- 1Department of Biology and Biotechnology “Lazzaro Spallanzani”, University of Pavia, Pavia, Italy
- 2Department of Biosciences, University of Milan, Milan, Italy
Bacterial species able to produce proteins that are toxic against insects have been discovered at the beginning of the last century. However, up to date only two of them have been used as pesticides in mosquito control strategies targeting larval breeding sites: Bacillus thuringensis var. israelensis and Lysinibacillus sphaericus. Aiming to expand the arsenal of biopesticides, bacterial cultures from 44 soil samples were assayed for their ability to kill larvae of Aedes albopictus. A method to select, grow and test the larvicidal capability of spore-forming bacteria from each soil sample was developed. This allowed identifying 13 soil samples containing strains capable of killing Ae. albopictus larvae. Among the active isolates, one strain with high toxicity was identified as Brevibacillus laterosporus by 16S rRNA gene sequencing and by morphological characterization using transmission electron microscopy. The new isolate showed a larvicidal activity significantly higher than the B. laterosporus LMG 15441 reference strain. Its genome was phylogenomically characterized and compared to the available Brevibacillus genomes. Thus, the new isolate can be considered as a candidate adjuvant to biopesticides formulations that would help preventing the insurgence of resistance.
Introduction
The vector control market, which includes chemical, biological, and physical (e.g., UV lights and traps) strategies, was valued at USD 15.12 billion in 2017 and is projected to reach 20.37 billion by 2023. The segment likely to grow at the highest rate is the one of biological control: globally the biopesticide market has an estimated value of USD 4.3 billion in 2020, and it is expected to reach USD 8.5 billion in the next five years (Markets and markets, 2018). This dramatic increase can be attributed to the growing concerns on the environmental impact of chemical insecticides and the continuous increase of insecticide-resistance populations in many vector species. Moreover, unlike conventional insecticides which often target a broad spectrum of insects, including beneficial species such as pollinators, bioinsecticides provide a more targeted activity toward selected species. Furthermore, these products are quickly biodegraded, leaving virtually no harmful residues and having limited long-term impact on the environment.
The biocontrol of mosquitoes began with the identification of bacteria active against Diptera, specifically Bacillus thuringiensis var. israelensis in 1977 (Goldberg and Margalit, 1977) and Lysinibacillus sphaericus strain 1593 (Singer, 1973). These two bacterial species achieved commercial success and are now broadly used.
The toxic activity of B. thuringiensis var. israelensis is due to four major crystal proteins, Cry4Aa, Cry4Ba, Cry11Aa, and Cyt1Aa, whose genes reside on a 128 kb plasmid (pBtoxis) (Berry et al., 2002; Ben-Dov, 2014). Other proteins, such as Cry10Aa, Cyt2Ba, Cyt1Ca, P19, and P20, contribute to the toxicity of B. thuringiensis var. israelensis (Palma et al., 2014). The larvicidal activity of the Cry and Cyt toxins is due to their ability to perforate midgut epithelial cells membranes, with the Crys binding to membrane receptors (Feldmann et al., 1995) and Cyt1Aa binding unsaturated phospholipids (Thomas and Ellar, 1983; Du et al., 1999). The major pitfalls of B. thuringiensis var. israelensis is its sensitivity to UV-damage (Myasnik et al., 2001), which required the development of stabilizing formulations (Lacey, 2007) and biotechnological approaches, including transgenic crops or recombinant bacteria expressing B. thuringiensis toxins (Federici et al., 2003; Sanahuja et al., 2011; Ursino et al., 2020).
One case of mosquito population resistant to B. thuringiensis var. israelensis has been described (Paul et al., 2005); however, most targeted studies have reported no resistance after long periods of treatment (Goldman et al., 1986; Becker and Ludwig, 1993). One of the obstacles to the development of resistance to B. thuringiensis var. israelensis is the synergism between Cry toxins and Cyt1Aa, with the latter functioning as a surrogate receptor able to promote toxin binding to host target membranes (Pérez et al., 2005). Nevertheless, moderate resistance may occur (Boyer et al., 2007; Paris et al., 2010), underlying the possibility that, in some instances, B. thuringiensis var. israelensis might persist in the environment for a long time, enhancing the likelihood of evolving resistant mosquito populations. Indeed, B. thuringiensis var. israelensis has low survivability in the environment but, in specific conditions, it persists and proliferates (Tilquin et al., 2008; Melo-Santos et al., 2010).
Lysinibacillus sphaericus is a second, potent mosquitocidal bioinsecticide. During sporulation it produces two separate proteins, BinA (42 kDa) and BinB (51 kDa), that form a binary toxin which accumulates as parasporal crystalline inclusions (Alexander and Priest, 1990). Some strains also produce non-crystal mosquitocidal toxins (Mtx1, Mtx2, and Mtx3) during vegetative growth. Upon binding to brush border membranes of midgut cells, L. sphaericus toxins are internalized and induce cell death either via ADP-ribosylation, as is the case of Mtx1 toxin (Thanabalu et al., 1993), or activation of apoptosis (Tangsongcharoen et al., 2015). As binding of Bin toxin to midgut cells is mediated by a single receptor (maltase), resistance is easily acquired as a consequence of mutations in this toxin binding protein (Darboux et al., 2002). The insurgence of L. sphaericus resistant populations has been described since 1997 (Nielsen-Leroux et al., 1997) and resistance in field populations of various countries has been reported (Su et al., 2018, 2019).
The highly effective results obtained with B. thuringiensis var. israelensis and L. sphaericus led to several screening campaigns allowing the identification of other useful bacterial biopesticide species, such as Chromobacterium subtsugae (Martin et al., 2007), Yersinia entomophaga (Hurst et al., 2011), and Brevibacillus laterosporus (de Oliveira et al., 2004; Ruiu, 2013).
Many strains of B. laterosporus have been isolated and the list of insects susceptible to their entomopathogenic activity includes Coleoptera, Lepidoptera (de Oliveira et al., 2004), mosquitoes, black flies (Favret and Yousten, 1985; Rivers et al., 1991), and house flies (Ruiu et al., 2007). Its pathogenicity against Diptera has been associated to the characteristic canoe-shaped parasporal body (CSPB) which consists of four major proteins: CpbA, CpbB, CHRD, and ExsC (Marche et al., 2017). Ingestion of lysates of recombinant Escherichia coli strains expressing these proteins results in the death of house flies, implying their role as insecticidal toxins (Marche et al., 2017).
Here we report the isolation of a novel B. laterosporus strain with high toxicity against mosquito larvae. This strain was isolated in a screening campaign of bacterial isolates active against Aedes albopictus, a globally distributed invasive species vector of many mosquito-borne diseases (Paupy et al., 2009). We carried out morphological, genomic, and insecticidal characterization of the new isolate, and determined that its larvicidal activity is significantly higher than that of the LMG 15441 reference strain. Thus, the new isolate characterized in this paper can be considered as a candidate for the development of novel biocontrol formulations, alone or in combination with B. thuringiensis var. israelensis and L. sphaericus preparations to enhance their efficacy and avert the insurgence of resistance.
Materials and Methods
Bacterial Strains and Growth Media
Lysinibacillus sphaericus strain 1593 (Bacillus Genetic Stock Center ID 13A1; Priest et al., 1997), B. laterosporus strains LMG 15441 (Bacillus Genetic Stock Center ID 40A1; Rivers et al., 1991) and DSM25 (Shida et al., 1996) were used as positive controls. Strains 1593 and LMG 15441 were purchased from the Bacillus Genetic Stock Center (Columbus, OH, United States); strain DSM25 was purchased from DSMZ – German Collection of Microorganisms and Cell Cultures GmbH (Braunschweig, Germany). Growth media employed in this work include Luria–Bertani medium (LB), T3 medium (3 g/L tryptone, 2 g/L tryptose, 1.5 g/L yeast extract, 0.05 M sodium phosphate (pH 6.8), 0.005 g/L MnCl2) (Martin and Travers, 1989) and BP medium (7 g/L Bactopeptone, 6.8 g/L KH2PO4, 0.12 g/L MgSO4⋅7H2O, pH 7.4; BP medium is completed by addition of 10 μM MnSO4⋅4H2O, 50 μM ZnSO4⋅7H2O, 50 μM FeSO4, 100 μM CaCl2⋅4H2O, 0.3% glucose just before use] (Lecadet et al., 1980).
Samples Collection, Screening, and Isolation of Bacteria With Larvicidal Activity Toward Ae. albopictus
Fourty-four soil samples were collected from different geographical areas [Supplementary Table 1: Italy (23), Cameroon (4), Zimbabwe (8), Philippines (3), United Kingdom (1), Cuba (1), Myanmar (1), Kenya (1), Pakistan (1), and Tajikistan (1)] for the isolation of bacteria with insecticidal activity toward larvae of Ae. albopictus. Soil samples (about 100 g each) were collected from 2 to 5 cm below the surface using a sterile spatula and transferred in sterile 50 mL Falcon tubes. Collected samples were transported to the laboratory and stored at 4°C.
A protocol for selecting and growing the sporulating and cultivable bacteria of each soil samples was developed. In order to enrich in entomopathogenic species, methods previously described for the isolation of B. thuringiensis from soil were combined and adapted (Travers et al., 1987; Santana et al., 2008; Patel et al., 2013). One gram of each sample was incubated at 80°C for 5 h before being inoculated in 20 mL LB medium supplemented with 0.25 M sodium-acetate. After 4 h of incubation at 30°C (200 rpm), 1 mL of culture was centrifuged at 1,000 rpm for 1 min to settle down soil particles. The supernatant was transferred into a sterile test tube and treated at 80°C for 10 min. Serial dilutions of each sample were spread on T3-agar and 0.25 mL of supernatant were used to inoculate 25 mL of T3 medium which is optimal for B. thuringiensis sporulation. After 48 h of growth at 30°C (200 rpm), each culture was diluted 50-fold in 25 mL of BP medium and incubated at 30°C, 200 rpm, for 72 h. BP is a complete medium for B. thuringiensis growth and sporulation. Appropriate dilutions (10–4, 10–6, 10–7, and 10–8) of each culture were spread onto T3-agar medium plates and the rest of the cultures were collected by centrifugation at 10,000 rpm for 10 min at 4°C. Pellets were washed three times with NaCl 1 M, EDTA 10 mM and twice with dH2O before being stored at −80°C or immediately used in larvicidal assays.
