used drug in veterinary medicine, PG resulted in the most substantial effect and presented self-induced synergism. Our findings agree with previous reports that PG has shown a synergistic effect with norfloxacin and gentamicin against S. aureus (Lima et al., 2016). Currently, there is a shortage of newly discovered antibiotics against multidrug-resistant bacteria and to worsen the situation, bacteria, particularly Gram-negative bacteria, develop resistance to most of the currently available antimicrobials (Lee et al., 2009; Freire-Moran et al., 2011). Thus, to alleviate this challenge, a combination of antimicrobial agents with other antimicrobials or other compounds is critical (Worthington and Melander, 2013). The potentiation effect of PG delivers a significant contribution to overcoming antimicrobial resistance. Hence, the antimicrobial activity observed by PG could have a significant contribution to reducing drug resistance and become an alternative to the currently available drugs (Worthington and Melander, 2013).
For successful treatment of intracellular bacteria, once the antimicrobial agents traverse the host cell membrane, they must be maintained and amass at adequate concentrations for a specified time (Kamaruzzaman et al., 2017). The accumulation of a drug is dependent on the suppression or expression of acrAB (Vergalli et al., 2017). PG increased the accumulation of fluoroquinolone in intact Salmonella Typhimurium cells. The presence of a higher concentration of fluoroquinolone suggests the inhibition of an active efflux pump (Okusu et al., 1996). This could be due to the downregulation of an efflux inhibitor acrA genes by PG. This indeed will increase the effectiveness of the drug in killing intracellular bacteria.
In addition to its antibacterial activity, the in vitro cell culture experiments demonstrated the potential of PG to inhibit Salmonella invasion and its intracellular survival. At the lowest concentration (30 μg/mL), four times less than its MIC, specifically in combination with sub-MIC of MAR, PG inhibited about 70% of Salmonella Typhimurium invasion in epithelial cells and intracellular survival in the macrophages. Methyl gallate, which is a polyphenol also inhibited bacterial adhesion, invasion and intracellular survival (Birhanu et al., 2018). This also agrees with the works of Tsou et al. (2016) who described the inhibition of Salmonella Typhimurium invasion by flavonoids like baicalein and quercetin. This inhibition targeted the SPI-1 T3SS substrates of the bacteria. This more considerably extends the role of PG in the production of a potent antimicrobial agent.
It is indicated that PG has downregulated the genetic expression of hilA and invF genes, critical for invading host cells and entering their cytoplasm. Salmonella Typhimurium activates the SPI-1 T3SS for entering the intestinal epithelium cells. HilA is the central regulator of invasion of epithelial cells and a transcriptional activator of SPI-1 genes including invF (Penheiter et al., 1997; Ellis et al., 2017). The most common mechanisms of inhibition by polyphenols are downregulating the genetic expression of virulence factors, quorum sensing signal inhibition, biofilm formation, and decreasing bacterial swarm motility (Kang-Mu et al., 2009; Daglia, 2012; Hossain et al., 2017; Birhanu et al., 2018). InvF is essential for the expression of the SPI-1 gene, which encodes effector proteins and their translocation into the cytosol (Darwin and Miller, 2000). Hence, the downregulation of these genes by PG could suppress the activity of other SPI-1 genes of Salmonella Typhimurium involved in cell invasion and intracellular survival and leads to the killing of the bacteria.
Furthermore, PG suppressed the expression of the sipB gene, which is critical for encoding and translocating other SPI-1 T3SS Salmonella Typhimurium secreted effector proteins and is essential for mammalian cell invasion (Hayward et al., 2000). Furthermore, sipB expedite adherance of Salmonella Typhimurium to target host cells (Lara-Tejero and Galán, 2009). Hence, the downregulation of sipB by PG might play a significant role in decreasing the invasion and intracellular bacteria.
