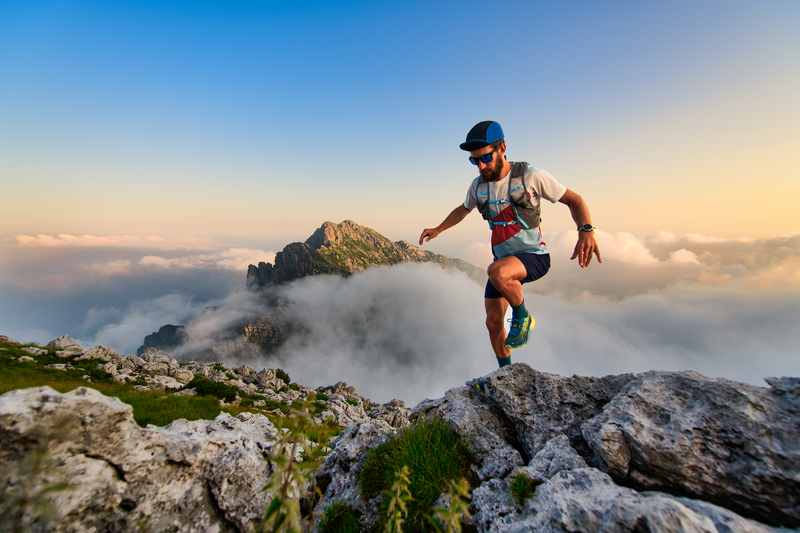
94% of researchers rate our articles as excellent or good
Learn more about the work of our research integrity team to safeguard the quality of each article we publish.
Find out more
ORIGINAL RESEARCH article
Front. Microbiol. , 14 January 2021
Sec. Antimicrobials, Resistance and Chemotherapy
Volume 11 - 2020 | https://doi.org/10.3389/fmicb.2020.626216
This article is part of the Research Topic Therapeutics Targeting Intracellular Bacteria View all 7 articles
Treatment outcomes using the standard regimen (a macrolide, ethambutol, and rifampicin) for Mycobacterium avium complex-pulmonary disease (MAC-PD) remain unsatisfactory. Thus, improved treatment regimens for MAC-PD are required. Clofazimine has recently been revisited as an effective drug against mycobacterial infection. We performed a comparison between the standard regimen and an alternative regimen (replacing the rifampicin of the standard regimen with clofazimine) based on the intracellular anti-MAC activities of the individual drugs in a murine model of chronic progressive MAC-pulmonary infection (MAC-PI). The intracellular anti-MAC activities of the individual drugs and their combinations in murine bone marrow-derived macrophages (BMDMs) were determined. The treatment efficacies of the standard and clofazimine-containing regimens were evaluated in mice chronically infected with M. avium by initiating 2- and 4-week treatment at 8 weeks post-infection. Bacterial loads in the lung, spleen, and liver were assessed along with lung inflammation. Insufficient intracellular anti-MAC activity of rifampicin in BMDMs was recorded despite its low in vitro minimum inhibitory concentrations (MICs), whereas optimal intracellular killing activity against all tested MAC strains was achieved with clofazimine. Compared to the standard regimen, the clofazimine-containing regimen significantly reduced CFUs in all organs and achieved marked reductions in lung inflammation. The replacement of rifampicin with clofazimine in the treatment regimen resulted in more favorable outcomes in an animal model of chronic progressive MAC-PI. Intriguingly, 2 weeks of treatment with the clofazimine-containing regimen reduced bacterial loads more effectively than 4 weeks of treatment with the standard regimen in M. avium-infected mice. Thus, the clofazimine-containing regimen also had a treatment-shortening effect.
Non-tuberculous mycobacteria (NTM), which are ubiquitous and opportunistic pathogens, comprise all the members of Mycobacterium except the Mycobacterium tuberculosis complex and Mycobacterium leprae (Baldwin et al., 2019; Daley et al., 2020). The incidence and prevalence of infectious disease caused by NTM have been increasing globally, leading to an emerging problem related to the difficulty of treatment (Loebinger, 2017; Lee et al., 2019).
The Mycobacterium avium complex (MAC), which comprises slow-growing Mycobacterium species, has been ranked as the most common group of NTM pathogens worldwide (Hoefsloot et al., 2013; Daley et al., 2020). In particular, M. avium and Mycobacterium intracellulare are the main species causing pulmonary disease (PD) and include two major forms: nodular bronchiectatic and fibrocavitary (Koh, 2017). Patients with drug-susceptible MAC-PD generally receive at least three antibiotics, a macrolide [azithromycin or clarithromycin (CLR)], ethambutol (EMB), and rifampicin (RIF), as a standard regimen for at least 12 months based on previous empirical clinical investigations (Griffith et al., 1996; Wallace et al., 1996; Wallace et al., 2014; Griffith, 2018). Although the clinical effectiveness of EMB and RIF in MAC-PD treatment has not been fully verified, these drugs are used as supportive treatments to prevent microbiological resistance to macrolide monotherapy (Kim et al., 2019). In addition, the minimum inhibitory concentrations (MICs) of EMB and RIF are associated with treatment efficacy, and 85.3% of patients whose MAC isolates have MICs of <8 mg/L for RIF and/or EMB have successful treatment (Kwon et al., 2018).
However, the serum concentrations of macrolides, the key drugs for the treatment of MAC disease, have been reported to be low because of the interplay with RIF (Koh et al., 2012; Shimomura et al., 2015; Jeong et al., 2016). RIF is a potent inducer of cytochrome P450 (CYP3A) enzymatic activity, and macrolides are CYP3A inhibitors. However, due to the prominent effect of RIF-mediated CYP3A activation, coadministration of RIF and a macrolide may lead to poor treatment outcomes (Shimomura et al., 2015; Akiyama et al., 2019). Moreover, increased serum concentrations of RIF can cause side effects, such as liver damage, leukopenia, and thrombocytopenia (Shimomura et al., 2015). In addition, a previous study revealed that a treatment regimen consisting only of a macrolide and EMB without RIF resulted in non-inferior outcomes compared with those of the standard three-drug regimen in selected cases (Miwa et al., 2014). Furthermore, Jarand et al. (2016) reported that patients treated with a macrolide, EMB, and clofazimine (CFZ) instead of RIF showed better treatment outcomes than those treated with a RIF-containing regimen. All these results have raised the question of whether RIF plays a sufficient role in MAC therapy despite the low in vitro MIC value of RIF against MAC strains.
