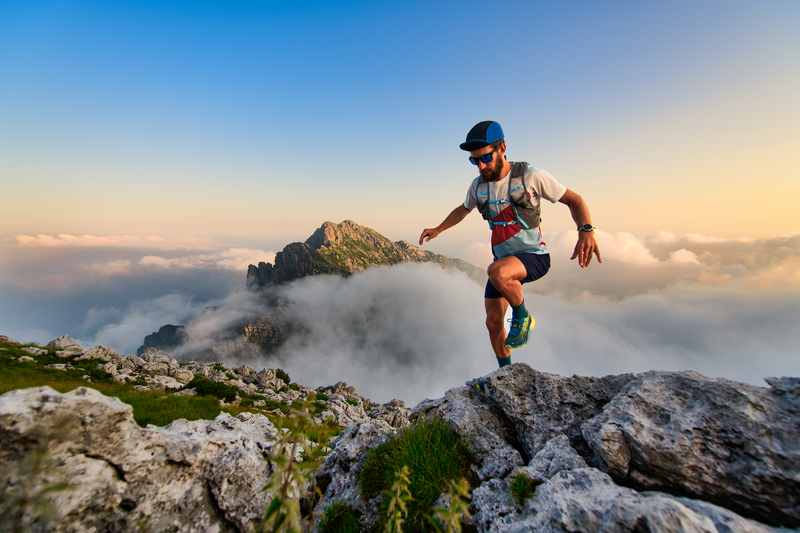
95% of researchers rate our articles as excellent or good
Learn more about the work of our research integrity team to safeguard the quality of each article we publish.
Find out more
REVIEW article
Front. Microbiol. , 25 November 2020
Sec. Infectious Agents and Disease
Volume 11 - 2020 | https://doi.org/10.3389/fmicb.2020.602305
This article is part of the Research Topic Cell Surface Proteins of Gram-positive Pathogenic Bacteria View all 15 articles
Streptococci are Gram-positive bacteria that belong to the natural microbiota of humans and animals. Certain streptococcal species are known as opportunistic pathogens with the potential to cause severe invasive disease. Antigen I/II (AgI/II) family proteins are sortase anchored cell surface adhesins that are nearly ubiquitous across streptococci and contribute to many streptococcal diseases, including dental caries, respiratory tract infections, and meningitis. They appear to be multifunctional adhesins with affinities to various host substrata, acting to mediate attachment to host surfaces and stimulate immune responses from the colonized host. Here we will review the literature including recent work that has demonstrated the multifaceted nature of AgI/II family proteins, focusing on their overlapping and distinct functions and their important contribution to streptococcal colonization and disease.
The genus Streptococcus is a heterogeneous group of Gram-positive bacteria that can be part of the natural microbiota. Various streptococci are commonly isolated from the oral cavity, intestines, or female reproductive tracts in healthy humans. These colonizing streptococci are often commensal organisms that persist without causing disease. In some cases, colonization by commensal streptococci can actually benefit the host through mechanisms such as niche competition or direct inhibition of more pathogenic organisms (Abranches et al., 2018; Nobbs and Kreth, 2019); however, there are also many streptococcal species that are opportunistic pathogens with the potential to cause severe invasive disease. Within the viridans group of streptococci, a heterogeneous group consisting of typically commensal α-hemolytic species, exists Streptococcus mutans. S. mutans is a major agent of dental caries (tooth decay) (Lehner et al., 1975) and infective endocarditis (Hoen et al., 2002). Streptococcus pyogenes (Group A Streptococcus, GAS) is a major cause of skin and soft tissue infections, as well as respiratory infections (Steer et al., 2012). Similarly, Streptococcus agalactiae (Group B Streptococcus, GBS) is known to colonize the female reproductive tract and is a leading cause of neonatal sepsis, pneumonia, and meningitis (Edmond et al., 2012). Understanding the shared mechanisms by which these diverse species colonize and cause disease is the first step toward treatment and prevention. One example of a shared virulence factor among these species is the family of multifunctional streptococcal surface anchored adhesins known as antigen I/II (AgI/II) proteins.
Mike Russell et al. and Roy Russell et al. identified the cell wall antigens I and II in Streptococcus mutans nearly simultaneously over 40 years ago (Russell and Lehner, 1978; Russell, 1979; Russell et al., 1980). Soon after, it was shown that antigen II was actually a breakdown product of antigen I (Kelly et al., 1989), giving rise to their new classification as antigen I/II proteins. These AgI/II proteins are widely distributed not only among various serotypes within S. mutans (Russell and Lehner, 1978; Russell et al., 1980; Ma et al., 1991), but orthologous proteins sharing similar structure and functions also exist within a number of other streptococcal species (Kelly et al., 1989; Jenkinson and Demuth, 1997; Brady et al., 2010). Due in part to the initial discovery of AgI/II proteins within S. mutans, their contribution to dental caries has been a major focus of AgI/II related research; however, more recent studies have investigated AgI/II proteins in other species and biological niches as well. The multifunctional nature of these adhesins in multiple niches may directly contribute to the transition toward pathogenesis as the same protein that promotes colonization of a commensal or beneficial organism in one niche may also allow persistence after that organism gains access to new and potentially more susceptible host tissues. Furthermore, discrete locations of various binding sites on AgI/II proteins for interaction with different microorganisms and host molecules ensures maximum protein functionality, which could be a key mechanism for generating diversity in the development of microbial communities. In addition to the cariogenic properties of AgI/II proteins, these studies have revealed diverse functions ranging from adherence to immune modulation, which in turn contribute to streptococcal colonization and disease.
Proteins belonging to the AgI/II family that have been identified to date are known by various names, many of which are shown in Table 1. The majority of these were independently studied and named by different groups prior to the widespread availability of sequencing technologies that eventually revealed that these were, in fact, the same proteins. For example, the single S. mutans AgI/II protein is known by at least seven different names. In some cases, the different names represent different proteins coded by unique genes within the same species; BspA-D in GBS, SspA/B in S. gordonii, and PAaA/B in S. criceti are examples of this. Standardization of the nomenclature in future publications would significantly clarify which proteins are being discussed. A few groups have used the “AgI/II” designation for antigen I/II proteins regardless of the species to which the protein belongs (Sciotti et al., 1997; Chuzeville et al., 2017; Auger et al., 2019).
AgI/II proteins are nearly ubiquitous within streptococci. In order to examine the relatedness of AgI/II proteins across species, representative genes from those listed in Table 1 were aligned, and their phylogenetic relationships are shown in Figure 1A. Orthologous genes, many of which have yet to be further characterized and may not be expressed at the protein level, were also identified in S. rattus, S. oralis, S. mitis, S. anginosus, S. constellatus, S. vestibularis, and S. parasanguinis via amplification from S. mutans SpaP based PCR probes (Ma et al., 1991). Another potential AgI/II protein was recently identified in S. salivarius as well, though it also remains to be characterized (Chaffanel et al., 2018). As AgI/II genes are so widely distributed among streptococci, it is likely that other species that were not listed here also contain AgI/II orthologs that have yet to be identified. For example, while there was previously no evidence for an AgI/II gene within S. pneumoniae (Brady et al., 2010), a BLASTP search for homologs of the GAS AgI/II gene AspA within S. pneumoniae strains revealed a homologous protein in a newly sequenced strain (NCBI RefSeq: WP_160544519.1). This protein has a similar primary structure with 69% pairwise identity to AspA, with 83% coverage, which is comparable to the variation that exists between AgI/II genes from different species. While this was the only S. pneumoniae strain we found containing an AgI/II homolog, its presence nonetheless highlights the widespread distribution of AgI/II proteins and their potential to provide pathogens with a selective advantage in certain niches.
Figure 1. Homology of AgI/II Family Proteins. (A) The evolutionary history was inferred by using the Maximum Likelihood method and JTT matrix-based model (Jones et al., 1992) using full-length amino acid sequences of the indicated proteins. The tree with the highest log likelihood (–21701.96) is shown. The tree is drawn to scale, with branch lengths measured in the number of substitutions per site. Evolutionary analyses were conducted in MEGA X (Kumar et al., 2018). (B) Conservation of individual domains is shown (top) as the pairwise identity percentage resulting from a MUSCLE protein alignment of each individual domain, as well as the primary structure and amino acid length of AgI/II proteins from select streptococci (bottom). The domains shown are the N-terminal region (N; blue), alanine-rich repeats (A; purple), the variable region (V; green), proline-rich repeats (P; yellow), the C-terminal region (C; orange), and the cell wall anchoring region (CWA; gray). The Uniprot IDs for protein sequences used in both (A,B) are as follows: S. mutans SpaP (P23504), S. gordonii SspA (Q54185), S. gordonii SspB (Q54186), S. agalactiae BspA (Q8E589), S. agalactiae BspC (A0A380IJX7), S. pyogenes AspA (Q48S75), S. intermedius Pas (Q9KW51), S. sobrinus SpaA (Q53414), S. sanguinis PAc (F3V086), S. criceti PAaA (Q9LBG3), S. downei PAh (Q59HN9), and S. suis SSP-5 (A0A0Z8CV71).
Previous studies comparing sequences across AgI/II genes from multiple species have revealed domain-based variation in conservation (Ma et al., 1991; Brady et al., 2010; Deng et al., 2019). This is shown to be especially true when protein sequences from a larger number of species are compared (Figure 1B). Comparisons of AgI/II genes from multiple strains within one species, however, reveal high levels of sequence conservation (Brady et al., 1991; Ma et al., 1991; Do et al., 2010; Chuzeville et al., 2015; Rego et al., 2016). For example, a study comparing 37 S. mutans SpaP genes observed non-synonymous polymorphisms in only 4% of sites (Do et al., 2010). This indicates both diversity within the AgI/II family of proteins, as well as specificity, where the AgI/II proteins within each species may have evolved to potentially better interact with niche specific receptors. The fact that AgI/II genes from more invasive streptococcal species cluster separately from the viridans streptococci supports this theory (Figure 1A). One factor contributing to the distribution and clustering of AgI/II genes is their ability to be shared via horizontal gene transfer. An example of this is seen with the GAS and GBS AgI/II genes. In GAS, the AgI/II genes are located on the integrative and conjugative element named region of difference 2 (RD2), which is thought to be horizontally shared between GBS, conferring pathogenic traits that support invasive disease in pregnant women and neonates (Zhang et al., 2006; Sitkiewicz et al., 2011; Chuzeville et al., 2015; Deng et al., 2019; Jain et al., 2019). It is especially concerning that this virulence factor is able to spread horizontally since this family of proteins has a wide variety of pathogenic functions, and their presence in streptococcal species occupying unique niches may correlate with the ability to cause disease as well.
The primary structure of AgI/II proteins is shown in Figure 1B. AgI/II proteins begin with an N-terminal domain containing a signal peptide mediating secretion via the general secretory pathway (Scott and Barnett, 2006). This is adjacent to the A-domain, a region containing multiple alanine-rich repeats (Kelly et al., 1989). The A-domain is connected to a globular domain termed the V-domain based on the variability observed in this region between strains (Brady et al., 1991). The V-domain contains a putative binding pocket, which will be elaborated on in greater detail further on. At the other end of the V-domain is the P-domain, termed for the presence of proline-rich repeats within this region (Kelly et al., 1995). Following the P-domain is the C-terminal region, which consists of two or three globular IgG-like domains. Finally, there is an LPXTG motif for sortase-mediated cell wall anchoring (Kelly et al., 1989). Overall, the AgI/II proteins adopt a cell wall-anchored stalk structure formed by interactions between the intertwined A- and P-domains, which lifts the globular V-domain over 50 nm away from the cell surface in the case of the S. mutans SpaP (Larson et al., 2010). The A- and P-domains of SpaP were shown to utilize a unique interlocking between tyrosine residues from heptad repeat motifs in the A-domain with hydrophobic pockets consisting of PxxP motifs within the P-region (Larson et al., 2010). The A- and P-domains are significantly smaller in some homologs such as the Bsp proteins of GBS (Figures 1B, 2), and it has also been shown that the interaction between A- and P-domains within these homologs is formed in a different manner. The A-domain of BspA contains an asparagine seam whose hydrogens interact with oxygen and nitrogen atoms within the P-domain (Rego et al., 2016). In addition to the A- and P-domains interacting, the tertiary stalk structure of AgI/II proteins is further stabilized by interactions between the N- and C-terminal domains in SpaP (Heim et al., 2014). As the structure of the N-terminal domain in other homologous proteins has not been experimentally determined thus far, the level of conservation of such a locking mechanism remains to be seen.
