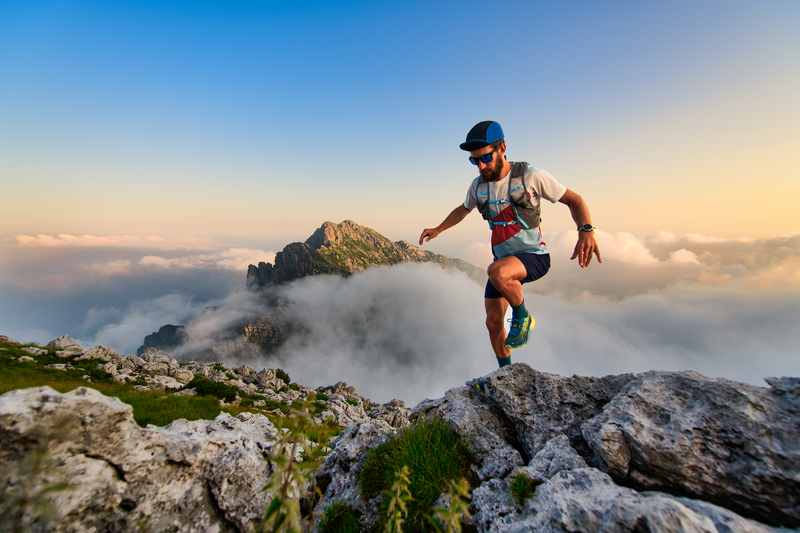
95% of researchers rate our articles as excellent or good
Learn more about the work of our research integrity team to safeguard the quality of each article we publish.
Find out more
REVIEW article
Front. Microbiol. , 25 February 2021
Sec. Microbiotechnology
Volume 12 - 2021 | https://doi.org/10.3389/fmicb.2021.628379
Biological nitrogen fixation (BNF) refers to a microbial mediated process based upon an enzymatic “Nitrogenase” conversion of atmospheric nitrogen (N2) into ammonium readily absorbable by roots. N2-fixing microorganisms collectively termed as “diazotrophs” are able to fix biologically N2 in association with plant roots. Specifically, the symbiotic rhizobacteria induce structural and physiological modifications of bacterial cells and plant roots into specialized structures called nodules. Other N2-fixing bacteria are free-living fixers that are highly diverse and globally widespread in cropland. They represent key natural source of nitrogen (N) in natural and agricultural ecosystems lacking symbiotic N fixation (SNF). In this review, the importance of Azotobacter species was highlighted as both important free-living N2-fixing bacteria and potential bacterial biofertilizer with proven efficacy for plant nutrition and biological soil fertility. In addition, we described Azotobacter beneficial plant promoting traits (e.g., nutrient use efficiency, protection against phytopathogens, phytohormone biosynthesis, etc.). We shed light also on the agronomic features of Azotobacter that are likely an effective component of integrated plant nutrition strategy, which contributes positively to sustainable agricultural production. We pointed out Azotobacter based-biofertilizers, which possess unique characteristics such as cyst formation conferring resistance to environmental stresses. Such beneficial traits can be explored profoundly for the utmost aim to research and develop specific formulations based on inoculant Azotobacter cysts. Furthermore, Azotobacter species still need to be wisely exploited in order to address specific agricultural challenges (e.g., nutrient deficiencies, biotic and abiotic constraints) taking into consideration several variables including their biological functions, synergies and multi-trophic interactions, and biogeography and abundance distribution.
Intensive agriculture relies on important application of N fertilizers, along with other essential nutrients for maximizing crop productivity. Generally, application of synthetic N-based fertilizers was estimated to produce approximately half of the global food supply and that consumption rate of N fertilizers is projected to trend upward from 80 to 180 Mt by 2050 (Bindraban et al., 2015). On the other hand, up to 50% of the application of conventional N-based fertilizers is subject to loss into the soil and the environment (Singh et al., 2014, 2015a). This could substantially inflict economic and environmental issues such as increasing greenhouse gas emissions (e.g., nitrous oxides volatilization accounts for approximately 10-fold emission of CO2-equivalent), soil acidification, depletion of non-renewable resources and nitrate leaching into the groundwater and surface water, which can cause devastating effects such as water eutrophication. Thus, there is a need to sustain use of N fertilizers in order to meet agriculture sustainability challenges consisting of a better crop nutrition and productivity needed for the ever-increasing world population. Most importantly, the soil ecosystem services with safe provision are, undoubtedly, a must for securing agroecosystems sustainability (Lescourret et al., 2015).
Meeting such an urgent and rapidly increasing demand for food, notably in developing nations, cannot be achieved without appropriate mineral fertilization supplies and best practices, especially where crops and resources hardly contribute to an efficient crop production. In fact, constant efforts are needed to intensify agricultural production in a sustainable manner, which consider the entire agro-ecosystem, bio-chemical diversity with the potential to mitigate the adverse impacts of low soil fertility, abiotic stresses, pathogens, and pests (Tilman et al., 2011; Bargaz et al., 2018).
In this context, there is a growing need to consider new innovative approaches for smart and sustainable “food and feed” production with a lesser reliance on conventional fertilizers, notably N. This is in line with meeting current and future changes in human needs within a sustainable context that will likely depend on best management practices and wiser exploitation of both biological and mineral resources, while maintaining environmental quality including preserving natural resources. However, securing adequate plant N nutrition for such a highly mobile nutrient in soils remains challenging. In this regard, biologically fixed N has been the major input of N in agroecosystems.
Atmospheric nitrogen (N2)-fixing bacteria inhabit both plant tissues (e.g., nodules, roots) and soil-root rhizosphere interface and can, consequently, supply significant N amounts for plant growth. This is mainly due to the “biological nitrogen fixation (BNF)” microbially mediated process through a highly sensitive bacterial enzymatic conversion of atmospheric N2 into ammonia (NH3). BNF can provide an ecologically acceptable complement or substitute for mineral N fertilizers. This process is controlled by the availability of some important resources such as phosphate (P), Molybdenum (Mo), and water (Schulze and Drevon, 2005; Alkama et al., 2012; Lazali and Bargaz, 2017; Timmusk et al., 2017). Published estimates regarding N derived from BNF indicated rates ranging approximately between 1.95 × 1011 kg of N-NH3 (Galloway et al., 2004) and 2.5 × 1011 kg of N-NH3 from BNF is fixed annually (Cheng, 2008). This study also reported that nearly 2 tons of industrially fixed N is needed as fertilizers for crop production to equal the effects of 1 ton of biologically fixed N by leguminous crops and cyanobacteria.
The process of BNF is widely known as (i) symbiotic N fixation (SNF) by bacteria living in symbiotic association with leguminous and higher plants that allocate carbon to N2-fixing bacteria in exchange for N and (ii) non-symbiotic BNF by heterotrophic or autotrophic bacteria inhabiting soils, water, rocks, and leaf litter or in association with plants. For example, rhizobia–legume symbiotic associations are known to be the most important BNF biosystem, contributing with an average of 227 kg N ha–1 annually (Herridge et al., 2008) and may reach up to 300 kg N ha–1 according to Roughley et al. (1995). Meanwhile, non-symbiotic BNF estimates for maize, rice, and wheat production systems reported an average contribution of 15.5 kg N ha–1 based on a 50-year assessment study (Ladha et al., 2016).
Large-scale data on non-symbiotic BNF estimates are scarce except for staple cereal crops such as maize, rice, and wheat (Ladha et al., 2016). However few reports indicated that BNF by free-living diazotrophs may be roughly estimated at up to 60 kg N ha–1 year–1 (Vadakattu et al., 2006; Reed et al., 2011). Among non-symbiotic N2-fixing bacteria; Beijerinckia, Azotobacter, Azospirillum, Herbaspirillum, Gluconacetobacter, Burkholderia, Clostridium, Methanosarcina, and Paenibacillus are well-known and have proven significant efficacy in cereals crops (e.g., growth and grain yield) (Malik et al., 2002; Kennedy et al., 2004; Ritika and Utpal, 2014; Ladha et al., 2016). In this review, we focused on Azotobacter being non-symbiotic N2-fixing bacteria that are highly diverse and globally widespread in soils. This bacterial group may represent the dominant natural source of N in ecosystems lacking SNF (Choudhury and Kennedy, 2004; Das and Saha, 2007). Moreover, the abundance of Azotobacter species in the soil could improve the availability not only of N through the BNF processes (Din et al., 2019), but also P as well (Velmourougane et al., 2019). Moreover, a study by Kizilkaya (2009) demonstrated that soil carbon and sulphur contents increased in response to inoculation with Azotobacter species by accelerating the mineralization of soil organic residues, which subsequently reduced heavy metals absorption by roots.
Recently, advancing applied research on Azotobacter species is of special interest as both agriculturally important plant growth promoting N2-Fixing rhizobacterium (PGPR) that can be used for improving plant N nutrition and a biofertilizer based products at large scale, having significant improvements in crop productivity and soil fertility. Besides BNF, Azotobacter species are able to influence directly plant growth by synthesizing plant growth hormones [e.g., Indole Acetic Acid (IAA), gibberellins, and cytokinins]. These hormones can not only enhance plant growth and nutrient uptake, but can also indirectly protect host plants from phytopathogens and stimulate other beneficial rhizosphere microorganisms (Sahoo et al., 2014; Arora et al., 2018). Furthermore, Azotobacter strains exhibited positive effects on plant growth, crop yield and plant N requirements of several economically important cereal and pulse crops, reaching significant yield improvement (up to 40%) (Yanni and El-Fattah, 1999; Choudhury and Kennedy, 2004; Kannan and Ponmurugan, 2010; Wani and Ali, 2013; Ritika and Utpal, 2014). These positive traits offer promising possibilities to ecologically engineer Azotobacter species likely providing significant N inputs, while reducing reliance to N-containing fertilizers such as urea (Wani et al., 2016; Bageshwar et al., 2017).
Bioformulation of microbial inoculants is still requiring fundamental and applied studies, allowing the transition to a larger scale supporting the approach “from industry to farm” (Bashan et al., 2014). Azotobacter species possess some unique features such as cysts formation confering resistance to environmental stresses (Sadoff, 1975). Such properties are reviewed with potentialities to develop specific Azotobacter cyst-based formulations. Furthermore, besides the agronomic potential of Azotobacter based biofertilizers species, their geographical distribution and diversity require additional specific studies even though global interest in beneficial Azotobacter species has slightly waned in recent decades with a few thousands of research investigations on free N2-Fixing bacteria and Azotobacter in particular. Particularly, this review provides necessary data on Azotobacter species occurrence in Moroccan soils. This review also sheds light on specific Azotobacter features that are highly beneficial for improved crop production, nutrient use efficiency (particularly P and N), and stress tolerance in staple crops while highlighting their abilities to reduce the need for synthetic N-based fertilizers. Another part of this review summarizes patents related to Azotobacter formulations and product development bringing to light future prospects toward Azotobacter product innovation. Market aspects of Azotobacter based products are also discussed, allowing an evaluation of investments and the inventiveness in this field.
The commercial history of microbial biofertilizers was launched with the Rhizobium-based bioinoculant named “Nitrogin,” which was considered the pioneer biofertilizer of all rhizobial inoculants (Patil and Solanki, 2016). Exploring the plant growth promoting abilities of soil N2-fixing microorganisms (including non-symbiotic bacteria such as Azotobacter) led to the development of the Azotobacter-based biofertilizer namely “azotobakterin” in Russia and East European countries, where ≃10 million ha of land was treated with microbial formulations in the middle of 19th century (Brown, 1974; Rovira, 1991). In Africa, the first studies on Azotobacter dated back to 1959, mainly reported by Becking, specifically on the genus Beijerinckia in South African soils (Becking, 1959). Few other studies published later by Makawi (1973) and Hegazi (1979) regarding the presence of Azotobacter species in Libyan and Egyptian soils (in both soils and roots).