CFZ has been used mainly to treat drug-resistant tuberculosis and leprosy (Tyagi et al., 2015). Moreover, it has been introduced for the treatment of NTM-PD caused by not only MAC but also Mycobacterium abscessus (MAB), with promising results (Roussel and Igual, 1998; Field and Cowie, 2003; Jarand et al., 2016; Yang et al., 2017). Particularly, in the treatment of MAC-PD, the CFZ-containing regimen showed favorable treatment outcomes in treatment-naïve patients as well as those with refractory disease (Jarand et al., 2016; Martiniano et al., 2017). In addition, under the guidelines from the Clinical and Laboratory Standards Institute, interest in combinatory regimens containing CFZ has re-emerged, even for the treatment of macrolide-resistant MAC infections (CLSI, 2018). Notably, CFZ had a bacteriostatic effect, and its use in combination with CLR had a synergistic ability to prevent regrowth of MAB and MAC in vitro (Ferro et al., 2016).
However, the clinical use of CFZ based on drug susceptibility testing (DST) still lacks sufficient evidence due to the unclear and unreliable breakpoint for CFZ susceptibility testing (Huang et al., 2018; Luo et al., 2018). Additionally, correlations between the results from in vitro DST, intracellular activity, and in vivo treatment outcomes have not been clearly investigated for MAC clinical isolates (Huang et al., 2018). Although a synergistic effect of CLR with CFZ was demonstrated in a murine model of M. avium ATCC 700898 infection (Lanoix et al., 2020), our present study, to the best of our knowledge, is the first to evaluate CFZ activity against a chronic and progressive infection in a murine model that reflects the clinical situation, along with an investigation of its intracellular anti-MAC activity. Generally, MAC-PD treatment is initiated once disease progression is noted radiologically or once symptoms worsen in patients who have chronic MAC lung infection, with significant bacterial loads and severe inflammation (Thomson and Yew, 2009). Thus, the establishment of chronic MAC-PI in animal models to mimic the condition of patients is necessary to rationally devise more effective MAC-PD chemotherapy regimens. However, the main limitation of in vivo studies is that chronic infection with MAC strains via aerosolization is not easily achieved in mice. Accordingly, the majority of experimental studies of MAC infection in vivo have employed intraperitoneal, intratracheal or intranasal injection rather than aerosol infection (Amaral et al., 2011; Bruffaerts et al., 2017; Abdissa et al., 2018; Kannan et al., 2019). Our previous study mimicked chronic infection with different MAC strains via aerosolization to investigate the possibility of reactivation of MAC infection, and it revealed that the different MAC strains in the mice exhibited different growth rates, which might be associated with differences in host-pathogen interactions (Cha et al., 2015).
In the present study, to compare efficacy between these regimens in MAC-infected mice, we used M. avium SMC #7, a clinical isolate, to cause a chronic progressive pulmonary infection (PI) in mice. The main purpose of this study was to evaluate the efficacy of the standard regimen and an alternative regimen that replaced RIF with CFZ in a murine model of chronic progressive MAC infection based on the in vitro and intracellular activities of individual drugs against MAC clinical isolates.
Eight Mycobacterium species were used in this study (Table 1). Briefly, reference strains were obtained from the American Type Culture Collection (ATCC; Manassas, VA, United States), and all clinical isolates were obtained from patients with the typical nodular bronchiectatic form of MAC-PD who had never been exposed to antimycobacterial antibiotics at the time of diagnosis (Samsung Medical Center, SMC; Seoul, South Korea). All strains were cultivated in Middlebrook 7H9 broth (BD-Difco, Pont-de-Claix, France) supplemented with 10% oleic acid-albumin-dextrose-catalase (OADC) at 37°C. Single-cell suspensions of each strain were prepared as previously described (Cha et al., 2015), and colony-forming units (CFUs) were determined on Middlebrook 7H10 agar plates (BD-Difco).
Table 1. Minimum inhibitory concentrations (MICs) of anti-mycobacterial drugs against Mycobacterium strains used in this study.
All drugs (CLR, EMB, RIF, and CFZ) were purchased from Sigma-Aldrich, Inc. (St. Louis, MO, United States) and were diluted in Dulbecco’s phosphate-buffered saline (DPBS; Biowest, Nuaillé, France) after complete dissolution in dimethyl sulfoxide for in vitro DST and intracellular activity testing. For in vivo oral administration, 0.5% carboxymethylcellulose was used as a vehicle.
Specific pathogen-free female BALB/c and C57BL/6 (6–8 weeks of age) mice were purchased from Japan SLC, Inc. (Shijuoka, Japan). All animal experiments were conducted in accordance with the Korean Food and Drug Administration (KFDA) guidelines and approved by the Ethics Committee and Institutional Animal Care and Use Committee of the Laboratory Animal Research Center at Yonsei University College of Medicine (Permit Number: 2015-0273 and 2018-0229).
In vitro susceptibility testing of MAC strains to individual drugs was determined by the broth microdilution resazurin assay after 7 days of incubation, as previously described. The MICs of the drugs were defined as the lowest concentration of the agents that inhibited bacterial growth. All MIC results for the tested MAC strains were verified by conducting each test twice (in duplicate wells).
Murine bone marrow-derived macrophages (BMDMs) from BALB/c and C57BL/6 mice were differentiated in Dulbecco’s modified Eagle’s medium (Biowest) supplemented with 10% fetal bovine serum (Biowest) and 10% L929 supernatant as previously described (Choi et al., 2019) and used for intracellular anti-MAC activities of the tested drugs at the indicated concentrations. Briefly, BMDMs (3 × 105 cells/ml) were cultured in a 48-well plate for 24 h, infected with the indicated MAC strains (Table 1) at multiplicity of infection of 3 for 4 h, and washed with DPBS. The cells were then cultured with or without drugs in duplicate or triplicate wells for 72 h. The cells were then lysed with 0.05% Triton X -100, and the lysates were serially diluted with DPBS and spotted a maximum of four times per well on 7H10 agar plates supplemented with 10% OADC to quantify the numbers of viable bacteria. Colonies were counted after 14 days of incubation at 37°C, and the values are reported as the mean CFUs ± standard deviations per ml of BMDMs. Each experiment was repeated at least twice independently.