Cultures showing larvicidal activity were selected for further experiments. Colonies grown on T3-agar plates on which dilutions of active cultures had been spread were isolated to single colonies. Single clones were grown in 20 mL of BP for 72 h (30°C, 200 rpm) and pellets were collected, washed, and stored as described above before being assayed for their larvicidal activity.
Larvicidal Assays and Determination of LC50
All experiments were performed using Ae. albopictus Rimini strain which was reared at 28°C, in a 12 h-light/12 h-dark photoperiod, with 70% of humidity.
The mixtures of cells and spores obtained after culture of soil inocula were screened for their larvicidal activity using 10 second-instar larvae of Ae. albopictus Rimini strain placed in 10 mL of dH2O without any nutritional supplement. Pellets were resuspended in dH2O and added at the final concentration of 2 g/L (expressed as biomass wet weight/litre). The same conditions were used in bioassays performed to screen individual bacteria isolates for potential larvicidal activity. In these screening assays, the concentration of 2 g/L was chosen to be able to detect activity even in soil samples with a low concentration of larvicidal bacteria and in bacterial isolates with low activity. A negative control group only exposed to distilled water was included in each experiment. Suspensions of L. sphaericus strain 1593 and B. laterosporus strain LMG 15441 collected after 72 h of growth in BP medium were used as positive controls. Mortality was recorded at 24 h intervals until 72 h from the beginning of the assay. The experiments were carried out at room temperature (22–25°C) in a laboratory location exposed to natural sunlight. Each test was performed in duplicate. Survival curves of each isolate were compared to those of the positive controls using a Long-Rank (Mantel–Cox) test in Prism GraphPad; P-values were corrected using False Discovery Rate.
Dose-response curves for strains SAM19, LMG 15441, and DSM25 were performed using 25 second-instar larvae of Ae. albopictus Rimini strain placed in 100 mL of dH2O. Larvae were exposed to serial dilutions (range from 320 to 5 mg/L) of cells-spores suspensions in distilled water, without adding food. For each strain, five to seven concentrations were tested alongside a negative control group. Each test was performed in duplicate (technical replicate) and the entire experiment was repeated three times. The number of cfu and spores per mL used in the assay was determined by plating appropriate dilutions of each suspension before and after heat treatment at 80°C for 10 min. The experiments were carried out at room temperature and mortality was recorded at 24 and 48 h. Mortality was calculated according to the following formula:
where X = percentage survival in the untreated control and Y = percentage survival in the treated sample.
Based on the obtained data, we performed a probit analysis using R studio (library ecotox and SciViews) and the results were visualized using ggplot2 (R Core Team, 2020).
Identification of Isolates by PCR and 16S rRNA Sequencing
Selected bacterial isolates showing larvicidal activity against Ae. albopictus were initially identified by performing colony PCRs targeting conserved regions of genes encoding known entomopathogenic toxins of L. sphaericus, B. thuringiensis var. israelensis and B. laterosporus. Primers employed in this work are reported in Table 1. Genes encoding the mosquito larvicidal toxins BinA, BinB, Mtx1, Mtx2, and Mtx3 of L. sphaericus were amplified by colony multiplex PCR (Jagtap et al., 2009). The same approach was used for the detection of B. thuringiensis var. israelensis toxin genes cry4, cry10, and cry11 (Vidal-Quist et al., 2009). For the identification of B. laterosporus strains, a set of primers targeting the gene encoding the conserved 28 kDa spore surface protein was employed (Marche et al., 2019). The taxonomic classification of SAM19 was determined by sequencing the V3–V4 hypervariable regions of the 16S rRNA gene. The fragment of interest was amplified using primers 16Sf (5′-CCTACGGGNGGCWGCAG) and 16Sr (5′-GACTACHVGGGTATCTAATCC) (Klindworth et al., 2013).
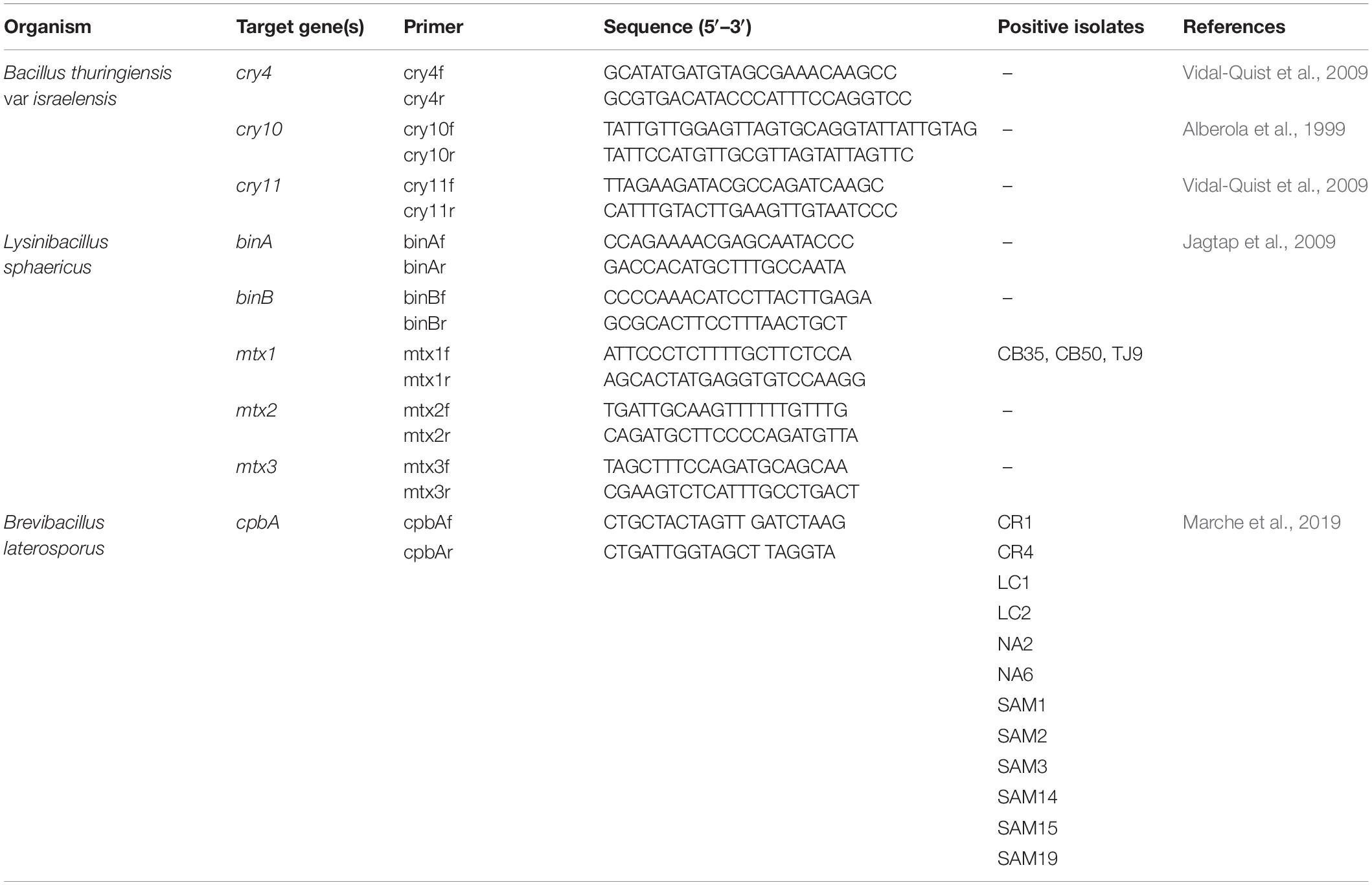
Table 1. Primers used for the detection of mosquitocidal toxin genes in the isolates with larvicidal activity against Ae. albopictus.
Transmission Electron Microscopy
Samples were collected at different time points during growth in BP medium at 30°C and pellets were prefixed in Karnowsky’s fixative in cacodylate buffer (pH 7.2). After post-fixation in 2% OsO4 in 0.1 M cacodylate buffer for 1.5 h at 4°C, samples were washed, dehydrated through a progressive ethanol gradient, transferred to propylene oxide and embedded in Epon 812. Thin sections (80 nm) were stained with saturated uranyl acetate, followed by Reynolds lead citrate and examined with Zeiss EM900 transmission electron microscope at 80 kV.
Genomic Analysis of SAM19
Genomic DNA was extracted from SAM19 using the DNeasy Blood and Tissue Kit (QIAGEN). DNA sequencing was performed by Illumina MiSeq with a Nextera-XT pair-end library. 1,308,373 read pairs were produced. After a preliminary quality check with FastQC (Wingett and Andrews, 2018), reads were assembled with SPAdes 3.10 (Bankevich et al., 2012). The genome was annotated with Prokka (Seemann, 2014). Accordingly, the genome assembly was polished by removing short (<500 bp) contigs bearing no annotated gene. The draft genome sequences of B. laterosporus SAM19 has been deposited in GenBank under accession number JADGMT000000000.
For phylogenomic analysis, the predicted protein sequences of a representative set of 12 B. laterosporus genomes, plus Bacillus agri as outgroup, were downloaded from NCBI. Maximum likelihood phylogenomic analyses were performed on a concatenated set of single copy orthogroups, as previously described (Floriano et al., 2018).
In order to perform comparative analyses on genome structure and content, the genome sequences of two closely related strains from phylogenomics (DSM25 and BGSP7) were downloaded from NCBI. After reciprocal reorientation of contigs in case of draft assemblies, synteny analyses were performed with MUMmer (Delcher et al., 2002), using the NUCmer aligner and filtering out alignments <1,000 bp and <80% identity. Average nucleotide identity (ANI) values were calculated with the Enveomics online suite (Rodriguez-R and Konstantinidis, 2016). Annotation of possible antimicrobial molecules was performed using the on-line software AntiSMASH (Antibiotics and Secondary Metabolite Analysis Shell; Medema et al., 2011) and BAGEL4 (de Jong et al., 2006).