Azotobacter-related research papers account for more than 4000 publications over the last two decades showing an exponential increase in the cumulative publication number, particularly from 1990 to 2020 reaching almost 4066 documents in 2020 found online using Azotobacter as a main key word (Figure 1A). As per research domain, most publications available on Azotobacter species were split between more than 20 research areas with most studies focused on biochemistry, genetics, molecular biology, agriculture, and overall biological sciences of Azotobacter (Figure 1B). Generally, Azotobacter-related publications are primarily research articles (88.7%) with more than 3600 publications, while reviews, conference papers and book chapters represent nearly 11.3%. As per country, the spatial distribution of Azotobacter-related documents revealed India and United States as the leading countries with more than 800 published items, whereas, research publications across African countries remain scarce, with the exception of Egypt wherein approximately 109 documents published between 1990 and 2020.
Figure 1. (A) Exponential growth in the number of scientific publications related to Azotobacter from 1990 to 2020 using Azotobacter as a key word from scopus (https://www.scopus.com). (B) Abundance and thematic distribution of the literature available on Azotobacter. Bibliometric research made in scopus (https://www.scopus.com) using Azotobacter as a keyword in June 2020.
Azotobacter representatives can commonly be found in soil, water, sediments, and plant roots (Aquilanti et al., 2004). Azotobacter species are generally found in slightly acidic to alkaline soils, which often governs the occurrence of certain species (Becking, 2006). For example, species belonging to Azotobacter chroococcum and Azotobacter vinelandii are more abundant in tropical soils, while Azotobacter beijerinckii species were often reported in acidic soils (Kennedy et al., 2015). However, only Azotobacter paspali was described to specifically associate with plant roots of Paspalum notatum cv Batatais (Kennedy et al., 2004).
The number of Azotobacter strains in soils is generally low (<104 CFU g–1 soil). However, they are found throughout the world typically in 30 to 80% of sampled soils (Kennedy et al., 2004). Considerations about whether Azotobacter is a rhizospheric or non-rhizospheric bacterium are still debated. However, based on most research investigations, Azotobacter prevalence is generally not higher in the rhizosphere compared to open locations (Bartholomew, 2015). Nevertheless, certain species are denser in the rhizosphere of higher plants than in the soil itself. This is in line with the fact that Azotobacter species were reported to be found in fertile than in sandy soils owing to their relatively high requirement for P (Brenner et al., 2005). Likewise, findings regarding the ability of Azotobacter species to enhance the growth of various crops should prompt a re-examination of whether Azotobacter abundance might be higher in the rhizosphere than in non-rhizosphere soils (Kennedy et al., 2004).
Progress in culture-independent approaches, mainly shotgun and amplicon sequencing, could improve substantially our understanding of diversity and function of diazotrophs including Azotobacter species in soils and plant compartments. Recently, Hassen et al. (2020) reported low abundance of Azotobacter (0.06%) in the rhizosphere microbiome of a South African indigenous legume “Cyclopia intermedia,” as revealed by shotgun metagenomics techniques. Microbiome analysis of Maize rhizosphere in Pakistan based on library constructions of 16S rRNA and functional nif-H gene revealed biases linked to culture media in the culture dependent techniques to investigate relative abundance of diazotrophs in the rhizosphere (Qaisrani et al., 2019). Nif-H gene clones confirmed a relatively low abundance of Azotobacter (5%) among the diazotrophs investigated in this study (Qaisrani et al., 2019).
According to Rana et al. (2020) there is no strong evidence that Azotobacter members could colonize internally plant tissues, even if endophytic microbes are, theoretically, able to fix more N2 as compared to rhizospheric microorganisms because of low partial oxygen pressure in tissues compared to external surrounding soil.
Meanwhile, either biogeographically or functionally, Azotobacter species have rarely been thoroughly investigated, mainly in Africa. Yet very few researches described the diversity and occurrence of Azotobacter species, which means that tremendous efforts are still to be deployed for mechanistic studies. In North Africa, only one main study was reported in Morocco by Sasson and Daste (1961), who published observations concerning Azotobacter ecology in dry soils of Morocco so far being the first evidence of the presence of Azotobacter occurrence strains in Moroccan soils. However, in a recent collaborative project aiming on isolation of Azotobacter from Moroccan soils will bring to light new evidence on the occurrence and diversity of Azotobacter and diazotrophs in Morocco (not published). Authors of this project (Dr. Issam Kadmiri. personal communication) adopted a molecular approach based on 16S rDNA and nif-H markers along with a conventional culture dependant approach and biochemical characterization. Promising findings showed significant variations in terms of both abundance and diversity of non-symbiotic N2-fixing isolates (Figure 2) in which, Azotobacter spp. representatives accounted for more than 22% of all strains isolated across Moroccan soils.
Figure 2. Number and distribution of major free N2-Fixing species identified in seven agricultural locations representative of Moroccan agricultural soils using 16S rDNA marker.
Despite various experimental data available on Azotobacter biostimulation traits on overall plant growth, the exact mode of action by which Azotobacter can enhance plant growth is not yet fully understood (Sumbul et al., 2020). However, the main mode of action evidently includes BNF, considering the capacity of these bacteria to fix N2, a vital macronutrient for plant growth. Moreover, these diazotrophs are capable to solubilize insoluble P forms in the soil (Nosrati et al., 2014). Other studies proposed other modes of action such as the production of phytohormone-like substances that alter plant growth and morphology and the bacterial mechanism of nitrate reduction that increases N accumulation in plants inoculated with Azotobacter strains (Deubel and Merbach, 2005; Wani and Ali, 2013; Wani et al., 2016).
Azotobacter species play an important role in maintaining soil N status. The estimated contribution of non-symbiotic BNF rates are subject to variations due to several factors including environmental variability, management and cropping practices, genotypic differences, and technical aspects related to methods used to estimate BNF (Peoples and Herridge, 2000; Ladha et al., 2016). The rates of these free-living N2-Fixing bacteria to N input of soil range from 0.3 to 15 kg ha–1 year–1 (Saha et al., 2017), other studies reported up to 60 kg ha–1 year–1 (Bhattacharyya and Jha, 2012).
This BNF process under aerobic conditions is the principal characteristic of the genus, which is extremely tolerant to oxygen during fixing N2 due to respiration protection of nitrogenase (Hakeem et al., 2017). The two component proteins of the Mo-dependent nitrogenase are called the iron (Fe) protein or dinitrogenase reductase. These two component proteins act together to catalyze the reduction of dinitrogen in a complex reaction with an ideal reaction stoichiometry shown as follows (1) (Kirn and Rees, 1992):
The Fe-protein is a homodimer that contains two nucleotide binding sites (MgATP or MgADP), one on each subunit and a single 4Fe–4S cluster that bridges the two subunits (Figure 3). The MoFe-nitrogenase is a α, β, heterotetramer. Each α, β dimeric unit contains two unique metalloclusters: a P-cluster (8Fe–7S) and a FeMo-cofactor (FeMo-co). During the catalytic cycle, a Fe-protein binds to one MoFe-protein αβ unit. During this encounter, one electron is transferred from the cluster 4Fe–4S to the MoFe protein. This electron transfer step is coupled to the hydrolysis of a minimum of two MgATP molecules. Following electron transfer and ATP hydrolysis, the Fe protein disengages from the MoFe protein and a new Fe protein binds in its place to repeat the cycle (Figure 3). Given that only one electron is transferred per cycle, a minimum of eight encounters must occur to reduce N as demonstrated by the Eq. 1. Detailed descriptions of the Fe-protein, P-clusters, and cofactor are available in Walker (2011). The major and minor clusters of genes encoding the nitrogenase complex enzymes were extensively studied in A. vinelandii. Detailed descriptions of the genome sequence of A. vinelandii and nif genes were reported by Setubal et al. (2009) and Poza-Carrión et al. (2015).
This is an energetically demanding process, requiring chemical energy in the form of phosphodiester bond energy from ATP (Setubal et al., 2009; Hamilton et al., 2011; Ledbetter et al., 2017; Segal et al., 2017; Batista Bueno and Dixon, 2019). Nevertheless, this process remains less demanding in energy and environmentally friendly compared to industrial N fixation through the energy-extensive Haber–Bosch method or geochemical processes, such as lightning (Gruber and Galloway, 2008; Thamdrup, 2012; Connor and Holland, 2017).
In addition, Azotobacter species could be considered as an evolving bacterium, because in addition to using the classic Mo-containing enzyme for BNF, Azotobacter species are able to synthetize one or more alternative nitrogenases under conditions where there is a lack of molybdenum. A. vinelandii for example was found to encode three different nitrogenase enzymes with different structural sub-units: (1) the traditional Mo-nitrogenase (2) a Vanadium containing enzyme (nitrogenase-2, encoded by the vnf-H, vnf-D, G, K genes), and (3) an iron containing nitrogenase (nitrogenase-3, encoded by the and H, D, G, K gene cluster) (Robson et al., 1986; Leigh, 2002).
Understanding the mechanisms employed by Azotobacter species to accomplish aerobic BNF could be integral to determining how to transfer this process into the aboveground bacteria, tissues of land plants, or better utilize this process in leaf endophytes or other endophytes associated with aboveground photosynthetic tissues in plants (Barney, 2020).
Besides N, P is a major nutrient, which plays an important role in plant physiology and biochemistry as well as in microorganism physiology such as BNF. Soils usually contain large amounts of total P in different available forms, including insoluble forms such as tri-calcium P (Ca3PO4)2, aluminum P (Al3PO4), and iron P (Fe3PO4). Unfortunately, compared to the other major nutrients, P is by far the least mobile and available nutrient to plants in most soils, even if the total soil P content is well beyond plant needs (400–1.200 mg/kg) (Nosrati et al., 2014). The poor mobility of soil P is due to the large reactivity of P ions with numerous soil constituents (Hinsinger, 2001), with only a small fraction of small P is available for plant growth (<1 mg P Kg–1) (Rodrìguez and Fraga, 1999; Richardson et al., 2009; Barker et al., 2015).
However, these forms may be converted to soluble P by soil phosphate-solubilizing microorganisms (PSMs) (Gupta et al., 2007; Song et al., 2008; Khan et al., 2013; Sharma et al., 2013; Kumawat et al., 2017). Numerous soil microflora were reported to solubilize insoluble P complexes into soluble forms readily absorbed by plants (Sashidhar and Podile, 2010). Among the phosphate-solubilizing bacteria (PSB), Bacillus and Pseudomonas are the most common along with some Azotobacter species also known for their P solubilizing capacity. A study by Hafez et al. (2016) demonstrated that A. vinelandii strain was able to solubilize up to 43% of the Abu Tartur phosphate rock in Egypt, while another study by Yi et al. (2008) showed that Azotobacter exopolysaccharides (EPS) were the main factor in the microbial solubilization of tricalcium P (TCP). Azotobacter species were also found to improve their P solubilizing through mutagenesis starting from soil isolates (Kumar et al., 2001). Therefore, these microorganisms are used as biofertilizers in order to compensate or even increase benefit of chemical fertilizers (Narula et al., 2000; Kumar et al., 2001; Nosrati et al., 2014).
The solubilization of insoluble P mechanism remains a research subject (Illmer and Schinner, 1995; Khan et al., 2007; Buch et al., 2008). Solubilization of P through low molecular weight organic acids has been a well-studied and a widely accepted theory being the main solubilization mechanism, and various studies have identified and quantified organic acids and defined their role in solubilization (Maliha et al., 2004; Khan et al., 2010; Marciano Marra et al., 2012; Azaroual et al., 2020). This process involves the acidification of microbial cells and their surroundings, leading to the release of P-ions from the P-mineral by H+ substitution for calcium (Trivedi and Sa, 2008). However, the efficiency of P solubilization process depends on the type and the amount of organic acids released, with assumption that the quality of the organic acid released is more important than the total amount of acids (Scervino et al., 2010). Other studies suggested that P solubilization can be done by other mechanisms besides the release of organic acids (Asea et al., 1988; Illmer and Schinner, 1992; Chen et al., 2006).
Another important aspect is the relation between BNF rates and soil nutrients availability (especially P). It is well established that BNF is often limited by the low P availability in soils, however Mills et al. (2004) found no exclusive P limitation at any of their experimental sites where the BNF seems to be limited by Mo alone in P rich soils and co-limited by both Molybdenum and P in P poor soils. Another study suggested that the BNF limitation by P and Mo is a dynamic process. P can likely limit BNF in the early stage of the growing season, while Mo is limiting factor in mid-season (Jean et al., 2013).