Figure 2. Comparison of SpaP and BspA Protein Structures. (A) PyMOL was used to combine the published models for domains of SpaP (N-terminus PDB:4TSH, A3VP1 PDB:3IOX, C-terminus PDB:6E3F) and BspA (V-domain, C-terminus PDB:5DZA). Additional SpaP A and P repeats were modeled from A3VP1, as were the A- and P-domains for BspA. As there is no structure for the N-terminal domain of any AgI/II protein among the cluster of invasive species shown in Figure 1A, the BspA N-terminus is depicted by an oval. Light blue indicates the N-terminal domain, purple indicates the A-domain, green indicates the V-domain, yellow indicates the P-domain, and the shades of orange indicate different globular domains at the C-terminus. The V-domain of SpaP (B) and BspA (C) have been enlarged with arrows pointing to binding pockets.
In addition to projecting the V-domain away from the cell surface to improve interactions with ligands, this stable stalk structure allows for a degree of flexibility that is known to promote bacterial attachment under shear conditions. While this tertiary structure is highly conserved, there is regional variability particular to individual species, especially at the V-domain. Interestingly, the full-length protein sequences used to generate the phylogenetic tree shown in Figure 1A cluster into a group of viridans streptococci and a separate group of more traditionally invasive species. Representatives of these two AgI/II protein structures, SpaP from S. mutans and BspA from GBS are shown in Figure 2. These two species were chosen because each has the largest number of domains with experimentally determined structures published within each cluster. The structural differences between homologs contribute to both overlapping and distinct functions for AgI/II proteins within streptococci. For example, AgI/II proteins from some species are able to bind to sialic acids, while those from other species cannot (Demuth et al., 1990a, b; Soell et al., 1994; Vernier et al., 1996; Chatenay-Rivauday et al., 1998). Structural differences exist even within the phylogenetic clusters, as seen by variations in the published V-domain structure from S. gordonii SspB (Forsgren et al., 2009) from that of SpaP. These types of species-dependent variations in AgI/II function will be discussed in greater detail in the following sections. It is likely that some of these functional variations also depend on structural differences in domains other than the V-domain. While the full structure of SpaP has been mostly solved (Heim et al., 2014, 2015), other than the C-domain of S. pyogenes AspA (Hall et al., 2014) and GBS BspA V- and C-domains (Rego et al., 2016), the structures of additional AgI/II domains from the cluster of invasive streptococci have not been experimentally determined. A better understanding of the structural differences between these AgI/II orthologs may reveal key insights regarding their unique mechanisms for colonization or disease progression. Additionally, the structure of Enterococcus faecalis Asc10 displays a primary structure akin to that of AgI/II proteins (Waters et al., 2004; Chuang et al., 2009). The region analogous to the AgI/II V-domain of Asc10 was recently shown to have a similar structure to the V-domain of S. gordonii SspB (Schmitt et al., 2018). As such, gaining a better understanding of the AgI/II structure may indirectly provide information regarding the mechanisms for pathogenesis utilized by other microorganisms as well.
One well known function of AgI/II proteins is their direct contribution to auto-aggregation of streptococci without intermediate molecules (Demuth et al., 1990a; Jenkinson and Demuth, 1997; Brady et al., 2010; Maddocks et al., 2011; Chuzeville et al., 2017; Deng et al., 2019). In recent years, it has also been shown that fragments of S. mutans SpaP interact with each other to form functional amyloid fibers (Oli et al., 2012). This discovery prompted investigations regarding the molecular interactions contributing to this phenomenon. As mentioned earlier, AgI/II proteins are known to fragment, releasing what was previously known as antigen II. These released fragments can interact with surface anchored AgI/II proteins, particularly through the V-domain of the surface anchored protein with either the V- or C-domains of the fragmented protein (Heim et al., 2015; Rego et al., 2016; Tang et al., 2016; Rivière et al., 2020). AgI/II fragments comprised of the C-domain are able to form the functional amyloid fibers in vitro (Tang et al., 2016; Barran-Berdon et al., 2020). Furthermore, AgI/II functional amyloids contribute to stabilization of S. mutans biofilms (Oli et al., 2012; Tang et al., 2016; Barran-Berdon et al., 2020). Together, this information suggests a critical role of AgI/II protein interactions for the stabilization of streptococcal communities, as well as in the formation of a structural foundation in biofilms that may be taken advantage of by other species. The direct role of AgI/II proteins in polymicrobial biofilms at various tissues will be discussed below.
In addition to auto-interactions, AgI/II proteins are able to interact with a large number of host ligands. As a class of multifunction adhesins, AgI/II proteins play a role in streptococcal interactions with extracellular matrix (ECM), or canonical ECM proteins outside of the context of ECM, as seen with some components of the salivary pellicle. These interactions potentially facilitate adherence to both mucosal surfaces such as the lung or vaginal epithelium, as well as non-mucosal surfaces such as endothelial cells or teeth. Thus far, interactions with the fibrous components of the ECM has been a major focus of research, especially collagen, fibrinogen, fibronectin, and laminin. As collagen is a major component of dentin (Linde, 1989), there has been a large interest in collagen-binding proteins and their role in mediating streptococcal access and invasion into tooth surfaces. It has been shown that S. gordonii SspA and SspB (Love et al., 1997; Heddle et al., 2003), S. suis AgI/II (Chuzeville et al., 2017), and S. mutans SpaP (Love et al., 1997; Sciotti et al., 1997; Beg et al., 2002; Petersen et al., 2002; Sullan et al., 2015; Esberg et al., 2017) interact with and promote adherence to surfaces coated with type I collagen, while S. intermedius Pas does not (Petersen et al., 2001, 2002). Some of these studies utilized human collagen (Sciotti et al., 1997; Beg et al., 2002; Chuzeville et al., 2017), while others used rat tail collagen (Love et al., 1997; Sullan et al., 2015). This is significant because a study that used type I collagen from both human and rat tail found that S. mutans SpaP interacts with rat and not human collagen (Petersen et al., 2002). Differences in experimental set up might explain these contradictory results, but additional investigation is required to definitively determine the role of an AgI/II – collagen interaction within the setting of human disease.
Streptococcal binding of laminin may also play a role in colonization or invasion of various tissues (Singh et al., 2012). Studies have shown that in addition to S. intermedius Pas (Petersen et al., 2001), S. mutans SpaP (Sciotti et al., 1997; Busscher et al., 2007) also interacts with laminin. Another study saw a minor decrease in the ability of a S. mutans SpaP knockout to bind human laminin but as the difference was not significant, the authors concluded that SpaP did not contribute to laminin binding (Beg et al., 2002); thus, additional investigations into the interactions of AgI/II proteins with laminin are still needed. Certain streptococcal species are also major agents of infective endocarditis (IE), and the ability to bind fibronectin and fibrinogen in addition to collagen may contribute to development of this disease (Baddour, 1994). S. mutans SpaP (Sciotti et al., 1997; Beg et al., 2002; Petersen et al., 2002; Kelemen et al., 2004; Sullan et al., 2015), S. intermedius Pas (Petersen et al., 2001, 2002), and S. suis AgI/II (Chuzeville et al., 2017) have all been shown to bind fibronectin. Additionally, S. mutans SpaP (Beg et al., 2002; Kelemen et al., 2004), S. suis AgI/II (Chuzeville et al., 2017), and GBS BspC (Chuzeville et al., 2015) have been shown to bind fibrinogen. The role of AgI/II proteins in binding of other ECM components such as elastin is still a significant knowledge gap that warrants future investigation.
Direct AgI/II interactions with host cells are also well documented. These proteins mediate attachment to epithelial (Vernier et al., 1996; Sciotti et al., 1997; Chuzeville et al., 2017; Chaffanel et al., 2018; Pidwill et al., 2018; Jain et al., 2019) and endothelial (Vernier et al., 1996; Al-Okla et al., 1999; Deng et al., 2019; Oho and Nagata, 2019) cells, in addition to monocytes and fibroblasts (Soell et al., 1994; Chatenay-Rivauday et al., 1998, 2000; Petersen et al., 2001; Hajishengallis et al., 2002; Engels-Deutsch et al., 2003). The diversity of different cell types that streptococci adhere to using AgI/II proteins highlights their dynamic role as adhesins. One ligand for S. mutans SpaP that was identified early on is the intermediate filament (IF) protein, keratin (Sciotti et al., 1997). More recently, vimentin, a type III IF protein, was also identified as the GBS BspC receptor mediating adherence to human cerebral microvascular endothelial cells (hCMECs) (Deng et al., 2019). Despite the ubiquitous nature of IF proteins as structural components in all cell types, they remain severely understudied as receptors for AgI/II proteins, and future studies should investigate the role of potential IF-AgI/II interactions in pathogenesis.
Salivary agglutinin (SAG) is perhaps the best documented host receptor for AgI/II proteins and will be discussed in a dedicated section below; however, these investigations have revealed the lectin-like properties of AgI/II proteins. For example, sialic acid (and to a lesser degree, fructose, and mannose) binding by S. sanguinis SSP-5 competitively inhibits aggregation and interaction with SAG (Demuth et al., 1990a). Conversely, S. mutans SpaP interaction with SAG was inhibited by fucose and lactose, but not by sialic acid (Demuth et al., 1990b). Interestingly, S. mutans AgI/II was shown to bind N-acetylneuraminic acid (a sialic acid) in addition to fucose on the surface of monocytes and KB cells (Soell et al., 1994; Vernier et al., 1996; Chatenay-Rivauday et al., 1998). Additionally, the V-domain of AgI/II proteins is thought to contain a carbohydrate-binding pocket (Troffer-Charlier et al., 2002; Forsgren et al., 2009; Rego et al., 2016), and another cleft between two of the globular C-terminal domains of SpaP was shown to bind glucose (Larson et al., 2011). Collectively, this information indicates that while members of the AgI/II family of proteins have similar and often overlapping specificity for host factors, many of these interactions may be unique to both the AgI/II protein expressed in certain species, as well as the cell type in which the interaction is taking place.
AgI/II proteins are also able to interact with a number of receptors involved in immune signaling. S. gordonii SspA and SspB have been shown to interact with α5β1 integrins on human lung epithelial A549 cells, HEp-2 cells, and coated plates (Nobbs et al., 2007; Andrian et al., 2012). Furthermore, the interaction between SspA/SspB with α5β1 integrins was shown to be mediated via the N-terminal portion of the protein, and this interaction contributed to invasion into HEp-2 cells in addition to adherence (Nobbs et al., 2007). Due to the widespread expression of α5β1 integrins in multiple cell types, it is likely that their interaction with AgI/II proteins contributes to adherence and invasion in other cell types as well, but this has yet to be confirmed.
AgI/II proteins are able to interact with or evade the host immune system, further impacting disease. One main niche where this occurs is within the bloodstream, as streptococci are often associated with blood-borne systemic diseases. AgI/II proteins have anti-phagocytic functions that may promote survival within the host and development of high levels of bacteremia. An S. suis AgI/II deficient mutant was more readily internalized by resident peritoneal macrophages but had no difference from wildtype in intracellular survival (Auger et al., 2019). The knockout strain also displayed increased internalization by dendritic cells, along with decreased intracellular survival within the dendritic cells. Furthermore, the S. suis AgI/II knockout was killed more readily when exposed to whole murine blood, collectively resulting in a dramatic difference in both rapid clearance of the knockout strain from murine blood and mouse survival upon intraperitoneal or intravenous infection (Auger et al., 2019). Similarly, deletion of AspA in GAS led to increased killing by the human neutrophil (HL60) and mouse macrophage (J774.2) cell lines, while expressing AspA in L. lactis led to decreased killing by the same cell lines (Franklin et al., 2013). Interestingly, heterologous expression of S. gordonii SspB within L. lactis had no impact on phagocytosis, indicating species specific differences in the sufficiency of AgI/II proteins to impact phagocytosis. This is also supported by the fact that the opposite trend was observed with S. mutans PAc, where a PAc knockout and S. mutans clinical strains with less AgI/II surface expression exhibited a decreased ability to be phagocytosed by human polymorphonuclear leukocytes (Nakano et al., 2006). Furthermore, the PAc deficient strain was not cleared as efficiently as wildtype S. mutans after intravenous injection within a rat model of bacteremia.