For in vivo experiments, a total of 44 BALB/c mice were infected with M. avium SMC #7 via aerosolization as previously described (Cha et al., 2015). Briefly, the mice were exposed to M. avium SMC #7 via aerosolization using an inhalation exposure system (Glas-Col, Terre Haute, IN, United States). Four to five mice per group were euthanized at 0, 2, 4, and 8 weeks for the establishment phase of the infection, two and four mice in the control group were euthanized at 10 weeks and 12 weeks post infection, and every five mice in the standard regimen group and CFZ-containing regimen group were euthanized at 10 and 12 weeks for evaluation of the bacterial loads in the lung, spleen and liver and histopathological analysis of the lung. At 8 weeks post-infection, treatment with each regimen 5 days per week for 2 and 4 weeks was initiated by oral gavage. The drug doses were as follows: 100 mg/kg for CLR and EMB, 10 mg/kg for RIF and 20 mg/kg for CFZ.
Organ homogenates were plated through serial dilution onto 7H10 agar plates supplemented with 10% OADC and 0.5% amphotericin B (Sigma-Aldrich) for CFU determination. Bacterial colonies were counted after incubation at 37°C for 2 weeks. For histopathological analysis, the right superior lobes of the lungs were preserved in 10% neutral buffered formalin, embedded in paraffin and sectioned at 4–5 μm, followed by hematoxylin and eosin staining. The extent of the affected inflamed area in the lungs was analyzed using open-source ImageJ software (National Institutes of Health, Bethesda, MD, United States).
The Mann–Whitney test was used to evaluate differences between two groups, and ANOVA corrected with Tukey’s test was used to evaluate differences among multiple groups using GraphPad Prism version 7 (San Diego, CA, United States). A p-value < 0.05 was considered statistically significant.
The MICs of CLR, EMB, RIF, and CFZ for the MAC strains used in this study are given in Table 1. Overall, the MAC strains were susceptible to CLR according to Clinical and Laboratory Standards Institute breakpoints (CLSI, 2018) but exhibited varying susceptibility to EMB, RIF, and CFZ, as previously reported (Huang et al., 2018; Wetzstein et al., 2020).
Next, we evaluated the intracellular activities of first-line antibiotics to determine whether a high dose of each drug is effective in inhibiting MAC growth inside macrophages. BMDMs from BALB/c mice were infected with M. avium ATCC 700898 and treated with 10 mg/L CLR, EMB, and RIF for 3 days (Figure 1A). Interestingly, a low MIC (1 mg/L) value was observed for RIF in vitro (Table 1), but RIF was insufficiently effective against M. avium ATCC 700898 at even a 10-fold higher concentration (10 mg/L) in BMDMs (Figure 1A). Furthermore, ineffective intracellular activity of RIF against M. intracellulare ATCC 13950 and M. avium SMC #7 in BMDMs were confirmed despite its low in vitro MICs (0.25 and 4 mg/L, respectively), whereas significant intracellular activity of RIF against M. tuberculosis ATCC 27294 was observed at only 1 mg/L (Figures 1B,C). In addition, M. avium ATCC 700898-infected BMDMs were treated with various concentrations of first-line antibiotics. The results revealed that compared to control treatment, RIF alone had no anti-MAC activity at any concentration in BMDMs (Figure 1D and Supplementary Figure 1). Similarly, M. intracellulare ATCC 13950 exhibited tolerance to high RIF concentrations, even at 40 mg/L, in BMDMs (Figure 1E). Next, to investigate whether the lack of intracellular anti-MAC activity of RIF was due to specific characteristics of macrophages from BALB/c mice, BMDMs from C57BL/6 mice were infected with M. avium ATCC 700898, M. avium 104 (CP000479.1) and M. intracellulare ATCC 13950 and treated with 10 mg/L RIF. Interestingly, RIF was again ineffective against those MAC strains, which was similar to the results obtained for BMDMs from BALB/c mice (Supplementary Figure 2A). Similar to M. avium ATCC 700898, M. avium 104 (CP000479.1) was inhibited by CLR concentrations over 10 mg/L but tolerated a high concentration of RIF (Supplementary Figures 2B,C). Taken together, these results show that the relatively low or absent intracellular anti-MAC activity of RIF did not result from its concentration or specific characteristics of different types of BMDMs, indicating the existence of inconsistent results between in vitro and intracellular activities.
Figure 1. Intracellular anti-MAC activities of first-line drugs in MAC-infected BMDMs. (A) BMDMs were infected with M. avium ATCC 700898 and treated with the indicated doses of CLR, EMB, and RIF. Unless otherwise indicated, all experiments investigating the intracellular activities of drugs in BMDMs were evaluated at 72 h post-infection by plating serially diluted cell lysates onto 7H10-OADC agar plates. (B) BMDMs were infected with M. intracellulare ATCC 13950 or M. avium SMC #7 and treated with 10 mg/L RIF. (C) BMDMs were infected with M. tuberculosis H37Rv and treated with 1 mg/L RIF as a control experiment. BMDMs were infected with (D) M. avium ATCC 700898 or (E) M. intracellulare ATCC 13950 and treated with the indicated doses of RIF. Each experiment was repeated at least twice independently with duplicate or triplicate wells; the results of a representative experiment are shown. Each dot represents the mean value ± S.D. of duplicate or triplicate wells, with four spots applied per well. The Mann–Whitney test was used to evaluate significance, and the results are represented as the mean value ± S.D. ***p < 0.001 vs. Pre-Tx. Pre-Tx, pre-treatment; CTL, untreated control.
To investigate the intracellular anti-MAC activity of CFZ in BMDMs, BMDMs were infected with M. avium ATCC 700898 and M. intracellulare ATCC 13950 and treated with different concentrations of CFZ (Figures 2A,B). Concentrations of CFZ over 5 mg/L exhibited significant intracellular activity against both strains at 72 h post-infection in BMDMs despite the relatively high MIC values for M. avium ATCC 700898 and M. intracellulare ATCC 13950 compared to those for RIF and CLR (Figure 2A,B). Next, BMDMs were infected with M. avium ATCC 700898, M. intracellulare ATCC 13950 or M. avium SMC #7 and treated with 5 mg/L CFZ or 10 mg/L CLR, and intracellular activity was assessed at 72 h post-infection (Figure 2C). Remarkably, CFZ inhibited intracellular bacterial growth to a similar extent as CLR.
Figure 2. Concentration-dependent intracellular activities of CFZ in MAC-infected BMDMs. BMDMs were infected with (A) M. avium ATCC 700898 or (B) M. intracellulare ATCC 13950 and treated with the indicated dose of CFZ. (C) BMDMs were infected with M. avium ATCC 700898, M. intracellulare ATCC 13950 or M. avium SMC #7 and treated with 5 mg/L CFZ or 10 mg/L CLR. After 72 h of cultivation, bacterial CFUs were enumerated by plating serially diluted cell lysates on 7H10-OADC agar plates. Each experiment was repeated at least twice independently with triplicate wells; the results of a representative experiment are shown. Each dot represents the mean value ± S.D. of duplicate or triplicate wells, with four spots applied per well. The Mann–Whitney test was used to evaluate significance, and the results are represented as the mean value ± S.D. ***p < 0.001 vs. Pre-Tx. Pre-Tx, pre-treatment; CTL, untreated control; hpi, h post-infection.