In the soil ecosystem, populations of Azotobacter sp. are affected by soil physicochemical parameters such as organic matter, pH, temperature, soil depth, soil moisture, and soil salinity (Kizilkaya, 2009). The NaCl concentrations affected the PGPR activities of Azotobacter, mainly BNF in soil. However, some species of Azotobacter are known to tolerate salt concentrations of up to 10% NaCl. e.g., Azotobacter salinestris was shown to tolerate 8% NaCl concentration, but the total CFU/mL values were reduced compared to lower NaCl concentrations. In response to temperature, Azotobacter is a typical mesophilic organism which thrives at optimum temperatures of 25–30°C for growth and physiological properties. The minimum temperature for the growth of Azotobacter evidently lies on little above 0°C. Azotobacter cells cannot tolerate high temperatures, although they can survive at 45–48°C by forming cysts which germinate under favorable conditions (Saribay, 2003). A. salinestris survived at 45°C and recorded an optimum growth rate at 35°C, the growth reduced with increasing temperatures above 35°C.
The presence of Azotobacter populations in soil ecosystems is controlled by pH. Generally, lower pH (<6.0) decreases Azotobacter population and in some cases, completely inhibits their growth. Acidic soils have unfavorable properties of poor and physiologically active nutrients and unsatisfactory air–water regime, so that the presence of Azotobacter in these soils was very low or even absent (Andjelković et al., 2018). An optimum pH of 7–7.5 is favorable for the physiological functions of Azotobacter. At this pH, population number may fall between 102 and 104 per gram of soil (Becking, 2006). Meanwhile, A. chroococcum survived at pH 9 and its growth was not inhibited at higher pH values, whereas A. salinestris was sensitive to pH above 9 and no growth was observed above this range.
Azotobacter species possess some unique features such as cysts formation (Sadoff, 1975). The formation of cysts is induced naturally in face of unfavorable and extreme conditions such as high or low temperatures, freezing, salinity, and drought. The cyst formation is induced also in response to changes in nutrients concentrations in the medium or the addition of some organic substances such as ethanol, n-butan-1-ol, or β-hydroxybutyrate. It is also affected by aldehyde dehydrogenase and the response regulator AlgR (Núñez et al., 1999). These morphological changes are accompanied by metabolic shifts, changes in catabolism, respiration and biosynthesis of macromolecules. Cysts of Azotobacter are spherical and consist of the so-called “central body,” a reduced copy of vegetative cells with several vacuoles and a “two-layer shell.” The inner part of the shell has a fibrous structure called intine, while the outer part has a hexagonal crystalline structure called exine. Numerous polyhydroxybutyrate granules are always observed within the central body, alginate is a major component of the capsule, and alkylresorcinols (a phenolic lipid) and alkylpyrones that are synthesized upon encystment induction replace the phospholipids of the cyst membranes and are components of the exine (Segura et al., 2014; Lara-López and Geiger, 2017). Some studies clearly indicated the role of small RNAs and LEA (Late embryogenesis abundant) proteins in the formation and resistance to desiccation and abiotic stresses in Azotobacter cysts (Castañeda et al., 2016; Rodriguez-Salazar et al., 2017). One of the main features of the cyst is its ability to withstand desiccation, being able to survive in dry soil for more than 10 years whereas vegetative cells stored under the same conditions were inactivated in less than 2 years (Vela, 1974). In particular, they are twice as resistant to UV light. They are also resistant to drying, ultrasound and gamma and solar irradiation, but not to heating (Wyss et al., 1961).
Encystment of Azotobacter strains in laboratory conditions can be induced upon induction of vegetative cells with specific reagents such as ethanol, n-butan-1-ol, or β-hydroxybutyrate. This process may be of great interest in Azotobacter bioformulation, mainly when fertilizer and phosphate rock are used in combination with the inoculant. It was shown that cyst formation at large-scale in biofertilizer product development using Sinorhizobium meliloti, Azospirillum brasilense, and Azospirillum lipoferum, extended the product shelf-life while maintaining its effectiveness (József et al., 2007). However, further studies are needed to investigate the behavior of cysts in natural soil conditions, since this feature makes Azotobacter species more resistant to soil and environment conditions and predators.
Besides BNF, the beneficial effects of Azotobacter on plant growth are also attributed to an improvement in root development, an increase in the rate of mineral uptake by roots as well as their antagonism against fungi and plant pathogenic bacteria. Azotobacter synthetizes and secretes considerable amounts of biologically active substances like B vitamins, nicotinic acid, pantothenic acid, biotin, heteroxins, and gibberellin, which enhance root growth of plants (Azcón and Barea, 1975; Patil et al., 2020). Inorganic and organic P solubilization by Azotobacter strains is another growth promoting trait which is characterized to screen efficient free-living N2-fixing bacteria (Narula et al., 2000; Nosrati et al., 2014; Hafez et al., 2016).
Another plant growth promoting trait showed by Azotobacter species is auxin (IAA) production. It is a fundamental phytohormone that modulates plant growth and development (Halliday et al., 2009; Grossmann, 2010). This phytohormone helps the production of longer roots and increases number of root hairs and lateral roots which are involved in nutrient uptake (Datta and Basu, 2000). It plays a central role in cell division, elongation, fruit development and senescence. Auxin initiates roots, leaves, and flowers (Phillips et al., 2011). Several works proposed that Azotobacter species can facilitate plant growth via synthetizing this phytohormone rather than N fixation (Behl et al., 2007; Ahmed and Holmström, 2014).
In addition to the production of these substances, some strains of Azotobacter (such as Atropicalis tropicalis, Azorhizophilus paspali, and A. vinelandii) have been characterized by their capacity to synthesize antifungal substances that inhibit the development of some phytopathogenic species such as Helminthosporium sp., Macrophomina sp., and Fusarium sp. (Bjelić et al., 2015). El_Komy et al. (2020) demonstrated that the use of a mixture of Azotobacter, Azospirillum, and Klebsiella significantly reduced the mycelial growth of certain pathogenic fungi such as Macrophomina phaseolina, Rhizoctonia solani, and Fusarium solani. Also, isolates of A. vinelandii have been characterized to have the ability to produce polysaccharides, such as alginate, at rates ranging from 4.88 to 5.26 g/L. Hydrogen cyanide (HCN) and siderophores production has been also characterized for Azotobacter species (Baars et al., 2015).
Potassium (K) and zinc (Zn) solubilization are part of the important potentials of how Azotobacter can promote plant growth. Wu et al. (2006) demonstrated the ability of the soil bacteria A. chroococcum to increase the bioavailability of Zn in the soil system. Various mechanisms are involved in this process, including the acidification. These microbes produce organic acids in soil which sequester the Zn cations and decrease the nearby soil pH (Alexander, 1997; Aung et al., 2020). Other mechanisms possibly involved in Zn solubilization include production of new siderophores family by A. chroococcum e.g., vibrioferrin, amphibactins, and crochelins which can bind iron in a hexadentate fashion using a new iron-chelating γ-amino acid. Such siderophores help bacteria to access iron resources but contribute also to control plant pathogens in the soil (Saravanan et al., 2011; Baars et al., 2018).
The capacity of Azotobacter species to solubilize K has been proven through several works (Singh et al., 2010; Sangeeth et al., 2012; Archana et al., 2013; Diep and Hieu, 2013). Other works suggested that Azotobacter species can not only solubilize K but also they can play an important role in improving K assimilation by plant (Wu et al., 2005; Singh et al., 2010).
Enzyme 1-aminocyclopropane1-carboxylate (ACC) deaminase is also a key trait produced by Azotobacter (Omer et al., 2016). ACC deaminase-producing organisms decrease plant ethylene levels which, when present in high concentrations, can lead to plant growth inhibition or even death (Honma and Shimomura, 1978; Glick et al., 2007). This enzyme is responsible for the cleavage of the plant ethylene precursor, ACC, into ammonia and -ketobutyrate by decreasing ACC levels in plants.
Many Azotobacter strains produce pigments that are involved in the metabolism of other microorganisms. For example, A. chroococcum forms dark-brown water-soluble pigment melanin which occurs at high levels of metabolism during BNF. This process is thought to protect the nitrogenase system from oxygen. Shivprasad and Page (1989) quantified the effect of Azotobacter on the overall microbial activity of the soil via the determination of soil dehydrogenase activity, which is an indication of the intensity of metabolic activity of microorganisms. In this research, dehydrogenase activity increased in all the variants where Azotobacter was applied.
The importance of Azotobacter as microbial inoculant is convincingly established throughout various experiments and large number of field trials. Ritika and Utpal (2014) showed in their review that the use of Azotobacter as N-biofertilizer increased the growth and yield of various crops under field conditions with a percentage increase of up to 40% for Cauliflower and 15–20% for Maize compared to conventional fertilizers. These beneficial effects can be attributed to the biosynthesis of biologically active substances, the stimulation of rhizospheric microorganisms, the production of phytopathogenic inhibitors and improved nutrient availability of N, P, carbon, and sulfur, through BNF and mineralization of organic residues in soil (Lévai et al., 2008; Lenart, 2012).
Numerous studies described crop responses to Azotobacter inoculation under greenhouse and field conditions. Plant responses ranged from increase in seed germination rates, root development, enhancement in nutrient uptake, root and shoot biomasses and leaf number and area (Wani et al., 2016). Quality attributes such as protein content, fruit total soluble solids and fruit stability after harvest have also been reported. Other studies also demonstrated that using Azotobacter species either alone as biofertilizer or in combination with other beneficial species like PSB and Azosprillum improved crop yield and quality of different crops. Table 1 summarizes the effect of Azotobacter based biofertilizers on yield and quality improvement of different crops and conditions. High percentage increases in both yield and quality attributes are reported in Table 1.
Table 1. Effect of Azotobacter based biofertilizers on yields and quality improvement of different crops.
Drought and salinity are among the major environmental constraints that limit growth, productivity, and quality of crops (Yang et al., 2009). Screening of various salt-tolerant strains of Azotobacter has revealed that some strains are able to colonize the rhizosphere successfully and promote plant growth under stress conditions. Multiple facets of Azotobacter mechanisms could explain their plant stress alleviation and may include additional properties beyond their characterized function of nitrogen fixation. All these properties could enhance the tolerance to abiotic and biotic stress in inoculated plants (Ruzzi and Aroca, 2015).
Azotobacter strains were found to enhance growth when applied with wheat under salt stress (Chaudhary et al., 2013). Additionally, inoculation of maize plants with Azotobacter has been reported to improve growth in saline stress conditions by improving sodium exclusion and potassium uptake (Rojas-Tapias et al., 2012; Latef et al., 2020). Moreover, Azotobacter species can protect several plants from biotic stress caused by plants’ pathogens. This capacity depends on their competition with the indigenous microbial and fungal strains and their colonization ability in the soil and rhizosphere (Goel et al., 1997). HCN and siderophores production agents (Ponmurugan et al., 2012) and the production of antimicrobial compounds such as 2,3-hydroxybenzoic acid, aminochelin, azotochelin, protochelin, and azotobactin are also known to inhibit the growth of many common plant pathogens such as Curvularia, Aspergillus, Fusarium, and Rhizoctonia species (Bhosale et al., 2013).
Several works on drought stress tolerance using Azotobacter species as a solution demonstrated the efficacy of their use (Creus et al., 2004; Shirinbayan et al., 2019). Sandhya et al. (2009) noted an increase of resistance to water stress in sunflower plants treated with EPS produced by Azotobacter, probably due to their ability to improve soil structure in the rhizosphere. The EPS produced by Azotobacter are essential molecules to maintain cellular hydration and biofilm formation under desiccating conditions. The polysaccharides are able to form various structures within a biofilm and may interact with a wide range of other molecules, including lectins, proteins, and lipids (Chang et al., 2007). They also revealed a high adsorption rate of metals (Gauri et al., 2011). EPS of Azotobacter directly bind and uptake heavy metals like Cd and Cr in the contaminated soils (Joshi and Juwarkar, 2009).
For the large-scale production of Azotobacter, it is necessary to take into consideration the improvement of some cultural and nutritional parameters in order to improve its growth in fermentation process avoiding contamination, as well as to improve its capacity as biofertilizer (Gomare et al., 2013).
