- Population Health Program, Tuberculosis Group, Texas Biomedical Research Institute, San Antonio, TX, United States
In the last two decades, multi (MDR), extensively (XDR), extremely (XXDR) and total (TDR) drug-resistant Mycobacterium tuberculosis (M.tb) strains have emerged as a threat to public health worldwide, stressing the need to develop new tuberculosis (TB) prevention and treatment strategies. It is estimated that in the next 35 years, drug-resistant TB will kill around 75 million people and cost the global economy $16.7 trillion. Indeed, the COVID-19 pandemic alone may contribute with the development of 6.3 million new TB cases due to lack of resources and enforced confinement in TB endemic areas. Evolution of drug-resistant M.tb depends on numerous factors, such as bacterial fitness, strain’s genetic background and its capacity to adapt to the surrounding environment, as well as host-specific and environmental factors. Whole-genome transcriptomics and genome-wide association studies in recent years have shed some insights into the complexity of M.tb drug resistance and have provided a better understanding of its underlying molecular mechanisms. In this review, we will discuss M.tb phenotypic and genotypic changes driving resistance, including changes in cell envelope components, as well as recently described intrinsic and extrinsic factors promoting resistance emergence and transmission. We will further explore how drug-resistant M.tb adapts differently than drug-susceptible strains to the lung environment at the cellular level, modulating M.tb–host interactions and disease outcome, and novel next generation sequencing (NGS) strategies to study drug-resistant TB.
Introduction
Tuberculosis (TB) kills one person every 21s, with ∼10 million cases and ∼1.5 million attributed deaths in 2018 (WHO, 2019). Caused by airborne pathogen Mycobacterium tuberculosis (M.tb), TB is the top disease killer worldwide due to a single infectious agent. After inhalation, M.tb reaches the alveolar space and is bathed in alveolar lining fluid (ALF), being in intimate contact with soluble components of the lung mucosa before interacting with the cellular compartment, including alveolar macrophages (AM) and other immune cells (Arcos et al., 2011; Torrelles and Schlesinger, 2017). This M.tb–host interplay during the initial stages of infection will determine TB disease outcome, driving clearance or progression to active (pulmonary or extrapulmonary TB) or latent TB, although the exact bacterial/host determinants and mechanisms that promote a successful infection are still poorly understood. The success of M.tb in establishing infection and evading the host immune system is in part due to its unique and dynamic cell envelope, composed mainly of lipids and carbohydrates, which changes to adapt to the different lung microenvironments in response to local environmental cues, and also protects the pathogen against harsh environments and many antimicrobial drugs due to its low permeability (Garcia-Vilanova et al., 2019; Maitra et al., 2019). And thus, most combined treatments for active TB work disrupting the mycobacterial cell envelope.
Standard treatment for drug-susceptible TB consists of administering four first-line drugs for 6 months (2 months of isoniazid, rifampicin, ethambutol and pyrazinamide, followed by 4 months of isoniazid and rifampicin), with a success rate of 85% (WHO, 2019). However, the worldwide emergence of drug-resistant strains in recent years, especially in TB endemic areas (Ellner, 2008; Lange et al., 2019), poses a global threat and compromises the goal of the End TB Strategy of reducing 90% the TB cases by 2035. Different causes have led to increased drug resistance, including insufficient healthcare infrastructure, prescription of the wrong treatment (either wrong dose or treatment length), poor quality drugs or drug unavailability, poor adherence to therapy, or M.tb re-infection, among others (Zaman, 2010). Indeed, in 2018, approximately half a million people developed drug-resistant TB (DR-TB) and of these, 78% were multidrug-resistant (MDR-TB), and only one in three confirmed cases were enrolled in treatment (WHO, 2019). Different categories of drug-resistant M.tb strains have been defined by the WHO: multi (MDR), extensively (XDR), and extremely (XXDR) drug-resistant TB. MDR-TB, which represent a 3.4% of new TB cases worldwide, are resistant to at least the two first-line drugs isoniazid and rifampicin, and require 9–20 months of costly treatment using second-line drugs, with decreased success rate of 56% when compared to susceptible TB (WHO, 2019). XDR-TB are also resistant to rifampicin and isoniazid, plus fluoroquinolone and at least one of the three injectable second-line drugs (amikacin, kanamycin, or capreomycin), with even a lower treatment success rate of 39% (WHO, 2019). Lastly, XXDR strains are resistant to all first and second-line drugs. More recently, a new category of M.tb strains not yet recognized by the WHO have been identified in Italy, India and Iran (Velayati et al., 2009b; Loewenberg, 2012), and named totally drug-resistant TB (TDR-TB) for being resistant to all tested antibiotics plus some of the ones currently in the discovery pipeline (Loewenberg, 2012; Parida et al., 2015). The emergence of these potentially incurable strains stresses the urgent need to develop new drug regimens and/or alternative anti-TB strategies to combat these superbugs (Lange et al., 2018).
Only a few studies have explored the cell envelope composition of drug-resistant M.tb strains and their metabolic responses and adaptation to the different environmental pressures during the course of pulmonary infection, which makes it challenging to develop new and effective treatments (Velayati et al., 2009b). Due to an altered cell envelope composition of drug-resistant isolates (Velayati et al., 2013), one can hypothesize that drug-susceptible and drug-resistant strains will adapt differently to the lung microenvironments, driving different infection outcomes. In recent years, new developments in next generation sequencing (NGS) technologies have allowed investigators to gain further insight not only in specific genetic determinants of drug resistance but have also shed some light on how particular M.tb strains adapt and interact with the host during infection through genome-wide transcriptomics, which is key in finding novel bacterial determinants that can be targeted in the development of new anti-TB drugs or host-directed therapies (Jeanes and O’Grady, 2016).
In this review, we will discuss different factors driving the emergence of drug-resistant M.tb strains, as well as what is known about the adaptation of MDR strains to the different lung environments encountered during the course of infection, and describe recent NGS strategies that could be used to gain a better understanding on M.tb–host interactions and key determinants involved in MDR evolution.
Origin and Evolution of Drug Resistance
Mycobacterium tuberculosis is a highly specialized human intracellular pathogen with an extremely conserved genome driving a paradigm of a near-perfect host-pathogen relationship (Brites and Gagneux, 2015). Currently, phylogenetic inferences reveal that there are seven global lineages of M.tb strains that have co-evolved with human populations under sympatric and allopatric host-pathogen combinations (Gagneux et al., 2006a; Al-Saeedi and Al-Hajoj, 2017). These M.tb strain lineages differ in their geographic distribution, biological fitness, virulence and their propensity to acquire drug resistance; specifically, lineages 2 to 4 have been associated with greater disease burden and drug resistance compared to ancient lineages, e.g., lineages 1, 5, and 6 (Al-Saeedi and Al-Hajoj, 2017; Nguyen et al., 2018).
The emergence of drug resistance in M.tb can be divided in two main factors and their interactions: extrinsic factors, which are related to social determinants of TB in populations and the quality of TB control and prevention services; and intrinsic factors, accounting for those related to the acquisition of genetic mutations in drug resistance-associated genes (Gygli et al., 2017; Nguyen et al., 2018; Swain et al., 2020). Regarding the intrinsic factors, mutations in genes coding for drug targets or drug activating enzymes are the primary mode of drug resistance, and they arise mainly through single nucleotide polymorphisms (SNPs) and insertion-deletions (indels). Unlike other bacterial pathogens, acquisition of drug resistance through horizontal gene transfer is not consistently reported in M.tb (Namouchi et al., 2012; Dookie et al., 2018). In addition, drug resistance in M.tb is commonly believed to be caused by single-step chromosomal mutations. However, there are now evidences suggesting that, at least for certain anti-TB drugs, acquired drug resistance is the result of a stepwise acquisition and fixation of mutations leading to a gradual increase in resistance, initiated with the acquisition of isoniazid resistance, subsequently followed by rifampicin or ethambutol resistance (Cohen et al., 2015). Indeed, resistance to isoniazid through the KatGS315T mutation is a common pattern that precedes rifampicin resistance and is conserved globally regardless of the M.tb lineage, geographic region and/or time (Manson et al., 2017). DR-TB can occur as primary drug resistance, when a person is directly infected by a drug-resistant M.tb strain, or as secondary or acquired drug resistance, occurring due to the acquisition of resistance-conferring mutations during failed treatment of drug-susceptible TB (Dookie et al., 2018). The latter is likely associated to M.tb metabolic adaptation to the host lung environment.
In addition to resistance caused by target mutations, several distinctive mechanisms of anti-M.tb innate resistance have been also described (Singh et al., 2020). These include: drug accessibility to the target due to the low permeability of the M.tb cell envelope, modification of drugs by M.tb enzymes, existence of M.tb efflux pumps removing out drugs that were able to cross the M.tb cell envelope, M.tb modulation of its gene expression to adapt to the drug’s effects (or to its presence), and a phenotypic drug tolerance linked to a state of slow growth rate and metabolic shut down. In this regard, during M.tb infection certain bacterial subpopulations, known as persisters, can become phenotypically tolerant to antimycobacterial drugs without acquiring genetic mutations (Kester and Fortune, 2014). This reversible phenomenon is usually induced by external stresses such as hypoxia or drug treatment, among others factors (Vilcheze and Jacobs, 2019), and is associated with a nonreplicating status of the bacilli. Several M.tb factors and metabolic traits are linked to M.tb persistence (Keren et al., 2011; Lee et al., 2019), although the evidence suggests that persistence consists of an array of heterogenous physiological states and mechanisms (Hartman et al., 2017). Mechanisms of M.tb persistence are still being elucidated; however, different bacterial metabolic status during early stages of the infection might explain why some M.tb subpopulations can become phenotypically drug-resistant.
Indeed, a novel genetically encoded mechanism that causes reversible drug tolerance was recently described in M.tb (Bellerose et al., 2019; Safi et al., 2019; Vargas and Farhat, 2020). This phase variation phenomenon is caused by transient frameshift mutations in a 5’ homopolymeric region in the glpK gene, which encodes the glycerol-3-kynase required for M.tb glycerol catabolism. This phenomenon has been observed in several clinical isolates which, as a consequence, generate bacterial variants (small smooth colonies) that exhibit a drug-tolerant phenotype easily reversible through the introduction of additional insertions/deletions in the same glpK region. This frameshift mutations are particularly enriched in MDR- and XDR-M.tb strains, and suggest that variation in GlpK might contribute to the development of drug resistance. Thus, limited efficacy of current TB treatments suggests that heterogeneity of both host and mycobacterial physiologies in the different lung compartments during the different stages of the infection can influence the emergence of persisters and ultimately, increased drug resistance (Warner and Mizrahi, 2006; Ben-Kahla and Al-Hajoj, 2016; Boldrin et al., 2020). Novel antimycobacterial drugs that target persisters populations are critical in order to shorten TB treatment and decrease the emergence and transmission of MDR-M.tb strains (Zhang et al., 2012; Mandal et al., 2019). Conversely, mixed clonal infections and/or genetic heterogeneity of M.tb populations with different drug susceptibility profiles within a patient can also lead to disparate responses during treatment, promoting drug resistance (Liu et al., 2015).
Factors Contributing to the Emergence of Drug Resistance
The mechanisms and pathways that result in the emergence and subsequent fixation of M.tb resistant strains and its dynamics are not fully understood. However, evidence suggest an interplay of several mechanisms involved during drug selection pressure, including clonal interference, mutation rates, efflux pumps, compensatory mutations, and epistasis (Al-Saeedi and Al-Hajoj, 2017). Exposure of M.tb to drugs induces a bacterial stress response while exerting a drug selection pressure; thus, only those M.tb strains able to adapt will prevail, initiating a competitive selection process between M.tb clones that may acquire different beneficial mutations to survive (clonal interference). In this regard, M.tb strain lineages can present different mutation rates and different capacity to acquire drug resistance (Ford et al., 2013), where clones of the Beijing/East Asian lineage (lineage 2) are associated to an hypermutability phenotype mediated through polymorphisms in anti-mutator genes (mut) involved in DNA repair systems (Ebrahimi-Rad et al., 2003). Recently, DNA methylation has been proposed as a mechanism for phenotypic plasticity in M.tb, aiding rapid adaptation to changing environmental pressures, with heterogeneous DNA methylation reported in MTBC clinical isolates (Modlin et al., 2020), which could have potential implications in the development of drug resistance. Further, because of the drug-induced stress response of M.tb, M.tb efflux pumps are rapidly upregulated within hours after drug exposure, being responsible of conferring a low-level resistance profile (described in detail below). Interestingly, efflux pump inhibitors reduce the minimum inhibitory concentrations (MICs) of key drugs such as isoniazid, rifampicin, linezolid and some fluoroquinolones (Escribano et al., 2007; Machado et al., 2012). Thus, this efflux pump mediated low-level resistance confers a selective advantage and allows M.tb to survive and replicate under sub-optimal drug concentrations, until further development of classical resistance-associated mutations that confer clinical drug-resistant phenotypes (Fonseca et al., 2015; Dheda et al., 2017).
Although beneficial against anti-TB treatment, drug resistance mutations may also impose a fitness cost to M.tb survival, as they can compromise genes involved in essential mycobacterial cell functions. This subject is a matter of debate aiming to elucidate the mechanisms involved in impaired fitness, transmissibility and virulence of DR-M.tb strains. The concept of reduced virulence of MDR-M.tb strains comes from early experiments in the Guinea pig infection model (Middlebrook and Cohn, 1953). More recently, clinical isolates with common mutations for isoniazid, rifampicin, and streptomycin were associated with low or absent in vivo fitness cost (Comas et al., 2011). Thus, clinical isolates undergo mutations to overcome fitness deficits associated to drug resistance, setting the basis for the compensatory evolutionary role in the spread of DR-TB (Nguyen et al., 2018). There is a wide diversity of resistance-conferring mutations in M.tb; however, during selective pressure, specific mutations in clinical isolates are associated with a high-level of resistance without losing fitness or transmissibility (Casali et al., 2014). Different compensatory mutations were demonstrated for rpoA and rpoC in rifampicin-resistant rpoB mutants (Comas et al., 2011; de Vos et al., 2013), for ahpC in isoniazid-resistant KatG mutants (Sherman et al., 1996), and 16S RNA for aminoglycoside resistance (Shcherbakov et al., 2010). This evidence supports the successful expansion of MDR and XDR-M.tb strains worldwide (Merker et al., 2018; Cohen et al., 2019a).
Epistasis, a form of interaction between genes or mutations that influences a phenotype, is also thought to drive the evolution of DR-TB (Borrell et al., 2013; Koch et al., 2014). In fact, some M.tb compensatory mutations are associated with epistatic interactions. Epistasis is conceptually linked to fitness and is divided into negative and positive epistasis; the latter meaning that the interaction between genes or mutations has a smaller fitness cost compared to the genetic determinants alone. Different positive and negative epistasis exist in M.tb between drug-resistant mutations and between drug resistance-associated mutations and compensatory mutations (Phillips, 2008; Nguyen et al., 2018). In this context, different lineages of M.tb carrying identical rifampicin-associated mutations showed different levels of fitness cost, supporting the idea that epistasis is influenced by M.tb strain’s genetic background (Gagneux et al., 2006b; Borrell and Gagneux, 2011; Li et al., 2017). Epistatic interactions may also determine the order in which drug resistance mutations and compensatory mutations arise, playing a key role in defining the evolutionary processes toward acquisition of MDR-TB (Salverda et al., 2011; Muller et al., 2013).
Adaptation of Drug-Resistant M.tb Strains to the Human Lung Environment
Mycobacterium tuberculosis evolved from a mycobacterial ancestor to effectively infect and persist in the human host (Brites and Gagneux, 2015; Jia et al., 2017). Its higher hydrophobicity compared to other mycobacterial species might have contributed to increased transmission through aerosolization (Jankute et al., 2017). After being inhaled and during the course of infection, M.tb gets in contact with very different host lung microenvironments, which can be extracellular (during the initial stages of infection and before interacting with alveolar phagocytes and other immune cells, after escaping necrotic cells, or after re-activation in cavities); or intracellular (in alveolar phagocytes such as alveolar macrophages (AMs) during primary infection, or within AMs in granulomas during the latency stage) (Figure 1). In these different environments, M.tb has evolved to use host resources to its advantage and adapt its metabolism in order to evade the host immune system, survive, and establish a successful active or latent infection.
