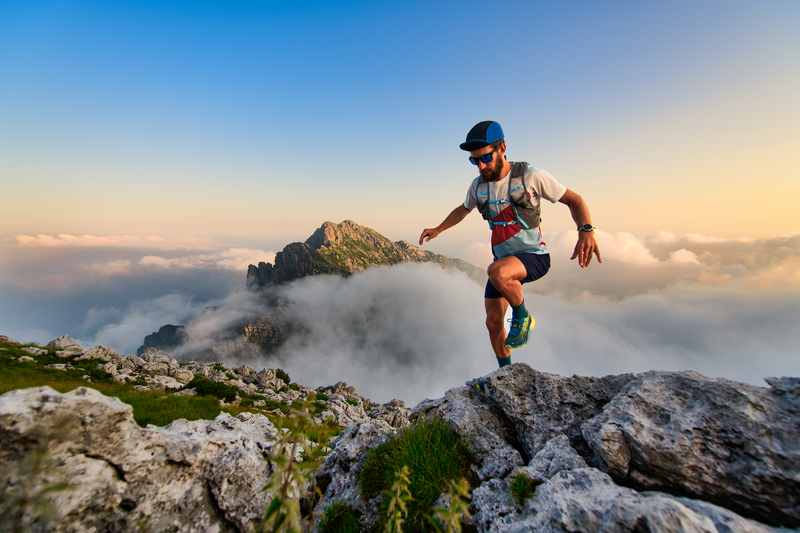
94% of researchers rate our articles as excellent or good
Learn more about the work of our research integrity team to safeguard the quality of each article we publish.
Find out more
ORIGINAL RESEARCH article
Front. Microbiol. , 08 December 2020
Sec. Microbial Immunology
Volume 11 - 2020 | https://doi.org/10.3389/fmicb.2020.608752
This article is part of the Research Topic The Role of Dietary Interventions in The Regulation of Host-Microbe Interactions View all 24 articles
Potential probiotic or immunobiotic effects of lactic acid bacteria (LAB) isolated from the milk of the South American camelid llama (Lama glama) have not been reported in published studies. The aim of the present work was to isolate beneficial LAB from llama milk that can be used as potential probiotics active against bacterial pathogens. LAB strains were isolated from llama milk samples. In vitro functional characterization of the strains was performed by evaluating the resistance against gastrointestinal conditions and inhibition of the pathogen growth. Additionally, the adhesive and immunomodulatory properties of the strains were assessed. The functional studies were complemented with a comparative genomic evaluation and in vivo studies in mice. Ligilactobacillus salivarius TUCO-L2 showed enhanced probiotic/immunobiotic potential compared to that of other tested strains. The TUCO-L2 strain was resistant to pH and high bile salt concentrations and demonstrated antimicrobial activity against Gram-negative intestinal pathogens and adhesion to mucins and epithelial cells. L. salivarius TUCO-L2 modulated the innate immune response triggered by Toll-like receptor (TLR)-4 activation in intestinal epithelial cells. This effect involved differential regulation of the expression of inflammatory cytokines and chemokines mediated by the modulation of the negative regulators of the TLR signaling pathway. Moreover, the TUCO-L2 strain enhanced the resistance of mice to Salmonella infection. This is the first report on the isolation and characterization of a potential probiotic/immunobiotic strain from llama milk. The in vitro, in vivo, and in silico investigation performed in this study reveals several research directions that are needed to characterize the TUCO-L2 strain in detail to position this strain as a probiotic or immunobiotic that can be used against infections in humans or animals, including llama.
The Camelidae family comprises the dromedary camel or camel of the plains (Camelus dromedarius), Bactrian camel or camel of the mountains (Camelus bactrianus), and four species of South American camelids, including guanaco (Lama guanicoe), vicuña (Vicugna vicugna), alpaca (Lama pacos), and llama (Lama glama) (Fukuda, 2013; Zarrin et al., 2020). The milk of camels has been a part of the daily diet of nomadic people living in the steppe and arid areas of central Asia for centuries because of its good nutritional properties (Shabo et al., 2005). The beneficial effects of the camel milk are attributed to its nutritional composition, physicochemical characteristics, and rich microbial population (Dong et al., 2012; Jans et al., 2012). Therefore, in the past decade, considerable efforts were aimed at characterizing the microbial populations of the camel milk to isolate the beneficial strains.
Several studies described the complex population of lactic acid bacteria (LAB) in the camel milk. Earlier studies reported the presence of Lactobacillus spp., Lactococcus spp., Streptococcus spp., and Enterococcus spp. in the milk of camels (Khedid et al., 2009). Moreover, a study reported that the most abundant cultivable species of Gram-positive cocci included Enterococcus faecium and Lactococcus lactis; the most abundant lactobacilli species included Lactobacillus helveticus, Lacticaseibacillus casei (basonym: Lactobacillus casei (Zheng et al., 2020), and Lactiplantibacillus plantarum (basonym: Lactobacillus plantarum). Subsequent studies confirmed the dominance of enterococci and lactococci in the cultivable bacteria from camel milk but detected certain differences in the predominant species of lactobacilli (Elbanna et al., 2018; Rahmeh et al., 2019; Zhao et al., 2020) that included Lacticaseibacillus paracasei (basonym: Lactobacillus paracasei), Lacticaseibacillus rhamnosus (basonym: Lactobacillus rhamnosus), and Limosilactobacillus reuteri (basonym: Lactobacillus reuteri). In addition to the characterization of microbial populations, certain strains with beneficial effects have been isolated from the camel milk. A strain isolated from fermented camel milk, L. casei TN-2, produces a bacteriocin that inhibits Escherichia coli and Staphylococcus aureus (Lü et al., 2014). The E. faecium LCW44 strain isolated from raw camel milk demonstrated a pronounced inhibitory effect against Listeria spp. and S. aureus (Vimont et al., 2017). The Lactobacillus acidophilus AA105 strain from camel milk is a potent inhibitor of Salmonella paratyphi, Shigella spp., and pathogenic E. coli (Abo-Amer, 2013), and L. brevis CM22 demonstrated inhibitory effects against Listeria spp. (Rahmeh et al., 2019). Interestingly, a recent report of Elbanna et al. (2018) showed that L. paracasei Pro4 and L. rhamnosus Pro7 isolated from fermented camel milk modulate the intestinal immunity after oral administration in mice by improving the expression of Toll-like receptor (TLR)-2, interferon (IFN)-γ, and secretory immunoglobulin A (IgA) indicating that LAB isolated from the milk of camelids can be beneficial modulators of the immune system.
In South America, the guanaco and vicuña camelids are wild animals, and alpaca and llama are domesticated species (Zarrin et al., 2020). It is estimated that there are approximately 5 million llamas in South America (FAOSTAT, 2019). Andean native communities mainly use llamas as pack animals, for meat production, and, to a lesser extent, for production of fiber and milk (Pérez et al., 2000; Larico et al., 2018). Llama meat has been characterized and was shown to be rich in iron and zinc (Polidori et al., 2007) and contain low fat and cholesterol compared with those in the meat of other livestock species (Cristofanelli et al., 2004). On the other hand, although milk yield in llamas is very low compared with that in dairy cows, llama milk has higher levels of protein, fat, and lactose than those in the cow milk; these properties suggest that llama milk can be used to develop new dairy products (Larico et al., 2018; Zarrin et al., 2020). These characteristics of llama milk suggest that it can be used as an alternative to cow milk; however, there are no in-depth studies on the biotechnological and functional potential of llama milk. Studies on the nutritional properties and impact on human health of llama milk are required to position it as a safe and healthy food that can expand globally in the future (Pauciullo and Erhardt, 2015). The composition of the microbial populations and characterization of derived strains are the important factors that should be analyzed in llama milk. These strains can be used: (a) to develop safe and varied dairy products, (b) to improve the health and productivity of llamas, and (c) to provide beneficial modulation of human health.
To the best of our knowledge, there are no published reports on the potential probiotic effects of bacteria isolated from the milk of llama. The aim this study was to isolate beneficial LAB from the llama milk and use them as potential probiotics against bacterial pathogens. LAB strains were isolated from the llama milk samples, and their functional properties were characterized in vitro based on their ability to resist gastrointestinal conditions, adhere to intestinal mucins and cells, inhibit the growth of the pathogens, and differentially modulate the TLR4-mediated innate immune responses in intestinal epithelial cells. These functional studies were complemented with a comparative genomic evaluation and in vivo studies in mice.
Five llama milk samples were collected aseptically by trained personnel of the Laboratory of Food Microbiology (Faculty of Veterinary Sciences, University of Concepción) in the Bio-Bio region of Chile and transported in cold containers. Resistance to pH 3 was used as a selection criterion to isolate LAB from llama milk as described previously (Quilodrán-Vega et al., 2016). Briefly, 150 μl of each milk sample was placed in MRS broth and incubated at 37°C at 5% CO2 for 12 h. Subsequently, 100 μl of the bacteria grown in MRS were transferred to new MRS broth adjusted to pH 3 with 5N HCl. The new MRS broth cultures were incubated for additional 3 h under the same conditions. The cultures were transferred to MRS agar, and grown colonies were subjected to the Gram staining and catalase test. Colonies of Gram-positive and catalase-negative microorganisms resistant to pH 3 were selected for subsequent studies.
The isolates confirmed as Gram-positive and catalase-negative bacilli were biochemically identified using the API 50 CH test as described elsewhere (Brolazo et al., 2011). Strains of interest were further identified using species-specific primers as described previously (Quilodrán-Vega et al., 2016). Briefly, genomic DNA extraction was performed using a ZR fungal/bacterial DNA miniprep kit (Zymo Research, CA, U.S.A., catalog no. D6005). Positive strains of the Lactobacillus genus were subjected to PCR analysis using the genera-specific primers as described previously (Quilodrán-Vega et al., 2016). Salmonella Typhimurium and L. rhamnosus CRL1505 DNA samples were used as negative and positive controls, respectively. A single bacterial strain, Ligilactobacillus salivarius TUCO-L2 (basonym: Lactobacillus salivarius TUCO-L2), showing potential probiotic properties was further identified by 16S RNA and complete genome sequencing (DDBJ/ENA/GenBank under the accession number SOPE01000000) (Albarracin et al., 2020b).
Screening for the selection of potential probiotic strains was based on resistance to NaCl and Oxgall (bile salts). LAB strains were cultivated in MRS broth containing 3, 6.5, or 9% w/v NaCl and in MRS broth containing 0.3, 0.6, 2, 3, or 5% w/v Oxgall as described previously (Quilodrán-Vega et al., 2016). Bacteria were incubated for 5 days at 37°C at 5% CO2, and 100 μl of each tube was streaked on MRS agar to test viability.
Various intestinal pathogens were used to evaluate the antagonistic effect of lactobacilli isolated from llama milk. The human intestinal pathogens Salmonella enterica ATCC 13076 and Escherichia coli ATCC 25922 were obtained from Oxoid (Argentina). The porcine pathogens enterotoxigenic E. coli TUCO-I5, enterohemorragic E. coli TUCO-I6, and Salmonella Typhimurium TUCO-I7 were obtained from the pathogen culture collection of the Laboratory of Food Microbiology (Faculty of Veterinary Sciences, University of Concepción). Escherichia coli and Salmonella strains were grown in BHI broth under the standard conditions (Quilodrán-Vega et al., 2016).
The study of inhibition of bacterial pathogens was performed as described previously (Quilodrán-Vega et al., 2016). Briefly, a 10 μl loop of a 2-day culture on MRS broth of the LAB strains was streaked on a line on MRS agar and incubated at 37°C at 5% CO2 for 24 h. After incubation, a 10 μl loop of overnight cultures from the pathogen strains was streaked perpendicular to the lactobacilli strains lines. The plates were incubated at 37°C for 48 h under aerobic conditions. The inhibition zones were measured in cm.
LAB were cultivated in MRS broth for 48 h at 37°C, and the cells were harvested by centrifugation for 10 min at 10,000 × g. The pellet was washed twice with Butterfield's buffer (pH 7.2) and resuspended in 4 ml of new MRS broth. The bacterial concentration in the new MRS broth was adjusted to 0.5 MacFarland in a DensiCHEK™ Plus instrument. The tubes were incubated for 6 or 24 h at 37°C. Then, samples were withdrawn from the top of the suspensions. Autoaggregation was calculated using the equation: autoaggregation (%) = ((A0 – At)/A0) × 100, where A0 indicates the absorbance at time 0 h and At indicates the absorbance at 6 or 24 h.
LAB suspensions for the coaggregation assay were prepared as described above. Additionally, the suspensions of pathogenic bacteria grown in BHI were prepared in a similar manner and adjusted to 0.5 MacFarland. Each LAB suspension (1 ml) was mixed with the same volume of each pathogen suspension. The mixtures were vortexed and left for gravity sedimentation. Tubes containing 2 ml of each pathogenic bacteria suspension without LAB were used as the controls. The concentration in MacFarland of the suspensions was determined after incubation at 37°C for 6 or 24 h. Coaggregation was calculated using the equation: coaggregation (%) = [(Alab + Apat)/2–A(lab+pat)]/[(Alab + Apat)/2], where lab and pat indicate the absorbance of the LAB and pathogen strains, respectively; and (lab+pat) indicates the absorbance of the mixtures.