There are various methods that can be followed to enhance Azotobacter species capabilities with the help of genome engineering and synthetic biology for either addition or deletion of targeted gene(s).
For Azotobacter nitrogen fixation, previous evidence suggested that nif-A acted as an activator of nitrogenase expression, while nif-L acted as an anti-activator (Little et al., 2006). With the presence of oxygen or ammonium, nif-L interacts with the nif-A and blocks its function (Das, 2019).
Several works have considered these properties to increase the level of ammonium released. Bali et al. (1992) were able to obtain high levels of release compared to wild strains by only disrupting a section of the nif-L gene and leaving nif-A intact. While Ortiz-Marquez et al. (2012) constructed an almost complete suppression of nif-L, resulting in a strain that also accumulated significant ammonium levels in the medium compared to unmodified strains. Bageshwar et al. (2017) deleted a part of the negative regulatory gene nif-L in A. chroococcum strain named HKD15 that was able to enhance wheat yield with 60% and reduce urea fertilizer.
Besides increasing Azotobacter nitrogen fixing capacity, improving other capacities of this species will be useful, such as generating strains that produce alginates with specific chemical characteristics (Galindo et al., 2007) or engineering their capacities to solubilize phosphate (Sashidhar and Podile, 2009).
Genetic engineering of Azotobacter species could be also adopted in the formulation processes, in order to improve encystment capacity and produce high resilient cysts with longer shelf life and resistance to contamination and harsh environment conditions. Such applications are still under-studied and need more scientific efforts in order to develop new generation of Azotobacter based inoculant.
Azotobacter has been known for their beneficial effects on crop growth and yield through BNF, biosynthesis of biologically active substances, stimulation of rhizospheric microbes and production of phyopathogenic inhibitors (Lenart, 2012). This kind of bacteria is capable of surviving under severe conditions of temperature and water availability by converting to a more resistant form than the vegetative cells (Sadoff, 1975). All these capacities give them a possibility to be applied as basis for biofertilizer products that can decrease the excessive use of chemical fertilizers.
The beneficial effects of Azotobacter and Azospirillum interaction on plants are mainly attributed to their capacity to improve root development, water and mineral uptake by roots, the displacement of fungi and plant pathogenic bacteria and to the BNF (El-Mokadem et al., 1989; Okon and Itzigsohn, 1995). Similarly, combined inoculation of Azotobacter and Rhizobium spp. has revealed a positive synergistic action resulting in significant increase in nodulation, increasing N content within roots and shoots of respiring/metabolizing plant cells, improving conditions within the rhizosphere and enhancing synergistic interactions between the host and Azotobacter sp. (Yadav and Vashishat, 1991).
Large scale inoculants production of Azotobacter sp. could be summuriszed in 4 steps (Figure 4). It ranges from the isolation and screening of effective strains according to several characteristics including N fixation, P solubilization, etc. to mass production with a suitable culture medium and finally the choice of the formulation according to the application mode sought. Solid formulations may be subdivided into powders and granules depending on their particle sizes. In general, they are applied as seed coatings or soil amendments (Bashan et al., 2014). Whearas, liquid formulations are suitable for a wide range of application technologies, they may be coated directly onto the seed (with the use of adhesive) immediately prior to sowing (Bashan et al., 2014) or used as a coating for chemical fertilizers (Hindersah et al., 2020). They may also be delivered to the soil in−furrow during sowing or at a later stage via fertigation systems (Malusá et al., 2012). Furthermore, liquid formulations allows the treatment of above−ground plant parts, for example in form of a foliar spray (Jambhulkar et al., 2016).
The genus Azotobacter has been used as a biofertilizer more than a century (Gerlach and Vogel, 1902). Using Azotobacter strains as a bioinoculant for a wide range of crops (cereals, tomato, eggplant, carrot, and sugarcane), has been reported to lead to better yield results (Mrkovacki and Milic, 2001). N2-Fixing biofertilizers like Rhizobium, Azotobacter, and Azospirillum, which are majorly used for BNF in seed and soil treatment applications, currently represent the largest segment of the global biofertilizer market. Global N2-Fixing biofertilizers market was valued at USD 800 million in 2016 and is expected to reach USD 3 billion by the end of 2024 (Soumare et al., 2020), growing at a CAGR of about 14.3% during the forecast period. The global market for Azotobacter-based biofertilizer was valued at USD 212.2 million in 2017 and is expected to register a CAGR of 8.7% during the period 2020–2025 (Mordor intelligence market, 2020)1. Some biofertilizers based on Azotobacter available in the market are summarized in Table 2.
Table 2. Benchmark of Azotobacter based biofertilizers used around the globe (modified from Mishra and Arora, 2016).
In order to evaluate Azotobacter biofertilizers IP trends (patenting activity), an analysis was made using “IP Business Intelligence” of “Orbit Intelligence” services. The research was carried out using the following query: « Azotobacter and biofertilizer », which generated 233 IP patent documents with a corresponding statistical study (Figure 5).
Figure 5. Technology investment and the evaluation dynamics of inventiveness of the studied of biofertilizers based on Azotobacter bacteria in the last 20 years.
The evaluation dynamics of inventiveness of the studied portfolio (Linear or exponential portfolio) indicates that the depositor is in the construction phase of its portfolio (more or less quickly). Contrariwise, a decrease in the number of patent families filed is generally symptomatic of a substantial decrease in R&D budgets and/or “intellectual property” budgets. Different profiles can be observed, and these profiles depend on the deposit strategy implemented by the depositor. Technology investment in the field of biofertilizers based on Azotobacter bacteria peaked in 2015, with 30 patent families as shown in Figure 5. This deposit peak can be explained by a massive deposit of actors at an instant t and in the opposite a hollow in patent deposit may be a result of repercussions of crisis or economic events on R&D budgets and consequently on patent filings. It should be noted that the last 2 years are incomplete, this is due to the publication period of 18 months between the filing of the application and its publication.
The results of our query showed that 17% of the resulting patent families belong to the top 10 IP players, besides that the International Patent Classification (IPC) has, in fact, been the subject of a grouping of work in 35 technological fields. “Basic materials chemistry” is predominant in the results, which belongs to IPC class of fertilizers and their manufacture (class C05). This class includes inorganic and organic fertilizers involving or not the addition of bacterial culture. Biotechnology is the second technological field that emerges from the statistical analysis of the patents research, which belongs to the IPC class of microbiology, enzymology, techniques of mutation, or genetic among others (class C12). Specifically, many patents resulting from the query are classified under the sub class C12N, which describes: micro-organisms or enzymes; compositions containing micro-organisms or enzymes; culture or preservation of micro-organisms, mutation or genetic techniques; culture media. Besides the distribution of these patenting activities, the extension strategies of the players in the sector were also studied. Thirty-nine patent families are applications filed at the European Patent Office and 23 are International patent applications [Patent Cooperation Treaty (PCT)]. The location of the extensions is a good indicator of the markets where players need to protect their invention in the location or region of interest. It should also be noted that some players protect the geographic areas where their competitors’ manufacturing sites are located (case of China and India).
The IP analysis showed that there is a need in investment in R&D in order to introduce new and innovative products. This analysis also identified the technological core of the actor in question. The least represented categories are future lines for identifying other potential applications of the actor’s patents.
It is clear that BNF can inexpensively supply an environmentally acceptable supplement for N resulting through the symbiotic and asymbiotic BNF either with legumes or other staple crops such as cereals. Azotobacter species are inevitably among the important contributors to BNF. Particularly, they are able to supply non-leguminous plants with significant amount of N, in addition to synthesizing plant growth promoting substances, which help increase availability of additional nutrients (P, K, and Zn) for better plant nutrition. Moreover, promising findings were highlighted regarding the ability of Azotobacter to be genetically modified in order to increase their capabilities to fix N2, to improve their colonization ability to plant, growth promotion traits and to improve their formulation effectiveness (Ambrosio et al., 2017; Bageshwar et al., 2017; Romero-Perdomo et al., 2017).
As per available knowledge gained so far, little is known about genes involved in plant-Azotobacter interactions and the key roles they likely perform. Further investigations, both basic and applied, are ultimately needed to find out whether BNF by Azotobacter species is a naturally occurring rhizosphere process that covers the bacteria need for N or a process induced in response to plant signals. Furthermore, the relationship between BNF rates and soil nutrients availability (especially P) needs to be unraveled. It is well established that BNF is limited by the P availability in soils, but the intervention and the limitation mechanism of BNF by P availability is not well discussed.
The compatibility of introduced Azotobacter species among the native microbes is still an unknown aspect to explore, but with the advance of omics technologies, there are opportunities to completely characterize and develop rhizosphere microbiome blueprints for individual crop species. This will help to understand changes in plant-rhizosphere microbiome composition and functions plausibly induced by Azotobacter either individually or in combination with other beneficial species. This will also improve the current understanding of how members of Azotobacter promote plant growth and nutrient use efficiency.
The response of crop to N-fertilizers is well understood, however, combination of microbial inoculants such as Azotobacter and N-fertilizers will require more investigations in order to determine whether the combined use of fertilizers and Azotobacter can ameliorate the BNF process or not. Another important aspect of required research is the production of Azotobacter microbial fertilizers, taking into consideration their ability to be transformed naturally to more resistant forms “cysts.” The induction of these forms could easily be integrated in fermentation processes, which produces a basic microbial bio-fertilizers materials. For this purpose, research must address several technological challenges such as the fermentation process, type of formulations, population of microorganisms and their release system. Thus, the development of a successful and environmentally friendly bioformulations should be made possible by combining interdisciplinary knowledge spanning microbiology and technological aspects. Promoting associative N2-Fixing Azotobacter for sustainable crops production and N nutrition has been an important biotechnological challenging interest. This is a growing and promising market and currently the focus should be on developing innovative and competitive Azotobacter based biofertilizers and this must go through a more substantial investment in R&D and IP.
All authors equally contributed to the preparation of the review, revised the text at different stages of the writing process, and read and approved the current manuscript.
This work was financially supported by OCP Group in a collaboration program between OCP Group, MAScIR Foundation, and Mohammed VI Polytechnic University.
YZ was employed by company Situation Innovation Group–OCP Group.
The remaining authors declare that the research was conducted in the absence of any commercial or financial relationships that could be construed as a potential conflict of interest.
This work is part of a research project, financed by OCP Group and conducted in the Moroccan Foundation for Advanced Science, Innovation and Research (MAScIR) in collaboration with Mohammed VI Polytechnic University.
SNF, symbiotic nitrogen fixation; N, nitrogen; N2, atmospheric nitrogen; BNF, biological nitrogen fixation; EPS, exopolysaccharide; PGPR, plant growth promoting rhizobacterium; P, phosphate; PSMs, phosphate-solubilizing microorganisms; PSB, phosphate-solubilizing bacteria; K, potassium; Zn, zinc; ACC, 1-aminocyclopropane1-carboxylate.
Ahmadi-Rad, S., Gholamhoseini, M., Ghalavand, A., Asgharzadeh, A., and Dolatabadian, A. (2016). Foliar application of nitrogen fixing bacteria increases growth and yield of canola grown under different nitrogen regimes. Rhizosphere 2, 34–37. doi: 10.1016/j.rhisph.2016.08.006
Ahmed, E., and Holmström, S. J. M. (2014). Siderophores in environmental research: roles and applications. Microb. Biotechnol. 7, 196–208. doi: 10.1111/1751-7915.12117
Alkama, N., Ounane, G., and Drevon, J. J. (2012). Is genotypic variation of H+ efflux under P deficiency linked with nodulated-root respiration of N2 fixing common bean (Phaseolus vulgaris L.). J. Plant Physiol. 169, 1084–1089. doi: 10.1016/j.jplph.2012.03.013
Ambrosio, R., Ortiz-Marquez, J. C. F., and Curatti, L. (2017). Metabolic engineering of a diazotrophic bacterium improves ammonium release and biofertilization of plants and microalgae. Metab. Eng. 40, 59–68. doi: 10.1016/j.ymben.2017.01.002
Andjelković, S., Vasića, T., Radovića, J., Babića, S., Markovića, J., Zornića, V., et al. (2018). Abundance of azotobacter in the soil of natural and artificial grasslands. Solut. Proj. Sustain. Soil Manage. 172.