AgI/II proteins have also been shown to modulate immune responses while in the bloodstream. In the same rat model for bacteremia that was mentioned above, significantly higher concentrations of serum sialic acid [a marker for systemic inflammation (Rajendiran et al., 2014)] were observed after injection with the PAc deficient mutant as compared to the wildtype control (Nakano et al., 2006). In this case, presence of the AgI/II protein appeared to dampen inflammation, although the opposite was observed in other S. mutans studies. As mentioned earlier, AgI/II proteins interact with THP-1 monocytes derived from human peripheral blood. S. mutans SpaP was able to induce release of the neutrophil chemoattractant IL-8 by THP-1 cells (Engels-Deutsch et al., 2003). SpaP also induced the pro-inflammatory cytokines TNFα and IL-1β from THP-1 cells, which was partially mediated by CD14 and TLR4, but not significantly by TLR2 (Hajishengallis et al., 2002). S. mutans Sr was similarly shown to bind human monocytes and induce the release of TNF, IL-1, and IL-6 (Soell et al., 1994). These seemingly contradictory results indicate complex interactions that strike a delicate balance with the host immune system. The mechanistic details regarding the contribution of S. mutans AgI/II to immune suppression or activation should be investigated in greater detail.
Despite the controversy involved with SpaP, most other AgI/II proteins appear to induce a pro-inflammatory response by cells found within the blood. S. intermedius Pas has been shown to induce IL-8 from THP-1 cells (Petersen et al., 2001). S. gordonii SspA and SspB both also induce TNF, IL-6, IL-10, and IL-12 release by bone marrow derived dendritic cells (Andrian et al., 2012). Intraperitoneal infection of mice with a S. suis AgI/II mutant strain led to decreased levels of a large number of pro-inflammatory mediators within the plasma, including IL-6, IL-12p70, IFN-γ, CCL2, CCL3, CCL4, CXCL1, and CXCL2 (Auger et al., 2019). Additionally, TLR2 and TLR4 were shown to contribute to MyD88 dependent induction of TNF, IL-1β, IL-6, and CCL3 in dendritic cells (Auger et al., 2019). TLR2 and TLR4 both interact with CD14 to sense extracellular factors and begin signal transduction (Arroyo-Espliguero et al., 2004). One study showed that THP-1 cells treated with vitamin D3 (known to increase expression of CD14) were not stimulated to a higher degree by S. mutans SpaP and concluded that CD14 was not a receptor for the protein (Chatenay-Rivauday et al., 1998); however, another study soon after showed that monoclonal antibodies specific to CD14 and TLR4 (but not TLR2), both decreased immune activation by SpaP in THP-1 cells (Hajishengallis et al., 2002). This indicates that SpaP does likely interact with CD14, but requires the intracellular signaling domain of TLR4 to induce the expected immune responses. Collectively, this information suggests an overwhelmingly pro-inflammatory response to AgI/II proteins by white blood cells. Still, as there is evidence for immune suppressive or anti-phagocytic characteristics of some of the AgI/II proteins, it remains possible that these AgI/II proteins have similarly complex interactions with the immune system under conditions or niches that have yet to be investigated.
AgI/II proteins also promote inflammatory signaling in other cell types, including epithelial and endothelial cells. As mentioned previously, AgI/II proteins can bind α5β1 integrins, which are known to sense the extracellular environment through adhesive interactions and initiate signaling cascades that alter a variety of host processes (Winograd-Katz et al., 2014). S. mutans SpaP is able to bind to α5β1 integrins on human saphenous vein endothelial cells (HSVECs) and osteoblasts, contributing to inflammatory signaling (Al-Okla et al., 1999; Son et al., 2012). AgI/II binding of α5β1 on the HSVEC surface was shown to induce the MAPK signaling pathway and tyrosine phosphorylation of phospholipase Cγ, focal adhesion kinase, and paxillin (Al-Okla et al., 1999). This eventually leads to IL-8 release in a PI3K independent manner. SpaP induction of HSVEC IL-8 release was confirmed by another study as well, along with release of IL-6 (Vernier et al., 1996). S. gordonii SspA and SspB also increased IL-6, MCP-1 and IL-8 production in A549 cells, and induction of both IL-6 and MCP-1 could be inhibited with antibodies that blocked β1 integrin. Furthermore, SspA and SspB induced IL-6 and IL-8 within HEp-2 cells, as well as CXCL1 and CXCL2 in lung homogenates (Andrian et al., 2012). Similarly, the GBS AgI/II protein known as BspC was shown to induce inflammatory signaling in hCMECs leading to meningitis-associated inflammation. BspC induced IL-8, IL-1β, and CXCL1 release by hCMECs, and also KC protein and IL-1β within a murine model of hematogenous meningitis (Deng et al., 2019). This is a clear case where the AgI/II mediated inflammatory signaling contributes to disease. Given the large body of data showing that similar immune induction occurs at other body sites, the direct role of AgI/II proteins in inflammatory disease should be investigated in greater detail.
As mentioned previously, due to the initial discovery of AgI/II proteins in S. mutans, the AgI/II proteins of streptococci that colonize the oral cavity are the most well studied. Within the oral cavity, AgI/II proteins are known to interact with one of the host innate defense proteins known as salivary agglutinin (SAG). Initial studies identified that S. mutans SpaP (Brady et al., 1992; Hajishengallis et al., 1994; Sciotti et al., 1997; Ahn et al., 2008), S. sanguinis PAc (Demuth et al., 1988, 1990a; Lamont et al., 1991), S. sobrinus SpaA (Lamont et al., 1991), and S. gordonii SspA (Jenkinson et al., 1993) all interacted with fluid-phase SAG, resulting in aggregation. The aggregation acts as a defense mechanism in this case as it promotes clearance of bacteria through actions such as swallowing. It has since been discovered that SAG and the lung scavenger receptor protein known as glycoprotein-340 (gp340) [also known as Deleted in Malignant Brain Tumors 1 or DMBT1 (Mollenhauer et al., 1997)] are the same protein (Prakobphol et al., 2000). In the context of mucosal colonization, the protein has historically been referred to as either SAG or more recently gp340, so it will be simply referred to as SAG/gp340 in this review. Within the oral cavity, SAG/gp340 can exist in fluid phase as a salivary component, or in an adsorbed phase that can mediate bacterial attachment to the tooth surface, thereby contributing to dental plaque biofilm formation (Madsen et al., 2010). Interestingly, it seems that AgI/II proteins have varying interactions with these two phases of SAG/gp340. S. gordonii SspA and SspB, S. intermedius Pas and S. mutans SpaP all contributed to aggregation by fluid-phase SAG/gp340 at comparable levels, but only the AgI/II proteins of S. gordonii mediated higher affinity binding to surface-bound SAG/gp340 (Loimaranta et al., 2005; Maddocks et al., 2011). Likewise, while GAS AspA contributes to attachment to immobilized SAG/gp340, it did not cause aggregation by fluid-phase SAG/gp340 (Maddocks et al., 2011). The AgI/II protein of S. suis has also been shown to interact with both fluid-phase and immobilized SAG/gp340, contributing to both aggregation and surface adherence, respectively (Chuzeville et al., 2017). While GAS and S. suis are not common colonizers of the oral cavity, the ability for their AgI/II proteins to interact with SAG/gp340 highlights the conservation of this particular function.
An early study using monoclonal blocking antibodies revealed that binding to fluid-phase and surface-immobilized SAG/gp340 is mediated by different regions of the AgI/II protein (Brady et al., 1992). This was the first indication that different portions of SAG/gp340 are accessible to AgI/II binding in its two physical states. Multiple studies have investigated this phenomenon in more detail, specifically examining the scavenger-rich cysteine repeat (SRCR) domain of SAG/gp340 that is targeted by AgI/II proteins (Bikker et al., 2002, 2004). It was shown that immobilized SRCR underwent a conformational change in the presence of calcium that allowed for AgI/II protein interaction with nanomolar affinity, whereas AgI/II protein interacted with fluid-phase SRCR with micromolar affinity (Purushotham and Deivanayagam, 2014). Additionally, SAG/gp340 size variants exist that have altered interactions with AgI/II proteins from different species (Jonasson et al., 2007). Furthermore, alternate AgI/II V-domain segments within a group of S. mutans human isolates were shown to confer differential saliva- and SAG/gp340-mediated adherence (Esberg et al., 2012, 2017). Taken together, this information suggests a complex co-evolution of AgI/II proteins and SAG/gp340. While fluid-phase SAG/gp340 normally functions as part of host innate immunity to cause aggregation and clearance of oral streptococci, certain species have evolved to interact with the alternative conformation of immobilized SAG/gp340 on tooth surfaces to promote adherence instead. This provides a mechanism by which both commensal and cariogenic streptococci maintain colonization of the oral cavity. The role of interactions between AgI/II proteins with SAG/gp340 in other diseases will be discussed further on.
Streptococcal AgI/II proteins are able to interact with other microbes known to colonize the oral cavity, contributing to polymicrobial biofilm formation. Often, these interactions stabilize the colonization of pathogens associated with the development of periodontitis or dental caries. While SAG/gp340 has been shown to increase adherence of both S. mutans and S. sobrinus to immobilized early plaque formers such as S. sanguinis and Actinomyces viscosus, both species were also shown to adhere to the plaque formers in an AgI/II dependent manner, even without the addition of saliva or purified SAG/gp340 (Lamont et al., 1991). S. gordonii SspA and SspB have similarly been shown to interact with Actinomyces oris (Back et al., 2015) and the periodontitis-associated pathogen Porphyromonas gingivalis (Brooks et al., 1997; Daep et al., 2006). In the case of P. gingivalis, it was determined that the minor fimbrial antigen (Mfa1) of P. gingivalis interacts with a specific sequence motif found in the C-terminal domain of the SspA/B proteins which is not highly conserved in SpaP (Demuth et al., 2001; Daep et al., 2006; Forsgren et al., 2010). The crystal structure of SspB revealed that this region protrudes from the protein, creating a handle for initial attachment of P. gingivalis and inclusion into a biofilm (Forsgren et al., 2010). Peptides mimicking this region have been shown to block the SspB-Mfa1 interaction and inhibit P. gingivalis virulence within a murine model for periodontitis (Daep et al., 2011). Furthermore, small molecule inhibitors were recently identified that similarly block the SspB-Mfa1 interaction and attenuate the ability of P. gingivalis to cause periodontitis in vivo (Roky et al., 2020). S. gordonii SspA and SspB, S. intermedius Pas, and S. mutans SpaP have also all been shown to contribute to aggregation with Actinomyces naeslundii, another organism associated with periodontitis (Jakubovics et al., 2005b). Additionally, S. mutans SpaP mediates interactions with Fusobacterium nucleatum ssp. polymorphum, which in turn is known to initiate adherence of other periodontal pathogens (Guo et al., 2017).
AgI/II proteins also contribute to interactions with pathogens associated with dental caries. For example, Lactobacillus casei is frequently isolated from dental caries. Co-culturing L. casei with S. mutans increases the ability of L. casei to form biofilms, and this increase was shown to be SpaP dependent (Wen et al., 2010, 2017). Additionally, the fungal pathogen Candida albicans is commonly associated with oral streptococcal biofilms, and its presence has recently been shown to be positively correlated with cariogenic traits of S. mutans in caries-related biofilms (Falsetta et al., 2014; Bachtiar and Bachtiar, 2018). AgI/II proteins are known to interact with the Agglutinin-like sequence family proteins Als1 and Als3 of C. albicans, contributing to the formation of polymicrobial communities. For example, S. gordonii SspB interactions with C. albicans Als3 have been shown to contribute to biofilm formation (Bamford et al., 2009; Silverman et al., 2010). S. mutans SpaP was also shown to be important for incorporation of C. albicans within a two-species biofilm, as well as for acid production within the biofilm, which may contribute to the progression of dental caries (Yang et al., 2018).
Despite the body of work indicating the role of AgI/II proteins in polymicrobial biofilm formation with oral pathogens, it has been shown that S. mutans spaP is significantly downregulated within two-species biofilms with S. sanguinis, S. oralis or L. casei (Wen et al., 2010). Very little is known regarding the regulation of AgI/II family proteins, but this indicates that at least within the context of biofilm formation, the regulation of AgI/II proteins is complex and warrants further investigation. Additionally, while it is known that AgI/II proteins contribute to oral disease through adherence or even invasion of dentinal tubules (Love et al., 1997), the role of AgI/II proteins in the immune response within the oral cavity remains largely unexplored.