The strain with good probiotic characteristics (L. salivarius TUCO-L2) was washed once and diluted two-fold with PBS. The bacterial suspension was dropped on a polycarbonate membrane (ADVANTEC) and filtered by vacuum filtration (Millipore). The membrane with lactobacilli on the surface was immersed in 2% (v/v) glutaraldehyde solution. After 1 h, the membrane was dehydrated by sequential immersion in 50, 60, 70, 80, 90, and 99% ethanol for 20 min at each step. The membrane was immersed in t-butyl alcohol and lyophilized. SEM was performed in an electron microscopy facility (CIME-CONICET-UNT, Tucuman, Argentina).
The PIE cell line was originally established at Tohoku University from intestinal epithelium of an unsuckled neonatal pig as described previously (Moue et al., 2008). After 3 days of culture, PIE cells form a monolayer with cobblestone- and epithelial-like morphology and close contacts between the cells. PIE cells are strongly positive for cytokeratin K8.13, a marker of porcine intestinal epithelial cells (Moue et al., 2008). PIE cells grow rapidly and are well-adapted to culture conditions even without transformation or immortalization. However, the proliferation of PIE cells diminishes after 50 passages in culture. Therefore, in the experiments, PIE cells only from passage 20 to 40 were used (Albarracin et al., 2017). DMEM medium supplemented with 10% fetal calf serum (FCS), penicillin (100 mg/ml), and streptomycin (100 U/ml) was used for maintenance of PIE cells. The cells (3.0 × 104 per well) were grown in 12 well type I collagen-coated plates at 37°C in a humidified atmosphere of 5% CO2. DMEM containing L. salivarius TUCO-L2 (5 × 107 cells/ml; 1 ml) was added to PIE cell monolayers. The cells were incubated for 48 h at 37°C at 5% CO2. PIE cells were washed with fresh medium to remove lactobacilli and stimulated with heat-stable pathogen associated molecular patterns (PAMPs) from enterotoxigenic Escherichia coli (ETEC) for 12 h to induce the activation of TLR4 as described previously (Shimazu et al., 2012; Garcia-Castillo et al., 2019). PIE cells not treated with lactobacilli and challenged with ETEC PAMPs were used as a control. PIE cells without any stimulation were used for comparison and are designated as a basal control.
The expression levels of interleukin (IL)-1β, IL-6, chemokine C-C motif ligand 8 (CCL8 or MCP2), C-X-C motif chemokine 5 (CXCL5 or ENA78), CXCL8 or IL-8, CXCL9 or MIG, CXCL10 or IP-9, and CXCL11 or IP-9 were evaluated after TLR4 activation by using RT-qPCR as detailed below. In addition, six negative regulators of TLR signaling were assayed after TLR4 activation: single immunoglobulin IL-1-related receptor (SIGIRR), Toll-interacting protein (Tollip), interleukin-1 receptor-associated kinase M (IRAK-M), ubiquitin-editing enzyme A20 (A20), B-cell lymphoma 3-encoded protein (Bcl-3), and mitogen-activated protein kinase 1 (MKP-1) (Shimazu et al., 2012; Garcia-Castillo et al., 2019).
The expression of immune factors in PIE cells was studied as described previously (Garcia-Castillo et al., 2019; Albarracin et al., 2020a). Briefly, total RNA was extracted with TRIzol reagent (Invitrogen) and its purity and quantity was analyzed by a Nano drop spectrophotometer ND-1000 UV-Vis (NanoDrop Technologies, USA). The RNA (500 ng) was used to synthesize cDNA in a thermal cycler (BIO-RAD, USA) by a Quantitect reverse transcription (RT) kit (Qiagen, Tokyo, Japan) following the manufacturer instructions. The qPCR was performed in a 7,300 real-time PCR system (Applied Biosystems, Warrington, UK) with platinum SYBR green (qPCR supermix containing uracil DNA glycosylase and 5-carboxy-X-rhodamine, Invitrogen). For PCR, 2.5 μl of cDNA was mixed with 7.5 μl of master mix that included SYBR green and forward and reverse primers (1 pmol/μl). The reaction was performed as follows: 50°C for 5 min; 95°C for 5 min; 40 cycles at 95°C for 15 s, 60°C for 30 s, and 72°C for 30 s. β-Actin was used as a housekeeping gene because of its high stability across various porcine tissues (Shimazu et al., 2012; Albarracin et al., 2017). The expression of the housekeeping gene was used to normalize the cDNA levels to account for the differences in total cDNA levels in the samples.
Porcine intestinal mucins were used to evaluate the adhesion of L. salivarius TUCO-L2. The L. salivarius FFIG79 strain highly adhesive to porcine mucin (Masumizu et al., 2019) was used for comparison. Crude mucus was scraped from porcine small intestine. Mucus was digested with 0.5 mg/ml proteinase K (TaKaRa Biotechnology, Shiga, Japan) overnight. After centrifugation (8,500 × g, 4°C, 10 min) and membrane filtration (DISMIC-25, 0.45 μm, Advantec, Tokyo, Japan), the supernatant was purified by gel filtration chromatography over a Toyopearl HW-65F column (90 × 2.6 cm; Tosoh, Tokyo, Japan) using distilled water as the mobile phase. The peptides were detected at 214 nm, and neutral sugar was measured at 490 nm using the phenol-sulfuric acid method. Fractions containing high concentrations of sugars and peptides were collected and concentrated before lyophilization. The purified soluble porcine mucins were used as the ligands for the Biacore analysis.
Biacore experiments were performed using a Biacore 1000 kit (GE Healthcare Bio-Sciences K.K.) at 25°C in HBS-EP buffer. The immobilization of purified porcine mucins on a CM5 sensor chip (GE Healthcare Bio-Sciences K.K.) was performed by the amine coupling reaction following the manufacturer's instructions. Mucins were dissolved at a concentration of 10 mg/ml in 10 mM sodium acetate buffer (pH 4.0) and immobilized using the reaction between N-hydroxysuccinimide (NHS) esters and primary amino groups present in the mucins molecules. The sensor chip was equilibrated in HBS-EP buffer.
Adhesion using Biacore 1,000 is based on the surface plasmon resonance (SPR). After washing and lyophilization, bacterial cells were suspended in HBS-EP buffer (3 mg protein/ml). Bacterial suspension was injected at a flow rate of 3 μl/min for 5 min, and the sensor chip was washed with HBS-EP buffer to remove unbound analyte and regenerated by elution with 1 M guanidine hydrochloride (GHCl) solution at a flow rate of 3 μl/min for 2 min. The resonance units (RU) were determined for 200 s after the end of sample addition. A response of 1 RU represents 1 pg protein/mm2 bound to the sensor chip surface at increasing concentrations of the analyte.
The adhesion of L. salivarius TUCO-L2 to PIE cells was assayed by using the microplate method and fluorescent bacteria. The L. salivarius FFIG58 strain highly adhesive to PIE cells (Masumizu et al., 2019) was used for comparison. Cultured lactobacilli were washed with PBS three times (6,000 rpm, 10 min). The pellet was resuspended in 1 ml of PBS, and 1 mM carboxyfluorescein diacetate (CFDA) was added for the fluorescent labeling reaction at 37°C for 1 h. Then, the bacteria were washed with PBS three times (6,000 rpm, 10 min) to remove CFDA on the bacterial surface. Fluorescent bacteria were counted by a hemocytometer.
PIE cells were seeded at 5,000 cells/well in a type I collagen-coated 96 well cell culture plate (Nippi Incorporated, Tokyo) and grown for 3 days. Cultured fluorescent lactobacilli were added to PIE cells at 100 MOI and cocultured for 48 h. After incubation, non-adherent bacteria were washed out with PBS. After lysis with 0.1 N NaOH, fluorescence was evaluated by a 2030 multilabel reader (Perkin Elmer, Madrid, Spain). Wells without PIE cells and labeled with bacteria only were used as the negative controls to determine non-specific fluorescence unrelated to specific adhesion to PIE cells.
The genome sequence of L. salivarius TUCO-L2 was published recently (Albarracin et al., 2020b). The genome sequences of other L. salivarius strains from human milk and intestine and pig and chicken intestine were downloaded from the GenBank database (https://www.ncbi.nlm.nih.gov/genome/1207) and used for comparison. Phylogenetic trees were constructed to evaluate the relationships between various microorganisms. The gene sequences were downloaded from the GenBank databases. The MUSCLE aligner (Edgar, 2004) available in the MEGAX (Kumar et al., 2018) software was used to align the gene sequences of all microbes before the construction of the phylogenetic tree according to the neighbor joining (NJ) distance algorithm (Saitou and Nei, 1987; Tamura et al., 2004) embedded in the MEGAX software. The multilocus sequence typing (MLST) analysis was used to construct the trees by the maximum likelihood method based on the sequences of the parB, rpsB, pheS, nrdB, groEL, and ftsQ genes (Harris et al., 2017; Lee et al., 2017). Heatmaps were constructed using the data plotting tools (Warnes et al., 2017) of R scripts. Pangenome analysis was performed using Roary (v. 3.6.0) (Page et al., 2015) with Prokka annotation (Seemann, 2014). The Venn diagrams were generated using InteractiVenn (Heberle et al., 2015).
Male 6-week-old Balb/c mice were obtained from CERELA (Tucuman, Argentina). The animals were housed in plastic cages at controlled room temperature (22 ± 2°C, 55 ± 2% humidity) and fed a conventional balanced diet ad libitum. Animal welfare was ensured by the investigators and special staff trained in animal care and handling at CERELA. Animal health and behavior were monitored twice a day. This study was carried out in strict accordance with the recommendations in the Guide for the Care and Use of Laboratory Animals of the Guidelines for Animal Experimentation of CERELA. The CERELA Institutional Animal Care and Use Committee prospectively approved this study under the protocol BIOT-CRL-17.
Animals were housed individually during the experiments, and the assays of the parameters were performed in the groups of 5–6 mice. L. salivarius TUCO-L2 was administered to mice for 5 consecutive days at a dose of 108 cells/mouse/day in drinking water. The dose was selected according to the preliminary experiments assessing the dose response (unpublished data). The treatment and untreated control groups were fed a conventional balanced diet ad libitum. Treated and control mice were challenged with 50 μl of 107 cells/mouse of S. typhimurium (20LD50) by oral administration (Quilodrán-Vega et al., 2016). Mice were sacrificed on day 2 after the infection. The liver and spleen of mice of various experimental groups were removed. The organs were homogenized in 0.1% peptone water, appropriately diluted, and plated in MacConkey agar. The plates were incubated at 37°C for 48 h. The results were expressed as the number (log) of CFU/g of organ weight. Bacteremia was monitored in blood samples obtained by cardiac puncture, which were plated on MacConkey agar. The results were expressed as the number (log) of CFU/ml of blood.
The concentrations of the cytokines were determined in the blood and intestinal samples of TUCO-L2-tretated and control mice as described previously (Garcia-Castillo et al., 2019). Briefly, blood samples were obtained by cardiac puncture at the end of each treatment using heparinized tubes. Intestinal fluid samples were obtained by flushing the small intestine with 5 ml of PBS, and the fluid was centrifuged (10,000 × g, 4°C for 10 min) to separate particulate material. The supernatant was stored frozen until use. Tumor necrosis factor α (TNF-α), interferon γ (IFN-γ), IL-1β, IL-6, and IL-10 concentrations in the serum and intestinal fluid were measured with commercially available enzyme-linked immunosorbent assay (ELISA) kits following the manufacturer's recommendations (R&D Systems, MN, USA).
Statistical analyses were performed using the GLM and REG procedures available in the SAS software (SAS, 1994, https://www.sas.com/en_us/legal/editorial-guidelines.html). Comparison between the mean values was performed using one-way analysis of variance and Fisher least significant difference (LSD) test. For these analyses, P < 0.05 were considered significant. For multiple comparisons between mean values, p < 0.05 was considered significant and indicated with different superscript letters (a < b < c). The Tukey-Kramer multi comparison was used.
A total of 105 different bacterial colonies were isolated from 5 samples of llama milk. The colonies included 41 strains able to grow in MRS broth at pH 3. Evaluation of these 41 LAB strains based on resistance to NaCl and bile salts and on inhibition of E. coli ATCC23922 (data not shown) resulted in the selection of four strains for additional experiments: TUCO-L1, TUCO-L2, TUCO-L3, and TUCO-L5. Two bacterial strains were Gram-positive bacilli (TUCO-L2 and TUCO-L5), and TUCO-L1 and TUCO-L3 were Gram-positive cocci. API 50 analysis and molecular biology assays demonstrated that both bacilli strains belong to the Lactobacillus genus and cocci belong to the Enterococcus genus (data not shown). The four strains had a remarkable ability to grow in the presence of all tested bile salt concentrations (Supplementary Table 1). The TUCO-L1 and TUCO-L2 strains were also resistant to all tested concentrations of NaCl; however, the growth of the TUCO-L3 and TUCO-L5 strains was inhibited by concentrations of NaCl over 6% (w/v) (Supplementary Table 1).
