- Department of Microbiology and Molecular Genetics, Michigan State University, East Lansing, MI, United States
Bacteria in the genus Geobacter thrive in iron- and manganese-rich environments where the divalent cobalt cation (CoII) accumulates to potentially toxic concentrations. Consistent with selective pressure from environmental exposure, the model laboratory representative Geobacter sulfurreducens grew with CoCl2 concentrations (1 mM) typically used to enrich for metal-resistant bacteria from contaminated sites. We reconstructed from genomic data canonical pathways for CoII import and assimilation into cofactors (cobamides) that support the growth of numerous syntrophic partners. We also identified several metal efflux pumps, including one that was specifically upregulated by CoII. Cells acclimated to metal stress by downregulating non-essential proteins with metals and thiol groups that CoII preferentially targets. They also activated sensory and regulatory proteins involved in detoxification as well as pathways for protein and DNA repair. In addition, G. sulfurreducens upregulated respiratory chains that could have contributed to the reductive mineralization of the metal on the cell surface. Transcriptomic evidence also revealed pathways for cell envelope modification that increased metal resistance and promoted cell-cell aggregation and biofilm formation in stationary phase. These complex adaptive responses confer on Geobacter a competitive advantage for growth in metal-rich environments that are essential to the sustainability of cobamide-dependent microbiomes and the sequestration of the metal in hitherto unknown biomineralization reactions.
Introduction
Metal micronutrients such as nickel (NiII), cobalt (CoII), manganese (MnII), and iron (FeII) are essential for life yet toxic above relatively low concentrations (Buccella et al., 2019). Not surprisingly, microorganisms have evolved numerous adaptive responses to import the essential metals from the environment while preventing their excessive intracellular accumulation and intoxication (Chandrangsu et al., 2017). Metal homeostasis is primarily achieved by the antagonistic activities of metal importers and exporters (Chandrangsu et al., 2017). Cells often use high affinity transporters to import the metals with specificity and rely on specialized proteins and chaperones to integrate them into pathways dedicated to the synthesis of metalloproteins and enzyme cofactors (Buccella et al., 2019). Collectively, biometals contribute to the synthesis of up to one third of the cell’s proteome and to metabolic functions essential to the growth and survival of the cell (Buccella et al., 2019). Each of these metals must be available in just the right intracellular concentration (i.e., the cellular metal quota) to prevent intoxication (Outten and O’Halloran, 2001). Thus, dedicated metalloregulatory systems monitor the intracellular metal levels and modulate the expression of transporters and other proteins essential for metal homeostasis (Chandrangsu et al., 2017). Metal exporters provide the primary mechanism to eliminate excess metal (Chandrangsu et al., 2017) but the cellular response to metal intoxication is often more extensive, as cells have to cope with the direct and indirect impacts of the reactive metals on proteins and DNA. For example, CoII can bind and inactivate numerous proteins non-specifically, displace other metals (particularly, FeII) from prosthetic groups and metal-binding sites, and generate free radicals (Valko et al., 2005). Its high affinity for thiol groups disrupts disulfide bonds in proteins, reduces the free thiol pool and can interfere with sulfur assimilation (Barras and Fontecave, 2011). Hence, CoII intoxication causes generalized damage in the cells, requiring extensive reprogramming to cope with multiple stressors.
The essentiality yet toxicity of metal micronutrients such as CoII exerts selective pressure on microorganisms to tune their metabolism to the fluctuating availability of the metal species from geochemical sources. Yet, many aspects of the biological cycling of metal micronutrients remain relatively obscure. This is particularly true for CoII, a metal micronutrient that some microorganisms assimilate to produce enzyme cofactors (cobamides) in the cobalamin (vitamin B12) family involved in metabolic reactions essential to all living cells (Shelton et al., 2019). Genes encoding cobamide-dependent enzymes are widespread in prokaryotes but only a fraction of surveyed genomes have complete pathways for de novo cobamide synthesis (Zhang et al., 2009; Shelton et al., 2019). As a result, most microorganisms need to salvage cobamides from the environment, a nutritional dependency that drives syntrophic interactions with cobamide producers (Seth and Taga, 2014). Cobamide-dependent microbiomes depend on the ability of cobamide producers to import and assimilate the soluble CoII cation. The divalent species, however, readily oxidizes to CoIII on the surface of MnIV oxide particles (Crowther et al., 1983; Kay et al., 2001). CoII mobility in soil and sediment systems is also limited by the tendency of the metal to coprecipitate with FeIII and MnIV oxide minerals (Krupka and Serne, 2002). Additionally, FeIII and MnIV oxides sorb large amounts of the metal cation, sequestering it in solid phases that reduce its bioavailability (Backes et al., 1995).
The absorption and co-precipitation of most of the available CoII into FeIII and MnIV minerals gives FeIII and MnIV-reducing bacteria, such as those in the genus Geobacter, a competitive advantage for growth in cobamide-dependent microbiomes (Figure 1). These bacteria gain energy for growth from the reductive dissolution of the metal oxides, which are reactions that solubilize FeII and MnII and remobilize CoII and CoIII (Reguera and Kashefi, 2019). Geobacter species are also important drivers of organic matter degradation, a process that generates organic chelators with affinity for CoIII. This keeps the trivalent species in solution and available for use as an electron acceptor (Reguera and Kashefi, 2019). The dissimilatory reduction of chelated forms of CoIII by Geobacter reduces CoIII to CoII (Reguera and Kashefi, 2019). The low reduction potential of the CoII species (−0.28 V versus standard hydrogen electrode, SHE) and its toxicity to bacteria at relatively low concentrations have been assumed to prevent its biological reduction to Co0 (Hau et al., 2008; Cosert and Reguera, 2019). Despite its toxicity, Geobacter species, including the model laboratory strain Geobacter sulfurreducens, assimilate CoII to synthesize cobamides, which they secrete to sustain several syntrophic partners (Yan et al., 2012) (Figure 1). These syntrophic interactions are favored in local epigenetic zones enriched in FeIII and MnIV oxides, where CoII preferentially accumulates (Burkhardt et al., 2009). This raises yet unexplored questions about the cellular tolerance of Geobacter species for CoII and the mechanisms that allow these microorganisms to survive and even thrive in CoII-rich environments.
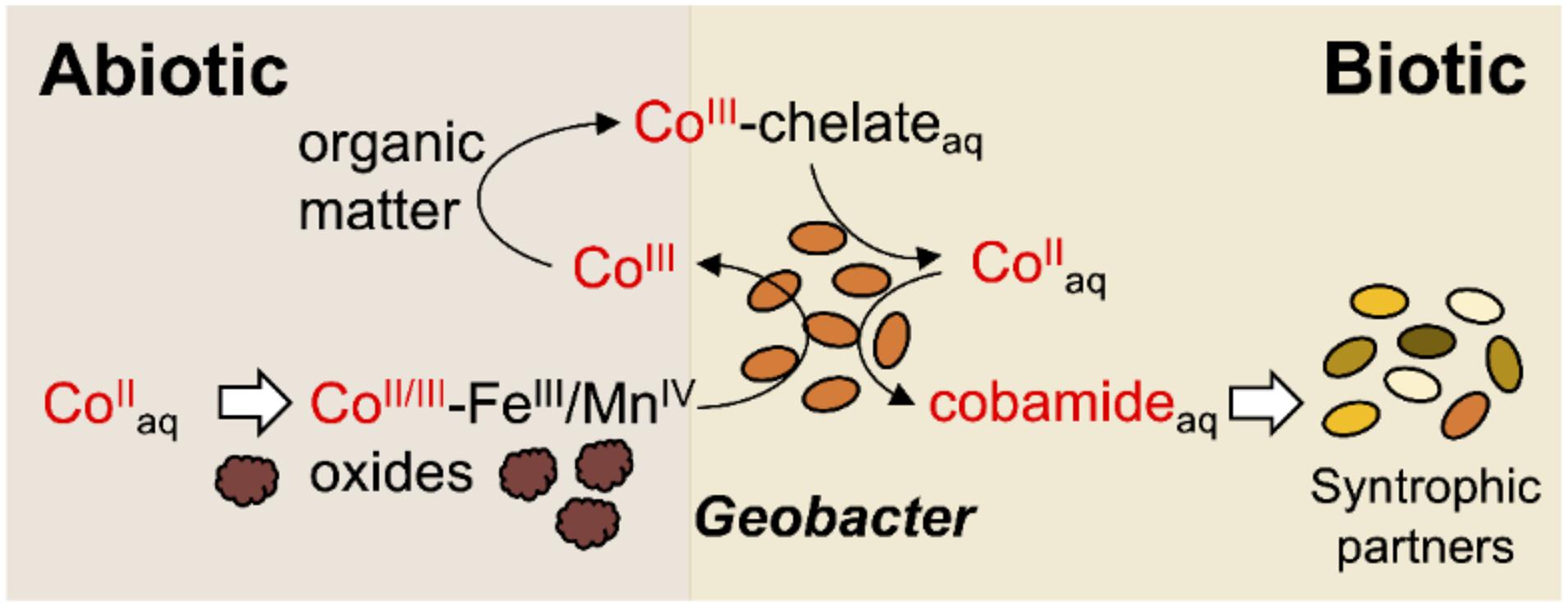
Figure 1. Known contribution of Geobacter species to the cycling of cobalt (Co). Geobacter bacteria reduce chelated and mineral forms of CoIII to CoII, whose bioavailability is limited by the tendency of the Co species to adsorb and/or co-precipitate with FeIII and MnIV oxides. The reduction of FeIII and MnIV oxides by Geobacter bacteria solubilizes CoII for the synthesis of cobamides that support the growth of syntrophic partners.
We gained insights into the environmental controls of Geobacter activities in cobamide-driven microbiomes by investigating the adaptive responses of G. sulfurreducens to growth and reproduction in the presence of CoII. Consistent with environmental exposure, we demonstrate high CoII resistance in this laboratory strain and describe pathways for protein and DNA repair, cell envelope modifications, and biofilm formation that allow the cells to effectively cope with CoII stress. Importantly, we show that metal acclimation activates respiratory chains that could participate in the reductive precipitation of the metal on the cell’s surface to alleviate toxicity. These adaptive responses allow Geobacter species to grow in CoII-rich environments, sustaining the productivity of the native microbiomes and contributing to hitherto unknown reactions of the Co cycle.