Ansari, M. F., Tipre, D. R., and Dave, S. R. (2015). Efficiency evaluation of commercial liquid biofertilizers for growth of Cicer aeritinum (chickpea) in pot and field study. Biocatal. Agric. Biotechnol. 4, 17–24. doi: 10.1016/j.bcab.2014.09.010
Aquilanti, L., Favilli, F., and Clementi, F. (2004). Comparison of different strategies for isolation and preliminary identification of Azotobacter from soil samples. Soil Biol. Biochem. 36, 1475–1483. doi: 10.1016/j.soilbio.2004.04.024
Archana, D. S., Nandish, M. S., Savalagi, V. P., and Alagawadi, A. R. (2013). Characterization of potassium solubilizing bacteria (KSB) from rhizosphere soil. Bioinfolet Q. J. Life Sci. 10, 248–257.
Arora, M., Saxena, P., Abdin, M. Z., and Varma, A. (2018). Interaction between Piriformospora indica and Azotobacter chroococcum governs better plant physiological and biochemical parameters in Artemisia annua L. plants grown under in vitro conditions. Symbiosis 75, 103–112. doi: 10.1007/s13199-017-0519-y
Asea, P. E. A., Kucey, R. M. N., and Stewart, J. W. B. (1988). Inorganic phosphate solubilization by two Penicillium species in solution culture and soil. Soil Biol. Biochem. 20, 459–464. doi: 10.1016/0038-0717(88)90058-2
Aung, A., Sev, T. M., Mon, A. A., and San Yu, S. (2020). Detection of abiotic stress tolerant Azotobacter species for enhancing plant growth promoting activities. J. Sci. Innov. Res. 9, 48–53.
Azaroual, S. E., Hazzoumi, Z., El Mernissi, N., Aasfar, A., Meftah Kadmiri, I., and Bouizgarne, B. (2020). Role of inorganic phosphate solubilizing Bacilli isolated from moroccan phosphate rock mine and rhizosphere soils in wheat (Triticum aestivum L) phosphorus uptake. Curr. Microbiol. 77, 2391–2404. doi: 10.1007/s00284-020-02046-8
Azcón, R., and Barea, J. M. (1975). Synthesis of auxins, gibberellins and cytokinins by Azotobacter vinelandii and Azotobacter beijerinckii related to effects produced on tomato plants. Plant Soil 43, 609–619. doi: 10.1007/BF01928522
Baars, O., Zhang, X., Gibson, M. I., Stone, A. T., Morel, F. M., and Seyedsayamdost, M. R. (2018). Crochelins: siderophores with an unprecedented iron-chelating moiety from the nitrogen-fixing bacterium Azotobacter chroococcum. Angew. Chem. 130, 545–550.
Baars, O., Zhang, X., Morel, F. M. M., and Seyedsayamdost, M. R. (2015). The siderophore metabolome of Azotobacter vinelandii. Appl. Environ. Microbiol. 82, 27–39. doi: 10.1128/AEM.03160-15
Bageshwar, U. K., Srivastava, M., Pardha-Saradhi, P., Paul, S., Gothandapani, S., Jaat, R. S., et al. (2017). An environmentally friendly engineered Azotobacter strain that replaces a substantial amount of urea fertilizer while sustaining the same wheat yield. Appl. Environ. Microbiol. 83:e00590-17. doi: 10.1128/AEM.00590-17
Bali, A., Blanco, G., Hill, S., and Kennedy, C. (1992). Excretion of ammonium by a nifL mutant of Azotobacter vinelandii fixing nitrogen. Appl. Environ. Microbiol. 58, 1711–1718.
Bargaz, A., Lyamlouli, K., Chtouki, M., Zeroual, Y., and Dhiba, D. (2018). Soil microbial resources for improving fertilizers efficiency in an integrated plant nutrient management system. Front. Microbiol. 9:1606. doi: 10.3389/fmicb.2018.01606
Barker, A. V., Pilbeam, D. J., Hopkins, B. G., and Hopkins, B. G. (2015). Phosphorus. (Boca Raton, FL: CRC Press). doi: 10.1201/b18458-6
Barney, B. M. (2020). Aerobic nitrogen-fixing bacteria for hydrogen and ammonium production: current state and perspectives. Appl. Microbiol. Biotechnol. 104, 1383–1399.
Bartholomew, W. V. (2015). “Mineralization and immobilization of nitrogen in the decomposition of plant and animal residues,” in Soil Nitrogen, eds W. V. Bartholomew and F. E. Clark (New York, NY: John Wiley & Sons, Ltd), 285–306. doi: 10.2134/agronmonogr10.c7
Bashan, Y., de-Bashan, L. E., Prabhu, S. R., and Hernandez, J.-P. (2014). Advances in plant growth-promoting bacterial inoculant technology: formulations and practical perspectives (1998–2013). Plant Soil 378, 1–33. doi: 10.1007/s11104-013-1956-x
Batista Bueno, M., and Dixon, R. (2019). Manipulating nitrogen regulation in diazotrophic bacteria for agronomic benefit. Biochem. Soc. Trans. 47, 603–614. doi: 10.1042/BST20180342
Becking, J. H. (1959). Nitrogen-fixing bacteria of the genus Beijerinckia in South African soils. Plant Soil 11, 193–206. doi: 10.1007/BF01435152
Becking, J. H. (2006). “The family Azotobacteraceae,” in The Prokaryotes: Volume 6: Proteobacteria: Gamma Subclass, eds M. Dworkin, S. Falkow, E. Rosenberg, K.-H. Schleifer, and E. Stackebrandt (New York, NY: Springer), 759–783. doi: 10.1007/0-387-30746-X_26
Behl, R. K., Ruppel, S., Kothe, E., and Narula, N. (2007). Wheat x Azotobacter x VA Mycorrhiza interactions towards plant nutrition and growth- a review. J. Appl. Bot. Food Qual. 81, 95–109.
Bhattacharyya, P., and Jha, D. (2012). Plant growth-promoting rhizobacteria (PGPR): emergence in agriculture. World J. Microbiol. Biotechnol. 28, 1327–1350. doi: 10.1007/s11274-011-0979-9
Bhosale, H. J., Kadam, T. A., and Bobade, A. R. (2013). Identification and production of Azotobacter vinelandii and its antifungal activity against Fusarium oxysporum. J. Environ. Biol. 34, 177–182.
Bindraban, P. S., Dimkpa, C., Nagarajan, L., Roy, A., and Rabbinge, R. (2015). Revisiting fertilisers and fertilisation strategies for improved nutrient uptake by plants. Biol. Fertil. Soils 51, 897–911. doi: 10.1007/s00374-015-1039-7
Bjelić, D., Marinković, J., Tintor, B., Tančić Živanov, S., Nastasic, A., and Mrkovacki, N. (2015). Screening of Azotobacter isolates for PGP properties and antifungal activity. Zb. Matice Srp. Prir. Nauk. 2015, 65–72. doi: 10.2298/ZMSPN1529065B
Brenner, D. J., Staley, J. T., and Krieg, N. R. (2005). “Classification of procaryotic organisms and the concept of bacterial speciation,” in Bergey’s Manual of Systematic Bacteriology: Volume Two: The Proteobacteria, Part A Introductory Essays, eds D. J. Brenner, N. R. Krieg, J. T. Staley, and G. M. Garrity (Boston, MA: Springer), 27–32. doi: 10.1007/0-387-28021-9_4
Buch, A., Archana, G., and Gattupalli, N. K. (2008). Metabolic channeling of glucose towards gluconate in phosphate-solubilizing Pseudomonas aeruginosa P4 under phosphorus deficiency. Res. Microbiol. 159, 635–642. doi: 10.1016/j.resmic.2008.09.012
Castañeda, M., López-Pliego, L., and Espín, G. (2016). “Azotobacter vinelandii Small RNAs: their roles in the formation of CystsCystand other processes,” in Non-coding RNAs and Inter-kingdom Communication, eds A. L. Leitão and F. J. Enguita (Cham: Springer), 67–82. doi: 10.1007/978-3-319-39496-1_4
Chang, W. S., Van De Mortel, M., Nielsen, L., De Guzman, G. N., Li, X., and Halverson, L. J. (2007). Alginate production by Pseudomonas putida creates a hydrated microenvironment and contributes to biofilm architecture and stress tolerance under water-limiting conditions. J. Bacteriol. 189, 8290–8299. doi: 10.1128/JB.00727-07
Chaudhary, D., Narula, N., Sindhu, S. S., and Behl, R. K. (2013). Plant growth stimulation of wheat (Triticum aestivum L.) by inoculation of salinity tolerant Azotobacter strains. Physiol. Mol. Biol. Plants 19, 515–519. doi: 10.1007/s12298-013-0178-2
Chen, Y. P., Rekha, P. D., Arun, A. B., Shen, F. T., Lai, W.-A., and Young, C. C. (2006). Phosphate solubilizing bacteria from subtropical soil and their tricalcium phosphate solubilizing abilities. Appl. Soil Ecol. 34, 33–41. doi: 10.1016/j.apsoil.2005.12.002
Cheng, Q. (2008). Perspectives in biological nitrogen fixation research. J. Integr. Plant Biol. 50, 786–798. doi: 10.1111/j.1744-7909.2008.00700.x
Choudhury, A. T. M. A., and Kennedy, I. R. (2004). Prospects and potentials for systems of biological nitrogen fixation in sustainable rice production. Biol. Fertil. Soils 39, 219–227. doi: 10.1007/s00374-003-0706-2
Connor, G. P., and Holland, P. L. (2017). Coordination chemistry insights into the role of alkali metal promoters in dinitrogen reduction. Catal. Today 286, 21–40. doi: 10.1016/j.cattod.2016.08.014
Creus, C. M., Sueldo, R. J., and Barassi, C. A. (2004). Water relations and yield in Azospirillum inoculated wheat exposed to drought in the field. Can. J. Bot. 82, 273–281.
Das, A. C., and Saha, D. (2007). Effect of diazotrophs on the mineralization of organic nitrogen in the rhizosphere soils of rice (Oryza sativa). J. Crop Weed 3, 69–74.
Datta, C., and Basu, P. S. (2000). Indole acetic acid production by a Rhizobium species from root nodules of a leguminous shrub, Cajanus cajan. Microbiol. Res. 155, 123–127. doi: 10.1016/S0944-5013(00)80047-6
Deshmukh, R. P., Nagre, P. K., Wagh, A. P., and Dod, V. (2014). Effect of different bio-fertilizers on growth, yield and quality of cluster bean. Indian J. Adv. Plant Res. 1, 39–42.
Deubel, A., and Merbach, W. (2005). “Influence of microorganisms on phosphorus bioavailability in soils,” in Microorganisms in Soils: Roles in Genesis and Functions. Soil Biology, Vol. 3, eds A. Varma and F. Buscot (Berlin: Springer), 177–191. doi: 10.1007/3-540-26609-7_9
Diep, C. N., and Hieu, T. N. (2013). Phosphate and potassium solubilizing bacteria from weathered materials of denatured rock mountain, Ha Tien, Kiên Giang province Vietnam. Am. J. Life Sci. 1, 88–92.
Din, M., Nelofer, R., Salman, M., Abdullah, Khan, F. H., Khan, A., et al. (2019). Production of nitrogen fixing Azotobacter (SR-4) and phosphorus solubilizing Aspergillus niger and their evaluation on Lagenaria siceraria and Abelmoschus esculentus. Biotechnol. Rep. 22:e00323. doi: 10.1016/j.btre.2019.e00323
El-Mokadem, M. T., Helemish, F. A., Abou-Bakr, Z. Y. M., and Sheteawi, S. A. (1989). Associative effect of Azospirillum lipoferum and Azotobacter chroococcum with Rhizobium spp. on mineral composition and growth of chickpea (Cicer arietinum) on sandy soils. Zentralbl. Mikrobiol. 144, 255–265. doi: 10.1016/S0232-4393(89)80087-3
El-sayed, S., Hassan, H., and El-Mogy, M. (2014). Impact of Bio- and organic fertilizers on potato yield, quality and tuber weight loss after harvest. Potato Res. 58, 67–81. doi: 10.1007/s11540-014-9272-2
El_Komy, M. H., Hassouna, M. G., Abou-Taleb, E. M., Al-Sarar, A. S., and Abobakr, Y. (2020). A mixture of Azotobacter, Azospirillum, and Klebsiella strains improves root-rot disease complex management and promotes growth in sunflowers in calcareous soil. Eur. J. Plant Pathol. 156, 713–726.