Streptococci are often colonizers of the nasopharyngeal tract and are a common cause of lung infections. The role of AgI/II proteins in streptococcal persistence within these two niches has been characterized mainly with S. suis, S. gordonii, and GAS. There is serotype-specific variation in the S. suis AgI/II contribution to respiratory infection. The S. suis AgI/II protein contributes to adherence to NPTr porcine tracheal cells in a serotype 9 strain, but not in a serotype 2 strain (Chuzeville et al., 2017). A mutant lacking the AgI/II protein in the serotype 9 strain also displayed decreased nasal cavity and tonsil bacterial burden within a porcine model for respiratory infection (Chuzeville et al., 2017). As S. suis is also able to cause human infections, it would be of interest to examine this in models for human disease. Studies with S. gordonii have used human cell-based models for disease. Some of these were done using HEp-2 cells which were initially thought to be derived from a human laryngeal epithelial carcinoma, however, it has been shown that the cell line was actually the result of HeLa cell contamination (Gartler, 1968). In order to provide historical context, these results will still be mentioned. S. gordonii SspA and SspB contribute to adherence and invasion of the HEp-2 cell line (Jakubovics et al., 2005a; Nobbs et al., 2007; Andrian et al., 2012). As mentioned earlier, this was shown to be mediated by recognition of β1 integrin by SspA and SspB (Nobbs et al., 2007). SspA and SspB contribute to S. gordonii adherence to a lung epithelial cell line (A549) through β1 integrin as well (Andrian et al., 2012). Additionally, AspA contributes to GAS colonization of the nasopharynx, as seen by its role in adherence to Detroit 562 laryngeal epithelial cells (Franklin et al., 2013). An AspA deficient mutant displayed decreased nasopharyngeal and lung bacterial burden within a murine model of respiratory infection (Franklin et al., 2013). While AspA has also been linked to innate immunity through repression of phagocytic killing (as discussed previously), continued investigation is required to determine its role in immune modulation specifically within the respiratory tract. Additionally, while β1 integrin was shown to be a receptor for SspA and SspB in HEp-2 cells, host respiratory epithelial cell receptors for AgI/II proteins have not been thoroughly characterized; however, since SAG/gp340 is known to be expressed in the lungs, this would be one logical interaction to examine.
Numerous streptococcal species are known to colonize the female reproductive tract. As seen with GBS, this can result in pregnancy-associated complications such as pre-term births. AgI/II proteins are beginning to be implicated in streptococcal colonization of these tissues as well. Recently, the role of the GBS Bsp proteins in colonization of the female reproductive tract has been described. Deletion of BspC from GBS strain 515 resulted in a modest decrease in adherence to vaginal epithelial cell (VECs); however, pretreatment with Bsp antisera resulted in a large decrease in adherence as compared to the preimmune sera control treatment (Pidwill et al., 2018). Additionally, heterologous expression of both BspA and BspC within Lactococcus lactis was shown to confer increased adherence to a VEC monolayer (Rego et al., 2016; Pidwill et al., 2018).
The GBS AgI/II proteins are also known to contribute to single species biofilm formation (Chuzeville et al., 2015), but there is evidence that these proteins mediate polymicrobial interactions as well. As mentioned earlier, AgI/II proteins are able to interact with the fungal pathogen C. albicans, which is also often co-isolated with GBS from the vaginal tract (Monif and Carson, 1998; Bayó et al., 2002; Cools et al., 2016). BspA and BspC were shown to mediate direct interaction between GBS and C. albicans (Rego et al., 2016; Pidwill et al., 2018). Furthermore, adherence of both GBS and L. lactis surrogate expression strains to VECs in the presence of C. albicans was largely mediated by expression of BspA and BspC. The association between GBS and C. albicans is dependent on the Als3 protein of C. albicans (Pidwill et al., 2018). Interestingly, Als3 is the same protein that was discussed earlier as being the receptor for AgI/II proteins of oral streptococci, indicating that these interactions are not niche specific. These experiments were all conducted in vitro. As such, the role of AgI/II interactions with C. albicans within the female reproductive tract in vivo remains unexplored.
Other reports of AgI/II interactions within the female reproductive tract are not as direct or as thoroughly studied as the Bsp interaction with vaginal epithelium, as is seen with GAS. Colonization of the genitourinary tract by GAS is a risk factor for puerperal sepsis, and it is possible that the GAS AgI/II protein contributes to colonization of this niche. GAS adherence to vaginal epithelium and colonization of murine vaginal tract was shown to be mediated by genes located on RD2 (Jain et al., 2019). As mentioned earlier, GAS RD2 contains the AgI/II protein AspA. The individual contribution of AspA to vaginal colonization, however, has not yet been shown. Future studies should additionally aim to determine what, if any, impact AgI/II proteins have on streptococcal ascending infection or immune modulation resulting in adverse pregnancy outcomes.
Several streptococcal species are able to penetrate the blood-brain barrier (BBB) in order to cause meningitis, including S. pneumoniae, S. suis and GBS (Doran et al., 2016). Only in GBS has the role of AgI/II been investigated. A recent study revealed that BspC promoted GBS adherence to hCMECs, a model of the BBB, by interacting with the C-terminus of the intermediate filament protein known as vimentin (Deng et al., 2019). BspC itself induced meningitis-associated inflammation, as discussed in the immune signaling section, and the BspC-vimentin interaction was also necessary for disease progression. A BspC-deficient mutant was unable to cause meningitis within a murine model, while vimentin-deficient mice were protected from infection by wildtype GBS. Furthermore, vimentin was shown to localize to the cell surface in response to interaction with BspC, although the mechanisms governing this reorganization are not yet understood. While S. suis AgI/II contributions to systemic infections (discussed within the “Interactions With the Immune System” section) have been previously investigated, their role specifically within central nervous system infections should be examined in future studies.
Another major example of AgI/II proteins interacting with host endothelial cells is during infective endocarditis (IE), which is characterized by the formation of a bacteria-containing vegetation on a heart valve (Ford and Douglas, 1997). Viridans group streptococci are the second largest cause of IE (Rajani and Klein, 2020) and as such, the role of AgI/II proteins in promoting IE has been widely investigated. The characteristic vegetation in IE is typically composed of fibronectin and fibrinogen, bacteria, and aggregated platelets (Baddour, 1994; Ford and Douglas, 1997). As mentioned previously, many AgI/II proteins are known to interact with both fibrinogen and fibronectin. In addition, S. mutans PAc binds platelets and causes their aggregation (Matsumoto-Nakano et al., 2009). S. gordonii SspA and SspB have also been shown to bind human erythrocytes (Demuth et al., 1996) and contribute to platelet aggregation, although they do not directly mediate platelet adhesion (Kerrigan et al., 2007).
The role of AgI/II proteins in interacting with endothelial cells is also relevant here, although many of these studies have used cell lines originating from regions distal to the heart. Interactions with brain endothelium were described in the previous section, but SpaP has also been shown to contribute to S. mutans adherence to the human saphenous vein endothelial cell (HSVEC) line (Vernier et al., 1996; Al-Okla et al., 1999). One study used human aortic endothelial cells (HAECs) to model S. mutans interactions during IE, and while they did not directly investigate the role of AgI/II proteins, they instead looked at expression of known AgI/II protein receptor DMBT1 (SAG/gp340) in response to infection (Oho and Nagata, 2019). The study found that SAG/gp340 release was upregulated in response to S. mutans infection, but this actually decreased adherence and invasion of the HAECs as compared to a SAG/gp340 knockdown cell line. In this case, it seems that the host utilizes the protein in a similar manner to fluid-phase SAG/gp340 to aggregate and promote clearance of S. mutans.
Interestingly, despite the role of AgI/II proteins in interacting with platelets, erythrocytes, and endothelial cells, as well as elevated levels of anti-PAc antibody titers in IE patients (Russell et al., 1992), the only study examining AgI/II proteins in an in vivo model of IE found no phenotype for an AgI/II-deficient mutant (Ryd et al., 1996). The study found no statistically significant difference in CFUs present in heart valve vegetations between the mutant and wildtype S. mutans strains 1-hour post inoculation, although there was a trend toward fewer CFU of the mutant strain. While they observed no difference in bacterial clearance from the blood, CFU counts were only examined for 1 hour post infection, which may have been too early in disease progression. Nonetheless, at 48 hours post infection, they still observed no difference between the wildtype and AgI/II-deficient mutant in CFU counts recovered from heart valve vegetations. Despite these results, due to the numerous differences between AgI/II protein interactions from different species that have been described thus far, revisiting an in vivo model for IE to investigate the contribution of AgI/II proteins from other species may provide novel insights into the development of the disease. Additionally, it is also possible that AgI/II proteins mediate IE disease through an altered immune response instead of through direct adherence. A recent study showed that there is a high level of peptide similarity between the S. mutans AgI/II protein and cardiovascular autoantigens (Lucchese, 2017). The role of the elevated anti-AgI/II antibody titers (Russell et al., 1992) in recognizing these autoantigens to promote IE should be investigated.
Given the growing recognition of their role in promoting streptococcal colonization and pathogenesis, the potential for AgI/II proteins to serve as therapeutic targets warrants consideration. Reflecting the timeline for discovery of this protein family, such investigations to date have primarily focused on exploiting the AgI/II protein of S. mutans to develop an anti-caries vaccine. Since it was first shown that antibodies against SpaP can impair adherence of S. mutans to SAG immobilized on the tooth surface (Hajishengallis et al., 1992), numerous vaccine strategies have been explored, with the ultimate goal of inducing a mucosal immune response that inhibits S. mutans colonization of the oral cavity. Approaches include immunization with recombinant SpaP peptides or chimeric proteins incorporating additional S. mutans antigens (Lehner et al., 1981; Katz et al., 1993; Yu et al., 1997; Hajishengallis et al., 1998; Zhang et al., 2002; Batista et al., 2017), attenuated Salmonella enterica serovar Typhimurium expressing a SpaP epitope (Huang et al., 2001; Jiang et al., 2017), or DNA vaccines (Guo et al., 2004; Jia et al., 2004, 2020; Shi et al., 2012), alongside passive immunotherapy studies using monoclonal antibodies (Lehner et al., 1985, 1992). While most of these studies have been at the preclinical phase using animal models, human studies involving topical application to the tooth surface of anti-SpaP monoclonal antibodies were shown to be effective in preventing colonization by exogenously administered or indigenous S. mutans (Ma et al., 1989, 1998). Protection was reported to last for up to 2 years (Ma and Lehner, 1990; Ma et al., 1990), although others failed to replicate such long-term effects (Weintraub et al., 2005), and no follow-up trials have been performed in recent years. Alongside the technical difficulties of vaccine development, another challenge with an anti-caries vaccine is that S. mutans is not the only cariogenic bacterium within the oral microbiota, which raises the question of the efficacy of targeting a single bacterium. However, this issue is less of a concern for the development of a vaccine to combat, for example, GBS disease. Sialylated capsular polysaccharide (CPS) has been a major focus of GBS vaccine strategies to date (Kobayashi et al., 2016). However, there are 10 distinct CPS serotypes, as well as non-typeable strains, meaning that any such vaccine design could face the challenge of potential serotype replacement/switching across geographical sites and over time. Vaccines comprising a combination of protein-based antigens have potential to confer broad protection that is serotype-independent, and these are currently under investigation. Based on SpaP, the Bsp proteins or domains thereof might well be anticipated to induce function-blocking antibodies, while their unique structural features offer the potential for the identification of epitopes that allow the selective targeting of GBS while not impacting other streptococci within the resident microbiota at a given ecological niche.
Alongside vaccination strategies, improved knowledge of structure-function relationships across the AgI/II proteins offers the potential for mimetics or small molecule inhibitors to be developed as a therapeutic route. As mentioned, proof-of-concept has been established with use of a peptide to block the interaction of S. gordonii AgI/II with P. gingivalis Mfa1, resulting in reduced alveolar bone resorption in a murine model of periodontitis (Daep et al., 2011). More recently, this peptide has been incorporated into polymeric nanoparticles to improve peptide dosage and persistence within the oral cavity (Mahmoud et al., 2018), while small molecule inhibitors of the AgI/II-MfaI engagement have also been found to effectively reduce P. gingivalis virulence (Roky et al., 2020). Protective effects have also been shown for a peptide (p1025) that blocks S. mutans AgI/II adhesion to SAG. In a human trial, topical application of p1025 to the teeth over a 3-week period prevented recolonization by S. mutans for up to 4 months but not by another member of the resident oral microbiota, Actinomyces (Kelly et al., 1999). With our growing understanding of the importance of the AgI/II protein family amongst pathogenic streptococci, opportunities for development of therapeutic agents that target them should remain an important area of investigation.