We next investigated the intracellular anti-MAC activities of each drug at different concentrations based on the in vitro MICs in BMDMs (Supplementary Figure 3). According to the in vitro MIC results (Table 1), the concentrations of all drugs except CFZ for monotherapy were fixed at 5 × MIC, and CFZ was fixed at 2 × MIC. The concentrations for the combined therapy were chosen to be 1 × MIC and 3 × MIC for each strain. CFZ exhibited an equally significant reduction in the viability of six MAC strains in BMDMs at 72 h post-infection (p < 0.001), even at only 2 × MIC. Finally, the intracellular anti-MAC activities of the standard regimen (CLR-EMB-RIF) versus the CFZ-containing regimen, as shown in Figure 3 and Supplementary Figure 4, were compared. The majority of MAC strains (i.e., all strains except M. avium SMC #7) displayed significantly lower viability at both 1 × MIC and 3 × MIC for the CFZ-containing regimen than for the standard regimen at 72 h in BMDMs (p < 0.001) (Figure 3 and Supplementary Figure 4). Interestingly, bacterial growth of M. intracellulare SMC #11 was naturally inhibited in BMDMs without anti-MAC drugs. However, we confirmed that it was not due to cytotoxic effect of M. intracellulare SMC #11 on BMDMs (data not shown). Taken together, these results suggest that a concentration of 1 × MIC was sufficient to control intracellular growth (Figure 3).
Figure 3. Comparative evaluation of the intracellular activities of drug combinations for the standard regimen and for the CFZ-containing regimen against a variety of MAC strains in BMDMs. BMDMs were infected with each MAC strain and treated with the drug regimen at 1 × MIC according to the data in Table 1. (A) M. avium ATCC 700898 and M. intracellulare ATCC 13950, (B) M. avium SMC #1 and M. avium SMC #7 and (C) M. intracellulare SMC #8 and M. intracellulare SMC #11 were assessed at 72 h post-infection by plating serially diluted cell lysates on 7H10-OADC agar plates. Each experiment was repeated at least twice independently with triplicate wells; the results of a representative experiment are shown. The Mann–Whitney test was used to evaluate significance, and the results are represented as the mean value ± S.D. ***p < 0.001 and n.s., not significant. CTL, untreated control; CLR-EMB-RIF, standard regimen; CLR-EMB-CFZ, CFZ-containing regimen; hpi, h post-infection.
Since treatment for patients with MAC-PD is initiated after disease progression becomes imminent, we established a murine model of chronic progressive MAC-PI using BALB/c mice by aerosol infection with M. avium SMC #7 (Figure 4A). At 8 weeks post-infection, the treatment efficacies of the regimen containing CFZ instead of RIF and the standard regimen were investigated for 2 and 4 weeks (Figure 4A). The impact of the CFZ-containing regimen on bacterial load is shown as the mean and log10 reduction in CFU. At both 2 and 4 weeks post-treatment, there was a significant reduction in the numbers of M. avium SMC #7 in the CFZ-containing regimen group, with a reduction of 2.24 log10 units at 2 weeks and 2.10 log10 units at 4 weeks compared with those in the standard regimen group (p < 0.001). Interestingly, the bacterial load of the CFZ-containing regimen group at 2 weeks was reduced effectively compared with that of the standard regimen group at 4 weeks, with a 1.04 log10 reduction (p < 0.05) (Figure 4B). In the spleen and liver, the antibiotic activities were similar, with a reduction in activity in the lung (Figures 4C,D). In the spleen, reductions of 1.68 log10 units at 2 weeks and 2.45 log10 units at 4 weeks were obtained for the CFZ-containing regimen group compared with the standard regimen group (p < 0.001). In the liver, reductions of 1.97 log10 units at 2 weeks (p < 0.001) and 1.16 log10 units at 4 weeks (p < 0.01) were obtained in the CFZ-containing regimen group compared with the standard regimen group.
Figure 4. Comparative assessment of treatment efficacy of the standard and CFZ-containing regimens in a murine model of chronic progressive MAC-PI. (A) Schematic design of the in vivo experiment. BALB/c mice were infected with the M. avium SMC #7 clinical isolate via aerosolization, achieving a mean initial bacterial number of 2 × 105 CFUs in the lungs. Two weeks and 4 weeks of therapy were initiated with the standard and CFZ-containing regimens at 8 weeks post-infection. The daily drug doses were 100 mg/kg for CLR and EMB, 10 mg/kg for RIF and 20 mg/kg for CFZ. The mice were euthanized, and the lungs were homogenized for histopathological examination and bacterial counts at 2 and 4 weeks post-treatment. Plotted infection data and mean CFU counts and bar graphs for the log10 reduction in CFU from treatment initiation in the (B) lungs, (C) spleens and (D) livers were assessed after 2 and 4 weeks of treatment with the two regimens by plating serially diluted tissue lysates onto 7H10-OADC agar plates. The broken vertical line of each plotted data indicates the day of treatment initiation. The statistical significance in (B–D) was calculated by one-way ANOVA followed by Tukey’s multiple comparison test, and the results are represented as the mean value ± S.D. **p < 0.01, ***p < 0.001 and n.s., not significant. MAC-PI, M. avium complex-pulmonary infection; CTL, untreated control; CLR-EMB-RIF, standard regimen; CLR-EMB-CFZ, CFZ-containing regimen.
Histopathological staining showed inflamed areas in M. avium-infected lungs (Figure 5A), consistent with the bacterial loads. Surprisingly, the inflamed area was diminished by the CFZ-containing regimen at only 2 weeks after starting treatment (p < 0.001) and was reduced even more than at treatment initiation (p < 0.001), but there was no significant difference in lung gross pathologic findings between the untreated control group and standard regimen group. At 4 weeks post-treatment, both the standard regimen group and CFZ-containing regimen group had significantly reduced inflamed areas (p < 0.001), and treatment with the CFZ-containing regimen resulted in more antibacterial activity than treatment with the standard regimen (p < 0.01) (Figure 5B).