PG also suppressed the level of expression of Salmonella Typhimurium acrA gene which is a resistance nodulation cell division gene (Guérin et al., 2016). It encodes for a membrane fusion protein, which is an efflux pump responsible for decreasing intracellular accumulation of drugs and results in antimicrobial resistance (Nishino et al., 2006; Yamasaki et al., 2011). The downregulation of acrA by PG will further confirm its paramount importance in preventing antimicrobial resistance and could be considered as an efflux pump inhibitor.
Furthermore, PG suppressed the quorum sensing related genes of Salmonella. SdiA, a LuxR homolog, is a quorum-sensing signal detector and regulator in Salmonella Typhimurium and activates srgE and rck genes (Dyszel et al., 2010; Li et al., 2014). Rck confers adhesiveness and invasiveness to intestinal epithelial cells (Cirillo et al., 1996; Michael et al., 2001). In addition, rck mediates the zipper-like internalization of Salmonella into the host cells (Rosselin et al., 2010). This further signifies the potential of PG in inhibiting bacterial invasion and combat clinical infections.
The decreased energy value of the protein-ligand interaction showed the high binding affinity of PG to the virulence and quorum sensing proteins of Salmonella Typhimurium. Although PG did not show to totally block the expression of virulence genes, it could have the potential to bind the remaining translated Salmonella effector proteins. This also indicates the likely chance of interfering with their activity and limiting the pathogenicity of the bacteria. Which further inhibits its invasion and intracellular survival.
Conclusion
In conclusion, in this study, we have indicated the antimicrobial and anti-invasive activity of PG against Salmonella Typhimurium in vitro study. PG is effective in killing intracellular bacteria alone and in combination with MAR. Furthermore, it increased the accumulation of fluoroquinolones. PG suppresses the virulence and quorum sensing genes of Salmonella Typhimurium, which are critical for invasion and intracellular survival of the bacteria and subsequently establish infection in the host cells. PG showed a self-induced potentiation effect to the most conserved drug of veterinary importance, MAR, in which certain bacterial agents are currently developing resistance against it. This suggests PG could be a potential efflux pump inhibitor and a candidate as an antimicrobial agent to prevent bacterial invasion, its intracellular survival, and antimicrobial resistance. However, further in vivo pharmacokinetic and pharmacodynamic studies should be conducted for comprehensive understanding before its preclinical trial.
Data Availability Statement
The original contributions presented in the study are included in the article/supplementary material, further inquiries can be directed to the corresponding author/s.
Author Contributions
BB designed and carried out the experiment, analyzed the results, and wrote the manuscript. E-BL analyzed the results and reviewed the manuscript. S-JL and S-CP designed the study and revised the manuscript. All authors read and approved the final draft.
Funding
This research is funded in part by the National Research Foundation of Korea (NRF) through the Ministry of Education (2019R1A2C2006277) and in part by a grant (Z-1543081-2020-22-02) from the Animal and Plant Quarantine Agency, Ministry of Agriculture, Food and Rural Affairs, Republic of Korea. The funders had no role in study design, data collection and interpretation, or the decision to submit the work for publication.
Conflict of Interest
The authors declare that the research was conducted in the absence of any commercial or financial relationships that could be construed as a potential conflict of interest.
References
Agbor, T. A., and Mccormick, B. A. (2011). Salmonella effectors: Important players modulating host cell function during infection. Cell. Microbiol. 13, 1858–1869. doi: 10.1111/j.1462-5822.2011.01701.x
Baruah, K., Duy Phong, H. P. P., Norouzitallab, P., Defoirdt, T., and Bossier, P. (2015). The gnotobiotic brine shrimp (Artemia franciscana) model system reveals that the phenolic compound pyrogallol protects against infection through its prooxidant activity. Free Radic. Biol. Med. 89, 593–601. doi: 10.1016/j.freeradbiomed.2015.10.397
Berman, H. M., Westbrook, J., Feng, Z., Gilliland, G., Bhat, T. N., Weissig, H., et al. (2000). The protein data bank. Nucleic Acids Res. 28, 235–242.
