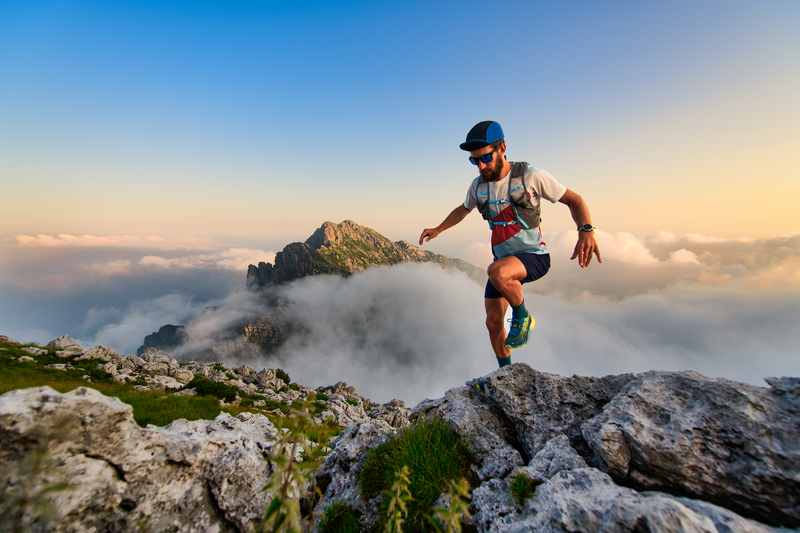
95% of researchers rate our articles as excellent or good
Learn more about the work of our research integrity team to safeguard the quality of each article we publish.
Find out more
REVIEW article
Front. Microbiol. , 26 March 2021
Sec. Microbial Symbioses
Volume 12 - 2021 | https://doi.org/10.3389/fmicb.2021.625752
This article is part of the Research Topic Multilateral Interactions In The Rhizosphere View all 13 articles
The rhizosphere is a dynamic ecosystem shaped by complex interactions between plant roots, soil, microbial communities and other micro- and macro-fauna. Although studied for decades, critical gaps exist in the study of plant roots, the rhizosphere microbiome and the soil system surrounding roots, partly due to the challenges associated with measuring and parsing these spatiotemporal interactions in complex heterogeneous systems such as soil. To overcome the challenges associated with in situ study of rhizosphere interactions, specialized plant growth chamber systems have been developed that mimic the natural growth environment. This review discusses the currently available lab-based systems ranging from widely known rhizotrons to other emerging devices designed to allow continuous monitoring and non-destructive sampling of the rhizosphere ecosystems in real-time throughout the developmental stages of a plant. We categorize them based on the major rhizosphere processes it addresses and identify their unique challenges as well as advantages. We find that while some design elements are shared among different systems (e.g., size exclusion membranes), most of the systems are bespoke and speaks to the intricacies and specialization involved in unraveling the details of rhizosphere processes. We also discuss what we describe as the next generation of growth chamber employing the latest technology as well as the current barriers they face. We conclude with a perspective on the current knowledge gaps in the rhizosphere which can be filled by innovative chamber designs.
Roots are not only vital for anchorage and for acquisition of water and nutrients from the soil, but are also engaged in complex physical and chemical interactions with the soil. Plant roots release approximately 11–40% of their photosynthetically fixed carbon, commonly known as root exudates, into the soil (Sasse et al., 2018; Zhalnina et al., 2018a). Root exudates and mucilage act as nutrient sources and as signaling molecules for soil microorganisms, thus shaping the microbial community in the immediate vicinity of the root system (Venturi and Keel, 2016). In turn, microbial processes promote plant growth by aiding in nutrient acquisition, plant growth hormone production and bio-control of plant pathogens (Afzal et al., 2019). The physicochemical characteristics of the surrounding soil are also affected by interactions between roots and the microbial community. This interplay between the different rhizosphere components is affected by spatio-temporal processes, which culminates in dynamic feedback loops that maintain the complex rhizosphere environment with physical, chemical and biological gradients that are distinct from the bulk soil (Six et al., 2004; Koebernick et al., 2017). Understanding these intricate rhizosphere relationships is vital in devising strategies to increase plant productivity and comprehend localized biogeochemical processes.
In many rhizosphere studies, the use of pots and containers is predominant as it allows the plants to be cultivated under controlled conditions and at low cost. Compared to field studies, growth of plants in defined spaces (e.g., pots) also offers advantages in ease of handling, monitoring and sampling (Neumann et al., 2009). Much of what we know of the rhizosphere microbiome has resulted from such pot-grown plants. However, since the rhizosphere and roots are still out of view in the soil, destructive sampling of the root is required prior to analysis. Destructive sampling may result in the loss of three-dimensional (3D) spatial information on rhizosphere processes over time, which is increasingly being recognized as a critical parameter.
On the other hand, soil free techniques such as hydroponics and aeroponics can provide visual access to the rhizosphere circumventing the need for destructive sampling. Other alternatives are gel-based substrates which can maintain rhizosphere transparency as well as the 3D architecture of roots and have been applied successfully in high throughput imaging, phenotyping and trait mapping platforms (Iyer-Pascuzzi et al., 2010; Topp et al., 2013). Nonetheless, the root phenotype and traits of plants grown under soil-free conditions are known to differ from those of soil-grown plants (Kuijken et al., 2015). These soil substitutes do not also accurately simulate the heterogeneous nature of soil aggregates, thus complicating extrapolations for field relevance. Sophisticated imaging approaches such as magnetic resonance imaging (Metzner et al., 2014; Popova et al., 2016; van Dusschoten et al., 2016) and X-ray computer tomography (Mooney et al., 2012; Helliwell et al., 2013) can be used to analyze root systems in the soil with minimal disturbance but they are low throughput, expensive and may not be easily accessible (Oburger and Schmidt, 2016; Morris et al., 2017). It is apparent that structural changes in design catered to solving specific challenges in the rhizosphere are indeed necessary.
To overcome these challenges relating to the rhizosphere in soil, specialized plant growth chamber systems have been designed, and successful implementation has led to multiple variations of similar designs. These specialized systems often have a visible rhizosphere which enables coupling with other technologies thereby increasing the breadth of experimental techniques applicable to the rhizosphere system. This review discusses representative growth chamber systems designed to study major rhizosphere processes and interactions in soil. Growth platforms resembling conventional containers such as pots and tubes are not covered. Specifically, the reviewed growth systems are selected based on the following criteria: (1) the growth chamber is amenable for use with soil/soil-like substrates (e.g., vermiculite or sand) and therefore, hydroponics, aeroponics and agar/gel-based systems are not discussed except in microfluidic-based platforms, (2) it is built with the intention to maintain growth of the plant and has architectural features distinct from conventional pots, and lastly (3) it is able to be set up in a laboratory; i.e., field measurement systems and observation platforms are not included. For instance, a minirhizotron, consisting of a camera mounted in a glass tube submerged in the soil which provides non-destructive root imaging over time (Taylor et al., 1990) will not be discussed as it is out of the scope of this review. Through our assessment of lab-based chamber systems, we identify unique advantages and challenges associated with each system (Table 1). We hope that future fabrication designs can benefit and improve on designs that work well. Lastly, we offer our perspectives on areas in which technological advances are needed to fill current knowledge gaps.
Table 1. Key attributes of different growth chambers designed to study rhizosphere processes and interactions.
Figure 1. Representative figure of major rhizosphere processes in the soil discussed in this review. (A) Root system architecture is concerned with structural features of the root and responds to with environmental stimuli. (B) The rhizosphere produces photosynthetically fixed carbon that exudes into the soil and influences soil physicochemical gradients. (C) Free-living or parasitic nematodes interact with the rhizosphere via signaling interactions. (D) Mycorrhizal fungi create intimate relationships with the roots and engage in nutrient exchange. (E) Bacterial composition is distinct upon different parts, age, type of the roots.
Figure 2. Schematic diagrams of representative growth chamber designs. Further description and characteristics are listed in Table 1. (A) Rhizotron/Rhizobox set up, (B) Rhizobox with side-compartment, (C) vertical root mat chambers; a modular option is show where the plant can be pre-grown in a separate compartment and transplanted afterward onto the main examination chamber, inset shows a modular set up option, (D) horizontal root mat in rhizobox, (E) Mycorrhizal compartments, (F) split-root systems shown here in a rhizobox set up; (G) Nylon bag to separate root and root-free soil; roots may be restricted in the bag or the soil may be protected from root penetration by the bag.
In studying rhizosphere processes, the myriad of complex interactions among members of the rhizosphere are often dissected to two interacting variables such as root-and-soil or root-and-microbes, etc. Each of these interactions inherently operates under distinct parameters and requires specifically designed platforms to effectively answer different research questions. This review is structured in a way that first describes each rhizosphere process briefly and then reports on the specific growth chamber systems designed to facilitate experiments for answering related research questions. The major rhizosphere processes discussed below include root system architecture, physicochemical gradients in the soil, exudation patterns by the roots and interactions between roots and nematodes, fungi or bacteria.
Root system architecture (RSA) encompasses structural features that provide spatial configuration such as root length, width, spread and number (Khan et al., 2016; Figure 1) and is an important rhizosphere parameter in regulating soil porosity, and nutrient and water uptake efficiency by plants (Helliwell et al., 2017; Fang et al., 2019). Plants have been observed to “sense” and direct root growth toward nutrient sources in soil, and the RSA of a plant exhibits great malleability in response to environmental stimuli (changes in nutrients, pH, soil moisture, and temperature) which in turn, influences microbial communities (Bao et al., 2014; Saleem et al., 2018). For instance, bean plants grew deeper roots under drought conditions to enhance water foraging capabilities while low phosphate (P) conditions stimulated the formation of dense lateral roots involved in P uptake from upper soil layers (Ho et al., 2005). Given that most soils are heterogenous, understanding the RSA of plants becomes critical in improving resource use efficiency and agricultural yields (Ingram and Malamy, 2010; Khan et al., 2016). Often, RSA in pot-grown plants is investigated by excising the roots via mechanical means such as root washing or blowing with compressed air (Judd et al., 2015). These methods are, however, time-consuming, cause inevitable damage of fine root hairs and result in loss of spatial and temporal information (Judd et al., 2015).