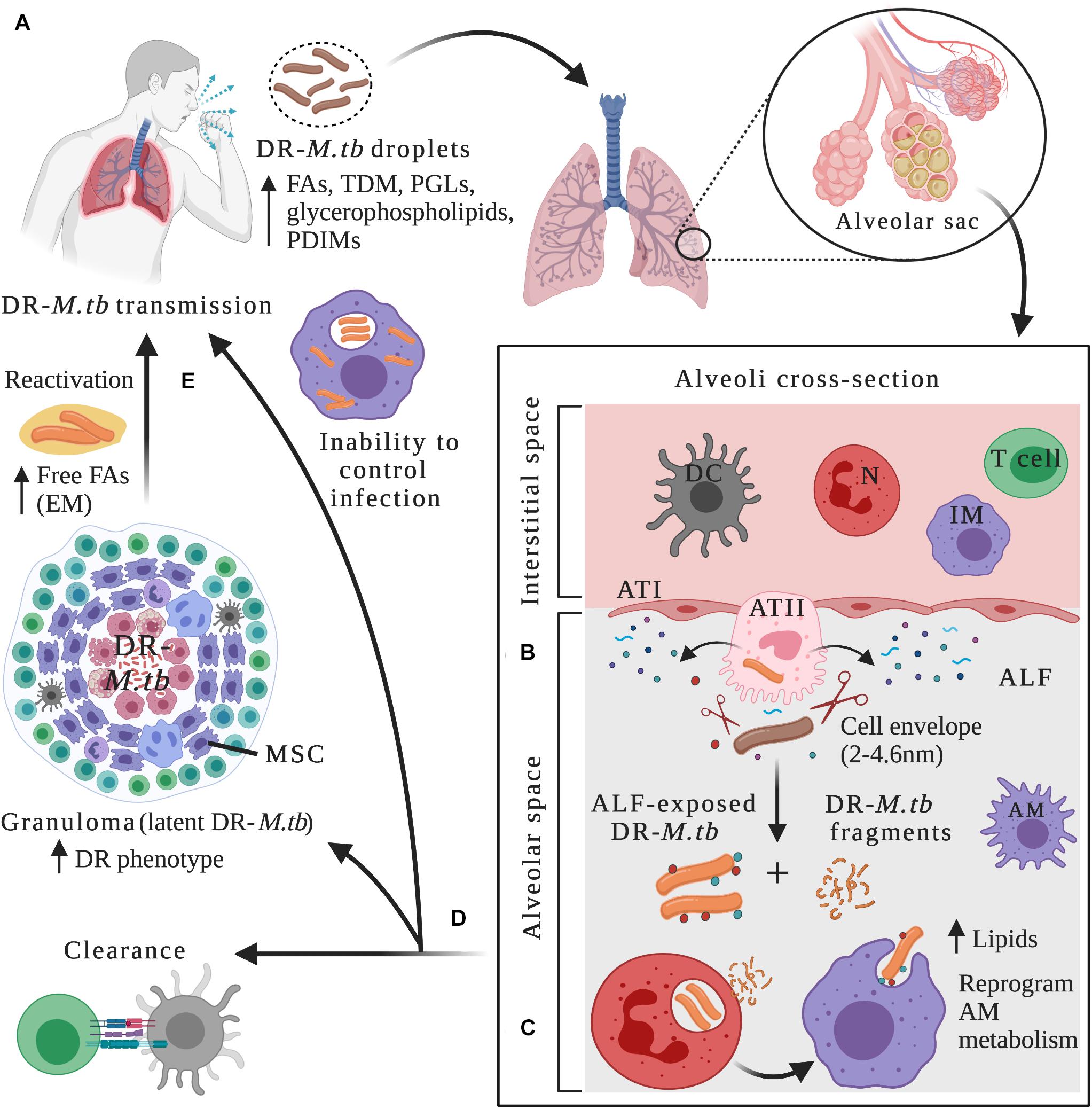
Figure 1. Drug-resistant M.tb–host interactions within lung microenvironments at different stages of the infection. (A) After being in close contact with an individual with active TB disease, infection can be initiated through inhalation of drug-resistant (DR) M.tb-containing droplets. DR-M.tb bacilli contain altered levels of cell envelope lipids such as free fatty acids (FAs), trehalose dimycolate (TDM), Phthiocerol dimycocerosates (PDIMs), phenolic glycolipids (PGLs) and glycerophospholipids, among others. Upon bypassing upper respiratory tract barriers, DR-M.tb will ultimately reach the alveoli, a sac-like structure composed of a thin layer of alveolar epithelial cells type I (ATIs, with structural and gas exchange function) and alveolar epithelial cells type II (ATII, with secretor function) surrounded by capillaries. Alveolar macrophages (AMs) are resident phagocytes that populate the alveolar space, while the interstitial space surrounding the alveoli contains interstitial macrophages (IMs), dendritic cells (DCs), neutrophils (N), and T cells, among other host cells. (B) In the alveolar space, DR-M.tb bacilli first interact with host soluble innate components present in the alveolar lining fluid (ALF), where hydrolases (represented as scissors) can cleave and modify the M.tb cell envelope, releasing cell envelope fragments into the alveolar space. (C) Subsequently, ALF-modified DR-M.tb bacilli will interact with AMs (professional phagocytes) and/or with ATs (non-professional phagocytes), as well as with other host innate immune cells (e.g., neutrophils, DCs). Released DR-M.tb fragments are immunogenic and could attract neutrophils to the infection site driving local oxidative stress and inflammation, which could assist resident resting AMs to clear the infection. (D) The outcome of these initial interactions will resolve in ALF-exposed DR-M.tb clearance, establishment of a successful infection driving primary active TB disease, or a latent M.tb infection defined by M.tb persisters within granulomas, a niche that provide a protective environment against anti-TB drugs, thus increasing the DR-M.tb phenotype. Surrounding mesenchymal stem cells (MSCs) can also dampen immune responses and provide a protective intracellular environment for M.tb persistence. (E) Reactivation and subsequent progression to active TB disease can happen when granulomas fail to contain DR-M.tb, with extracellular DR-M.tb growth that leads to lung tissue destruction and cavity formation. It has been suggested that in this scenario, DR-M.tb secretes free fatty acids, creating some kind of extracellular matrix (EM) that further shields the DR-M.tb against TB drugs. Figure created with BioRender.com.
The M.tb cell envelope provides structural support and protection to osmotic changes, and is composed of four main layers: an inner plasma membrane and periplasmic space, a cell envelope core composed of peptidoglycan (PG) covalently linked to arabinogalactan (AG) and mycolic acids (MAs), the peripheral lipid layer [formed by non-covalently linked lipids and glycolipids such as trehalose dimycolate (TDM), phthiocerol dimycocerosates (PDIMs), mannose-capped lipoarabinomannan (ManLAM), sulfolipids (SLs), phosphatidyl-myo-inositol mannosides (PIMs), and phenolic glycolipids (PGLs), among others], and the outermost layer (also named capsule) (Kalscheuer et al., 2019). Although lipids and carbohydrates constitute ∼80% of the cell wall, proteins are also important components of the M.tb envelope, being studied as potential drug targets (Gu et al., 2003; Mawuenyega et al., 2005; Niederweis et al., 2010; Chiaradia et al., 2017; Hermann et al., 2020). Several described proteins regulate the permeability of the M.tb cell envelope, with important implications in drug resistance. Among many, we find mycobacterial membrane protein Large 3 (MmpL3), Rv3143/Rv1524 axis, and decaprenylphosphoryl-D-ribose 2′-epimerase (DprE), which are involved in the export and synthesis of the M.tb cell wall, regulating its permeability (Dong et al., 2020). Other M.tb cell wall proteins act directly inactivating the drug. This is the case of Rv2170, a putative acetyltransferase that acetylates isoniazid (INH), inducing its breakdown into acetylhydrazine and isonicotinic acid, thus defining a mechanism by which some M.tb strains could bypass INH toxicity (Arun et al., 2020).
Besides providing structural support, the M.tb cell envelope also plays a critical immunomodulatory role in the bacterium-host crosstalk, where several cell envelope outer molecules are known to participate in different stages of the infection with key implications in M.tb pathogenesis and development of drug resistance (Sonawane et al., 2012; Gago et al., 2018; Garcia-Vilanova et al., 2019; Kalscheuer et al., 2019). Indeed, the mycobacterial cell envelope is considered a highly dynamic structure, tightly regulated and remodeled in response to changing host environmental pressures. Consequently, a wide range of cell envelope compositions in different bacterial cells can be found during the course of infection (Kalscheuer et al., 2019). These will determine how M.tb interacts with the host immune system and its potential implications for antibiotic resistance (Dulberger et al., 2020).
However, little is known about the temporal changes of the M.tb cell envelope in response to local environmental cues and how these can lead to different disease outcomes, especially in DR-M.tb strains, since most of the data come from in vitro studies and/or specific stages of infection, not necessarily reflecting the actual temporal dynamics of this complex and heterogeneous structure. M.tb–host interactions and subsequent infection outcome might also be influenced by M.tb metabolic and/or physiologic status before and/or during infection. Below we will discuss recent developments on MDR-M.tb adaptation to the different human lung microenvironments, and how the dynamics of the M.tb cell envelope might determine disease outcome during the M.tb-host interplay.
Initial M.tb–Host Interactions Within the Lung Mucosa
Mycobacterium tuberculosis infection requires close contact with an infectious individual with active TB, occurring through airborne transmission of droplets containing viable bacteria (Figure 1A). Infection in the lungs is initiated when the pathogen is inhaled and bypasses bacterial clearance mechanisms present in the first interaction points that act as barriers, including nose and sinuses, tracheobronchial tree and bronchioles, ultimately reaching the alveoli (Torrelles and Schlesinger, 2017) (Figure 1A). In the alveolar space, M.tb first comes in close contact with soluble elements present in the lung alveolar mucosa, which is composed of surfactant lipids and a hypophase layer or ALF (Figure 1B). ALF contains surfactant proteins, hydrolytic enzymes, and complement proteins, among others, all critical components of the host soluble innate immune response. Indeed, ALF hydrolases are known to interact and modify the cell envelope of M.tb, significantly reducing the amount of two major virulence factors, ManLAM and TDM on the bacterial cell envelope surface (Arcos et al., 2011) (Figure 1B and Table 1). This ALF-driven changes on the M.tb cell envelope are correlated with a significantly better control of M.tb intracellular growth by professional phagocytes in vitro by increasing phagosome-lysosome fusion, as well as long-term effects in vivo (Arcos et al., 2011, 2015; Moliva et al., 2019) (Table 1), suggesting a critical role of these first interactions between M.tb and host innate soluble proteins in determining infection and disease outcome. In fact, M.tb exposure to ALF, besides modifying the M.tb cell envelope, can also drive differential M.tb infection outcome in alveolar epithelial cells (ATs). In this regard, two distinct subsets of human ALFs are defined, the ones that upon exposure drove M.tb to replicate faster (H-ALF), and the ones that upon exposure slowed down M.tb replication (L-ALF) within ATs. This M.tb differential replication within ATs is further correlated with an altered human ALF composition and function, where people with H-ALF could be more susceptible to M.tb infection (Scordo et al., 2019) (Table 1).
Indeed, altered ALF composition and functionality in certain human populations such as the elder, HIV-infected, diabetic and/or individuals with underlying chronic lung diseases might be a contributing factor related to increased susceptibility to M.tb infection (Moliva et al., 2014, 2019; Bell and Noursadeghi, 2018; Segura-Cerda et al., 2019), demonstrating the protective and unique role of ALF in determining M.tb infection control and/or progression to disease. In this context, the elderly population is characterized by a pro-inflammatory lung environment, with increased protein oxidation levels in the ALF linked to decreased soluble innate immune function (Moliva et al., 2014, 2019). Thus, M.tb exposed to ALF obtained from elderly people and subsequently used to infect human macrophages shows altered trafficking (e.g., decreased phagosome-lysosome fusion) and increased M.tb intracellular growth (Moliva et al., 2019). This phenomenon is also observed in vivo, when elderly ALF exposed M.tb infected mice presented significantly higher bacterial burden and tissue damage in their lungs (Moliva et al., 2019). This increase in virulence when M.tb is exposed to elderly ALF is further linked to surfactant protein-D (SP-D) dysfunctionality in the lungs of elderly individuals (Moliva et al., 2019). In this context, polymorphisms in SP-D are linked to TB susceptibility (Azad et al., 2012; Hsieh et al., 2018). SP-D is shown to bind and cluster M.tb bacilli, influencing uptake by macrophages and intracellular killing by increased phagosome-lysosome fusion (Ferguson et al., 1999, 2006). These studies demonstrate the importance of the first M.tb interactions with soluble host innate immune components and the host ALF composition and functional status in dictating infection progression and disease outcome (Ferguson et al., 1999; Torrelles et al., 2008a). Still, the overall contribution of human ALF as an environmental pressure determinant in the development of DR-TB is a relatively unexplored research area. How M.tb changes its metabolism in response to these initial ALF interactions is still largely unknown, as well as which are the specific mycobacterial and host determinants that play a role in establishing a successful infection. Outcomes of M.tb infection after interacting with some ALF components [e.g., complement component 3 (C3), mannose binding lectin (MBL), SP-A, SP-D, etc., are described in detail elsewhere (reviewed in Torrelles et al., 2008a; Torrelles and Schlesinger, 2017; Garcia-Vilanova et al., 2019)].
The M.tb cell envelope surface composition plays a key role in defining the interactions between M.tb and its surroundings, with differences known to exist among M.tb clinical isolates (Torrelles et al., 2008b). Indeed, differential glycosylation patterns observed in membrane- and cell envelope-associated M.tb proteins, lipids and lipoglycans might contribute to virulence and phenotypic variability across M.tb lineages, including drug resistance development (Birhanu et al., 2019). Although little is known about the cell envelope composition of drug-resistant M.tb, some studies observed differences in cell envelope thickness between susceptible and resistant strains (Table 1). A study using electron transmission microscopy (TEM) showed differences of almost 2 nm and up to 4.6 nm in cell envelope thickness between MDR and XDR strains, respectively, when compared to susceptible isolates, with a denser peptidoglycan layer in XDR-M.tb strains (Velayati et al., 2009a) (Figure 1B). Further, XXDR-M.tb strains in exponential phase studied by atomic force microscopy present alterations in cell morphology (increased roughness and striations, with tubular extensions), probably induced by drug treatment; where a subpopulation of XXDR-M.tb bacteria (5–7%) had extraordinarily thick cell envelopes, independent of strain genotype (Velayati et al., 2010). Little information is provided about the drug-resistant strains tested in these studies, and it is still unknown if this thicker cell envelope is a general feature of all DR-M.tb strains; however, these microscopy studies suggest that the M.tb cell envelope composition is altered in these strains (Table 1).
Other studies attempted to analyze differences in lipid profiles between susceptible and DR-M.tb strains, as well as to study the role of surface lipids remodeling contributing to the emergence of MDR-M.tb phenotypes. Using a high-throughput mass spectrometry-based lipidomic approach, it is shown that MDR-M.tb strains have increased levels of free fatty acids, TDM, glycerophospholipids, altered glycerolipids, and unique and distinct lipid signatures when compared to drug-susceptible isolates (Pal et al., 2017) (Figure 1A and Table 1). Indeed, a potential link between low PDIM and PGL levels in the M.tb cell envelope with increased drug susceptibility has been reported using a tesA (encoding a type II thioesterase) mutant strain of Mycobacterium marinum (Chavadi et al., 2011). On the other hand, a recent study used two genetically distinct M.tb clonal pairs (laboratory and clinical drug-sensitive strains and their derived isoniazid-resistant (INHr) mutants) to determine specific in vitro changes related to the isoniazid-resistant phenotype through proteomic and lipidomic analyses (Nieto et al., 2018). These INHr isolates presented 26 proteins with altered levels, which were mainly associated with energy metabolism and respiration, but also with lipid metabolism, virulence, adaptation, and cell envelope remodeling. These strains presented activation of an alternative mycolic acid biosynthesis route, which is also observed in DR-M.tb strains from other lineages (Singh et al., 2015; Nieto et al., 2016b). Interestingly, of the two INHr mutants studied, only the clinical INHr isolate presented low levels of TDM, TMM (trehalose monomycolate) and PDIM, the latter associated with reduced virulence of this strain (Nieto et al., 2016a). This is in contrast with previous studies, indicating that M.tb genetic background may play and important role in determining the remodeling of the M.tb cell envelope protein and lipid constituents after acquisition of drug resistance (Table 1).
How differently DR-M.tb strains interact with the human alveolar environment is still unknown, and it is an active branch of research in our laboratory. In this context, during infection ALF soluble components could modify and shape differently the DR-M.tb cell envelope, as well as different DR-M.tb strains could have different metabolic responses to the alveolar environment, being an unexplored contributing factor in the development of drug resistance and/or persistence within the host (Table 1). Further investigation of a larger number of DR-M.tb isolates with resistance to different drugs is needed to support this hypothesis of a generally thickened and altered cell envelope and to determine interactions with ALF components.
Active TB Disease: Interaction With Professional and Non-professional Phagocytes and Other Immune Cells in the Lung
After susceptible or DR-M.tb are bathed in immune soluble components of the ALF for an undetermined period of time, AMs are thought to be the first host cellular line of defense against M.tb, participating in the pathogen elimination directly or indirectly by activating host innate and adaptive immune responses (Figure 1C). AMs are defined as alternative activated, highly phagocytic cells with tightly regulated balance of pro-and anti-inflammatory responses that avoid the destruction of lung tissue due to excessive inflammation (Evren et al., 2020). M.tb has evolved to exploit AM resources as a strategy for host immune evasion and survival (Guirado et al., 2013).
Pathogen-associated molecular patterns (PAMPs) present in the M.tb cell envelope are recognized by macrophages and other phagocytic cells like lung submucosal and interstitial dendritic cells (DCs) directly or after being ALF-opsonized through an array of phagocytic receptors [e.g., C-type lectin receptors (CLRs), the mannose receptor (MR), Fc and complement receptors (CRs), DC-specific intercellular adhesion molecule-3-Grabbing non-integrin (DC-SIGN)], and signaling receptors [e.g., Toll-like receptors (TLRs)], among others (Leemans et al., 2003; Sasindran and Torrelles, 2011; Ishikawa et al., 2017; Rajaram et al., 2017; Lugo-Villarino et al., 2018) (Figure 1C and Table 1). After recognition and binding by a phagocytic receptor, the internalization of M.tb will initiate a series of trafficking and signaling cascades and the activation of numerous cellular processes such as M.tb-containing phagosome maturation, oxidative and inflammatory responses, antigen processing and presentation, autophagy, and cellular apoptosis, all essential phagocyte killing mechanisms and innate immune responses leading to clearance of M.tb infection (Mortaz et al., 2015) (Figure 1D). Although there are multiple scenarios of interaction with phagocytic cells via different receptors, it is unclear how M.tb uptake through these different phagocytic routes will affect disease progression, or if alternative routes lead to different outcomes.