Galindo, E., Peña, C., Núñez, C., Segura, D., and Espín, G. (2007). Molecular and bioengineering strategies to improve alginate and polydydroxyalkanoate production by Azotobacter vinelandii. Microb. Cell Fact. 6:7.
Galloway, J. N., Dentener, F. J., Capone, D. G., Boyer, E. W., Howarth, R. W., Seitzinger, S. P., et al. (2004). Nitrogen cycles: past, present, and future. Biogeochemistry 70, 153–226. doi: 10.1007/s10533-004-0370-0
Gauri, S. S., Archanaa, S., Mondal, K. C., Pati, B. R., Mandal, S. M., and Dey, S. (2011). Removal of arsenic from aqueous solution using pottery granules coated with cyst of Azotobacter and Portland cement: characterization, kinetics and modeling. Bioresour. Technol. 102, 6308–6312. doi: 10.1016/j.biortech.2011.02.037
Glick, B. R., Todorovic, B., Czarny, J., Cheng, Z., Duan, J., and McConkey, B. (2007). Promotion of plant growth by bacterial ACC deaminase. Crit. Rev. Plant Sci. 26, 227–242. doi: 10.1080/07352680701572966
Goel, A., Sindu, S., and Dadarwal, K. (1997). Nodule competence between bacteriocin producing effective and ineffective Rhizobium strains of Vigna. Indian J. Microbiol. 37, 51–52.
Gomare, K. S., Mese, M., and Shetkar, Y. (2013). Isolation of Azotobacter and cost effective production of biofertilizer. Indian J App. Res. 3, 54–56.
Grossmann, K. (2010). Auxin herbicides: current status of mechanism and mode of action. Pest Manag. Sci. 66, 113–120. doi: 10.1002/ps.1860
Gruber, N., and Galloway, J. N. (2008). An Earth-system perspective of the global nitrogen cycle. Nature 451, 293–296.
Gupta, N., Sabat, J., Parida, R., and Kerkatta, D. (2007). Solubilization of tricalcium phosphate and rock phosphate by microbes isolated from chromite, iron and manganese mines. Acta Bot. Croat. 66, 197–204.
Hafez, M., Elbarbary, T. A., Ibrahim, I., and Abdel-Fatah, Y. (2016). Azotobacter vinelandii evaluation and optimization of Abu Tartur Egyptian phosphate ore dissolution. Saudi J. Pathol. Microbiol. 1, 80–93. doi: 10.21276/sjpm.2016.1.3.2
Hakeem, K. R., Sabir, M., Ozturk, M., Akhtar, M. S., and Ibrahim, F. H. (2017). “Nitrate and nitrogen oxides: sources, health effects and their remediation,” in Reviews of Environmental Contamination and Toxicology, Vol. 242, ed. P. de Voogt (Cham: Springer), 183–217. doi: 10.1007/398_2016_11
Halliday, K. J., Martínez-García, J. F., and Josse, E.-M. (2009). Integration of light and auxin signaling. Cold Spring Harb. Perspect. Biol. 1:a001586. doi: 10.1101/cshperspect.a001586
Hamilton, T. L., Ludwig, M., Dixon, R., Boyd, E. S., Dos Santos, P. C., Setubal, J. C., et al. (2011). Transcriptional profiling of nitrogen fixation in Azotobacter vinelandii. J. Bacteriol. 193, 4477–4486. doi: 10.1128/JB.05099-11
Hassen, M. I., Pierneef, R., Swanevelder, Z. H., and Bopape, F. L. (2020). Microbial and functional diversity of Cyclopia intermedia rhizosphere microbiome revealed by analysis of shotgun metagenomics sequence data. Data Brief. 32:106288. doi: 10.1016/j.dib.2020.106288
Hegazi, N. A. (1979). Ecological studies on Azotobacter in Egyptian soils. Zbl. Bakteriol. Naturwiss. 134, 489–497. doi: 10.1016/S0323-6056(79)80072-5
Herridge, D. F., Peoples, M. B., and Boddey, R. M. (2008). Global inputs of biological nitrogen fixation in agricultural systems. Plant Soil 311, 1–18. doi: 10.1007/s11104-008-9668-3
Hindersah, R., Setiawati, M. R., Asmiran, P., and Fitriatin, B. N. (2020). Formulation of Bacillus and Azotobacter consortia in liquid cultures: preliminary research on microbes-coated urea. Int. J. Agric. Syst. 8, 1–10.
Hinsinger, P. (2001). Bioavailability of soil inorganic P in the rhizosphere as affected by root-induced chemical changes: a review. Plant Soil 237, 173–195. doi: 10.1023/A:1013351617532
Honma, M., and Shimomura, T. (1978). Metabolism of 1-aminocyclopropane-1-carboxylic acid. Agric. Biol. Chem. 42, 1825–1831.
Illmer, P., and Schinner, F. (1992). Solubilization of inorganic phosphates by microorganisms isolated from forest soils. Soil Biol. Biochem. 24, 389–395. doi: 10.1016/0038-0717(92)90199-8
Illmer, P., and Schinner, F. (1995). Solubilization of inorganic calcium phosphates—Solubilization mechanisms. Soil Biol. Biochem. 27, 257–263. doi: 10.1016/0038-0717(94)00190-C
Jaipaul, S., Dixit, A., and Sharma, A. (2011). Growth and yield of capsicum (Capsicum annum) and garden pea (Pisum sativum) as influenced by organic manures and biofertilizers. Indian J. Agric. Sci. 81, 637–642.
Jambhulkar, P. P., Sharma, P., and Yadav, R. (2016). “Delivery systems for introduction of microbial inoculants in the field,” in Microbial Inoculants in Sustainable Agricultural Productivity, eds D. Singh, H. Singh, and R. Prabha (New Delhi: Springer), 199–218.
Jean, M.-E., Phalyvong, K., Forest-Drolet, J., and Bellenger, J.-P. (2013). Molybdenum and phosphorus limitation of asymbiotic nitrogen fixation in forests of Eastern Canada: influence of vegetative cover and seasonal variability. Soil Biol. Biochem. 67, 140–146. doi: 10.1016/j.soilbio.2013.08.018
Joshi, P. M., and Juwarkar, A. A. (2009). In vivo studies to elucidate the role of extracellular polymeric substances from Azotobacter in immobilization of heavy metals. Environ. Sci. Technol. 43, 5884–5889. doi: 10.1021/es900063b
József, K., Éva, K., and Ilona, D. (2007). Highly effective rhizobacterial soil inoculants: large-scale production of cyst form cultures in hollow fibre filters. J. Biotechnol. 2:S151. doi: 10.1016/j.jbiotec.2007.07.866
Kannan, T., and Ponmurugan, P. (2010). Response of paddy (Oryza sativa L.) varieties to Azospirillum brasilense inoculation. J. Phytol. 2, 8–13.
Kennedy, C., Rudnick, P., MacDonald, M. L., Melton, T. (2015). “Azotobacter,” in Bergey’s Manual of Systematics of Archaea and Bacteria, eds M. E. Trujillo, S. Dedysh, P. DeVos, B. Hedlund, P. Kämpfer, F. A. Rainey and W. B. Whitman (Atlanta, GA: American Cancer Society), 1–33. doi: 10.1002/9781118960608.gbm01207
Kennedy, I. R., Choudhury, A. T. M., and Kecskés, M. L. (2004). Non-symbiotic bacterial diazotrophs in crop-farming systems: can their potential for plant growth promotion be better exploited? Soil Biol. Biochem. 36, 1229–1244. doi: 10.1016/j.soilbio.2004.04.006
Khan, M., Ahmad, E., Zaidi, A., and Oves, M. (2013). “Functional aspect of phosphate-solubilizing bacteria: importance in crop production,” in Bacteria in Agrobiology: Crop Productivity, eds D. Maheshwari, M. Saraf, and A. Aeron (Berlin: Springer), 237–263. doi: 10.1007/978-3-642-37241-4_10
Khan, M. S., Zaidi, A., Ahemad, M., Oves, M., and Wani, P. A. (2010). Plant growth promotion by phosphate solubilizing fungi – current perspective. Arch. Agron. Soil Sci. 56, 73–98. doi: 10.1080/03650340902806469
Khan, M. S., Zaidi, A., and Wani, P. A. (2007). Role of phosphate-solubilizing microorganisms in sustainable agriculture — A review. Agron. Sustain. Dev. 27, 29–43. doi: 10.1051/agro:2006011
Kirn, J., and Rees, D. C. (1992). Crystallographic structure and functional implications of the nitrogenase molybdenum–iron protein from Azotobacter vinelandii. Nature 360, 553–560. doi: 10.1038/360553a0
Kizilkaya, R. (2009). Nitrogen fixation capacity of Azotobacter spp. strains isolated from soils in different ecosystems and relationship between them and the microbiological properties of soils. J. Environ. Biol. 30, 73–82.
Kumar, V., Behl, R., and Narula, N. (2001). Establishment of phosphate-solubilizing strains of Azotobacter chroococcum in the rhizosphere and their effect on wheat cultivars under green house conditions. Microbiol. Res. 156, 87–93. doi: 10.1078/0944-5013-00081
Kumawat, N., Kumar, R., Kumar, S., and Meena, V. S. (2017). “Nutrient solubilizing microbes (NSMs): its role in sustainable crop production bt - agriculturally important microbes for sustainable agriculture: volume 2: applications in crop production and protection,” in Applications in Crop Production and Protection, eds V. S. Meena, P. K. Mishra, J. K. Bisht, and A. Pattanayak (Singapore: Springer), 25–61. doi: 10.1007/978-981-10-5343-6_2
Ladha, J. K., Tirol-Padre, A., Reddy, C. K., Cassman, K. G., Verma, S., Powlson, D. S., et al. (2016). Global nitrogen budgets in cereals: a 50-year assessment for maize, rice and wheat production systems. Sci. Rep. 6, 19355. doi: 10.1038/srep19355
Lara-López, I. M., and Geiger, O. (2017). Bacterial lipid diversity. Biochim. Biophys. Acta 1862, 1287–1299.
Latef, A. A. H. A., Alhmad, M. F. A., Kordrostami, M., Abo-Baker, A. B. A. E., and Zakir, A. (2020). Inoculation with Azospirillum lipoferum or Azotobacter chroococcum reinforces maize growth by improving physiological activities under saline conditions. J. Plant Growth Regul. 39, 1293–1306.
Lazali, M., and Bargaz, A. (2017). “Examples of belowground mechanisms enabling legumes to mitigate phosphorus deficiency,” in Legume Nitrogen Fixation in Soils with Low Phosphorus Availability: Adaptation and Regulatory Implication, eds S. Sulieman and L.-S. P. Tran (Cham: Springer), 135–152. doi: 10.1007/978-3-319-55729-8_7
Ledbetter, R., Garcia-Costas, A. M., Lubner, C. E., Mulder, D. W., TokminaLukaszewska, M., Artz, J. H., et al. (2017). The electron bifurcating FixABCX protein complex from Azotobacter vinelandii: generation of low-potential reducing equivalents for nitrogenase catalysis. Biochemistry 56, 4177–4190.
Leigh, G. J. (ed.) (2002). “Front matter,” in Nitrogen Fixation at the Millennium (Amsterdam: Elsevier Science), iii. doi: 10.1016/B978-0-444-50965-9.50017-3
Lenart, A. (2012). Occurrence, characteristics, and genetic diversity of Azotobacter chroococcum in various soils of Southern Poland. Polish J. Environ. Stud. 21, 415–424.
Lescourret, F., Magda, D., Richard, G., Adam-Blondon, A.-F., Bardy, M., Baudry, J., et al. (2015). A social–ecological approach to managing multiple agro-ecosystem services. Curr. Opin. Environ. Sustain. 14, 68–75. doi: 10.1016/j.cosust.2015.04.001
Lévai, L., Veres, S., Meszaros, I., Bakonyi, N., and Gajdos, É. (2008). “Interaction between wood Ash and bio fertilizer in crop nutrition,” in Proceedings of the 43rd Croatian and 3rd International Symposium on Agriculture, Opatija.