Clusters of Orthology Groups (COGs) in the predicted proteins of SAM19 and the two references were predicted with the NCBI pipeline (Galperin et al., 2015), and the respective COG repertoires directly compared.
Results
Isolation of Bacterial Strains With Larvicidal Activity Against Ae. albopictus
The sporulating and cultivable bacterial communities of 44 soil samples were grown and screened for their larvicidal activity against second instar larvae of Ae. albopictus. To this purpose, based on methods previously described for the isolation of B. thuringiensis from soil samples (Travers et al., 1987; Santana et al., 2008; Patel et al., 2013), a new strategy for the selection and cultivation of sporulating bacteria enriched in entomopathogenic strains was developed (described in section “Materials and Methods” and Figure 1). The mixtures of cells and spores obtained from each soil sample were resuspended in dH2O and used in larvicidal assays at the final concentration of 2 g/L (Figure 1). After 24 h of treatment, eight mixtures of soil bacteria (YA, CB, AR, LC, TC, VV, NA, and TJ) caused more than 30% larval mortality. Larvicidal activity increased over time and, 48 h after the beginning of the assay, five additional suspensions of soil bacteria (CR, LB, SAM, ZBC, and ZBD) killed at least 30% of Ae. albopictus larvae (Figure 2A). For each of the final 13 active samples, purified clones were grown in BP medium and the mixtures of cells and spores were harvested after 72 h of growth. Pellets were used in larvicidal assays at the final concentration of 2 g/L. After 24 h from the beginning of the assay, 10 bacterial clones isolated from three different soil samples induced more than 30% mortality against second instar larvae of Ae. albopictus. Five additional clones isolated from Cuba, Kenya, and Tajikistan also displayed larvicidal activity, but the percentage of larval mortality induced at 24 h was below 30% (Figure 2B). Larval mortality induced by each strain increased over time, reaching 100% in the case of clones isolated from soil collected in the Sampaloc lake area (Philippines) (Figure 2B). Furthermore, statistical analysis of the survival curves indicated that the Philippine isolates, with the exception of SAM3, were the only strains not being significantly different (p > 0.05) from the two positive controls (L. sphaericus and B. laterosporus), suggesting a similar larvicidal activity.
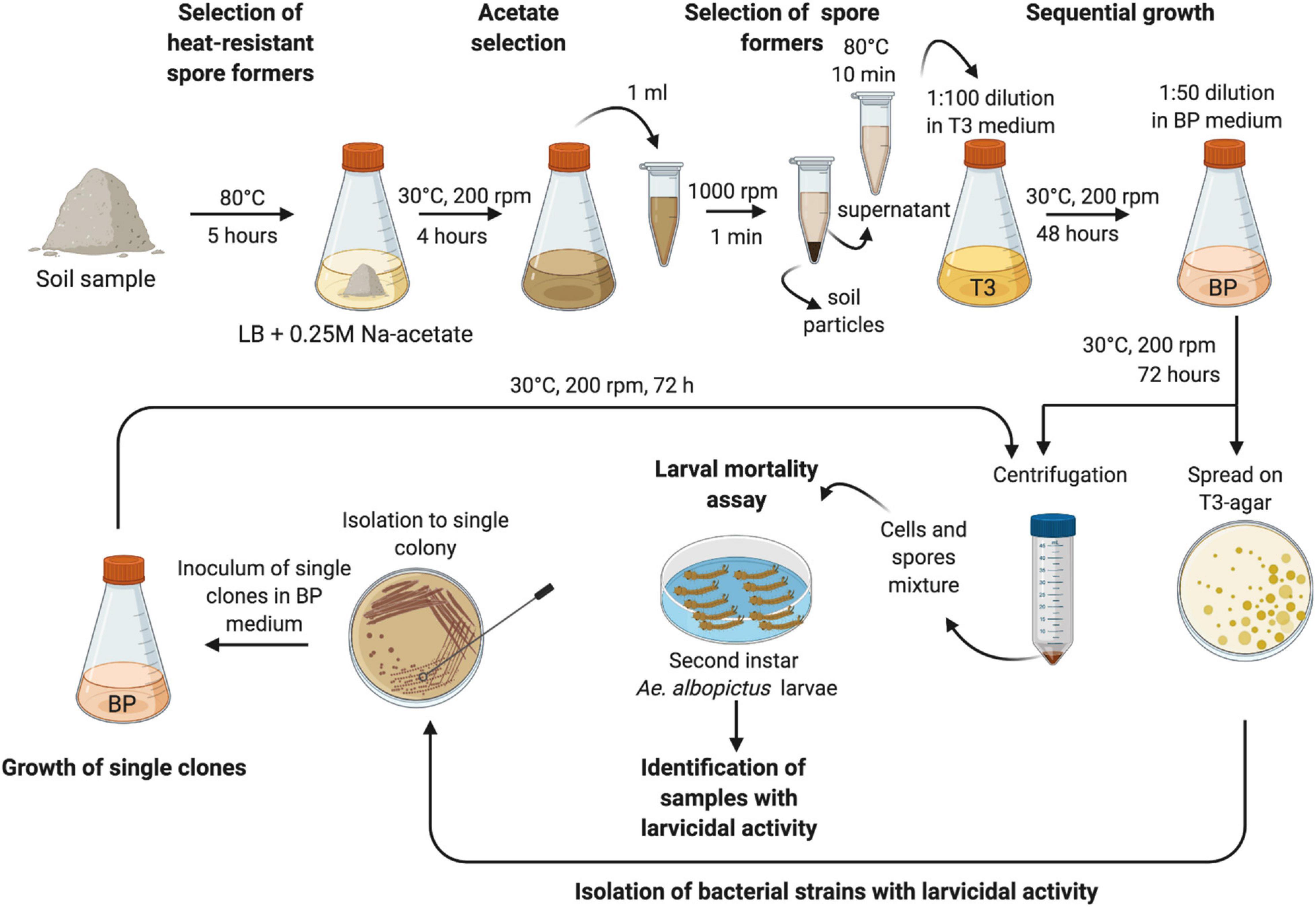
Figure 1. Schematic representation of the protocol used for the identification of soil samples enriched with bacteria with insecticidal activity and for the isolation of bacterial strains with larvicidal activity against Ae. albopictus. Created with BioRender.com.
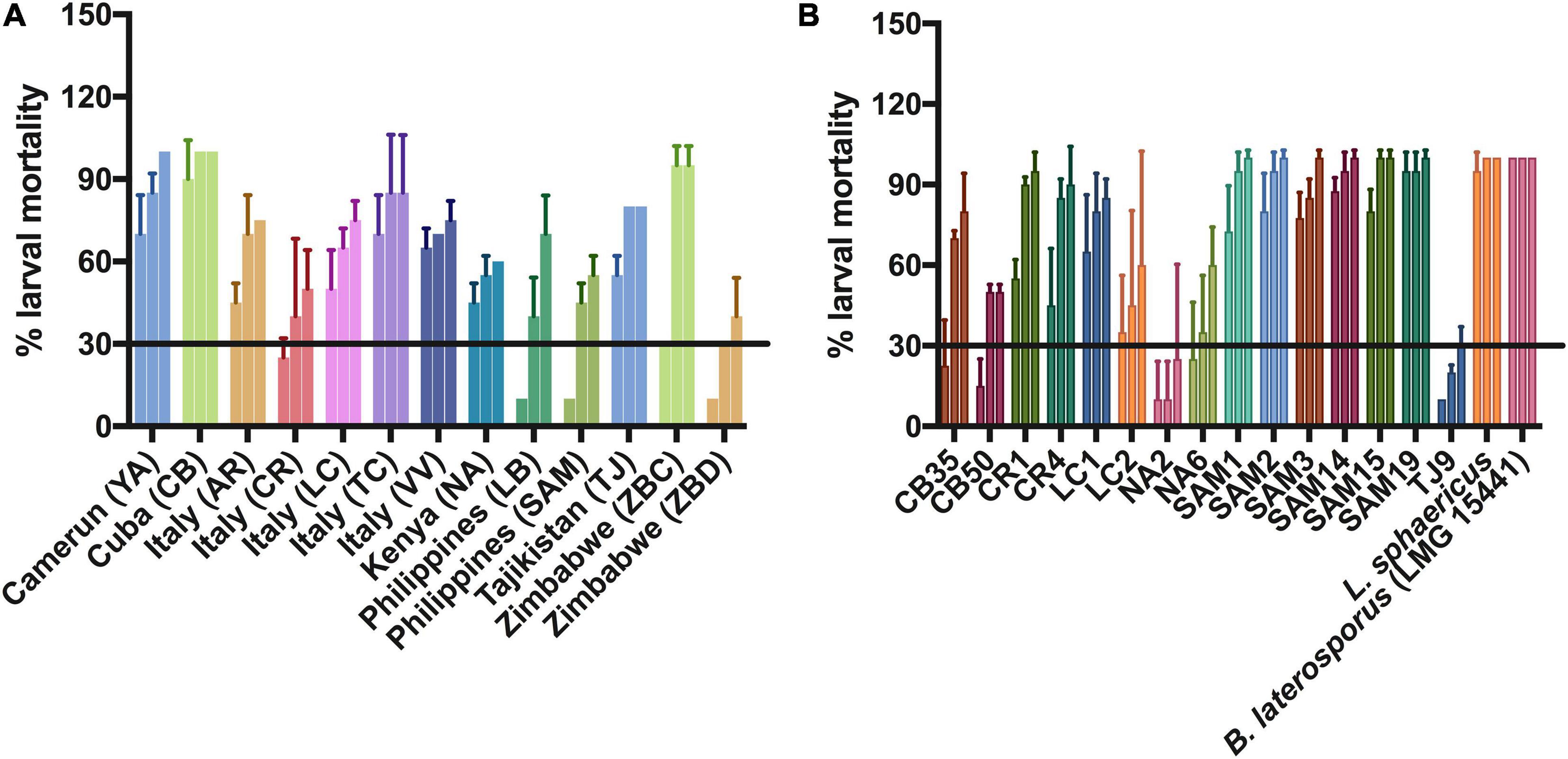
Figure 2. Percentage of Ae. albopictus larval mortality at 24, 48, and 72 h after treatment with mixtures of cells and spores of cultures originating from the inoculum of 13 soil samples (A) or of single bacterial isolates (B). Suspensions were added at the final concentration of 2 g/L. For each sample, the first, second and third bar represent larval mortality at 24, 48, and 72 h, respectively. Under the employed conditions, treatment with B. laterosporus strain LMG 15441 induced 100% of larval mortality in all experiments [Standard deviation (SD) = 0]. Data are the average ± SD of two independent experiments, each performed in duplicate.