The AgI/II family of proteins display both overlapping and diverse functions that vary between streptococcal species as well as the niche within the host (Figure 3). The protein structure itself has many unique features, with multiple intramolecular interactions forming an elongated fibrillar stalk that projects the V-domain containing a binding cleft away from the cell surface. This cleft may be used to promote streptococcal colonization of various tissues via both direct cellular adherence or interaction with ECM components. Here we provide a historical perspective of the existing literature and as such, the data presented represent over 40 years of work. During this time, the field has progressed significantly. Some of the pitfalls in the earlier studies that have become clear in hindsight were discussed. In general, validated cell lines, antibodies, recombinant proteins made with the benefit of current structural knowledge, and other modern tools and techniques could provide clarification or new insights into some of the previously identified functions of AgI/II proteins. In addition to the roles for AgI/II proteins in mucosal colonization and blood-borne diseases that were discussed, there are also functions that have yet to be investigated in detail. For example, SspA and SspB protect S. gordonii from the antibiotic polymyxin B, as well as the antibacterial peptides nisin and histatin-5 (Andrian et al., 2012). AgI/II proteins may also play a role in streptococcal gut colonization, as the S. suis AgI/II was shown to protect against acid stress (Chuzeville et al., 2017), and a potential AgI/II protein identified in S. salivarius contributed to adherence to intestinal epithelium (Chaffanel et al., 2018). Each of these potential functions should be further investigated. Additionally, future studies should aim to elucidate the potential for post-translational modifications of AgI/II proteins such as glycosylation to contribute to their functions. Understanding the diverse nature of these multifunctional adhesins will provide novel insights into the mechanisms by which streptococci and other organisms with structurally similar adhesins cause disease, as well as provide targets for therapeutic intervention.
Figure 3. Summary of Site-Specific AgI/II Interactions. (A) AgI/II proteins promote direct interactions with vaginal epithelial cells, and interact with Als3 protein of C. albicans, promoting fungal and streptococcal adherence to the vaginal epithelium. (B) AgI/II proteins bind fluid-phase SAG/gp340, resulting in aggregation and clearance through mechanisms such as swallowing, as well as to immobilized SAG/gp340 on the tooth pellicle, facilitating adherence and colonization. AgI/II proteins mediate direct adherence to tooth components and invasion into dentinal tubules, resulting in caries formation, and also facilitate colonization and polymicrobial biofilm formation with cariogenic pathogens, as well as with pathogens associated with periodontitis. (C) AgI/II proteins interact with vimentin on the surface of brain endothelium, and also induce chemokine signaling and neutrophil chemotaxis characteristic of meningitis. (D) AgI/II proteins interact with β1 integrin on the nasopharynx epithelium, resulting in adherence and invasion of the cells and consequently increased bacterial burden in the nasopharynx and lungs. (E) AgI/II proteins promote aggregation of blood-borne bacteria, as well as attachment to and aggregation of platelets; however, there is currently insufficient evidence to show this promotes increased vegetation formation on heart valves that is characteristic of infective endocarditis, as indicated by the question mark.
HM, AN, and KD contributed to writing, reviewing, and editing the manuscript. HM generated the table and figures. All authors contributed to the article and approved the submitted version.
This work was supported by KD’s laboratory NIH/NINDS R01 NS116716 and HM’s T32 5T32AI052066-18.
The authors declare that the research was conducted in the absence of any commercial or financial relationships that could be construed as a potential conflict of interest.
The authors appreciate the contribution of all of the researchers whose work has been reviewed here as well as those whose work may not have been discussed in detail due to space constraints.
Abranches, J., Zeng, L., Kajfasz, J. K., Palmer, S. R., Chakraborty, B., Wen, Z. T., et al. (2018). Biology of Oral Streptococci. Microbiol. Spectr. 6:0042. doi: 10.1128/microbiolspec
Ahn, S. J., Ahn, S. J., Wen, Z. T., Brady, L. J., and Burne, R. A. (2008). Characteristics of biofilm formation by Streptococcus mutans in the presence of saliva. Infect. Immun. 76, 4259–4268. doi: 10.1128/iai.00422-08
Al-Okla, S., Chatenay-Rivauday, C., Klein, J. P., and Wachsmann, D. (1999). Involvement of alpha5beta1 integrins in interleukin 8 production induced by oral viridans streptococcal protein I/IIf in cultured endothelial cells. Cell Microbiol. 1, 157–168. doi: 10.1046/j.1462-5822.1999.00016.x
Andrian, E., Qi, G., Wang, J., Halperin, S. A., and Lee, S. F. (2012). Role of surface proteins SspA and SspB of Streptococcus gordonii in innate immunity. Microbiology 158, 2099–2106. doi: 10.1099/mic.0.058073-0
Arroyo-Espliguero, R., Avanzas, P., Jeffery, S., and Kaski, J. C. (2004). CD14 and toll-like receptor 4: a link between infection and acute coronary events? Heart 90, 983–988. doi: 10.1136/hrt.2002.001297
Auger, J. P., Boa, A. C., Segura, M., and Gottschalk, M. (2019). Antigen I/II Participates in the Interactions of Streptococcus suis Serotype 9 With Phagocytes and the Development of Systemic Disease. Front. Cell Infect. Microbiol. 9:124. doi: 10.3389/fcimb.2019.00124
Bachtiar, E. W., and Bachtiar, B. M. (2018). Relationship between Candida albicans and Streptococcus mutans in early childhood caries, evaluated by quantitative PCR. F1000Res. 7:1645. doi: 10.12688/f1000research.16275.2
Back, C. R., Douglas, S. K., Emerson, J. E., Nobbs, A. H., and Jenkinson, H. F. (2015). Streptococcus gordonii DL1 adhesin SspB V-region mediates coaggregation via receptor polysaccharide of Actinomyces oris T14V. Mole. Microbiol. 30, 411–424. doi: 10.1111/omi.12106
Baddour, L. M. (1994). Virulence factors among gram-positive bacteria in experimental endocarditis. Infect. Immun. 62, 2143–2148.
Bamford, C. V., d’Mello, A., Nobbs, A. H., Dutton, L. C., Vickerman, M. M., and Jenkinson, H. F. (2009). Streptococcus gordonii modulates Candida albicans biofilm formation through intergeneric communication. Infect. Immun. 77, 3696–3704. doi: 10.1128/iai.00438-09
Barran-Berdon, A. L., Ocampo, S., Haider, M., Morales-Aparicio, J., Ottenberg, G., Kendall, A., et al. (2020). Enhanced purification coupled with biophysical analyses shows cross-β structure as a core building block for Streptococcus mutans functional amyloids. Sci. Rep. 10:5138. doi: 10.1038/s41598-020-62115-7
Batista, M. T., Ferreira, E. L., Pereira, G. S., Stafford, P., Maeda, D., Rodrigues, J. F., et al. (2017). LT adjuvant modulates epitope specificity and improves the efficacy of murine antibodies elicited by sublingual vaccination with the N-terminal domain of Streptococcus mutans P1. Vaccine 35, 7273–7282. doi: 10.1016/j.vaccine.2017.11.007
Bayó, M., Berlanga, M., and Agut, M. (2002). Vaginal microbiota in healthy pregnant women and prenatal screening of group B streptococci (GBS). Int. Microbiol. 5, 87–90. doi: 10.1007/s10123-002-0064-1
Beg, A. M., Jones, M. N., Miller-Torbert, T., and Holt, R. G. (2002). Binding of Streptococcus mutans to extracellular matrix molecules and fibrinogen. Biochem. Biophys. Res. Commun. 298, 75–79. doi: 10.1016/s0006-291x(02)02390-2
Bikker, F. J., Ligtenberg, A. J., End, C., Renner, M., Blaich, S., Lyer, S., et al. (2004). Bacteria binding by DMBT1/SAG/gp-340 is confined to the VEVLXXXXW motif in its scavenger receptor cysteine-rich domains. J. Biol. Chem. 279, 47699–47703. doi: 10.1074/jbc.M406095200
Bikker, F. J., Ligtenberg, A. J., Nazmi, K., Veerman, E. C., van’t Hof, W., Bolscher, J. G., et al. (2002). Identification of the bacteria-binding peptide domain on salivary agglutinin (gp-340/DMBT1), a member of the scavenger receptor cysteine-rich superfamily. J. Biol. Chem. 277, 32109–32115. doi: 10.1074/jbc.M203788200
Bleiweis, A. S., Oyston, P. C., and Brady, L. J. (1992). Molecular, immunological and functional characterization of the major surface adhesin of Streptococcus mutans. Adv. Exp. Med. Biol. 327, 229–241. doi: 10.1007/978-1-4615-3410-5_25
Brady, L. J., Crowley, P. J., Ma, J. K., Kelly, C., Lee, S. F., Lehner, T., et al. (1991). Restriction fragment length polymorphisms and sequence variation within the spaP gene of Streptococcus mutans serotype c isolates. Infect. Immun. 59, 1803–1810.
Brady, L. J., Maddocks, S. E., Larson, M. R., Forsgren, N., Persson, K., Deivanayagam, C. C., et al. (2010). The changing faces of Streptococcus antigen I/II polypeptide family adhesins. Mol. Microbiol. 77, 276–286. doi: 10.1111/j.1365-2958.2010.07212.x
Brady, L. J., Piacentini, D. A., Crowley, P. J., Oyston, P. C., and Bleiweis, A. S. (1992). Differentiation of salivary agglutinin-mediated adherence and aggregation of mutans streptococci by use of monoclonal antibodies against the major surface adhesin P1. Infect. Immun. 60, 1008–1017.
Brooks, W., Demuth, D. R., Gil, S., and Lamont, R. J. (1997). Identification of a Streptococcus gordonii SspB domain that mediates adhesion to Porphyromonas gingivalis. Infect. Immun. 65, 3753–3758.
Busscher, H. J., van de Belt-Gritter, B., Dijkstra, R. J., Norde, W., Petersen, F. C., Scheie, A. A., et al. (2007). Intermolecular forces and enthalpies in the adhesion of Streptococcus mutans and an antigen I/II-deficient mutant to laminin films. J. Bacteriol. 189, 2988–2995. doi: 10.1128/JB.01731-06
Chaffanel, F., Charron-Bourgoin, F., Soligot, C., Kebouchi, M., Bertin, S., Payot, S., et al. (2018). Surface proteins involved in the adhesion of Streptococcus salivarius to human intestinal epithelial cells. Appl. Microbiol. Biotechnol. 102, 2851–2865. doi: 10.1007/s00253-018-8794-y
Chatenay-Rivauday, C., Yamodo, I., Sciotti, M. A., Ogier, J. A., and Klein, J. P. (1998). The A and the extended V N-terminal regions of streptococcal protein I/IIf mediate the production of tumour necrosis factor alpha in the monocyte cell line THP-1. Mol. Microbiol. 29, 39–48. doi: 10.1046/j.1365-2958.1998.00881.x
Chatenay-Rivauday, C., Yamodo, I., Sciotti, M. A., Troffer-Charlier, N., Klein, J. P., and Ogier, J. A. (2000). TNF-alpha release by monocytic THP-1 cells through cross-linking of the extended V-region of the oral streptococcal protein I/II. J. Leukoc. Biol. 67, 81–89. doi: 10.1002/jlb.67.1.81
Chuang, O. N., Schlievert, P. M., Wells, C. L., Manias, D. A., Tripp, T. J., and Dunny, G. M. (2009). Multiple functional domains of Enterococcus faecalis aggregation substance Asc10 contribute to endocarditis virulence. Infect. Immun. 77, 539–548. doi: 10.1128/iai.01034-08
Chuzeville, S., Auger, J. P., Dumesnil, A., Roy, D., Lacouture, S., Fittipaldi, N., et al. (2017). Serotype-specific role of antigen I/II in the initial steps of the pathogenesis of the infection caused by Streptococcus suis. Vet. Res. 48:39. doi: 10.1186/s13567-017-0443-4
Chuzeville, S., Dramsi, S., Madec, J. Y., Haenni, M., and Payot, S. (2015). Antigen I/II encoded by integrative and conjugative elements of Streptococcus agalactiae and role in biofilm formation. Microb. Pathog. 88, 1–9. doi: 10.1016/j.micpath.2015.07.018
Cools, P., Jespers, V., Hardy, L., Crucitti, T., Delany-Moretlwe, S., Mwaura, M., et al. (2016). A Multi-Country Cross-Sectional Study of Vaginal Carriage of Group B Streptococci (GBS) and Escherichia coli in Resource-Poor Settings: Prevalences and Risk Factors. PLoS One 11:e0148052. doi: 10.1371/journal.pone.0148052
Daep, C. A., James, D. M., Lamont, R. J., and Demuth, D. R. (2006). Structural characterization of peptide-mediated inhibition of Porphyromonas gingivalis biofilm formation. Infect. Immun. 74, 5756–5762. doi: 10.1128/iai.00813-06
Daep, C. A., Novak, E. A., Lamont, R. J., and Demuth, D. R. (2011). Structural Dissection and In Vivo Effectiveness of a Peptide Inhibitor of Porphyromonas gingivalis Adherence to Streptococcus gordonii. Infect. Immun. 79, 67–74. doi: 10.1128/iai.00361-10
Demuth, D. R., Davis, C. A., Corner, A. M., Lamont, R. J., Leboy, P. S., and Malamud, D. (1988). Cloning and expression of a Streptococcus sanguis surface antigen that interacts with a human salivary agglutinin. Infect. Immun. 56, 2484–2490.