Results
Genomic Determinants of CoII Homeostasis in G. sulfurreducens
Metal ions bridge the outer membrane of Gram-negative bacteria by simple diffusion through nonselective pores (Nikaido, 2003) but require specific transporters to traverse the inner membrane (Figure 2). We identified in G. sulfurreducens complete NikMNQO and CbiMNQO importers, the most widespread prokaryotic systems for NiII and CoII uptake (Rodionov et al., 2006). Although both systems can import NiII and CoII, specific amino acid signatures in the M subunits make CbiMNQO the high affinity importer of CoII (Rodionov et al., 2006). At high enough concentrations, however, CoII could selectively outcompete NiII and enter the cytoplasm via the NikMNQO system. These importers are annotated as ATP-binding Cassette (ABC) transporters, but they are part of the prokaryotic family of energy-coupling factor (ECF) systems that also transport water-soluble vitamins and cofactors (Cracan and Banerjee, 2013). The metal ECF subclass has a distinct modular architecture (A, T, and S components) to bind the substrate (S component) without assistance from extracytoplasmic solute-binding proteins (Cracan and Banerjee, 2013). In most Nik/CbiMNQO systems, the S component is a heterodimer of M and N subunits (Rodionov et al., 2006) but these subunits are fused in a single gene in G. sulfurreducens (nikMN, GSU1279; cbiMN, GSU3004). The end result is the same: the assembly of a MN subcomplex (S component) that binds the metal and transports it across the membrane in a reaction energized by the O subunit dimer (A, or ATPase, component) and rate-modulated via interactions with the transmembrane Q subunit (T component) (Cracan and Banerjee, 2013).
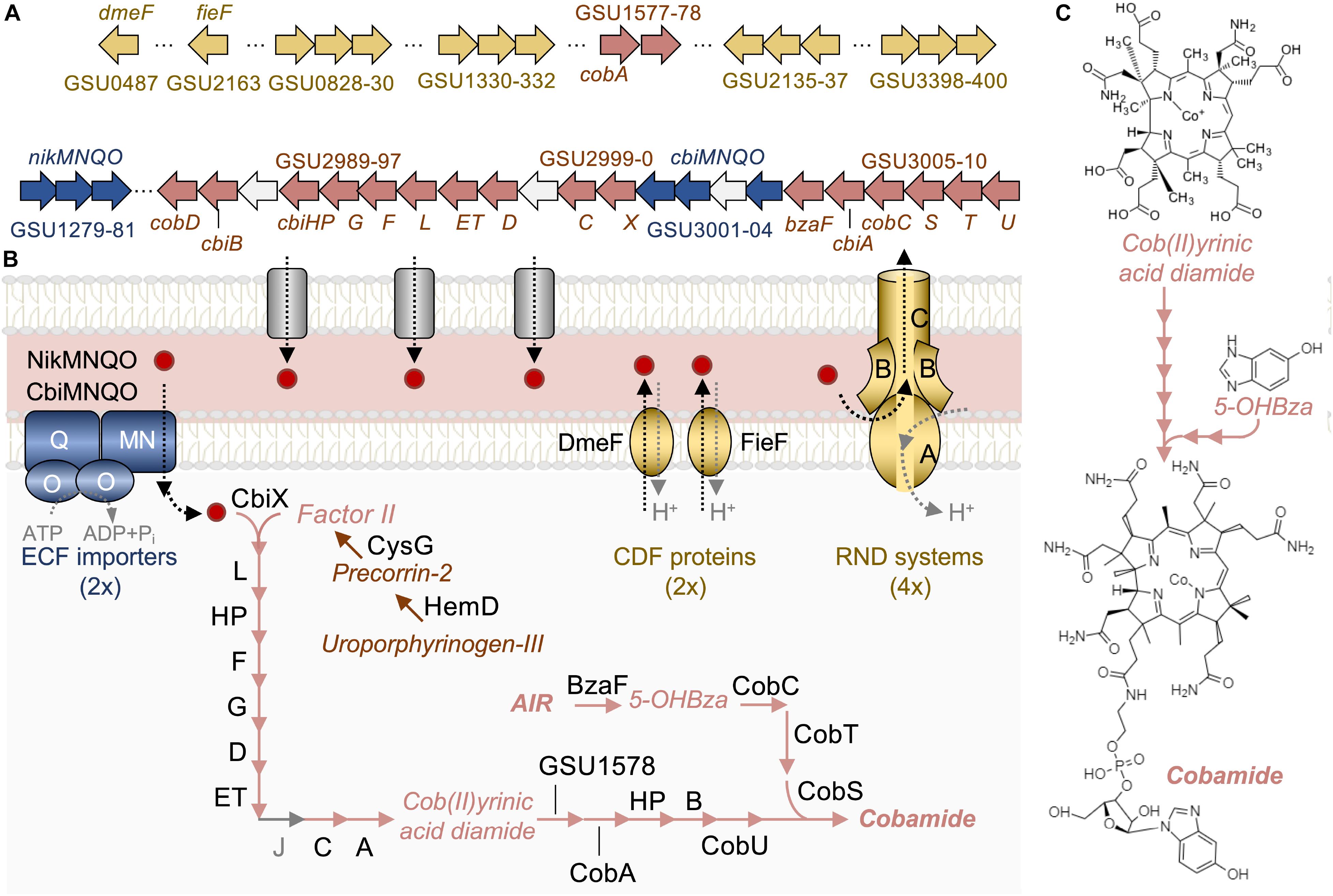
Figure 2. Genomic reconstruction of potential pathways for CoII transport and assimilation in G. sulfurreducens. The genes (A) and model (B) show transmembrane ECF importers (in blue) as well as two CDF proteins for transmembrane export and four RND systems for exporting periplasmic metal across the outer membrane (gold color). Panel (B) also shows generic outer membrane porins (in gray) for the simple diffusion of metal cations. The genome of G. sulfurreducens also encodes for Cbi (letter designation), Cob (full name) and other enzymes needed for the anaerobic synthesis of cobamide (pink arrows). The pathway starts with the incorporation by the cobaltochelatase CbiX of the metal into Factor II, which is synthesized from heme intermediates such as uroporphyrinogen-III. The genome encodes Cbi proteins that convert the CoII-Factor II into cob(II)yrinic acid diamide, except for CbiJ (in gray) whose role in the pathway is yet to be experimentally validated. Cob (full name) and Cbi (only letters) proteins and other enzymes complete the synthesis of a cobamide with a 5-OHBza lower ligand (formula in C).
As shown in Figure 2, the cbiMNQO genes are part of a large cluster (GSU2989–3010) encoding most of the enzymes needed for the anaerobic synthesis of a cobinamide intermediate (Cbi proteins) and its conversion into cobamide (Cob and Cbi proteins) (Moore and Warren, 2012). We identified in a separate genomic location two additional cobamide biosynthetic enzymes, GSU1578 and CobA (GSU1577). Also unlinked were two genes encoding enzymes for the methylation (HemD, GSU3286) and oxidation (CysG, GSU3282) of uroporphyrinogen III, the common precursor of cobamide and heme biosynthesis (Moore and Warren, 2012). These two enzymes convert uroporphyrinogen III into Factor II, the preferred substrate for the anaerobic synthesis of cobamide (Moore and Warren, 2012). The anaerobic cobaltochelatase CbiX (GSU3000) incorporates the metal into Factor II, while several Cbi proteins methylate, contract, amidate, and decarboxylate the molecule to generate a cobyrinic acid diamide intermediate (Figure 2C). All of the proteins needed for these reactions were annotated or had a clear homolog in the genome of G. sulfurreducens, except for the precorrin-6X reductase CbiJ (highlighted in gray in Figure 2B). This enzyme is often assigned to this reaction based on its homology with the aerobic enzyme CobK, but its biological role has never been confirmed (Moore and Warren, 2012). The cob(II)yrinic acid diamide intermediate is then converted into adenosyl cobinamide in sequential reactions initiated by an adenosylcobamide-binding subunit of an (R)-methylmalonyl-CoA mutase (GSU1578). The step catalyzed by CobU (GSU3010) generates an adenosine-GDP-cobinamide substrate for attachment of the cobamide lower ligand. G. sulfurreducens produces a cobamide with a 5-hydroxybenzimidazole (5-OHBza) lower ligand (Figure 2C) that is synthesized from 5-amino-imidazole ribonucleotide (AIR) by BzaF (GSU3005) (Hazra et al., 2015). The bzaF gene, which is unique to the Geobacteraceae and other members of the order Desulfurococcales, is a functional homolog of the bzaA and bzaB genes that catalyze the synthesis of 5-OHBza in other bacteria (Hazra et al., 2015). The attachment of the lower ligand to adenosine-GDP-cobinamide completes the synthesis of the cobamide (Figure 2C).
At high enough concentrations, CoII can also enter the inner membrane non-specifically via magnesium (MgII) uptake systems (Nies, 1999). To compensate for the uncontrolled influx of the metal, cells express metal exporters (Chandrangsu et al., 2017). We identified in the genome two genes (GSU0487 and GSU2613) encoding the cation diffusion facilitator (CDF) proteins, DmeF and FieF (Figure 2). These proton-driven antiporters export a broad range of divalent cations (CoII, ZnII, FeII, CdII, and NiII) across the inner membrane (Kolaj-Robin et al., 2015). However, the preferred substrate for DmeF is CoII (Munkelt et al., 2004) while FieF specializes in the export of excess FeII (Grass et al., 2005). The intracellular accumulation of CoII can disrupt the homeostatic balance with FeII and allow FieF to move more CoII than FeII across the inner membrane. The genome also contains four tripartite metal efflux systems of the Resistance-Nodulation-Division (RND) superfamily (Munkelt et al., 2004) (Figure 2). RND efflux pumps use the proton gradient to energize the export of cytoplasmic or periplasmic substrates (Kim et al., 2011). Some of these exporters function as multidrug efflux pumps (Nikaido and Takatsuka, 2009) while others specialize in proton-dependent transport of divalent metal cations (Bagai et al., 2008; Pak et al., 2013). As in other Gram-negative bacteria (Nikaido and Takatsuka, 2009), the Geobacter metal RND systems contain a transmembrane pump, a periplasmic adaptor protein and an outer membrane porin. This trimeric configuration facilitates the export of periplasmic metals using the electrochemical gradient (Nikaido and Takatsuka, 2009). Collectively, inner and outer metal exporters ensure that CoII does not accumulate to toxic levels inside the cytoplasm (Chandrangsu et al., 2017).