Nevertheless, M.tb has learned to circumvent host defenses (Kang et al., 2005; Singh et al., 2009; Barczak et al., 2017; Cambier et al., 2017; Liu et al., 2018; Peterson et al., 2019), even to limit its exposure to sub-lethal concentrations of antimicrobial drugs potentially promoting the emergence of drug resistance (Wu et al., 2019). Indeed, it is shown that M.tb cell envelope surface mimics mammalian mannoproteins by having terminal mannose-containing molecules (e.g., ManLAM, higher-order PIMs, 19 kDa and 24 kDa mannoproteins, PstS-1, Apa, etc.). It is suggested that some strains of M.tb use these heavily mannosylated cell surface molecules to interact with lung surfactant proteins such as SP-A (Ragas et al., 2007), and phagocytic receptors including MR (Kang et al., 2005; Torrelles et al., 2006; Torrelles and Schlesinger, 2010; Esparza et al., 2015), Dectin-2 (Decout et al., 2018), and DC-SIGN (Pitarque et al., 2005; Tailleux et al., 2005; Driessen et al., 2009), providing a safe portal of entry for M.tb inside alveolar host cells without driving inflammation and limiting tissue pathology (Ehlers, 2010) (Table 1). In addition, collectins such as mannose-binding lectin (MBL) promote M.tb phagocytosis through bacterial opsonization by binding to ManLAM and PIMs, resulting in establishment of the infection (Denholm et al., 2010; Kalscheuer et al., 2019).
To date, multiple mycobacterial mannoproteins have been identified, with potential roles in virulence, cell invasion, evasion of host defense, and host immunomodulation, reviewed in Mehaffy et al. (2019), Deng et al. (2020), and Tonini et al. (2020). Many of these antigenic glycoproteins contain acyl groups and are defined as lipoglycoproteins (Becker and Sander, 2016). This is the case of the 19 kDa lipoglycoprotein LpqH, which has been shown to have multiple immunomodulatory roles such as: promoting the activation of neutrophils and CD4+ T cells, acting as an adhesin and promoting phagocytosis through lectin receptors, altering macrophage antigen presentation functions, or favoring M.tb immune evasion and dissemination by promoting TLR2-dependent macrophage apoptosis, among others (Parra et al., 2017). Another example is the 24 kDa lipoglycoprotein LprG, which binds and transports PIM and ManLAM to the cell surface of M.tb, acts as an inhibitor of MHC-II antigen processing in human macrophages in vitro (Gehring et al., 2004), although evidence suggests that LprG-reactive T cells are activated through TLR2 and glycosylation of specific MHC-II restriction molecules in vivo (Sieling et al., 2008). LprG is also associated with regulating TAG levels, M.tb growth rate and virulence in combination with the efflux pump Rv1410 (Martinot et al., 2016). Also, the 38 kDa mannosylated glycoprotein PstS-1 acts as adhesin by binding to the macrophage MR, promoting phagocytosis and intracellular survival (Esparza et al., 2015), and glycosylated lipoprotein SodC (Sartain and Belisle, 2009), a superoxide dismutase produced by M.tb, is known to act as a B-cell antigen and contribute to M.tb virulence, with a potential role in the defense against the oxidative burst produced in vivo inside macrophages (Piddington et al., 2001).
Other groups of glycoproteins involved in M.tb–host interactions are the ones belonging to the MCE family, MPT64, or Apa, among others, involved in colonization and invasion of host cells (Sonawane et al., 2012), as well as other proteins such as Rv0227c, HtrA-like serine protease Rv1223, TatA, GlnA1, and the disulfide oxidase DsbA-like enzyme Rv2969c, recently described as mannosylated (Tonini et al., 2020). In addition, GroEL2, a chaperone-like M.tb capsule-associated glycoprotein, is shown to contribute to the suboptimal antigen presentation during mycobacterial infection by modulating macrophage and DC proinflammatory responses (Georgieva et al., 2018), supporting the immunogenic role of M.tb cell envelope-associated glycoproteins during M.tb infection. Other strategies used by M.tb to counteract host defense mechanisms are the blockade of phagolysosome biogenesis and phagosomal acidification, where cell envelope components such as ManLAM and TDM among others, play important roles (Fratti et al., 2003; Vergne et al., 2004; Kang et al., 2005) (Table 1). A recent study also showed that M.tb surface protein Rv1468c binds to ubiquitin, triggering host xenophagy and promoting a controlled persistent intracellular infection while restricting host immune responses (Chai et al., 2019). Increased PIM expression is also reported during in vitro macrophage infection, although its involvement in M.tb virulence and intracellular survival is still unclear (Glass et al., 2017). The presence of DIM/PDIM lipids in the cell envelope is also associated with arrest of phagosomal acidification and macrophage death (Quigley et al., 2017).
Another important group of cell envelope glycoproteins are the ones associated with drug efflux pumps and mycobacterial cell wall permeability, with key implications in the development of drug resistance and M.tb persistence, especially when no genomic mutations are involved [reviewed in Singh et al. (2020)]. A clear example is the MmpL family, a group of inner membrane proteins associated with the transport of cell envelope lipids such as TMM, PDIM, sulfolipids, and TAG, among others (Melly and Purdy, 2019). Several MmpL proteins also act as drug efflux pumps, e.g., overexpression of MmpL5/S5 and MmpL7 increases mycobacterial resistance to clozafimine, bedaquiline, azole drugs, and isoniazid (Rodrigues et al., 2012; Hartkoorn et al., 2014). Indeed, antibiotic stress induces expression of efflux pumps in clinical M.tb strains (Gupta et al., 2010), which can contribute to the emergence of MDR-M.tb phenotypes. This is evidenced by the significantly increased expression of multiple efflux pumps, including Tap, among others, in MDR- and XDR-M.tb isolates when compared to drug-susceptible strains such as H37Rv (Li et al., 2015; Kardan-Yamchi et al., 2019; Liu et al., 2019) (Table 1). Conversely, the outer membrane channel protein CpnT that mediates efficient nutrient uptake during infection, can act as a major drug susceptibility determinant for M.tb, where mutations in cpnT appear to be associated with increased drug resistance in M.tb clinical isolates (Danilchanka et al., 2015). Other transporters involved in drug susceptibility/resistance have been identified in M.tb (da Silva et al., 2011; Singh et al., 2020). Overall, a systematic screening of cell surface-exposed lipids and glycoproteins and their functions is key for a better understanding of the pathogenesis and survival strategies adopted by DR-M.tb strains, including the development of drug-resistance in vivo.
At the end, the balance between macrophage capacity to control intracellular replication and M.tb ability to escape macrophage killing may dictate the outcome of the infection. In this delicate interplay, both macrophage phenotypic and functional heterogeneity, as well as M.tb genotypic and phenotypic differences displayed by the infecting strain will play important roles in disease progression (Guirado et al., 2013). In this regard, a distinct CD11c+ CD11b+ AM subpopulation with a unique inflammatory signature was shown to preferentially harbor M.tb during infection (Lafuse et al., 2019). Conversely, infection with different M.tb genotypes and strains induce different cytokine production and immune responses in in vitro and in vivo models (Lopez et al., 2003; Chacon-Salinas et al., 2005; Sousa et al., 2020). Indeed, there are more than 2,000 genes differentially expressed by macrophages during infection with a lineage 2 MDR-M.tb Beijing strain (Leisching et al., 2016). Thus, the biological properties and the metabolic status of infecting M.tb bacilli, as well as the surface composition of their cell envelope, will determine how M.tb interacts and activates AMs to respond to the infection (Torrelles et al., 2008b; Kalscheuer et al., 2019).
Some evidence indicate that DR-M.tb strains may have a different cell envelope thickness and composition than their counterparts, drug-susceptible strains (Velayati et al., 2009a, 2010; Singh et al., 2015; Nieto et al., 2016b, 2018). These potential differences in their cell envelope composition and thickness might be further accentuated by host ALF hydrolases and other host innate immune soluble components, differently impacting the interaction of these strains with AMs and subsequent infection progression. Indeed, MDR-M.tb isolates are described to present morphological differences in their cell envelope, that correlate with their selective advantage over other circulating M.tb isolates (Silva et al., 2013) (Table 1). Several high-throughput transcriptomic, proteomic and lipidomic approaches focused on the cell wall of rpoB mutant strains, showing upregulation of enzymes involved in the biosynthesis of PDIM (Bisson et al., 2012), decreased synthesis of fatty acids (du Preez and Loots, 2012), and altered levels of sulfoglycolipids and mycobactin (Lahiri et al., 2016). Other studies indicate that the rpoB mutation H526D is associated with altered cell wall physiology and resistance to cell-wall related stresses (Campodonico et al., 2018), and defined rpoB mutations as drivers of altered cytokine and chemokine production in macrophages (Ravan et al., 2019) (Table 1). Recently, MDR W-Beijing M.tb lineage 2 strains with rifampicin-conferring mutations in the rpoB gene were shown to overexpress cell envelope lipids such as PDIMs, bypassing the IL-1 receptor type I (IL-1R1) pathway associated with M.tb control and inducing INF-β that drives less effective aerobic glycolysis, ultimately reprogramming the metabolism of host macrophages (Howard et al., 2018; Howard and Khader, 2020) (Figure 1C and Table 1). Still, global effects of rpoB mutations on M.tb metabolism and cell envelope constitution, and subsequent interactions with host immune cells are still not well characterized, and further studies are needed to determine if the known effects are common to all DR-M.tb strains. Finally, there are some clinical evidences indicating that MDR-M.tb strains could be remodeling host transcriptional programming through epigenetic manipulation (Marimani et al., 2018; Crimi et al., 2020) (Table 1). Indeed, some XDR-M.tb strains seem to promote aberrant epigenetic modifications in macrophages, showing increased methylation levels of inflammatory genes in the TLR2 signaling pathway (Behrouzi et al., 2019).
Neutrophils are also key players in the host immune response against M.tb, being one of the first recruited innate effector cells to arrive at the infection site, found in large numbers in the lung during active TB disease (Eum et al., 2010) (Figure 1C). Upon M.tb contact, neutrophils can activate different intracellular and extracellular killing mechanisms to clear the infection, such as phagocytosis, production and release of reactive oxygen intermediates, and secretion of neutrophil extracellular traps (NETs) and granules containing hydrolytic enzymes and antimicrobial peptides (Kroon et al., 2018). Despite neutrophil’s protective role against M.tb, a regulation of how many neutrophils migrate to the infection site is important. Indeed, increased number of neutrophils in the alveolar space is associated with lung pathology during active TB due to excessive inflammation and tissue destruction, reviewed in detail elsewhere (Dallenga and Schaible, 2016; Muefong and Sutherland, 2020). Furthermore, neutrophil’s activity during M.tb infection is influenced by the human lung mucosa. In this regard, M.tb exposed to ALF enhanced the neutrophil’s innate capacity to recognize and kill M.tb intracellularly by enhancing phagosome-lysosome fusion events and producing higher levels of TNF and IL-8, while limiting excessive extracellular inflammatory responses and tissue damage by reducing oxidative burst, apoptosis, and degranulation (Arcos et al., 2015). Conversely, released M.tb cell envelope fragments after ALF exposure activate neutrophils, leading to an increased local oxidative response and the production of inflammatory cytokines, which regulate the activity of resting macrophages and thus, contribute to the control of M.tb infection (Arcos et al., 2017; Scordo et al., 2017) (Figure 1C). Thus, deciphering the effects of drug resistance in the M.tb–neutrophil interactions is a research area that needs further investigation.
ATs also play important roles in the early recognition and internalization of M.tb in the alveolar compartment (Scordo et al., 2016; Olmo-Fontanez and Torrelles, 2020). Upon M.tb recognition, ATs uptake M.tb and secrete cytokines, antimicrobial peptides, nitric oxide, surfactant proteins and other soluble components into the ALF, facilitating cell-to-cell crosstalk with other alveolar compartment cells such as AMs and neutrophils, and thus ATs are active players initiating the innate immune response (Garcia-Perez et al., 2003; Boggaram et al., 2013; Chuquimia et al., 2013; Reuschl et al., 2017; Gupta et al., 2018) (Figure 1C). Evidence supports that M.tb can replicate within ATs type II cells (ATII), localized inside late endosomal vesicles, and in some instances, ATs can process and present M.tb antigens via MHC class I, which are efficiently recognized by IFN-γ CD8+ T cells (Harriff et al., 2014), defining also ATs as contributors of the adaptive immune response initiation during M.tb infection and TB disease.
Mycobacterium tuberculosis–ATs interactions can occur during: the very first initial stages of primary infection when M.tb reaches the alveolar space or after M.tb is exposed to soluble host factors present in the lung mucosa (Scordo et al., 2019) (Figure 1C), following M.tb release from dying necrotic infected cells in advanced disease stages, and/or following release from granulomas during TB reactivation. The role of ATs might differ based on the stage of infection, although most of the studies are focused on initial M.tb–AT interactions and little is known about the other potential scenarios. Still, it is postulated that invasion of ATIIs could be beneficial for M.tb, as these cells constitute a protective intracellular niche optimal for undetected M.tb growth and replication, in part due to M.tb-containing late endosomes in ATs that fail to acidify, while evading recognition and clearance by professional alveolar phagocytes (Scordo et al., 2016). Indeed, virulence and pathogenesis of M.tb is correlated with their ability to infect ATs (McDonough and Kress, 1995), and differences in the M.tb cell envelope composition, pre-determined by the nature of host ALF, might be modulating M.tb–ATs interactions (Table 1). Supporting this, a M.tb mce1 mutant strain accumulating mycolic acids in its cell envelope can bypass the TLR-2-mediated pro-inflammatory response in ATs (Sequeira et al., 2014). Studies also show that during infection, different M.tb genotypic strains induce different immune responses and gene expression patterns and activated pathways in ATs (Mvubu et al., 2016, 2018). Specifically, XDR-M.tb strains show increased adhesion and invasion of ATs compared to other genotypes, including drug-susceptible virulent M.tb H37Rv and attenuated H37Ra strains, suggesting that successful dissemination of DR-M.tb strains might be related to their interaction with the alveolar epithelium (Ashiru et al., 2010) (Table 1). We are just beginning to understand the role of ATs during M.tb infection and thus, it still remains unclear the differential adaptation of DR-M.tb strains to this alveolar cellular environment and its impact in M.tb infection and TB disease progression.
Differences in MDR-M.tb cell envelope and physiology may lead to different interactions with phagocytic and other immune cells, promoting characteristic host immune responses that might be different than the ones induced by susceptible M.tb strains. Indeed, several studies defined an array of different immune responses correlated to DR-M.tb strains. For example, a case-control study showed that MDR-TB patients had higher levels of CD3 and CD4 cells, as well as IgM, when compared to drug-susceptible TB patients (Sun et al., 2017). Another study showed that MDR-TB patient’s dendritic cells stimulated with MDR-M.tb antigens can mediate a significant higher production of IFN-γ by T cells when compared to DCs from uninfected individuals (Hadizadeh Tasbiti et al., 2018), although this study did not address how this MDR-M.tb induced immune responses compare to drug-susceptible M.tb stimulation. In this regard, another study showed that individuals infected with MDR-M.tb Haarlem strains had higher levels of IL-17+ IFN-γ– CD4+ T cells through an IL-23 and TGF-β-dependent mechanisms when compared to latently TB-infected and uninfected individuals (Basile et al., 2017). Other host immune correlates have been associated with MDR- and XDR-TB patients when compared to both drug-susceptible TB and uninfected individuals: decreased levels of CD4, CD3/HLA-DR+ and Fas+ T cells, and increased levels of NKT and γδ T cells (Kiran et al., 2010); altered CD4/CD8 ratio and higher TNF levels in serum (Song et al., 2018); and low plasma concentrations of human neutrophil peptides (HNP1-3) (Zhu et al., 2011). T-regs might be also contributing to immune dysfunction and M.tb persistence in XDR-TB, although it is not clear whether this response is specific to XDR-TB or if it is actually associated with treatment failure independent of the strain’s drug resistance profile (Davids et al., 2018).
Overall, these data suggest that DR-M.tb strains induce different host immune responses (Table 1), and thus; there is a need of more robust clinical studies in order to establish whether the alteration of the host immune responses is an effect or a cause of DR-TB development.
Latent TB: Granuloma Environment
After M.tb infection, host innate and adaptive immune responses are mounted, resulting in bacterial clearance, active TB disease or establishment of a latent M.tb infection (LTBI), the latter characterized by persistent M.tb bacilli contained inside complex structures called granulomas, a highly heterogeneous and dynamic cellular environment in the lung (Ehlers and Schaible, 2012; Guirado and Schlesinger, 2013) (Figure 1D). Depending of the granuloma stage (formation, maintenance, maturation, disruption leading to M.tb reactivation), these can be studied in vitro (Guirado et al., 2015) and in vivo using animal models such as mouse, Guinea pigs, rabbit and non-human primate models (NHPs) (Bucsan et al., 2019). Granulomas are composed of immune host cells including AMs, interstitial macrophages, foamy macrophages, monocytes, multi-nucleated giant cells, epithelial-like cells, DCs, NK cells, and neutrophils, surrounded by B and T cells, which tightly control M.tb replication and dissemination (Torrelles and Schlesinger, 2017; Muefong and Sutherland, 2020) (Figure 1D). Host determinants such as TNF, IL-6 and complement are important for cellular recruitment and granuloma maintenance (Dutta and Karakousis, 2014). Further, laser microdissection of individual granulomas, followed by confocal microscopy and proteomics, indicated that the granuloma’s center is a pro-inflammatory environment constituted of antimicrobial molecules, ROS and pro-inflammatory eicosanoids, whereas the tissue environment surrounding the granuloma possesses an anti-inflammatory profile (Marakalala et al., 2016).
Indeed, the granuloma environment has been characterized by low pH, hypoxia, oxidative stress, and nutrient starvation, where persisting bacilli are found in a metabolically adapted and non-replicating state (Keren et al., 2011; Eoh et al., 2017; Peterson et al., 2020). Using the in vitro granuloma model, M.tb is known to undergo metabolic changes, including having a preference toward using free fatty acids as energy source, in detriment of using carbohydrates (Guirado et al., 2015) (Table 1). Induction of the DosR regulon is also observed as a contributing factor in response to starvation and adaptation to other environmental stresses, suggesting a relevant role for the DosR regulon on the adaptation and persistence of M.tb within granulomas (Mehra et al., 2015; Zheng et al., 2020). Indeed, a proteome-wide study shows a highly dynamic remodeling of the M.tb proteome during exponential growth, dormancy and resuscitation (Table 1), where the stress-induced DosR regulon contributes 20% to cellular protein content during dormancy (Schubert et al., 2015). Remodeling of the M.tb cell envelope composition during dormancy seems to depend on phosphorylation by serine/threonine protein kinases (STPKs), which modulate lipid biosynthetic enzymes through altered exporter function (Table 1) (described in detail in Stokas et al., 2020).