Demuth, D. R., Duan, Y., Brooks, W., Holmes, A. R., McNab, R., and Jenkinson, H. F. (1996). Tandem genes encode cell-surface polypeptides SspA and SspB which mediate adhesion of the oral bacterium Streptococcus gordonii to human and bacterial receptors. Mol. Microbiol. 20, 403–413. doi: 10.1111/j.1365-2958.1996.tb02627.x
Demuth, D. R., Golub, E. E., and Malamud, D. (1990a). Streptococcal-host interactions. Structural and functional analysis of a Streptococcus sanguis receptor for a human salivary glycoprotein. J. Biol. Chem. 265, 7120–7126.
Demuth, D. R., Irvine, D. C., Costerton, J. W., Cook, G. S., and Lamont, R. J. (2001). Discrete Protein Determinant Directs the Species-Specific Adherence of Porphyromonas gingivalis to Oral Streptococci. Infect. Immun. 69, 5736–5741. doi: 10.1128/iai.69.9.5736-5741.2001
Demuth, D. R., Lammey, M. S., Huck, M., Lally, E. T., and Malamud, D. (1990b). Comparison of Streptococcus mutans and Streptococcus sanguis receptors for human salivary agglutinin. Microb. Pathog. 9, 199–211. doi: 10.1016/0882-4010(90)90022-i
Deng, L., Spencer, B. L., Holmes, J. A., Mu, R., Rego, S., Weston, T. A., et al. (2019). The Group B Streptococcal surface antigen I/II protein, BspC, interacts with host vimentin to promote adherence to brain endothelium and inflammation during the pathogenesis of meningitis. PLoS Pathog. 15:e1007848. doi: 10.1371/journal.ppat.1007848
Do, T., Gilbert, S. C., Clark, D., Ali, F., Fatturi Parolo, C. C., Maltz, M., et al. (2010). Generation of diversity in Streptococcus mutans genes demonstrated by MLST. PLoS One 5:e9073. doi: 10.1371/journal.pone.0009073
Doran, K. S., Fulde, M., Gratz, N., Kim, B. J., Nau, R., Prasadarao, N., et al. (2016). Host-pathogen interactions in bacterial meningitis. Acta Neuropathol. 131, 185–209. doi: 10.1007/s00401-015-1531-z
Edmond, K. M., Kortsalioudaki, C., Scott, S., Schrag, S. J., Zaidi, A. K., Cousens, S., et al. (2012). Group B streptococcal disease in infants aged younger than 3 months: systematic review and meta-analysis. Lancet 379, 547–556. doi: 10.1016/s0140-6736(11)61651-6
Engels-Deutsch, M., Pini, A., Yamashita, Y., Shibata, Y., Haikel, Y., Schöller-Guinard, M., et al. (2003). Insertional inactivation of pac and rmlB genes reduces the release of tumor necrosis factor alpha, interleukin-6, and interleukin-8 induced by Streptococcus mutans in monocytic, dental pulp, and periodontal ligament cells. Infect. Immun. 71, 5169–5177. doi: 10.1128/iai.71.9.5169-5177.2003
Esberg, A., Lofgren-Burstrom, A., Ohman, U., and Stromberg, N. (2012). Host and bacterial phenotype variation in adhesion of Streptococcus mutans to matched human hosts. Infect. Immun. 80, 3869–3879. doi: 10.1128/IAI.00435-12
Esberg, A., Sheng, N., Mårell, L., Claesson, R., Persson, K., Borén, T., et al. (2017). Streptococcus mutans Adhesin Biotypes that Match and Predict Individual Caries Development. EBio Med. 24, 205–215. doi: 10.1016/j.ebiom.2017.09.027
Falsetta, M. L., Klein, M. I., Colonne, P. M., Scott-Anne, K., Gregoire, S., Pai, C. H., et al. (2014). Symbiotic relationship between Streptococcus mutans and Candida albicans synergizes virulence of plaque biofilms in vivo. Infect. Immun. 82, 1968–1981. doi: 10.1128/iai.00087-14
Ford, I., and Douglas, C. W. (1997). The role of platelets in infective endocarditis. Platelets 8, 285–294. doi: 10.1080/09537109777159
Forsgren, N., Lamont, R. J., and Persson, K. (2009). Crystal structure of the variable domain of the Streptococcus gordonii surface protein SspB. Protein. Sci. 18, 1896–1905. doi: 10.1002/pro.200
Forsgren, N., Lamont, R. J., and Persson, K. (2010). Two intramolecular isopeptide bonds are identified in the crystal structure of the Streptococcus gordonii SspB C-terminal domain. J. Mol. Biol. 397, 740–751. doi: 10.1016/j.jmb.2010.01.065
Franklin, L., Nobbs, A. H., Bricio-Moreno, L., Wright, C. J., Maddocks, S. E., Sahota, J. S., et al. (2013). The AgI/II family adhesin AspA is required for respiratory infection by Streptococcus pyogenes. PLoS One 8:e62433. doi: 10.1371/journal.pone.0062433
Gartler, S. M. (1968). Apparent Hela cell contamination of human heteroploid cell lines. Nature 217, 750–751. doi: 10.1038/217750a0
Guo, J. H., Jia, R., Fan, M. W., Bian, Z., Chen, Z., and Peng, B. (2004). Construction and immunogenic characterization of a fusion anti-caries DNA vaccine against PAc and glucosyltransferase I of Streptococcus mutans. J. Dent. Res. 83, 266–270. doi: 10.1177/154405910408300316
Guo, L., Shokeen, B., He, X., Shi, W., and Lux, R. (2017). Streptococcus mutans SpaP binds to RadD of Fusobacterium nucleatum ssp. polymorphum. Mole. Microbiol. 32, 355–364. doi: 10.1111/omi.12177
Hajishengallis, G., Koga, T., and Russell, M. W. (1994). Affinity and specificity of the interactions between Streptococcus mutans antigen I/II and salivary components. J. Dent. Res. 73, 1493–1502. doi: 10.1177/00220345940730090301
Hajishengallis, G., Martin, M., Sojar, H. T., Sharma, A., Schifferle, R. E., DeNardin, E., et al. (2002). Dependence of Bacterial Protein Adhesins on Toll-Like Receptors for Proinflammatory Cytokine Induction. Clin. Diagnos. Lab. Immunol. 9, 403–411. doi: 10.1128/cdli.9.2.403-411.2002
Hajishengallis, G., Nikolova, E., and Russell, M. W. (1992). Inhibition of Streptococcus mutans adherence to saliva-coated hydroxyapatite by human secretory immunoglobulin A (S-IgA) antibodies to cell surface protein antigen I/II: reversal by IgA1 protease cleavage. Infect. Immun. 60, 5057–5064.
Hajishengallis, G., Russell, M. W., and Michalek, S. M. (1998). Comparison of an adherence domain and a structural region of Streptococcus mutans antigen I/II in protective immunity against dental caries in rats after intranasal immunization. Infect. Immun. 66, 1740–1743.
Hall, M., Nylander, S., Jenkinson, H. F., and Persson, K. (2014). Structure of the C-terminal domain of AspA (antigen I/II-family) protein from Streptococcus pyogenes. FEBS Open Bio. 4, 283–289. doi: 10.1016/j.fob.2014.02.012
Heddle, C., Nobbs, A. H., Jakubovics, N. S., Gal, M., Mansell, J. P., Dymock, D., et al. (2003). Host collagen signal induces antigen I/II adhesin and invasin gene expression in oral Streptococcus gordonii. Mol. Microbiol. 50, 597–607. doi: 10.1046/j.1365-2958.2003.03711.x
Heim, K. P., Crowley, P. J., Long, J. R., Kailasan, S., McKenna, R., and Brady, L. J. (2014). An intramolecular lock facilitates folding and stabilizes the tertiary structure of Streptococcus mutans adhesin P1. Proc. Natl. Acad. Sci. U S A 111, 15746–15751. doi: 10.1073/pnas.1413018111
Heim, K. P., Sullan, R. M., Crowley, P. J., El-Kirat-Chatel, S., Beaussart, A., Tang, W., et al. (2015). Identification of a supramolecular functional architecture of Streptococcus mutans adhesin P1 on the bacterial cell surface. J. Biol. Chem. 290, 9002–9019. doi: 10.1074/jbc.M114.626663
Hoen, B., Alla, F., Selton-Suty, C., Beguinot, I., Bouvet, A., Briancon, S., et al. (2002). Changing profile of infective endocarditis: results of a 1-year survey in France. JAMA 288, 75–81. doi: 10.1001/jama.288.1.75
Huang, Y., Hajishengallis, G., and Michalek, S. M. (2001). Induction of protective immunity against Streptococcus mutans colonization after mucosal immunization with attenuated Salmonella enterica serovar typhimurium expressing an S. mutans adhesin under the control of in vivo-inducible nirB promoter. Infect. Immun. 69, 2154–2161. doi: 10.1128/IAI.69.4.2154-2161.2001
Jain, I., Sarkar, P., Danger, J. L., Medicielo, J., Roshika, R., Calfee, G., et al. (2019). A Mobile Genetic Element Promotes the Association Between Serotype M28 Group A Streptococcus Isolates and Cases of Puerperal Sepsis. J. Infect. Dis. 220, 882–891. doi: 10.1093/infdis/jiz195
Jakubovics, N. S., Kerrigan, S. W., Nobbs, A. H., Stromberg, N., van Dolleweerd, C. J., Cox, D. M., et al. (2005a). Functions of cell surface-anchored antigen I/II family and Hsa polypeptides in interactions of Streptococcus gordonii with host receptors. Infect. Immun. 73, 6629–6638. doi: 10.1128/IAI.73.10.6629-6638.2005
Jakubovics, N. S., Stromberg, N., van Dolleweerd, C. J., Kelly, C. G., and Jenkinson, H. F. (2005b). Differential binding specificities of oral streptococcal antigen I/II family adhesins for human or bacterial ligands. Mol. Microbiol. 55, 1591–1605. doi: 10.1111/j.1365-2958.2005.04495.x
Jenkinson, H. F., and Demuth, D. R. (1997). Structure, function and immunogenicity of streptococcal antigen I/II polypeptides. Mol. Microbiol. 23, 183–190. doi: 10.1046/j.1365-2958.1997.2021577.x
Jenkinson, H. F., Terry, S. D., McNab, R., and Tannock, G. W. (1993). Inactivation of the gene encoding surface protein SspA in Streptococcus gordonii DL1 affects cell interactions with human salivary agglutinin and oral actinomyces. Infect. Immun. 61, 3199–3208.
Jia, R., Guo, J. H., Fan, M. W., Bian, Z., Chen, Z., Peng, B., et al. (2004). Mucosal immunization against dental caries with plasmid DNA encoding pac gene of Streptococcus mutans in rats. Vaccine 22, 2511–2516. doi: 10.1016/j.vaccine.2004.01.025
Jia, R., Yan, L., and Guo, J. (2020). Enhancing the immunogenicity of a DNA vaccine against Streptococcus mutans by attenuating the inhibition of endogenous miR-9. Vaccine 38, 1424–1430. doi: 10.1016/j.vaccine.2019.11.083
Jiang, H., Hu, Y., Yang, M., Liu, H., and Jiang, G. (2017). Enhanced immune response to a dual-promoter anti-caries DNA vaccine orally delivered by attenuated Salmonella typhimurium. Immunobiology 222, 730–737. doi: 10.1016/j.imbio.2017.01.007
Jonasson, A., Eriksson, C., Jenkinson, H. F., Källestål, C., Johansson, I., and Strömberg, N. (2007). Innate immunity glycoprotein gp-340 variants may modulate human susceptibility to dental caries. BMC Infect. Dis. 7:57. doi: 10.1186/1471-2334-7-57
Jones, D. T., Taylor, W. R., and Thornton, J. M. (1992). The rapid generation of mutation data matrices from protein sequences. Comput. Appl. Biosci. 8, 275–282. doi: 10.1093/bioinformatics/8.3.275
Katz, J., Harmon, C. C., Buckner, G. P., Richardson, G. J., Russell, M. W., and Michalek, S. M. (1993). Protective salivary immunoglobulin A responses against Streptococcus mutans infection after intranasal immunization with S. mutans antigen I/II coupled to the B subunit of cholera toxin. Infect. Immun. 61, 1964–1971.