High CoII Tolerance in G. sulfurreducens Suggests Significant Environmental Exposure to the Metal
As metal resistance evolves under selective pressure, we determined the growth efficiency of G. sulfurreducens in the presence of CoII (Figure 3). For these experiments, we inoculated cells at low densities (OD660, ∼0.03) in a medium optimized for growth of G. sulfurreducens (DB medium) (Speers and Reguera, 2012) with acetate and fumarate (DBAF medium) and supplemented with various concentrations of CoCl2. We measured growth with up to 1 mM CoCl2 (Figure 3A), a concentration commonly used to enrich for metal-resistant bacteria from soils and industrial wastes contaminated with heavy metals (Schmidt and Schlegel, 1989). Generation times increased (Figure 3B) and planktonic biomass yields (maximum OD660 during entry in stationary phase) decreased (Figure 3C) in a dose-dependent manner, as cells coped with higher levels of metal toxicity. For example, G. sulfurreducens cells doubled every 4.58 (± 0.05) hours in the untreated cultures, which we estimated to have approximately 27 μM of CoII using an assay we developed for the colorimetric detection of the metal in the culture medium. Supplementation with an additional 100 or 250 μM CoCl2 increased generation times to 5.41 (± 0.25) and 10.13 (± 0.54) hours, respectively (Figure 3B). Generation times increased even more at higher CoCl2 concentrations (500 and 1,000 μM) and, on average, one out of three replicate cultures did not resume growth after a week (Figure 3B). Furthermore, cultures that resumed growth did so after extended phases of acclimation (long lag phases before entering exponential phase) and reached lowest biomass yields (Figure 3C).
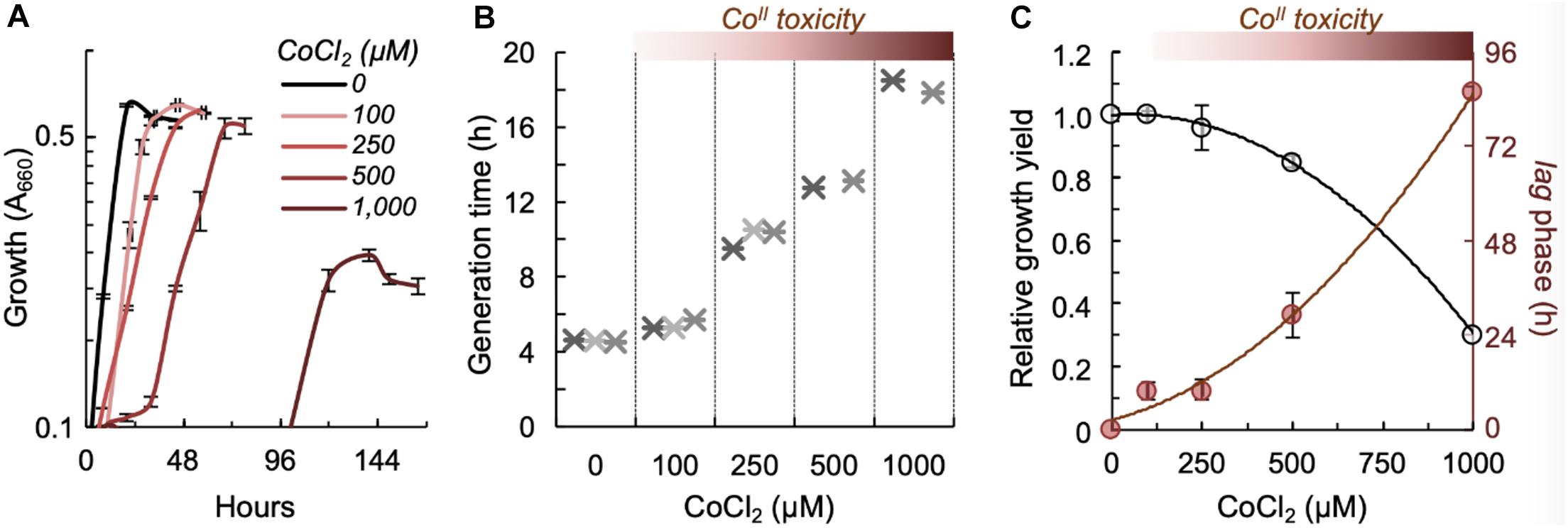
Figure 3. Effect of CoII on G. sulfurreducens growth. (A) Cell growth (absorbance at 660 nm) in acetate-fumarate cultures with or without CoCl2 supplementation (up to 1,000 μM). Data points for 0–250 μM CoCl2 treatments show average and standard deviation of triplicate cultures. Treatments with 500 and 1,000 μM CoCl2 show average and standard error of the only two replicates that resumed growth within a week. (B,C) Effect of CoII toxicity on growth efficiency. Panel (B) shows generation (doubling) times for each of the replicates in the untreated (0 μM) and treated (100–1,000 μM) cultures shown in (A). Panel (C) shows the effect of the CoCl2 treatment in reducing the culture’s growth yields (OD660 in early stationary phase relative to the untreated 0 μM cultures) or in extending the lag phase (time before entry in exponential phase). The trendlines in (C) are the polynomial fits for the average data points of relative growth yields (R2 = 0.999) and lag phases (R2 = 0.994) from the cultures shown in (A).
Transcriptomic Analysis Reveals Multiple Mechanisms for CoII Detoxification
We gained insights into the mechanisms that allow G. sulfurreducens to cope with CoII stress by comparing the transcript abundance of mid-log phase cells grown with or without 250 μM CoCl2 supplementation (Figure 4). CoII stress led to the differential expression of 47 genes. Of them, 32 were upregulated (Table 1) and 15 were downregulated (Table 2). This is approximately 0.9% (upregulated) and 0.4% (downregulated) of the genes annotated in the genome of G. sulfurreducens. Most of the upregulated genes encoded proteins with predicted roles in metal detoxification such as efflux pumps, protein and DNA repair enzymes, cell envelope modification pathways, and transcriptional regulation (Table 1). We also identified among the upregulated genes pathways for extracellular electron transfer that could provide a mechanism for energy transduction and CoII mineralization on the cell surface. By contrast, most of the downregulated genes coded for non-essential proteins with metal-binding domains or amino acids that CoII is known to bind strongly (Table 2). Thus, their downregulation reduces the burden of CoII retention in the cell’s proteome.
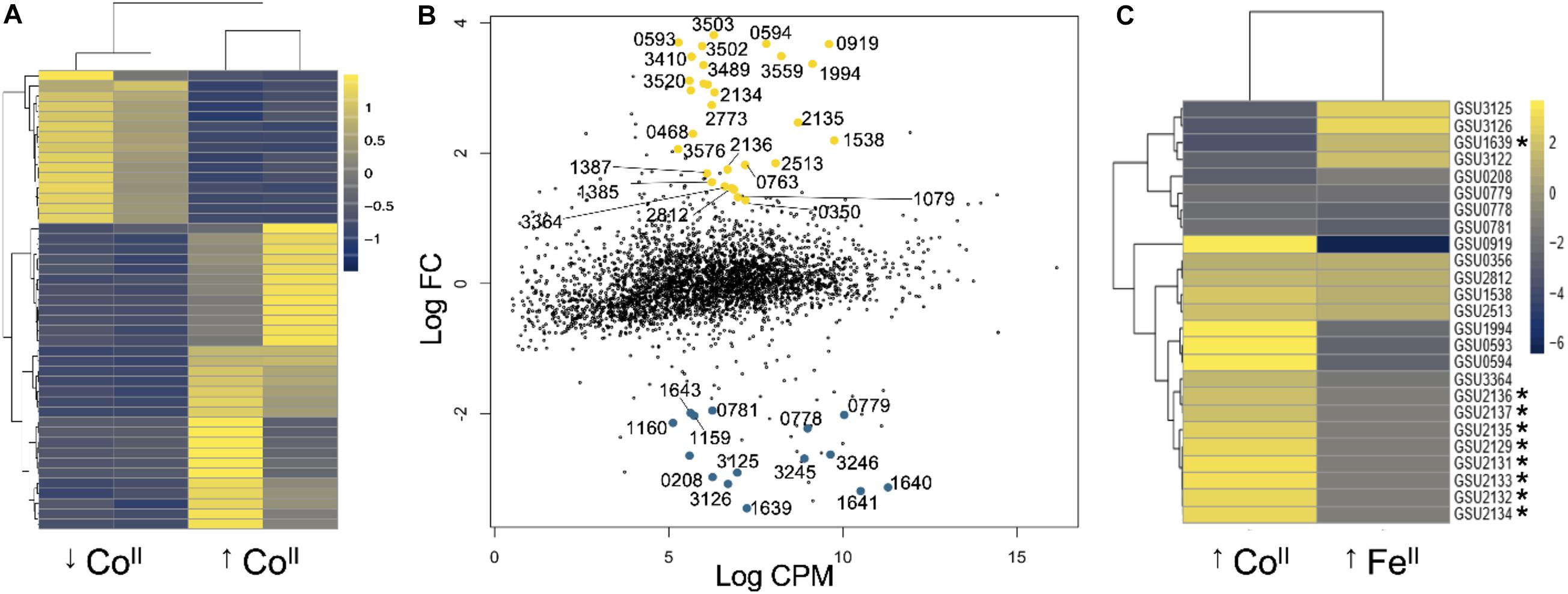
Figure 4. Transcriptional response of G. sulfurreducens to CoII. (A) Heatmap of the transcriptional response in two replicates for the untreated (↓CoII) and treated (↑CoII) cultures. The datasets and statistical analyses of the expression data are provided in Supplementary Data 1. (B) Dispersion plot of transcript abundance (log fold change [logFC] versus log counts per million [logCPM]) identifying the significantly upregulated (yellow) and downregulated (blue) genes. (C) Heatmap of genes differentially expressed with excess CoII or FeII. The latter was calculated as the inverse ratio of the log2 fold transcriptional changes reported for G. sulfurreducens cultures growing with sufficient versus excess FeII (Embree et al., 2014). The asterisk shows genes under Fur control (Embree et al., 2014). The calculations and data comparisons are provided in Supplementary Data 2.