Birhanu, B. T., Park, N. H., Lee, S. J., Hossain, M. A., and Park, S. C. (2018). Inhibition of Salmonella Typhimurium adhesion, invasion, and intracellular survival via treatment with methyl gallate alone and in combination with marbofloxacin. Vet Res. 49:101.
Burley, S. K., Berman, H. M., Bhikadiya, C., Bi, C., Chen, L., Di Costanzo, L., et al. (2019). RCSB Protein Data Bank: Biological macromolecular structures enabling research and education in fundamental biology, biomedicine, biotechnology and energy. Nucleic Acids Res. 47, D464–D474.
Cirillo, D. M., Heffernan, E. J., Wu, L., Harwood, J., Fierer, J., and Guiney, D. G. (1996). Identification of a domain in Rck, a product of the Salmonella typhimurium virulence plasmid, required for both serum resistance and cell invasion. Infect. Immun. 64, 2019–2023. doi: 10.1128/iai.64.6.2019-2023.1996
CLSI (2017). M100-S23 Performance Standards for Antimicrobial Susceptibility Testing; Twenty-Third Informational Supplement An informational supplement for global application developed through the Clinical and Laboratory Standards Institute consensus process, 27 Edn, eds J. Patel, M. Weinstein, E. George, S. Jenkins, J. Lewis, and B. Limbago (Wayne, PA: Clinical and Laboratory Standards Institute).
Daglia, M. (2012). Polyphenols as antimicrobial agents. Curr. Opin. Biotechnol. 23, 174–181. doi: 10.1016/j.copbio.2011.08.007
Darwin, K. H., and Miller, V. L. (2000). The putative invasion protein chaperone SicA acts together with InvF to activate the expression of Salmonella typhimurium virulence genes. Mol. Microbiol. 35, 949–960. doi: 10.1046/j.1365-2958.2000.01772.x
de Jong, A., Stephan, B., and Silley, P. (2012). Fluoroquinolone resistance of Escherichia coli and Salmonella from healthy livestock and poultry in the EU. J. Appl. Microbiol. 112, 239–245. doi: 10.1111/j.1365-2672.2011.05193.x
Dyszel, J. L., Smith, J. N., Lucas, D. E., Soares, J. A., Swearingen, M. C., Vross, M. A., et al. (2010). Salmonella enterica serovar typhimurium can detect acyl homoserine lactone production by Yersinia enterocolitica in mice. J. Bacteriol. 192, 29–37. doi: 10.1128/jb.01139-09
Ellis, M. J., Trussler, R. S., Charles, O., and Haniford, D. B. (2017). A transposon-derived small RNA regulates gene expression in Salmonella Typhimurium. Nucleic Acids Res. 45, 5470–5486. doi: 10.1093/nar/gkx094
Fischbach, M. A. (2011). Combination therapies for combating antimicrobial resistance. Curr. Opin. Microbiol. 14, 519–523. doi: 10.1016/j.mib.2011.08.003
Freire-Moran, L., Aronsson, B., Manz, C., Gyssens, I. C., So, A. D., Monnet, D. L., et al. (2011). Critical shortage of new antibiotics in development against multidrug-resistant bacteria – Time to react is now. Drug Resist Updat. 2011, 118–124. doi: 10.1016/j.drup.2011.02.003
Friedman, M., Henika, P. R., Levin, C. E., Mandrell, R. E., and Kozukue, N. (2006). Antimicrobial activities of tea catechins and theaflavins and tea extracts against Bacillus cereus. J. Food Protect. 2006, 354–361. doi: 10.4315/0362-028x-69.2.354
Guérin, F., Lallement, C., Isnard, C., Dhalluin, A., Cattoir, V., and Giard, J. C. (2016). Landscape of resistance-nodulation-cell division (RND)-type efflux pumps in Enterobacter cloacae complex. Antimicrob Agents Chemother. 60, 2373–2382. doi: 10.1128/aac.02840-15
Haraga, A., Ohlson, M. B., and Miller, S. I. (2008). Salmonellae interplay with host cells. Nat. Rev. Microbiol. 6, 53–66.