The inhibitory potency of the four LAB strains from llama milk against intestinal pathogens is shown in Figure 1. The four strains were able to inhibit the human intestinal pathogens S. enterica ATCC 13076 and E. coli ATCC 25922. The four strains were equally effective in the inhibition of S. enterica ATCC 13076; however, the TUCO-L5 strain showed the highest inhibition of E. coli ATCC 25922. Additionally, the four TUCO strains were able to inhibit the growth of the porcine pathogens enterotoxigenic (ETEC) E. coli TUCO-I5, enterohemorragic (EHEC) E. coli TUCO-I6, and Salmonella Typhimurium TUCO-I7 (Figure 1). The four strains were equally effective inhibitors of EHEC. The TUCO-L1 and TUCO-L5 strains had lower ability to inhibit ETEC and Salmonella Typhimurium, respectively, compared to that of the other strains isolated from the llama milk (Figure 1). Neutralization of acid did not reduce inhibition of the pathogen growth by llama milk strains indicating that other factors rather than lactic acid are responsible for the beneficial effect (data not shown).
Figure 1. Inhibition of Gram-negative bacterial pathogens by lactic acid bacteria (LAB) isolated from llama (Lama glama) milk. The antimicrobial activities of the llama milk strains of lactobacilli (TUCO-L2 and TUCO-L5) and enterococci (TUCO-L1 and TUCO-L3) were evaluated against the human intestinal pathogens Salmonella enterica ATCC 13076 and Escherichia coli ATCC 25922 and porcine pathogens enterotoxigenic E. coli TUCO-I5, enterohemorragic E. coli TUCO-I6, and Salmonella Typhimurium TUCO-I7. The results represent the data of three independent experiments. Letters indicate significant differences (P < 0.05), a < b < c.
The ability of the TUCO strains to coaggregate with intestinal pathogens was investigated. The TUCO-L2 and TUCO-L3 strains had the highest coaggregation with the tested intestinal pathogens (Figure 2). The ability of the TUCO-L2 strain to coaggregate with intestinal pathogens was substantially higher than that of other strains from llama milk at 6 h incubation. Similarly, autoaggregation of the TUCO-L2 and TUCO-L3 strains was higher than that of other strains (Figure 3A). The TUCO-L2 strain had the highest autoaggregation than that of other LAB strains at 6 h. Then, the TUCO-12 strain was used for SEM analysis. The microscopic observations demonstrated that the bacterial cells are bound by two clearly visible structures: short fibril-like structures that connect the cells to each other and the extracellular matrix that forms a cement surrounding the cells (Figure 3B). These structures may account for high aggregation of TUCO-L2 cells in the broth media and formation of rigid colonies in the agar media (Supplementary Figure 1).
Figure 2. Coaggregation of Gram-negative bacterial pathogens with lactic acid bacteria (LAB) isolated from llama (Lama glama) milk. Coaggregate of llama milk strains of lactobacilli (TUCO-L2 and TUCO-L5) and enterococci (TUCO-L1 and TUCO-L3) with the pathogens was evaluated using the human intestinal pathogens Salmonella enterica ATCC 13076 and Escherichia coli ATCC 25922 and the porcine pathogens enterotoxigenic E. coli TUCO-I5, enterohemorragic E. coli TUCO-I6, and Salmonella Typhimurium TUCO-I7 at 6 and 24 h. The results represent the data of three independent experiments. Letters indicate significant differences (P < 0.05), a < b < c.
Figure 3. Autoaggregation of lactic acid bacteria (LAB) isolated from llama (Lama glama) milk. (A) Autoaggregation at 6 and 24 h of llama milk lactobacilli (TUCO-L2 and TUCO-L5) and enterococci (TUCO-L1 and TUCO-L3). The results represent the data of three independent experiments. Letters indicate significant differences (P < 0.05), a < b < c. (B) Scanning electron microscopy (SEM) images of aggregates of Ligilactobacillus salivarius TUCO-L2 showing putative exopolysaccharide (blue arrows) and fibril-like structures (yellow arrows).
Thus, the TUCO-L2 strain was selected for subsequent experiments. The complete genome of the TUCO-L2 strain was sequenced (accession number SOPE01000000), and the bacterium was identified as Ligilactobacillus salivarius (basonym: Lactobacillus salivarius) (Zheng et al., 2020). Therefore, the strain was designated as L. salivarius TUCO-L2 (Albarracin et al., 2020b).
Then, the adhesion of L. salivarius TUCO-L2 to porcine mucins was evaluated. Porcine mucin from the small intestine was purified as described in Materials and Methods and used to evaluate the adhesion of the TUCO-L2 strain (Figure 4A). L. salivarius FFIG58 and FFIG79 isolated from the intestinal tract of wakame-fed pigs were used for comparison; these strains have been demonstrated to have low and high binding to porcine mucins, respectively (Zhou et al., 2020). L. salivarius TUCO-L2 demonstrated strong binding to porcine mucins that was not different from the binding of the FFIG79 strain (Figure 4A). Additionally, the ability of L. salivarius TUCO-L2 to adhere to porcine intestinal epithelial (PIE) cells was assessed. Comparison of the TUCO-L2 strain with the FFIG58 and FFIG79 strains, which have been demonstrated to have high and low adhesion to PIE cells, respectively (Zhou et al., 2020) indicated that L. salivarius TUCO-L2 adheres to PIE cells, and the adhesion was significantly different from that of the two control strains (Figure 4B).
Figure 4. Adhesion of Ligilactobacillus salivarius TUCO-L2 isolated from llama (Lama glama) milk to porcine mucin and epithelial cells. The adhesion of the TUCO-L2 strain to porcine mucins and porcine intestinal epithelial (PIE) cells was compared with the porcine L. salivarius strains FFIG58 and FFIG79. Letters indicate significant differences (P < 0.05), a < b < c.
The ability of L. salivarius TUCO-L2 to differentially modulate the innate immune response in PIE cells was evaluated by triggering the activation of TLR4 in lactobacilli-treated epithelial cells (Figure 5). PIE cells challenged with ETEC PAMPs in the absence of lactobacilli were used as a control. As reported previously, TLR4 activation by ETEC PAMPs resulted in an increase in the expression of proinflammatory cytokines IL-1β and IL-6 in PIE cells compared to cells that were not stimulated with ETEC PAMPs (basal group) (Shimazu et al., 2012; Garcia-Castillo et al., 2019). L. salivarius TUCO-L2 significantly increased the levels of both inflammatory cytokines compared to those in the control (Figure 5A). The expression levels of proinflammatory chemokines CCL8, CXCL5, CXCL8, CXCL9, CXCL10, and CXCL11 were increased in ETEC PAMPs-treated PIE cells after TLR4 activation. L. salivarius TUCO-L2 significantly reduced the expression of these chemokines compared to those in the ETEC PAMPs-challenged control PIE cells (Figure 5B).
Figure 5. Immunomodulatory activity of Ligilactobacillus salivarius TUCO-L2 isolated from llama (Lama glama) milk in porcine intestinal epithelial (PIE) cells. PIE cells were stimulated with the TUCO-L2 strain and then challenged with pathogen-associated molecular patterns (PAMPs) from enterotoxigenic Escherichia coli to induce the activation of Toll-like receptor (TLR)-4. The expression levels of the cytokines (A) and chemokines (B) were assayed by RT-PCR 12 h after the ETEC PAMPs challenge. Letters indicate significant differences (P < 0.05), a < b < c.
Differential modulation of the expression of the negative regulators of the TLR4 signaling pathway by L. salivarius TUCO-L2 was assessed in PIE cells (Figure 6). The treatment of PIE cells with ETEC PAMPs significantly augmented the expression of A20, Bcl-3, Tollip, and IRAK-M, while the expression levels of MKP-1 and SIGIRR were not modified compared to those in the unchallenged control cells (basal group). The treatment of PIE cells with L. salivarius TUCO-L2 significantly increased the expression of A20, IRAK-M, and MKP-1 compared to those in the ETEC PAMPs-challenged control PIE cells (Figure 6). The expression level of Bcl-3 in TUCO-L2-treated PIE cells was significantly lower than that in the ETEC PAMPs-challenged control PIE cells.
Figure 6. Immunomodulatory activity of Ligilactobacillus salivarius TUCO-L2 isolated from llama (Lama glama) milk in porcine intestinal epithelial (PIE) cells. PIE cells were stimulated with the TUCO-L2 strain and then challenged with pathogen-associated molecular patterns (PAMPs) from enterotoxigenic Escherichia coli to induce the activation of Toll-like receptor (TLR)-4. The expression levels of the negative regulators of the TLR signaling pathway were evaluated by RT-PCR 12 h after the ETEC PAMPs challenge. Letters indicate significant differences (P < 0.05), a < b < c.
The complete genome of L. salivarius TUCO-L2 was sequenced by Illumina HiSeq, annotated, and recently published (Albarracin et al., 2020b) to provide detailed characterization of the probiotic/immunobiotic properties. The genomic analysis of the TUCO-L2 strain was performed by comparison with publicly available genomes of L. salivarius strains isolated from human milk (LPM01 and CECT5713), human intestinal tract (UCC118 and REN), porcine intestine (JCM1046 and ZSL006), and chicken intestine (DJ-sa-01 and CICC23174). The general genomic features of L. salivarius strains used in the present study are summarized in the Supplementary Table 2. The TUCO-L2 draft genome sequence has an average GC content of 33%, a total estimated size of 1,600,747 bp, and 1,691 protein-coding genes. These general genomic features were similar to those of the L. salivarius strains used for comparison. However, the TUCO-L2 strain had a smaller genome size compared with that of other L. salivarius strains. Their genome size varied from 1,746,897 bp in the chicken strain CICC 23174 to 2,177,581 bp in the porcine strain ZLS006 (Supplementary Table 2). The number of protein-coding genes in the TUCO-L2 strain was among the lowest of all strains, and only the CICC 23174 strain had lower number of protein-coding genes than that in L. salivarius TUCO-L2.
Phylogenetic trees were constructed based on the sequences of the 16s rRNA genes (Figure 7A) and the maximum likelihood analysis of MLST of the sequences of the parB, rpsB, pheS, nrdB, groEL, and ftsQ genes (Figure 7B) (Harris et al., 2017; Lee et al., 2017). Both methods of analysis indicated that L. salivarius TUCO-L2 clustered separately from other evaluated strains. Notably, the MLST analysis indicated that the TUCO-L2 strain is clustered close to the two strains originally isolated from the chicken intestine (DJ-sa-01 and CICC23174).
Figure 7. Hierarchical clustering of Ligilactobacillus salivarius TUCO-L2 isolated from llama (Lama glama) milk compared with the strains of the same species isolated from different origins. (A) Phylogenetic tree constructed using 16s RNA extracted from the genome of TUCO-L2 strain and from the publicly available complete genomes of the L. salivarius strains isolated from human milk (LPM01 and CECT5713), human intestinal tract (UCC118 and REN), porcine intestine (JCM1046 and ZSL006), and chicken intestine (DJ-sa-01 and CICC23174). (B) Molecular phylogenetic analysis by maximum likelihood method of MLST of L. salivarius strains. The phylogenetic trees were constructed based on the MLST analysis by using the parB, rpsB, pheS, nrdB, groEL, and ftsQ genes present the genomes of the TUCO-L2 strain and publicly available genomes of the L. salivarius strains.
Comparison of the complete genomes of L. salivarius TUCO-L2 and the control strains revealed a core genome of 997 genes and an accessory genome of 2,891 genes (Figure 8A). The TUCO-L2 strain has 154 unique genes that are not shared with other strains. However, this number was not the highest number of unique genes since the ZLS006, DJ-sa-01, JCM1046, and REN strains had 432, 243, 194, and 192 unique genes, respectively. Unique genes of the TUCO-L2 strain included genes of the inositol catabolism pathway [methylmalonate semialdehyde dehydrogenase (iolA), 5-deoxy-glucuronate isomerase (iolB), 3D-(3,5/4)-trihydroxycyclohexane-1,2-dione hydrolase (iolD), inosose dehydratase (iolE), inositol 2-dehydrogenase/D-chiro-inositol 3-dehydrogenase (iolG), and inosose isomerase (iolI)]. The genes encoding for the iron-sulfur cluster assembly were also detected, including PaaD-like protein, cysteine desulfurase, iron-sulfur cluster assembly proteins SufB, SufE2, and SufD, and iron-sulfur cluster assembly ATPase protein SufC. Unique genes of the TUCO-L2 strain encode for a high affinity phosphate transporter and control of PHO regulon, including a phosphate ABC transporter, periplasmic phosphate-binding protein (PstS), phosphate transport ATP-binding protein (PstB), and phosphate transport system permease proteins PstA (PstA) and PstC (PstC), and components of the common pathway for the synthesis of aromatic compounds (3-dehydroquinate dehydratase I and shikimate/quinate 5-dehydrogenase I beta) (Supplementary Tables 3–6).