An appealing alternative for studying RSA is the use of rhizotrons. Rhizotrons were initially constructed as underground facilities designed for viewing and measuring roots in the field (Klepper and Kaspar, 1994). In the lab, the rhizotron implies a chamber constructed using two vertical sheets with at least one or both of the sheets being transparent and/or removable (Figure 2A). This allows repeated visual inspections of individual roots; a feature unachievable with destructive sampling. In some cases, the word “rhizobox” is used for a similar set up although this was first introduced in as compartmentalized systems to separate the root and soil compartments (Kuchenbuch and Jungk, 1982). Rhizotrons/rhizoboxes are often constructed with PVC or acrylic materials and come in many sizes to accommodate different plants with soil or soil-less substrates (Neumann et al., 2009). Root growth and morphology in the rhizotron can be tracked by a variety of methods ranging from manual tracing onto a plastic sheet, using handheld or flatbed scanners to fully automated time-lapse imaging camera systems (Mohamed et al., 2017). Data can be subsequently analyzed with a wide range of software packages (Kuijken et al., 2015). Affordable and robust RSA imaging platforms using rhizotrons have also been developed for increased accessibility in low-income countries (Bontpart et al., 2020).
The versatile construction of a rhizotron design for RSA studies has inspired many variations. For instance, ara-rhizotrons were designed to enable the study of 3D canopy competition with simultaneous root growth observation in an Arabidopsis plant population (Devienne-Barret et al., 2006). The horizontal and radial design of HorhizotronTM and mini-Horhizotron consisting of transparent quadrants attached to a central chamber were developed to study lateral growth of roots in a semi-3D space and to perform post-transplant assessment (Wright and Wright, 2004; Judd et al., 2014). The separated quadrants can also be used with different soil substrates simultaneously to study substrate effects on root growth (Wright and Wright, 2004). A rhizotron fitted with water-tight gasket seals has also been used successfully to investigate the RSA of plants under water-logged conditions (Busch et al., 2006).
Despite the continuous real-time visual read-out, most rhizotron designs suffer from inevitable loss of information from roots occluded by soil particles. The GLO-Roots system overcomes this by imaging from both sides of the rhizotron while using bioluminescent roots to create higher contrast against the soil, enabling quantitative studies on RSA (Rellán-Álvarez et al., 2015). Following advances in engineering and device fabrication, more rhizotron variants adapted to specific plant growth conditions can be envisioned.
In a typical topsoil, approximately half is composed of solid minerals and organic matter while the rest is a fluctuating composition of water and gas filled spaces influenced by environmental conditions and uptake/release of solutes from plants (O’Donnell et al., 2007). Changes in gaseous and hydrologic parameters, such as ions, O2 and moisture among others, create a spatially complex environment that influences microbial communities and overall plant health. These physicochemical fluxes are heterogeneously distributed along roots and vary with root types and zones (Neumann et al., 2009). Often, they exist as gradients in the rhizosphere (Kuzyakov and Razavi, 2019), thus emphasizing the need for non-destructive sampling in order to accurately capture processes occurring at biologically relevant times and scales.
Rhizotron chambers with a visually accessible rhizosphere allows in situ and continuous mapping of these gradients in the soil through the use of different types of imaging methods. For instance, photoluminescence-based optical sensors enable in situ, repeated detection of small molecule analytes in addition to pH (Blossfeld et al., 2010), O2 (Frederiksen and Glud, 2006) and NH4 (Santner et al., 2015). Methods like zymography to detect enzyme activity (Spohn et al., 2013) and diffusive gradients in thin film (Santner et al., 2012; Valentinuzzi et al., 2015) can be used to map solute concentrations in the soil down to sub-mm scales with high spatial resolution more realistically than traditional destructive approaches. For example, transport and distribution of water in the rhizosphere soil has been imaged on both 2D and 3D planes by coupling a rhizotron with neutron radiography and tomography, respectively (Esser et al., 2010) and showed varying moisture gradients along the root system with higher water uptake at the rhizosphere compared to bulk soil. On the other hand, if the rhizotron slabs are thin enough (∼4 mm), even simple imaging solutions based on light transmission can be set up to capture water uptake by roots in sand (Garrigues et al., 2006). Despite trade-offs in method sensitivity between these two studies, a rhizotron set up is critical in both designs and illustrates its adaptability to multiple equipment.
Roots exude a substantial amount of photosynthetically fixed organic carbon into the soil consisting of a wide variety of compounds such as sugars, organic acids, and primary and secondary metabolites (Sasse et al., 2018; de la Fuente Cantó et al., 2020). Together with mucilage and border cells (which are mainly expelled from root tips), root exudates provide a major source of nutrients for the rhizosphere microbiome (Figure 1). Root exudation is regulated under genetic control (i.e., genotype, root type and developmental stage) (Canarini et al., 2019) as well as in response to environmental conditions in the soil such as nutrient limitations or increase in toxicity (van Dam and Bouwmeester, 2016). Exudate patterns are also recognized as one of the strongest drivers shaping the rhizosphere microbiome (Dessaux et al., 2016; Zhalnina et al., 2018b; de la Fuente Cantó et al., 2020). As a central player in the rhizosphere ecosystem, it is imperative to understand root exudation patterns to unravel subsequent impacts to the surrounding soil and microbial community.
Improvements in analytical instrumentation have made it possible to move from targeted to untargeted explorations with mass spectrometry to create root exudate fingerprints in its entire complexity (Oburger and Schmidt, 2016). Regardless, the impact of such techniques relies partly on our exudate sampling techniques. Detection of exudates in real-time is difficult due to rapid biotransformation and sorption to the soil matrix. As such, common collection methods rely on root washing in hydroponic systems to overcome complications in the soil matrix and preserve native exudation profiles. However, a comparison between a soil-based collection method and hydroponic methods showed varied responses particularly in amino acid exudation although the underlying cause was not elucidated (Oburger et al., 2013). It is possible that the differing growth conditions between hydroponics and soil, which include differences in gas concentrations, mechanical impedance and microbial spatial composition, can elicit differing root exudation responses to the same environmental stimuli.
Rhizoboxes offer the advantage of localized sampling in soil using sorption media such as paper and membrane filters, compound specific ion exchange binding resin or micro-suction cups placed closed to root zones of interest to collect exudates (Kamh et al., 1999; Neumann et al., 2009, 2014). Moreover, in a rhizobox fitted at the bottom with a porous root-impenetrable membrane, a root mat is allowed to be formed which is then further transferred onto a collection compartment (Figure 2D; Chaignon et al., 2002; Chaignon and Hinsinger, 2003). The collection compartment containing soil could then be cut into thin slices (1–3 mm) parallel to the membrane to represent differing distances from the rhizosphere (Neumann et al., 2009). While this approach can be used to investigate exudate release and sorption under soil conditions, the root mat growth generalizes exudate production in terms of the whole root system and occludes spatial exudation patterns. In a hybrid set up by Oburger et al. (2013), the rhizobox is transplanted to a second specialized rhizobox for continued vertical root growth. This specialized rhizobox consists of a nylon membrane (30 μm pore size) close to the transparent side to restrict root growth into the soil except for root hairs (Figure 2C). This creates a vertical flat root mat onto which localized exudate samples can be collected. A comparison of this novel set up to conventional collection methods showed that amino acid exudation rates were most varied among the different methods (Oburger et al., 2013), further highlighting the need for specialized chambers.
Nonetheless, successful implementation of these chambers is still limited to fast-growing plants which can form active root mats. The high density of root mats could also lead to unnatural root exudate levels and an overestimation of rhizosphere effects. In addition, care has to be given to the choice of membrane as selective sorption of certain root exudates onto the membrane may also occur (Neumann et al., 2009).
Free-living nematodes are ubiquitous in the soil. They are beneficial to the plants by playing a role in nutrient cycling and in defense against insects and microbial infections through signaling interactions with the roots (Rasmann et al., 2005; Manosalva et al., 2015; Figure 1). Conversely, infections by parasitic nematodes in the roots increase the plant’s susceptibility to stress and other pathogenic bacteria, fungi, and viruses creating major losses in crop productivity (Powell, 1971; O’Callaghan et al., 2018). With an impending rise in nematode infections due to climate change, understanding nematode behavior and interactions in the rhizosphere becomes important to develop appropriate biocontrol methods to ensure long term food security (O’Callaghan et al., 2018).
Traditional nematode studies are performed in petri dishes with agar or culture media (Dinh et al., 2014; O’Callaghan et al., 2018). However, these substrates do not accurately emulate the physical textures and heterogeneity of soil and create homogenous solute and temperature gradients which could impact nematode behavior and interactions with the roots (Lockery et al., 2008). Indeed, nematode motility speed and dispersal decreased in substrates more closely mimicking sand (Hapca et al., 2007). On the other hand, studying nematode behavior in the soil is a difficult endeavor as its near-transparent body and small size makes it almost indistinguishable from soil particles. Cross-sectioning and staining infected roots make it possible for nematode visualization but they are destructive and provide only static snapshots of cellular changes or nematode behavior during infections (Dinh et al., 2014).