Hayward, R. D., McGhie, E. J., and Koronakis, V. (2000). Membrane fusion activity of purified SipB, a Salmonella surface protein essential for mammalian cell invasion. Mol. Microbiol. 37, 727–739. doi: 10.1046/j.1365-2958.2000.02027.x
Hossain, M. A., Lee, S. J., Park, N. H., Mechesso, A. F., Birhanu, B. T., Kang, J., et al. (2017). Impact of phenolic compounds in the acyl homoserine lactone-mediated quorum sensing regulatory pathways. Sci. Rep. 7, 1–16.
Hrvatin, V. (2017). Combating antibiotic resistance: New drugs or alternative therapies? CMAJ? 189:E1199. doi: 10.1002/9783527622931.ch1
Johnson, M. B., and Criss, A. K. (2013). Fluorescence microscopy methods for determining the viability of bacteria in association with mammalian cells. J. Vis. Exp. 50729. doi: 10.3791/50729
Kamaruzzaman, N. F., Kendall, S., and Good, L. (2017). Targeting the hard to reach: challenges and novel strategies in the treatment of intracellular bacterial infections. Br. J. Pharmacol. 174, 2225–2236. doi: 10.1111/bph.13664
Kang-Mu, L. E. E., Wan-Seok, K. I. M., Jeesun, L. I. M., Sunyoung, N. A. M., Youn, M. I. N., Seong-Won, N. A. M., et al. (2009). Antipathogenic properties of green tea polyphenol epigallocatechin gallate at concentrations below the MIC against enterohemorrhagic escherichia coli O157:H7. J. Food Prot. 72, 325–331. doi: 10.4315/0362-028x-72.2.325
Kim, S., Chen, J., Cheng, T., Gindulyte, A., He, J., He, S., et al. (2019). PubChem 2019 update: Improved access to chemical data. Nucleic Acids Res. 47, D1102–D1109.
Kocaçalişkan, I., Talan, I., and Terzi, I. (2006). Antimicrobial activity of catechol and pyrogallol as allelochemicals. Zeitschr. Naturf. Sect. C J. Biosci. 61, 639–642. doi: 10.1515/znc-2006-9-1004
Lara-Tejero, M., and Galán, J. E. (2009). Salmonella enterica serovar typhimurium pathogenicity island 1-encoded type III secretion system translocases mediate intimate attachment to nonphagocytic cells. Infect Immun. 77, 2635–2642. doi: 10.1128/iai.00077-09
Laupland, K. B., Schønheyder, H. C., Kennedy, K. J., Lyytikäinen, O., Valiquette, L., Galbraith, J., et al. (2010). Salmonella enterica bacteraemia: a multi-national population-based cohort study. BMC Infect Dis. 10:95.
Leber, A. L. (2016). Clinical Microbiology Procedures Handbook, 4 Edn, ed. A. L. Leber (Columbus, OH: ASM Press).
Lee, J. H., Jeong, S. H., Cha, S.-S., and Lee, S. H. (2009). New Disturbing trend in antimicrobial resistance of gram-negative pathogens. PLoS Pathog. 5:e1000221. doi: 10.1371/journal.ppat.1000221
Lee, S. J., Park, N. H., Mechesso, A. F., Lee, K. J., and Park, S. C. (2017). The phenotypic and molecular resistance induced by a single-exposure to sub-mutant prevention concentration of marbofloxacin in Salmonella typhimurium isolates from swine. Vet. Microbiol. 207, 29–35. doi: 10.1016/j.vetmic.2017.05.026
Li, G., Yan, C., Xu, Y., Feng, Y., Wu, Q., Lv, X., et al. (2014). Punicalagin inhibits Salmonella virulence factors and has anti-quorum-sensing potential. Appl. Environ. Microbiol. 80, 6204–6211. doi: 10.1128/aem.01458-14
Lim, J. Y., Kim, C.-M., Rhee, J. H., and Kim, Y. R. (2016). Effects of pyrogallol on growth and cytotoxicity of wild-type and katg mutant strains of vibrio vulnificus. PLoS One 11:e0167699. doi: 10.1371/journal.pone.0167699
Lima, V. N., Oliveira-Tintino, C. D. M., Santos, E. S., Morais, L. P., Tintino, S. R., Freitas, T. S., et al. (2016). Antimicrobial and enhancement of the antibiotic activity by phenolic compounds: Gallic acid, caffeic acid and pyrogallol. Microb. Pathog. 99, 56–61. doi: 10.1016/j.micpath.2016.08.004
Liu, Y., Jia, Y., Yang, K., and Wang, Z. (2020). Heterogeneous strategies to eliminate intracellular bacterial pathogens. Front. Microbiol. 11:563.