Identification of the Mosquitocidal Isolates
In order to assess whether the isolated clones belonged to known entomopathogenic bacterial species, their genomic DNA was used as a template in PCR reactions targeting known entomopathogenic toxin-encoding genes of the species B. thuringiensis var. israelensis, L. sphaericus, and B. laterosporus (Table 1). While no amplification products were obtained using primers pairs annealing to conserved regions of the B. thuringiensis var. israelensis toxin genes cry4, cry10, and cry11, an amplicon corresponding to the L. sphaericus mtx1 toxin gene was obtained in three (CB35, CB50, and TJ9) of the five clones with lower larvicidal activity (Figure 2B and Table 1). More interestingly, the use of primers targeting a gene encoding a highly conserved 28 kDa spore surface protein of B. laterosporus yielded an amplification product in all other active clones (Table 1; Marche et al., 2019).
Due to their low level of activity, the L. sphaericus isolates CB35, CB50, and TJ9 (Figure 2B) were not studied any further. Among the B. laterosporus clones, those isolated from soil collected in the Sampaloc lake area (Philippines, SAM) induced higher mortality; clone SAM19, displaying the highest larvicidal activity after 24 h of treatment, was selected for further characterization.
BLAST analysis of its 16S rRNA gene sequence confirmed its classification as B. laterosporus. Cell and spore morphology were examined by transmission electron microscopy (TEM). To this purpose, SAM19 and the wild type B. laterosporus reference strain LMG 15441 were grown in synchronized cultures in BP medium and cultures were collected at different time points. As shown in Figure 3, during late stationary phase (24 h), an electrodense structure, probably related to the nascent parasporal body, could be observed at one pole of SAM19 spores, which were still contained within their mother cells. At 48 h, when sporulation was complete, a lamellar CSPB, firmly anchored to one side of the spore coat, was observed. The morphology of the mature spore appears similar to that of the reference strain LMG 15441 and to other B. laterosporus strains with entomopathogenic activity against Diptera (Marche et al., 2017).
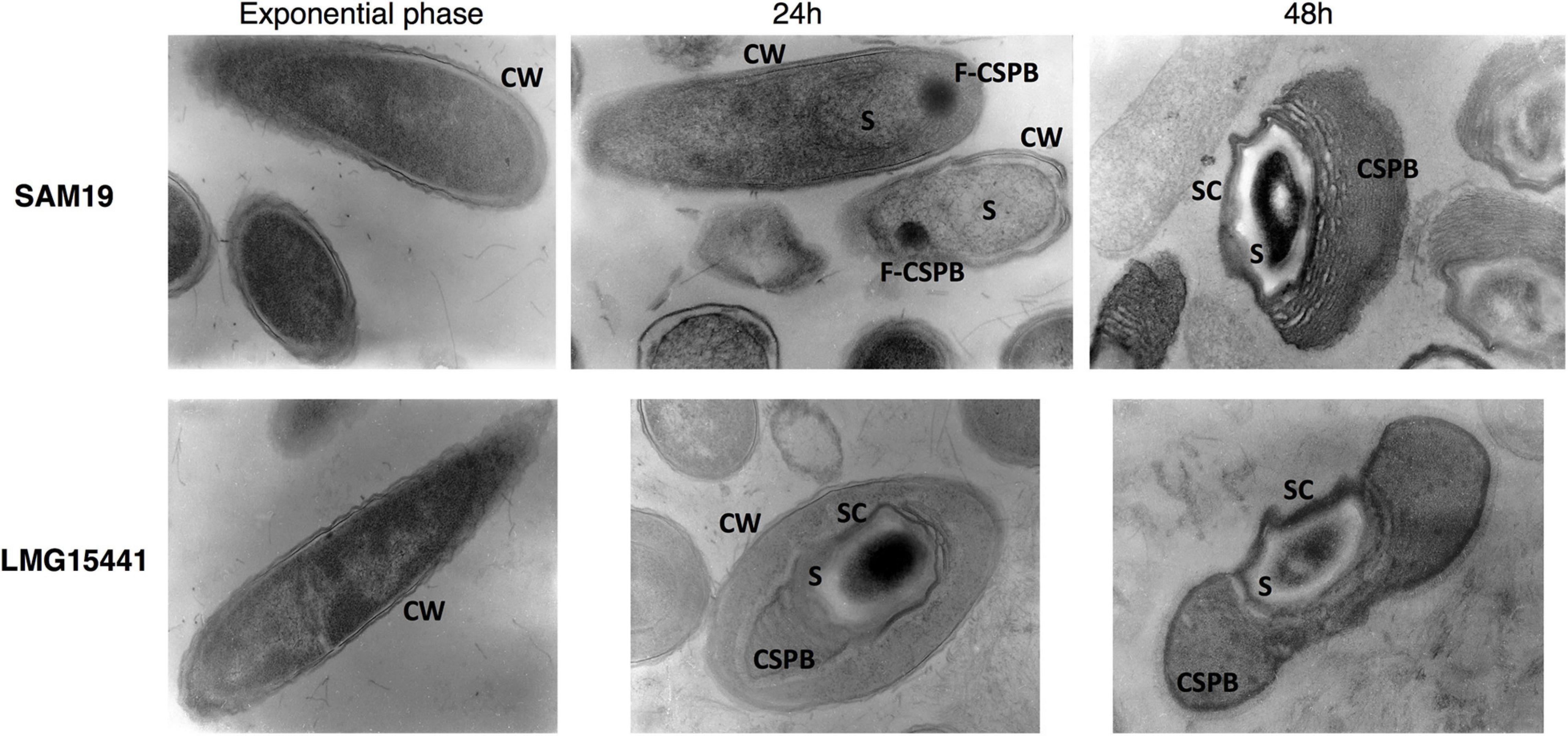
Figure 3. Transmission electron microscopy (TEM) images of Brevibacillus laterosporus strains SAM19 and LMG 15441 at different growth stages: Vegetative cell during exponential phase; Sporangium including forming spore at 24 h after inoculum; Free spore. CW, cell wall; S, spore; SC, spore coat; F-CSPB, forming canoe-shaped parasporal body; CSPB, canoe-shaped parasporal body.
Genome Sequence and Phylogenomic Analysis of B. laterosporus SAM19
The final draft genome assembly suggests that the chromosome of SAM19 is 5,550,463 bp-long (174 contigs; N50 = 90,219 bp; L50 = 20, largest contig = 316,450 bp; GC = 40.1%): 5,252 protein coding genes and 108 ncRNA genes were annotated.
Phylogenomic analyses confirmed the assignment of SAM19 to the B. laterosporus species (Figure 4). In particular, this new isolate forms a monophyletic lineage (100% support) with strains BGSP7 and DSM25, being more closely related with BGSP7 (93% support). These two strains were thus selected for more detailed comparative analyses.
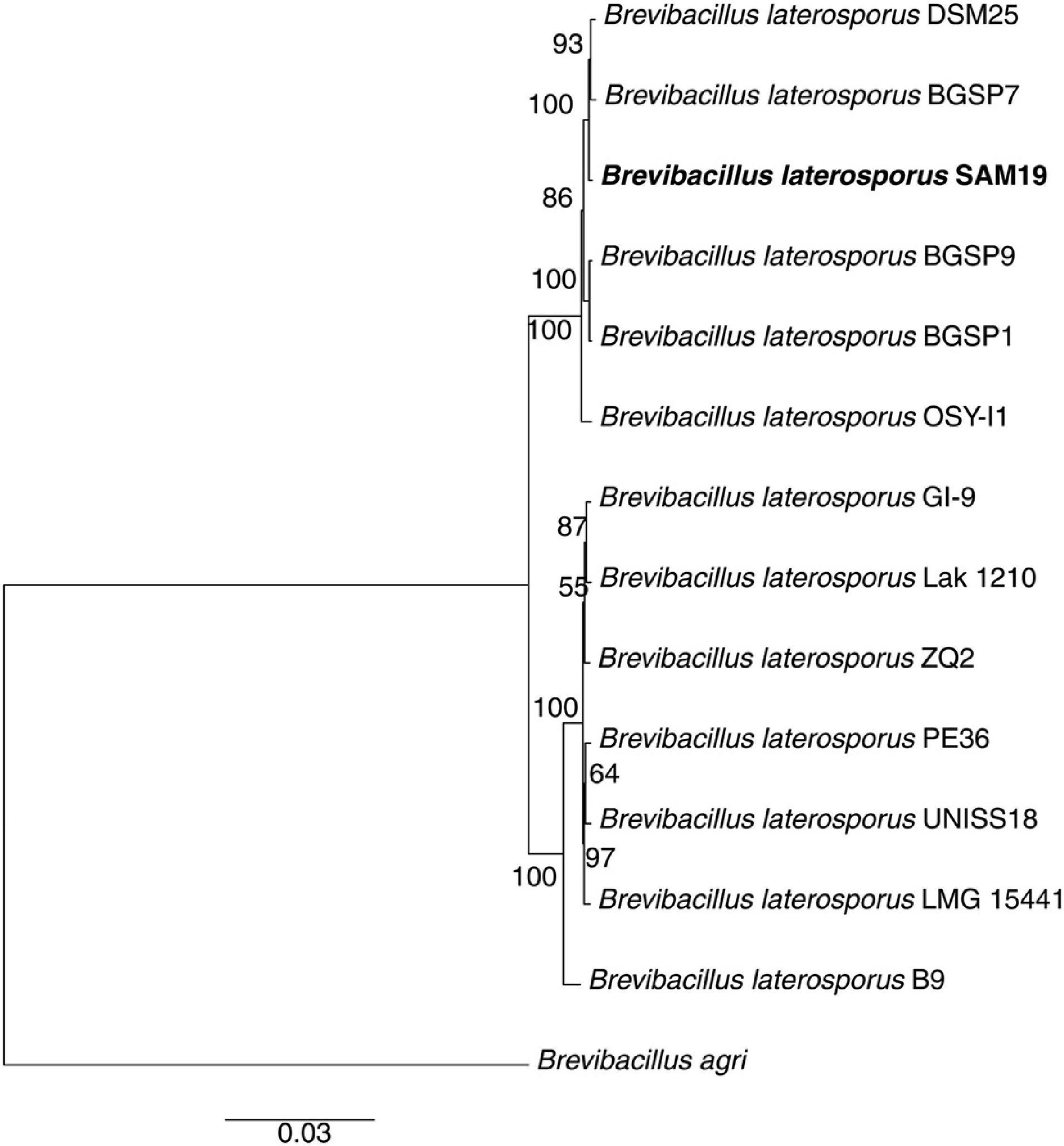
Figure 4. Maximum likelihood phylogenomic tree obtained with RAxML (Stamatakis, 2015) showing the relationships of SAM19 with representative B. laterosporus strains. Numbers of branches stand for bootstrap supports with 100 pseudo-replicates, scale bar for estimated proportional sequence divergence.