Figure 8. Genomic comparison of Ligilactobacillus salivarius TUCO-L2 isolated from llama (Lama glama) milk with the strains of the same species isolated from various sources. (A) Venn diagram of the number of genes in the core genome and accessory genome and unique genes in the genome of TUCO-L2 strain and in the genomes of L. salivarius strains isolated from human milk (LPM01 and CECT5713), human intestinal tract (UCC118 and REN), porcine intestine (JCM1046 and ZSL006), and chicken intestine (DJ-sa-01 and CICC23174). (B) Venn diagrams comparing L. salivarius TUCO-L2 with various strains based on the origin of the strains.
Subsequent comparison accounted for different origins of the control strains (Figure 8B). Genomic comparison of L. salivarius TUCO-L2 with the human milk isolates revealed that the llama milk strain shares a total of 1,130 genes with the CECT 5713 and LPM01 strains and has 303 unique genes. Comparison with the CECT 5713 and LPM01 strains isolated from human milk indicated that unique genes of the L. salivarius TUCO-L2 genome (Supplementary Table 3) include genes of the accessory SecA2-SecY2 system (secA2, secY2, asp1, asp2, and asp3), exopolysaccharide (EPS) biosynthesis (cap8A, epsE1, epsE2, epsJ1-J5, and epsL), glycosyltransferases (gtf1, gtf2, gtfC, wfgD, wbbI2, and wbbI3), glucosidases (bglH1, bglH2, and bglK), and choloylglycine hydrolase (cbh1). Similar results were obtained by comparison of the L. salivarius TUCO-L2 genome with the genomes of the UCC118 and REN strains with the exception of the cbh1 gene that was also detected in the human intestinal strains (Supplementary Table 4).
Comparison with the JCM1046 and ZLS006 strains isolated from porcine intestine (Supplementary Table 5) and the DJ-sa-01 and CICC23174 strains isolated from chicken intestine (Supplementary Table 6) identified the following unique genes in the L. salivarius TUCO-L2 genome: EPS biosynthesis (cap8A, epsE1, epsE2, epsJ1-J5, and epsL), glycosyltransferases (gtf1, gtf2, gtfC, wfgD, wbbI2, and wbbI3), and glucosidases (bglH1, bglH2, and bglK). In contrast to human strains, the genes of the accessory SecA2-SecY2 system (secA2, secY2, asp1, asp2, and asp3) were detected in all L. salivarius strains of animal origin. Notably, the cbh1 gene of the TUCO-L2 strain was not detected in the genomes of the strains isolated from chicken intestine.
Considering that some of the genes specific for L. salivarius TUCO-L2 are involved in EPS biosynthesis, the EPS-related genes in the genomes of the TUCO-L2 and L. salivarius strains were compared. The EPS clusters described in the L. salivarius strains UCC118 (Harris et al., 2017) and JCM1046 (Raftis et al., 2011) were used as a reference. Two EPS gene clusters were detected in L. salivarius UCC118, including EPS cluster 1 containing 20 genes and EPS cluster 2 containing 27 genes, and a single EPS gene cluster containing 28 genes was detected in L. salivarius JCM1046 (Figure 9A). The EPS cluster of the JCM1046 strain was designated as EPS cluster 3 in this study. None of the genes of EPS cluster 1 were detected in the genome of L. salivarius TUCO-L2. On the other hand, the conserved genes of EPS clusters 2 and 3 (Raftis et al., 2011; Harris et al., 2017) were detected in the TUCO-L2 strain, including undecaprenyl-phosphate β-glucose phosphotransferase, transcriptional regulator LytR, a phosphotyrosine protein phosphatase, a tyrosine protein kinase, a chain length regulator, β-N-acetylhexosaminidase, dTDP-4-dehydrorhamnose reductase, dTDP-glucose 4,6-dehydratase, dTDP-4-dehydrorhamnose 3,5-epimerase, and glucose-1-phosphate thymidylyltransferase (Figure 9A). The gene LSL_1549 (glycosyltransferase) and the genes LSL_1555 to LSL_1565 mainly corresponding to glycosyltransferases and transposases were not detected in the genome of the TUCO-L2 strain. Similar results were obtained when EPS cluster 3 was used to analyze the TUCO-L2 genome. These results were anticipated since EPS clusters 2 and 3 have substantial similarities (Raftis et al., 2011; Harris et al., 2017). Interestingly, the genes LSJ_1604c, LSJ_1606c, and LSJ_1633c that encode for glycosyltransferases in EPS cluster 3 were not detected in the TUCO-L2 genome. Analysis of the conserved genes in EPS clusters 2 and 3 identified 12 EPS core genes that were used for subsequent analysis of EPS in all genomes of the L. salivarius strains. The phylogenetic tree constructed based on the sequences of 12 EPS core genes demonstrated that the TUCO-L2 strain is clustered separately from other L. salivarius strains (Figure 9B).
Figure 9. Genomic comparison of the exopolysaccharides (EPS) cluster of Ligilactobacillus salivarius TUCO-L2 isolated from llama (Lama glama) milk with that of the strains of the same species. (A) The EPS clusters from L. salivarius UCC118 (EPS1: from LSL_077 to LSL_097; EPS2: from LSL_1547 to LSL_1574) and JCM1046 (EPS3: from LSJ_1603c to LSJ_1633c) were used for comparison. (B) Phylogenetic tree was constructed by using the sequences of twelve conserved EPS genes shared by the strains isolated from human milk (LPM01 and CECT5713), human intestinal tract (UCC118 and REN), porcine intestine (JCM1046 and ZSL006), chicken intestine (DJ-sa-01 and CICC23174), and llama milk (TUCO-L2).
Various types of glycosyltransferases encoded by the genomes of EPS-producing bacteria can modify the structure of the EPS molecule and consequently change its functionality. Then, the abundance of the genes encoding for glycosyltransferase families in the TUCO-L2 strain was evaluated and compared with that in the other eight L. salivarius strains (Figure 10A). Interestingly, the results of the clustering analysis accounting for the number and type of glycosyltransferases showed that the TUCO-L2 strain is clustered separately from most of the other strains and is close only to the porcine intestinal JCM1046 strain. Comparison of glycosylhydrolases in the genomes of the L. salivarius strains (Figure 10B) indicated that the TUCO-L2 strain is clustered separately from most of the other strains and is close only to the chicken intestine CICC23174 strain. Notably, L. salivarius TUCO-L2 had significantly higher number of glycosylhydrolases of the GH1 and GH70 families.
Figure 10. Genomic comparison of glycosyltransferases and glycosylhydrolases of Ligilactobacillus salivarius TUCO-L2 isolated from llama (Lama glama) milk with those of the strains of the same species isolated from different origins. Numbers of glycosyltransferases (A) and glycosylhydrolases (B) identified in the genomes of TUCO-L2 strain and in the genomes of L. salivarius strains isolated from human milk (LPM01 and CECT5713), human intestinal tract (UCC118 and REN), porcine intestine (JCM1046 and ZSL006), and chicken intestine (DJ-sa-01 and CICC23174). Heatmaps were constructed based on the numbers of glycosyltransferases or glycosylhydrolases in each family.
The whole genome sequence of L. salivarius TUCO-L2 was analyzed to identify antimicrobial substance(s) responsible for the antipathogenic effect demonstrated in the present study. Therefore, the BAGEL 4 platform and blastp were used to search for bacteriocins in the TUCO-L2 genome. The analysis indicated the absence of any similarity to known bacteriocin genes. Thus, the whole genome sequence of TUCO-L2 was annotated to identify two genes encoding for a bacteriocin-prepeptide or an inducing factor for bacteriocin synthesis and bacteriocin immunity indicating the presence of a novel bacteriocin in this strain from llama milk (Supplementary Table 7).
Comparative genomic analysis was used to characterize the adhesion factors of L. salivarius TUCO-L2 by investigating several proteins and systems that were described to be involved in the adhesion of lactobacilli to the intestinal mucosa. Several mucus-binding proteins (MucBP) have been identified in lactic acid bacteria and are associated with their ability to colonize the gastrointestinal tract (Latousakis and Juge, 2018). Then, we searched for MucBP genes in the genome of L. salivarius TUCO-L2 (Figure 11). As described previously (Lee et al., 2017), a common mucus-binding protein (designated here as MucBP1) was detected in all L. salivarius strains, including the TUCO-L2 strain. Four MucBP were detected in the genomes of the tested L. salivarius strains, and only a single MucBP gene WP_087118522.1 (designated here as MucBP2) was detected in the genome of the TUCO-L-2 strain (Figure 11A). This protein is similar but not identical to MucBP of the LPM01, UCC118, DJ-sa-01, JCM1046, and ZLS006 strains (Figure 11B).
Figure 11. Genomic comparison of the mucus-binding proteins (MucBPs) of Ligilactobacillus salivarius TUCO-L2 isolated from llama (Lama glama) milk with the strains of the same species. (A) MucPBs genes of the TUCO-L2 strain were compared with those of the L. salivarius strains isolated from human milk (LPM01 and CECT5713), human intestinal tract (UCC118 and REN), porcine intestine (JCM1046 and ZSL006), and chicken intestine (DJ-sa-01 and CICC23174). (B) Functional domains present in MucBP2 of L. salivarius TUCO-L2 and compared with those of the proteins encoded by the genomes of the JCM1046 and LPM01 strains.
Our data indicate that similar to other L. salivarius strains from animals, the TUCO-L2 strain possesses the genes for the accessory Sec system. The SecA2-SecY2 secretion system is associated with adhesion of certain lactobacilli strains to mucosal tissues (Frese et al., 2013; De Boeck et al., 2020). Then, the presence of this cluster was investigated by manual search for the genes encoding for motor protein SecA2, membrane translocation complex SecY2, chaperones Asp1-3, and glycosyltransferases GtfA and GtfB. Complete cluster was detected in the genome of L. salivarius TUCO-L2 and in the genomes of the strains isolated from animal intestine (Figure 12A). Moreover, in agreement with our previous results (Supplementary Tables 3, 4) and the data of other authors (Lee et al., 2017), the SecA2-SecY2 cluster was not detected in the human-related L. salivarius strains LPM01, CECT5713, UCC118, and REN that were used as a reference in the present study (Figure 12A). Notably, the secA2, secY2, and gtfB genes of the TUCO-L2 strain showed 99.8, 100, and 97.5% homology, respectively, with the similar genes of the porcine JCM1046 strain (Supplementary Figure 3). Additionally, the asp1 and asp3 genes showed 98.7% homology with the similar genes in the chicken DJ-sa-01 strain. Although all genomes of the L. salivarius strains of animal origin contained the genes of the SecA2-SecY2 system, our results of evaluation of the phylogenetic clustering based on the nucleotide sequences of the conserved secA2, secY2, asp1, asp2, asp3, gtfA, and gtfB genes indicate some degree of divergence (Figure 12B).
Figure 12. Genomic comparison of the SeA2-SecY2 accessory secretion system of Ligilactobacillus salivarius TUCO-L2 isolated from llama (Lama glama) milk with the strains of the same species. (A) The SecA2-SecY2 cluster of the TUCO-L2 strain compared with that of the L. salivarius strains isolated from human milk (LPM01 and CECT5713), human intestinal tract (UCC118 and REN), porcine intestine (JCM1046 and ZSL006), and chicken intestine (DJ-sa-01 and CICC23174). (B) Phylogenetic tree was constructed by using the sequences of the secA2, secY2, asp1, asp2, asp3, gtfA, and gtfB genes shared by the strains of animal origin.
Then, the presence of accessory Sec-dependent serine-rich glycoprotein adhesion proteins or srr adhesins was assessed within the SecA2-SecY2 system cluster of the TUCO-L2 strain. We detected the presence of two partial sequences of srr proteins: WP_134354846.1 and WP_134354365.1. Both sequences contained the KxYKxGKxW signal peptide, N-terminal serine-rich repeat adhesion glycoprotein AST domain, and partial regions of serine-rich repeats (data not shown). The blast analysis of these two sequences revealed a 45.6% identity with a 59% query cover suggesting that the sequences represent two different srr adhesins. These putative adhesins WP_134354846.1 and WP_134354365.1 were designated srr1 and srr2, respectively. Unfortunately, due to the fragmentation of the contigs, we are unable to identify the C terminal regions of these two putative adhesins.
Pili are involved in the intestinal colonization of lactobacilli associated with probiotic effects (Kankainen et al., 2009). Previous genomic studies by Harris et al. (2017) showed that only 5 of 43 L. salivarius genomes contained the genes encoding for extra sortase A, sortase C, and putative pilin subunits. These strains include L. salivarius JCM1047, DSM20555, ATCC11741, gul1, and gul2. Additional search of the NCBI genome bank for other L. salivarius strains with a pili operon detected this cluster only in the genome of L. salivarius A3iob originally isolated from the bee intestine (Audisio et al., 2018). Then, the pilus operon of these strains was compared with that of L. salivarius TUCO-L2 (Supplementary Figure 3). The genes encoding for extra sortase A, sortase C, or pilin subunits were not detected in the genome of the TUCO-L2 strain.