Periplasmic Detoxification of CoII
The diffusion of CoII through non-selective outer membrane porins (Nikaido, 2003) leads to its rapid accumulation in the periplasmic space and risks disruption of essential cellular functions such as protein secretion and respiration. CoII toxicity in the periplasm is consistent with the upregulation of two periplasmic cytochromes (GSU1538 and GSU2513) with predicted roles in hydrogen peroxide (H2O2) detoxification (Figure 5). This suggests that CoII accumulated in the periplasm at levels high enough to catalyze Fenton-chemistry reactions yielding reactive oxygen species (ROS) (Barras and Fontecave, 2011). GSU1538 has the conserved domain of di-heme cytochrome c peroxidases (PF03150), a group of periplasmic enzymes that reduce H2O2 to prevent oxidative stress (Pettigrew et al., 2006). Bacterial cytochrome c peroxidases can receive electrons from small monoheme cytochromes (Pettigrew et al., 2006). The upregulation of GSU2513, a periplasmic monoheme cytochrome c protein, suggests a similar redox partnership with the GSU1538 peroxidase.
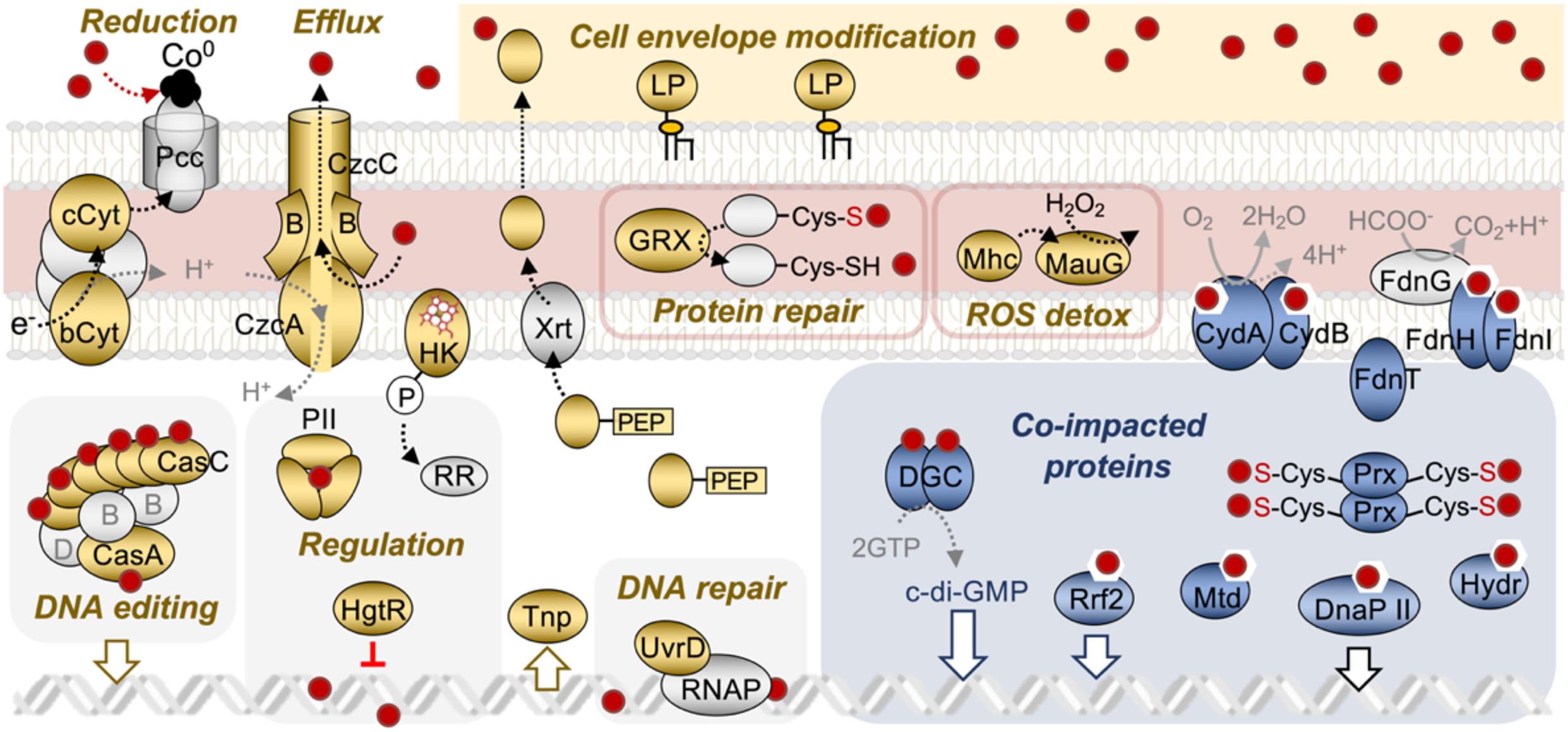
Figure 5. Model for the transcriptional response of G. sulfurreducens during growth under CoII stress. The figure illustrates pathways (upregulated, gold; downregulated, blue) differentially expressed under CoII stress. Proteins in gray represent proteins predicted to participate in the detoxification pathways that did not undergo differential expression. Additional information for the proteins and encoding genes is available in Tables 1, 2. bCyt, Cytochrome b; CasABCD, CRISPR-Cas complex; b/cCyt, Cytochrome b or c; CydAB, Cytochrome bc oxidase complex; DGC, diguanylate cyclase; FdhT, formate dehydrogenase chaperone; FdnGHI, formate dehydrogenase; GRX, glutaredoxin; HgtR, hydrogen-dependent growth transcriptional regulator; HK, heme-binding histidine kinase; Hydr, Hydrolase; LP, lipoprotein; MauG, MauG-like diheme peroxidase; Mhc, monoheme cytochrome c; Mtd, mannitol dehydrogenase; PII, P-II family nitrogen regulator (PII-NG); Pcc, Porin-cytochrome complex; RNAP, RNA polymerase; UvrD, UV repair protein D (DNA helicase of the nucleotide excision repair pathway); Xrt, exosortase.
CoII-stressed cells also upregulated GSU2812, a glutaredoxin-family protein (glutaredoxin motif, PF00462) containing a signal peptide (amino acids 1–27) for export to the periplasm. Glutaredoxins, like thioredoxins, are thioldisulfide oxidoreductases that reduce or oxidize disulfide bonds depending on the redox potential of the cellular compartment (cytoplasm or periplasm) where they operate (Eser et al., 2009). For example, E. coli secretes several thioredoxin proteins (e.g., DsbA and DsbC) to the periplasm to form disulfide bonds and fold proteins (Hatahet et al., 2014). A periplasmic monothiol glutaredoxin (glutaredoxin 3, Grx3) complements the activities of DsbA and DsbC in reactions dependent on the glutathione biosynthetic pathway (Eser et al., 2009). The high affinity of CoII for thiol groups in cysteines leads to the rapid oxidation of the amino acid and the formation of non-native disulfide bonds, which glutaredoxins can resolve to prevent protein inactivation (Hiniker et al., 2005).
We also identified among the upregulated genes an operon containing the three subunits of one of the four RND systems (GSU2135–2137) identified in the genome of G. sulfurreducens (Figure 2). This RND transporter has a membrane-bound metal pump (GSU2135) homologous to CzcA from Cupriavidus metallidurans strain CH34 and CusA from E. coli (Kim et al., 2011). The pump binds the metal in the periplasm and undergoes conformational changes that move one proton into the cytoplasm and translocate the metal through a channel formed by the B and C subunits (Figure 5). CusABC complexes often work coordinately with periplasmic metal chaperones (CusF) to transport monovalent cations (CuI and AgI). The lack of CusF chaperones in the G. sulfurreducens genome suggests that the CoII RND transporter is a CzcABC system. Indeed, CzcABC transporters receive their name for their ability to mobilize the divalent cations cobalt, zinc, and cadmium (Kim et al., 2011). Furthermore, this metal efflux system plays roles in CoII detoxification and resistance in other bacteria (Ma et al., 2020). Thus, we designated the GSU2135–2137 genes as czcABC (Table 1).
Cytoplasmic Detoxification of CoII
The first gene in the czcABC operon (GSU2134) codes for a protein with the conserved PII domain (PF00543) of nitrogen regulatory proteins. These proteins form homotrimers to bind metabolites signaling the energy (ATP, ADP), carbon (2-oxogluratate) and nitrogen (glutamine and 2-oxoglutarate) levels inside the cell (Huergo et al., 2013). GSU2134 belongs to a phylogenetically distinct clade of proteobacterial PII proteins (PII-NG) that evolved from the canonical nitrogen regulators GlnB and GlnK (Sant’Anna et al., 2009). Like most of the PII-NG proteins (Sant’Anna et al., 2009), GSU2134 clusters in the genome with the genes encoding a proton-cation CzcABC antiporter. Furthermore, PII-NG is a structural homolog of the metal-binding protein CutA1 of E. coli (Arnesano et al., 2003). CutA1 binds the divalent copper cation (CuII) at a site structurally equivalent to the ATP binding site of PII-NG proteins (Arnesano et al., 2003) and uses metal binding to regulate genes involved in CuII tolerance (Fong et al., 1995). The structural homology of PII-NG and metal sensors together with its cytoplasmic location are consistent with a role in intracellular CoII sensing and modulation of the regulatory cascade needed for cell acclimation to metal stress.