Larvicidal Activity of B. laterosporus SAM19
The larvicidal activity of SAM19 was determined and compared with that of the phylogenetically proximal strain DSM25. Strain LMG 15441 was included in the analysis: despite being on a different phylogenetic branch with respect to SAM19, it shows phylogenetic proximity with UNISS18, a B. laterosporus strain with entomopathogenic activity (Ruiu et al., 2007; Figure 4). As reported in Figure 5, the lethal effects were concentration dependent and at 24 h post infection SAM19 displayed the highest larvicidal activity, with a LC50 of 101.233 mg/L (confidential limits 101.154–101.307), corresponding to 105.287 cfu/mL (105.192–105.381) and 105.153 spores/mL (105.077–105.229) (Supplementary Table 2). SAM19 LC50 values did not change significantly after 48 h (Supplementary Figure 1 and Supplementary Table 2), confirming that the effect on larval viability is exerted within 24 h since exposure to the strain suspension. Interestingly, for all three strains LC50 values expressed as cfu/mL and spores/mL are similar to each other (Supplementary Table 2), consistent with the fact that larvicidal activity is associated with the spore.
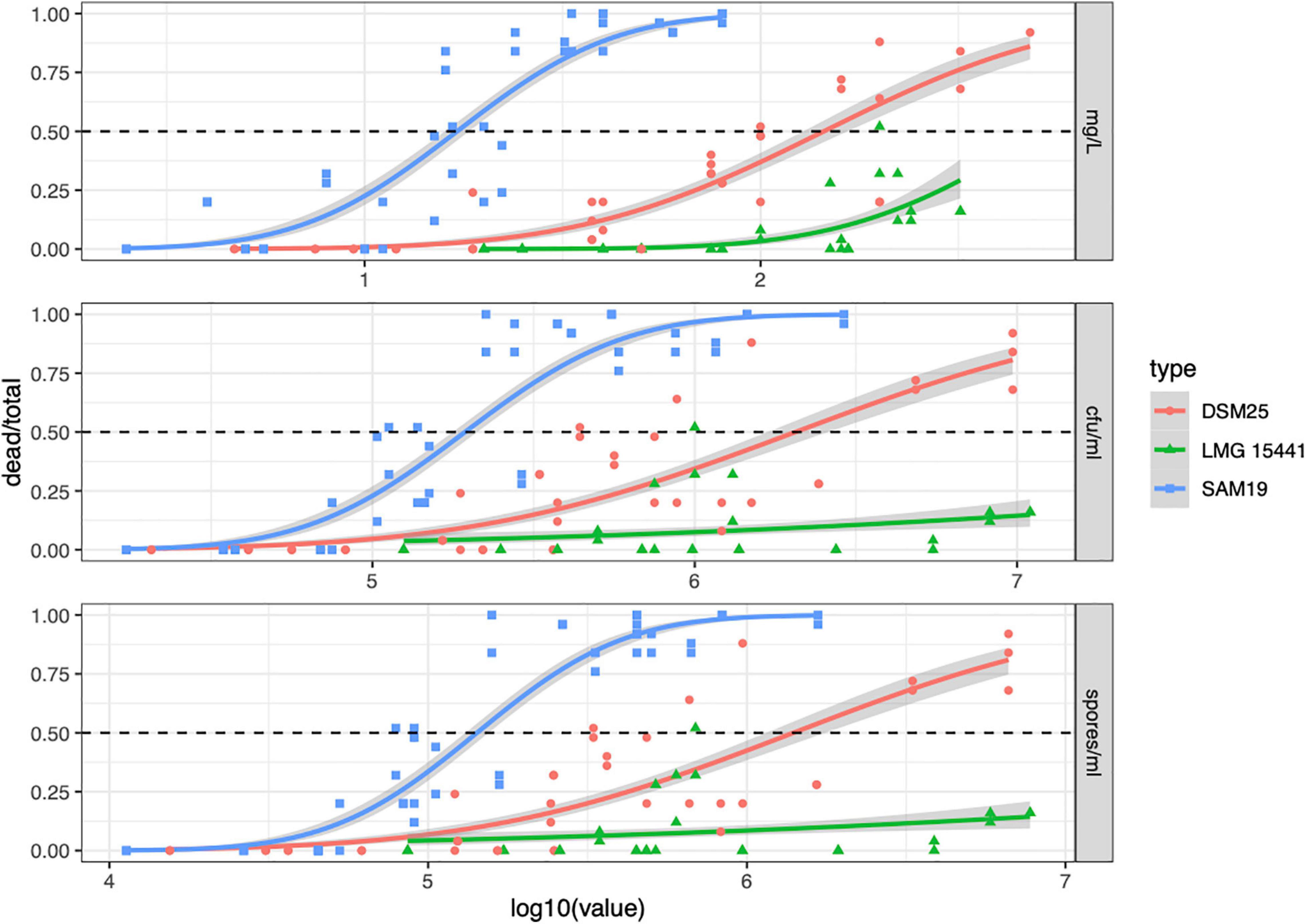
Figure 5. Probit analysis of mortality data of Aedes albopictus larvae treated with Brevibacillus laterosporus strains SAM19, DSM25, and LMG 15441. The analysis was performed on data collected at 24 h after exposure to bacteria. The dead/total ratio is reported as a function of the concentration (log10) of each suspension expressed as mg/L (upper panel), cfu/mL (middle panel) or spores/mL (lower panel).
Gene Content Analysis
In order to investigate whether the lower LC50 of SAM19 could be associated to unique functions encoded by the new isolate, its genome was compared with those of DSM25 and BGSP7. Coherently with phylogenetic proximity, SAM19 showed a very high level of synteny (14 and 8 inversions) and an ANI of 99.11 and 99.13% with DSM 25 and BGSP7, respectively (Supplementary Figure 2).
93.3% of the 1,910 COGs present on the genome of SAM19 were shared with the other two strains; only 75 were unique to this new isolate. BLAST analysis of the corresponding proteins revealed that homologues with >95% sequence identity were present in other B. laterosporus strains. No clear candidates with a potential involvement in virulence could be identified (Supplementary Datasheet 1).
As SAM19 is phylogenetically close to the antimicrobials producer strain BGSP7, its genome was also searched for the presence of genes coding for secondary-metabolites biosynthesis and bacteriocins using the online bioinformatic tools AntiSMASH (Antibiotics and Secondary Metabolite Analysis Shell; Medema et al., 2011) and BAGEL4 (de Jong et al., 2006). Non-ribosomal peptide synthetase clusters encoding bogorol A and brevicidine were identified by AntiSMASH analysis. Moreover, SAM19 genome displayed the potential to produce numerous bacteriocins, including laterosporulin, sactipeptides, UviB, and lanthipeptide class I (Supplementary Table 3).
Discussion
Bacillus thuringensis is the most widely employed biopesticide. Its high insecticidal activity, the specificity of each subspecies for a limited clade of insects (Ehling-Schulz et al., 2019), its low environmental impact and the possibility of using genes encoding toxic proteins to generate transgenic organisms make the use of B. thuringiensis a successful strategy to control insect pests. Currently, there are more than 98 formulated bacterial pesticides commercially available (Lacey et al., 2015).
The two main pitfalls of the large use of B. thuringiensis are its short lifetime and concerns about public health. As the stability of insecticidal crystalline proteins is hampered by solar radiation, effective control of insect pests requires continuous spraying of B. thuringensis formulations. Furthermore, B. thuringensis belongs to the Bacillus cereus group together with Bacillus cereus sensu stricto, Bacillus anthracis, Bacillus weihenstephanensis, Bacillus mycoides, Bacillus pseudomycoides, Bacillus cytotoxicus, and Bacillus toyonensis. B. cereus is recognized to secrete the emetic toxin cereulide, coded by the ces operon (Agata et al., 1995). Although there is no evidence that B. thuringiensis secretes it, concerns were raised by the European Food Safety Authority on the possibility that some B. thuringensis strains might contain genes for its synthesis, or that some formulations might contain other B. cereus species, requiring the full sequencing of the B. thuringensis strains used (EFSA Panel on Biological Hazards (Biohaz), 2016). Notably, ces genes are located in a transposable element in B. weihenstephanensis (Mei et al., 2014), raising questions about the possible horizontal transfer between species.
In view of these premises, the search for possible alternative biopesticides is increasing, particularly to control vector-borne diseases. It is worth noting that vector control is still the most effective strategy for the prevention of many insect-borne diseases, particularly for mosquito-borne diseases, including malaria, dengue and Zika. Their endemic area are the tropical and subtropical regions, but climate change and intense human commercial activities are expanding the geographical distribution of many mosquito species, allowing the transmission of mosquito-borne diseases in new countries. This is the case of Ae. albopictus, which spread from South-East Asia to all over the world and which caused in the last decade several outbreaks of chikungunya and dengue in Europe (Benedict et al., 2007; Gossner et al., 2018).