Several in vitro models of hypoxia/nutrient depletion have also been used to study the cell wall of M.tb during forced dormancy [e.g., using media with no detergent or exogenous lipids, or M.tb cultured in the Hampshire model chemostat under gradual nutrient depletion in an oxygen-controlled environment (Galagan et al., 2013; Bacon et al., 2014)]. When compared to exponentially growing M.tb, dormant M.tb has a thicker cell envelope with increased content of TAGs, free mycolic acids, and lipoglycans, in detriment of having less TDMs, TMMs, and PDIMs, but keeping its infectivity and pathogenesis intact in a guinea pig model (Galagan et al., 2013; Bacon et al., 2014). Conversely, in vitro lipidomic studies using a modified Wayne’s model comparing exponentially grown M.tb in normoxic conditions vs. hypoxia-induced dormant M.tb (Gopinath et al., 2015) pointed a decrease in mycolic acids production and degradation of cell envelope-associated and cytoplasmic lipids during dormancy, which was attributed to a late onset of dormancy achieved in this model compared to previous studies. This lipid depletion was reversed to normal levels after bacterial re-aeration/resuscitation (Raghunandanan et al., 2019). Based on this model, reduction of mycolic acid content during dormancy could explain why M.tb is unable to elicit an appropriate host immune response and clearance during its dormancy state.
Granulomas also provide a protective environment for M.tb against anti-TB drugs, and delivery of drugs inside the core of the granuloma becomes challenging (Grobler et al., 2016). M.tb adaptation to the granuloma environment is also related to the thickening of the M.tb cell envelope (Cunningham and Spreadbury, 1998), where originally drug-susceptible M.tb bacillus become phenotypically drug-resistant (Deb et al., 2009; Rodriguez et al., 2014) or persisters (Figure 1D and Table 1). Further studies also showed M.tb overexpressing several efflux pumps within granulomas, contributing to M.tb drug tolerance during latency (Adams et al., 2011; Smith et al., 2013) (Table 1). In addition, stresses associated to the granuloma microenvironment (inflammation, hypoxia, starvation, among others) are correlated with increased M.tb mutation rates and the generation of drug resistance-conferring mutations in the M.tb genome, which have important implications in the emergence of DR-M.tb phenotypes in vivo. For example, host immune-mediated M.tb DNA damage suggests a potential role of error-prone DNA repair synthesis in the generation of chromosomally encoded drug resistance mutations, and emergence of persister populations might be associated to an altered metabolic state mediated by the (p)ppGpp alarmone system (Warner and Mizrahi, 2006). Actually, a study based on mathematical modeling estimated that the prevalence of latent MDR-TB is increasing worldwide, where one in every 83 individuals with latent TB is harboring an MDR-M.tb strain (Knight et al., 2019). Even though the increased cell envelope thickness observed in DR-M.tb strains resembles the appearance of dormant M.tb bacilli within granulomas, it is still unclear how DR-M.tb strains adapt to the granuloma environment, although transient drug tolerance and permanent drug resistance is associated with the bacterial trehalose-catalytic shift using an M.tb persister-like bacilli (PLB) model (Lee et al., 2019) (Table 1). Further research is needed in this area in order to develop novel anti-DR-TB strategies.
Finally, other type of host cells associated with granulomas are the mesenchymal stem cells. These host cells are reported to dampen the immune response to M.tb infection in tissues surrounding granulomas and thus, can provide a protective intracellular niche for M.tb replication during chronic stages of the infection, sheltering M.tb bacilli from anti-TB drugs and inflammatory cytokines, via increased PGE2 host signaling (Jain et al., 2020) (Figure 1D).
LTBI can persist for decades and thus, it is important to understand the balance between the host cells and M.tb creating this immune-controlled environment, especially in the case of DR-TB. When the host immune control is lost due to a variety of intrinsic (e.g., TNF blockade treatments) and external factors (e.g., malnutrition), which are still poorly understood, granulomas fail to contain M.tb, and latent infection reactivates and progresses to active TB disease (Figure 1E), with the formation of necrotic granulomas, extracellular M.tb growth and tissue destruction resulting in lung cavities and M.tb dissemination and/or escape to the upper airways to propagate the infection elsewhere. Decreased levels of TNF might be associated with this process, as well as T cell exhaustion or impaired functions (Lin et al., 2007; Garcia-Vilanova et al., 2019), although bacterial and other host factors leading to reactivation remain to be elucidated. It is suggested that during reactivation, M.tb increases the production of mycolic acids and other cell envelope components such as PDIM, SL-1, and pentaacyl trehaloses (PATs), increasing cell envelope hydrophobicity and subsequent transmission (Garcia-Vilanova et al., 2019) (Table 1). It is also shown that M.tb can form biofilms in vitro by creating a type of highly hydrophobic extracellular matrix composed of free mycolic acids (Ojha et al., 2008), which might shield the bacterium against host immune responses and high dose of TB drugs in necrotic areas such as cavities in the lungs (Orme, 2011, 2014), allowing bacterial persistence and transmission (Figure 1E and Table 1).
Concluding Remarks
The emergence of DR-TB may be worsened by the current COVID-19 pandemic, exacerbating the global health crisis and undermining TB prevention and control strategies. The development of NGS technologies in the past 20 years has revolutionized our understanding of pathogen–host interactions in the infectious disease field, providing genome-wide information in an increasingly time- and cost-effective manner. Thus, whole-genome sequencing (WGS) has been used to gain better insight of M.tb biology and TB disease, including epidemiologic investigations, strain identification, TB transmission dynamics, pathogen–host interactions, and as a fast point-of-care (POC) diagnostic test (Wlodarska et al., 2015; Dookie et al., 2018; Brown et al., 2019) (Table 2). In this regard, numerous studies demonstrate the value of WGS in predicting DR-M.tb phenotypes from the M.tb genome sequenced directly from clinical specimens (e.g., sputum) (Groschel et al., 2018; Cohen et al., 2019b) (Table 2). Importantly, WGS has provided new insights into the complex underlying molecular mechanisms of M.tb drug resistance thanks to its nucleotide-level resolution (Gonzalez-Escalante et al., 2015; Yu et al., 2015; Coll et al., 2018) (Table 2). Of interest, studies of M.tb clinical isolates collected from the same patient at a single time point (parallel isolates) or at a different time points (serial isolates) during active TB disease progression and/or drug treatment, revealed within-host M.tb genomic diversity and microevolution events (O’Neill et al., 2015; Ley et al., 2019; Vargas et al., 2020). This observation was associated to M.tb drug resistance genes, lipid synthesis and regulation, and host innate immunity regulation. Thus, studying within-host M.tb diversity may assist in identifying novel adaptation strategies to drug and immune pressures in the different lung microenvironments (Nimmo et al., 2020).
Importantly, during infection, DR-M.tb encounters very different human lung microenvironments, modifying its metabolism to adapt and persist in the human host. Evidence suggests that the initial M.tb–host interactions in the alveolar space, such as ALF exposure, play a decisive role in the establishment of M.tb infection. Indeed, ALF-derived changes in the M.tb cell envelope drive interactions with host innate immune cells, modulating the immune response and infection outcome. Although only a few studies have focused on the in vitro and in vivo pathogenesis and adaptation of DR-M.tb strains, a differential host response to DR-M.tb when compared to drug-susceptible strains is reported, which might be due, in part, to an altered M.tb envelope composition leading to M.tb clearance, active TB disease or latent M.tb infection.
In order to develop new strategies to combat the emergence of DR-M.tb clinical isolates, it is imperative to decipher how DR-M.tb navigates through the different lung tissue microenvironments, adapting its metabolic status to persist. Transcriptomic approaches allow us to better understand the mode of action of particular drugs (Table 2), as well as mechanisms of drug tolerance and, in particular, those associated to bacterial metabolic/physiologic changes and to the host immune response, reviewed in detail elsewhere (Liu et al., 2016; Singhania et al., 2018; Briffotaux et al., 2019; Li et al., 2020). In the last decade, dual RNA sequencing has been investigated as an unbiased and global strategy to simultaneously characterize the transcriptional profiling of host and pathogen in infected cells/tissues (Avraham et al., 2016; Westermann et al., 2016, 2017) (Table 2). In this regard, dual RNA-Seq of M. bovis BCG-infected macrophages showed upregulation of mycobacterial cholesterol degradation and iron acquisition pathways, and recycling of mycolic acids, while host cells upregulated de novo cholesterol biosynthesis likely to compensate for the loss of this metabolite due to bacterial catabolism (Rienksma et al., 2015). Further, dual RNA-seq in M.tb infected macrophages isolated from mice show that the growth advantage of M.tb in AMs compared to interstitial macrophages (IMs) is a direct consequence of the metabolic interplay between M.tb and AMs (Pisu et al., 2020). A similar strategy could be applied to study how DR-M.tb strains adapt to the lung environment in comparison to drug-susceptible M.tb strains, and clearly define transcriptional profiles during infection that could be used in DR-TB diagnostics and/or therapy.
Single-cell RNA sequencing (scRNA-seq) has also gained popularity in recent years as a means to study individual transcriptional responses of different host cell populations during altered physiological states (e.g., infection) at an unprecedented level of resolution (Hosokawa et al., 2017; Kolodziejczyk and Lonnberg, 2018; Vegh and Haniffa, 2018; Hashimoto et al., 2019) (Table 2). Thanks to its ability to amplify hundreds to thousands of individual cells in a single preparation, it allows the identification of rare cell populations that otherwise might go undetected in bulk RNA-seq (Tirosh et al., 2016). However, only a few studies have been conducted in the TB field (Gierahn et al., 2017), due to numerous challenges associated with the single-cell isolation and cell wall disruption of M.tb, capturing M.tb non-polyadenylated mRNA, and amplifying the low amount of prokaryotic starting material [reviewed in detail (Chen et al., 2017; Zhang et al., 2018)] (Table 2). Several methods are being developed in order to overcome these microbial scRNA-seq issues (Sheng et al., 2017; Blattman et al., 2020; Imdahl et al., 2020). In this context, scRNA-seq would be critical to study M.tb persisters within granulomas, where a small fraction of the total bacterial population is able to survive drug exposure and killing, likely due to different metabolic/physiological state leading to DR-M.tb.
Paired dual scRNA-seq, which combines the dual transcriptional profiling of host and pathogen in infected tissues with the high resolution of single-cell analysis, represents the next level in the study of complex host–pathogen interactions (reviewed in Penaranda and Hung, 2019) (Table 2). To date, only one study has attempted to simultaneously characterize the transcriptomes of both host and pathogen at a single-cell level in macrophages infected with Salmonella typhimurium (Avital et al., 2017), although it was difficult to extract meaningful biological information due to the low bacterial coverage, plus interpretation of results becomes increasingly complex (Table 2). Although there is still much to accomplish in this area, this methodology will become invaluable to study the adaptive capabilities of M.tb to drug treatments, as well as to study M.tb-ALF and M.tb-host cell responses during infection. We would be able to respond questions such as why during the same infection some M.tb bacterial subpopulations enter drug tolerant states during treatment and others don’t, why some M.tb bacilli but not others are cleared by the same host cell type during infection, and/or what is the spatial-time relationship between M.tb and the host cell defining the infection outcome.
Ultimately, we expect that single-cell multimodal ‘Omics’ approaches will be the future for dissecting the complex biological networks within each cell, providing a systematic and global picture by analyzing and combining multiple modalities such as genomics, transcriptomics, epigenomics, proteomics, lipidomics, and glycomics, among others (Khan et al., 2019; Zhu et al., 2020) (Table 2). Indeed, metabolomics and dual RNA-seq data from M.tb-infected macrophages were collected using a multi-omics approach to identify metabolic sub-networks regulated during early M.tb infection, showing that M.tb consumes up to 33 different host-derived substrates to establish its intracellular niche (Zimmermann et al., 2017).
These novel NGS strategies, including scRNA-seq, dual RNA-seq, and single-cell multi-Omics could provide systematic and global approaches to fully understand the complex DR M.tb-host interplay and dynamics during the different stages of the infection, and to uncover regulatory networks critical for DR-M.tb survival in the host that could potentially be exploited as novel anti-DR-TB strategies.
Author Contributions
AA-G, JIG, and JBT made substantial contributions to the conception, writing, and editing of this review. All authors contributed to the article and approved the submitted version.
Funding
This study was supported by the Ruth Chapman Cowles and Andrew G. Cowles Memorial Trust to JIG, and the Robert J. Kleberg Jr. and Helen C. Kleberg Foundation, and the National Institutes of Health/National Institute of Allergy and Infectious Diseases (NIH/NIAID) Grant Number RO1 AI-146340 to JBT.
Conflict of Interest
The authors declare that the research was conducted in the absence of any commercial or financial relationships that could be construed as a potential conflict of interest.
Acknowledgments
We thank the Texas Biomedical Research Institute family for their efforts to maintain the institute functional during the COVID-19 pandemic.