Manach, C., Scalbert, A., Morand, C., Rémésy, C., and Jiménez, L. (2004). Polyphenols: food sources and bioavailability. Am. J. Clin. Nutr. 79, 727–747. doi: 10.1093/ajcn/79.5.727
Mason, T. L., and Bruce, P. W. (1987). Inactivation of red beet β-glucan synthase by native and oxidized phenolic compounds. Phytochemistry 26, 2197–2202. doi: 10.1016/s0031-9422(00)84683-x
Mendes, V., Vilaça, R., De Freitas, V., Ferreira, P. M., Mateus, N., and Costa, V. (2015). Effect of myricetin, pyrogallol, and phloroglucinol on yeast resistance to oxidative stress. Oxid Med. Cell Longev. 2015:782504.
Michael, B., Smith, J. N., Swift, S., Heffron, F., and Ahmer, B. M. M. (2001). SdiA of Salmonella enterica is a LuxR homolog that detects mixed microbial communities. J. Bacteriol. 183, 5733–5742. doi: 10.1128/jb.183.19.5733-5742.2001
Middleton, E., Kandaswami, C., and Theoharides, T. (2000). The effects of plant flavonoids on mammalian cells: implications for inflammation, heart disease, and cancer. Pharmacol. Rev. 52, 673–751.
Moyle, C. W. A., Cerezo, A. B., Winterbone, M. S., Hollands, W. J., Alexeev, Y., Needs, P. W., et al. (2015). Potent inhibition of VEGFR-2 activation by tight binding of green tea epigallocatechin gallate and apple procyanidins to VEGF: Relevance to angiogenesis. Mol. Nutr. Food Res. 59, 401–412. doi: 10.1002/mnfr.201400478
Ni, N., Choudhary, G., Li, M., and Wang, B. (2008). Pyrogallol and its analogs can antagonize bacterial quorum sensing in Vibrio harveyi. Bioorganic. Med. Chem. Lett. 18, 1567–1572. doi: 10.1016/j.bmcl.2008.01.081
Nishino, K., Latifi, T., and Groisman, E. A. (2006). Virulence and drug resistance roles of multidrug efflux systems of Salmonella enterica serovar typhimurium. Mol. Microbiol. 59, 126–141. doi: 10.1111/j.1365-2958.2005.04940.x
Okusu, H., Ma, D., and Nikaido, H. (1996). AcrAB efflux pump plays a major role in the antibiotic resistance phenotype of Escherichia coli multiple-antibiotic-resistance (Mar) mutants. J. Bacteriol. 178, 306–308. doi: 10.1128/jb.178.1.306-308.1996
Ozturk Sarikaya, S. B. (2015). Acethylcholinesterase inhibitory potential and antioxidant properties of pyrogallol. J. Enzyme Inhib. Med. Chem. 30, 761–766. doi: 10.3109/14756366.2014.965700
Pandey, K. B., and Rizvi, S. I. (2009). Plant polyphenols as dietary antioxidants in human health and disease. Oxidat. Med. Cell. Long. 2, 270–278. doi: 10.4161/oxim.2.5.9498
Patel, J. C., and Galán, J. E. (2005). Manipulation of the host actin cytoskeleton by Salmonella – All in the name of entry. Curr. Opin. Microbiol. 8, 10–15. doi: 10.1016/j.mib.2004.09.001
Penheiter, K. L., Mathur, N., Giles, D., Fahlen, T., and Jones, B. D. (1997). Non-invasive Salmonella typhimurium mutants are avirulent because of an inability to enter and destroy M cells of ileal Peyer’s patches. Mol. Microbiol. 24, 697–709. doi: 10.1046/j.1365-2958.1997.3741745.x