The in vitro and in silico data indicated the beneficial effects of the TUCO-L2 strain in the protection against intestinal pathogens. Thus, we performed in vivo studies to demonstrate the probiotic potential of this strain. The ability of L. salivarius TUCO-L2 to reduce Salmonella infection was studied in a mouse model (Figure 13). The TUCO-L2 strain was administered to mice for 5 consecutive days at a dose of 108 cells/mouse/day in drinking water, and the animals were orally challenged with pathogenic Salmonella Typhimurium as described previously (Quilodrán-Vega et al., 2016). The administration of L. salivarius TUCO-L2 induced a significant decrease in the number of Salmonella in the liver and spleen of treated mice compared to that in the control animals (Figure 13). Moreover, the pathogen was not detected in the blood of animals treated with the TUCO-L2 strain (Figure 13).
Figure 13. The effect of Ligilactobacillus salivarius TUCO-L2 isolated from llama (Lama glama) milk on the resistance of adult mice to Salmonella infection. L. salivarius TUCO-L2 was administered to male 6-week-old Balb/c mice for 5 consecutive days at a dose of 108 cells/mouse/day in drinking water. Untreated mice were used as a control. TUCO-L2-treated and control mice were challenged by oral administration of 107 cells/mouse of S. typhimurium (20LD50) on day 6. Mice were sacrificed on day 2 after the infection, and the bacterial pathogen counts were determined in the liver, spleen, and blood of mice. Untreated and uninfected mice were used for comparison (basal group). Each parameter was assayed in 5–6 mice per group. Letters indicate significant differences (P < 0.05), a < b < c.
The levels of the blood and intestinal cytokines were evaluated after the challenge with Salmonella in untreated and TUCO-L2-treated mice. The challenge with the intestinal pathogen significantly increased the levels of all tested cytokines in the intestinal tract (Figure 14) and blood (Supplementary Figure 4). The intestinal levels of TNF-α, IL-1β, and IL-6 were increased in both groups after the challenge with Salmonella; however, the mice treated with L. salivarius TUCO-L2 had significantly lower levels of TNF-α and higher levels of IL-1β and IL-6 compared to those in the control animals (Figure 14). Similar results were obtained when the blood levels of TNF-α, IL-1β, and IL-6 were assayed (Supplementary Figure 4). The treatment of mice with the TUCO-L2 strain increased the levels of IFN-γ and IL-10 in the intestinal tract (Figure 15) and blood (Supplementary Figure 4) compared to those in the control groups after the challenge with Salmonella.
Figure 14. The effect of Ligilactobacillus salivarius TUCO-L2 isolated from llama (Lama glama) milk on the intestinal immune response of adult mice to Salmonella infection. L. salivarius TUCO-L2 was administered to male 6-week-old Balb/c mice for 5 consecutive days at a dose of 108 cells/mouse/day in drinking water. Untreated mice were used as a control. TUCO-L2-treated and control mice were challenged by oral administration of 107 cells/mouse of S. typhimurium (20LD50) on day 6. Mice were sacrificed on day 2 after the infection, and the levels of intestinal TNF-α, IFN-γ, IL-1β, IL-6, and IL-10 were measured by ELISA. Untreated and uninfected mice were used for comparison (basal group). Each parameter was assayed in 5–6 mice per group. Letters indicate significant differences (P < 0.05), a < b < c.
Figure 15. Proposed mechanism of the immunomodulatory activity of Ligilactobacillus salivarius TUCO-L2 isolated from llama (Lama glama) milk in porcine intestinal epithelial (PIE) cells. PIE cells were stimulated with the TUCO-L2 strain and then challenged with pathogen-associated molecular patterns (PAMPs) from enterotoxigenic Escherichia coli to induce the activation of Toll-like receptor (TLR)-4 resulting in differential expression of inflammatory cytokines and chemokines compared to that in ETEC PAMPs-challenged control cells. The immunomodulatory effect of L. salivarius TUCO-L2 is mediated by the modulation of the negative regulators of the TLR signaling pathway.
To the best of our knowledge, this is the first report describing the isolation of LAB from the milk of Lama glama. We showed that llama milk contains a population of LAB, including lactobacilli and cocci, similar to the milk of other camelids (Khedid et al., 2009; Dong et al., 2012; Rahmeh et al., 2019; Zhao et al., 2020). Studies of cultivable LAB from raw dromedary milk demonstrated the presence of L. lactis (17.5%), S. thermophilus (9.2%), and lactobacilli (20.8%) (Khedid et al., 2009) or E. faecium (20.7%), L. lactis (17.2%), and lactobacilli (12%) (Rahmeh et al., 2019). Similarly, we observed predominance of Gram-positive cocci in the bacterial isolates from llama milk samples with a minor population of lactobacilli (data not shown). Additional studies of the microbial composition of llama milk in a greater number of samples using a combination of culture-dependent and culture-independent techniques will enable more accurate characterization of the microbial populations present in this particular ecological niche. Although the results of the present study cannot be used to draw definitive conclusions about the microbiota of llama milk, we were able to isolate strains with considerable probiotic potential. One of the isolated strains, L. salivarius TUCO-L2, demonstrated several characteristics that would allow the use of this strain as a probiotic against intestinal infections. Our in vitro, in vivo, and in silico results indicate that L. salivarius TUCO-L2 can (a) resist adverse gastrointestinal conditions, (b) adhere to the intestinal mucosa, (c) antagonize intestinal bacterial pathogens, and (d) modulate the intestinal immune response.
a) L. salivarius TUCO-L2 resists adverse gastrointestinal conditions. Probiotic strains should be able to overcome extremely low pH of the gastric juice and the detergent effect of the bile salts to reach the site of action in a viable physiological state. Our functional experiments demonstrated that L. salivarius TUCO-L2 has these characteristics. Moreover, bioinformatics investigation detected two choloylglycine hydrolase genes in the genome of the TUCO-L2 strain, including cbh1 that was not detected in the genomes of L. salivarius strains isolated from human milk. Interestingly, L. salivarius strains isolated from the intestinal tract of humans and chickens have one or two choloylglycine hydrolase genes, while most of the isolates from the porcine intestinal mucosa have three choloylglycine hydrolase genes (Lee et al., 2017). This difference has been associated with required higher resistance of the porcine strains to the toxic effect of bile salts since it was demonstrated that pigs secrete more bile per day compared to humans (Boyer, 2013). Our results indicate that the TUCO-L2 strain has higher similarity to the human and chicken intestinal strains than to the porcine strains with regard to its genetic resistance to bile salts. Our results suggest that the TUCO-L2 strain should be able to have probiotic effects after in vivo administration due to the presence of the choloylglycine hydrolase genes and ability to resist bile salts. This hypothesis is supported by a recent study that demonstrated that oral administration of L. salivarius FXJCJ7-2, HN26-4, or NT4-8 in mice protected against intestinal inflammatory damage induce by LPS administration (Zhai et al., 2020). These three L. salivarius strains improved the barrier function of the gut, reduced the intestinal histological alterations, and differentially modulated the Treg/Th17 balance in mice; however, the FXJCJ7-2 strain was more efficient in inducing the protective effect against LPS challenge. Notably, genomic comparison of the FXJCJ7-2, HN26-4, and NT4-8 strains demonstrated that L. salivarius FXJCJ7-2 has 19 unique genes that are not shared with the NT4-8 and HN26-4 strains. Among these genes, researchers detected several genes associated to the resistance to gastrointestinal tract conditions including those related to selenocysteine synthesis and transportation as well as to the production of the osmolyte proline (Zhai et al., 2020), which have been associated to the protection against bile salt actions and environmental stress (Wang et al., 2015).
Genomic comparison of the TUCO-L2 strain with L. salivarius from various host types and niches indicated that the strain from llama milk has genes that are not detected in the genomes of bacteria from human, porcine, or chicken origin. Unique genes of the TUCO-L2 strain include the genes encoding for the iron-sulfur cluster assembly and high affinity phosphate transporter and control of the PHO regulon. The PHO regulon is involved in the uptake of inorganic phosphate and is controlled by a two-component signal transduction system. Studies in E. coli demonstrated that this regulon is important for phosphate uptake and influences the genes involved in stress responses (Santos-Beneit, 2015). Studies in L. casei BL23 showed that the inactivation of the two-component signal transduction system of the PHO regulon results in a decrease in growth at pH 3 (Alcántara et al., 2011). On the other hand, LAB are presumed to be a rare bacterial group that has no iron requirement. However, the sequencing of the complete genomes of several LAB demonstrated that certain species harbor genes encoding for the components involved in iron transport (Makarova et al., 2006). The iron-sulfur cluster system of E. coli is activated under oxidative stress, i.e., in the presence of H2O2 (Outten et al., 2004). The sensitivity of the iron-sulfur cluster system to oxygen allows the system to act as an environmental sensor that conditions the responses of the bacteria. This system was suggested to be an important mechanism of resistance to oxidative stress in lactobacilli (Martinez et al., 2008). The ability to sense and respond to extracellular stresses is essential for bacterial survival under adverse conditions, such as low pH or high H2O2 concentrations; hence, the presence of these gene clusters in L. salivarius TUCO-L2 may be advantageous because of enhanced colonization of the mucosal surfaces or resistance to certain biotechnological processes used during food manufacturing.
b) L. salivarius TUCO-L2 adheres to the intestinal mucosa. Colonization of the gastrointestinal tract of the host is considered one of the most important properties of probiotic lactobacilli. In the present study, the results obtained using a porcine model host demonstrated that L. salivarius TUCO-L2 can adhere to porcine mucins and PIE cells in a manner similar to that of L. salivarius strains originally isolated from the porcine gastrointestinal tract (Masumizu et al., 2019). Furthermore, the genomic analysis of the TUCO-L2 strain identified two types of molecules that may be involved in adhesion: MucBP and srr adhesins. MucBPs were detected in LAB colonizing the gastrointestinal tract (Latousakis and Juge, 2018). These proteins contain an N-terminal secretion signal peptide YSIRK and a C-terminal LPxTG anchoring motif. Variable number of mub repeats in the center of the molecule mediate the binding to mucin glycans due to interactions with terminal sialic acid (Etzold et al., 2014; Gunning et al., 2016). MucBP are shaped as fiber-like structures of variable length that form appendices similar to pili (Etzold and Juge, 2014). The genomes of Lactobacillus species can harbor one or more types of MucBPs (Latousakis and Juge, 2018). Up to seven different MucBP orthologs were detected in the pangenome of L. salivarius (Lee et al., 2017). The study analyzed the genomes of L. salivarius strains isolated from the intestine of human, pigs, and chickens and identified a common MucBP (designated here as MucBP1) in all strains independently of the host origin. The results of the present study indicate that the genome of the L. salivarius strain isolated from the llama milk also contains this common MucBP1. Additionally, L. salivarius TUCO-L2 has a second MucBP of approximately 2,500 amino acids (designated here as MucBP2) that was also detected in the genomes of the LPM01, UCC118, DJ-sa-01, JCM1046, and ZLS006 strains.
On the other hand, the SecA2-SecY2 secretion system is associated with adherence of certain lactobacilli strains to mucosal tissues (Frese et al., 2013; De Boeck et al., 2020). This system facilitates the glycosylation of srr proteins and export of glycosylated adhesins that are involved in adhesion to the surface of the host cells (Feltcher and Braunstein, 2012; Bensing et al., 2014; Wegmann et al., 2015). Previous studies identified the genes encoding for the SecA2-SecY2 system in L. salivarius strains isolated from pig and chicken intestines (Lee et al., 2017). However, the analysis of the associated srr proteins did not detect any known adhesin-associated binding domain. In the present study, two srr proteins associated with the SecA2-SecY2 system of L. salivarius TUCO-L2 were incompletely characterized due to limitations in contig fragmentation.