References
Adams, K. N., Takaki, K., Connolly, L. E., Wiedenhoft, H., Winglee, K., Humbert, O., et al. (2011). Drug tolerance in replicating mycobacteria mediated by a macrophage-induced efflux mechanism. Cell 145, 39–53. doi: 10.1016/j.cell.2011.02.022
Al-Saeedi, M., and Al-Hajoj, S. (2017). Diversity and evolution of drug resistance mechanisms in Mycobacterium tuberculosis. Infect. Drug Resist. 10, 333–342. doi: 10.2147/IDR.S144446
Arcos, J., Diangelo, L. E., Scordo, J. M., Sasindran, S. J., Moliva, J. I., Turner, J., et al. (2015). Lung mucosa lining fluid modification of Mycobacterium tuberculosis to reprogram human neutrophil killing mechanisms. J. Infect. Dis. 212, 948–958. doi: 10.1093/infdis/jiv146
Arcos, J., Sasindran, S. J., Fujiwara, N., Turner, J., Schlesinger, L. S., and Torrelles, J. B. (2011). Human lung hydrolases delineate Mycobacterium tuberculosis-macrophage interactions and the capacity to control infection. J. Immunol. 187, 372–381. doi: 10.4049/jimmunol.1100823
Arcos, J., Sasindran, S. J., Moliva, J. I., Scordo, J. M., Sidiki, S., Guo, H., et al. (2017). Mycobacterium tuberculosis cell wall released fragments by the action of the human lung mucosa modulate macrophages to control infection in an IL-10-dependent manner. Mucosal Immunol. 10, 1248–1258. doi: 10.1038/mi.2016.115
Arun, K. B., Madhavan, A., Abraham, B., Balaji, M., Sivakumar, K. C., Nisha, P., et al. (2020). Acetylation of isoniazid is a novel mechanism of isoniazid resistance in Mycobacterium tuberculosis. Antimicrob. Agents Chemother. 65:e00456-20. doi: 10.1128/AAC.00456-20
Ashiru, O. T., Pillay, M., and Sturm, A. W. (2010). Adhesion to and invasion of pulmonary epithelial cells by the F15/LAM4/KZN and Beijing strains of Mycobacterium tuberculosis. J. Med. Microbiol. 59(Pt 5), 528–533. doi: 10.1099/jmm.0.016006-0
Avital, G., Avraham, R., Fan, A., Hashimshony, T., Hung, D. T., and Yanai, I. (2017). scDual-Seq: mapping the gene regulatory program of Salmonella infection by host and pathogen single-cell RNA-sequencing. Genome Biol. 18:200. doi: 10.1186/s13059-017-1340-x
Avraham, R., Haseley, N., Fan, A., Bloom-Ackermann, Z., Livny, J., and Hung, D. T. (2016). A highly multiplexed and sensitive RNA-seq protocol for simultaneous analysis of host and pathogen transcriptomes. Nat. Protoc. 11, 1477–1491. doi: 10.1038/nprot.2016.090
Azad, A. K., Sadee, W., and Schlesinger, L. S. (2012). Innate immune gene polymorphisms in tuberculosis. Infect. Immun. 80, 3343–3359. doi: 10.1128/IAI.00443-12
Bacon, J., Alderwick, L. J., Allnutt, J. A., Gabasova, E., Watson, R., Hatch, K. A., et al. (2014). Non-replicating Mycobacterium tuberculosis elicits a reduced infectivity profile with corresponding modifications to the cell wall and extracellular matrix. PLoS One 9:e87329. doi: 10.1371/journal.pone.0087329
Barczak, A. K., Avraham, R., Singh, S., Luo, S. S., Zhang, W. R., Bray, M. A., et al. (2017). Systematic, multiparametric analysis of Mycobacterium tuberculosis intracellular infection offers insight into coordinated virulence. PLoS Pathog. 13:e1006363. doi: 10.1371/journal.ppat.1006363
Basile, J. I., Kviatcovsky, D., Romero, M. M., Balboa, L., Monteserin, J., Ritacco, V., et al. (2017). Mycobacterium tuberculosis multi-drug-resistant strain M induces IL-17(+) IFNgamma(-) CD4(+) T cell expansion through an IL-23 and TGF-beta-dependent mechanism in patients with MDR-TB tuberculosis. Clin. Exp. Immunol. 187, 160–173. doi: 10.1111/cei.12873
Becker, K., and Sander, P. (2016). Mycobacterium tuberculosis lipoproteins in virulence and immunity - fighting with a double-edged sword. FEBS Lett. 590, 3800–3819. doi: 10.1002/1873-3468.12273
Behrouzi, A., Hadifar, S., Amanzadeh, A., Riazi Rad, F., Vaziri, F., and Siadat, S. D. (2019). Aberrant methylation of host macrophages induced by tuberculosis infection. World J. Microbiol. Biotechnol. 35:168. doi: 10.1007/s11274-019-2733-7
Bell, L. C. K., and Noursadeghi, M. (2018). Pathogenesis of HIV-1 and Mycobacterium tuberculosis co-infection. Nat. Rev. Microbiol. 16, 80–90. doi: 10.1038/nrmicro.2017.128
Bellerose, M. M., Baek, S. H., Huang, C. C., Moss, C. E., Koh, E. I., Proulx, M. K., et al. (2019). Common variants in the glycerol kinase gene reduce tuberculosis drug efficacy. mBio 10:e663-19. doi: 10.1128/mBio.00663-19
Ben-Kahla, I., and Al-Hajoj, S. (2016). Drug-resistant tuberculosis viewed from bacterial and host genomes. Int. J. Antimicrob. Agents 48, 353–360. doi: 10.1016/j.ijantimicag.2016.07.010
Birhanu, A. G., Yimer, S. A., Kalayou, S., Riaz, T., Zegeye, E. D., Holm-Hansen, C., et al. (2019). Ample glycosylation in membrane and cell envelope proteins may explain the phenotypic diversity and virulence in the Mycobacterium tuberculosis complex. Sci. Rep. 9:2927. doi: 10.1038/s41598-019-39654-9
Bisson, G. P., Mehaffy, C., Broeckling, C., Prenni, J., Rifat, D., Lun, D. S., et al. (2012). Upregulation of the phthiocerol dimycocerosate biosynthetic pathway by rifampin-resistant, rpoB mutant Mycobacterium tuberculosis. J. Bacteriol. 194, 6441–6452. doi: 10.1128/JB.01013-12
Blattman, S. B., Jiang, W., Oikonomou, P., and Tavazoie, S. (2020). Prokaryotic single-cell RNA sequencing by in situ combinatorial indexing. Nat. Microbiol. 5, 1192–1201. doi: 10.1038/s41564-020-0729-6
Boggaram, V., Gottipati, K. R., Wang, X., and Samten, B. (2013). Early secreted antigenic target of 6 kDa (ESAT-6) protein of Mycobacterium tuberculosis induces interleukin-8 (IL-8) expression in lung epithelial cells via protein kinase signaling and reactive oxygen species. J. Biol. Chem. 288, 25500–25511. doi: 10.1074/jbc.M112.448217
Boldrin, F., Provvedi, R., Cioetto Mazzabo, L., Segafreddo, G., and Manganelli, R. (2020). Tolerance and persistence to drugs: a main challenge in the fight against Mycobacterium tuberculosis. Front. Microbiol. 11:1924. doi: 10.3389/fmicb.2020.01924
Borrell, S., and Gagneux, S. (2011). Strain diversity, epistasis and the evolution of drug resistance in Mycobacterium tuberculosis. Clin. Microbiol. Infect 17, 815–820. doi: 10.1111/j.1469-0691.2011.03556.x
Borrell, S., Teo, Y., Giardina, F., Streicher, E. M., Klopper, M., Feldmann, J., et al. (2013). Epistasis between antibiotic resistance mutations drives the evolution of extensively drug-resistant tuberculosis. Evol. Med. Public Health 2013, 65–74. doi: 10.1093/emph/eot003
Briffotaux, J., Liu, S., and Gicquel, B. (2019). Genome-wide transcriptional responses of Mycobacterium to antibiotics. Front. Microbiol. 10:249. doi: 10.3389/fmicb.2019.00249
Brites, D., and Gagneux, S. (2015). Co-evolution of Mycobacterium tuberculosis and Homo sapiens. Immunol. Rev. 264, 6–24. doi: 10.1111/imr.12264
Brown, T. S., Challagundla, L., Baugh, E. H., Omar, S. V., Mustaev, A., Auld, S. C., et al. (2019). Pre-detection history of extensively drug-resistant tuberculosis in KwaZulu-Natal, South Africa. Proc. Natl. Acad. Sci. U.S.A. 116, 23284–23291. doi: 10.1073/pnas.1906636116
Bucsan, A. N., Mehra, S., Khader, S. A., and Kaushal, D. (2019). The current state of animal models and genomic approaches towards identifying and validating molecular determinants of Mycobacterium tuberculosis infection and tuberculosis disease. Pathog. Dis. 77:ftz037. doi: 10.1093/femspd/ftz037
Cambier, C. J., O’Leary, S. M., O’Sullivan, M. P., Keane, J., and Ramakrishnan, L. (2017). Phenolic Glycolipid facilitates mycobacterial escape from microbicidal tissue-resident macrophages. Immunity 47, 552.e554–565.e554. doi: 10.1016/j.immuni.2017.08.003
Campodonico, V. L., Rifat, D., Chuang, Y. M., Ioerger, T. R., and Karakousis, P. C. (2018). Altered Mycobacterium tuberculosis cell wall metabolism and physiology associated with RpoB mutation H526D. Front. Microbiol. 9:494. doi: 10.3389/fmicb.2018.00494
Casali, N., Nikolayevskyy, V., Balabanova, Y., Harris, S. R., Ignatyeva, O., Kontsevaya, I., et al. (2014). Evolution and transmission of drug-resistant tuberculosis in a Russian population. Nat. Genet. 46, 279–286. doi: 10.1038/ng.2878
Chacon-Salinas, R., Serafin-Lopez, J., Ramos-Payan, R., Mendez-Aragon, P., Hernandez-Pando, R., Van Soolingen, D., et al. (2005). Differential pattern of cytokine expression by macrophages infected in vitro with different Mycobacterium tuberculosis genotypes. Clin. Exp. Immunol. 140, 443–449. doi: 10.1111/j.1365-2249.2005.02797.x
Chai, Q., Wang, X., Qiang, L., Zhang, Y., Ge, P., Lu, Z., et al. (2019). A Mycobacterium tuberculosis surface protein recruits ubiquitin to trigger host xenophagy. Nat. Commun. 10:1973. doi: 10.1038/s41467-019-09955-8
Chavadi, S. S., Edupuganti, U. R., Vergnolle, O., Fatima, I., Singh, S. M., Soll, C. E., et al. (2011). Inactivation of tesA reduces cell wall lipid production and increases drug susceptibility in mycobacteria. J. Biol. Chem. 286, 24616–24625. doi: 10.1074/jbc.M111.247601
Chen, Z., Chen, L., and Zhang, W. (2017). Tools for genomic and transcriptomic analysis of microbes at single-cell level. Front. Microbiol. 8:1831. doi: 10.3389/fmicb.2017.01831
Chiaradia, L., Lefebvre, C., Parra, J., Marcoux, J., Burlet-Schiltz, O., Etienne, G., et al. (2017). Dissecting the mycobacterial cell envelope and defining the composition of the native mycomembrane. Sci. Rep. 7:12807. doi: 10.1038/s41598-017-12718-4
Chuquimia, O. D., Petursdottir, D. H., Periolo, N., and Fernandez, C. (2013). Alveolar epithelial cells are critical in protection of the respiratory tract by secretion of factors able to modulate the activity of pulmonary macrophages and directly control bacterial growth. Infect. Immun. 81, 381–389. doi: 10.1128/IAI.00950-12
Cohen, K. A., Abeel, T., Manson McGuire, A., Desjardins, C. A., Munsamy, V., Shea, T. P., et al. (2015). Evolution of extensively drug-resistant tuberculosis over four decades: whole genome sequencing and dating analysis of Mycobacterium tuberculosis isolates from KwaZulu-Natal. PLoS Med. 12:e1001880. doi: 10.1371/journal.pmed.1001880
Cohen, K. A., Manson, A. L., Abeel, T., Desjardins, C. A., Chapman, S. B., Hoffner, S., et al. (2019a). Extensive global movement of multidrug-resistant M. tuberculosis strains revealed by whole-genome analysis. Thorax 74, 882–889. doi: 10.1136/thoraxjnl-2018-211616
Cohen, K. A., Manson, A. L., Desjardins, C. A., Abeel, T., and Earl, A. M. (2019b). Deciphering drug resistance in Mycobacterium tuberculosis using whole-genome sequencing: progress, promise, and challenges. Genome Med. 11:45. doi: 10.1186/s13073-019-0660-8
Coll, F., Phelan, J., Hill-Cawthorne, G. A., Nair, M. B., Mallard, K., Ali, S., et al. (2018). Genome-wide analysis of multi- and extensively drug-resistant Mycobacterium tuberculosis. Nat. Genet. 50, 307–316. doi: 10.1038/s41588-017-0029-0
Comas, I., Borrell, S., Roetzer, A., Rose, G., Malla, B., Kato-Maeda, M., et al. (2011). Whole-genome sequencing of rifampicin-resistant Mycobacterium tuberculosis strains identifies compensatory mutations in RNA polymerase genes. Nat. Genet. 44, 106–110. doi: 10.1038/ng.1038
Crimi, E., Benincasa, G., Cirri, S., Mutesi, R., Faenza, M., and Napoli, C. (2020). Clinical epigenetics and multidrug-resistant bacterial infections: host remodelling in critical illness. Epigenetics 15, 1021–1034. doi: 10.1080/15592294.2020.1748918
Cunningham, A. F., and Spreadbury, C. L. (1998). Mycobacterial stationary phase induced by low oxygen tension: cell wall thickening and localization of the 16-kilodalton alpha-crystallin homolog. J. Bacteriol. 180, 801–808. doi: 10.1128/JB.180.4.801-808.1998
da Silva, P. E. A., Von Groll, A., Martin, A., and Palomino, J. C. (2011). Efflux as a mechanism for drug resistance in Mycobacterium tuberculosis. FEMS Immunol. Med. Microbiol. 63, 1–9. doi: 10.1111/j.1574-695X.2011.00831.x
Dallenga, T., and Schaible, U. E. (2016). Neutrophils in tuberculosis–first line of defence or booster of disease and targets for host-directed therapy? Pathog. Dis. 74:ftw012. doi: 10.1093/femspd/ftw012
Danilchanka, O., Pires, D., Anes, E., and Niederweis, M. (2015). The Mycobacterium tuberculosis outer membrane channel protein CpnT confers susceptibility to toxic molecules. Antimicrob. Agents Chemother. 59, 2328–2336. doi: 10.1128/AAC.04222-14
Davids, M., Pooran, A. S., Pietersen, E., Wainwright, H. C., Binder, A., Warren, R., et al. (2018). Regulatory T cells subvert mycobacterial containment in patients failing extensively drug-resistant tuberculosis treatment. Am. J. Respir. Crit. Care Med. 198, 104–116. doi: 10.1164/rccm.201707-1441OC
de Vos, M., Muller, B., Borrell, S., Black, P. A., van Helden, P. D., Warren, R. M., et al. (2013). Putative compensatory mutations in the rpoC gene of rifampin-resistant Mycobacterium tuberculosis are associated with ongoing transmission. Antimicrob. Agents Chemother. 57, 827–832. doi: 10.1128/AAC.01541-12
Deb, C., Lee, C. M., Dubey, V. S., Daniel, J., Abomoelak, B., Sirakova, T. D., et al. (2009). A novel in vitro multiple-stress dormancy model for Mycobacterium tuberculosis generates a lipid-loaded, drug-tolerant, dormant pathogen. PLoS One 4:e6077. doi: 10.1371/journal.pone.0006077
Decout, A., Silva-Gomes, S., Drocourt, D., Blattes, E., Riviere, M., Prandi, J., et al. (2018). Deciphering the molecular basis of mycobacteria and lipoglycan recognition by the C-type lectin Dectin-2. Sci. Rep. 8:16840. doi: 10.1038/s41598-018-35393-5
Deng, G., Zhang, W., Ji, N., Zhai, Y., Shi, X., Liu, X., et al. (2020). Identification of secreted O-mannosylated proteins from BCG and characterization of immunodominant antigens BCG_0470 and BCG_0980. Front. Microbiol. 11:407. doi: 10.3389/fmicb.2020.00407
Denholm, J. T., McBryde, E. S., and Eisen, D. P. (2010). Mannose-binding lectin and susceptibility to tuberculosis: a meta-analysis. Clin. Exp. Immunol. 162, 84–90. doi: 10.1111/j.1365-2249.2010.04221.x
Dheda, K., Gumbo, T., Maartens, G., Dooley, K. E., McNerney, R., Murray, M., et al. (2017). The epidemiology, pathogenesis, transmission, diagnosis, and management of multidrug-resistant, extensively drug-resistant, and incurable tuberculosis. Lancet Respir Med. doi: 10.1016/S2213-2600(17)30079-6 Online ahead of print
Dong, W., Wang, R., Li, P., Wang, G., Ren, X., Feng, J., et al. (2020). Orphan response regulator Rv3143 increases antibiotic sensitivity by regulating cell wall permeability in Mycobacterium smegmatis. Arch. Biochem. Biophys. 692:108522. doi: 10.1016/j.abb.2020.108522
Dookie, N., Rambaran, S., Padayatchi, N., Mahomed, S., and Naidoo, K. (2018). Evolution of drug resistance in Mycobacterium tuberculosis: a review on the molecular determinants of resistance and implications for personalized care. J. Antimicrob. Chemother. 73, 1138–1151. doi: 10.1093/jac/dkx506
Driessen, N. N., Ummels, R., Maaskant, J. J., Gurcha, S. S., Besra, G. S., Ainge, G. D., et al. (2009). Role of phosphatidylinositol mannosides in the interaction between mycobacteria and DC-SIGN. Infect. Immun. 77, 4538–4547. doi: 10.1128/Iai.01256-08
du Preez, I., and Loots, D. (2012). Altered fatty acid metabolism due to rifampicin-resistance conferring mutations in the rpoB gene of Mycobacterium tuberculosis: mapping the potential of pharmaco-metabolomics for global health and personalized medicine. OMICS 16, 596–603. doi: 10.1089/omi.2012.0028
Dulberger, C. L., Rubin, E. J., and Boutte, C. C. (2020). The mycobacterial cell envelope - a moving target. Nat. Rev. Microbiol. 18, 47–59. doi: 10.1038/s41579-019-0273-7
Dutta, N. K., and Karakousis, P. C. (2014). Latent tuberculosis infection: myths, models, and molecular mechanisms. Microbiol. Mol. Biol. Rev. 78, 343–371. doi: 10.1128/MMBR.00010-14
Ebrahimi-Rad, M., Bifani, P., Martin, C., Kremer, K., Samper, S., Rauzier, J., et al. (2003). Mutations in putative mutator genes of Mycobacterium tuberculosis strains of the W-Beijing family. Emerg. Infect. Dis. 9, 838–845. doi: 10.3201/eid0907.020803
Ehlers, S. (2010). DC-SIGN and mannosylated surface structures of Mycobacterium tuberculosis: a deceptive liaison. Eur. J. Cell Biol. 89, 95–101. doi: 10.1016/j.ejcb.2009.10.004
Ehlers, S., and Schaible, U. E. (2012). The granuloma in tuberculosis: dynamics of a host-pathogen collusion. Front. Immunol. 3:411. doi: 10.3389/fimmu.2012.00411
Ellner, J. J. (2008). The emergence of extensively drug-resistant tuberculosis: a global health crisis requiring new interventions: part I: the origins and nature of the problem. Clin. Transl. Sci. 1, 249–254. doi: 10.1111/j.1752-8062.2008.00060.x
Eoh, H., Wang, Z., Layre, E., Rath, P., Morris, R., Branch Moody, D., et al. (2017). Metabolic anticipation in Mycobacterium tuberculosis. Nat. Microbiol. 2:17084. doi: 10.1038/nmicrobiol.2017.84
Escribano, I., Rodriguez, J. C., Llorca, B., Garcia-Pachon, E., Ruiz, M., and Royo, G. (2007). Importance of the efflux pump systems in the resistance of Mycobacterium tuberculosis to fluoroquinolones and linezolid. Chemotherapy 53, 397–401. doi: 10.1159/000109769
Esparza, M., Palomares, B., Garcia, T., Espinosa, P., Zenteno, E., and Mancilla, R. (2015). PstS-1, the 38-kDa Mycobacterium tuberculosis glycoprotein, is an adhesin, which binds the macrophage mannose receptor and promotes phagocytosis. Scand. J. Immunol. 81, 46–55. doi: 10.1111/sji.12249
Eum, S. Y., Kong, J. H., Hong, M. S., Lee, Y. J., Kim, J. H., Hwang, S. H., et al. (2010). Neutrophils are the predominant infected phagocytic cells in the airways of patients with active pulmonary TB. Chest 137, 122–128. doi: 10.1378/chest.09-0903
Evren, E., Ringqvist, E., and Willinger, T. (2020). Origin and ontogeny of lung macrophages: from mice to humans. Immunology 160, 126–138. doi: 10.1111/imm.13154
Ferguson, J. S., Martin, J. L., Azad, A. K., McCarthy, T. R., Kang, P. B., Voelker, D. R., et al. (2006). Surfactant protein D increases fusion of Mycobacterium tuberculosis-containing phagosomes with lysosomes in human macrophages. Infect. Immun. 74, 7005–7009. doi: 10.1128/IAI.01402-06
Ferguson, J. S., Voelker, D. R., McCormack, F. X., and Schlesinger, L. S. (1999). Surfactant protein D binds to Mycobacterium tuberculosis bacilli and lipoarabinomannan via carbohydrate-lectin interactions resulting in reduced phagocytosis of the bacteria by macrophages. J. Immunol. 163, 312–321.