Indirect Effects of CoII Stress on FeII Homeostasis
In G. sulfurreducens, the operon encoding the PII-NG regulator (GSU2134) and CzcABC proton/metal antiporter (GSU2135–2137) is also upregulated under FeII limitation via the master regulon of FeII homeostasis, Fur (Embree et al., 2014). To test if CoII intoxication could indirectly limit the availability of FeII, we used published transcriptomic data for G. sulfurreducens grown with sufficient versus excess FeII (Embree et al., 2014) to identify genes differentially expressed under FeII intoxication. More than half of the genes responding to CoII stress (24 in total) were also differentially expressed during FeII intoxication (Figure 4C). However, most of the genes had opposite patterns of expression, supporting the idea that CoII intoxication limits FeII availability. For example, a cluster of Fur-regulated genes comprised of the PII-NG-czcABC operon (GSU2134–2137) and upstream genes (GSU2129 and GSU2131–33) were upregulated by CoII stress but downregulated in cells growing with excess FeII.
We also identified a protein (GSU1639) with the conserved Rrf2 domain (PF02082) of FeII-dependent transcriptional regulators (Keon et al., 1997; Schwartz et al., 2001) that was downregulated under CoII stress but upregulated during FeII intoxication (Figure 4C). The Rrf2 domain ligates Fe or Fe-S clusters via redox-sensitive cysteine residues to tune the protein’s DNA specificity to FeII homeostasis (Rajagopalan et al., 2013). For example, the Rrf2 domain of E. coli IscR has three cysteines and one glutamic acid that bind Fe-S clusters to regulate genes involved in Fe-S cluster biosynthesis as a function of FeII availability (Schwartz et al., 2001). GSU1639 shares 55% similarity (33% identity) with IscR and has the conserved cysteines and glutamic acid needed for Fe-S cluster coordination at the Rrf2 domain. Furthermore, it is under direct control of Fur, the master regulator of FeII homeostasis (Embree et al., 2014). This suggests that GSU1639 binds Fe-S clusters to co-regulate the cluster’s biosynthetic pathways to FeII homeostasis. CoII infiltration in Fe-S clusters and/or its high affinity for the cysteines in the Rrf2 binding site could prevent the regulator from sensing the Fe-S cluster signal and impair the ability of the cells to sense FeII availability.
CoII but not FeII toxicity upregulated the hydrogen-dependent growth transcriptional repressor HgtR (GSU3364), a master regulator of central metabolism (Figure 4C). HgtR downregulates genes involved in energy generation and biosynthesis such as gtlA (citrate synthase in TCA cycle), atpG (ATP synthase F0 β’ subunit), and nuoA (NADH dehydrogenase I, A subunit) to tune growth rates to the cell’s nutritional status (Ueki and Lovley, 2010). The overexpression of the repressor provides a mechanism to adjust growth to the energy demands of cells coping with CoII intoxication and low FeII availability. FeII limitation may have also triggered the induction of GSU0356, a heme-binding sensor histidine kinase that could regulate the cellular response to the accumulation of metal-free or CoII-impacted heme groups (Table 1). This histidine kinase lacks a signal peptide but contains three internal helices for insertion in the inner leaflet of the inner membrane, a subcellular localization optimal for cytoplasmic sensing. In addition to phosphoacceptor (HisKinA, PF00512) and ATPase (HATPase, PF02518) domains, the GSU0356 histidine kinase has a domain of unknown function (DUF3365, PF11845) with a heme-binding site (CXXCH sequence). Heme-responsive histidine kinases typically bind the heme group reversibly (Girvan and Munro, 2013). This sensory capacity allows the cells to prevent the toxic build-up of metal-free hemes (Dailey et al., 2017). The upregulation of the heme sensor during CoII and FeII intoxication (Figure 4C) suggests that both conditions may have resulted in heme toxicity.
Evidence for DNA Damage
CoII-stressed cells upregulated components of one of the two Type I CRISPR loci (CRISPR2) in G. sulfurreducens (GSU1385 and GSU1387) (Table 1). The CRISPR2 locus (GSU1384–1393) contains 8 CRISPR-associated (Cas) proteins and an array with 143 spacers. The locus lacks a Cas4 protein but has Cse1 and Cse2 (named after the CRISPR system of E. coli) components, meeting the criterion for classification as a subtype I-E CRISPR (Makarova and Koonin, 2015). CoII-stressed cells upregulated Cse1 (GSU1385, also known as CasA), the large subunit of the antiviral defense Cas complex (Cascade) that facilitates RNA-guided recognition of complementary DNA (Makarova and Koonin, 2015). Cse1 recognizes a short protospacer adjacent motif (PAM) in the crRNA and discriminates self from foreign DNA targets (Westra et al., 2013). A Zn-finger motif in Cse1 binds ZnII to control interactions with the target DNA (Gorelsky et al., 2005). This structural motif is sensitive to infiltration by CoII, a metal that changes the selectivity of the Cas complex for the target DNA and stimulates its nicking activity (Sundaresan et al., 2017). CoII also upregulated Cse4 (GSU1387, also known as CasC or Cas7), a protein that polymerizes as a hexameric arch along the spacer region of the crRNA within the Cascade complex (Zheng et al., 2020). Cse4 has a ferredoxin-like fold in its RNA recognition motif (Makarova and Koonin, 2015) with a conserved metal-binding βαββαβ fold that could bind CoII (Waldron and Robinson, 2009; Smith et al., 2015). The final result is a CoII-compromised Cascade complex with increased nicking activity that could lead to DNA damage. CoII can also infiltrate the DNA helix and cause structural changes and breaks in the strands (Kanellis and Dos Remedios, 2018). Consistent with the need to repair damaged DNA, cells upregulated a UvrD helicase (GSU0763) of the nucleotide excision repair pathway. UvrD can also bind RNA polymerase (RNAP) stalled on the DNA lesions and backtrack the enzyme to expose the damaged site to DNA repair proteins (Epshtein et al., 2014). This mechanism allows the RNAP to resume transcription as soon as the repair has concluded.
Downregulation of Non-essential Metalloproteins
Most of the downregulated genes encoded non-essential proteins with prosthetic groups, metal-binding motifs and amino acids sensitive to CoII inactivation (Table 2). Almost all of the targets where cytoplasmic or periplasmic redox-active proteins with FeII-prosthetic groups (e.g., hemes and Fe-S clusters) or proteins with thiol-containing cysteines that CoII readily binds and inactivates (Figure 5). For example, metal-stressed cells downregulated the two subunits (CydAB) of the cytochrome bd complex (GSU1640–1641), a respiratory quinol:O2 oxidoreductase widespread in prokaryotes that conserves energy from the transfer of electrons from the menaquinone pool to O2 (Borisov et al., 2011). Thus, the CydAB complex is not needed under the strictly anaerobic conditions used in our study. Similarly, cells downregulated two subunits (GSU0778–0779) of the trimeric formate dehydrogenase enzyme, FdnGHI, and the associated secretory protein FdnT (GSU0781), which are only needed when growing with formate as electron donor. Another example of a downregulated protein is Prx-2 (GSU3246), a cytoplasmic thioredoxin peroxidase of the 2-cysteine peroxiredoxin subfamily (Poole and Nelson, 2016). These thiol-based peroxidases scavenge the low levels of H2O2 produced intracellularly during normal growth and transduce the H2O2 signal to control cellular homeostasis (Toledano and Huang, 2016). When hyperoxidized, however, the enzymes aggregate and become chaperone holdases to protect proteins from denaturation (Toledano and Huang, 2016). The affinity of CoII for the thiol groups of peroxiredoxins could impair these functions. Thus, cells downregulate its expression to minimize negative impacts of metal inactivation on the cellular stress response.
Reductive Precipitation of CoII as a Detoxification Mechanism
We identified among the most upregulated genes two cytochrome-encoding genes (GSU0593 and GSU0594) that could participate in the extracellular reduction of CoII. GSU0593 and GSU0594 are the cytochrome b (CbcB) and cytochrome c (CbcA) subunits, respectively, of the Cbc5 menaquinol:ferricytochrome c oxidoreductase, a pentasubunit complex expressed during the reduction of FeIII oxide minerals (Aklujkar et al., 2013). The cytochromes (b and c1) and Fe-S proteins in cytochrome bc complexes transfer electrons from the menaquinone pool via a proton motive Q-cycle pathway (Trumpower, 1990). To complete the Q cycle, Cbc5 catalyzes two “redox turnovers” that consume two protons in the cytoplasm and release four protons in the periplasm. Thus, each Q cycle transfers two electrons and contributes two protons to the transmembrane proton gradient. Spanning the inner and outer membranes, the Cbc5 complex could electronically connect the menaquinone carriers with Geobacter outer membrane porin-cytochrome c complexes (Pcc) (Shi et al., 2016). Given the known role of Pcc complexes in the reductive precipitation of some divalent cations to their elemental, metallic form (Hu et al., 2013), we examined untreated and CoII-treated cells by transmission electron microscopy (TEM) for the extracellular formation of metal nanoclusters (Figure 6A). To prevent artifactual mineralization of heavy metal salts used to negatively stain cells for TEM (Brenner and Horne, 1959), we examined unstained cells. This approach allowed us to visualize numerous electron dense nanoparticles on the surface of CoII-stressed cells that were absent in untreated samples (Figure 6A). The homogenous dispersal of the nanoclusters is consistent with the distribution of outer membrane cytochrome foci in G. sulfurreducens (Shi et al., 2016). Further, X-ray energy dispersive spectroscopy (EDS) analyses confirmed the presence of Co on the outer surface of the treated cells but not in cell-free controls, consistent with the immobilization of the metal on the cell surface (Figure 6B). Using a colorimetric assay based on the color response of CoII when bound by 2-beta-mercaptoethanol (Figure 6B), we estimated that CoII-stressed cells had removed from the solution an average of 25 μM of the metal (Figure 6C). These results suggest that the detoxification response of G. sulfurreducens also included pathways for CoII mineralization, as reported for the uranyl cation (Cologgi et al., 2011).