Figure 5. Histopathological evaluation of lung inflammation between the standard and CFZ-containing regimens at 2 and 4 weeks post-treatment. (A) Representative 10× and 100× magnification photomicrographs of H&E-stained lung tissue from the superior lobe of the right lung are shown. Each treatment regimen is indicated below the representative lung pathology. (B) Quantitation of inflamed areas of the H&E-stained samples of (A) is presented as bar graphs. All data for inflamed areas were analyzed by one-way ANOVA followed by Tukey’s multiple comparison test to evaluate significance, and the results are represented as the mean value ± S.D. **p < 0.01, ***p < 0.001 and n.s., not significant. Pre-Tx, pre-treatment; CTL, untreated control; CLR-EMB-RIF, standard regimen; CLR-EMB-CFZ, CFZ-containing regimen.
Although previous studies showed that a treatment regimen containing CFZ resulted in favorable outcomes for patients with MAC-PD, there have been few studies elucidating its effect on macrophages and relevant animal models. In the present study, CFZ showed effective intracellular anti-MAC activity against all MAC strains, and the CFZ-containing regimen provided significantly improved treatment efficacy in a murine model of chronic progressive MAC-PI, with greater efficacy after 2 weeks of treatment with this regimen than after 4 weeks of treatment with the standard regimen, by rapidly reducing bacterial loads and lung inflammation. Recently, due to a lack of compatible new drugs with low toxicity and high effectiveness, existing drugs have been repurposed or repositioned as the current candidate drugs for the treatment of MAC disease (Wu et al., 2018).
Macrolides have been considered the cornerstone drugs in the treatment of MAC disease, followed by EMB and RIF (Daley et al., 2020). Kim et al. (2019) reported that MAC-PD patients who maintained treatment with either EMB or RIF with a macrolide had a higher probability of culture conversion than patients who maintained treatment with a macrolide only, but maintenance with EMB was superior to maintenance with RIF in terms of treatment outcomes and microbiological cure rates. In addition, Miwa et al. (2014) reported that a double regimen without RIF was not inferior to a standard regimen with respect to culture conversion after 12 months of treatment in patients with MAC. Additionally, clinical research regarding the CFZ-containing regimen and RIF-containing regimen demonstrated that 100% of patients treated with CFZ achieved negative culture results, while only 71% of patients treated with RIF had successful sputum culture conversion (Jarand et al., 2016). For this reason, we investigated the individual efficacy of first-line antibiotics through intracellular activity in MAC-infected BMDMs. The main limitation of in vitro DST is that fixed breakpoints of EMB and RIF have not been clearly established, although those drugs have been considered first-line drugs (CLSI, 2018). Therefore, we attempted to evaluate the intracellular anti-MAC activities of these drugs prior to conducting in vivo efficacy testing since in vitro DST results varied according to MAC strains. To evaluate the intracellular efficacies of antibiotics, seven different MAC strains showing different MIC values against antibiotics were used. Intriguingly, RIF did not confer anti-MAC activity in BMDMs despite its low MIC, regardless of the macrophage source (i.e., different mouse strains). Surprisingly, few studies have investigated the intracellular activity of RIF against MAC infection in macrophages. Moreover, no consistent results among studies have been reported; Rastogi et al. (1987) and Rastogi and Labrousse (1991) showed that RIF was bactericidal for M. avium in the J774 mouse macrophage cell line, whereas Yajko et al. (1989) reported that 11 of 13 MAC strains survived in the same J774 cells (with more than 90% of the initial number in infected cells) after treatment with 8 mg/L RIF for 7 days.
The in vitro activity of CFZ against the MAC is also still controversial. Huang et al. (2018) reported CFZ MIC ranges from 1 to 4 mg/L for 83 clinical MAC isolates of M. avium, with MICs ≤ 1 mg/L against the majority of M. intracellulare isolates. In addition, Kwak et al. (2020) reported that the MICs of CFZ for 133 MAC strains ranged from 0.031 to 8 mg/L, and more than 75% of the MAC isolates had MIC values ≤ 1 mg/L. The cut-off values of several studies could be variable due to the use of different MAC species and different culture conditions. In our study, a total of seven MAC strains had MIC values from 1 to 4 mg/L, consistent with the range reported for CFZ in previous studies (Huang et al., 2018; Kwak et al., 2020). Since drug options have been limited for MAC-PD treatment, the general choice of drug regimen has been empirically selected based on MIC determination (Renvoise et al., 2014). However, unexpectedly, RIF showed the weakest correlation between its in vitro MIC and intracellular anti-MAC activity. In contrast, in our investigation, CFZ could be an effective drug displaying positive correlations between the in vitro MIC and intracellular anti-MAC activity, similar to CLR. In addition, CFZ had better synergistic effects with CLR than with RIF on growth inhibition of all strains except M. avium SMC #7 in macrophages. Indeed, many previous studies have reported variation in virulence among MAC strains and different host responses in macrophages and mice (Amaral et al., 2011; Agdestein et al., 2014; Bruffaerts et al., 2017; Abdissa et al., 2018; Rindi et al., 2018; Kannan et al., 2019). Moreover, antibiotics are well known to be associated with immune responses (Labro and Abdelghaffar, 2001; Tamaoki, 2004; Yuhas et al., 2007; Yuhas et al., 2011; Singh et al., 2014). Hence, variation in virulence and host responses and the influence of antibiotics might explain why intracellular M. avium SMC #7 was inhibited by both regimens with the same efficacy. Therefore, to evaluate the efficacies of the regimens for chronic progressive disease, mice were infected via aerosol infection with a variety of MAC strains including M. avium ATCC 700898 and M. avium SMC #7. However, mice infected with various MAC strains and M. avium ATCC 700898 underwent bacterial clearance, and chronic progressive infection could not be established (data not shown), whereas mice infected with M. avium SMC #7 established a chronic pulmonary infection with granulomatous inflammation. Although we found that the CFZ-containing regimen was superior to the standard regimen through the in vivo study with M. avium SMC #7, the intracellular activity of CFZ-containing regimen displayed a lesser extent to the other MAC strains in BMDMs. We assume that the difference in results between intracellular activity of CFZ and its in vivo efficacy testing against M. avium SM #7 might be from the frequencies of treatment and immune status between BMDMs and animals. Thus, the refined intracellular drug assays that mimic in vivo conditions should ensure more accurate results, particularly, when testing new drugs. Nevertheless, our results clearly displayed the effective intracellular activity of CFZ against various clinical MAC strains in BMDMs.