Fonseca, J. D., Knight, G. M., and McHugh, T. D. (2015). The complex evolution of antibiotic resistance in Mycobacterium tuberculosis. Int. J. Infect. Dis. 32, 94–100. doi: 10.1016/j.ijid.2015.01.014
Ford, C. B., Shah, R. R., Maeda, M. K., Gagneux, S., Murray, M. B., Cohen, T., et al. (2013). Mycobacterium tuberculosis mutation rate estimates from different lineages predict substantial differences in the emergence of drug-resistant tuberculosis. Nat. Genet. 45, 784–790. doi: 10.1038/ng.2656
Fratti, R. A., Chua, J., Vergne, I., and Deretic, V. (2003). Mycobacterium tuberculosis glycosylated phosphatidylinositol causes phagosome maturation arrest. Proc. Natl. Acad. Sci. U.S.A. 100, 5437–5442. doi: 10.1073/pnas.0737613100
Gagneux, S., DeRiemer, K., Van, T., Kato-Maeda, M., de Jong, B. C., Narayanan, S., et al. (2006a). Variable host-pathogen compatibility in Mycobacterium tuberculosis. Proc. Natl. Acad. Sci. U.S.A. 103, 2869–2873. doi: 10.1073/pnas.0511240103
Gagneux, S., Long, C. D., Small, P. M., Van, T., Schoolnik, G. K., and Bohannan, B. J. (2006b). The competitive cost of antibiotic resistance in Mycobacterium tuberculosis. Science 312, 1944–1946. doi: 10.1126/science.1124410
Gago, G., Diacovich, L., and Gramajo, H. (2018). Lipid metabolism and its implication in mycobacteria-host interaction. Curr. Opin. Microbiol. 41, 36–42. doi: 10.1016/j.mib.2017.11.020
Galagan, J. E., Minch, K., Peterson, M., Lyubetskaya, A., Azizi, E., Sweet, L., et al. (2013). The Mycobacterium tuberculosis regulatory network and hypoxia. Nature 499, 178–183. doi: 10.1038/nature12337
Garcia-Perez, B. E., Mondragon-Flores, R., and Luna-Herrera, J. (2003). Internalization of Mycobacterium tuberculosis by macropinocytosis in non-phagocytic cells. Microb. Pathog. 35, 49–55. doi: 10.1016/s0882-4010(03)00089-5
Garcia-Vilanova, A., Chan, J., and Torrelles, J. B. (2019). Underestimated manipulative roles of Mycobacterium tuberculosis cell envelope glycolipids during infection. Front. Immunol. 10:2909. doi: 10.3389/fimmu.2019.02909
Gehring, A. J., Dobos, K. M., Belisle, O. T., Harding, C. V., and Boom, W. H. (2004). Mycobacterium tuberculosis LprG (Rv1411c): a novel TLR-2 ligand that inhibits human macrophage class II MHC antigen processing. J. Immunol. 173, 2660–2668. doi: 10.4049/jimmunol.173.4.2660
Georgieva, M., Sia, J. K., Bizzell, E., Madan-Lala, R., and Rengarajan, J. (2018). Mycobacterium tuberculosis GroEL2 modulates dendritic cell responses. Infect. Immun. 86:e387-17. doi: 10.1128/IAI.00387-17
Gierahn, T. M., Wadsworth, M. H. II, Hughes, T. K., Bryson, B. D., Butler, A., Satija, R., et al. (2017). Seq-Well: portable, low-cost RNA sequencing of single cells at high throughput. Nat. Methods 14, 395–398. doi: 10.1038/nmeth.4179
Glass, L. N., Swapna, G., Chavadi, S. S., Tufariello, J. M., Mi, K., Drumm, J. E., et al. (2017). Mycobacterium tuberculosis universal stress protein Rv2623 interacts with the putative ATP binding cassette (ABC) transporter Rv1747 to regulate mycobacterial growth. PLoS Pathog. 13:e1006515. doi: 10.1371/journal.ppat.1006515
Gonzalez-Escalante, L., Penuelas-Urquides, K., Said-Fernandez, S., Silva-Ramirez, B., and Bermudez de Leon, M. (2015). Differential expression of putative drug resistance genes in Mycobacterium tuberculosis clinical isolates. FEMS Microbiol. Lett. 362:fnv194. doi: 10.1093/femsle/fnv194
Gopinath, V., Raghunandanan, S., Gomez, R. L., Jose, L., Surendran, A., Ramachandran, R., et al. (2015). Profiling the proteome of Mycobacterium tuberculosis during dormancy and reactivation. Mol. Cell. Proteomics 14, 2160–2176. doi: 10.1074/mcp.M115.051151
Grobler, A., Perez Sierra, Z., and Viljoen, H. J. (2016). Modeling nanoparticle delivery of TB drugs to granulomas. J. Theor. Biol. 388, 85–95. doi: 10.1016/j.jtbi.2015.10.004
Groschel, M. I., Walker, T. M., van der Werf, T. S., Lange, C., Niemann, S., and Merker, M. (2018). Pathogen-based precision medicine for drug-resistant tuberculosis. PLoS Pathog. 14:e1007297. doi: 10.1371/journal.ppat.1007297
Gu, S., Chen, J., Dobos, K. M., Bradbury, E. M., Belisle, J. T., and Chen, X. (2003). Comprehensive proteomic profiling of the membrane constituents of a Mycobacterium tuberculosis strain. Mol. Cell. Proteomics 2, 1284–1296. doi: 10.1074/mcp.M300060-MCP200
Guirado, E., Mbawuike, U., Keiser, T. L., Arcos, J., Azad, A. K., Wang, S. H., et al. (2015). Characterization of host and microbial determinants in individuals with latent tuberculosis infection using a human granuloma model. mBio 6:e2537-14. doi: 10.1128/mBio.02537-14
Guirado, E., and Schlesinger, L. S. (2013). Modeling the Mycobacterium tuberculosis granuloma - the critical battlefield in host immunity and disease. Front. Immunol. 4:98. doi: 10.3389/fimmu.2013.00098
Guirado, E., Schlesinger, L. S., and Kaplan, G. (2013). Macrophages in tuberculosis: friend or foe. Semin. Immunopathol. 35, 563–583. doi: 10.1007/s00281-013-0388-2
Gupta, A. K., Katoch, V. M., Chauhan, D. S., Sharma, R., Singh, M., Venkatesan, K., et al. (2010). Microarray analysis of efflux pump genes in multidrug-resistant Mycobacterium tuberculosis during stress induced by common anti-tuberculous drugs. Microb. Drug Resist. 16, 21–28. doi: 10.1089/mdr.2009.0054
Gupta, N., Kumar, R., and Agrawal, B. (2018). New players in immunity to tuberculosis: the host microbiome, lung epithelium, and innate immune cells. Front. Immunol. 9:709. doi: 10.3389/fimmu.2018.00709
Gygli, S. M., Borrell, S., Trauner, A., and Gagneux, S. (2017). Antimicrobial resistance in Mycobacterium tuberculosis: mechanistic and evolutionary perspectives. FEMS Microbiol. Rev. 41, 354–373. doi: 10.1093/femsre/fux011
Hadizadeh Tasbiti, A., Yari, S., Siadat, S. D., Tabarsi, P., Saeedfar, K., and Yari, F. (2018). Cellular immune response in MDR-TB patients to different protein expression of MDR and susceptible Mycobacterium tuberculosis: Rv0147, a novel MDR-TB biomarker. Immunol. Res. 66, 59–66. doi: 10.1007/s12026-017-8971-6
Harriff, M. J., Cansler, M. E., Toren, K. G., Canfield, E. T., Kwak, S., Gold, M. C., et al. (2014). Human lung epithelial cells contain Mycobacterium tuberculosis in a late endosomal vacuole and are efficiently recognized by CD8(+) T cells. PLoS One 9:e97515. doi: 10.1371/journal.pone.0097515
Hartkoorn, R. C., Uplekar, S., and Cole, S. T. (2014). Cross-resistance between clofazimine and bedaquiline through upregulation of MmpL5 in Mycobacterium tuberculosis. Antimicrob. Agents Chemother. 58, 2979–2981. doi: 10.1128/Aac.00037-14
Hartman, T. E., Wang, Z., Jansen, R. S., Gardete, S., and Rhee, K. Y. (2017). Metabolic perspectives on persistence. Microbiol. Spectr. 5:10.1128/microbiolspec.TBTB2-0026-2016. doi: 10.1128/microbiolspec.TBTB2-0026-2016
Hashimoto, K., Kouno, T., Ikawa, T., Hayatsu, N., Miyajima, Y., Yabukami, H., et al. (2019). Single-cell transcriptomics reveals expansion of cytotoxic CD4 T cells in supercentenarians. Proc. Natl. Acad. Sci. U.S.A. 116, 24242–24251. doi: 10.1073/pnas.1907883116
Hermann, C., Karamchand, L., Blackburn, J. M., and Soares, N. C. (2020). Cell envelope proteomics of mycobacteria. J Proteome Res. 20, 94–109. doi: 10.1021/acs.jproteome.0c00650
Hosokawa, M., Nishikawa, Y., Kogawa, M., and Takeyama, H. (2017). Massively parallel whole genome amplification for single-cell sequencing using droplet microfluidics. Sci. Rep. 7:5199. doi: 10.1038/s41598-017-05436-4
Howard, N. C., and Khader, S. A. (2020). Immunometabolism during Mycobacterium tuberculosis Infection. Trends Microbiol. 28, 832–850. doi: 10.1016/j.tim.2020.04.010
Howard, N. C., Marin, N. D., Ahmed, M., Rosa, B. A., Martin, J., Bambouskova, M., et al. (2018). Mycobacterium tuberculosis carrying a rifampicin drug resistance mutation reprograms macrophage metabolism through cell wall lipid changes. Nat. Microbiol. 3, 1099–1108. doi: 10.1038/s41564-018-0245-0
Hsieh, M. H., Ou, C. Y., Hsieh, W. Y., Kao, H. F., Lee, S. W., Wang, J. Y., et al. (2018). Functional analysis of genetic variations in surfactant protein D in mycobacterial infection and their association with tuberculosis. Front. Immunol. 9:1543. doi: 10.3389/fimmu.2018.01543
Imdahl, F., Vafadarnejad, E., Homberger, C., Saliba, A. E., and Vogel, J. (2020). Single-cell RNA-sequencing reports growth-condition-specific global transcriptomes of individual bacteria. Nat. Microbiol. 5, 1202–1206. doi: 10.1038/s41564-020-0774-1
Ishikawa, E., Mori, D., and Yamasaki, S. (2017). Recognition of mycobacterial lipids by immune receptors. Trends Immunol. 38, 66–76. doi: 10.1016/j.it.2016.10.009
Jain, N., Kalam, H., Singh, L., Sharma, V., Kedia, S., Das, P., et al. (2020). Mesenchymal stem cells offer a drug-tolerant and immune-privileged niche to Mycobacterium tuberculosis. Nat. Commun. 11:3062. doi: 10.1038/s41467-020-16877-3
Jankute, M., Nataraj, V., Lee, O. Y., Wu, H. H. T., Ridell, M., Garton, N. J., et al. (2017). The role of hydrophobicity in tuberculosis evolution and pathogenicity. Sci. Rep. 7:1315. doi: 10.1038/s41598-017-01501-0
Jeanes, C., and O’Grady, J. (2016). Diagnosing tuberculosis in the 21st century - Dawn of a genomics revolution? Int. J. Mycobacteriol. 5, 384–391. doi: 10.1016/j.ijmyco.2016.11.028
Jia, X., Yang, L., Dong, M., Chen, S., Lv, L., Cao, D., et al. (2017). The bioinformatics analysis of comparative genomics of Mycobacterium tuberculosis complex (MTBC) provides insight into dissimilarities between intraspecific groups differing in host association, virulence, and epitope diversity. Front. Cell Infect. Microbiol. 7:88. doi: 10.3389/fcimb.2017.00088
Kalscheuer, R., Palacios, A., Anso, I., Cifuente, J., Anguita, J., Jacobs, W. R. Jr., et al. (2019). The Mycobacterium tuberculosis capsule: a cell structure with key implications in pathogenesis. Biochem. J. 476, 1995–2016. doi: 10.1042/BCJ20190324
Kang, P. B., Azad, A. K., Torrelles, J. B., Kaufman, T. M., Beharka, A., Tibesar, E., et al. (2005). The human macrophage mannose receptor directs Mycobacterium tuberculosis lipoarabinomannan-mediated phagosome biogenesis. J. Exp. Med. 202, 987–999. doi: 10.1084/jem.20051239
Kardan-Yamchi, J., Kazemian, H., Haeili, M., Harati, A. A., Amini, S., and Feizabadi, M. M. (2019). Expression analysis of 10 efflux pump genes in multidrug-resistant and extensively drug-resistant Mycobacterium tuberculosis clinical isolates. J. Glob. Antimicrob. Resist. 17, 201–208. doi: 10.1016/j.jgar.2019.01.003
Keren, I., Minami, S., Rubin, E., and Lewis, K. (2011). Characterization and transcriptome analysis of Mycobacterium tuberculosis persisters. mBio 2:e100-11. doi: 10.1128/mBio.00100-11
Kester, J. C., and Fortune, S. M. (2014). Persisters and beyond: mechanisms of phenotypic drug resistance and drug tolerance in bacteria. Crit. Rev. Biochem. Mol. Biol. 49, 91–101. doi: 10.3109/10409238.2013.869543
Khan, M. M., Ernst, O., Manes, N. P., Oyler, B. L., Fraser, I. D. C., Goodlett, D. R., et al. (2019). Multi-omics strategies uncover host-pathogen interactions. ACS Infect. Dis. 5, 493–505. doi: 10.1021/acsinfecdis.9b00080
Kiran, B., Cagatay, T., Clark, P., Kosar, F., Cagatay, P., Yurt, S., et al. (2010). Can immune parameters be used as predictors to distinguish between pulmonary multidrug-resistant and drug-sensitive tuberculosis? Arch. Med. Sci. 6, 77–82. doi: 10.5114/aoms.2010.13511
Knight, G. M., McQuaid, C. F., Dodd, P. J., and Houben, R. (2019). Global burden of latent multidrug-resistant tuberculosis: trends and estimates based on mathematical modelling. Lancet Infect. Dis. 19, 903–912. doi: 10.1016/S1473-3099(19)30307-X
Koch, A., Mizrahi, V., and Warner, D. F. (2014). The impact of drug resistance on Mycobacterium tuberculosis physiology: what can we learn from rifampicin? Emerg. Microbes Infect. 3:e17. doi: 10.1038/emi.2014.17
Kolodziejczyk, A. A., and Lonnberg, T. (2018). Global and targeted approaches to single-cell transcriptome characterization. Brief. Funct. Genomics 17, 209–219. doi: 10.1093/bfgp/elx025
Kroon, E. E., Coussens, A. K., Kinnear, C., Orlova, M., Moller, M., Seeger, A., et al. (2018). Neutrophils: innate effectors of TB resistance? Front. Immunol. 9:2637. doi: 10.3389/fimmu.2018.02637
Lafuse, W. P., Rajaram, M. V. S., Wu, Q., Moliva, J. I., Torrelles, J. B., Turner, J., et al. (2019). Identification of an increased alveolar macrophage subpopulation in old mice that displays unique inflammatory characteristics and is permissive to Mycobacterium tuberculosis infection. J. Immunol. 203, 2252–2264. doi: 10.4049/jimmunol.1900495
Lahiri, N., Shah, R. R., Layre, E., Young, D., Ford, C., Murray, M. B., et al. (2016). Rifampin resistance mutations are associated with broad chemical remodeling of Mycobacterium tuberculosis. J. Biol. Chem. 291, 14248–14256. doi: 10.1074/jbc.M116.716704
Lange, C., Chesov, D., Heyckendorf, J., Leung, C. C., Udwadia, Z., and Dheda, K. (2018). Drug-resistant tuberculosis: an update on disease burden, diagnosis and treatment. Respirology 23, 656–673. doi: 10.1111/resp.13304
Lange, C., Dheda, K., Chesov, D., Mandalakas, A. M., Udwadia, Z., and Horsburgh, C. R. Jr. (2019). Management of drug-resistant tuberculosis. Lancet 394, 953–966. doi: 10.1016/S0140-6736(19)31882-3
Lee, J. J., Lee, S. K., Song, N., Nathan, T. O., Swarts, B. M., Eum, S. Y., et al. (2019). Transient drug-tolerance and permanent drug-resistance rely on the trehalose-catalytic shift in Mycobacterium tuberculosis. Nat. Commun. 10:2928. doi: 10.1038/s41467-019-10975-7
Leemans, J. C., Florquin, S., Heikens, M., Pals, S. T., van der Neut, R., and van der Poll, T. (2003). CD44 is a macrophage binding site for Mycobacterium tuberculosis that mediates macrophage recruitment and protective immunity against tuberculosis. J. Clin. Invest. 111, 681–689. doi: 10.1172/Jci200316936
Leisching, G., Pietersen, R. D., Mpongoshe, V., van Heerden, C., van Helden, P., Wiid, I., et al. (2016). The host response to a clinical MDR mycobacterial strain cultured in a detergent-free environment: a global transcriptomics approach. PLoS One 11:e0153079. doi: 10.1371/journal.pone.0153079
Ley, S. D., de Vos, M., Van Rie, A., and Warren, R. M. (2019). Deciphering within-host microevolution of Mycobacterium tuberculosis through whole-genome sequencing: the phenotypic impact and way forward. Microbiol. Mol. Biol. Rev. 83:e00062-18.