Most bacteria with the SecA2-SecY2 systems produce a unique srr glycoprotein (Frese et al., 2013; De Boeck et al., 2020). However, certain Gram-positive bacteria can produce two or three srr proteins associated with the SecA2-SecY2 system. Human commensal bacterium Streptococcus salivarius JIM8777, which is an efficient colonizer of the oral and intestinal mucosa, expresses three non-homologous glycosylated surface proteins that have characteristics of srr adhesins and are secreted through the accessory SecA2-SecY2 system (Couvigny et al., 2017, 2018). Electron microscopy detected fibril-like structures partially covering the surface of JIM8777, which were eliminated from the bacterial surface in the secA2 mutants. Moreover, the adhesive abilities of S. salivarius were partly related to the presence of cell wall-associated fibril-like structures since the secA2 mutants had less efficient adhesion to epithelial cells or proteins of the extracellular matrix (Couvigny et al., 2017, 2018). The srr proteins mediate several interactions of S. salivarius with the environment and have thus emerged as the crucial host attachment factors involved in the colonization of mucosal surfaces by this commensal bacterium. Electron microscopy analysis of the TUCO-L2 strain demonstrated the presence of fibrin-like structures on bacterial surface; however, the density of the structures was significantly lower than that reported in S. salivarius (Couvigny et al., 2017, 2018). It is tempting to speculate that two srr proteins may have a similar function in L. salivarius TUCO-L2 that enables colonization of the bacteria in various mucosal tissues in llamas. Additional studies evaluating the functional properties of the putative adhesins srr1 and srr2 in the TUCO-L2 strain in the context of adhesion to mucins and PIE cells may be able to determine whether these proteins are involved in the adhesion of the L. salivarius strain isolated from the llama milk.
c) L. salivarius TUCO-L2 antagonizes intestinal bacterial pathogens. The antimicrobial effects against the major gastric and enteric bacterial pathogens are one of the most desirable properties for a probiotic strain (Liévin-Le Moal and Servin, 2014). Investigation of the antibacterial activities of L. salivarius TUCO-L2 demonstrated that this strain inhibits the growth of pathogenic Gram-negative bacteria of human and porcine origin. The antimicrobial activities of LAB have been associated with several molecules, including lactic acid and bacteriocin or non-ribosomal peptides (Liévin-Le Moal and Servin, 2014). Neutralization of the TUCO-L2 supernatant eliminated lactic acid responsible for pathogen inhibition. Thus, in silico analysis was performed by using Bagel 4, blastp, and the gene annotation RAST platform to identify antibacterial substances produced by the TUCO-L2 strain. In silico analysis detected the presence of bacteriocin-related immunity genes possibly related to a prepeptide or a synthesis-inducing factor. Interestingly, we were unable to detect similarities of these molecules with any know bacteriocin by blastp (peptide sequences) or Bagel 4 (gene sequences) comparison. This result suggests the presence of a new bacteriocin synthetized by L. salivarius TUCO-L2 strain, which has an anti-Gram-negative effect. These findings strongly encourage further studies aimed to purify, sequence, and characterize this bacteriocin.
Probiotics strains with good adhesion to intestinal epithelial cells and mucins can block the adherence of the pathogens by competition for the host cell binding sites (Monteagudo-Mera et al., 2019). This transient colonization of probiotic bacteria can lead to competitive exclusion of the pathogens. Adhesion includes attachment of the bacterial cells to the host cells and to other bacterial cells of different species (coaggregation) or same species (autoaggregation). Moreover, multiple studies have shown that a potential protective role of probiotic LAB is mediated by binding of the pathogens into coaggregates thus inhibiting the biofilm formation that is frequently involved in infections (reviewed in Monteagudo-Mera et al., 2019). The results of this study indicate that L. salivarius TUCO-L2 binds intestinal epithelial cells and mucins and is capable of autoaggregation and coaggregation with intestinal pathogens. These data suggest that this strain can potentially promote competitive exclusion and prevent the biofilm formation by the pathogens in the intestinal mucosa.
The colonization of the intestinal surface and production of a bacteriocin by L. salivarius TUCO-L2 may help to avoid the colonization by enteropathogens. These antimicrobial effects of probiotic bacteria are unlikely to have a deleterious effect on the commensal intestinal bacteria (reviewed in Monteagudo-Mera et al., 2019). Our results confirm this assertion since we did not detect any signs of intestinal dysbiosis when the TUCO-L2 strain was administered to mice.
d) L. salivarius TUCO-L2 modulates the intestinal immune response. In addition to adherence to PIE cells, our data indicate that L. salivarius TUCO-L2 differentially modulates the innate immune response triggered by TLR4 activation in this cell line (Figure 15). We have previously used the PIE cell line to study the influence of immunomodulatory probiotic (immunobiotic) lactobacilli on the TLR4-mediated immune response induced by ETEC PAMPs. We demonstrated that the challenge of PIE cells with ETEC PAMPs activates the MAPK and NF-kB signaling pathways leading to the expression of inflammatory cytokines, including IL-1β and IL-6 (Shimazu et al., 2012; Wachi et al., 2014; Garcia-Castillo et al., 2019), and several chemokines, including CCL8, CXCL5, CXCL8, CXCL9, CXCL10, and CXCL11 (Kobayashi et al., 2016). Then, these cytokines and chemokines were assayed to evaluate the potential immunomodulatory properties of the TUCO-L2 strain from llama milk. L. salivarius TUCO-L2 increased the expression of IL-1β and IL-6 and reduced the expression of all tested chemokines compared to those in the control cells (Figure 15). Previously, we demonstrated that prestimulation of PIE cells with the immunobiotic strain Lactobacillus jensenii TL2937 differentially modulated the expression of inflammatory factors produced in response to ETEC PAMPs challenge. The TL2937 strain increased the expression of IL-1β and significantly reduced the expression levels of IL-6, CCL8, CXCL5, CXCL8, CXCL9, CXCL10, and CXCL11 (Kobayashi et al., 2016). This strong anti-inflammatory effect was related to the upregulation of the negative regulators MKP-1, A20, and Bcl-3 in PIE cells (Shimazu et al., 2012). Moreover, L. jensenii TL2937 efficiently reduced intestinal inflammation associated with weaning in piglets (Suda et al., 2014). We also reported that immunobiotic Limosilactobacillus fermentum UCO-979c (basonym: Lactobacillus fermentum UCO-979c) significantly reduced the levels of CXCL8, CXCL9, CXCL10, and CXCL11 and increased the expression levels of IL-6 and CCL8 in PIE cells challenged with ETEC PAMPs (Garcia-Castillo et al., 2019). This mixed stimulant/anti-inflammatory effect induced by L. fermentum UCO-979c was associated with a reduction in the expression of Tollip and MKP-1 and upregulation of SIGIRR and Bcl-3 in ETEC PAPMs-challenged PIE cells. The UCO-979c strain was able to protect against intestinal pathogens in vitro and in vivo (Garcia-Castillo et al., 2019). The results of this work indicated that L. salivarius TUCO-L2 has a mixed stimulant/anti-inflammatory effect similar to the UCO-979c strain. In fact, the analysis of the expression of the negative regulators of the TRL4 signaling pathway in PIE cells indicated that the TUCO-L2 strain enhances the expression of IRAK-M, MKP-1, and A20 and reduces the expression of Bcl-3 in PIE cells. Moreover, in vivo experiments in mice clearly demonstrated that the TUCO-L2 strain increases the resistance to Salmonella infection by differentially modulating the immune response. L. salivarius TUCO-L2 demonstrated a mixed stimulant/anti-inflammatory effect in vivo because it reduced the levels of TNF-α and increased the levels of IL-1β, IL-6, IFN-γ, and IL-10 in the intestine and blood of treated animals compared to those in the control mice.
The intestinal mucosa needs to maintain balanced TLR activation to protect against invading pathogens and avoid uncontrolled inflammatory responses that can lead to tissue damage (Allaire et al., 2018). Appropriate balance of inflammatory cytokines and chemokines is necessary to efficiently manifest this function. The changes in the cytokine profiles induced by L. salivarius TUCO-L2 in PIE cells after the stimulation with ETEC PAPMs and in mice after the challenge with Salmonella indicate that this strain positively influences the intestinal immune response triggered by Gram-negative pathogens. On the one hand, reduced chemokines expression and increased levels of the immunoregulatory cytokine IL-10 may account for the effects of the TUCO-L2 strain on the regulation of the activation and recruitment of inflammatory cells, such as neutrophils, thus limiting the inflammatory damage in the intestinal mucosa. On the other hand, an increase in IL-1β and IL-6 may help to eliminate the pathogens by modulating the effector functions of T cells (Siegmund et al., 2001; Kuhn et al., 2018). Activation of the inflammasome in intestinal epithelial cells is involved in the production of the active form of IL-1β, which collaborate in the activation and differentiation of T cells that produce IL-17 or IFN-γ. Additionally, IL-6 in the gut promotes T cell expansion and stimulates Th1 cell-mediated inflammation. Then, it is tempting to speculate that differential expression of IL-1β and IL-6 induced by the TUCO-L2 strain in intestinal epithelial cells favors efficient development of functional Th1. This hypothesis is in agreement with an increase in the levels of IFN-γ detected in mice treated with L. salivarius TUCO-L2 and challenged with Salmonella. Additional studies are needed to characterize the influence of the TUCO-L2 strain on intestinal immunity, including qualitative and quantitative evaluation of antigen-presenting cells, effector T cells, and Treg cells.
In addition to the effect on intestinal immune cell populations, it would be of interest to identify bacterial molecules of the TUCO-L2 strain that are involved in molecular interaction with the immune system. The results of electron microscopy analysis in the present study demonstrated that L. salivarius TUCO-L2 can produce EPS. Genomic analysis was used to characterize EPS of the llama milk strain. Genes of EPS cluster 1 were not detected in the genome of L. salivarius TUCO-L2. This result is in agreement with previous genomic studies of Harris et al. (2017) that demonstrated that EPS cluster 1 is mainly detected in L. salivarius strains of human origin suggesting that this gene cluster encodes a trait involved in adaptation to the human gastrointestinal tract. All L. salivarius strains investigated in the present study, including the TUCO-L2 strain, contained genes of EPS clusters 2 and 3; however, evaluation of the phylogenetic clustering based in the nucleotide sequences of 12 conserved genes indicated a certain divergence. These results are in agreement with previous genomics studies of Lee et al. (2017) that used 24 conserved EPS genes to evaluate the differences between L. salivarius isolated from humans, pigs, and chickens and that detected divergence associated with the hosts. The study suggested that the point mutations in the EPS genes rather than gene acquisition/loss may explain the production of different EPS by L. salivarius strains. Moreover, Harris et al. (2017) demonstrated variable abundance of the genes of glycosyltransferase families in 42 L. salivarius strains emphasizing that strain-specific sets of glycosyltransferases may be involved in the generation of variable structures of EPS associated with different interaction with abiotic and biotic environmental factors. Previous findings and genomic analysis performed in the present study suggest that L. salivarius TUCO-L2 may produce an EPS with unique characteristics. Further characterization of the TUCO-L2 EPS may be able to determine its role in the probiotic or immunobiotic properties of this strain considering that EPS of some lactobacilli are the key molecules associated with bacterial adhesion (Živković et al., 2016) or immunomodulatory effects (Wachi et al., 2014; Kanmani et al., 2018a,b).
In conclusion, this is the first report on the isolation and characterization of a potential probiotic/immunobiotic strain from llama milk. In vitro functional studies complemented with genomic analysis and in vivo studies in mice demonstrated that L. salivarius TUCO-L2 has several characteristics of probiotic strains including resistance to pH and high bile salt concentrations, antimicrobial activity against Gram-negative intestinal pathogens, and adherence to mucins and epithelial cells. Moreover, similar to other immunobiotic strains, L. salivarius TUCO-L2 differentially modulates the innate immune response triggered by TLR4 activation and improves the protection against Gram-negative intestinal pathogens. Thus, this study provides access to several directions of investigations to expand the characterization of the TUCO-L2 strain and position it as a probiotic or immunobiotic strain that can be used against infections in humans or animals, such as llamas.
The datasets generated for this study can be found in online repositories. The names of the repository/repositories and accession number(s) can be found at: https://www.ncbi.nlm.nih.gov/genbank/, SOPE01000000.
The animal study was reviewed and approved by The CERELA Institutional Animal Care and Use Committee (San Miguel de Tucuman, Tucuman, Argentina).
SQ-V, YS, HK, and JV designed the study. SQ-V, FM, LAr, BZ, and MT performed in vitro studies. LAl, IA, and LAr, performed the bioinformatics studies. LAr and JV performed in vivo studies. SQ-V, YS, LAr, and LAl performed the statistical analysis. SQ-V, HK, and JV analyzed the data. HK and JV wrote the manuscript. All authors read and approved the manuscript.
This study was supported by ANPCyT–FONCyT grant PICT-2016-0410 to JV, grants from the project of NARO Bio-oriented Technology Research Advancement Institution (research program on the development of innovative technology, no. 01002A), and by a Grant-in-Aid for Scientific Research (A) (19H00965) from the Japan Society for the Promotion of Science (JSPS) to HK. This work was also supported by JSPS Core-to-Core Program, A. Advanced Research Networks entitled Establishment of international agricultural immunology research-core for a quantum improvement in food safety. MI was supported by JSPS (Postdoctoral Fellowship for Foreign Researchers, Program No. 18F18081). MT was supported by Tohoku University Global Hagi Scholarship.
The authors declare that the research was conducted in the absence of any commercial or financial relationships that could be construed as a potential conflict of interest.
The Supplementary Material for this article can be found online at: https://www.frontiersin.org/articles/10.3389/fmicb.2020.608752/full#supplementary-material
Supplementary Figure 1. Microbiological characterization of Ligilactobacillus salivarius TUCO-L2. The TUCO-L2 strain is a short rod-shaped Gram-positive bacterium that aggregates in MRS broth and grows as white pearl-like colonies in MRS agar.
Supplementary Figure 2. Genomic comparison of the pilus operon of Ligilactobacillus salivarius TUCO-L2 isolated from llama (Lama glama) milk with that of the strains of the same species. The pilus operon genes in the genomes of L. salivarius JCM1047, DSM20555, ATCC11741, gul1, gul2, and A3iob were identified by search of the genome of the TUCO-L2 strain.