In this context, this work was aimed at isolating novel cultivable bacterial strains with insecticidal activity toward larvae of Ae. albopictus. To this purpose, a novel method to rapidly identify soil samples colonized by bacteria with larvicidal activity was developed. In order to have a collection as diversified as possible, samples were collected from different areas of Italy, from African (Kenya, Zimbabwe, and Camerun) and Asian countries (Pakistan, Tajikistan, Philippines, and Myanmar) as well as from Cuba and the United Kingdom. Different environments such as city, rural areas, agricultural lands, river basins, and lake areas were sampled (Supplementary Table 1). The classical protocol for the isolation of strains of B. thuringiensis var. israelensis is based on acetate selection followed by heat treatment: soil is inoculated in rich liquid medium supplemented with 0.25 M sodium acetate and, after 4 h of incubation at 30°C with aeration, culture supernatant is heated at 80°C. Since B. thuringiensis spores do not germinate in the presence of 0.25 M sodium acetate, germinated bacteria of species other than B. thuringiensis are heat killed (Travers et al., 1987). However, since B. thuringiensis concentration in soil can be very low, this strategy was reported to have low efficiency and alternative methodologies, including dry-heat pre-treatment of soil samples and enrichment method, were developed (Santana et al., 2008; Patel et al., 2013). All these methods require microscopic examinations of single clones for their ability to produce spores and crystals after at least 72 h of growth on sporulation agar-medium. Isolating, cultivating, and assaying the larvicidal activity of a high number of single clones for each soil sample is a time-consuming procedure. To overcome this limitation, we developed a new two steps procedure. In the first step, a mixed population of sporulating cultivable bacteria was obtained and tested for its larvicidal capacity. In the second step, single bacterial clones obtained from active soil samples were tested for their entomopathogenic activity. This method provides two major advantages: (i) multiple soil samples can be assayed in parallel and (ii) the bacterial population obtained from each soil contains a high percentage of active bacteria. We cannot exclude that entomopathogenic bacteria could be present at low density even in soil samples that displayed low or null larvicidal activity under employed conditions. Surprisingly, the majority of active clones were not B. thuringiensis var. israelensis strains but were identified as L. sphaericus and B. laterosporus, two known entomopathogenic species. While L. sphaericus isolates showed lower activities compared to the control reference strain 1593, isolated B. laterosporus strains displayed high toxicity.
Brevibacillus laterosporus has attracted increasing attention as a producer of antimicrobial compounds and secondary metabolites and is used as probiotic for humans (Ruiu, 2013). Importantly, strains with insecticidal activities against Diptera, Lepidoptera, and Coleoptera have been reported, making it an important candidate for the biocontrol of different pests with very limited risks for public health (de Oliveira et al., 2004; Ruiu, 2013).
The genome of the newly isolated strain was sequenced and the phylogenomic analysis revealed its close proximity to strains DSM25 and BGSP7. DSM25 is a known entomopathogen active against Culex quinquefasciatus and Aedes aegypti (Favret and Yousten, 1985; Rivers et al., 1991). BGSP7 was recently isolated from silage and selected for its ability to produce antimicrobial molecules active against Gram-negative (Klebsiella pneumoniae Ni9 and Pseudomonas aeruginosa MMA83) and Gram-positive (Staphylococcus aureus ATCC25923 and Listeria monocytogenes ATCC19111) multi-drug resistant pathogens (Miljkovic et al., 2019). Moreover, antifungal activity and toxicity toward larvae and adults of the potato beetle Leptinotarsa decemlineata were reported for BGSP7 (Miljkovic et al., 2019).
Even though the possible antimicrobial activity of SAM19 was not investigated in this work, bioinformatic analysis of its genome provided evidence of the presence of genes encoding antimicrobials.
The genome of SAM19 revealed the presence of 75 COGs not shared with its closest relatives DSM25 and BGSP7. However, analysis of the corresponding proteins did not highlight any specific feature suggesting higher toxicity of SAM19 compared to DSM25. Interestingly, SAM19 unique COGs included different phage related proteins, suggesting the possibility that phage functions and lysogenic conversion could play a role in the increased entomopathogenic activity.
The insecticidal activity of some strains of B. laterosporus has been associated to the presence of cytoplasmic inclusions containing insecticidal crystal proteins (Orlova et al., 1998). As revealed by TEM observations, SAM19 does not display intracellular crystalline inclusions. Entomopathogenic B. laterosporus strains lacking parasporal crystals have been previously described. Among these, the strain UNISS18 is characterized by insecticidal activity against Musca domestica and Ae. aegypti (Ruiu et al., 2007). This strain, however, is phylogenomically distant from SAM19. Its pathogenicity has been correlated to different virulence factors, including two surface proteins, CbpA and CbpB, associated to the CSPB. While a gene (LICBGMMG_04116) encoding a protein with 91.89% identity to UNISS18 CpbA was identified in SAM19, the new isolate did not display any CpbB homologue. A BLAST search analysis revealed that SAM19 encodes proteins previously identified as putative virulence factors expressed by UNISS18 during growth in the insect body (Marche et al., 2018). These proteins, with a high level of sequence identity (81.41–100%) with their UNISS18 homologues (Supplementary Table 4) include chitinases ChiA and ChiB (LICBGMMG_02893 Chitinase A1; LICBGMMG_01497 Chitodextrinase), a collagenase-like protease PrtC (LICBGMMG_01889), GlcNAc-binding protein (LICBGMMG_01411), protective antigen proteins (LICBGMMG_05212; LICBGMMG_04772; LICBGMMG_05296), bacillolysin (LICBGMMG_04689), thermophilic serine proteinase (LICBGMMG_02557), and the insecticidal toxin Mtx (LICBGMMG_04980 Epsilon-toxin type B). The toxicity of the new isolate against Ae. albopictus could therefore result from the concerted activity of a variety of virulence factors.
Further studies are needed to better characterize the toxicity of the newly identified SAM19 B. laterosporus strain against other mosquito and, more generally, Diptera species and to test the strain stability in field settings, using formulated or unformulated preparations. The higher toxicity of SAM19, compared with other B. laterosporus strains used as reference, supports the possibility of adding this strain to the arsenal of eco-compatible tools that can be used to control mosquitoes and to limit the spread of invasive species and their associated diseases. Noteworthy, since B. laterosporus strains have been previously described for their toxicity to insects (Coleoptera, Lepidoptera, and Diptera), nematodes and mollusks (Ruiu, 2013), it will be important to assess possible off-target effects of SAM 19 to other species and organisms. Furthermore, the identification of genes encoding antimicrobial molecules suggests its potential use for antimicrobial production.
Data Availability Statement
The datasets presented in this study can be found in online repositories. The names of the repository/repositories accession number(s) can be found below: https://www.ncbi.nlm.nih.gov/genbank/, JADGMT000000000; https://www.ncbi.nlm.nih.gov/biosample/, SAMN16515084.
Author Contributions
GB, PG, EF, GG, and AA contributed to the conception and design of the study. GB, SM, PG, VS, and EU screened the collection of soil samples, isolated bacterial strains with larvicidal activity against Ae. albopictus, and identified and characterized SAM19. CF, MC, and DS performed SAM19 genome assembly, annotation, and phylogenomic analysis. GR, EC, and LS performed TEM analysis of SAM19. GB, PG, MC, and DS drafted the manuscript. GB, PG, and EF finalized the manuscript. All authors read and approved the final manuscript.
Funding
This work was supported by a Fondazione Bussolera-Branca research grant to AA and GG and by the Italian Ministry of Education, University and Research (MIUR): Dipartimenti di Eccellenza Program (2018–2022) – Department of Biology and Biotechnology “L. Spallanzani”, University of Pavia (GB, DS, GG, and AA).
Conflict of Interest
The authors declare that the research was conducted in the absence of any commercial or financial relationships that could be construed as a potential conflict of interest.
Acknowledgments
We are grateful to Mrs. Elisabetta Andreoli (Department of Biology and Biotechnology, University of Pavia) for expert technical assistance.
Supplementary Material
The Supplementary Material for this article can be found online at: https://www.frontiersin.org/articles/10.3389/fmicb.2021.624014/full#supplementary-material
References
Agata, N., Ohta, M., Mori, M., and Isobe, M. (1995). A novel dodecadepsipeptide, cereulide, is an emetic toxin of Bacillus cereus. FEMS Microbiol. Lett. 129, 17–20. doi: 10.1016/0378-1097(95)00119-P
Alberola, T. M., Aptosoglou, S., Arsenakis, M., Bel, Y., Delrio, G., Ellar, D. J., et al. (1999). Insecticidal activity of strains of Bacillus thuringiensis on larvae and adults of Bactrocera oleae Gmelin (Dipt. Tephritidae). J Invertebr Pathol. 74, 127–136. doi: 10.1006/jipa.1999.4871
Alexander, B., and Priest, F. G. (1990). Numerical classification and identification of Bacillus sphaericus including some strains pathogenic for mosquito larvae. J. Gen. Microbiol. 136, 367–376. doi: 10.1099/00221287-136-2-367
Bankevich, A., Nurk, S., Antipov, D., Gurevich, A. A., Dvorkin, M., Kulikov, A. S., et al. (2012). SPAdes: a new genome assembly algorithm and its applications to single-cell sequencing. J. Comput. Biol. 19, 455–477. doi: 10.1089/cmb.2012.0021
Becker, N., and Ludwig, M. (1993). Investigations on possible resistance in Aedes vexans field populations after a 10-year application of Bacillus thuringiensis israelensis. J. Am. Mosq. Control. Assoc. 9, 221–224.