Since effective MAC-PD treatment requires at least 12 months of combination therapy (Daley et al., 2020) and since disease progression may worsen radiologically due to severe lung inflammation (Thomson and Yew, 2009), evaluating lung inflammation during antibiotic treatment is necessary to devise more effective MAC-PD chemotherapy regimens. Accordingly, we showed that lung inflammation and bacterial loads declined much earlier in mice treated with the CFZ-containing regimen than in mice treated with the standard regimen. Lung inflammation in mice treated with the CFZ-containing regimen was significantly reduced at 2 weeks post-treatment, whereas lung inflammation in mice treated with the standard regimen was not significantly reduced at this time point. Moreover, treatment with a CFZ-containing regimen at 2 weeks post-treatment was more effective than treatment with a standard regimen for 4 weeks, indicating that the CFZ-containing regimen also had a treatment-shortening effect.
Overall, we evaluated a CFZ-containing regimen for the treatment of a murine model of chronic progressive MAC-PI based on its intracellular activity against a variety of MAC strains in BMDMs. These results provide a rationale for the use of CFZ as a component of an optimal treatment regimen instead of the currently used standard regimen. Furthermore, investigations of treatment regimens combining macrolides and CFZ with other drugs to enhance treatment success in a short duration are needed. Another important finding is that RIF generally had no intracellular anti-MAC activity despite its low MICs against MAC strains, indicating that the host-mediated mechanism of RIF tolerance in macrophages is worth further investigation.
The original contributions generated for this study are included in the article/Supplementary Material, further inquiries can be directed to the corresponding author/s.
The animal study was reviewed and approved by the Ethics Committee and Institutional Animal Care and Use Committee of the Laboratory Animal Research Center at Yonsei University College of Medicine (Permit Numbers: 2015-0273 and 2018-0229).
L-HK and SS conceived, designed, and supervised the work. JL and JP together with L-HK and SC analyzed the data and interpreted the results. BJ and S-YK provided the clinical MAC isolates obtained from MAC-PD patients. JL and JP drafted and prepared the manuscript while BJ, S-YK, K-WJ, JH, L-HK, and SS were involved in the editing of the manuscript. All authors read and approved the final version.
This work was supported by the Korea Health Technology R&D Project through the Korea Health Industry Development Institute (KHIDI), funded by the Ministry of Health and Welfare, Republic of Korea (HI20C0017 to SS and BJ) and the Korea Research Institute of Bioscience and Biotechnology Research Initiative Program, Republic of Korea (KGM4572013 to SS and JH).
The authors declare that the research was conducted in the absence of any commercial or financial relationships that could be construed as a potential conflict of interest.
We would like our sincere gratitude to Dr. Won-Jung Koh for his contribution to initiate, conceive, and design this work. He was our mentor and project leader of our team.
The Supplementary Material for this article can be found online at: https://www.frontiersin.org/articles/10.3389/fmicb.2020.626216/full#supplementary-material
Abdissa, K., Nerlich, A., Beineke, A., Ruangkiattikul, N., Pawar, V., Heise, U., et al. (2018). Presence of infected Gr-1(int)CD11b(hi)CD11c(int) monocytic myeloid derived suppressor cells subverts T Cell response and is associated with impaired dendritic cell function in Mycobacterium avium-infected mice. Front. Immunol. 9:2317. doi: 10.3389/fimmu.2018.02317
Agdestein, A., Jones, A., Flatberg, A., Johansen, T. B., Heffernan, I. A., Djonne, B., et al. (2014). Intracellular growth of Mycobacterium avium subspecies and global transcriptional responses in human macrophages after infection. BMC Genom. 15:58. doi: 10.1186/1471-2164-15-58
Akiyama, N., Inui, N., Mori, K., Nakamura, Y., Hayakawa, H., Tanaka, S., et al. (2019). Effect of rifampicin and clarithromycin on the CYP3A activity in patients with Mycobacterium avium complex. J. Thorac. Dis. 11, 3814–3821. doi: 10.21037/jtd.2019.09.06
Amaral, E. P., Kipnis, T. L., de Carvalho, E. C., da Silva, W. D., Leao, S. C., and Lasunskaia, E. B. (2011). Difference in virulence of Mycobacterium avium isolates sharing indistinguishable DNA fingerprint determined in murine model of lung infection. PLoS One 6:e21673. doi: 10.1371/journal.pone.0021673
Baldwin, S. L., Larsen, S. E., Ordway, D., Cassell, G., and Coler, R. N. (2019). The complexities and challenges of preventing and treating nontuberculous mycobacterial diseases. PLoS Negl. Trop. Dis. 13:e0007083. doi: 10.1371/journal.pntd.0007083
Bruffaerts, N., Vluggen, C., Roupie, V., Duytschaever, L., Van den Poel, C., Denoel, J., et al. (2017). Virulence and immunogenicity of genetically defined human and porcine isolates of M. avium subsp. hominissuis in an experimental mouse infection. PLoS One 12:e0171895. doi: 10.1371/journal.pone.0171895
Cha, S. B., Jeon, B. Y., Kim, W. S., Kim, J. S., Kim, H. M., Kwon, K. W., et al. (2015). Experimental reactivation of pulmonary Mycobacterium avium complex infection in a modified cornell-like murine model. PLoS One 10:e0139251. doi: 10.1371/journal.pone.0139251
Choi, H. H., Kwon, K. W., Han, S. J., Kang, S. M., Choi, E., Kim, A., et al. (2019). PPE39 of the Mycobacterium tuberculosis strain Beijing/K induces Th1-cell polarization through dendritic cell maturation. J. Cell Sci. 132:jcs228700. doi: 10.1242/jcs.228700
CLSI (2018). Susceptibility Testing of Mycobacteria, Nocardia spp., and Other Aerobic Actinomycetes, M24Ed3. Wayne, PA: CLSI.