On the other hand, microscopy rhizosphere chambers provide non-invasive detection and observation of nematode activity in the rhizosphere (Froelich et al., 2011; Kooliyottil et al., 2017). The roots in these chambers grow between a glass slide and a nylon membrane (unknown pore size) (Figure 2C). The membrane restricts movement of roots except root hairs into the soil while the transparent glass enables microscopy of the roots at high resolution (Froelich et al., 2011). Coupled with fluorescently stained nematodes, microscopy rhizosphere chambers allowed for non-destructive in situ observations of nematode infection in its host species over the entire life of the parasite (Dinh et al., 2014; Kooliyottil et al., 2016).
Nonetheless, staining nematodes is an additional challenge as nematode cuticles are impermeable to stains (O’Callaghan et al., 2018). This can, however, be alleviated by using advanced imaging technologies which eliminates the need for staining. A recent study demonstrated live screening of nematode-root interactions in a transparent soil-like substrate through the use of label-free light sheet imaging termed Biospeckle Selective Plane Illumination Microscopy (BSPIM) coupled with Confocal Laser Scanning Microscopy (Downie et al., 2014; O’Callaghan et al., 2018). Using this set up, researchers were able to monitor roots for nematode activity at high resolution and suggest its possible use in rapid testing of chemical control agents against parasitic nematodes in soil-like conditions (O’Callaghan et al., 2018).
Fungal communities in the rhizosphere are involved in the degradation of organic matter in the soil and subsequent nutrient turnover affecting plant health as well as the microbial community (Buée et al., 2009). Fungal biomass often reaches a third of total microbial biomass carbon (Joergensen, 2000) and almost all terrestrial plants are able to form symbiotic associations with mycorrhizal fungi (Van Der Heijden et al., 2016; Figure 1). The majority of these associations are with arbuscular mycorrhiza fungi (AMF) (Smith and Read, 2008) which penetrate into root cortex cells to form highly branched structures (Harrison, 2005). The investment of photosynthetic carbon by plants to AMF is rewarded with increased nutrient availability made possible by the extended hyphal network in the soil. For instance, up to 90% of phosphorus uptake in plants can be contributed by symbiosis with AMF (Ferrol et al., 2019). AMF networks in the soil also influence water retention and soil aggregation further impacting plant growth (Augé, 2004). Moreover, next-generation sequencing technologies and advances in imaging techniques have greatly improved our knowledge on the taxonomical and functional properties of fungal communities in the rhizosphere (Oburger and Schmidt, 2016). However, these methods are optimized for fine scale (millimeter) analysis and are not capable of assessing the foraging capabilities of hyphal networks which can span across centimeter to meter scales.
Toward this end, several researchers have used compartment setups with physical barriers created by 20–37 μm nylon membranes (Figure 2E) which restrict movement of roots but not mycorrhizal fungi. This separation creates root-free and plant-free soil compartments connected only by mycorrhizal fungi to examine the transport of various compounds across these compartments. Using this set up, the importance of mycorrhizal fungi in the flow of different elements such as carbon (Kaiser et al., 2015), nitrogen (Tanaka and Yano, 2005) and phosphorus (Wang et al., 2016) between plants, soil and microbes over centimeter distances have been validated. Repeated disruption of the hyphal connections also led to a decreased resistance in plants to drought stress (Zou et al., 2015). The membranes can also be placed horizontally to create different depth gradients to investigate hyphal contributions to water uptake (Ruiz-Lozano and Azcón, 1995). In some studies, an additional 1.5–3 mm air gap is created between two membranes with a wire net to restrict solute movement between two chambers (Tanaka and Yano, 2005; Zhang et al., 2010; Koegel et al., 2013; Figure 2E). A common feature of these set ups is the size-exclusion membranes which proved to be critical in distinguishing fungal hyphae processes in the rhizosphere soil.
In addition to AMF interactions, a split root set up, which separates the roots of one plant into halves, can be introduced to investigate the systemic response of plants (Figure 2F; Vierheilig et al., 2000). In essence, the split-root system directs the growth of the roots to generally two different growth conditions and enables the investigation of whether a local stimuli (microbial interactions, nutrient limitations, etc.) have a local or global response which can be observed at the root or shoot level (Agapit et al., 2020). Split-root systems are widely studied (Larrainzar et al., 2014; Saiz-Fernández et al., 2021) and have been adapted to rhizoboxes (Zhu and Yao, 2004; Mitchell et al., 2018) as well as to pots and tubes (Kosslak and Bohlool, 1984; Marschner and Baumann, 2003).
In the rhizosphere, plants host a wide diversity of bacteria on the surface of the root (epiphytes) as well as within roots in the vascular tissue (endophytes). Due to its abundance and importance, the bacterial community in the rhizosphere is perhaps the most widely studied among other microbial members in the rhizosphere ecosystem. While the study of endophytic bacteria requires inevitable destructive sampling due to its localization, several non-destructive approaches have been developed to study microbes inhabiting the rhizoplane.
One of the most widely studied plant-microbe interactions in the rhizosphere is that of the symbiotic relationship between legumes and rhizobia (Hirsch et al., 2001). Once a potential nodule forming bacteria is isolated, it is often required to authenticate its nodule forming phenotype by inoculating on host plants. However, conventional methods such as the use of soil pouches do not allow long term incubation, while “Leonard jars,” consisting of two stacked glass jars forming the top soil layer and the bottom nutrient solution layer, can be expensive and time consuming (Yates et al., 2016). A recent study challenges this by describing the use of clear plastic CD cases as mini-rhizotrons with potential for use in phenotyping root traits such as legume formation, and demonstrated innovation that democratizes research opportunities in rhizosphere research (Cassidy et al., 2020).
Other microbial interactions in the rhizosphere, however, may not result in visible changes to the root system and often rely on next-generation omics technologies. As such, physical separation of the rhizosphere from the bulk soil becomes paramount in elucidating changes to microbial community and interactions. One approach to this end is the use of nylon bags with differing pore sizes (10–50 μm) (Figure 2G). The nylon bag restricts the movement of roots and the soil inside the bag is then regarded as the rhizosphere soil to compare against the surrounding root-free bulk soil (Yevdokimov et al., 2006; Shrestha et al., 2010; Nie et al., 2015). Developing further on this concept, Wei et al. (2019) designed a specialized rhizobox that allowed repeated non-destructive sampling by adding individual nylon bags of root-free soil surrounding the root compartment which are then used as a proxy for the rhizosphere (Wei et al., 2019).
These methods allowed easy distinction of the rhizosphere and the bulk soil but, we now know that the rhizosphere community is not only distinct from the bulk soil but also varies with type, part and age of the root, largely as a consequence of varying root exudation patterns (Sasse et al., 2018). Studying this phenomenon in situ in the soil requires separation of desired roots from others without disturbance to plant growth or soil. To address this, researchers have used a modified rhizobox design with a side compartment to regulate root growth and quarantine specific roots from the main plant chamber (Figure 2B). This additionally creates easy distinction between old and new roots and allows testing on specific quarantined roots despite plant age. A study using this set up showed specific microbial chemotaxis toward different exudates (sucrose or tryptophan) on an individual root (Jaeger et al., 1999) whereas another showed spatial and temporal regulation of niche differentiation in microbial rhizosphere guilds (Nuccio et al., 2020). Similar physical perturbations to regulate root growth in response to microbial stimuli have also been applied in the microscale and are explored in the next section.
Our assessment of the major growth chambers showed that most of the systems applied share similarities in basic structural components such as in the use of two parallel sheets in rhizobox-based devices. While these growth chambers brought many of the rhizosphere processes to light, limitations do exist. One limitation is with the scale of applicability. Most of these growth systems are mesoscale and can easily reproduce pot-scale studies (Devienne-Barret et al., 2006) but may not be easily translatable to interactions occurring at the microscale nor recapitulate processes occurring at field-relevant scale. The next section describes advances in technology resulting in a new wave of unique devices making use of microfluidic processes and fabricated ecosystems which are specifically made to investigate specific rhizosphere processes.
A complex web of biochemical processes and interactions occur in microscale dimensions in the rhizosphere. Having the ability to interrogate and manipulate these microscale processes and environmental conditions with high spatiotemporal resolution will elucidate mechanistic understanding of the processes. Microfluidics has proven to be a powerful approach to minimize reagent usage and to automate the often-repetitive steps. The microscale of the channels also allows precise control of reproducible conditions utilizing the laminar flow and automated fluidic operations (Figures 3A,B). In addition, the microfluidic devices are well integrated with conventional imaging techniques by using a glass slide or coverslip as a substrate bonded with polydimethylsiloxane (PDMS). These characteristics, as well as the ability to rapidly prototype and reproducibly manufacture using soft lithography technique, have enabled new ways of interrogating and studying the rhizosphere environment in a reproducible manner.
Figure 3. Next generation of growth chamber systems developed to study rhizosphere interactions. (A) Schematic diagram of a representative microfluidic device in studying root response to environmental stimuli. The media inlet is controlled by various pneumatic methods such as syringes and automated Quake-valve system. (B) An image of a RootChip, demonstrating the increased throughput by the parallel growth of 8 Arabidopsis seedlings on a single device (a figure by Massalha et al., 2017). (C) Schematic diagram of an EcoPOD showing three dedicated units with different features; sensors monitor operational parameters closely and the central hub located at the base of the atmospheric unit controls the EcoPOD. (D) Schematic diagram of an EcoFAB with soil sealed to a glass slide. (E) An image of the EcoFAB bonded to glass slide with Brachypodium distachyon grown in soil.