Li, G., Zhang, J., Guo, Q., Jiang, Y., Wei, J., Zhao, L. L., et al. (2015). Efflux pump gene expression in multidrug-resistant Mycobacterium tuberculosis clinical isolates. PLoS One 10:e0119013. doi: 10.1371/journal.pone.0119013
Li, H. C., Guo, H. X., Chen, T., Wang, W., Wu, Z. H., Chen, L., et al. (2020). potential genes related to levofloxacin resistance in Mycobacterium tuberculosis based on transcriptome and methylome overlap analysis. J. Mol. Evol. 88, 202–209. doi: 10.1007/s00239-019-09926-z
Li, Q. J., Jiao, W. W., Yin, Q. Q., Li, Y. J., Li, J. Q., Xu, F., et al. (2017). Positive epistasis of major low-cost drug resistance mutations rpoB531-TTG and katG315-ACC depends on the phylogenetic background of Mycobacterium tuberculosis strains. Int. J. Antimicrob. Agents 49, 757–762. doi: 10.1016/j.ijantimicag.2017.02.009
Lin, P. L., Plessner, H. L., Voitenok, N. N., and Flynn, J. L. (2007). Tumor necrosis factor and tuberculosis. J. Investig. Dermatol. Symp. Proc. 12, 22–25. doi: 10.1038/sj.jidsymp.5650027
Liu, F., Chen, J., Wang, P., Li, H., Zhou, Y., Liu, H., et al. (2018). MicroRNA-27a controls the intracellular survival of Mycobacterium tuberculosis by regulating calcium-associated autophagy. Nat. Commun. 9:4295. doi: 10.1038/s41467-018-06836-4
Liu, J., Shi, W., Zhang, S., Hao, X., Maslov, D. A., Shur, K. V., et al. (2019). Mutations in efflux Pump Rv1258c (Tap) cause resistance to pyrazinamide, isoniazid, and streptomycin in M. tuberculosis. Front. Microbiol. 10:216. doi: 10.3389/fmicb.2019.00216
Liu, Q. Y., Via, L. E., Luo, T., Liang, L. L., Liu, X., Wu, S. F., et al. (2015). Within patient microevolution of Mycobacterium tuberculosis correlates with heterogeneous responses to treatment. Sci. Rep. 5:17507.
Liu, Y. C., Tan, S. M., Huang, L., Abramovitch, R. B., Rohde, K. H., Zimmerman, M. D., et al. (2016). Immune activation of the host cell induces drug tolerance in Mycobacterium tuberculosis both in vitro and in vivo. J. Exp. Med. 213, 809–825. doi: 10.1084/jem.20151248
Loewenberg, S. (2012). India reports cases of totally drug-resistant tuberculosis. Lancet 379:205. doi: 10.1016/s0140-6736(12)60085-3
Lopez, B., Aguilar, D., Orozco, H., Burger, M., Espitia, C., Ritacco, V., et al. (2003). A marked difference in pathogenesis and immune response induced by different Mycobacterium tuberculosis genotypes. Clin. Exp. Immunol. 133, 30–37. doi: 10.1046/j.1365-2249.2003.02171.x
Lugo-Villarino, G., Troegeler, A., Balboa, L., Lastrucci, C., Duval, C., Mercier, I., et al. (2018). The C-type lectin receptor DC-SIGN has an anti-inflammatory role in human M(IL-4) macrophages in response to Mycobacterium tuberculosis. Front. Immunol. 9:1123. doi: 10.3389/fimmu.2018.01123
Machado, D., Couto, I., Perdigao, J., Rodrigues, L., Portugal, I., Baptista, P., et al. (2012). Contribution of efflux to the emergence of isoniazid and multidrug resistance in Mycobacterium tuberculosis. PLoS One 7:e34538. doi: 10.1371/journal.pone.0034538
Maitra, A., Munshi, T., Healy, J., Martin, L. T., Vollmer, W., Keep, N. H., et al. (2019). Cell wall peptidoglycan in Mycobacterium tuberculosis: an Achilles’ heel for the TB-causing pathogen. FEMS Microbiol. Rev. 43, 548–575. doi: 10.1093/femsre/fuz016
Mandal, S., Njikan, S., Kumar, A., Early, J. V., and Parish, T. (2019). The relevance of persisters in tuberculosis drug discovery. Microbiology 165, 492–499. doi: 10.1099/mic.0.000760
Manson, A. L., Cohen, K. A., Abeel, T., Desjardins, C. A., Armstrong, D. T., Barry, C. E., et al. (2017). Genomic analysis of globally diverse Mycobacterium tuberculosis strains provides insights into the emergence and spread of multidrug resistance. Nat. Genet. 49, 395–402. doi: 10.1038/ng.3767
Marakalala, M. J., Raju, R. M., Sharma, K., Zhang, Y. J., Eugenin, E. A., Prideaux, B., et al. (2016). Inflammatory signaling in human tuberculosis granulomas is spatially organized. Nat. Med. 22, 531–538. doi: 10.1038/nm.4073
Marimani, M., Ahmad, A., and Duse, A. (2018). The role of epigenetics, bacterial and host factors in progression of Mycobacterium tuberculosis infection. Tuberculosis 113, 200–214. doi: 10.1016/j.tube.2018.10.009
Martinot, A. J., Farrow, M., Bai, L., Layre, E., Cheng, T. Y., Tsai, J. H., et al. (2016). Mycobacterial metabolic syndrome: LprG and Rv1410 regulate triacylglyceride levels, growth rate and virulence in Mycobacterium tuberculosis. PLoS Pathog. 12:e1005351. doi: 10.1371/journal.ppat.1005351
Mawuenyega, K. G., Forst, C. V., Dobos, K. M., Belisle, J. T., Chen, J., Bradbury, E. M., et al. (2005). Mycobacterium tuberculosis functional network analysis by global subcellular protein profiling. Mol. Biol. Cell 16, 396–404. doi: 10.1091/mbc.e04-04-0329
McDonough, K. A., and Kress, Y. (1995). Cytotoxicity for lung epithelial cells is a virulence-associated phenotype of Mycobacterium tuberculosis. Infect. Immun. 63, 4802–4811. doi: 10.1128/IAI.63.12.4802-4811.1995
Mehaffy, C., Belisle, J. T., and Dobos, K. M. (2019). Mycobacteria and their sweet proteins: an overview of protein glycosylation and lipoglycosylation in M-tuberculosis. Tuberculosis 115, 1–13. doi: 10.1016/j.tube.2019.01.001
Mehra, S., Foreman, T. W., Didier, P. J., Ahsan, M. H., Hudock, T. A., Kissee, R., et al. (2015). The DosR regulon modulates adaptive immunity and is essential for Mycobacterium tuberculosis persistence. Am. J. Respir. Crit. Care Med. 191, 1185–1196. doi: 10.1164/rccm.201408-1502OC
Melly, G., and Purdy, G. E. (2019). MmpL proteins in physiology and pathogenesis of M. tuberculosis. Microorganisms 7:70. doi: 10.3390/microorganisms7030070
Merker, M., Barbier, M., Cox, H., Rasigade, J. P., Feuerriegel, S., Kohl, T. A., et al. (2018). Compensatory evolution drives multidrug-resistant tuberculosis in central Asia. Elife 7:e38200. doi: 10.7554/eLife.38200
Middlebrook, G., and Cohn, M. L. (1953). Some observations on the pathogenicity of isoniazid-resistant variants of tubercle Bacilli. Science 118, 297–299. doi: 10.1126/science.118.3063.297
Modlin, S. J., Conkle-Gutierrez, D., Kim, C., Mitchell, S. N., Morrissey, C., Weinrick, B. C., et al. (2020). Drivers and sites of diversity in the DNA adenine methylomes of 93 Mycobacterium tuberculosis complex clinical isolates. Elife 9:e58542. doi: 10.7554/eLife.58542
Moliva, J. I., Duncan, M. A., Olmo-Fontanez, A., Akhter, A., Arnett, E., Scordo, J. M., et al. (2019). The lung mucosa environment in the elderly increases host susceptibility to Mycobacterium tuberculosis infection. J. Infect. Dis. 220, 514–523. doi: 10.1093/infdis/jiz138
Moliva, J. I., Rajaram, M. V., Sidiki, S., Sasindran, S. J., Guirado, E., Pan, X. J., et al. (2014). Molecular composition of the alveolar lining fluid in the aging lung. Age 36:9633. doi: 10.1007/s11357-014-9633-4
Mortaz, E., Adcock, I. M., Tabarsi, P., Masjedi, M. R., Mansouri, D., Velayati, A. A., et al. (2015). Interaction of pattern recognition receptors with Mycobacterium Tuberculosis. J. Clin. Immunol. 35, 1–10. doi: 10.1007/s10875-014-0103-7
Muefong, C. N., and Sutherland, J. S. (2020). Neutrophils in tuberculosis-associated inflammation and lung pathology. Front. Immunol. 11:962. doi: 10.3389/fimmu.2020.00962
Muller, B., Borrell, S., Rose, G., and Gagneux, S. (2013). The heterogeneous evolution of multidrug-resistant Mycobacterium tuberculosis. Trends Genet. 29, 160–169. doi: 10.1016/j.tig.2012.11.005
Mvubu, N. E., Pillay, B., Gamieldien, J., Bishai, W., and Pillay, M. (2016). Canonical pathways, networks and transcriptional factor regulation by clinical strains of Mycobacterium tuberculosis in pulmonary alveolar epithelial cells. Tuberculosis 97, 73–85. doi: 10.1016/j.tube.2015.12.002
Mvubu, N. E., Pillay, B., McKinnon, L. R., and Pillay, M. (2018). Mycobacterium tuberculosis strains induce strain-specific cytokine and chemokine response in pulmonary epithelial cells. Cytokine 104, 53–64. doi: 10.1016/j.cyto.2017.09.027
Namouchi, A., Didelot, X., Schock, U., Gicquel, B., and Rocha, E. P. (2012). After the bottleneck: genome-wide diversification of the Mycobacterium tuberculosis complex by mutation, recombination, and natural selection. Genome Res. 22, 721–734. doi: 10.1101/gr.129544.111
Nguyen, Q. H., Contamin, L., Nguyen, T. V. A., and Banuls, A. L. (2018). Insights into the processes that drive the evolution of drug resistance in Mycobacterium tuberculosis. Evol. Appl. 11, 1498–1511. doi: 10.1111/eva.12654
Niederweis, M., Danilchanka, O., Huff, J., Hoffmann, C., and Engelhardt, H. (2010). Mycobacterial outer membranes: in search of proteins. Trends Microbiol. 18, 109–116. doi: 10.1016/j.tim.2009.12.005
Nieto, R. L., Mehaffy, C., Creissen, E., Troudt, J., Troy, A., Bielefeldt-Ohmann, H., et al. (2016a). Virulence of Mycobacterium tuberculosis after acquisition of isoniazid resistance: individual nature of katG mutants and the possible role of AhpC. PLoS One 11:e0166807. doi: 10.1371/journal.pone.0166807
Nieto, R. L., Mehaffy, C., and Dobos, K. M. (2016b). Comparing isogenic strains of Beijing genotype Mycobacterium tuberculosis after acquisition of Isoniazid resistance: a proteomics approach. Proteomics 16, 1376–1380. doi: 10.1002/pmic.201500403
Nieto, R. L., Mehaffy, C., Islam, M. N., Fitzgerald, B., Belisle, J., Prenni, J., et al. (2018). Biochemical characterization of isoniazid-resistant Mycobacterium tuberculosis: can the analysis of clonal strains reveal novel targetable pathways? Mol. Cell Proteomics 17, 1685–1701. doi: 10.1074/mcp.RA118.000821
Nimmo, C., Brien, K., Millard, J., Grant, A. D., Padayatchi, N., Pym, A. S., et al. (2020). Dynamics of within-host Mycobacterium tuberculosis diversity and heteroresistance during treatment. EBioMedicine 55:102747. doi: 10.1016/j.ebiom.2020.102747
Ojha, A. K., Baughn, A. D., Sambandan, D., Hsu, T., Trivelli, X., Guerardel, Y., et al. (2008). Growth of Mycobacterium tuberculosis biofilms containing free mycolic acids and harbouring drug-tolerant bacteria. Mol. Microbiol. 69, 164–174. doi: 10.1111/j.1365-2958.2008.06274.x
Olmo-Fontanez, A. M., and Torrelles, J. B. (2020). “Alveolar epithelial cells,” in Advances in Host-Directed Therapies Against Tuberculosis, 1st Edn, eds P. C. Karakousis, R. Hafner, and M. L. Gennaro (Berlin: Springer).
O’Neill, M. B., Mortimer, T. D., and Pepperell, C. S. (2015). Diversity of Mycobacterium tuberculosis across evolutionary scales. PLoS Pathog. 11:e1005257. doi: 10.1371/journal.ppat.1005257
Orme, I. M. (2011). Development of new vaccines and drugs for TB: limitations and potential strategic errors. Future Microbiol. 6, 161–177. doi: 10.2217/fmb.10.168
Orme, I. M. (2014). A new unifying theory of the pathogenesis of tuberculosis. Tuberculosis 94, 8–14. doi: 10.1016/j.tube.2013.07.004
Pal, R., Hameed, S., Kumar, P., Singh, S., and Fatima, Z. (2017). Comparative lipidomics of drug sensitive and resistant Mycobacterium tuberculosis reveals altered lipid imprints. 3 Biotech 7:325. doi: 10.1007/s13205-017-0972-6
Parida, S. K., Axelsson-Robertson, R., Rao, M. V., Singh, N., Master, I., Lutckii, A., et al. (2015). Totally drug-resistant tuberculosis and adjunct therapies. J. Intern. Med. 277, 388–405. doi: 10.1111/joim.12264
Parra, J., Marcoux, J., Poncin, I., Canaan, S., Herrmann, J. L., Nigou, J., et al. (2017). Scrutiny of Mycobacterium tuberculosis 19 kDa antigen proteoforms provides new insights in the lipoglycoprotein biogenesis paradigm. Sci. Rep. 7:43682. doi: 10.1038/srep43682
Penaranda, C., and Hung, D. T. (2019). Single-cell RNA sequencing to understand host-pathogen interactions. ACS Infect Dis. 5, 336–344. doi: 10.1021/acsinfecdis.8b00369
Peterson, E. J., Bailo, R., Rothchild, A. C., Arrieta-Ortiz, M. L., Kaur, A., Pan, M., et al. (2019). Path-seq identifies an essential mycolate remodeling program for mycobacterial host adaptation. Mol. Syst. Biol. 15:e8584. doi: 10.15252/msb.20188584
Peterson, E. J. R., Abidi, A. A., Arrieta-Ortiz, M. L., Aguilar, B., Yurkovich, J. T., Kaur, A., et al. (2020). Intricate genetic programs controlling dormancy in Mycobacterium tuberculosis. Cell Rep. 31:107577. doi: 10.1016/j.celrep.2020.107577
Phillips, P. C. (2008). Epistasis–the essential role of gene interactions in the structure and evolution of genetic systems. Nat. Rev. Genet. 9, 855–867. doi: 10.1038/nrg2452
Piddington, D. L., Fang, F. C., Laessig, T., Cooper, A. M., Orme, I. M., and Buchmeier, N. A. (2001). Cu, Zn superoxide dismutase of Mycobacterium tuberculosis contributes to survival in activated macrophages that are generating an oxidative burst. Infect. Immun. 69, 4980–4987. doi: 10.1128/Iai.69.8.4980-4987.2001
Pisu, D., Huang, L., Grenier, J. K., and Russell, D. G. (2020). Dual RNA-seq of Mtb-infected macrophages in vivo reveals ontologically distinct host-pathogen interactions. Cell Rep. 30, 335.e334–350.e334. doi: 10.1016/j.celrep.2019.12.033
Pitarque, S., Herrmann, J. L., Duteyrat, J. L., Jackson, M., Stewart, G. R., Lecointe, F., et al. (2005). Deciphering the molecular bases of Mycobacterium tuberculosis binding to the lectin DC-SIGN reveals an underestimated complexity. Biochem. J. 392(Pt 3), 615–624. doi: 10.1042/BJ20050709
Quigley, J., Hughitt, V. K., Velikovsky, C. A., Mariuzza, R. A., El-Sayed, N. M., and Briken, V. (2017). The cell wall lipid PDIM contributes to phagosomal escape and host cell exit of Mycobacterium tuberculosis. mBio 8:e148-17. doi: 10.1128/mBio.00148-17
Ragas, A., Roussel, L., Puzo, G., and Riviere, M. (2007). The Mycobacterium tuberculosis cell-surface glycoprotein Apa as a potential adhesin to colonize target cells via the innate immune system pulmonary C-type lectin surfactant protein A. J. Biol. Chem. 282, 5133–5142. doi: 10.1074/jbc.M610183200
Raghunandanan, S., Jose, L., Gopinath, V., and Kumar, R. A. (2019). Comparative label-free lipidomic analysis of Mycobacterium tuberculosis during dormancy and reactivation. Sci. Rep. 9:3660. doi: 10.1038/s41598-019-40051-5
Rajaram, M. V. S., Arnett, E., Azad, A. K., Guirado, E., Ni, B., Gerberick, A. D., et al. (2017). M. tuberculosis-initiated human mannose receptor signaling regulates macrophage recognition and Vesicle trafficking by FcRgamma-Chain, Grb2, and SHP-1. Cell Rep. 21, 126–140. doi: 10.1016/j.celrep.2017.09.034
Ravan, P., Nejad Sattari, T., Siadat, S. D., and Vaziri, F. (2019). Evaluation of the expression of cytokines and chemokines in macrophages in response to rifampin-monoresistant Mycobacterium tuberculosis and H37Rv strain. Cytokine 115, 127–134. doi: 10.1016/j.cyto.2018.12.004
Reuschl, A. K., Edwards, M. R., Parker, R., Connell, D. W., Hoang, L., Halliday, A., et al. (2017). Innate activation of human primary epithelial cells broadens the host response to Mycobacterium tuberculosis in the airways. PLoS Pathog. 13:e1006577. doi: 10.1371/journal.ppat.1006577
Rienksma, R. A., Suarez-Diez, M., Mollenkopf, H. J., Dolganov, G. M., Dorhoi, A., Schoolnik, G. K., et al. (2015). Comprehensive insights into transcriptional adaptation of intracellular mycobacteria by microbe-enriched dual RNA sequencing. BMC Genomics 16:34. doi: 10.1186/s12864-014-1197-2
Rodrigues, L., Machado, D., Couto, I., Amaral, L., and Viveiros, M. (2012). Contribution of efflux activity to isoniazid resistance in the Mycobacterium tuberculosis complex. Infect. Genet. Evol. 12, 695–700. doi: 10.1016/j.meegid.2011.08.009