Daley, C. L., Iaccarino, J. M., Lange, C., Cambau, E., Wallace, R. J., Andrejak, C., et al. (2020). Treatment of nontuberculous mycobacterial pulmonary disease: an official ATS/ERS/ESCMID/IDSA clinical practice guideline: executive summary. Clin. Infect. Dis. 71, e1–e36. doi: 10.1093/cid/ciaa241
Ferro, B. E., Meletiadis, J., Wattenberg, M., de Jong, A., van Soolingen, D., Mouton, J. W., et al. (2016). Clofazimine prevents the regrowth of Mycobacterium abscessus and Mycobacterium avium type strains exposed to Amikacin and Clarithromycin. Antimicrob Agents Chemother 60, 1097–1105. doi: 10.1128/AAC.02615-2615
Field, S. K., and Cowie, R. L. (2003). Treatment of Mycobacterium avium-intracellulare complex lung disease with a macrolide, ethambutol, and clofazimine. Chest 124, 1482–1486. doi: 10.1378/chest.124.4.1482
Griffith, D. E. (2018). Treatment of Mycobacterium avium complex (MAC). Semin. Respir. Crit. Care Med. 39, 351–361. doi: 10.1055/s-0038-1660472
Griffith, D. E., Brown, B. A., Girard, W. M., Murphy, D. T., et al. (1996). Azithromycin activity against Mycobacterium avium complex lung disease in patients who were not infected with human immunodeficiency virus. Clin. Infect. Dis. 23, 983–989. doi: 10.1093/clinids/23.5.983
Hoefsloot, W., van Ingen, J., Andrejak, C., Angeby, K., Bauriaud, R., Bemer, P., et al. (2013). The geographic diversity of nontuberculous mycobacteria isolated from pulmonary samples: an NTM-NET collaborative study. Eur. Respir. J. 42, 1604–1613. doi: 10.1183/09031936.00149212
Huang, C. C., Wu, M. F., Chen, H. C., and Huang, W. C. (2018). In vitro activity of aminoglycosides, clofazimine, d-cycloserine and dapsone against 83 Mycobacterium avium complex clinical isolates. J. Microbiol. Immunol. Infect. 51, 636–643. doi: 10.1016/j.jmii.2017.05.001
Jarand, J., Davis, J. P., Cowie, R. L., Field, S. K., and Fisher, D. A. (2016). Long-term follow-up of Mycobacterium avium complex lung disease in patients treated with regimens including clofazimine and/or Rifampin. Chest 149, 1285–1293. doi: 10.1378/chest.15-0543
Jeong, B. H., Jeon, K., Park, H. Y., Moon, S. M., Kim, S. Y., Lee, S. Y., et al. (2016). Peak plasma concentration of azithromycin and treatment responses in Mycobacterium avium complex lung disease. Antimicrob Agents Chemother 60, 6076–6083. doi: 10.1128/AAC.00770-716
Kannan, N., Lai, Y. P., Haug, M., Lilleness, M. K., Bakke, S. S., Marstad, A., et al. (2019). Genetic Variation/Evolution and differential host responses resulting from in-patient adaptation of Mycobacterium avium. Infect. Immun. 87, e00323-318. doi: 10.1128/IAI.00323-18
Kim, H. J., Lee, J. S., Kwak, N., Cho, J., Lee, C. H., Han, S. K., et al. (2019). Role of ethambutol and rifampicin in the treatment of Mycobacterium avium complex pulmonary disease. BMC Pulm Med. 19:212. doi: 10.1186/s12890-019-0982-988
Koh, W. J., Jeong, B. H., Jeon, K., Lee, S. Y., and Shin, S. J. (2012). Therapeutic drug monitoring in the treatment of Mycobacterium avium complex lung disease. Am. J. Respir. Crit. Care Med. 186, 797–802. doi: 10.1164/rccm.201206-1088OC
Koh, W. J. (2017). Nontuberculous mycobacteria-overview. Microbiol. Spectr. 5, doi: 10.1128/microbiolspec.TNMI7-0024-2016
Kwak, N., Whang, J., Yang, J. S., Kim, T. S., Kim, S. A., and Yim, J. J. (2020). Minimal inhibitory concentration of clofazimine among clinical isolates of nontuberculous mycobacteria and its impact on treatment outcome. Chest doi: 10.1016/j.chest.2020.07.040
CrossRef Full Text Online ahead of print. Google Scholar
Kwon, B. S., Kim, M. N., Sung, H., Koh, Y., Kim, W. S., Song, J. W., et al. (2018). In vitro MIC values of rifampin and ethambutol and treatment outcome in Mycobacterium avium complex lung disease. Antimicrob Agents Chemother 62, e00491-18. doi: 10.1128/AAC.00491-18
Labro, M. T., and Abdelghaffar, H. (2001). Immunomodulation by macrolide antibiotics. J. Chemother 13, 3–8. doi: 10.1179/joc.2001.13.1.3
Lanoix, J. P., Joseph, C., Peltier, F., Castelain, S., and Andrejak, C. (2020). Synergistic activity of clofazimine and clarithromycin in an aerosol mouse model of Mycobacterium avium infection. Antimicrob Agents Chemother 64, e02349-19. doi: 10.1128/AAC.02349-2319
Lee, H., Myung, W., Koh, W. J., Moon, S. M., and Jhun, B. W. (2019). Epidemiology of nontuberculous mycobacterial infection, South Korea, 2007-2016. Emerg. Infect. Dis. 25, 569–572. doi: 10.3201/eid2503.181597
Loebinger, M. R. (2017). Mycobacterium avium complex infection: phenotypes and outcomes. Eur. Respir. J. 50:1701380. doi: 10.1183/13993003.01380-2017
Luo, J., Yu, X., Jiang, G., Fu, Y., Huo, F., Ma, Y., et al. (2018). In vitro activity of clofazimine against nontuberculous mycobacteria isolated in Beijing, China. Antimicrob Agents Chemother 62:e00072-18. doi: 10.1128/AAC.00072-18
Martiniano, S. L., Wagner, B. D., Levin, A., Nick, J. A., Sagel, S. D., and Daley, C. L. (2017). Safety and effectiveness of clofazimine for primary and refractory nontuberculous mycobacterial infection. Chest 152, 800–809. doi: 10.1016/j.chest.2017.04.175
Miwa, S., Shirai, M., Toyoshima, M., Shirai, T., Yasuda, K., Yokomura, K., et al. (2014). Efficacy of clarithromycin and ethambutol for Mycobacterium avium complex pulmonary disease. a preliminary study. Ann. Am. Thorac. Soc. 11, 23–29. doi: 10.1513/AnnalsATS.201308-266OC
Rastogi, N., and Labrousse, V. (1991). Extracellular and intracellular activities of clarithromycin used alone and in association with ethambutol and rifampin against Mycobacterium avium complex. Antimicrob Agents Chemother 35, 462–470. doi: 10.1128/aac.35.3.462
Rastogi, N., Potar, M. C., and David, H. L. (1987). Intracellular growth of pathogenic mycobacteria in the continuous murine macrophage cell-line j-774 - ultrastructure and drug-susceptibility studies. Curr. Microbiol. 16, 79–92. doi: 10.1007/Bf01588176
Renvoise, A., Bernard, C., Veziris, N., Galati, E., Jarlier, V., and Robert, J. (2014). Significant difference in drug susceptibility distribution between Mycobacterium avium and Mycobacterium intracellulare. J. Clin. Microbiol. 52, 4439–4440. doi: 10.1128/JCM.02127-2114
Rindi, L., Lari, N., and Garzelli, C. (2018). Virulence of Mycobacterium avium Subsp. hominissuis human isolates in an in vitro macrophage infection model. Int. J. Mycobacteriol. 7, 48–52. doi: 10.4103/ijmy.ijmy_11_18
Roussel, G., and Igual, J. (1998). Clarithromycin with minocycline and clofazimine for Mycobacterium avium intracellulare complex lung disease in patients without the acquired immune deficiency syndrome. GETIM. Groupe d’Etude et de traitement des infections a mycobacteries. Int. J. Tuberc. Lung. Dis. 2, 462–470.