Little, R., Martinez-Argudo, I., and Dixon, R. (2006). Role of the central region of Nif-L in conformational switches that regulate nitrogen fixation. Biochem. Soc. Trans. 34, 162–164.
Makawi, A. A. M. (1973). The density of Azotobacter in root-free soils and in the rhizosphere of several plants in arid and semi-arid Libyan regions. Z. Bakteriol. Parasitenkd. Infektionskr. Hyg. 128, 135–139. doi: 10.1016/S0044-4057(73)80051-6
Mal, B., Mahapatra, P., and Mohanty, S. (2014). Effect of Diazotrophs and Chemical Fertilizers on Production and Economics of Okra (Abelmoschus esculentus. L.) Cultivars. Am. J. Plant Sci. 05, 168–174. doi: 10.4236/ajps.2014.51022
Maliha, R., Samina, K., Najma, A., Alam, S., and Farooq, L. (2004). Organic acids production and phosphate solubilization by Phosphate Solubilizing Microorganisms (PSM) under in vitro conditions. Pakistan J. Biol. Sci. 7, 187–196. doi: 10.3923/pjbs.2004.187.196
Malik, A. I., Colmer, T., Lambers, H., Setter, T., and Schortemeyer, M. (2002). Short-term waterlogging has long-term effects on the growth and physiology of wheat. New Phytol. 153, 225–236. doi: 10.1046/j.0028-646X.2001.00318.x
Malusá, E., Sas-Paszt, L., and Ciesielska, J. (2012). Technologies for beneficial microorganisms inocula used as biofertilizers. Sci. World J. 2012:491206.
Marciano Marra, L., Fonsêca Sousa Soares, C. R., de Oliveira, S. M., Avelar Ferreira, P. A., Lima Soares, B., de Fráguas Carvalho, R., et al. (2012). Biological nitrogen fixation and phosphate solubilization by bacteria isolated from tropical soils. Plant Soil 357, 289–307. doi: 10.1007/s11104-012-1157-z
Mills, M. M., Ridame, C., Davey, M., La Roche, J., and Geider, R. J. (2004). Iron and phosphorus co-limit nitrogen fixation in the eastern tropical North Atlantic. Nature 429, 292–294. doi: 10.1038/nature02550
Milošević, N., Tintor, B., Protić, R., Cvijanović, G., and Dimitrijević, T. (2012). Effect of inoculation with Azotobacter chroococcum on wheat yield and seed quality. Rom. Biotechnol. Lett. 17, 7352–7357.
Mirzakhani, M., Ardakani, M. R., Rejali, F., Rad, A. H. S., and Miransari, M. (2014). “Safflower (Carthamus tinctorius L.) oil content and yield components as affected by co-inoculation with Azotobacter chroococcum and Glomusintraradices at various N and P levels in a dry climate,” in Use of Microbes for the Alleviation of Soil Stresses: Volume 2: Alleviation of Soil Stress by PGPR and Mycorrhizal Fungi, ed. M. Miransari (New York, NY: Springer), 153–164. doi: 10.1007/978-1-4939-0721-2_9
Mishra, J. and Arora, N. K. (2016). “Bioformulations for plant growth promotion and combating phytopathogens: a sustainable approach,” Bioformulations: For sustainable agriculture. eds (New Delhi: Springer), 3–33. doi: 10.1007/978-81-322-2779-3_1
Mrkovacki, N., and Milic, V. (2001). Use of Azotobacter chroococcum as potentially useful in agricultural application. Ann. Microbiol. 51, 145–158.
Narula, N., Kumar, V., Behl, R., Deubel, A., Gransee, A., and Merbach, W. (2000). Effect of P-solubilizing Azotobacter chroococcum on N, P, K uptake in P-responsive wheat genotypes grown under greenhouse conditions. J. Plant Nutr. Soil Sci. 163, 393–398.
Nosrati, R., Owlia, P., Saderi, H., Rasooli, I., and Ali Malboobi, M. (2014). Phosphate solubilization characteristics of efficient nitrogen fixing soil Azotobacter strains. Iran. J. Microbiol. 6, 285–295.
Núñez, C., Moreno, S., Soberón-Chávez, G., and Espín, G. (1999). The Azotobacter vinelandii response regulator AlgR is essential for cyst formation. J. Bacteriol. 181, 141–148.
Okon, Y., and Itzigsohn, R. (1995). The development of Azospirillum as a commercial inoculant for improving crop yields. Biotechnol. Adv. 13, 415–424. doi: 10.1016/0734-9750(95)02004-M
Omer, A., Emara, H., Zaghloul, R., Abdel, M., and Dawwam, G. (2016). Potential of Azotobacter salinestris as plant growth promoting rhizobacteria under saline stress conditions. Res. J. Pharm. Biol. Chem. Sci. 7, 2572–2583.
Ortiz-Marquez, J. C. F., Do Nascimento, M., de los Angeles Dublan, M., and Curatti, L. (2012). Association with an ammonium-excreting bacterium allows diazotrophic culture of oil-rich eukaryotic microalgae. Appl. Environ. Microbiol. 78, 2345–2352.
Patil, H. J., and Solanki, M. K. (2016). “Microbial inoculant: modern era of fertilizers and pesticides,” in Microbial Inoculants in Sustainable Agricultural Productivity: Vol. 1: Research Perspectives, eds D. P. Singh, H. B. Singh, and R. Prabha (New Delhi: Springer), 319–343. doi: 10.1007/978-81-322-2647-5_19
Patil, S. V., Mohite, B. V., Patil, C. D., Koli, S. H., Borase, H. P., and Patil, V. S. (2020). “Azotobacter,” in Beneficial Microbes in Agro-Ecology, ed. Academic Press (Berlin: Springer), 397–426.
Peoples, M. B., and Herridge, D. F. (2000). “Quantification of biological nitrogen fixation in agricultural systems,” in Nitrogen Fixation: From Molecules to Crop Productivity, eds F. O. Pedrosa, M. Hungria, G. Yates, and W. E. Newton (Dordrecht: Springer), 519–524. doi: 10.1007/0-306-47615-0_293
Phillips, K. A., Skirpan, A. L., Liu, X., Christensen, A., Slewinski, T. L., Hudson, C., et al. (2011). vanishing tassel encodes a grass-specific tryptophan aminotransferase required for vegetative and reproductive development in maize. Plant Cell 23, 550–566. doi: 10.1105/tpc.110.075267
Ponmurugan, K., Sankaranarayanan, A., and Al-Dharbi, N. A. (2012). Biological activities of plant growth promoting azotobacter sp. isolated from vegetable crops rhizosphere soils. J. Pure Appl. Microbiol. 6, 1689–1698.
Poza-Carrión, C., Echavarri-Erasun, C., and Rubio, L. M. (2015). “Regulation of nif gene expression in Azotobacter vinelandii,” in Biological Nitrogen Fixation, (Hoboken, NJ: John Wiley & Sons, Ltd), 99–108. doi: 10.1002/9781119053095.ch9
Qaisrani, M. M., Zaheer, A., Mirza, M. S., Naqqash, T., Qaisrani, T. B., Hanif, M. K., et al. (2019). A comparative study of bacterial diversity based on culturable and culture-independent techniques in the rhizosphere of maize (Zea mays L.). Saudi J. Biol. Sci. 26, 1344–1351.
Rana, K. L., Kour, D., Kaur, T., Devi, R., Yadav, A. N., Yadav, N., et al. (2020). Endophytic microbes: biodiversity, plant growth-promoting mechanisms and potential applications for agricultural sustainability. Antonie Van Leeuwenhoek 113, 1075–1107.
Reed, S. C., Cleveland, C. C., and Townsend, A. R. (2011). Functional ecology of free-living nitrogen fixation: a contemporary perspective. Annu. Rev. Ecol. Evol. Syst. 42, 489–512. doi: 10.1146/annurev-ecolsys-102710-145034
Richardson, A. E., Barea, J.-M., McNeill, A. M., and Prigent-Combaret, C. (2009). Acquisition of phosphorus and nitrogen in the rhizosphere and plant growth promotion by microorganisms. Plant Soil 321, 305–339. doi: 10.1007/s11104-009-9895-2
Ritika, B., and Utpal, D. (2014). Biofertilizer, a way towards organic agriculture: a review. Afr. J. Microbiol. Res. 8, 2332–2343. doi: 10.5897/AJMR2013.6374
Robson, R. L., Eady, R. R., Richardson, T. H., Miller, R. W., Hawkins, M., and Postgate, J. R. (1986). The alternative nitrogenase of Azotobacter chroococcum is a vanadium enzyme. Nature 322, 388–390. doi: 10.1038/322388a0
Rodriguez-Salazar, J., Moreno, S., and Espín, G. (2017). LEA proteins are involved in cyst desiccation resistance and other abiotic stresses in Azotobacter vinelandii. Cell Stress Chaperones 22, 397–408. doi: 10.1007/s12192-017-0781-1
Rodrìguez, H., and Fraga, R. (1999). Phosphate solubilizing bacteria and their role in plant growth promotion. Biotechnol. Adv. 17, 319–339. doi: 10.1016/S0734-9750(99)00014-2
Rojas-Tapias, D., Moreno-Galván, A., Pardo-Díaz, S., Obando, M., Rivera, D., and Bonilla, R. (2012). Effect of inoculation with plant growth-promoting bacteria (PGPB) on amelioration of saline stress in maize (Zea mays). Appl. Soil Ecol. 61, 264–272. doi: 10.1016/j.apsoil.2012.01.006
Romero-Perdomo, F., Abril, J., Camelo, M., Moreno-Galván, A., Pastrana, I., Rojas-Tapias, D., et al. (2017). Azotobacter chroococcum as a potentially useful bacterial biofertilizer for cotton (Gossypium hirsutum): effect in reducing N fertilization. Rev. Argent. Microbiol. 49, 377–383. doi: 10.1016/j.ram.2017.04.006
Roughley, R. J., Gault, R. R., Gemell, L. G., Andrews, J. A., Brockwell, J., Dunn, B. W., et al. (1995). Autecology of Bradyrhizobium japonicum in soybean-rice rotations. Plant Soil 176, 7–14. doi: 10.1007/BF00017670
Rovira, A. D. (1991). “Rhizosphere research - 85 years of progress and frustration,” in The Rhizosphere and Plant Growth: Beltsville Agricultural Research Center (BARC), Beltsville, Maryland, eds D. L. Keister and P. B. Cregan (Dordrecht: Springer), 3–13. doi: 10.1007/978-94-011-3336-4_1
Ruzzi, M., and Aroca, R. (2015). Plant growth-promoting rhizobacteria act as biostimulants in horticulture. Sci. Hortic. 196, 124–134.
Sadoff, H. L. (1975). Encystment and germination in Azotobacter vinelandii. Bacteriol. Rev. 39, 516–539.
Saeed, K., Ahmed, S. A., Hassan, I. A., and Ahmed, P. H. (2015). Effect of bio-fertilizer and chemical fertilizer on growth and yield in cucumber (Cucumis sativus L.) in green house condition. Am. Eurasian J. Agric. Environ. Sci. 15, 353–358.
Saha, B., Saha, S., Das, A., Bhattacharyya, P., Basak, N., Sinha, A., et al. (2017). “Biological nitrogen fixation for sustainable agriculture,” in Agriculturally Important Microbes for Sustainable Agriculture, eds V. S. Meena et al. (Singapore: Springer), 81–128. doi: 10.1007/978-981-10-5343-6_4
Sahoo, R. K., Ansari, M. W., Dangar, T. K., Mohanty, S., and Tuteja, N. (2014). Phenotypic and molecular characterisation of efficient nitrogen-fixing Azotobacter strains from rice fields for crop improvement. Protoplasma 251, 511–523. doi: 10.1007/s00709-013-0547-2
Sandhya, V. Z. A. S., Grover, M., Reddy, G., and Venkateswarlu, B. (2009). Alleviation of drought stress effects in sunflower seedlings by the exopolysaccharides producing Pseudomonas putida strain GAP-P45. Biol. Fertil. Soils 46, 17–26. doi: 10.1007/s00374-009-0401-z
Sangeeth, K. P., Bhai, R. S., and Srinivasan, V. (2012). Paenibacillus glucanolyticus, a promising potassium solubilizing bacterium isolated from black pepper (Piper nigrum L.) rhizosphere. J. Spices Aromat. Crops 21, 118–124.