Pettersen, E. F., Goddard, T. D., Huang, C. C., Couch, G. S., Greenblatt, D. M., Meng, E. C., et al. (2004). UCSF Chimera – A visualization system for exploratory research and analysis. J. Comput. Chem. 25, 1605–1612. doi: 10.1002/jcc.20084
Ribet, D., and Cossart, P. (2015). How bacterial pathogens colonize their hosts and invade deeper tissues. Microbes Infect. 17, 173–183. doi: 10.1016/j.micinf.2015.01.004
Rosselin, M., Virlogeux-Payant, I., Roy, C., Bottreau, E., Sizaret, P. Y., Mijouin, L., et al. (2010). Rck of Salmonella enterica, subspecies enterica serovar Enteritidis, mediates Zipper-like internalization. Cell Res. 20, 647–664. doi: 10.1038/cr.2010.45
Sendi, P., Ruppen, C., and Sendi, P. (2015). Time kill assays for Streptococcus agalactiae and synergy testing. Protoc. Exch. 28, 126.
Taguri, T., Tanaka, T., and Kouno, I. (2006). Antibacterial spectrum of plant polyphenols and extracts depending upon hydroxyphenyl structure. Biol. Pharm. Bull. 29, 2226–2235. doi: 10.1248/bpb.29.2226
Thiennimitr, P., Winter, S. E., and Bäumler, A. J. (2012). Salmonella, the host and its microbiota. Curr. Opin. Microbiol. 12, 108–114. doi: 10.1016/j.mib.2011.10.002
Tinh, T. H., Nuidate, T., Vuddhakul, V., and Rodkhum, C. (2016). Antibacterial activity of pyrogallol, a polyphenol compound against vibrio parahaemolyticus isolated from the central region of thailand. Proc. Chem. 18, 162–168. doi: 10.1016/j.proche.2016.01.025
Tsou, L. K., Lara-Tejero, M., Rosefigura, J., Zhang, Z. J., Wang, Y. C., Yount, J. S., et al. (2016). Antibacterial flavonoids from medicinal plants covalently inactivate type iii protein secretion substrates. J. Am. Chem. Soc. 138, 2209–2218. doi: 10.1021/jacs.5b11575
Van Bambeke, F., Michot, J. M., Van Eldere, J., and Tulkens, P. M. (2005). Quinolones in 2005: An update. Clin. Microbiol. Infect. 11, 256–280. doi: 10.1111/j.1469-0691.2005.01131.x
Varma, J. K., Greene, K. D., Ovitt, J., Barrett, T. J., Medalla, F., and Angulo, F. J. (2005). Hospitalization and antimicrobial resistance in Salmonella outbreaks, 1984-2002. Emerg. Infect. Dis. 11, 943–946. doi: 10.3201/eid1106.041231
Velge, P., Wiedemann, A., Rosselin, M., Abed, N., Boumart, Z., Chaussé, A. M., et al. (2012). Multiplicity of Salmonella entry mechanisms, a new paradigm for Salmonella pathogenesis. Microbiologyopen 1, 243–258.
Vergalli, J., Atzori, A., Pajovic, J., Dumont, E., Malloci, G., Masi, M., et al. (2020). The challenge of intracellular antibiotic accumulation, a function of fluoroquinolone influx versus bacterial efflux. Commun. Biol. 3:198.
Vergalli, J., Dumont, E., Cinquin, B., Maigre, L., Pajovic, J., Bacqué, E., et al. (2017). Fluoroquinolone structure and translocation flux across bacterial membrane. Sci. Rep. 7:9821.