Kelemen, L., Rizk, S., Debreczeny, M., Ogier, J., and Szalontai, B. (2004). Streptococcal antigen I/II binds to extracellular proteins through intermolecular beta-sheets. FEBS Lett. 566, 190–194. doi: 10.1016/j.febslet.2004.04.029
Kelly, C. G., Todryk, S., Kendal, H. L., Munro, G. H., and Lehner, T. T. - (1995). cell, adhesion, and B-cell epitopes of the cell surface Streptococcus mutans protein antigen I/II. Infect. Immun. 63, 3649–3658.
Kelly, C. G., Younson, J. S., Hikmat, B. Y., Todryk, S. M., Czisch, M., Haris, P. I., et al. (1999). A synthetic peptide adhesion epitope as a novel antimicrobial agent. Nat. Biotechnol. 17, 42–47. doi: 10.1038/5213
Kelly, C., Evans, P., Bergmeier, L., Lee, S. F., Progulske-Fox, A., Harris, A. C., et al. (1989). Sequence analysis of the cloned streptococcal cell surface antigen I/II. FEBS Lett. 258, 127–132. doi: 10.1016/0014-5793(89)81632-1
Kerrigan, S. W., Jakubovics, N. S., Keane, C., Maguire, P., Wynne, K., Jenkinson, H. F., et al. (2007). Role of Streptococcus gordonii surface proteins SspA/SspB and Hsa in platelet function. Infect. Immun. 75, 5740–5747. doi: 10.1128/iai.00909-07
Kobayashi, M., Vekemans, J., Baker, C. J., Ratner, A. J., Le Doare, K., and Schrag, S. J. (2016). Group B Streptococcus vaccine development: present status and future considerations, with emphasis on perspectives for low and middle income countries. F1000Res. 5:2355. doi: 10.12688/f1000research.9363.1
Kumar, S., Stecher, G., Li, M., Knyaz, C., and Tamura, K. (2018). MEGA X: Molecular Evolutionary Genetics Analysis across Computing Platforms. Mol. Biol. Evol. 35, 1547–1549. doi: 10.1093/molbev/msy096
Lamont, R. J., Demuth, D. R., Davis, C. A., Malamud, D., and Rosan, B. (1991). Salivary-agglutinin-mediated adherence of Streptococcus mutans to early plaque bacteria. Infect. Immun. 59, 3446–3450.
LaPolla, R. J., Haron, J. A., Kelly, C. G., Taylor, W. R., Bohart, C., Hendricks, M., et al. (1991). Sequence and structural analysis of surface protein antigen I/II (SpaA) of Streptococcus sobrinus. Infect. Immun. 59, 2677–2685.
Larson, M. R., Rajashankar, K. R., Crowley, P. J., Kelly, C., Mitchell, T. J., Brady, L. J., et al. (2011). Crystal structure of the C-terminal region of Streptococcus mutans antigen I/II and characterization of salivary agglutinin adherence domains. J. Biol. Chem. 286, 21657–21666. doi: 10.1074/jbc.M111.231100
Larson, M. R., Rajashankar, K. R., Patel, M. H., Robinette, R. A., Crowley, P. J., Michalek, S., et al. (2010). Elongated fibrillar structure of a streptococcal adhesin assembled by the high-affinity association of alpha- and PPII-helices. Proc. Natl. Acad. Sci. U S A 107, 5983–5988. doi: 10.1073/pnas.0912293107
Lehner, T., Caldwell, J., and Smith, R. (1985). Local passive immunization by monoclonal antibodies against streptococcal antigen I/II in the prevention of dental caries. Infect. Immun. 50, 796–799.
Lehner, T., Challacombe, S. J., and Caldwell, J. (1975). Immunological and bacteriological basis for vaccination against dental caries in rhesus monkeys. Nature 254, 517–520. doi: 10.1038/254517a0
Lehner, T., Ma, J. K., and Kelly, C. G. (1992). A mechanism of passive immunization with monoclonal antibodies to a 185,000 M(r) streptococcal antigen. Adv. Exp. Med. Biol. 327, 151–163. doi: 10.1007/978-1-4615-3410-5_17
Lehner, T., Russell, M. W., Caldwell, J., and Smith, R. (1981). Immunization with purified protein antigens from Streptococcus mutans against dental caries in rhesus monkeys. Infect. Immun. 34, 407–415.
Linde, A. (1989). Dentin matrix proteins: composition and possible functions in calcification. Anat. Rec. 224, 154–166. doi: 10.1002/ar.1092240206
Loimaranta, V., Jakubovics, N. S., Hytönen, J., Finne, J., Jenkinson, H. F., and Strömberg, N. (2005). Fluid- or Surface-Phase Human Salivary Scavenger Protein gp340 Exposes Different Bacterial Recognition Properties. Infect. Immun. 73, 2245–2252. doi: 10.1128/iai.73.4.2245-2252.2005
Love, R. M., McMillan, M. D., and Jenkinson, H. F. (1997). Invasion of dentinal tubules by oral streptococci is associated with collagen recognition mediated by the antigen I/II family of polypeptides. Infect. Immun. 65, 5157–5164.
Lucchese, A. (2017). Streptococcus mutans antigen I/II and autoimmunity in cardiovascular diseases. Autoimmun. Rev. 16, 456–460. doi: 10.1016/j.autrev.2017.03.009
Ma, J. K., and Lehner, T. (1990). Prevention of colonization of Streptococcus mutans by topical application of monoclonal antibodies in human subjects. Arch. Oral Biol. 35(Suppl.), 115S–122S. doi: 10.1016/0003-9969(90)90140-6
Ma, J. K., Hikmat, B. Y., Wycoff, K., Vine, N. D., Chargelegue, D., Yu, L., et al. (1998). Characterization of a recombinant plant monoclonal secretory antibody and preventive immunotherapy in humans. Nat. Med. 4, 601–606. doi: 10.1038/nm0598-601
Ma, J. K., Hunjan, M., Smith, R., and Lehner, T. (1989). Specificity of monoclonal antibodies in local passive immunization against Streptococcus mutans. Clin. Exp. Immunol. 77, 331–337.
Ma, J. K., Hunjan, M., Smith, R., Kelly, C., and Lehner, T. (1990). An investigation into the mechanism of protection by local passive immunization with monoclonal antibodies against Streptococcus mutans. Infect. Immun. 58, 3407–3414.
Ma, J. K., Kelly, C. G., Munro, G., Whiley, R. A., and Lehner, T. (1991). Conservation of the gene encoding streptococcal antigen I/II in oral streptococci. Infect. Immun. 59, 2686–2694.
Maddocks, S. E., Wright, C. J., Nobbs, A. H., Brittan, J. L., Franklin, L., Strömberg, N., et al. (2011). Streptococcus pyogenes antigen I/II-family polypeptide AspA shows differential ligand-binding properties and mediates biofilm formation. Mole. Microbiol. 81, 1034–1049. doi: 10.1111/j.1365-2958.2011.07749.x
Madsen, J., Mollenhauer, J., and Holmskov, U. (2010). Review: Gp-340/DMBT1 in mucosal innate immunity. Innate Immun. 16, 160–167. doi: 10.1177/1753425910368447
Mahmoud, M. Y., Demuth, D. R., and Steinbach-Rankins, J. M. (2018). BAR-encapsulated nanoparticles for the inhibition and disruption of Porphyromonas gingivalis-Streptococcus gordonii biofilms. J. Nanobiotechnol. 16:69. doi: 10.1186/s12951-018-0396-4
Matsumoto-Nakano, M., Tsuji, M., Inagaki, S., Fujita, K., Nagayama, K., Nomura, R., et al. (2009). Contribution of cell surface protein antigen c of Streptococcus mutans to platelet aggregation. Oral Microbiol. Immunol. 24, 427–430. doi: 10.1111/j.1399-302X.2009.00521.x
Mollenhauer, J., Wiemann, S., Scheurlen, W., Korn, B., Hayashi, Y., Wilgenbus, K. K., et al. (1997). DMBT1, a new member of the SRCR superfamily, on chromosome 10q25.3-26.1 is deleted in malignant brain tumours. Nat. Genet. 17, 32–39. doi: 10.1038/ng0997-32
Monif, G. R., and Carson, H. J. (1998). Female genital tract bacterial coisolates with Candida albicans in patients without clinical vaginitis. Infect. Dis. Obstet. Gynecol. 6, 52–56. doi: 10.1002/(sici)1098-099719986:2<52::Aid-idog4<3.0.Co;2-9
Nakano, K., Tsuji, M., Nishimura, K., Nomura, R., and Ooshima, T. (2006). Contribution of cell surface protein antigen PAc of Streptococcus mutans to bacteremia. Microb. Infect. 8, 114–121. doi: 10.1016/j.micinf.2005.06.005
Nobbs, A. H., Shearer, B. H., Drobni, M., Jepson, M. A., and Jenkinson, H. F. (2007). Adherence and internalization of Streptococcus gordonii by epithelial cells involves beta1 integrin recognition by SspA and SspB (antigen I/II family) polypeptides. Cell Microbiol. 9, 65–83. doi: 10.1111/j.1462-5822.2006.00768.x
Nobbs, A., and Kreth, J. (2019). Genetics of sanguinis-Group Streptococci in Health and Disease. Microbiol. Spectr. 7:0052. doi: 10.1128/microbiolspec.GPP3-0052-2018
Ogier, J. A., Scholler, M., Leproivre, Y., Pini, A., Sommer, P., and Klein, J. P. (1990). Complete nucleotide sequence of the sr gene from Streptococcus mutans OMZ 175. FEMS Microbiol. Lett. 56, 223–227. doi: 10.1016/0378-1097(90)90155-j
Oho, T., and Nagata, E. (2019). DMBT1 involvement in the human aortic endothelial cell response to Streptococcus mutans. Mole. Microbiol. 34, 108–117. doi: 10.1111/omi.12257
Oli, M. W., Otoo, H. N., Crowley, P. J., Heim, K. P., Nascimento, M. M., Ramsook, C. B., et al. (2012). Functional amyloid formation by Streptococcus mutans. Microbiology 158, 2903–2916. doi: 10.1099/mic.0.060855-0
Petersen, F. C., Assev, S., van der Mei, H. C., Busscher, H. J., and Scheie, A. A. (2002). Functional variation of the antigen I/II surface protein in Streptococcus mutans and Streptococcus intermedius. Infect. Immun. 70, 249–256. doi: 10.1128/iai.70.1.249-256.2002
Petersen, F. C., Pasco, S., Ogier, J., Klein, J. P., Assev, S., and Scheie, A. A. (2001). Expression and functional properties of the Streptococcus intermedius surface protein antigen I/II. Infect. Immun. 69, 4647–4653. doi: 10.1128/IAI.69.7.4647-4653.2001
Pidwill, G. R., Rego, S., Jenkinson, H. F., Lamont, R. J., and Nobbs, A. H. (2018). Coassociation between Group B Streptococcus and Candida albicans Promotes Interactions with Vaginal Epithelium. Infect. Immun. 86:e00669-17. doi: 10.1128/iai.00669-17
Prakobphol, A., Xu, F., Hoang, V. M., Larsson, T., Bergstrom, J., Johansson, I., et al. (2000). Salivary Agglutinin, Which Binds Streptococcus mutans and Helicobacter pylori, Is the Lung Scavenger Receptor Cysteine-rich Protein gp-340. J. Biol. Chem. 275, 39860–39866. doi: 10.1074/jbc.M006928200
Purushotham, S., and Deivanayagam, C. (2014). The calcium-induced conformation and glycosylation of scavenger-rich cysteine repeat (SRCR) domains of glycoprotein 340 influence the high affinity interaction with antigen I/II homologs. J. Biol. Chem. 289, 21877–21887. doi: 10.1074/jbc.M114.565507
Rajani, R., and Klein, J. L. (2020). Infective endocarditis: A contemporary update. Clin. Med. 20, 31–35. doi: 10.7861/clinmed.cme.20.1.1
Rajendiran, K. S., Ananthanarayanan, R. H., Satheesh, S., and Rajappa, M. (2014). Elevated levels of serum sialic acid and high-sensitivity C-reactive protein: markers of systemic inflammation in patients with chronic heart failure. Br. J. Biomed. Sci. 71, 29–32. doi: 10.1080/09674845.2014.11669959
Rego, S., Heal, T. J., Pidwill, G. R., Till, M., Robson, A., Lamont, R. J., et al. (2016). Structural and Functional Analysis of Cell Wall-anchored Polypeptide Adhesin BspA in Streptococcus agalactiae. J. Biol. Chem. 291, 15985–16000. doi: 10.1074/jbc.M116.726562
Rivière, G., Peng, E. Q., Brotgandel, A., Andring, J. T., Lakshmanan, R. V., Agbandje-McKenna, M., et al. (2020). Characterization of an intermolecular quaternary interaction between discrete segments of the Streptococcus mutans adhesin P1 by NMR spectroscopy. FEBS J. 287, 2597–2611. doi: 10.1111/febs.15158
Roky, M., Tan, J., Sztukowska, M. N., Trent, J. O., and Demuth, D. R. (2020). Identification of small molecule inhibitors targeting Porphyromonas gingivalis interspecies adherence and determination of their in vitro and in vivo efficacy. Antimicrob. Agent Chemother. 2020:00884-20. doi: 10.1128/aac.00884-20
Russell, M. W., and Lehner, T. (1978). Characterisation of antigens extracted from cells and culture fluids of Streptococcus mutans serotype c. Arch. Oral. Biol. 23, 7–15. doi: 10.1016/0003-9969(78)90047-x
Russell, M. W., Bergmeier, L. A., Zanders, E. D., and Lehner, T. (1980). Protein antigens of Streptococcus mutans: purification and properties of a double antigen and its protease-resistant component. Infect. Immun. 28, 486–493.