Many of the microfluidic devices used for studying the rhizosphere share a similar design concept (Khan et al., 2019). They have an opening port, sometimes with pipette tips inserted into the PDMS body where the seed of the seedling rests and a microchannel where the primary root grows into. The dimension of the channel depends on the type and age of the plant. For example, an Arabidopsis thaliana’s seedling is typically grown in a microfluidic device up to 10 days, with chamber dimension around 150 to 200 μm in height, whereas the Brachypodium distachyon seedling chamber is 1 mm in height due to its thicker roots (Massalha et al., 2017; Khan et al., 2019). Media and/or inoculation of the microbiome is achieved through additional channels to the main chamber. The PDMS body with the channels is typically bonded on a 50 mm by 75 mm microscope slide, and is made to accommodate multiple plants to increase throughput. Automated control offers the ability for continuous imaging and manipulation of media conditions with high temporal resolution.
One notable example of a microfluidic device for rhizosphere studies is the RootChip, which uses the micro-valves in a PDMS device to control the fluidics (Unger et al., 2000; Grossmann et al., 2011). The first study using the RootChip grew 8 Arabidopsis plants on a single device with micro-valves (Grossmann et al., 2011) but by the second iteration, the throughput has been doubled (Jones et al., 2014; Keinath et al., 2015) indicating rapid technological advances in the field. In addition, all these studies demonstrated spatiotemporal imaging at single-cell resolution and dynamic control of the abiotic environments in the rhizosphere.
Another microfluidics-specific application to rhizosphere study is to use the laminar flow to generate the spatially precise and distinct microenvironment to a section of the root as demonstrated by Meier et al. (2010). A young Arabidopsis’ seedling was sandwiched and clamped between two layers of PDMS slabs with microchannel features to tightly control synthetic plant hormone flow with 10 to 800 μm resolution to the root tip area, enabling observations of root tissues’ response to the hormones. As many root bacteria produce auxin to stimulate the interactions with the root, this study showed the possible mechanism of microbiome inducing the interaction by stimulating root hair growth. Another application of laminar flow utilized the RootChip architecture by adding the two flanking input channels to generate two co-laminar flows in the root chamber, subjecting a root to two different environmental conditions along the axial direction to study root cells adaptation to the microenvironment at a local level (Stanley et al., 2018). These studies revealed locally asymmetrical growth and gene pattern regulations in Arabidopsis root in response to different environmental stimuli.
Microfluidic platforms have also been successfully employed to study the interactions between the root, microbiome and nematodes in real time (Parashar and Pandey, 2011; Massalha et al., 2017; Aufrecht et al., 2018). In the systems, additional vertical side channels are connected perpendicularly to the main microchannel to enable introduction of microorganisms and solutes to the roots in a spatially and temporally defined manner (Parashar and Pandey, 2011; Aufrecht et al., 2018). A recent microfluidic design incorporated a nano-porous interface which confines the root in place while enabling metabolite sampling from different parts of the root (Patabadige et al., 2019). These studies demonstrated the potential of microfluidics in achieving spatiotemporal insights into the complex interaction networks in the rhizosphere.
Despite several advantages of microfluidics in rhizosphere research as described above, some challenges remain. All the microfluidic applications grow plants in hydroponic systems where clear media is necessary for the imaging applications and packing solid substrates in the micro-channels is not trivial. The microscale of the channels limits the applications of these devices to young seedlings. Thus, interrogating the microscale interactions in bigger, more developed plants is not possible with current microfluidic channel configurations. In addition, technical challenges such as operating the micro-valves and microfabrication present a barrier to device design and construction for non-specialists.
Fabricated ecosystems aim to capture critical aspects of ecosystem dynamics within highly controlled laboratory environments (Zengler et al., 2019). They hold promise in accelerating the translation of lab-based studies to field applications and advance science from correlative and observational insights to mechanistic understanding. Pilot scale enclosed ecosystem chambers such as EcoPODs, EcoTrons and EcoCELLs have been developed for such a purpose (Griffin et al., 1996; Lawton, 1996; Ke et al., 2020). These state-of-the-art systems offer the ability to manipulate many parameters such as temperature, humidity, gas composition, etc., to mimic field conditions and are equipped with multiple analytical instruments to link below ground rhizosphere processes to above ground observations and vice versa (Figure 3C; Griffin et al., 1996; Lawton, 1996; Ke et al., 2020). Currently, however, accessibility to such systems is low as there are only several places in the world which can host such multifaceted facilities due to the requirement of significant financial investments.
Switching back to lab-scale systems, a recent perspective paper calls for the need to standardize devices, microbiomes and laboratory techniques to create model ecosystems (Zengler et al., 2019) to enable elucidation of molecular mechanisms mediating observed plant-microbe interactions e.g., exudate driven bacterial recruitment (Zhalnina et al., 2018a, b). Toward this goal, open source 3D printable chambers, termed Ecosystem Fabrication (EcoFAB) devices, have been released with detailed protocols to provide controlled laboratory habitats aimed at promoting mechanistic studies of plant-microbe interactions (Gao et al., 2018). Similar to a rhizotron setup, these flow-through systems are designed to provide clear visual access to the rhizosphere with flexibility of use with either soil or liquid substrates (Figures 3D,E). Certainly, there are many limitations to these devices (discussed in more in Table 1) in that they are limited to relatively small plants and limit the 3D architecture of the root system. Still, an advantage with the EcoFAB is that its 3D printable nature allows for adaptations and modifications to be made and shared on public data platforms such as Github for ease of standardization across different labs and experiments (Sasse et al., 2019). In fact, a recent multi-lab effort showed high reproducibility of root physiological and morphological traits in EcoFAB-grown Brachypodium distachyon plants (Sasse et al., 2019). The development of comparable datasets through the use of standardized systems is crucial to advancing our understanding of complex rhizosphere interactions. Open science programs such as the EcoFAB foster a transparent and collaborative network in an increasingly multi-disciplinary scientific community.
Specialized plant chamber systems are necessary for non-destructive visualization of rhizosphere processes and interactions as all destructive sampling approaches tend to overestimate the rhizosphere extent by 3–5 times compared to those based on visualization techniques (Kuzyakov and Razavi, 2019). Nonetheless, plants in such chambers are still grown in defined boundaries and suffer from inherent container impacts. For instance, studies have pointed out that container design (size, density, depth) significantly influences root growth during early developmental stages and leaves lasting impacts on plant health and phenotype (Howell and Harrington, 2004; South et al., 2005; Tsakaldimi et al., 2005; Kostopoulou et al., 2011). The majority of the lab-based chambers are also centimeter scale and are unlikely to replicate exact field conditions in terms of soil structure, water distribution, redox potential or root zone temperatures (Neumann et al., 2009). While comparisons between chamber-grown (e.g., rhizobox) and pot-grown plants show similar outputs (Devienne-Barret et al., 2006), studies comparing plants grown in confined spaces to those directly grown in the field are missing.
A recent review mapped the gradient boundaries for different rhizosphere aspects (physico-chemical gradients, root exudates and microbial communities, etc.) and found that despite the dynamic nature of each trait, the rhizosphere size and shape exist in a quasi-stationary state due to the opposing directions of their formation processes (Kuzyakov and Razavi, 2019). The generalized rhizosphere boundaries were deducted to be within 0.5–4 mm for most rhizosphere processes except for gases (e.g., O2) which exceeds > 4 mm and interestingly, they are independent of plant type, root type, age or soil (Kuzyakov and Razavi, 2019). Bearing this in mind, our assessment of the different growth chambers revealed possible overestimation of rhizosphere ranges in some chamber set ups. For instance, the use of root-free soil pouches representing rhizosphere soil despite being cm-distance away from the rhizoplane. This prompts the need for careful evaluation of new growth chamber designs to ensure accurate simulation of natural rhizosphere conditions.
To date, many rhizosphere microbiome studies and growth chambers systems focus on the impact of plant developmental stage, genotype and soil type on microbial composition and function (Chaparro et al., 2014; Edwards et al., 2015; Wagner et al., 2016). On the other hand, predation as a driver in the rhizosphere microbiome remains understudied. For instance, protists are abundant in the soil and are active consumers of bacteria and fungi and play a role in nutrient cycling yet remain an overlooked part of the rhizosphere (Gao et al., 2019). Viruses are also pivotal in modulating host communities thereby affecting biogeochemical cycles but their influence in the rhizosphere is poorly studied (Bi et al., 2020). These predator-prey interactions in the rhizosphere deserve in-depth studies which can be facilitated by these specialized growth chambers.
Another area worth investigating in the rhizosphere is in anaerobic microbial ecology. At microbially relevant scales, soils primarily exist as aggregates (<2 mm). Aggregation creates conditions different from bulk soil, particularly in terms of oxygen diffusion and water flow resulting in anoxic spaces within aggregates and influences the microbial community (Wilpiszeski et al., 2019). The rhizosphere is also rich in a wide range of compounds which can serve as alternative electron acceptors such as nitrate, iron, sulfate and humic substances in the absence of oxygen (Lecomte et al., 2018). However, most anaerobic studies in the rhizosphere focus only on aqueous environments such as water-logged paddy soils despite biochemical and metatranscriptomic evidence pointing to the possibility of anaerobic respiration in the rhizosphere (Lecomte et al., 2018). To fully understand biogeochemical cycles in the rhizosphere, it is imperative to investigate rhizosphere processes in the microscale and to include localized redox conditions as one of the influencing parameters. Microfluidic platforms with its fast prototyping capabilities can be helpful in creating growth chambers designed to stimulate these redox changes.
In the study of the rhizosphere microbiome, genetic manipulation strategies are foundational in deep characterization of microbial mechanisms and current manipulation techniques require axenic isolates. However, the uncultivability of a significant portion of soil microorganisms continues to hamper efforts in gaining mechanistic knowledge. Even for culturable isolates, the process of isolation introduces selective pressure and disturbance to the community with inevitable loss of information on spatial interactions. A recent innovation in gene editing technologies using CRISPR-cas systems demonstrated in situ editing of genetically tractable bacteria within a complex community (Rubin et al., 2020). Coupled with the use of transparent soil-like substrates (Downie et al., 2014), the application of such a technique for the editing of in situ rhizosphere microbiome while preserving spatial and temporal associations would indeed bring invaluable insights. Specialized growth chambers using 3D fabrication and microfluidic technologies are primed to facilitate such innovations.