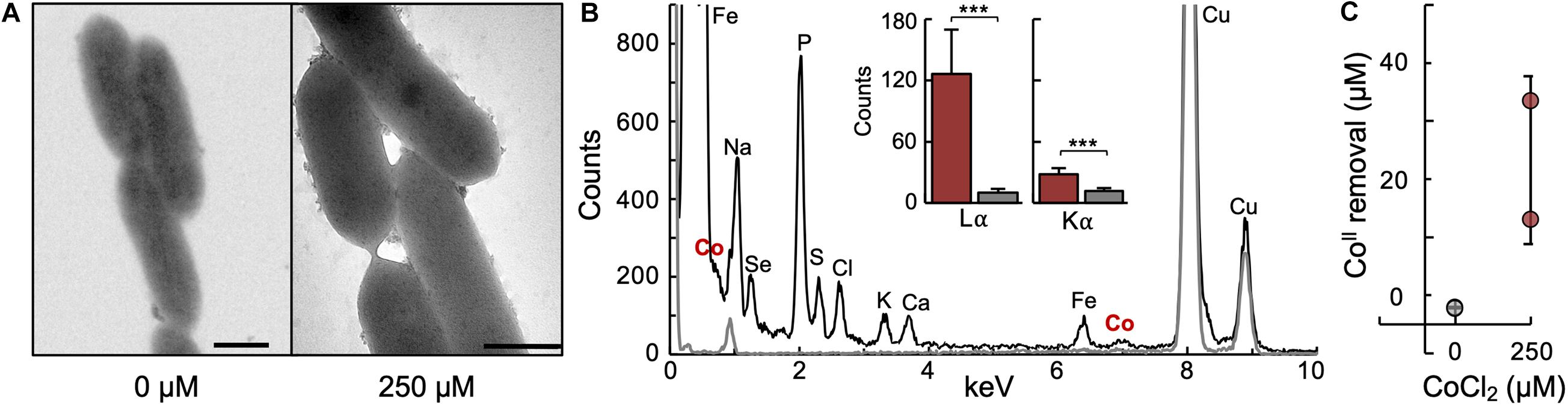
Figure 6. CoII mineralization at the cell outer surface. (A) Transmission electron microscopy (TEM) images of unstained cells from untreated (0 μM CoCl2) and treated (250 μM CoCl2) cultures. Scale bar, 500 nm. (B) Representative X-ray energy dispersive (EDS) spectra of cells from 250 μM CoCl2 cultures (black) and cell-free control areas examined by TEM identifying Co energy signatures from the cells. The inset shows the average energy intensity (counts) detected for the primary X-ray Co emission peaks (Lα, 0.776 keV; Kα, 6.924 keV) for four different cells (maroon) and control (gray) samples. Pairwise comparisons (t-test) between the cell and cell-free Lα and Kα average intensities produced p values below 0.0001 (***). (C) CoII removal by cells in cultures with or without 250 μM CoCl2 supplementation. The initial and final (early stationary phase) CoII concentrations in culture supernatant fluids were calculated colorimetrically after complexation with 2-beta-mercaptoethanol and using a standard curve (linear fit from 0 to 500 μM CoCl2; R2 = 0.9947). The difference between the final and initial concentration of CoII estimated the amount of metal removed by the cells.
Cell Envelope Modifications to Prevent CoII Infiltration and Form Biofilms
The transcriptomics analyses identified lipoproteins (GSU2133 and 3576) and EPS-associated proteins (GSU1079 and GSU1994) that could have modulated the properties of the cell surface to prevent metal infiltration and promote its extracellular immobilization (Cologgi et al., 2014). At least one of the lipoproteins was predicted to be targeted to the outer membrane, the other one could only be confirmed as non-cytoplasmic (Table 1). Lipid modification of exported hydrophilic proteins facilitates their anchoring to the inner leaflet of the outer membrane yet most, if not all, of the lipoproteins get translocated to the outer leaflet (Schulze and Zuckert, 2006; Konovalova and Silhavy, 2015). Surface-exposed lipoproteins control the permeability of the cell to soluble substrates and can also mediate cell adhesion (Konovalova and Silhavy, 2015). Additional modifications to the cell envelope are expected from the upregulation of two proteins (GSU1079 and GSU1994) carrying the PEP-CTERM motif (PF07589) of EPS-associated proteins (Haft et al., 2006). The motif comprises a carboxy-terminal (CTERM) Pro-Glu-Pro (PEP) recognition peptide, a transmembrane helix and an arginine-rich cluster (Haft et al., 2006). The protein sorting signal is recognized and cleaved by a dedicated exosortase (Xrt) in the inner membrane and the mature protein is then exported to the EPS matrix by yet unknown secretory pathways (Haft et al., 2006). Except for the presence of the conserved sorting signal, PEP-CTERM proteins have little homology to other proteins. Most are, however, rich in serine and threonine, suggesting they are glycosylated during export (Haft et al., 2012). The genome of G. sulfurreducens encodes 5 PEP-CTERM proteins, an EpsH family Xrt exosortase (GSU1979) and a two-component system (PrsK histidine kinase, GSU1941; PrsR response regulator, GSU1940) predicted to regulate export (Haft et al., 2006).
The widespread presence of PEP-CTERM proteins in Gram-negative bacteria that form biofilms suggests a role for these proteins in the development of surface-attached communities (Haft et al., 2006). In support of this, the expression of EPS-associated proteins in CoII-stressed cells of G. sulfurreducens preceded the formation of thick biofilms at the bottom of the tube once the cultures reached stationary-phase (Figure 7A). We did not identify in the transcriptome any of the genes that encode proteins required for the synthesis of the biofilm EPS, Xap (Rollefson et al., 2011). This is not unexpected, because G. sulfurreducens expresses the xap genes during exponential growth in acetate-fumarate cultures (Rollefson et al., 2011). The Xap EPS anchors outer membrane cytochromes (Rollefson et al., 2011) and provides a mechanical and redox barrier to the permeation of soluble divalent metal cations in biofilms (Cologgi et al., 2014). To test for a similar protective effect by the EPS produced by planktonic cells, we challenged mid-log phase cultures with up to 1 mM concentrations of CoCl2 and monitored the effect of the metal treatments in growth (OD660). As shown in Figure 7B, metal shock had little effect on growth efficiency in any of the cultures. Such high levels of metal resistance are consistent with the role of the EPS matrix in preventing metal permeation. Furthermore, planktonic cultures challenged with the metal did not form thick biofilms in stationary phase (Figure 7A). Thus, biofilm formation appears to be an adaptive response to persistent metal toxicity.
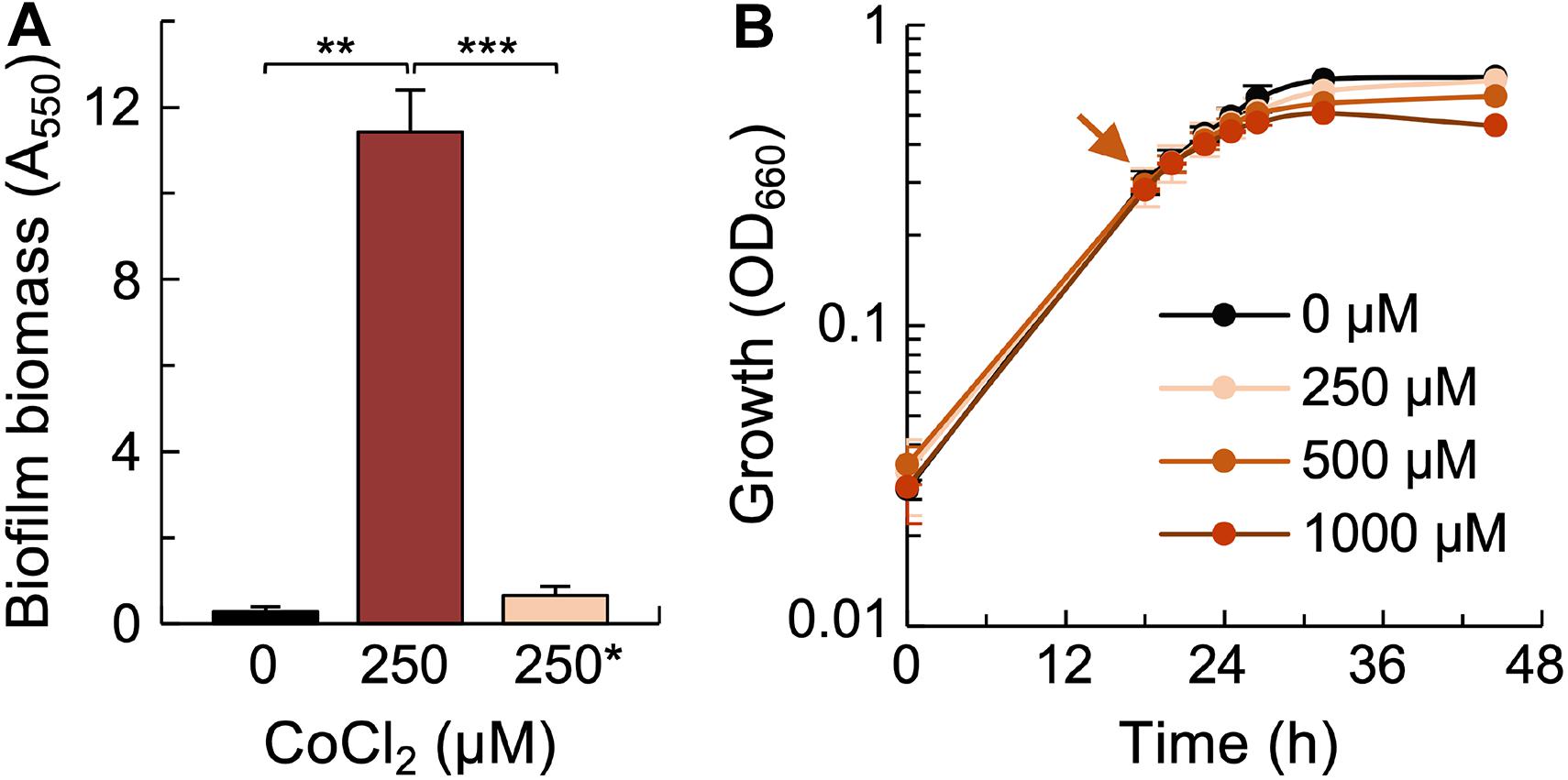
Figure 7. Adaptive responses of cells to CoII stress. (A) Biofilm formation (absorbance at 550 nm of biofilm-associated crystal violet) in stationary phase cultures growing with 250 μM CoCl2 compared to the lack of biofilms in cultures growing without CoCl2 supplementation (0 μM) or challenged with the CoCl2 in mid-log phase (∼0.3 OD660) (250∗). Statistically significant differences in pairwise comparisons (t-test) are highlighted with asterisks (∗∗p < 0.0002 or ∗∗∗p < 0.0001). (B) Planktonic growth of cultures challenged with increasing concentrations of CoCl2 (arrow) in mid-log phase (∼0.3 OD660), including the ones used for the biofilm assays shown in panel (A) (250∗).