Russell, M. W., Wu, H. Y., White, P. L., Kilian, M., and Henrichsen, J. (1992). Serum antibody responses to Streptococcus mutans antigens in humans systemically infected with oral streptococci. Oral Microbiol. Immunol. 7, 321–325. doi: 10.1111/j.1399-302x.1992.tb00630.x
Russell, R. R. (1979). Wall-associated protein antigens of Streptococcus mutans. J. Gen. Microbiol. 114, 109–115. doi: 10.1099/00221287-114-1-109
Ryd, M., Schennings, T., Flock, M., Heimdahl, A., and Flock, J. I. (1996). Streptococcus mutans major adhesion surface protein, P1 (I/II), does not contribute to attachment to valvular vegetations or to the development of endocarditis in a rat model. Arch. Oral Biol. 41, 999–1002. doi: 10.1016/s0003-9969(96)00035-0
Schmitt, A., Jiang, K., Camacho, M. I., Jonna, V. R., Hofer, A., Westerlund, F., et al. (2018). PrgB promotes aggregation, biofilm formation, and conjugation through DNA binding and compaction. Mol. Microbiol. 109, 291–305. doi: 10.1111/mmi.13980
Sciotti, M. A., Yamodo, I., Klein, J. P., and Ogier, J. A. (1997). The N-terminal half part of the oral streptococcal antigen I/IIf contains two distinct binding domains. FEMS Microbiol. Lett. 153, 439–445. doi: 10.1111/j.1574-6968.1997.tb12608.x
Scott, J. R., and Barnett, T. C. (2006). Surface proteins of gram-positive bacteria and how they get there. Annu. Rev. Microbiol. 60, 397–423. doi: 10.1146/annurev.micro.60.080805.142256
Shi, W., Li, Y. H., Liu, F., Yang, J. Y., Zhou, D. H., Chen, Y. Q., et al. (2012). Flagellin enhances saliva IgA response and protection of anti-caries DNA vaccine. J. Dent. Res. 91, 249–254. doi: 10.1177/0022034511424283
Silverman, R. J., Nobbs, A. H., Vickerman, M. M., Barbour, M. E., and Jenkinson, H. F. (2010). Interaction of Candida albicans cell wall Als3 protein with Streptococcus gordonii SspB adhesin promotes development of mixed-species communities. Infect. Immun. 78, 4644–4652. doi: 10.1128/IAI.00685-10
Singh, B., Fleury, C., Jalalvand, F., and Riesbeck, K. (2012). Human pathogens utilize host extracellular matrix proteins laminin and collagen for adhesion and invasion of the host. FEMS Microbiol. Rev. 36, 1122–1180. doi: 10.1111/j.1574-6976.2012.00340.x
Sitkiewicz, I., Green, N. M., Guo, N., Mereghetti, L., and Musser, J. M. (2011). Lateral gene transfer of streptococcal ICE element RD2 (region of difference 2) encoding secreted proteins. BMC Microbiol. 11:65. doi: 10.1186/1471-2180-11-65
Soell, M., Holveck, F., Schöller, M., Wachsmann, R. D., and Klein, J. P. (1994). Binding of Streptococcus mutans SR protein to human monocytes: production of tumor necrosis factor, interleukin 1, and interleukin 6. Infect. Immun. 62, 1805–1812.
Son, Y.-O., Jeon, Y.-M., Kim, Y.-S., Park, S.-S., Park, S.-M., Kim, J.-H., et al. (2012). Streptococcus mutans GS-5 antigen I/II stimulates cell survival in serum deprived-cultures through PI3K/Akt Pathways. J. Cell. Biochem. 113, 1724–1732. doi: 10.1002/jcb.24042
Steer, A. C., Lamagni, T., Curtis, N., and Carapetis, J. R. (2012). Invasive group a streptococcal disease: epidemiology, pathogenesis and management. Drugs 72, 1213–1227. doi: 10.2165/11634180-000000000-00000
Sullan, R. M., Li, J. K., Crowley, P. J., Brady, L. J., and Dufrene, Y. F. (2015). Binding forces of Streptococcus mutans P1 adhesin. ACS Nano 9, 1448–1460. doi: 10.1021/nn5058886
Tamura, H., Kikuchi, T., Shirato, R., and Kato, H. (2001). Cloning and DNA sequencing of the surface protein antigen I/II (PAa) of Streptococcus cricetus. FEMS Microbiol. Lett. 196, 251–256. doi: 10.1111/j.1574-6968.2001.tb10573.x
Tamura, H., Yamada, A., and Kato, H. (2008). Identification and characterization of an antigen I/II homologous gene, pah, from Streptococcus downei. Curr. Microbiol. 56, 518–523. doi: 10.1007/s00284-008-9117-9
Tamura, H., Yamada, A., Saito, H., Murai, S., and Kato, H. (2004). Identification of another surface protein antigen I/II gene, paaB, and a putative transcriptional regulator gene, par, from Streptococcus cricetus. Gene Genet. Syst. 79, 129–137. doi: 10.1266/ggs.79.129
Tang, W., Bhatt, A., Smith, A. N., Crowley, P. J., Brady, L. J., and Long, J. R. (2016). Specific binding of a naturally occurring amyloidogenic fragment of Streptococcus mutans adhesin P1 to intact P1 on the cell surface characterized by solid state NMR spectroscopy. J. Biomol. NMR 64, 153–164. doi: 10.1007/s10858-016-0017-1
Tettelin, H., Masignani, V., Cieslewicz, M., Donati, C., Medini, D., Ward, N., et al. (2005). Genome analysis of multiple pathogenic isolates of Streptococcus agalactiae: Implications for the microbial “pan-genome”. Proc. Natl. Acad. Sci. U S A 102, 13950–13955. doi: 10.1073/pnas.0506758102
Troffer-Charlier, N., Ogier, J., Moras, D., and Cavarelli, J. (2002). Crystal structure of the V-region of Streptococcus mutans antigen I/II at 2.4 A resolution suggests a sugar preformed binding site. J. Mol. Biol. 318, 179–188. doi: 10.1016/S0022-2836(02)00025-6
Vernier, A., Diab, M., Soell, M., Haan-Archipoff, G., Beretz, A., Wachsmann, D., et al. (1996). Cytokine production by human epithelial and endothelial cells following exposure to oral viridans streptococci involves lectin interactions between bacteria and cell surface receptors. Infect. Immun. 64, 3016–3022.
Waters, C. M., Hirt, H., McCormick, J. K., Schlievert, P. M., Wells, C. L., and Dunny, G. M. (2004). An amino-terminal domain of Enterococcus faecalis aggregation substance is required for aggregation, bacterial internalization by epithelial cells and binding to lipoteichoic acid. Mol. Microbiol. 52, 1159–1171. doi: 10.1111/j.1365-2958.2004.04045.x
Weintraub, J. A., Hilton, J. F., White, J. M., Hoover, C. I., Wycoff, K. L., Yu, L., et al. (2005). Clinical trial of a plant-derived antibody on recolonization of mutans streptococci. Caries Res. 39, 241–250. doi: 10.1159/000084805
Wen, Z. T., Liao, S., Bitoun, J. P., De, A., Jorgensen, A., Feng, S., et al. (2017). Streptococcus mutans Displays Altered Stress Responses While Enhancing Biofilm Formation by Lactobacillus casei in Mixed-Species Consortium. Front. Cell. Infect. Microbiol. 7:524. doi: 10.3389/fcimb.2017.00524
Wen, Z. T., Yates, D., Ahn, S. J., and Burne, R. A. (2010). Biofilm formation and virulence expression by Streptococcus mutans are altered when grown in dual-species model. BMC Microbiol. 10:111. doi: 10.1186/1471-2180-10-111
Winograd-Katz, S. E., Fässler, R., Geiger, B., and Legate, K. R. (2014). The integrin adhesome: from genes and proteins to human disease. Nat. Rev. Mole. Cell Biol. 15, 273–288. doi: 10.1038/nrm3769
Yang, C., Scoffield, J., Wu, R., Deivanayagam, C., Zou, J., and Wu, H. (2018). Antigen I/II mediates interactions between Streptococcus mutans and Candida albicans. Mole. Microbiol. 33, 283–291. doi: 10.1111/omi.12223
Yu, H., Nakano, Y., Yamashita, Y., Oho, T., and Koga, T. (1997). Effects of antibodies against cell surface protein antigen PAc-glucosyltransferase fusion proteins on glucan synthesis and cell adhesion of Streptococcus mutans. Infect. Immun. 65, 2292–2298.
Zhang, P., Jespersgaard, C., Lamberty-Mallory, L., Katz, J., Huang, Y., Hajishengallis, G., et al. (2002). Enhanced immunogenicity of a genetic chimeric protein consisting of two virulence antigens of Streptococcus mutans and protection against infection. Infect. Immun. 70, 6779–6787. doi: 10.1128/iai.70.12.6779-6787.2002
Keywords: antigen I/II, Streptococcus, adhesin, dental caries, respiratory infection, vaginal colonization, meningitis, biofilms
Citation: Manzer HS, Nobbs AH and Doran KS (2020) The Multifaceted Nature of Streptococcal Antigen I/II Proteins in Colonization and Disease Pathogenesis. Front. Microbiol. 11:602305. doi: 10.3389/fmicb.2020.602305
Received: 03 September 2020; Accepted: 29 October 2020;
Published: 25 November 2020.
Edited by:
Magnus Hook, Texas A&M Health Science Center, United StatesReviewed by:
Mark Joseph Walker, The University of Queensland, AustraliaCopyright © 2020 Manzer, Nobbs and Doran. This is an open-access article distributed under the terms of the Creative Commons Attribution License (CC BY). The use, distribution or reproduction in other forums is permitted, provided the original author(s) and the copyright owner(s) are credited and that the original publication in this journal is cited, in accordance with accepted academic practice. No use, distribution or reproduction is permitted which does not comply with these terms.
*Correspondence: Kelly S. Doran, a2VsbHkuZG9yYW5AY3VhbnNjaHV0ei5lZHU=
Disclaimer: All claims expressed in this article are solely those of the authors and do not necessarily represent those of their affiliated organizations, or those of the publisher, the editors and the reviewers. Any product that may be evaluated in this article or claim that may be made by its manufacturer is not guaranteed or endorsed by the publisher.
Research integrity at Frontiers
Learn more about the work of our research integrity team to safeguard the quality of each article we publish.