Finally, this review revealed that while similarities exist among the different growth chamber systems, many of these systems are bespoke. This makes it difficult to replicate experiments and determine reproducibility which are important cornerstones of scientific advancement. The complexity of rhizosphere interactions also warrant that computational models are essential to gain a better understanding of system level processes (Darrah et al., 2006; Zengler et al., 2019). However, predictive modeling requires data from standardized approaches to be comparable between experiments. Thus, future growth chamber systems and designs are encouraged to follow the open science framework to enable standardization to an extent, such as in the case of EcoFABs (Sasse et al., 2019).
Studying the rhizosphere is a challenge due to the complex and dynamic interactions between many of its members, made further complicated by the opaque soil. Specialized plant chambers have been and continue to be an important tool in investigating these rhizosphere spatiotemporal processes in the soil. We identified representative growth systems used to study various rhizosphere interactions and processes such as root system architecture, exudation and microbial communities and found that they share common features but most are custom made to answer specific research questions. A major benefit of these specialized chambers is the ability to visualize the rhizosphere which allows for coupling with various analytical instruments to probe in situ processes through non-destructive sampling. Modern developments in growth chamber systems utilizing 3D fabrication and microfluidic technologies are also gaining prominence in understanding microscale interactions. These chambers also present the opportunity for both top down (community engineering and characterization) and bottom up (isolation-based) approaches to investigate rhizosphere communities. However, it should be noted that as these specialized chambers have been developed for model systems, the findings should ultimately be verified at field relevant conditions for truly predictive ecological understanding. Nonetheless, it is clear that the use of specialized chambers would continue to play a central role in our effort to gain a mechanistic understanding of the rhizosphere ecosystem.
RC conceptualized the idea. MY developed and wrote the manuscript. PK and YL contributed to specific sections of the review. All authors contributed to drafting and editing of the manuscript.
The authors declare that the research was conducted in the absence of any commercial or financial relationships that could be construed as a potential conflict of interest.
We thank Lauren K. Jabusch for the use of EcoFAB image in Figure 3E. This material by m-CAFEs Microbial Community Analysis & Functional Evaluation in Soils (m-CAFEs@lbl.gov), an SFA led by Lawrence Berkeley National Laboratory is based upon work supported by the U.S. Department of Energy, Office of Science, Office of Biological & Environmental Research under contract number DE-AC02-05CH11231.
Afzal, I., Shinwari, Z. K., Sikandar, S., and Shahzad, S. (2019). Plant beneficial endophytic bacteria: mechanisms, diversity, host range and genetic determinants. Microbiol. Res. 221, 36–49. doi: 10.1016/j.micres.2019.02.001
Agapit, C., Gigon, A., Girin, T., Leitao, L., and Blouin, M. (2020). Split-root system optimization based on the survival, growth and development of the model Poaceae brachypodium distachyon. Physiol. Plant. 168, 227–236. doi: 10.1111/ppl.12971
Aufrecht, J. A., Timm, C. M., Bible, A., Morrell-Falvey, J. L., Pelletier, D. A., Doktycz, M. J., et al. (2018). Quantifying the spatiotemporal dynamics of plant root colonization by beneficial bacteria in a microfluidic habitat. Adv. Biosyst. 2:1800048. doi: 10.1002/adbi.201800048
Augé, R. M. (2004). Arbuscular mycorrhizae and soil/plant water relations. Can. J. Soil Sci. 84, 373–381. doi: 10.4141/S04-002
Bao, Y., Aggarwal, P., Robbins, N. E., Sturrock, C. J., Thompson, M. C., Tan, H. Q., et al. (2014). Plant roots use a patterning mechanism to position lateral root branches toward available water. Proc. Natl. Acad. Sci. U.S.A. 111, 9319–9324. doi: 10.1073/pnas.1400966111
Bi, L., Yu, D. T., Du, S., Zhang, L. M., Zhang, L. Y., Wu, C. F., et al. (2020). Diversity and potential biogeochemical impacts of viruses in bulk and rhizosphere soils. Environ. Microbiol. 23, 588–599. doi: 10.1111/1462-2920.15010
Blossfeld, S., Perriguey, J., Sterckeman, T., Morel, J. L., and Lösch, R. (2010). Rhizosphere pH dynamics in trace-metal-contaminated soils, monitored with planar pH optodes. Plant Soil 330, 173–184. doi: 10.1007/s11104-009-0190-z
Bontpart, T., Concha, C., Giuffrida, M. V., Robertson, I., Admkie, K., Degefu, T., et al. (2020). Affordable and robust phenotyping framework to analyse root system architecture of soil-grown plants. Plant J. 103, 2330–2343. doi: 10.1111/tpj.14877
Buée, M., de Boer, W., Martin, F., van Overbeek, L., and Jurkevitch, E. (2009). The rhizosphere zoo: an overview of plant-associated communities of microorganisms, including phages, bacteria, archaea, and fungi, and of some of their structuring factors. Plant Soil 321, 189–212. doi: 10.1007/s11104-009-9991-3
Busch, J., Mendelssohn, I. A., Lorenzen, B., Brix, H., and Miao, S. L. (2006). A rhizotron to study root growth under flooded conditions tested with two wetland Cyperaceae. Flora Morphol. Distrib. Funct. Ecol. Plants 201, 429–439. doi: 10.1016/j.flora.2005.08.007
Canarini, A., Kaiser, C., Merchant, A., Richter, A., and Wanek, W. (2019). Root exudation of primary metabolites: mechanisms and their roles in plant responses to environmental stimuli. Front. Plant Sci. 10:157. doi: 10.3389/fpls.2019.00157
Cassidy, S. T., Burr, A. A., Reeb, R. A., Melero Pardo, A. L., Woods, K. D., and Wood, C. W. (2020). Using clear plastic CD cases as low-cost mini-rhizotrons to phenotype root traits. Appl. Plant Sci. 8, 1–7. doi: 10.1002/aps3.11340
Chaignon, V., Di Malta, D., and Hinsinger, P. (2002). Fe-deficiency increases Cu acquisition by wheat cropped in a Cu-contaminated vineyard soil. New Phytol. 154, 121–130. doi: 10.1046/j.1469-8137.2002.00349.x
Chaignon, V., and Hinsinger, P. (2003). A biotest for evaluating copper bioavailability to plants in a contaminated soil. J. Environ. Qual. 32, 824–833. doi: 10.2134/jeq2003.8240
Chaparro, J. M., Badri, D. V., and Vivanco, J. M. (2014). Rhizosphere microbiome assemblage is affected by plant development. ISME J. 8, 790–803. doi: 10.1038/ismej.2013.196
Darrah, P. R., Jones, D. L., Kirk, G. J. D., and Roose, T. (2006). Modelling the rhizosphere: a review of methods for “upscaling” to the whole-plant scale. Eur. J. Soil Sci. 57, 13–25. doi: 10.1111/j.1365-2389.2006.00786.x
de la Fuente Cantó, C., Simonin, M., King, E., Moulin, L., Bennett, M. J., Castrillo, G., et al. (2020). An extended root phenotype: the rhizosphere, its formation and impacts on plant fitness. Plant J. 103, 951–964. doi: 10.1111/tpj.14781
Dessaux, Y., Grandclément, C., and Faure, D. (2016). Engineering the rhizosphere. Trends Plant Sci. 21, 266–278. doi: 10.1016/j.tplants.2016.01.002
Devienne-Barret, F., Richard-Molard, C., Chelle, M., Maury, O., and Ney, B. (2006). Ara-rhizotron: an effective culture system to study simultaneously root and shoot development of Arabidopsis. Plant Soil 280, 253–266. doi: 10.1007/s11104-005-3224-1
Dinh, P. T. Y., Knoblauch, M., and Elling, A. A. (2014). Nondestructive imaging of plant-parasitic nematode development and host response to nematode pathogenesis. Phytopathology 104, 497–506. doi: 10.1094/PHYTO-08-13-0240-R
Downie, H. F., Valentine, T. A., Otten, W., Spiers, A. J., and Dupuy, L. X. (2014). Transparent soil microcosms allow 3D spatial quantification of soil microbiological processes in vivo. Plant Signal. Behav. 9:e970421. doi: 10.4161/15592316.2014.970421
Edwards, J., Johnson, C., Santos-Medellín, C., Lurie, E., Podishetty, N. K., Bhatnagar, S., et al. (2015). Structure, variation, and assembly of the root-associated microbiomes of rice. Proc. Natl. Acad. Sci. U.S.A. 112, E911–E920. doi: 10.1073/pnas.1414592112
Esser, H. G., Carminati, A., Vontobel, P., Lehmann, E. H., and Oswald, S. E. (2010). Neutron radiography and tomography of water distribution in the root zone. J. Plant Nutr. Soil Sci. 173, 757–764. doi: 10.1002/jpln.200900188