Shimomura, H., Andachi, S., Aono, T., Kigure, A., Yamamoto, Y., Miyajima, A., et al. (2015). Serum concentrations of clarithromycin and rifampicin in pulmonary Mycobacterium avium complex disease: long-term changes due to drug interactions and their association with clinical outcomes. J. Pharm. Health Care Sci. 1:32. doi: 10.1186/s40780-015-0029-20
Singh, S., Kubler, A., Singh, U. K., Singh, A., Gardiner, H., Prasad, R., et al. (2014). Antimycobacterial drugs modulate immunopathogenic matrix metalloproteinases in a cellular model of pulmonary tuberculosis. Antimicrob Agents Chemother 58, 4657–4665. doi: 10.1128/AAC.02141-2113
Tamaoki, J. (2004). The effects of macrolides on inflammatory cells. Chest 125, 41S–50S. doi: 10.1378/chest.125.2_suppl.41s
Thomson, R. M., and Yew, W. W. (2009). When and how to treat pulmonary non-tuberculous mycobacterial diseases. Respirology 14, 12–26. doi: 10.1111/j.1440-1843.2008.01408.x
Tyagi, S., Ammerman, N. C., Li, S. Y., Adamson, J., Converse, P. J., Swanson, R. V., et al. (2015). Clofazimine shortens the duration of the first-line treatment regimen for experimental chemotherapy of tuberculosis. Proc. Natl. Acad. Sci. U S A. 112, 869–874. doi: 10.1073/pnas.1416951112
Wallace, R. J. Jr., Brown, B. A., Griffith, D. E., Girard, W. M., and Murphy, D. T. (1996). Clarithromycin regimens for pulmonary Mycobacterium avium complex. the first 50 patients. Am. J. Respir. Crit. Care Med. 153(6 Pt 1), 1766–1772. doi: 10.1164/ajrccm.153.6.8665032
Wallace, R. J. Jr., Brown-Elliott, B. A., McNulty, S., Philley, J. V., Killingley, J., et al. (2014). Macrolide/Azalide therapy for nodular/bronchiectatic Mycobacterium avium complex lung disease. Chest 146, 276–282. doi: 10.1378/chest.13-2538
Wetzstein, N., Kohl, T. A., Andres, S., Schultze, T. G., Geil, A., Kim, E., et al. (2020). Comparative analysis of phenotypic and genotypic antibiotic susceptibility patterns in Mycobacterium avium complex. Int. J. Infect. Dis. 93, 320–328. doi: 10.1016/j.ijid.2020.02.059
Wu, M. L., Aziz, D. B., Dartois, V., and Dick, T. (2018). NTM drug discovery: status, gaps and the way forward. Drug Discov. Today 23, 1502–1519. doi: 10.1016/j.drudis.2018.04.001
Yajko, D. M., Nassos, P. S., Sanders, C. A., and Hadley, W. K. (1989). Killing by antimycobacterial agents of AIDS-derived strains of Mycobacterium avium complex inside cells of the mouse macrophage cell line J774. Am. Rev. Respir. Dis. 140, 1198–1203. doi: 10.1164/ajrccm/140.5.1198
Yang, B., Jhun, B. W., Moon, S. M., Lee, H., Park, H. Y., Jeon, K., et al. (2017). Clofazimine-Containing regimen for the treatment of Mycobacterium abscessus Lung Disease. Antimicrob Agents Chemother 61:e02052-16. doi: 10.1128/AAC.02052-2016
Yuhas, Y., Azoulay-Alfaguter, I., Berent, E., and Ashkenazi, S. (2007). Rifampin inhibits prostaglandin E2 production and arachidonic acid release in human alveolar epithelial cells. Antimicrob Agents Chemother 51, 4225–4230. doi: 10.1128/AAC.00985-987
Keywords: Mycobacterium avium complex-pulmonary disease, clofazimine, clofazimine-containing regimen, standard treatment regimen, minimum inhibitory concentrations, intracellular drug susceptibility test, in vivo drug susceptibility test, chronic progressive murine model
Citation: Lee JM, Park J, Choi S, Jhun BW, Kim S-Y, Jo K-W, Hong JJ, Kim L-H and Shin SJ (2021) A Clofazimine-Containing Regimen Confers Improved Treatment Outcomes in Macrophages and in a Murine Model of Chronic Progressive Pulmonary Infection Caused by the Mycobacterium avium Complex. Front. Microbiol. 11:626216. doi: 10.3389/fmicb.2020.626216
Received: 05 November 2020; Accepted: 22 December 2020;
Published: 14 January 2021.
Edited by:
Nagendran Tharmalingam, Rhode Island Hospital, United StatesReviewed by:
Durai Sellegounder, Buck Institute for Research on Aging, United StatesCopyright © 2021 Lee, Park, Choi, Jhun, Kim, Jo, Hong, Kim and Shin. This is an open-access article distributed under the terms of the Creative Commons Attribution License (CC BY). The use, distribution or reproduction in other forums is permitted, provided the original author(s) and the copyright owner(s) are credited and that the original publication in this journal is cited, in accordance with accepted academic practice. No use, distribution or reproduction is permitted which does not comply with these terms.
*Correspondence: Sung Jae Shin, c2pzaGluQHl1aHMuYWM=; Lee-Han Kim, bGVlaGFuMjY1MkB5dWhzLmFj
†These authors have contributed equally to this work and share first authorship
Disclaimer: All claims expressed in this article are solely those of the authors and do not necessarily represent those of their affiliated organizations, or those of the publisher, the editors and the reviewers. Any product that may be evaluated in this article or claim that may be made by its manufacturer is not guaranteed or endorsed by the publisher.
Research integrity at Frontiers
Learn more about the work of our research integrity team to safeguard the quality of each article we publish.