Supplementary Figure 3. Genomic comparison of the SeA2-SecY2 accessory secretion system of Ligilactobacillus salivarius TUCO-L2 isolated from llama (Lama glama) milk with the gene clusters of the L. salivarius strains from the intestinal tract of pig (JCM1046) and chicken (DJ-sa-01). Gene organization of the SeA2-SecY2 accessory secretion system of selected strains is shown. Conserved glycosyltransferases are shown in orange, and non-conserved glycosyltransferases are shown in light green. Putative srr adhesins are shown in dark green. Gray connections indicate high homology between the genes.
Supplementary Figure 4. The effect of Ligilactobacillus salivarius TUCO-L2 isolated from llama (Lama glama) milk on the systemic immune response of adult mice to Salmonella infection. L. salivarius TUCO-L2 was administered to male 6-week-old Balb/c mice for 5 consecutive days at a dose of 108 cells/mouse/day in drinking water. Untreated mice were used as a control. TUCO-L2-treated and control mice were challenged by oral administration of 107 cells/mouse of S. typhimurium (20LD50) on day 6. Mice were sacrificed on day 2 after the infection, and the levels of serum TNF-α, IFN-γ, IL-1β, IL-6, and IL-10 were measured by ELISA. Untreated and uninfected mice were used for comparison (basal group). Each parameter was assayed in 5–6 mice per group. Letters indicate significant differences (P < 0.05), a < b < c.
Supplementary Table 1. Evaluation of general probiotic properties. Resistance to NaCl and Oxgall.
Supplementary Table 2. General genomic features of Ligilactobacillus salivarius strains used in this work.
Supplementary Table 3. List of unique genes found in the genome of Ligilactobacillus salivarius TUCO-L2 compared with the strains isolated from human milk L. salivarius CECT 5713 and LPM01.
Supplementary Table 4. List of unique genes found in the genome of Ligilactobacillus salivarius TUCO-L2 compared with the strains isolated from human intestine L. salivarius UCC118 and REN.
Supplementary Table 5. List of unique genes found in the genome of Ligilactobacillus salivarius TUCO-L2 compared with the strains isolated from porcine intestine L. salivarius JCM1046 and ZLS006.
Supplementary Table 6. List of unique genes found in the genome of Ligilactobacillus salivarius TUCO-L2 compared with the strains isolated from chicken intestine L. salivarius DJ-sa-01 and CICC23174.
Supplementary Table 7. BAGEL 4 and blastp analysis for the search of bacteriocins in the genome of Ligilactobacillus salivarius TUCO-L2.
Abo-Amer, A. E. (2013). Inhibition of foodborne pathogens by a bacteriocin-like substance produced by a novel strain of Lactobacillus acidophilus isolated from camel milk. Appl. Biochem. Microbiol. 49, 270–279. doi: 10.1134/S0003683813030174
Albarracin, L., García-Castillo, V., Masumizu, Y., Indo, Y., Islam, M. A., Suda, Y., et al. (2020a). Efficient selection of new immunobiotic strains with antiviral effects in local and distal mucosal sites by using porcine intestinal epitheliocytes. Front. Immunol. 11:543. doi: 10.3389/fimmu.2020.00543
Albarracin, L., Kobayashi, H., Iida, H., Sato, N., Nochi, T., Aso, H., et al. (2017). Transcriptomic analysis of the innate antiviral immune response in porcine intestinal epithelial cells: influence of immunobiotic lactobacilli. Front. Immunol. 8:57. doi: 10.3389/fimmu.2017.00057
Albarracin, L., Quilodrán-Vega, S., Fukuyama, K., Tomokiyo, M., Islam, M. A., Arce, L., et al. (2020b). Draft genome sequence of Ligilactobacillus salivarius TUCO-L2, isolated from Lama glama milk. Microbiol. Resour. Announc. 9:e00784–20. doi: 10.1128/MRA.00784-20
Alcántara, C., Revilla-Guarinos, A., and Zúiga, M. (2011). Influence of two-component signal transduction systems of Lactobacillus casei BL23 on tolerance to stress conditions. Appl. Environ. Microbiol. 77, 1516–1519. doi: 10.1128/AEM.02176-10
Allaire, J. M., Crowley, S. M., Law, H. T., Chang, S. Y., Ko, H. J., and Vallance, B. A. (2018). The intestinal epithelium: central coordinator of mucosal immunity. Trends Immunol. 39, 677–696. doi: 10.1016/j.it.2018.04.002
Audisio, M. C., Albarracín, L., Torres, M. J., Saavedra, L., Hebert, E. M., and Villena, J. (2018). Draft genome sequences of Lactobacillus salivarius A3iob and Lactobacillus johnsonii CRL1647, novel potential probiotic strains for honeybees (Apis mellifera L.). Microbiol. Resour. Announc. 7, e00975–e00918. doi: 10.1128/MRA.00975-18
Bensing, B. A., Seepersaud, R., Yen, Y. T., and Sullam, P. M. (2014). Selective transport by SecA2: an expanding family of customized motor proteins. Biochim. Biophys. Acta 1843, 1674–1686. doi: 10.1016/j.bbamcr.2013.10.019
Boyer, J. L. (2013). Bile formation and secretion. Compr. Physiol. 3, 1035–1078. doi: 10.1002/cphy.c120027
Brolazo, E. M., Leite, D. S., Tiba, M. R., Villarroel, M., Marconi, C., and Simoes, J. A. (2011). Correlation between API 50 CH and multiplex polymerase chain reaction for the identification of vaginal lactobacilli in isolates. Braz. J. Microbiol. 42, 225–232. doi: 10.1590/S1517-83822011000100028
Couvigny, B., Kulakauskas, S., Pons, N., Quinquis, B., Abraham, A. L., Meylheuc, T., et al. (2018). Identification of new factors modulating adhesion abilities of the pioneer commensal bacterium Streptococcus salivarius. Front. Microbiol. 9:273. doi: 10.3389/fmicb.2018.00273
Couvigny, B., Lapaque, N., Rigottier-Gois, L., Guillot, A., Chat, S., Meylheuc, T., et al. (2017). Three glycosylated serine-rich repeat proteins play a pivotal role in adhesion and colonization of the pioneer commensal bacterium, Streptococcus salivarius. Environ. Microbiol. 19, 3579–3594. doi: 10.1111/1462-2920.13853
Cristofanelli, S., Antonini, M., Torres, D., Polidori, P., and Renieri, C. (2004). Meat and carcass quality from Peruvian llama (Lama glama) and alpaca (Lama pacos). Meat. Sci. 66, 589–593. doi: 10.1016/S0309-1740(03)00174-8
De Boeck, I., van den Broek, M. F. L., Allonsius, C. N., Spacova, I., Wittouck, S., Martens, K., et al. (2020). Lactobacilli have a niche in the human nose. Cell Rep. 31:107674. doi: 10.1016/j.celrep.2020.107674
Dong, F., Hennessy, D. A., and Jensen, H. H. (2012). Factors determining milk quality and implications for production structure under somatic cell count standard modification. J. Dairy Sci. 95, 6421–6435. doi: 10.3168/jds.2012-5522
Edgar, R. C. (2004). MUSCLE: multiple sequence alignment with high accuracy and high throughput. Nucleic Acids Res. 32, 1792–1797. doi: 10.1093/nar/gkh340
Elbanna, K., El Hadad, S., Assaeedi, A., Aldahlawi, A., Khider, M., and Alhebshi, A. (2018). In vitro and in vivo evidences for innate immune stimulators lactic acid bacterial starters isolated from fermented camel dairy products. Sci. Rep. 8:12553. doi: 10.1038/s41598-018-31006-3
Etzold, S., and Juge, N. (2014). Structural insights into bacterial recognition of intestinal mucins. Curr. Opin. Struct. Biol. 28, 23–31. doi: 10.1016/j.sbi.2014.07.002
Etzold, S., Kober, O. I., Mackenzie, D. A., Tailford, L. E., Gunning, A. P., Walshaw, J., et al. (2014). Structural basis for adaptation of lactobacilli to gastrointestinal mucus. Environ. Microbiol. 16, 888–903. doi: 10.1111/1462-2920.12377
FAOSTAT (2019) FAOSTAT. Available online at: http://www.fao.org/faostat/en/#home (accessed September 19, 2020).
Feltcher, M. E., and Braunstein, M. (2012). Emerging themes in SecA2-mediated protein export. Nat. Rev. Microbiol. 10, 779–789. doi: 10.1038/nrmicro2874
Frese, S. A., MacKenzie, D. A., Peterson, D. A., Schmaltz, R., Fangman, T., Zhou, Y., et al. (2013). Molecular characterization of host-specific biofilm formation in a vertebrate gut symbiont. PLoS Genet. 9:e1004057. doi: 10.1371/journal.pgen.1004057
Fukuda, K. (2013). “Camel Milk,” in Milk and Dairy Products in Human Nutrition, eds Y. W. Park and G. F. W. Haenlein (Chichester: Wiley), 578–593. doi: 10.1002/9781118534168.ch26
Garcia-Castillo, V., Komatsu, R., Clua, P., Indo, Y., Takagi, M., Salva, S., et al. (2019). Evaluation of the immunomodulatory activities of the probiotic strain Lactobacillus fermentum UCO-979C. Front. Immunol. 10:1376. doi: 10.3389/fimmu.2019.01376
Gunning, A. P., Kavanaugh, D., Thursby, E., Etzold, S., Mackenzie, D. A., and Juge, N. (2016). Use of atomic force microscopy to study the multi-modular interaction of bacterial adhesins to mucins. Int. J. Mol. Sci. 17:1854. doi: 10.3390/ijms17111854
Harris, H. M. B., Bourin, M. J. B., Claesson, M. J., and O'Toole, P. W. (2017). Phylogenomics and comparative genomics of Lactobacillus salivarius, a mammalian gut commensal. Microb. Genomics 3:e000115. doi: 10.1099/mgen.0.000115
Heberle, H., Meirelles, V. G., da Silva, F. R., Telles, G. P., and Minghim, R. (2015). InteractiVenn: a web-based tool for the analysis of sets through Venn diagrams. BMC Bioinformatics 16:169. doi: 10.1186/s12859-015-0611-3
Jans, C., Bugnard, J., Njage, P. M. K., Lacroix, C., and Meile, L. (2012). Lactic acid bacteria diversity of African raw and fermented camel milk products reveals a highly competitive, potentially health-threatening predominant microflora. LWT 47, 371–379. doi: 10.1016/j.lwt.2012.01.034
Kankainen, M., Paulin, L., Tynkkynen, S., Von Ossowski, I., Reunanen, J., Partanen, P., et al. (2009). Comparative genomic analysis of Lactobacillus rhamnosus GG reveals pili containing a human-mucus binding protein. Proc. Natl. Acad. Sci. U.S.A. 106, 17193–17198. doi: 10.1073/pnas.0908876106
Kanmani, P., Albarracin, L., Kobayashi, H., Hebert, E. M., Saavedra, L., Komatsu, R., et al. (2018a). Genomic characterization of Lactobacillus delbrueckii TUA4408L and evaluation of the antiviral activities of its extracellular polysaccharides in porcine intestinal epithelial cells. Front. Immunol. 9:2178. doi: 10.3389/fimmu.2018.02178
Kanmani, P., Albarracin, L., Kobayashi, H., Iida, H., Komatsu, R., Humayun Kober, A. K. M., et al. (2018b). Exopolysaccharides from Lactobacillus delbrueckii OLL1073R-1 modulate innate antiviral immune response in porcine intestinal epithelial cells. Mol. Immunol. 93, 253–265. doi: 10.1016/j.molimm.2017.07.009
Khedid, K., Faid, M., Mokhtari, A., Soulaymani, A., and Zinedine, A. (2009). Characterization of lactic acid bacteria isolated from the one humped camel milk produced in Morocco. Microbiol. Res. 164, 81–91. doi: 10.1016/j.micres.2006.10.008
Kobayashi, H., Albarracin, L., Sato, N., Kanmani, P., Kober, A. K. M. H., Ikeda-Ohtsubo, W., et al. (2016). Modulation of porcine intestinal epitheliocytes immunetranscriptome response by Lactobacillus jensenii TL2937. Benef. Microbes 7, 769–782. doi: 10.3920/BM2016.0095
Kuhn, K. A., Schulz, H. M., Regner, E. H., Severs, E. L., Hendrickson, J. D., Mehta, G., et al. (2018). Bacteroidales recruit IL-6-producing intraepithelial lymphocytes in the colon to promote barrier integrity. Mucosal. Immunol. 11, 357–368. doi: 10.1038/mi.2017.55
Kumar, S., Stecher, G., Li, M., Knyaz, C., and Tamura, K. (2018). MEGA X: molecular evolutionary genetics analysis across computing platforms. Mol. Biol. Evol. 35, 1547–1549. doi: 10.1093/molbev/msy096