Discussion
The high CoII tolerance and complex acclimation response of G. sulfurreducens is consistent with selection mechanisms during long-term environmental exposure to the metal. FeIII and MnIV oxides form heterogenous mixes with natural organic matter and metal micronutrients (Huang and Zhang, 2020) that provide optimal conditions for the growth of Geobacter species (Reguera and Kashefi, 2019). The high reactivity of the FeIII and MnIV (hydr)oxides sequesters CoII and other metal cations in the mineral phases (Backes et al., 1995; Krupka and Serne, 2002), concentrating them in the metal oxide-rich epigenetic zones (Burkhardt et al., 2009). The reductive dissolution of the metal-bearing minerals mobilizes the metal cations (Huang and Zhang, 2020) and increases their concentration in the pore-water to toxic levels (Weber et al., 2009). CuII, for example, can be mobilized to levels (∼20 μM) above the minimum concentration (10 μM) that inhibits the growth of G. sulfurreducens in the laboratory (Kimber et al., 2020). Yet, this bacterium grew from low cell densities, albeit with trade-offs in growth efficiency, in the presence of up to 1 mM CoCl2 (Figure 3). Furthermore, it was relatively unaffected when exposed to the same metal concentrations during the exponential phase of growth (Figure 7). We attributed this to the expression in exponentially growing cells of the biofilm EPS (Rollefson et al., 2011), which can shield the cells from metal infiltration. Cell density can also affect cellular metabolism and the secretion of metabolites that change the chemical speciation, bioavailability, and toxicity of metals (Franklin et al., 2002). Furthermore, increases in cell numbers activate stress responses that acclimate the population and increase tolerance to a number of stressors (Li et al., 2001). By contrast, cells inoculated at low densities must first reprogram their physiology to acclimate to and initiate growth in the presence of the metal stressor. Acclimation is evident in the extended periods of growth arrest (lag phase) that G. sulfurreducens cultures initially experienced when growing with sublethal concentrations of CoCl2 (Figure 3) and in the multiple cellular pathways that were activated to couple growth to CoII detoxification (Figure 4).
The transcriptomic studies provided insights into the extensive transcriptional reprogramming that allowed G. sulfurreducens to cope with CoII stress (Figure 5). Transcript levels for CoII importers remained constant, consistent with the absence in G. sulfurreducens of transcriptional regulators (e.g., CzrA and CoaR) that other bacteria use to directly control CoII uptake for metal homeostasis (Waldron and Robinson, 2009). Instead, G. sulfurreducens acclimation involved metal (PII-NG) and heme (GSU0356 histidine kinase) sensors and a transcriptional regulator of central metabolism (HgtR) (Figure 5). Cells also upregulated a CzcABC pump for proton-driven export of metal traversing the outer membrane, a canonical mechanism used by other Gram-negative bacteria to increase metal resistance (Ma et al., 2020). In addition, CoII upregulated a periplasmic glutaredoxin, which repairs and rejoins cysteines oxidized by CoII to refold proteins to their native and functional conformation (Ezraty et al., 2017). The activation of a periplasmic MauG-like di-heme cytochrome c peroxidase (GSU1538) suggested that CoII accumulated in the periplasm at levels sufficiently high to generate H2O2 (Barras and Fontecave, 2011). Di-heme cytochrome c peroxidases detoxify H2O2 in the periplasm by reducing it to two H2O molecules (Pettigrew et al., 2006). This reaction receives electrons from a dedicated electron donor such as the monoheme cytochrome GSU2513, which was also upregulated by CoII (Table 1). Without the peroxidase-cytochrome pair, H2O2 would oxidize solvent-exposed [4Fe-4S]2+ clusters in proteins, producing inactive [3Fe-4S]3+ species that abolish the redox activity of the metalloprotein (Imlay, 2008). The detoxification of H2O2 is also important to prevent Fenton-like reactions that generate highly toxic ∙OH radicals and exacerbate oxidative stress (Leonard et al., 1998).
Despite mechanisms for periplasmic detoxification, CoII may have infiltrated the cytoplasm and damaged essential macromolecules. The presence of cytoplasmic chelators such as glutathione facilitates reactions between CoII and H2O that generate ROS and oxidatively damage DNA (Leonard et al., 1998). CoII can also bind components of the CRISPR Cascade complex that mediates antiviral defense, changing its specificity for target DNA and stimulating its RNA-independent DNA cleavage activity (Sundaresan et al., 2017). To cope with DNA damage, G. sulfurreducens activated the expression of UvrD, a helicase of the nucleotide excision repair pathway (Kamarthapu and Nudler, 2015) and transcription-coupled repair (Epshtein et al., 2014). The latter is particularly important to maintain the transcriptional activity of the cell during metal intoxication. This is because UvrD associates with NusA to backtrack RNAP when stalled at a DNA lesion. The helicase then recruits the UvrAB repair complex to the damaged site (Epshtein et al., 2014). This intervention allows the RNAP to resume transcription as soon the lesion is repaired (Kamarthapu and Nudler, 2015).
The Irving-Williams series (MnII < FeII < CoII < NiII < CuII > ZnII) predicts greater stability for CoII than FeII or MnII complexes independently of the ligand (Hill and Sadler, 2016). As a result, CoII intoxication preferentially impacts FeII and MnII metalloproteins. To prevent the retention of the toxic metal in the metalloproteome, G. sulfurreducens downregulated non-essential proteins with FeII prosthetic groups (Figure 5). Nearly all of the downregulated proteins contained Fe-S clusters or metallocenters coordinating FeII atoms (Table 2). The chemical similarities with FeII facilitate the infiltration of CoII into Fe-S clusters but the greater electron density of CoII alters the coordination of the metal with the enzyme and its activity (Thorgersen and Downs, 2007; Waldron and Robinson, 2009). CoII is also able to compete with FeII for binding to the porphyrin ring of heme groups such as those in cytochromes (Thorgersen and Downs, 2007). This could be catastrophic in the periplasm, where heme-containing respiratory chains are particularly abundant. CoII-hemes are weaker transporters of charges than the native FeII-hemes (Majtan et al., 2011), impairing, or even abolishing, respiratory growth. To compensate for this, G. sulfurreducens downregulated non-essential heme-containing proteins such as the cytochrome bd oxidase subunits CydAB required for aerobic respiration (Figure 5). Similarly, cells downregulated genes encoding the formate dehydrogenase complex (the Fe-S cluster protein FdnH and the cytochrome b FdnI) and the secretory accessory protein FdnT, as these proteins are only needed for formate-dependent growth. Cells also downregulated an Rrf2 protein (GSU1639), which uses cysteine residues to bind Fe-S clusters and co-regulate Fe-S cluster biosynthesis and FeII homeostasis (Schwartz et al., 2001). The high affinity of CoII for cysteines may prevent Rrf2 protein from sensing Fe-S cluster availability in the cytoplasm. To prevent further deregulation of FeII homeostasis, cells downregulated the rrf2 gene (Table 2).
The principles of the Irving-Williams series (Hill and Sadler, 2016) also explain the high affinity of CoII for FeII-heme. Downregulating non-essential proteins with FeII-hemes can provide some partial relief (Table 2). However, CoII can also infiltrate the FeII-hemes during their biosynthesis and prevent their incorporation into proteins. This leads to the accumulation of free CoII-hemes in the cytoplasm and cytotoxicity (Lin and Everse, 1987). The upregulation of a heme-containing histidine kinase (GSU0356) (Table 1) could provide a mechanism to sense the impact of CoII on the heme pool and coordinate the heme detoxification response, as reported in other bacteria (Anzaldi and Skaar, 2010). The advantage of this heme-sensing mechanism is that cells can simultaneously co-regulate heme biosynthesis to CoII and FeII homeostasis (Dailey et al., 2017). We initially reasoned that CoII infiltration in the free hemes could have increased the intracellular levels of FeII and exacerbate metal toxicity (Lin and Everse, 1987; Anzaldi and Skaar, 2010). For example, free FeII, like CoII, can generate ROS via Fenton chemistry and cause intracellular damage (Everse and Hsia, 1997). However, although CoII and FeII intoxication had overlapping transcriptional responses, most of the shared gene targets were reversely regulated (Figure 4C). Thus, cells faced conditions of FeII limitation during CoII intoxication. The accumulation of CoII in the periplasm could competitively exclude FeII from import across the inner membrane, reducing its intracellular availability. Furthermore, once removed from metalloproteins and prosthetic groups, FeII can be sequestered non-specifically by cytoplasmic chelators, effectively reducing its intracellular availability.
In addition to mechanisms for metal detoxification in the periplasm and cytoplasm, G. sulfurreducens induced pathways that could have promoted the extracellular immobilization of the metal. For example, cells upregulated outer membrane lipoproteins that could have modulated the permeability of the outer membrane (Nikaido, 2003) and/or function as adhesins to promote cell-cell aggregation (Konovalova and Silhavy, 2015). Additionally, CoII triggered the expression of EPS-associated proteins (PEP-CTERM proteins) typically expressed by biofilm-forming bacteria (Haft et al., 2006). The synthesis by planktonic cells of G. sulfurreducens of the biofilm EPS (Xap) precedes biofilm formation and allows the cell to anchor to the Xap matrix cytochromes needed for metal reduction (Rollefson et al., 2011). This redox activity could allow the planktonic cells to reductively precipitate CoII on the cell surface, generating the metal nanoclusters visualized by TEM (Figure 6A). The mineral particles resolved by TEM formed on discreet foci on the cell surface, similarly to the distribution of outer membrane cytochromes of the Pcc complexes (Qian et al., 2007). Furthermore, the Pcc outer membrane cytochromes can bind and reductively precipitate divalent metal cations to their elemental form (e.g., HgII to Hg0) (Hu et al., 2013). A similar reaction could allow the cytochromes to reductively precipitate CoII to Co0 on the cell surface. The Pcc outer membrane cytochrome complexes contain periplasmic and extracellular c-type cytochromes within an outer membrane porin to electronically connect periplasmic carriers to extracellular electron acceptors (Shi et al., 2016). The upregulation by CoII of a respiratory cytochrome bc complex (Cbc5) could provide a mechanism for energy conservation from the reduction of CoII at the Pcc foci (Figure 5). The Cbc5 complex is anchored to the inner and outer membranes and could interact with the periplasmic cytochrome of the Pcc complex to complete the electron transfer pathway to CoII (Figure 5). Although none of the Pcc genes were differentially expressed by CoII, we confirmed the upregulation of the Pcc outer membrane c-cytochrome OmcC (GSU2731) when the false discovery rate (FDR) threshold was increased from 0.05 to 0.08. This could indicate that some cells may be upregulating the PccC cytochrome. Alternatively, cells may constitutively produce the Pcc complexes under the culture conditions used in our study. Experimental testing of this hypothesis is warranted.