Wang, S., Li, Z., Yu, Y., and Xu, J. (2017a). Folding membrane proteins by deep transfer learning. Cell Syst. 5, 202–211.e3.
Wang, S., Sun, S., Li, Z., Zhang, R., and Xu, J. (2017b). Accurate de novo prediction of protein contact map by ultra-deep learning model. PLoS Comput. Biol. 13:e1005324. doi: 10.1371/journal.pcbi.1005324
Wang, S., Sun, S., and Xu, J. (2018). Analysis of deep learning methods for blind protein contact prediction in CASP12. Prot. Struct. Funct. Bioinforma. 86(Suppl. 1) 67–77. doi: 10.1002/prot.25377
Worthington, R. J., and Melander, C. (2013). Combination approaches to combat multidrug-resistant bacteria. Trends Biotechnol. 31, 177–184. doi: 10.1016/j.tibtech.2012.12.006
Wu, J., Pugh, R., Laughlin, R. C., Andrews-Polymenis, H., McClelland, M., Bäumler, A. J., et al. (2014). High-throughput assay to phenotype salmonella enterica typhimurium association, invasion, and replication in macrophages. J. Vis. Exp. 14:51759.
Xiao, Z. P., Ma, T. W., Fu, W. C., Peng, X. C., Zhang, A. H., and Zhu, H. L. (2010). The synthesis, structure and activity evaluation of pyrogallol and catechol derivatives as Helicobacter pylori urease inhibitors. Eur. J. Med. Chem. 45, 5064–5070. doi: 10.1016/j.ejmech.2010.08.015
Xu, J. (2019). Distance-based protein folding powered by deep learning. Proc. Natl. Acad. Sci. U.S.A. 116, 16856–16865. doi: 10.1073/pnas.1821309116
Xu, J., and Wang, S. (2019). Analysis of distance−based protein structure prediction by deep learning in CASP13. Prot. Struct. Funct. Bioinform. 87, 1069–1081. doi: 10.1002/prot.25810
Yamasaki, S., Nagasawa, S., Hayashi-Nishino, M., Yamaguchi, A., and Nishino, K. (2011). AcrA dependency of the AcrD efflux pump in Salmonella enterica serovar typhimurium. J. Antibiot (Tokyo). 64, 433–437. doi: 10.1038/ja.2011.28
Yanagawa, Y., Yamamoto, Y., Hara, Y., and Shimamura, T. (2003). A combination effect of epigallocatechin gallate, a major compound of green tea catechins, with antibiotics on Helicobacter pylori growth in vitro. Curr. Microbiol. 47, 244–249. doi: 10.1007/s00284-002-3956-6
Keywords: intracellular inhibition, invasion, marbofloxacin, pharmacodynamic, pyrogallol
Citation: Birhanu BT, Lee E-B, Lee S-J and Park S-C (2021) Targeting Salmonella Typhimurium Invasion and Intracellular Survival Using Pyrogallol. Front. Microbiol. 12:631426. doi: 10.3389/fmicb.2021.631426
Received: 20 November 2020; Accepted: 07 January 2021;
Published: 02 February 2021.
Edited by:
Nagendran Tharmalingam, Rhode Island Hospital, United StatesReviewed by:
Paul Ugalde Silva, Rhode Island Hospital, United StatesSelvaraj Arokiyaraj, Sejong University, South Korea
Debayan Dey, Emory University, United States
Copyright © 2021 Birhanu, Lee, Lee and Park. This is an open-access article distributed under the terms of the Creative Commons Attribution License (CC BY). The use, distribution or reproduction in other forums is permitted, provided the original author(s) and the copyright owner(s) are credited and that the original publication in this journal is cited, in accordance with accepted academic practice. No use, distribution or reproduction is permitted which does not comply with these terms.
*Correspondence: Seung-Jin Lee, bGVlLnNldW5namluQGtpdG94LnJlLmty; Seung-Chun Park, cGFya3NjaEBrbnUuYWMua3I=
†These authors have contributed equally to this work