Saravanan, V. S., Kumar, M. R., and Sa, T. M. (2011). “Microbial zinc solubilization and their role on plants,” in Bacteria in Agrobiology: Plant Nutrient Management, ed. D. K. Maheshwari (Berlin: Springer), 47–63.
Saribay, G. F. (2003). Growth and Nitrogen Fixationdynamics of Azotobacter chroococcum in Nitrogen –Free and OMW Containing Medium. Master’s thesis, Middle East Technical University, Ankara.
Sarkar, A., Mandal, A. R., Prasad, P. H., Maity, T. K., Chandra, B., Viswavidyalaya, K., et al. (2010). Influence of nitrogen and biofertilizer on growth and yield of cabbage. J. Crop Weed 6, 72–73.
Sarma, I., Phookan, D. B., and Boruah, S. (2015). Influence of manures and biofertilizers on carrot (Daucus carota L.) cv. Early Nantes growth, yield and quality. J. Ecofriendly Agric. 10, 25–27.
Sashidhar, B., and Podile, A. R. (2009). Transgenic expression of glucose dehydrogenase in azotobacter vinelandii enhances mineral phosphate solubilization and growth of sorghum seedlings. Microb. Biotechnol. 2, 521–529. doi: 10.1111/j.1751-7915.2009.00119.x
Sashidhar, B., and Podile, A. R. (2010). Mineral phosphate solubilization by rhizosphere bacteria and scope for manipulation of the direct oxidation pathway involving glucose dehydrogenase. J. Appl. Microbiol. 109, 1–12. doi: 10.1111/j.1365-2672.2009.04654.x
Sasson, A., and Daste, P. (1961). Observations concerning the ecology of Azotobacter in the dry soils of Morocco.]. C. R. Seances Soc. Biol. Fil. 155, 1997–2002.
Scervino, J. M., Mesa, M. P., Della Mónica, I., Recchi, M., Sarmiento Moreno, N., and Godeas, A. (2010). Soil fungal isolates produce different organic acid patterns involved in phosphate salts solubilization. Biol. Fertil. Soils 46, 755–763. doi: 10.1007/s00374-010-0482-8
Schulze, J., and Drevon, J.-J. (2005). P-deficiency increases the O2 uptake per N2 reduced in alfalfa. J. Exp. Bot. 56, 1779–1784. doi: 10.1093/jxb/eri166
Segal, H. M., Spatzal, T., Hill, M. G., Udit, A. K., and Rees, D. C. (2017). Electrochemical and structural characterization of Azotobacter vinelandii flavodoxin II. Protein Sci. 26, 1984–1993. doi: 10.1002/pro.3236
Segura, D., Núñez, C., and Espín, G. (2014). “Azotobacter Cysts,” in eLS, ed. John Wiley & Sons, Ltd (Chichester: Wiley). doi: 10.1002/9780470015902.a0000295.pub3
Setubal, J., Dos Santos, P., Goldman, B., Ertesvåg, H., Espin, G., Rubio, L., et al. (2009). Genome sequence of Azotobacter vinelandii, an obligate aerobe specialized to support diverse anaerobic metabolic processes. J. Bacteriol. 191, 4534–4545. doi: 10.1128/JB.00504-09
Sharma, S. B., Sayyed, R. Z., Trivedi, M. H., and Gobi, T. A. (2013). Phosphate solubilizing microbes: sustainable approach for managing phosphorus deficiency in agricultural soils. Springerplus 2:587. doi: 10.1186/2193-1801-2-587
Shirinbayan, S., Khosravi, H., and Malakouti, M. J. (2019). Alleviation of drought stress in maize (Zea mays) by inoculation with Azotobacter strains isolated from semi-arid regions. Appl. Soil Ecol. 133, 138–145.
Shivprasad, S., and Page, W. J. (1989). Catechol formation and melanization by Na -Dependent Azotobacter chroococcum: a protective mechanism for Aeroadaptation? Appl. Environ. Microbiol. 55, 1811–1817.
Singh, A., Maji, S., and Kumar, S. (2014). Effect of biofertilizers on yield and biomolecules of anti-cancerous vegetable broccoli. Int. J. Bioresour. Stress Manag. 5:262. doi: 10.5958/0976-4038.2014.00565.x
Singh, B., Ryan, J., Singh, B., and Ryan, J. (2015a). Managing Fertilizers to Enhance Soil Health Managing Fertilizers to Enhance Soil Health. (Paris: IFA), 1–24.
Singh, S. K., Sharma, H. R., Shukla, A., Singh, U., and Thakur, A. (2015b). Effect of biofertilizers and mulch on growth, yield and quality of tomato in mid-hills of Himachal Pradesh. Int. J. Farm Sci. 5, 98–110.
Singh, G., Biswas, D. R., and Marwaha, T. S. (2010). mobilization of potassium from waste mica by plant growth promoting rhizobacteria and its assimilation by maize (Zea mays) and wheat (Triticum aestivum.): a hydroponics study under phytotron growth chamber. J. Plant Nutr. 33, 1236–1251. doi: 10.1080/01904161003765760
Song, O.-R., Lee, S.-J., Lee, Y.-S., Lee, S.-C., Kim, K.-K., and Choi, Y.-L. (2008). Solubilization of insoluble inorganic phosphate by Burkholderia cepacia DA23 isolated from cultivated soil. Braz. J. Microbiol. 39, 151–156. doi: 10.1590/S1517-838220080001000030
Soumare, A., Diedhiou, A. G., Thuita, M., and Hafidi, M. (2020). Exploiting biological nitrogen fixation: a route towards a sustainable agriculture. Plants 9:1011. doi: 10.3390/plants9081011
Sumbul, A., Ansari, R. A., Rizvi, R., and Mahmood, I. (2020). Azotobacter: a potential bio-fertilizer for soil and plant health management. Saudi J. Biol. Sci. 27, 3634–3640. doi: 10.1016/j.sjbs.2020.08.004
Thamdrup, B. (2012). New pathways and processes in the global nitrogen cycle. Annu. Rev. Ecol. Evol. Syst. 43, 407–428. doi: 10.1146/annurev-ecolsys-102710-145048
Tilman, D., Balzer, C., Hill, J., and Befort, B. L. (2011). Global food demand and the sustainable intensification of agriculture. Proc. Natl. Acad. Sci. U.S.A. 108, 20260–20264. doi: 10.1073/pnas.1116437108
Timmusk, S., Behers, L., Muthoni, J., Muraya, A., and Aronsson, A.-C. (2017). Perspectives and challenges of microbial application for crop improvement. Front. Plant Sci. 8:49. doi: 10.3389/fpls.2017.00049
Trivedi, P., and Sa, T. (2008). Pseudomonas corrugata (NRRL B-30409) mutants increased phosphate solubilization, organic acid production, and plant growth at lower temperatures. Curr. Microbiol. 56, 140–144. doi: 10.1007/s00284-007-9058-8
Vadakattu, G., Roper, M., and Roget, D. (2006). Potential for non-symbiotic N-2-fixation in different agroecological zones of southern Australia. Aust. J. Soil Res. 44, 343–354. doi: 10.1071/SR05122
Velmourougane, K., Prasanna, R., Chawla, G., Nain, L., Kumar, A., and Saxena, A. K. (2019). Trichoderma–Azotobacter biofilm inoculation improves soil nutrient availability and plant growth in wheat and cotton. J. Basic Microbiol. 59, 632–644. doi: 10.1002/jobm.201900009
Walker, J. M. (2011). “Nitrogen fixation,” in Methods in Molecular Biology (Methods and Protocols), Vol. 766, ed. M. Ribbe (Totowa, NJ: Humana Press), 326. doi: 10.1007/978-1-61779-194-9
Wani, S., and Ali, T. (2013). Potential use of Azotobacter chroococcum in crop production: an overview. Curr. Agric. Res. J. 1, 35–38. doi: 10.12944/CARJ.1.1.04
Wani, S. A., Chand, S., Wani, M. A., Ramzan, M., and Hakeem, K. R. (2016). “Azotobacter chroococcum – a potential biofertilizer in agriculture: an overview,” in Soil Science: Agricultural and Environmental Prospectives, eds K. R. Hakeem, J. Akhtar, and M. Sabir (Cham: Springer), 333–348. doi: 10.1007/978-3-319-34451-5_15
Wu, S. C., Cao, Z. H., Li, Z. G., Cheung, K. C., and Wong, M. H. (2005). Effects of biofertilizer containing N-fixer, P and K solubilizers and AM fungi on maize growth: a greenhouse trial. Geoderma 125, 155–166.
Wu, S. C., Luo, Y. M., Cheung, K. C., and Wong, M. H. (2006). Influence of bacteria on Pb and Zn speciation, mobility and bioavailability in soil: a laboratory study. Environ. Pollut. 144, 765–773. doi: 10.1016/j.envpol.2006.02.022
Wyss, O., Neumnn, M. G., and Socolofsky, M. D. (1961). Development and Germination of the Azotobacter cyst. J. Biophys. Biochem. Cytol. 10, 549–573.
Yadav, A. S., and Vashishat, R. (1991). Associative effect of Bradyrhizobium and Azotobacter inoculation on nodulation, nitrogen fixation and yield of mungbean(Vigna radiata(L.) Wilczek). Indian J. Microbiol. 31, 297–299.
Yang, J., Kloepper, J. W., and Ryu, C. M. (2009). Rhizosphere bacteria help plants tolerate abiotic stress. Trends Plant Sci. 14, 1–4. doi: 10.1016/j.tplants.2008.10.004
Yanni, Y., and El-Fattah, F. K. (1999). Towards integrated biofertilization management with free living and associative dinitrogen fixers for enhancing rice performance in the Nile delta. Symbiosis 27, 319–331.
Yi, Y., Huang, W., and Ge, Y. (2008). Exopolysaccharide: a novel important factor in the microbial dissolution of tricalcium phosphate. World J. Microbiol. Biotechnol. 24, 1059–1065. doi: 10.1007/s11274-007-9575-4
Keywords: Azotobacter, biological nitrogen fixation, nitrogenase, nitrogen, plant nutrition, phosphate
Citation: Aasfar A, Bargaz A, Yaakoubi K, Hilali A, Bennis I, Zeroual Y and Meftah Kadmiri I (2021) Nitrogen Fixing Azotobacter Species as Potential Soil Biological Enhancers for Crop Nutrition and Yield Stability. Front. Microbiol. 12:628379. doi: 10.3389/fmicb.2021.628379
Received: 11 November 2020; Accepted: 05 February 2021;
Published: 25 February 2021.
Edited by:
Ashwani Kumar, Dr. Harisingh Gour Central University, IndiaReviewed by:
Jay Prakash Verma, Banaras Hindu University, IndiaCopyright © 2021 Aasfar, Bargaz, Yaakoubi, Hilali, Bennis, Zeroual and Meftah Kadmiri. This is an open-access article distributed under the terms of the Creative Commons Attribution License (CC BY). The use, distribution or reproduction in other forums is permitted, provided the original author(s) and the copyright owner(s) are credited and that the original publication in this journal is cited, in accordance with accepted academic practice. No use, distribution or reproduction is permitted which does not comply with these terms.
*Correspondence: Abderrahim Aasfar, YS5hYXNmYXJAbWFzY2lyLm1h; Issam Meftah Kadmiri, aS5rYWRtaXJpQG1hc2Npci5tYQ==
Disclaimer: All claims expressed in this article are solely those of the authors and do not necessarily represent those of their affiliated organizations, or those of the publisher, the editors and the reviewers. Any product that may be evaluated in this article or claim that may be made by its manufacturer is not guaranteed or endorsed by the publisher.
Research integrity at Frontiers
Learn more about the work of our research integrity team to safeguard the quality of each article we publish.