Rodriguez, J. G., Hernandez, A. C., Helguera-Repetto, C., Aguilar Ayala, D., Guadarrama-Medina, R., Anzola, J. M., et al. (2014). Global adaptation to a lipid environment triggers the dormancy-related phenotype of Mycobacterium tuberculosis. mBio 5:e1125-14. doi: 10.1128/mBio.01125-14
Safi, H., Gopal, P., Lingaraju, S., Ma, S., Levine, C., Dartois, V., et al. (2019). Phase variation in Mycobacterium tuberculosis glpK produces transiently heritable drug tolerance. Proc. Natl. Acad. Sci. U.S.A. 116, 19665–19674. doi: 10.1073/pnas.1907631116
Salverda, M. L., Dellus, E., Gorter, F. A., Debets, A. J., van der Oost, J., Hoekstra, R. F., et al. (2011). Initial mutations direct alternative pathways of protein evolution. PLoS Genet. 7:e1001321. doi: 10.1371/journal.pgen.1001321
Sartain, M. J., and Belisle, J. T. (2009). N-Terminal clustering of the O-glycosylation sites in the Mycobacterium tuberculosis lipoprotein SodC. Glycobiology 19, 38–51. doi: 10.1093/glycob/cwn102
Sasindran, S. J., and Torrelles, J. B. (2011). Mycobacterium Tuberculosis infection and inflammation: what is beneficial for the host and for the bacterium? Front. Microbiol. 2:2. doi: 10.3389/fmicb.2011.00002
Schubert, O. T., Ludwig, C., Kogadeeva, M., Zimmermann, M., Rosenberger, G., Gengenbacher, M., et al. (2015). Absolute proteome composition and dynamics during dormancy and resuscitation of Mycobacterium tuberculosis. Cell Host Microbe 18, 96–108. doi: 10.1016/j.chom.2015.06.001
Scordo, J. M., Arcos, J., Kelley, H. V., Diangelo, L., Sasindran, S. J., Youngmin, E., et al. (2017). Mycobacterium tuberculosis cell wall fragments released upon bacterial contact with the human lung mucosa alter the neutrophil response to infection. Front. Immunol. 8:307. doi: 10.3389/fimmu.2017.00307
Scordo, J. M., Knoell, D. L., and Torrelles, J. B. (2016). Alveolar epithelial cells in Mycobacterium tuberculosis infection: active players or innocent bystanders? J. Innate Immun. 8, 3–14. doi: 10.1159/000439275
Scordo, J. M., Olmo-Fontanez, A. M., Kelley, H. V., Sidiki, S., Arcos, J., Akhter, A., et al. (2019). The human lung mucosa drives differential Mycobacterium tuberculosis infection outcome in the alveolar epithelium. Mucosal Immunol. 12, 795–804. doi: 10.1038/s41385-019-0156-2
Segura-Cerda, C. A., Lopez-Romero, W., and Flores-Valdez, M. A. (2019). Changes in host response to Mycobacterium tuberculosis infection associated with type 2 diabetes: beyond hyperglycemia. Front. Cell Infect. Microbiol. 9:342. doi: 10.3389/fcimb.2019.00342
Sequeira, P. C., Senaratne, R. H., and Riley, L. W. (2014). Inhibition of toll-like receptor 2 (TLR-2)-mediated response in human alveolar epithelial cells by mycolic acids and Mycobacterium tuberculosis mce1 operon mutant. Pathog. Dis. 70, 132–140. doi: 10.1111/2049-632X.12110
Sharma, D., Sharma, S., and Sharma, J. (2020). Potential strategies for the management of drug-resistant tuberculosis. J. Glob. Antimicrob. Resist. 22, 210–214. doi: 10.1016/j.jgar.2020.02.029
Shcherbakov, D., Akbergenov, R., Matt, T., Sander, P., Andersson, D. I., and Bottger, E. C. (2010). Directed mutagenesis of Mycobacterium smegmatis 16S rRNA to reconstruct the in vivo evolution of aminoglycoside resistance in Mycobacterium tuberculosis. Mol. Microbiol. 77, 830–840. doi: 10.1111/j.1365-2958.2010.07218.x
Sheng, K. W., Cao, W. J., Niu, Y. C., Deng, Q., and Zong, C. H. (2017). Effective detection of variation in single-cell transcriptomes using MATQ-seq. Nat. Methods 14, 267–270. doi: 10.1038/Nmeth.4145
Sherman, D. R., Mdluli, K., Hickey, M. J., Arain, T. M., Morris, S. L., Barry, C. E., et al. (1996). Compensatory ahpC gene expression in isoniazid-resistant Mycobacterium tuberculosis. Science 272, 1641–1643. doi: 10.1126/science.272.5268.1641
Sieling, P. A., Hill, P. J., Dobos, K. M., Brookman, K., Kuhlman, A. M., Fabri, M., et al. (2008). Conserved mycobacterial lipoglycoproteins activate TLR2 but also require glycosylation for MHC class II-Restricted T cell activation. J. Immunol. 180, 5833–5842. doi: 10.4049/jimmunol.180.9.5833
Silva, C., Perdigao, J., Alverca, E., de Matos, A. P. A., Carvalho, P. A., Portugal, I., et al. (2013). Exploring the contribution of mycobacteria characteristics in their interaction with human macrophages. Microsc. Microanal. 19, 1159–1169. doi: 10.1017/S1431927613001906
Singh, A., Crossman, D. K., Mai, D., Guidry, L., Voskuil, M. I., Renfrow, M. B., et al. (2009). Mycobacterium tuberculosis WhiB3 maintains redox homeostasis by regulating virulence lipid anabolism to modulate macrophage response. PLoS Pathog. 5:e1000545. doi: 10.1371/journal.ppat.1000545
Singh, A., Gopinath, K., Sharma, P., Bisht, D., Sharma, P., Singh, N., et al. (2015). Comparative proteomic analysis of sequential isolates of Mycobacterium tuberculosis from a patient with pulmonary tuberculosis turning from drug sensitive to multidrug resistant. Indian J. Med. Res. 141, 27–45. doi: 10.4103/0971-5916.154492
Singh, R., Dwivedi, S. P., Gaharwar, U. S., Meena, R., Rajamani, P., and Prasad, T. (2020). Recent updates on drug resistance in Mycobacterium tuberculosis. J. Appl. Microbiol. 128, 1547–1567. doi: 10.1111/jam.14478
Singhania, A., Wilkinson, R. J., Rodrigue, M., Haldar, P., and O’Garra, A. (2018). The value of transcriptomics in advancing knowledge of the immune response and diagnosis in tuberculosis. Nat. Immunol. 19, 1159–1168. doi: 10.1038/s41590-018-0225-9
Smith, T., Wolff, K. A., and Nguyen, L. (2013). Molecular biology of drug resistance in Mycobacterium tuberculosis. Curr. Top. Microbiol. Immunol. 374, 53–80. doi: 10.1007/82_2012_279
Sonawane, A., Mohanty, S., Jagannathan, L., Bekolay, A., and Banerjee, S. (2012). Role of glycans and glycoproteins in disease development by Mycobacterium tuberculosis. Crit. Rev. Microbiol. 38, 250–266. doi: 10.3109/1040841X.2011.653550
Song, T., Li, L. J., Liu, J. L., and Geng, S. J. (2018). Study on the relationship between changes of immune cells and TNF-alpha in peripheral blood of patients with multidrug-resistant and extensively drug-resistant tuberculosis. Eur. Rev. Med. Pharmacol. Sci. 22, 1061–1065. doi: 10.26355/eurrev_201802_14389
Sousa, J., Ca, B., Maceiras, A. R., Simoes-Costa, L., Fonseca, K. L., Fernandes, A. I., et al. (2020). Mycobacterium tuberculosis associated with severe tuberculosis evades cytosolic surveillance systems and modulates IL-1beta production. Nat. Commun. 11:1949. doi: 10.1038/s41467-020-15832-6
Stokas, H., Rhodes, H. L., and Purdy, G. E. (2020). Modulation of the M. tuberculosis cell envelope between replicating and non-replicating persistent bacteria. Tuberculosis 125:102007. doi: 10.1016/j.tube.2020.102007
Sun, E. T., Xia, D., Li, B. H., Ma, J., Dong, Y. Y., Ding, S. S., et al. (2017). Association of immune factors with drug-resistant tuberculosis: a case-control study. Med. Sci. Monit. 23, 5330–5336. doi: 10.12659/msm.904309
Swain, S. S., Sharma, D., Hussain, T., and Pati, S. (2020). Molecular mechanisms of underlying genetic factors and associated mutations for drug resistance in Mycobacterium tuberculosis. Emerg. Microbes Infect. 9, 1651–1663. doi: 10.1080/22221751.2020.1785334
Tailleux, L., Pham-Thi, N., Bergeron-Lafaurie, A., Herrmann, J. L., Charles, P., Schwartz, O., et al. (2005). DC-SIGN induction in alveolar macrophages defines privileged target host cells for mycobacteria in patients with tuberculosis. PLoS Med. 2:e381. doi: 10.1371/journal.pmed.0020381
Tirosh, I., Izar, B., Prakadan, S. M., Wadsworth, M. H. II, Treacy, D., Trombetta, J. J., et al. (2016). Dissecting the multicellular ecosystem of metastatic melanoma by single-cell RNA-seq. Science 352, 189–196. doi: 10.1126/science.aad0501
Tonini, L., Sadet, B., Stella, A., Bouyssie, D., Nigou, J., Burlet-Schiltz, O., et al. (2020). Potential plasticity of the mannoprotein repertoire associated to Mycobacterium tuberculosis virulence unveiled by mass spectrometry-based glycoproteomics. Molecules 25:2348. doi: 10.3390/molecules25102348
Torrelles, J. B., Azad, A. K., Henning, L. N., Carlson, T. K., and Schlesinger, L. S. (2008a). Role of C-type lectins in mycobacterial infections. Curr. Drug Targets 9, 102–112. doi: 10.2174/138945008783502467
Torrelles, J. B., Knaup, R., Kolareth, A., Slepushkina, T., Kaufman, T. M., Kang, P., et al. (2008b). Identification of Mycobacterium tuberculosis clinical isolates with altered phagocytosis by human macrophages due to a truncated lipoarabinomannan. J. Biol. Chem. 283, 31417–31428. doi: 10.1074/jbc.M806350200
Torrelles, J. B., Azad, A. K., and Schlesinger, L. S. (2006). Fine discrimination in the recognition of individual species of phosphatidyl-myo-inositol mannosides from Mycobacterium tuberculosis by C-type lectin pattern recognition receptors. J. Immunol. 177, 1805–1816. doi: 10.4049/jimmunol.177.3.1805
Torrelles, J. B., and Schlesinger, L. S. (2010). Diversity in Mycobacterium tuberculosis mannosylated cell wall determinants impacts adaptation to the host. Tuberculosis 90, 84–93. doi: 10.1016/j.tube.2010.02.003
Torrelles, J. B., and Schlesinger, L. S. (2017). Integrating lung physiology, immunology, and tuberculosis. Trends Microbiol. 25, 688–697. doi: 10.1016/j.tim.2017.03.007
Vargas, R. Jr., and Farhat, M. R. (2020). Antibiotic treatment and selection for glpK mutations in patients with active tuberculosis disease. Proc. Natl. Acad. Sci. U.S.A. 117, 3910–3912. doi: 10.1073/pnas.1920788117
Vargas, R. Jr., Freschi, L., Marin, M., Epperson, L. E., Smith, M., Oussenko, I., et al. (2020). In-host population dynamics of M. tuberculosis during treatment failure. bioRxiv [Preprint] doi: 10.1101/726430
Vegh, P., and Haniffa, M. (2018). The impact of single-cell RNA sequencing on understanding the functional organization of the immune system. Brief. Funct. Genomics 17, 265–272. doi: 10.1093/bfgp/ely003
Velayati, A. A., Farnia, P., Ibrahim, T. A., Haroun, R. Z., Kuan, H. O., Ghanavi, J., et al. (2009a). Differences in cell wall thickness between resistant and nonresistant strains of Mycobacterium tuberculosis: using transmission electron microscopy. Chemotherapy 55, 303–307. doi: 10.1159/000226425
Velayati, A. A., Masjedi, M. R., Farnia, P., Tabarsi, P., Ghanavi, J., ZiaZarifi, A. H., et al. (2009b). Emergence of new forms of totally drug-resistant tuberculosis bacilli super extensively drug-resistant tuberculosis or totally drug-resistant strains in Iran. Chest 136, 420–425. doi: 10.1378/chest.08-2427
Velayati, A. A., Farnia, P., and Masjedi, M. R. (2013). The totally drug resistant tuberculosis (TDR-TB). Int. J. Clin. Exp. Med. 6, 307–309.
Velayati, A. A., Farnia, P., Merza, M. A., Zhavnerko, G. K., Tabarsi, P., Titov, L. P., et al. (2010). New insight into extremely drug-resistant tuberculosis: using atomic force microscopy. Eur. Respir. J. 36, 1490–1493. doi: 10.1183/09031936.00064510
Vergne, I., Fratti, R. A., Hill, P. J., Chua, J., Belisle, J., and Deretic, V. (2004). Mycobacterium tuberculosis phagosome maturation arrest: mycobacterial phosphatidylinositol analog phosphatidylinositol mannoside stimulates early endosomal fusion. Mol. Biol. Cell 15, 751–760. doi: 10.1091/mbc.e03-05-0307
Vilcheze, C., and Jacobs, W. R. Jr. (2019). The isoniazid paradigm of killing, resistance, and persistence in Mycobacterium tuberculosis. J. Mol. Biol. 431, 3450–3461. doi: 10.1016/j.jmb.2019.02.016
Warner, D. F., and Mizrahi, V. (2006). Tuberculosis chemotherapy: the influence of bacillary stress and damage response pathways on drug efficacy. Clin. Microbiol. Rev. 19, 558–570. doi: 10.1128/CMR.00060-05
Westermann, A. J., Barquist, L., and Vogel, J. (2017). Resolving host-pathogen interactions by dual RNA-seq. PLoS Pathog. 13:e1006033. doi: 10.1371/journal.ppat.1006033
Westermann, A. J., Forstner, K. U., Amman, F., Barquist, L., Chao, Y., Schulte, L. N., et al. (2016). Dual RNA-seq unveils noncoding RNA functions in host-pathogen interactions. Nature 529, 496–501. doi: 10.1038/nature16547
Wlodarska, M., Johnston, J. C., Gardy, J. L., and Tang, P. (2015). A microbiological revolution meets an ancient disease: improving the management of tuberculosis with genomics. Clin. Microbiol. Rev. 28, 523–539. doi: 10.1128/CMR.00124-14
Wu, Q., Hossfeld, A., Gerberick, A., Saljoughian, N., Tiwari, C., Mehra, S., et al. (2019). Effect of Mycobacterium tuberculosis enhancement of macrophage P-glycoprotein expression and activity on intracellular survival during antituberculosis drug treatment. J. Infect. Dis. 220, 1989–1998. doi: 10.1093/infdis/jiz405
Yu, G. H., Cui, Z. L., Sun, X., Peng, J. F., Jiang, J., Wu, W., et al. (2015). Gene expression analysis of two extensively drug-resistant tuberculosis isolates show that two-component response systems enhance drug resistance. Tuberculosis 95, 303–314. doi: 10.1016/j.tube.2015.03.008
Zaman, K. (2010). Tuberculosis: a global health problem. J. Health Popul. Nutr. 28, 111–113. doi: 10.3329/jhpn.v28i2.4879
Zhang, Y., Gao, J., Huang, Y., and Wang, J. (2018). Recent developments in single-Cell RNA-Seq of microorganisms. Biophys. J. 115, 173–180. doi: 10.1016/j.bpj.2018.06.008
Zhang, Y., Yew, W. W., and Barer, M. R. (2012). Targeting persisters for tuberculosis control. Antimicrob. Agents Chemother. 56, 2223–2230. doi: 10.1128/AAC.06288-11
Zheng, H., Williams, J. T., Aleiwi, B., Ellsworth, E., and Abramovitch, R. B. (2020). Inhibiting Mycobacterium tuberculosis DosRST signaling by targeting response regulator DNA binding and sensor kinase heme. ACS Chem. Biol. 15, 52–62. doi: 10.1021/acschembio.8b00849
Zhu, C., Preissl, S., and Ren, B. (2020). Single-cell multimodal omics: the power of many. Nat. Methods 17, 11–14. doi: 10.1038/s41592-019-0691-5
Zhu, L. M., Liu, C. H., Chen, P., Dai, A. G., Li, C. X., Xiao, K., et al. (2011). Multidrug-resistant tuberculosis is associated with low plasma concentrations of human neutrophil peptides 1-3. Int. J. Tuberc. Lung Dis. 15, 369–374.
Keywords: Mycobacterium tuberculosis, drug resistance, evolution, bacterial–host interactions, next generation sequencing
Citation: Allué-Guardia A, García JI and Torrelles JB (2021) Evolution of Drug-Resistant Mycobacterium tuberculosis Strains and Their Adaptation to the Human Lung Environment. Front. Microbiol. 12:612675. doi: 10.3389/fmicb.2021.612675
Received: 30 September 2020; Accepted: 15 January 2021;
Published: 04 February 2021.
Edited by:
Jianying Gu, College of Staten Island, United StatesReviewed by:
John T. Belisle, Colorado State University, United StatesOana Dumitrescu, Hospices Civils de Lyon, France
Copyright © 2021 Allué-Guardia, García and Torrelles. This is an open-access article distributed under the terms of the Creative Commons Attribution License (CC BY). The use, distribution or reproduction in other forums is permitted, provided the original author(s) and the copyright owner(s) are credited and that the original publication in this journal is cited, in accordance with accepted academic practice. No use, distribution or reproduction is permitted which does not comply with these terms.
*Correspondence: Anna Allué-Guardia, YWFsbHVlZ3VhcmRpYUB0eGJpb21lZC5vcmc=; Jordi B. Torrelles, anRvcnJlbGxlc0B0eGJpb21lZC5vcmc=