Ben-Dov, E. (2014). Bacillus thuringiensis subsp. israelensis and its dipteran-specific toxins. Toxins (Basel) 6, 1222–1243. doi: 10.3390/toxins6041222
Benedict, M. Q., Levine, R. S., Hawley, W. A., and Lounibos, L. P. (2007). Spread of the tiger: global risk of invasion by the mosquito Aedes albopictus. Vector Borne Zoonotic Dis. 7, 76–85. doi: 10.1089/vbz.2006.0562
Berry, C., O’Neil, S., Ben-Dov, E., Jones, A. F., Murphy, L., Quail, M. A., et al. (2002). Complete sequence and organization of pBtoxis, the toxin-coding plasmid of Bacillus thuringiensis subsp. israelensis. Appl. Environ. Microbiol. 68, 5082–5095. doi: 10.1128/aem.68.10.5082-5095.2002
Boyer, S., Tilquin, M., and Ravanel, P. (2007). Differential sensitivity to Bacillus thuringiensis var. israelensis and temephos in field mosquito populations of Ochlerotatus cataphylla (Diptera: Culicidae): toward resistance? Environ. Toxicol. Chem. 26, 157–162. doi: 10.1897/06-205r.1
Darboux, I., Pauchet, Y., Castella, C., Silva-Filha, M. H., Nielsen-LeRoux, C., Charles, J. F., et al. (2002). Loss of the membrane anchor of the target receptor is a mechanism of bioinsecticide resistance. Proc. Natl. Acad. Sci. USA. 99, 5830–5835. doi: 10.1073/pnas.092615399
de Jong, A., van Hijum, S. A., Bijlsma, J. J., Kok, J., and Kuipers, O. P. (2006). BAGEL: a web-based bacteriocin genome mining tool. Nucleic. Acids Res. 34, W273–W279. doi: 10.1093/nar/gkl237
de Oliveira, E. J., Rabinovitch, L., Monnerat, R. G., Passos, L. K., and Zahner, V. (2004). Molecular characterization of Brevibacillus laterosporus and its potential use in biological control. Appl. Environ. Microbiol. 70, 6657–6664. doi: 10.1128/AEM.70.11.6657-6664.2004
Delcher, A. L., Phillippy, A., Carlton, J., and Salzberg, S. L. (2002). Fast algorithms for large-scale genome alignment and comparison. Nucleic. Acids Res. 30, 2478–2483. doi: 10.1093/nar/30.11.2478
Du, J., Knowles, B. H., Li, J., and Ellar, D. J. (1999). Biochemical characterization of Bacillus thuringiensis cytolytic toxins in association with a phospholipid bilayer. Biochem. J. 338 (Pt 1), 185–193. doi: 10.1042/0264-6021:3380185
EFSA Panel on Biological Hazards (Biohaz). (2016). Risks for public health related to the presence of Bacillus cereus and other Bacillus spp. including Bacillus thuringiensis in foodstuffs. EFSA J. 14, 4524. doi: 10.2903/j.efsa.2016.4524
Ehling-Schulz, M., Lereclus, D., and Koehler, T. M. (2019). The Bacillus cereus Group: Bacillus species with Pathogenic Potential. Microbiol. Spectr. 7, doi: 10.1128/microbiolspec.GPP3-0032-2018 ∗∗pgQ,
Favret, M. E., and Yousten, A. A. (1985). Insecticidal activity of Bacillus laterosporus. J. Invertebr. Pathol. 45, 195–203. doi: 10.1016/0022-2011(85)90009-6
Federici, B. A., Park, H. W., Bideshi, D. K., Wirth, M. C., and Johnson, J. J. (2003). Recombinant bacteria for mosquito control. J Exp Biol. 206, 3877–3885. doi: 10.1242/jeb.00643
Feldmann, F., Dullemans, A., and Waalwijk, C. (1995). Binding of the CryIVD Toxin of Bacillus thuringiensis subsp. israelensis to Larval Dipteran Midgut Proteins. Appl. Environ. Microbiol. 61, 2601–2605. doi: 10.1128/AEM.61.7.2601-2605.1995
Floriano, A. M., Castelli, M., Krenek, S., Berendonk, T. U., Bazzocchi, C., Petroni, G., et al. (2018). The Genome Sequence of “Candidatus Fokinia solitaria”: Insights on Reductive Evolution in Rickettsiales. Genome. Biol. Evol. 10, 1120–1126. doi: 10.1093/gbe/evy072
Galperin, M. Y., Makarova, K. S., Wolf, Y. I., and Koonin, E. V. (2015). Expanded microbial genome coverage and improved protein family annotation in the COG database. Nucleic. Acids Res. 43, D261–D269. doi: 10.1093/nar/gku1223
Goldberg, L., and Margalit, J. (1977). A bacterial spore demonstrating rapid larvicidal activity against Anopheles sergentii, Uranotaenia unguiculata, Culex univitatus, Aedes aegypti and Culex pipiens. Mosq. News 37, 355–358.
Goldman, I. F., Arnold, J., and Carlton, B. C. (1986). Selection for resistance to Bacillus thuringiensis subspecies israelensis in field and laboratory populations of the mosquito Aedes aegypti. J. Invertebr. Pathol. 47, 317–324. doi: 10.1016/0022-2011(86)90102-3
Gossner, C. M., Ducheyne, E., and Schaffner, F. (2018). Increased risk for autochthonous vector-borne infections transmitted by Aedes albopictus in continental Europe. Euro Surveill. 23, 1800268. doi: 10.2807/1560-7917.ES.2018.23.24.1800268
Hurst, M. R. H., Becher, S. A., Young, S. D., Nelson, T. L., and Glare, T. R. (2011). Yersinia entomophaga sp. nov., isolated from the New Zealand grass grub Costelytra zealandica. Int. J. Syst. Evol. Microbiol. 61, 844–849. doi: 10.1099/ijs.0.024406-0
Jagtap, S. C., Jagtap, C. B., Kumar, P., and Srivastava, R. B. (2009). Detection of Bacillus sphaericus mosquitocidal toxin genes by multiplex colony PCR. Can. J. Microbiol. 55, 207–209. doi: 10.1139/w08-113
Klindworth, A., Pruesse, E., Schweer, T., Peplies, J., Quast, C., Horn, M., et al. (2013). Evaluation of general 16S ribosomal RNA gene PCR primers for classical and next-generation sequencing-based diversity studies. Nucleic. Acids Res. 41, e1. doi: 10.1093/nar/gks808
Lacey, L. A. (2007). Bacillus thuringiensis serovariety israelensis and Bacillus sphaericus for mosquito control. J. Am. Mosq. Control. Assoc. 23, 133–163. doi: 10.2987/8756-971x(2007)23[133:btsiab]2.0.co;2
Lacey, L. A., Grzywacz, D., Shapiro-Ilan, D. I., Frutos, R., Brownbridge, M., and Goettel, M. S. (2015). Insect pathogens as biological control agents: Back to the future. J. Invertebr. Pathol. 132, 1–41. doi: 10.1016/j.jip.2015.07.009
Lecadet, M. M., Blondel, M. O., and Ribier, J. (1980). Generalized transduction in Bacillus thuringiensis var. berliner 1715 using bacteriophage CP-54Ber. J. Gen. Microbiol. 121, 203–212. doi: 10.1099/00221287-121-1-203
Marche, M. G., Camiolo, S., Porceddu, A., and Ruiu, L. (2018). Survey of Brevibacillus laterosporus insecticidal protein genes and virulence factors. J. Invertebr. Pathol. 155, 38–43. doi: 10.1016/j.jip.2018.05.002
Marche, M. G., Mura, M. E., Falchi, G., and Ruiu, L. (2017). Spore surface proteins of Brevibacillus laterosporus are involved in insect pathogenesis. Sci. Rep. 7, 43805. doi: 10.1038/srep43805
Marche, M. G., Mura, M. E., and Ruiu, L. (2019). Rapid polymerase chain reaction assays for Brevibacillus laterosporus detection. J. Basic Microbiol. 59, 853–857. doi: 10.1002/jobm.201900188
Markets and markets. (2018). Vector Control Market by Vector Type (Insects and Rodents), End-use Sector (Commercial & Industrial and Residential), Method of Control (Chemical, Physical & Mechanical, and Biological), and Region – Global Forecast to 2023. Available online at: https://www.marketsandmarkets.com/Market-Reports/vector-control-market-265146798.html?gclid=Cj0KCQjwreT8BRDTARIsAJLI0KLDmuNNqvf4KJJzu MUZCM8GoAWAKD8CVWtBtp617UwaPeAwKGJmed4aAsD_EALw_wcB(accessed October 28, 2020).