Fang, Y., Yabusaki, S. B., Ahkami, A. H., Chen, X., and Scheibe, T. D. (2019). An efficient three-dimensional rhizosphere modeling capability to study the effect of root system architecture on soil water and reactive transport. Plant Soil 441, 33–48. doi: 10.1007/s11104-019-04068-z
Ferrol, N., Azcón-Aguilar, C., and Pérez-Tienda, J. (2019). Review: arbuscular mycorrhizas as key players in sustainable plant phosphorus acquisition: an overview on the mechanisms involved. Plant Sci. 280, 441–447. doi: 10.1016/j.plantsci.2018.11.011
Frederiksen, M. S., and Glud, R. N. (2006). Oxygen dynamics in the rhizosphere of Zostera marina: a two-dimensional planar optode study. Limnol. Oceanogr. 51, 1072–1083. doi: 10.4319/lo.2006.51.2.1072
Froelich, D. R., Mullendore, D. L., Jensen, K. H., Ross-Elliott, T. J., Anstead, J. A., Thompson, G. A., et al. (2011). Phloem ultrastructure and pressure flow: sieve-element-occlusion-related agglomerations do not affect translocation. Plant Cell 23, 4428–4445. doi: 10.1105/tpc.111.093179
Gao, J., Sasse, J., Lewald, K. M., Zhalnina, K., Cornmesser, L. T., Duncombe, T. A., et al. (2018). Ecosystem fabrication (EcoFAB) protocols for the construction of laboratory ecosystems designed to study plant-microbe interactions. J. Vis. Exp. 2018:e57170. doi: 10.3791/57170
Gao, Z., Karlsson, I., Geisen, S., Kowalchuk, G., and Jousset, A. (2019). Protists: puppet masters of the rhizosphere microbiome. Trends Plant Sci. 24, 165–176. doi: 10.1016/j.tplants.2018.10.011
Garrigues, E., Doussan, C., and Pierret, A. (2006). Water uptake by plant roots: I - formation and propagation of a water extraction front in mature root systems as evidenced by 2D light transmission imaging. Plant Soil 283, 83–98. doi: 10.1007/s11104-004-7903-0
Griffin, K. L., Ross, P. D., Sims, D. A., Luo, Y., Seemann, J. R., Fox, C. A., et al. (1996). EcoCELLs: tools for mesocosm scale measurements of gas exchange. Plant Cell Environ. 19, 1210–1221. doi: 10.1111/j.1365-3040.1996.tb00437.x
Grossmann, G., Guo, W. J., Ehrhardt, D. W., Frommer, W. B., Sit, R. V., Quake, S. R., et al. (2011). The Rootchip: an integrated microfluidic chip for plant science. Plant Cell 23, 4234–4240. doi: 10.1105/tpc.111.092577
Hapca, S. M., Budha, P., Crawford, J. W., and Young, I. M. (2007). Movement of the nematode, Phasmarhabditis hermaphrodita, in a structurally heterogeneous environment. Nematology 9, 731–738. doi: 10.1163/156854107782024811
Harrison, M. J. (2005). Signaling in the arbuscular mycorrhizal symbiosis. Annu. Rev. Microbiol. 59, 19–42. doi: 10.1146/annurev.micro.58.030603.123749
Helliwell, J. R., Sturrock, C. J., Grayling, K. M., Tracy, S. R., Flavel, R. J., Young, I. M., et al. (2013). Applications of X-ray computed tomography for examining biophysical interactions and structural development in soil systems: a review. Eur. J. Soil Sci. 64, 279–297. doi: 10.1111/ejss.12028
Helliwell, J. R., Sturrock, C. J., Mairhofer, S., Craigon, J., Ashton, R. W., Miller, A. J., et al. (2017). The emergent rhizosphere: imaging the development of the porous architecture at the root-soil interface. Sci. Rep. 7:14875. doi: 10.1038/s41598-017-14904-w
Hirsch, A. M., Lum, M. R., and Downie, J. A. (2001). What makes the Rhizobia-legume symbiosis so special? Plant Physiol. 127, 1484–1492. doi: 10.1104/pp.010866
Ho, M. D., Rosas, J. C., Brown, K. M., and Lynch, J. P. (2005). Root architectural tradeoffs for water and phosphorus acquisition. Funct. Plant Biol. 32, 737–748. doi: 10.1071/FP05043
Howell, K. D., and Harrington, T. B. (2004). Nursery practices influence seedling morphology, field performance, and cost efficiency of containerized Cherrybark Oak. S. J. Appl. For. 28, 152–162. doi: 10.1093/sjaf/28.3.152
Iyer-Pascuzzi, A. S., Symonova, O., Mileyko, Y., Hao, Y., Belcher, H., Harer, J., et al. (2010). Imaging and analysis platform for automatic phenotyping and trait ranking of plant root systems. Plant Physiol. 152, 1148–1157. doi: 10.1104/pp.109.150748
Jaeger, C. H., Lindow, S. E., Miller, W., Clark, E., and Firestone, M. K. (1999). Mapping of sugar and amino acid availability in soil around roots with bacterial sensors of sucrose and tryptophan. Appl. Environ. Microbiol. 65, 2685–2690. doi: 10.1128/aem.65.6.2685-2690.1999
Joergensen, R. G. (2000). Ergosterol and microbial biomass in the rhizosphere of grassland soils. Soil Biol. Biochem. 32, 647–652. doi: 10.1016/S0038-0717(99)00191-1
Jones, A. M., Danielson, J. ÅH., Manojkumar, S. N., Lanquar, V., Grossmann, G., and Frommer, W. B. (2014). Abscisic acid dynamics in roots detected with genetically encoded FRET sensors. eLife 3:e01741. doi: 10.7554/eLife.01741.001
Judd, L. A., Jackson, B. E., and Fonteno, W. C. (2015). Advancements in root growth measurement technologies and observation capabilities for container-grown plants. Plants 4, 369–392. doi: 10.3390/plants4030369
Judd, L. A., Jackson, B. E., Yap, T. C., and Fonteno, W. C. (2014). Mini-horhizotron: an apparatus for observing and measuring root growth of container-grown plant material in Situ. HortScience 49, 1424–1431. doi: 10.21273/hortsci.49.11.1424
Kaiser, C., Kilburn, M. R., Clode, P. L., Fuchslueger, L., Koranda, M., Cliff, J. B., et al. (2015). Exploring the transfer of recent plant photosynthates to soil microbes: mycorrhizal pathway vs direct root exudation. New Phytol. 205, 1537–1551. doi: 10.1111/nph.13138
Kamh, M., Horst, W. J., Amer, F., Mostafa, H., and Maier, P. (1999). Mobilization of soil and fertilizer phosphate by cover crops. Plant Soil 211, 19–27. doi: 10.1023/A:1004543716488
Ke, J., Wang, B., and Yoshikuni, Y. (2020). Microbiome engineering: synthetic biology of plant-associated microbiomes in sustainable agriculture. Trends Biotechnol. 39, 244–261. doi: 10.1016/j.tibtech.2020.07.008
Keinath, N. F., Waadt, R., Brugman, R., Schroeder, J. I., Grossmann, G., Schumacher, K., et al. (2015). Live cell imaging with R-GECO1 sheds light on flg22- and chitin-induced transient [Ca2+]cyt patterns in Arabidopsis. Mol. Plant 8, 1188–1200. doi: 10.1016/j.molp.2015.05.006
Khan, M. A., Gemenet, D. C., and Villordon, A. (2016). Root system architecture and abiotic stress tolerance: current knowledge in root and tuber crops. Front. Plant Sci. 7:1584. doi: 10.3389/fpls.2016.01584
Khan, Z., Karamahmutoğlu, H., Elitaş, M., Yüce, M., and Budak, H. (2019). Through the looking glass: real-time imaging in brachypodium roots and osmotic stress analysis. Plants 8:14. doi: 10.3390/plants8010014
Klepper, B., and Kaspar, T. C. (1994). Rhizotrons: their development and use in agricultural research. Agron. J. 86, 745–753. doi: 10.2134/agronj1994.00021962008600050002x
Koebernick, N., Daly, K. R., Keyes, S. D., George, T. S., Brown, L. K., Raffan, A., et al. (2017). High-resolution synchrotron imaging shows that root hairs influence rhizosphere soil structure formation. New Phytol. 216, 124–135. doi: 10.1111/nph.14705
Koegel, S., Boller, T., Lehmann, M. F., Wiemken, A., and Courty, P. E. (2013). Rapid nitrogen transfer in the sorghum bicolor-glomus mosseae arbuscular mycorrhizal symbiosis. Plant Signal. Behav. 8, 10–13. doi: 10.4161/psb.25229
Kooliyottil, R., Dandurand, L.-M., Govindan, B. N., and Knudsen, R. (2016). Microscopy method to compare cyst nematode infection of different plant species. Adv. Biosci. Biotechnol. 07, 311–318. doi: 10.4236/abb.2016.76029
Kooliyottil, R., Dandurand, L. M., Kuhl, J. C., Caplan, A., and Xiao, F. (2017). Microaspiration of Solanum tuberosum root cells at early stages of infection by Globodera pallida. Plant Methods 13:68. doi: 10.1186/s13007-017-0219-x
Kosslak, R. M., and Bohlool, B. B. (1984). Suppression of nodule development of one side of a split-root system of soybeans caused by prior inoculation of the other side. Plant Physiol. 75, 125–130. doi: 10.1104/pp.75.1.125
Kostopoulou, P., Radoglou, K., Papanastasi, O. D., and Adamidou, C. (2011). Effect of mini-plug container depth on root and shoot growth of four forest tree species during early developmental stages. Turk. J. Agric. For. 35, 379–390. doi: 10.3906/tar-1104-11
Kuchenbuch, R., and Jungk, A. (1982). A method for determining concentration profiles at the soil-root interface by thin slicing rhizospheric soil. Plant Soil 68, 391–394. doi: 10.1007/BF02197944