Larico, H. M., Fernández, E. R., Olarte, C. D., Rodrigo, Y. V., Machaca, P. T., Sumari, R. M., et al. (2018). Alpaca cheese: a new alternative. Rev. Investig. Vet. del Peru 29, 848–857. doi: 10.15381/rivep.v29i3.14015
Latousakis, D., and Juge, N. (2018). How sweet are our gut beneficial bacteria? A focus on protein glycosylation in Lactobacillus. Int. J. Mol. Sci. 19:136. doi: 10.3390/ijms19010136
Lee, J. Y., Han, G. G., Kim, E. B., and Choi, Y. J. (2017). Comparative genomics of Lactobacillus salivarius strains focusing on their host adaptation. Microbiol. Res. 205, 48–58. doi: 10.1016/j.micres.2017.08.008
Liévin-Le Moal, V., and Servin, A. L. (2014). Anti-infective activities of Lactobacillus strains in the human intestinal microbiota: From probiotics to gastrointestinal anti- infectious biotherapeutic agents. Clin. Microbiol. Rev. 27, 167–199. doi: 10.1128/CMR.00080-13
Lü, X., Hu, P., Dang, Y., and Liu, B. (2014). Purification and partial characterization of a novel bacteriocin produced by Lactobacillus casei TN-2 isolated from fermented camel milk (Shubat) of Xinjiang Uygur Autonomous region, China. Food Control 43, 276–283. doi: 10.1016/j.foodcont.2014.03.020
Makarova, K., Slesarev, A., Wolf, Y., Sorokin, A., Mirkin, B., Koonin, E., et al. (2006). Comparative genomics of the lactic acid bacteria. Proc. Natl. Acad. Sci. U.S.A. 103, 15611–15616. doi: 10.1073/pnas.0607117103
Martinez, R. C. R., Franceschini, S. A., Patta, M. C., Quintana, S. M., Nunes, Á. C., Moreira, J. L. S., et al. (2008). Analysis of vaginal lactobacilli from healthy and infected Brazilian women. Appl. Environ. Microbiol. 74, 4539–4542. doi: 10.1128/AEM.00284-08
Masumizu, Y., Zhou, B., Kober, A. K. M. H., Islam, M. A., Iida, H., Ikeda-Ohtsubo, W., et al. (2019). Isolation and immunocharacterization of Lactobacillus salivarius from the intestine of wakame-fed pigs to develop novel “immunosynbiotics.” Microorganisms 7:167. doi: 10.3390/microorganisms7060167
Monteagudo-Mera, A., Rastall, R. A., Gibson, G. R., Charalampopoulos, D., and Chatzifragkou, A. (2019). Adhesion mechanisms mediated by probiotics and prebiotics and their potential impact on human health. Appl. Microbiol. Biotechnol. 103, 6463–6472. doi: 10.1007/s00253-019-09978-7
Moue, M., Tohno, M., Shimazu, T., Kido, T., Aso, H., Saito, T., et al. (2008). Toll-like receptor 4 and cytokine expression involved in functional immune response in an originally established porcine intestinal epitheliocyte cell line. Biochim. Biophys. Acta. 1780, 134–144. doi: 10.1016/j.bbagen.2007.11.006
Outten, F. W., Djaman, O., and Storz, G. (2004). A suf operon requirement for Fe-S cluster assembly during iron starvation in Escherichia coli. Mol. Microbiol. 52, 861–872. doi: 10.1111/j.1365-2958.2004.04025.x
Page, A. J., Cummins, C. A., Hunt, M., Wong, V. K., Reuter, S., Holden, M. T. G., et al. (2015). Roary: rapid large-scale prokaryote pan genome analysis. Bioinformatics 31, 3691–3693. doi: 10.1093/bioinformatics/btv421
Pauciullo, A., and Erhardt, G. (2015). Molecular characterization of the llamas (Lama glama) casein cluster genes transcripts (CSN1S1, CSN2, CSN1S2, CSN3) and regulatory regions. PLoS ONE 10:e0124963. doi: 10.1371/journal.pone.0124963
Pérez, P., Maino, M., Guzmán, R., Vaquero, A., Köbrich, C., and Pokniak, J. (2000). Carcass characteristics of llamas (Lama glama) reared in Central Chile. Small Rumin. Res. 37, 93–97. doi: 10.1016/S0921-4488(99)00127-3
Polidori, P., Renieri, C., Antonini, M., Passamonti, P., and Pucciarelli, F. (2007). Meat fatty acid composition of llama (Lama glama) reared in the Andean highlands. Meat Sci. 75, 356–358. doi: 10.1016/j.meatsci.2006.07.010
Quilodrán-Vega, S. R., Villena, J., Valdebenito, J., Salas, M. J., Parra, C., Ruiz, A., et al. (2016). Isolation of lactic acid bacteria from swine milk and characterization of potential probiotic strains with antagonistic effects against swine-associated gastrointestinal pathogens. Can. J. Microbiol. 62, 514–524. doi: 10.1139/cjm-2015-0811
Raftis, E. J., Salvetti, E., Torriani, S., Felis, G. E., and O'Toole, P. W. (2011). Genomic diversity of Lactobacillus salivarius. Appl. Environ. Microbiol. 77, 954–965. doi: 10.1128/AEM.01687-10
Rahmeh, R., Akbar, A., Kishk, M., Al-Onaizi, T., Al-Azmi, A., Al-Shatti, A., et al. (2019). Distribution and antimicrobial activity of lactic acid bacteria from raw camel milk. New Microbes New Infect. 30:100560. doi: 10.1016/j.nmni.2019.100560
Saitou, N., and Nei, M. (1987). The neighbor-joining method: a new method for reconstructing phylogenetic trees. Mol. Biol. Evol. 4, 406–425
Santos-Beneit, F. (2015). The pho regulon: a huge regulatory network in bacteria. Front. Microbiol. 6:402. doi: 10.3389/fmicb.2015.00402
Seemann, T. (2014). Prokka: rapid prokaryotic genome annotation. Bioinformatics 30, 2068–2069. doi: 10.1093/bioinformatics/btu153
Shabo, Y., Barzel, R., Margoulis, M., and Yagil, R. (2005). Camel milk for food allergies in children. Isr. Med. Assoc. J. 7, 796–798
Shimazu, T., Villena, J., Tohno, M., Fujie, H., Hosoya, S., Shimosato, T., et al. (2012). Immunobiotic Lactobacillus jensenii elicits anti-inflammatory activity in porcine intestinal epithelial cells by modulating negative regulators of the toll-like receptor signaling pathway. Infect. Immun. 80, 276–288. doi: 10.1128/IAI.05729-11
Siegmund, B., Lehr, H. A., Fantuzzi, G., and Dinarello, C. A. (2001). IL-1β-converting enzyme (caspase-1) in intestinal inflammation. Proc. Natl. Acad. Sci. U.S.A. 98, 13249–13254. doi: 10.1073/pnas.231473998
Suda, Y., Villena, J., Takahashi, Y., Hosoya, S., Tomosada, Y., Tsukida, K., et al. (2014). Immunobiotic Lactobacillus jensenii as immune-health promoting factor to improve growth performance and productivity in post-weaning pigs. BMC Immunol. 15, 1–18. doi: 10.1186/1471-2172-15-24
Tamura, K., Nei, M., and Kumar, S. (2004). Prospects for inferring very large phylogenies by using the neighbor-joining method. Proc. Natl. Acad. Sci. U.S.A. 101, 11030–11035. doi: 10.1073/pnas.0404206101
Vimont, A., Fernandez, B., Hammami, R., Ababsa, A., Daba, H., and Fliss, I. (2017). Bacteriocin-producing Enterococcus faecium LCW 44: A high potential probiotic candidate from raw camel milk. Front. Microbiol. 8:865. doi: 10.3389/fmicb.2017.00865
Wachi, S., Kanmani, P., Tomosada, Y., Kobayashi, H., Yuri, T., Egusa, S., et al. (2014). Lactobacillus delbrueckii TUA4408L and its extracellular polysaccharides attenuate enterotoxigenic Escherichia coli- induced inflammatory response in porcine intestinal epitheliocytes via toll-like receptor-2 and 4. Mol. Nutr. Food Res. 58, 2080–2093. doi: 10.1002/mnfr.201400218
Wang, G., Li, D., Ma, X., An, H., Zhai, Z., Ren, F., et al. (2015). Functional role of oppA encoding an oligopeptide-binding protein from Lactobacillus salivarius Ren in bile tolerance. J. Ind. Microbiol. Biotechnol. 42, 1167–1174. doi: 10.1007/s10295-015-1634-5
Warnes, G. R., Bolker, B., Gorjanc, G., Grothendieck, G., Korosec, A., Lumley, T., et al. (2017). CRAN - Package gdata. Available online at: https://cran.r-project.org/web/packages/gdata/index.html (accessed August 19, 2020)
Wegmann, U., MacKenzie, D. A., Zheng, J., Goesmann, A., Roos, S., Swarbreck, D., et al. (2015). The pan-genome of Lactobacillus reuteri strains originating from the pig gastrointestinal tract. BMC Genomics 16:1023. doi: 10.1186/s12864-015-2216-7
Zarrin, M., Riveros, J. L., Ahmadpour, A., de Almeida, A. M., Konuspayeva, G., Vargas-Bello-Pérez, E., et al. (2020). Camelids: new players in the international animal production context. Trop. Anim. Health Prod. 52, 903–913. doi: 10.1007/s11250-019-02197-2
Zhai, Q., Shen, X., Cen, S., Zhang, C., Tian, F., Zhao, J., et al. (2020). Screening of: Lactobacillus salivarius strains from the feces of Chinese populations and the evaluation of their effects against intestinal inflammation in mice. Food Func. 11, 221–235. doi: 10.1039/C9FO02116G
Zhao, J., Fan, H., Kwok, L. Y., Guo, F., Ji, R., Ya, M., et al. (2020). Analyses of physicochemical properties, bacterial microbiota, and lactic acid bacteria of fresh camel milk collected in inner Mongolia. J. Dairy Sci. 103, 106–116. doi: 10.3168/jds.2019-17023
Zheng, J., Wittouck, S., Salvetti, E., Franz, C. M. A. P., Harris, H. M. B., Mattarelli, P., et al. (2020). A taxonomic note on the genus Lactobacillus: description of 23 novel genera, emended description of the genus Lactobacillus Beijerinck 1901, and union of Lactobacillaceae and Leuconostocaceae. Int. J. Syst. Evol. Microbiol. 70, 2782–2858. doi: 10.1099/ijsem.0.004107
Zhou, B., Albarracin, L., Indo, Y., Arce, L., Masumizu, Y., Tomokiyo, M., et al. (2020). Selection of Immunobiotic Ligilactobacillus salivarius strains from the intestinal tract of wakame-fed pigs: functional and genomic studies. Microorganisms 8:1659. doi: 10.3390/microorganisms8111659
Živković, M., Miljković, M. S., Ruas-Madiedo, P., Markelić, M. B., Veljović, K., Tolinački, M., et al. (2016). EPS-SJ exopolisaccharide produced by the strain Lactobacillus paracasei subsp. paracasei BGSJ2-8 is involved in adhesion to epithelial intestinal cells and decrease on E. coli association to Caco-2 cells. Front. Microbiol. 7:286. doi: 10.3389/fmicb.2016.00286
Keywords: Ligilactobacillus salivarius TUCO-L2, Lama glama milk, bacterial infection, probiotic, immunomodulation, intestinal epithelia cell
Citation: Quilodrán-Vega S, Albarracin L, Mansilla F, Arce L, Zhou B, Islam MA, Tomokiyo M, Al Kassaa I, Suda Y, Kitazawa H and Villena J (2020) Functional and Genomic Characterization of Ligilactobacillus salivarius TUCO-L2 Isolated From Lama glama Milk: A Promising Immunobiotic Strain to Combat Infections. Front. Microbiol. 11:608752. doi: 10.3389/fmicb.2020.608752
Received: 21 September 2020; Accepted: 16 November 2020;
Published: 08 December 2020.
Edited by:
Xinglin Zhang, Zhejiang University, ChinaReviewed by:
Felix Ngosa Toka, Warsaw University of Life Sciences, PolandCopyright © 2020 Quilodrán-Vega, Albarracin, Mansilla, Arce, Zhou, Islam, Tomokiyo, Al Kassaa, Suda, Kitazawa and Villena. This is an open-access article distributed under the terms of the Creative Commons Attribution License (CC BY). The use, distribution or reproduction in other forums is permitted, provided the original author(s) and the copyright owner(s) are credited and that the original publication in this journal is cited, in accordance with accepted academic practice. No use, distribution or reproduction is permitted which does not comply with these terms.
*Correspondence: Haruki Kitazawa, aGFydWtpLmtpdGF6YXdhLmM3QHRvaG9rdS5hYy5qcA==; Julio Villena, amN2aWxsZW5hQGNlcmVsYS5vcmcuYXI=
†These authors have contributed equally to this work
Disclaimer: All claims expressed in this article are solely those of the authors and do not necessarily represent those of their affiliated organizations, or those of the publisher, the editors and the reviewers. Any product that may be evaluated in this article or claim that may be made by its manufacturer is not guaranteed or endorsed by the publisher.
Research integrity at Frontiers
Learn more about the work of our research integrity team to safeguard the quality of each article we publish.