Martin, P. A., and Travers, R. S. (1989). Worldwide Abundance and Distribution of Bacillus thuringiensis Isolates. Appl. Environ. Microbiol. 55, 2437–2442. doi: 10.1128/AEM.55.10.2437-2442.1989
Martin, P. A. W., Gundersen-Rindal, D., Blackburn, M., and Buyer, J. (2007). Chromobacterium subtsugae sp. nov., a betaproteobacterium toxic to Colorado potato beetle and other insect pests. Int. J. Syst. Evol. Microbiol. 57, 993–999. doi: 10.1099/ijs.0.64611-0
Medema, M. H., Blin, K., Cimermancic, P., de Jager, V., Zakrzewski, P., Fischbach, M. A., et al. (2011). antiSMASH: rapid identification, annotation and analysis of secondary metabolite biosynthesis gene clusters in bacterial and fungal genome sequences. Nucleic. Acids Res. 39, W339–W346. doi: 10.1093/nar/gkr466
Mei, X., Xu, K., Yang, L., Yuan, Z., Mahillon, J., and Hu, X. (2014). The genetic diversity of cereulide biosynthesis gene cluster indicates a composite transposon Tnces in emetic Bacillus weihenstephanensis. BMC Microbiol. 14:149. doi: 10.1186/1471-2180-14-149
Melo-Santos, M. A., Varjal-Melo, J. J., Araújo, A. P., Gomes, T. C., Paiva, M. H., Regis, L. N., et al. (2010). Resistance to the organophosphate temephos: mechanisms, evolution and reversion in an Aedes aegypti laboratory strain from Brazil. Acta Trop 113, 180–189. doi: 10.1016/j.actatropica.2009.10.015
Miljkovic, M., Jovanovic, S., O’Connor, P. M., Mirkovic, N., Jovcic, B., Filipic, B., et al. (2019). Brevibacillus laterosporus strains BGSP7, BGSP9 and BGSP11 isolated from silage produce broad spectrum multi-antimicrobials. PLoS One 14:e0216773. doi: 10.1371/journal.pone.0216773
Myasnik, M., Manasherob, R., Ben-Dov, E., Zaritsky, A., Margalith, Y., and Barak, Z. (2001). Comparative sensitivity to UV-B radiation of two Bacillus thuringiensis subspecies and other Bacillus sp. Curr. Microbiol. 43, 140–143. doi: 10.1007/s002840010276
Nielsen-Leroux, C., Pasquier, F., Charles, J. F., Sinègre, G., Gaven, B., and Pasteur, N. (1997). Resistance to Bacillus sphaericus involves different mechanisms in Culex pipiens (Diptera:Culicidae) larvae. J. Med. Entomol. 34, 321–327. doi: 10.1093/jmedent/34.3.321
Orlova, M. V., Smirnova, T. A., Ganushkina, L. A., Yacubovich, V. Y., and Azizbekyan, R. R. (1998). Insecticidal activity of Bacillus laterosporus. Appl. Environ. Microbiol. 64, 2723–2725. doi: 10.1128/aem.64.7.2723-2725.1998
Palma, L., Muñoz, D., Berry, C., Murillo, J., and Caballero, P. (2014). Bacillus thuringiensis toxins: an overview of their biocidal activity. Toxins (Basel) 6, 3296–3325. doi: 10.3390/toxins6123296
Paris, M., Boyer, S., Bonin, A., Collado, A., David, J. P., and Despres, L. (2010). Genome scan in the mosquito Aedes rusticus: population structure and detection of positive selection after insecticide treatment. Mol. Ecol. 19, 325–337. doi: 10.1111/j.1365-294X.2009.04437.x
Patel, K. D., Bhanshali, F. C., Chaudhary, A. V., and Ingle, S. S. (2013). A new enrichment method for isolation of Bacillus thuringiensis from diverse sample types. Appl Biochem Biotechnol. 170, 58–66. doi: 10.1007/s12010-013-0145-y
Paul, A., Harrington, L. C., Zhang, L., and Scott, J. G. (2005). Insecticide resistance in Culex pipiens from New York. J. Am. Mosq. Control. Assoc. 21, 305–309. doi: 10.2987/8756-971x(2005)21[305:iricpf]2.0.co;2
Paupy, C., Delatte, H., Bagny, L., Corbel, V., and Fontenille, D. (2009). Aedes albopictus, an arbovirus vector: from the darkness to the light. Microbes Infect. 11, 1177–1185. doi: 10.1016/j.micinf.2009.05.005
Pérez, C., Fernandez, L. E., Sun, J., Folch, J. L., Gill, S. S., Soberón, M., et al. (2005). Bacillus thuringiensis subsp. israelensis Cyt1Aa synergizes Cry11Aa toxin by functioning as a membrane-bound receptor. Proc. Natl. Acad. Sci. USA 102, 18303–18308. doi: 10.1073/pnas.0505494102
Priest, F. G., Ebdrup, L., Zahner, V., and Carter, P. E. (1997). Distribution and characterization of mosquitocidal toxin genes in some strains of Bacillus sphaericus. Appl. Environ. Microbiol. 63, 1195–1198. doi: 10.1128/AEM.63.4.1195-1198.1997
R Core Team. (2020). R: A language and environment for statistical computing. Vienna: R Foundation for Statistical Computing.
Rivers, D. B., Vann, C. N., Zimmack, H. L., and Dean, D. H. (1991). Mosquitocidal activity of Bacillus laterosporus. J. Invertebr. Pathol. 58, 444–447. doi: 10.1016/0022-2011(91)90191-r
Rodriguez-R, L., and Konstantinidis, K. (2016). The enveomics collection: a toolbox for specialized analyses of microbial genomes and metagenomes. PeerJ Preprints 4, e1900v1901.
Ruiu, L. (2013). Brevibacillus laterosporus, a Pathogen of Invertebrates and a Broad-Spectrum Antimicrobial Species. Insects 4, 476–492. doi: 10.3390/insects4030476
Ruiu, L., Floris, I., Satta, A., and Ellar, D. (2007). Toxicity of a Brevibacillus laterosporus strain lacking parasporal crystals against Musca domestica and Aedes aegypti. Biol. Control 43, 136–143. doi: 10.1016/j.biocontrol.2007.07.002
Sanahuja, G., Banakar, R., Twyman, R. M., Capell, T., and Christou, P. (2011). Bacillus thuringiensis: a century of research, development and commercial applications. Plant. Biotechnol. J. 9, 283–300. doi: 10.1111/j.1467-7652.2011.00595.x
Santana, M. A., Moccia-V, C. C., and Gillis, A. E. (2008). Bacillus thuringiensis improved isolation methodology from soil samples. J Microbiol Methods. 75, 357–358. doi: 10.1016/j.mimet.2008.06.008
Seemann, T. (2014). Prokka: rapid prokaryotic genome annotation. Bioinformatics 30, 2068–2069. doi: 10.1093/bioinformatics/btu153
Shida, O., Takagi, H., Kadowaki, K., and Komagata, K. (1996). Proposal for two new genera, Brevibacillus gen. nov. and Aneurinibacillus gen. nov. Int. J. Syst. Bacteriol. 46, 939–946. doi: 10.1099/00207713-46-4-939
Singer, S. (1973). Insecticidal activity of recent bacterial isolates and their toxins against mosquito larvae. Nature 244, 110–111. doi: 10.1038/244110a0
Stamatakis, A. (2015). Using RAxML to Infer Phylogenies. Curr. Protoc. Bioinformatics 51, 6.14.1–6.14.14. doi: 10.1002/0471250953.bi0614s51 ∗∗6.14.1-6.14.14,
Su, T., Thieme, J., Ocegueda, C., Ball, M., and Cheng, M. L. (2018). Resistance to Lysinibacillus sphaericus and Other Commonly Used Pesticides in Culex pipiens (Diptera: Culicidae) from Chico. California. J. Med. Entomol. 55, 423–428. doi: 10.1093/jme/tjx235
Su, T., Thieme, J., White, G. S., Lura, T., Mayerle, N., Faraji, A., et al. (2019). High Resistance to Bacillus sphaericus and Susceptibility to Other Common Pesticides in Culex pipiens (Diptera: Culicidae) from Salt Lake City. UT. J. Med. Entomol. 56, 506–513. doi: 10.1093/jme/tjy193
Tangsongcharoen, C., Chomanee, N., Promdonkoy, B., and Boonserm, P. (2015). Lysinibacillus sphaericus binary toxin induces apoptosis in susceptible Culex quinquefasciatus larvae. J. Invertebr. Pathol. 128, 57–63. doi: 10.1016/j.jip.2015.04.008
Thanabalu, T., Berry, C., and Hindley, J. (1993). Cytotoxicity and ADP-ribosylating activity of the mosquitocidal toxin from Bacillus sphaericus SSII-1: possible roles of the 27- and 70-kilodalton peptides. J. Bacteriol. 175, 2314–2320. doi: 10.1128/jb.175.8.2314-2320.1993
Thomas, W. E., and Ellar, D. J. (1983). Mechanism of action of Bacillus thuringiensis var israelensis insecticidal delta-endotoxin. FEBS Lett. 154, 362–368. doi: 10.1016/0014-5793(83)80183-5
Tilquin, M., Paris, M., Reynaud, S., Despres, L., Ravanel, P., Geremia, R. A., et al. (2008). Long lasting persistence of Bacillus thuringiensis Subsp. israelensis (Bti) in mosquito natural habitats. PLoS One 3:e3432. doi: 10.1371/journal.pone.0003432
Travers, R. S., Martin, P. A., and Reichelderfer, C. F. (1987). Selective Process for Efficient Isolation of Soil Bacillus spp. Appl. Environ. Microbiol. 53, 1263–1266. doi: 10.1128/aem.53.6.1263-1266.1987
Ursino, E., Albertini, A. M., Fiorentino, G., Gabrieli, P., Scoffone, V. C., Pellegrini, A., et al. (2020). Bacillus subtilis as a host for mosquitocidal toxins production. Microb. Biotechnol. 13, 1972–1982. doi: 10.1111/1751-7915.13648
Vidal-Quist, J. C., Castañera, P., and González-Cabrera, J. (2009). Simple and rapid method for PCR characterization of large Bacillus thuringiensis strain collections. Curr. Microbiol. 58, 421–425. doi: 10.1007/s00284-008-9328-0
Keywords: Aedes albopictus, Brevibacillus laterosporus, biopesticides, genome sequencing, soil microbiota community
Citation: Barbieri G, Ferrari C, Mamberti S, Gabrieli P, Castelli M, Sassera D, Ursino E, Scoffone VC, Radaelli G, Clementi E, Sacchi L, Ferrari E, Gasperi G and Albertini AM (2021) Identification of a Novel Brevibacillus laterosporus Strain With Insecticidal Activity Against Aedes albopictus Larvae. Front. Microbiol. 12:624014. doi: 10.3389/fmicb.2021.624014
Received: 30 October 2020; Accepted: 19 January 2021;
Published: 17 February 2021.
Edited by:
Jeremy Keith Herren, International Centre of Insect Physiology and Ecology (ICIPE), KenyaReviewed by:
Bo Zhu, Shanghai Jiao Tong University, ChinaMaria Vittoria Mancini, MRC-University of Glasgow Centre For Virus Research (MRC), United Kingdom
Copyright © 2021 Barbieri, Ferrari, Mamberti, Gabrieli, Castelli, Sassera, Ursino, Scoffone, Radaelli, Clementi, Sacchi, Ferrari, Gasperi and Albertini. This is an open-access article distributed under the terms of the Creative Commons Attribution License (CC BY). The use, distribution or reproduction in other forums is permitted, provided the original author(s) and the copyright owner(s) are credited and that the original publication in this journal is cited, in accordance with accepted academic practice. No use, distribution or reproduction is permitted which does not comply with these terms.
*Correspondence: Giulia Barbieri, Z2l1bGlhLmJhcmJpZXJpQHVuaXB2Lml0