Kuijken, R. C. P., Van Eeuwijk, F. A., Marcelis, L. F. M., and Bouwmeester, H. J. (2015). Root phenotyping: from component trait in the lab to breeding. J. Exp. Bot. 66, 5389–5401. doi: 10.1093/jxb/erv239
Kuzyakov, Y., and Razavi, B. S. (2019). Rhizosphere size and shape: temporal dynamics and spatial stationarity. Soil Biol. Biochem. 135, 343–360. doi: 10.1016/j.soilbio.2019.05.011
Larrainzar, E., Gil-Quintana, E., Arrese-Igor, C., González, E. M., and Marino, D. (2014). Split-root systems applied to the study of the legume-rhizobial symbiosis: what have we learned? J. Integr. Plant Biol. 56, 1118–1124. doi: 10.1111/jipb.12231
Lawton, J. H. (1996). The ecotron facility at silwood park: the value of “Big Bottle” experiments. Ecology 77, 665–669. doi: 10.2307/2265488
Lecomte, S. M., Achouak, W., Abrouk, D., Heulin, T., Nesme, X., and Haichar, F. Z. (2018). Diversifying anaerobic respiration strategies to compete in the rhizosphere. Front. Environ. Sci. 6:139. doi: 10.3389/fenvs.2018.00139
Lockery, S. R., Lawton, K. J., Doll, J. C., Faumont, S., Coulthard, S. M., Thiele, T. R., et al. (2008). Artificial dirt: microfluidic substrates for nematode neurobiology and behavior. J. Neurophysiol. 99, 3136–3143. doi: 10.1152/jn.91327.2007
Manosalva, P., Manohar, M., Von Reuss, S. H., Chen, S., Koch, A., Kaplan, F., et al. (2015). Conserved nematode signalling molecules elicit plant defenses and pathogen resistance. Nat. Commun. 6, 1–8. doi: 10.1038/ncomms8795
Marschner, P., and Baumann, K. (2003). Changes in bacterial community structure induced by mycorrhizal colonisation in split-root maize. Plant Soil 251, 279–289. doi: 10.1023/A:1023034825871
Massalha, H., Korenblum, E., Malitsky, S., Shapiro, O. H., and Aharoni, A. (2017). Live imaging of root-bacteria interactions in a microfluidics setup. Proc. Natl. Acad. Sci. U.S.A. 114, 4549–4554. doi: 10.1073/pnas.1618584114
Meier, M., Lucchetta, E. M., and Ismagilov, R. F. (2010). Chemical stimulation of the Arabidopsis thaliana root using multi-laminar flow on a microfluidic chip. Lab. Chip 10, 2147–2153. doi: 10.1039/c004629a
Metzner, R., van Dusschoten, D., Bühler, J., Schurr, U., and Jahnke, S. (2014). Belowground plant development measured with magnetic resonance imaging (MRI): exploiting the potential for non-invasive trait quantification using sugar beet as a proxy. Front. Plant Sci. 5:469. doi: 10.3389/fpls.2014.00469
Mitchell, K., French, E., Beckerman, J., Iyer-Pascuzzi, A., Volenec, J., and Gibson, K. (2018). Biochar alters the root systems of large crabgrass. HortScience 53, 354–359. doi: 10.21273/HORTSCI12690-17
Mohamed, A., Monnier, Y., Mao, Z., Lobet, G., Maeght, J. L., Ramel, M., et al. (2017). An evaluation of inexpensive methods for root image acquisition when using rhizotrons. Plant Methods 13:11. doi: 10.1186/s13007-017-0160-z
Mooney, S. J., Pridmore, T. P., Helliwell, J., and Bennett, M. J. (2012). Developing X-ray computed tomography to non-invasively image 3-D root systems architecture in soil. Plant Soil 352, 1–22. doi: 10.1007/s11104-011-1039-9
Morris, E. C., Griffiths, M., Golebiowska, A., Mairhofer, S., Burr-Hersey, J., Goh, T., et al. (2017). Shaping 3D root system architecture. Curr. Biol. 27, R919–R930. doi: 10.1016/j.cub.2017.06.043
Neumann, G., Bott, S., Ohler, M. A., Mock, H. P., Lippmann, R., Grosch, R., et al. (2014). Root exudation and root development of lettuce (lactuca sativa l. Cv. Tizian) as affected by different soils. Front. Microbiol. 5:2. doi: 10.3389/fmicb.2014.00002
Neumann, G., George, T. S., and Plassard, C. (2009). Strategies and methods for studying the rhizosphere-the plant science toolbox. Plant Soil 321, 431–456. doi: 10.1007/s11104-009-9953-9
Nie, S., Li, H., Yang, X., Zhang, Z., Weng, B., Huang, F., et al. (2015). Nitrogen loss by anaerobic oxidation of ammonium in rice rhizosphere. ISME J. 9, 2059–2067. doi: 10.1038/ismej.2015.25
Nuccio, E. E., Starr, E., Karaoz, U., Brodie, E. L., Zhou, J., Tringe, S. G., et al. (2020). Niche differentiation is spatially and temporally regulated in the rhizosphere. ISME J. 14, 999–1014. doi: 10.1038/s41396-019-0582-x
Oburger, E., Dell’mour, M., Hann, S., Wieshammer, G., Puschenreiter, M., and Wenzel, W. W. (2013). Evaluation of a novel tool for sampling root exudates from soil-grown plants compared to conventional techniques. Environ. Exp. Bot. 87, 235–247. doi: 10.1016/j.envexpbot.2012.11.007
Oburger, E., and Schmidt, H. (2016). New methods to unravel rhizosphere processes. Trends Plant Sci. 21, 243–255. doi: 10.1016/j.tplants.2015.12.005
O’Callaghan, F. E., Braga, R. A., Neilson, R., MacFarlane, S. A., and Dupuy, L. X. (2018). New live screening of plant-nematode interactions in the rhizosphere. Sci. Rep. 8:1440. doi: 10.1038/s41598-017-18797-7
O’Donnell, A. G., Young, I. M., Rushton, S. P., Shirley, M. D., and Crawford, J. W. (2007). Visualization, modelling and prediction in soil microbiology. Nat. Rev. Microbiol. 5, 689–699. doi: 10.1038/nrmicro1714
Parashar, A., and Pandey, S. (2011). Plant-in-chip: microfluidic system for studying root growth and pathogenic interactions in Arabidopsis. Appl. Phys. Lett. 98:263703. doi: 10.1063/1.3604788
Patabadige, D. E. W., Millet, L. J., Aufrecht, J. A., Shankles, P. G., Standaert, R. F., Retterer, S. T., et al. (2019). Label-free time- and space-resolved exometabolite sampling of growing plant roots through nanoporous interfaces. Sci. Rep. 9, 1–10. doi: 10.1038/s41598-019-46538-5
Popova, L., Van Dusschoten, D., Nagel, K. A., Fiorani, F., and Mazzolai, B. (2016). Plant root tortuosity: an indicator of root path formation in soil with different composition and density. Ann. Bot. 118, 685–698. doi: 10.1093/aob/mcw057
Powell, N. T. (1971). Interactions between nematodes and fungi in disease complexes. Annu. Rev. Phytopathol. 9, 253–274. doi: 10.1146/annurev.py.09.090171.001345
Rasmann, S., Köllner, T. G., Degenhardt, J., Hiltpold, I., Toepfer, S., Kuhlmann, U., et al. (2005). Recruitment of entomopathogenic nematodes by insect-damaged maize roots. Nature 434, 732–737. doi: 10.1038/nature03451
Rellán-Álvarez, R., Lobet, G., Lindner, H., Pradier, P. L., Sebastian, J., Yee, M. C., et al. (2015). GLO-roots: an imaging platform enabling multidimensional characterization of soil-grown root systems. eLife 4:e07597. doi: 10.7554/eLife.07597
Rubin, B., Diamond, S., Cress, B., Crits-Christoph, A., He, C., Xu, M., et al. (2020). Targeted genome editing of bacteria within microbial communities. bioRxiv [Preprint]. doi: 10.1101/2020.07.17.209189
Ruiz-Lozano, J. M., and Azcón, R. (1995). Hyphal contribution to water uptake in mycorrhizal plants as affected by the fungal species and water status. Physiol. Plant. 95, 472–478. doi: 10.1111/j.1399-3054.1995.tb00865.x
Saiz-Fernández, I., Černý, M., Skalák, J., and Brzobohatý, B. (2021). Split-root systems: detailed methodology, alternative applications, and implications at leaf proteome level. Plant Methods 17:7. doi: 10.1186/s13007-020-00706-1
Saleem, M., Law, A. D., Sahib, M. R., Pervaiz, Z. H., and Zhang, Q. (2018). Impact of root system architecture on rhizosphere and root microbiome. Rhizosphere 6, 47–51. doi: 10.1016/j.rhisph.2018.02.003
Santner, J., Larsen, M., Kreuzeder, A., and Glud, R. N. (2015). Two decades of chemical imaging of solutes in sediments and soils - a review. Anal. Chim. Acta 878, 9–42. doi: 10.1016/j.aca.2015.02.006
Santner, J., Zhang, H., Leitner, D., Schnepf, A., Prohaska, T., Puschenreiter, M., et al. (2012). High-resolution chemical imaging of labile phosphorus in the rhizosphere of Brassica napus L. cultivars. Environ. Exp. Bot. 77, 219–226. doi: 10.1016/j.envexpbot.2011.11.026
Sasse, J., Kant, J., Cole, B. J., Klein, A. P., Arsova, B., Schlaepfer, P., et al. (2019). Multilab EcoFAB study shows highly reproducible physiology and depletion of soil metabolites by a model grass. New Phytol. 222, 1149–1160. doi: 10.1111/nph.15662
Sasse, J., Martinoia, E., and Northen, T. (2018). Feed your friends: do plant exudates shape the root microbiome? Trends Plant Sci. 23, 25–41. doi: 10.1016/j.tplants.2017.09.003
Shrestha, M., Shrestha, P. M., Frenzel, P., and Conrad, R. (2010). Effect of nitrogen fertilization on methane oxidation, abundance, community structure, and gene expression of methanotrophs in the rice rhizosphere. ISME J. 4, 1545–1556. doi: 10.1038/ismej.2010.89
Six, J., Bossuyt, H., Degryze, S., and Denef, K. (2004). A history of research on the link between (micro)aggregates, soil biota, and soil organic matter dynamics. Soil Tillage Res. 79, 7–31. doi: 10.1016/j.still.2004.03.008
South, D. B., Harris, S. W., Barnett, J. P., Hainds, M. J., and Gjerstad, D. H. (2005). Effect of container type and seedling size on survival and early height growth of Pinus palustris seedlings in Alabama, U.S.A. For. Ecol. Manage. 204, 385–398. doi: 10.1016/j.foreco.2004.09.016
Spohn, M., Carminati, A., and Kuzyakov, Y. (2013). Soil zymography - A novel in situ method for mapping distribution of enzyme activity in soil. Soil Biol. Biochem. 58, 275–280. doi: 10.1016/j.soilbio.2012.12.004
Stanley, C. E., Grossmann, G., CasadevallI Solvas, X., and DeMello, A. J. (2016). Soil-on-a-chip: microfluidic platforms for environmental organismal studies. Lab Chip 16, 228–241. doi: 10.1039/c5lc01285f
Stanley, C. E., Shrivastava, J., Brugman, R., Heinzelmann, E., van Swaay, D., and Grossmann, G. (2018). Dual-flow-RootChip reveals local adaptations of roots towards environmental asymmetry at the physiological and genetic levels. New Phytol. 217, 1357–1369. doi: 10.1111/nph.14887
Tanaka, Y., and Yano, K. (2005). Nitrogen delivery to maize via mycorrhizal hyphae depends on the form of N supplied. Plant Cell Environ. 28, 1247–1254. doi: 10.1111/j.1365-3040.2005.01360.x
Taylor, H. M., Upchurch, D. R., and McMichael, B. L. (1990). Applications and limitations of rhizotrons and minirhizotrons for root studies. Plant Soil 129, 29–35. doi: 10.1007/BF00011688
Topp, C. N., Iyer-Pascuzzi, A. S., Anderson, J. T., Lee, C. R., Zurek, P. R., Symonova, O., et al. (2013). 3D phenotyping and quantitative trait locus mapping identify core regions of the rice genome controlling root architecture. Proc. Natl. Acad. Sci. U.S.A. 110, E1695–E1704. doi: 10.1073/pnas.1304354110
Tsakaldimi, M., Zagas, T., Tsitsoni, T., and Ganatsas, P. (2005). Root morphology, stem growth and field performance of seedlings of two Mediterranean evergreen oak species raised in different container types. Plant Soil 278, 85–93. doi: 10.1007/s11104-005-2580-1
Unger, M. A., Chou, H. P., Thorsen, T., Scherer, A., and Quake, S. R. (2000). Monolithic microfabricated valves and pumps by multilayer soft lithography. Science 288, 113–116. doi: 10.1126/science.288.5463.113
Valentinuzzi, F., Mimmo, T., Cesco, S., Al Mamun, S., Santner, J., Hoefer, C., et al. (2015). The effect of lime on the rhizosphere processes and elemental uptake of white lupin. Environ. Exp. Bot. 118, 85–94. doi: 10.1016/j.envexpbot.2015.06.010
van Dam, N. M., and Bouwmeester, H. J. (2016). Metabolomics in the rhizosphere: tapping into belowground chemical communication. Trends Plant Sci. 21, 256–265. doi: 10.1016/j.tplants.2016.01.008
Van Der Heijden, M. G. A., Bruin, S., De, Luckerhoff, L., Van Logtestijn, R. S. P., and Schlaeppi, K. (2016). A widespread plant-fungal-bacterial symbiosis promotes plant biodiversity, plant nutrition and seedling recruitment. ISME J. 10, 389–399. doi: 10.1038/ismej.2015.120
van Dusschoten, D., Metzner, R., Kochs, J., Postma, J. A., Pflugfelder, D., Bühler, J., et al. (2016). Quantitative 3D analysis of plant roots growing in soil using magnetic resonance imaging. Plant Physiol. 170, 1176–1188. doi: 10.1104/pp.15.01388
Venturi, V., and Keel, C. (2016). Signaling in the rhizosphere. Trends Plant Sci. 21, 187–198. doi: 10.1016/j.tplants.2016.01.005
Vierheilig, H., Garcia-Garrido, J. M., Wyss, U., and Piché, Y. (2000). Systemic suppression of mycorrhizal colonization of barley roots already colonized by AM fungi. Soil Biol. Biochem. 32, 589–595. doi: 10.1016/S0038-0717(99)00155-8
Wagner, M. R., Lundberg, D. S., Del Rio, T. G., Tringe, S. G., Dangl, J. L., and Mitchell-Olds, T. (2016). Host genotype and age shape the leaf and root microbiomes of a wild perennial plant. Nat. Commun. 7, 1–15. doi: 10.1038/ncomms12151
Wang, F., Shi, N., Jiang, R., Zhang, F., and Feng, G. (2016). In situ stable isotope probing of phosphate-solubilizing bacteria in the hyphosphere. J. Exp. Bot. 67, 1689–1701. doi: 10.1093/jxb/erv561
Wei, Z., Gu, Y., Friman, V. P., Kowalchuk, G. A., Xu, Y., Shen, Q., et al. (2019). Initial soil microbiome composition and functioning predetermine future plant health. Sci. Adv. 5:eaaw0759. doi: 10.1126/sciadv.aaw0759
Wilpiszeski, R. L., Aufrecht, J. A., Retterer, S. T., Sullivan, M. B., Graham, D. E., Pierce, E. M., et al. (2019). Soil aggregate microbial communities: towards understanding microbiome interactions at biologically relevant scales. Appl. Environ. Microbiol. 85:e00324-19. doi: 10.1128/AEM.00324-19
Wright, A. N., and Wright, R. D. (2004). The horhizotronTM: a new instrument for measuring root growth. Horttechnology 14, 560–563. doi: 10.21273/horttech.14.4.0560
Yates, R. J., Howieson, J. G., Hungria, M., Bala, A., O’Hara, G. W., and Terpolilli, J. (2016). “Authentication of rhizobia and assessment of the legume symbiosis in controlled plant growth systems,” in Working with rhizobia, eds J. G. Howieson and M. J. Dilworth (Canberra: Australian centre for international agricultural research).
Yevdokimov, I., Ruser, R., Buegger, F., Marx, M., and Munch, J. C. (2006). Microbial immobilisation of 13C rhizodeposits in rhizosphere and root-free soil under continuous 13C labelling of oats. Soil Biol. Biochem. 38, 1202–1211. doi: 10.1016/j.soilbio.2005.10.004
Zengler, K., Hofmockel, K., Baliga, N. S., Behie, S. W., Bernstein, H. C., Brown, J. B., et al. (2019). EcoFABs: advancing microbiome science through standardized fabricated ecosystems. Nat. Methods 16, 567–571. doi: 10.1038/s41592-019-0465-0
Zhalnina, K., Louie, K. B., Hao, Z., Mansoori, N., Da Rocha, U. N., Shi, S., et al. (2018a). Dynamic root exudate chemistry and microbial substrate preferences drive patterns in rhizosphere microbial community assembly. Nat. Microbiol. 3, 470–480. doi: 10.1038/s41564-018-0129-3
Zhalnina, K., Zengler, K., Newman, D., and Northen, T. R. (2018b). Need for laboratory ecosystems to unravel the structures and functions of soil microbial communities mediated by chemistry. mBio 9:e01175-18. doi: 10.1128/mBio.01175-18
Zhang, Q., Yang, R., Tang, J., Yang, H., Hu, S., and Chen, X. (2010). Positive feedback between mycorrhizal fungi and plants influences plant invasion success and resistance to invasion. PLoS One 5:e12380. doi: 10.1371/journal.pone.0012380
Zhu, H. H., and Yao, Q. (2004). Localized and Systemic Increase of Phenols in Tomato Roots Induced by Glomus versiforme Inhibits Ralstonia solanacearum. J. Phytopathol. 152, 537–542. doi: 10.1111/j.1439-0434.2004.00892.x
Keywords: rhizosphere, interactions, plant growth chamber, soil, chamber design
Citation: Yee MO, Kim P, Li Y, Singh AK, Northen TR and Chakraborty R (2021) Specialized Plant Growth Chamber Designs to Study Complex Rhizosphere Interactions. Front. Microbiol. 12:625752. doi: 10.3389/fmicb.2021.625752
Received: 03 November 2020; Accepted: 19 February 2021;
Published: 26 March 2021.
Edited by:
Soumitra Paul Chowdhury, Helmholtz-Gemeinschaft Deutscher Forschungszentren (HZ), GermanyReviewed by:
Siu Mui Tsai, University of São Paulo, BrazilCopyright © 2021 Yee, Kim, Li, Singh, Northen and Chakraborty. This is an open-access article distributed under the terms of the Creative Commons Attribution License (CC BY). The use, distribution or reproduction in other forums is permitted, provided the original author(s) and the copyright owner(s) are credited and that the original publication in this journal is cited, in accordance with accepted academic practice. No use, distribution or reproduction is permitted which does not comply with these terms.
*Correspondence: Romy Chakraborty, cmNoYWtyYWJvcnR5QGxibC5nb3Y=
Disclaimer: All claims expressed in this article are solely those of the authors and do not necessarily represent those of their affiliated organizations, or those of the publisher, the editors and the reviewers. Any product that may be evaluated in this article or claim that may be made by its manufacturer is not guaranteed or endorsed by the publisher.
Research integrity at Frontiers
Learn more about the work of our research integrity team to safeguard the quality of each article we publish.