The expression of lipoprotein adhesins and a redox-active EPS could also have allowed cells to aggregate and form biofilms (Figure 7A), an adaptive response that confers on G. sulfurreducens increased resistance to soluble, toxic metals (Cologgi et al., 2014). The downregulation of a cytoplasmic diguanylate cyclase (DGC) with a canonical GGDEF domain (GSU1643) (Table 2) in CoII-stressed cells may have reduced the intracellular levels of c-di-GMP in order to regulate the planktonic-to-biofilm transition. Most DGC enzymes contain sensory domains that modulate the synthesis of the bacterial second messenger bis-(3′,5′)-cyclic dimeric guanosine monophosphate (c-di-GMP) to specific input signals, including metals. For example, ZnII reversibly binds the subunits of the E. coli DgcZ dimer (formerly YdeH) to allosterically regulate the synthesis of c-di-GMP (Zähringer et al., 2013). The Geobacter DGC enzyme does not have metal-binding domains but has instead the N-terminal phosphoreceiver (REC) domain of DGCs in the PelD superfamily (Table 2). The best studied PelD-like DGC is WspR, the response regulator of the Wsp chemosensory pathway that regulates cell-cell aggregation and biofilm formation in Pseudomonas aeruginosa (D’Argenio et al., 2002). Phosphorylation of the receiver domain in the WspR dimer activates the synthesis of c-di-GMP and autoaggregative/biofilm phenotypes (Hickman et al., 2005). MgII cations bind near the receiver’s active site of the WspR dimer and contribute to its activity (De et al., 2008). The downregulation in CoII-stressed cells of the DGC enzyme could reflect a feedback mechanism to the infiltration of CoII in the protein (Waldron and Robinson, 2009). Alternatively, CoII-stressed cells may have downregulated the WspR-like DGC to reduce GTP demand for c-di-GMP and increase the availability of the nucleotide triphosphate for EPS synthesis (Rehm, 2010). The EPS matrix can then promote cell-cell aggregation and biofilm formation as a protective mechanism against metal toxicity (Cologgi et al., 2014).
Biofilm formation in G. sulfurreducens embeds the cells in an electroactive matrix of cytochromes and conductive pili that effectively immobilizes soluble metals (Cologgi et al., 2014). The conductive pili are particularly important to overcome metal toxicity in biofilms because they provide a large redox surface area for the extracellular immobilization and reductive precipitation of toxic metals (Cologgi et al., 2011, 2014). The pilus surface is decorated with specialized motifs optimal for the coordination of divalent metal cations (Feliciano et al., 2015). These metal traps have high affinity for CoII and, at high enough potentials, can reductively precipitate it as Co0 nanoparticles (Cosert and Reguera, 2019). Furthermore, the conductive pili are retractable appendages (Speers et al., 2016), a dynamic feature that allows cells to detach the minerals and recycle the structural peptides in the membrane for a new cycle of pilus polymerization and metal reduction (Reguera, 2018). We did not identify in the CoII transcriptome any of the genes encoding proteins of the pilus biosynthetic apparatus (Table 1) nor did we observe pilus filaments by TEM (Figure 6). This was not unexpected because we used growth temperatures (30°C) that prevent pilus assembly in planktonic cells (Reguera et al., 2005; Cologgi et al., 2011). Under these conditions, cytochrome respiratory chains involving outer membrane Pcc complexes provided the primary pathway for extracellular electron transfer in CoII-stressed cells. Thus, Pcc cytochromes could have promoted the mineralization of CoII on discreet surface foci as a detoxification mechanism (Figure 6).
The presence of metal nanoclusters on the surface of CoII-treated cells suggests that hitherto unknown biological reactions could contribute to the geochemical cycling of this important metal. We estimated that, on average, cells removed from the solvent 25 μM concentrations of CoII (Figure 6C). As a comparison, the intracellular CoII quota is in the low to sub-μM range and typically below the limits of detection of mass spectrometry assays (Outten and O’Halloran, 2001). CoII biomineralization may be more significant in biofilms, thanks to the concentration in the biofilm matrix of conductive pili (Cologgi et al., 2014; Steidl et al., 2016) with high affinity motifs for CoII binding and reduction to Co0 (Cosert and Reguera, 2019). These adaptive responses confer on Geobacter a competitive advantage for growth in metal-rich environments despite the mobilization of CoII during the reductive dissolution of metal oxide mineral phases. The ability of Geobacter bacteria to reductively precipitate CoII could also alleviate metal stress on syntrophic partners that depend on interspecies cobamide transfer to sustain their metabolism. Furthermore, the formation of Co0 nanoparticles effectively metallizes the cell surface and could allow Geobacter cells to gain energy from the reduction of low potential electron acceptors and to transfer respiratory electrons to syntrophic partners. Hence, CoII mineralization may help define the niche space of Geobacter-driven microbiomes and provide molecular markers to predict the impact of their activities in the fate of this and other essential elements.
Materials and Methods
Genomic Reconstruction of Pathways for CoII Transport and Assimilation
We performed a literature survey and used the KEGG database and BLAST searches to identify genes in the G. sulfurreducens genome with a predicted role in CoII homeostasis and assimilation into cobamide synthesis. The subcellular localization of the protein products was predicted with PSORTb 3.0 (Yu et al., 2010).
Bacterial Strains and Culture Conditions
Geobacter sulfurreducens strain PCA was obtained from our laboratory culture collection and routinely maintained in anaerobic mineral medium DB with 20 mM acetate and 40 mM fumarate, as described elsewhere (Speers and Reguera, 2012). The cultures were incubated at 30°C while periodically monitoring growth as optical density at 660 nm (OD660). Unless otherwise indicated, culture transfers to fresh medium were in mid-log phase (OD660, 0.3–0.4) and diluting the cells to an initial OD660 of 0.03. When indicated, the media was supplemented with CoCl2 from stock anaerobic solutions of 5 and 50 mM CoCl2 prior to cell inoculation. For some experiments, CoCl2 was added to mid-log phase cultures (∼0.3 OD660) incubated at 30°C. All growth experiments were performed in triplicate cultures. Cultures that did not initiate growth for 7 days were discarded (routinely one out of three replicates grown from low cell densities with 500 and 1,000 μM CoCl2, as shown in Figure 3). Growth curves (OD660) for each of the replicate cultures were analyzed to calculate the length of the lag phase (time before start of exponential growth), generation (doubling) time in exponential phase, and biomass yields (OD660 reached once the cultures entered stationary phase). The latter was expressed as relative growth yield in the treated (with 100–1,000 μM CoCl2) versus the untreated (0 μM CoCl2) cultures.
RNA Extraction and Sequencing (RNAseq)
Cells were grown to mid-log phase (∼0.3 OD660) in the presence or absence of sublethal concentrations of CoCl2 (250 μM) before adding 1 ml of water saturated phenol (5% [v/v] Ambion® water saturated phenol, pH 6.6 in ethanol) to stop transcription. We harvested the cells by centrifugation (3,800 rpm, 8 min, 4°C) and extracted their RNA with the QIAGEN RNeasy kit (QIAGEN) following manufacturer’s recommendations. DNA digestion in the RNA samples used the QIAGEN RNase-free DNase Set and was confirmed by Reverse Transcriptase (RT)-PCR (Verso 1-step kit, Ambion). After assessing RNA integrity in a BioAnalyzer 2100 (Agilent), we selected the samples with the best RNA quality (two biological replicates from each treatment, 0 or 250 μM CoCl2) for RNA sequencing at Michigan State’s Research Technology Support Facility (RTSF, Genomic core). The facility uses validated procedures for rRNA depletion, library preparation, and Illumina Hi-Seq 4000 sequencing. Briefly, rRNA depletion used an Illumina TruSeq Total RNA Library Preparation kit with QIAseq FastSelect – 5S/16S/23S rRNA depletion (QIAGEN). Libraries were quantified using Qubit and Advanced Analytical Fragment Analyzer High Sensitivity DNA NGS assays. The libraries were then pooled in equimolar amounts for multiplexed sequencing and the pool was quantified using the Kapa Biosystems Illumina Library Quantification qPCR kit. Sequencing was in one lane of the Illumina HiSeq 4000 flow cell in 1 × 50 bp single read format and using SBS reagents. Base calling was with the Illumina Real Time Analysis (RTA) v2.7.7 software while demultiplexing and conversion to FastQ format was with Illumina Bcl2fastq v2.19.1 package.
We analyzed the RNAseq data from the CoII-treated and untreated samples with the SPARTA pipeline (Johnson et al., 2016), using FastQC and Trimmomatic tools for quality control and trimming and Bowtie for sequence alignment to the reference genome (GCA_000007985.2 G. sulfurreducens PCA). Gene-level transcript level abundance was calculated with the HTSeq software while the edgeR tool provided the differential expression values. Data filtering used a FDR < 0.05, log CPM > 5, and a log2 FC < −1 (downregulated genes) or >1 (upregulated genes) (Johnson et al., 2016). We used the R software1 with pheatmap function to draw clustered heatmaps of differentially expressed genes. Individual searches in BioCyc 24.0 (Karp et al., 2019) predicted the operon organization of the genes and identified one gene in an RND efflux pump operon (GSU2137) that did not make the maximum FDR value yet met the log CPM and fold-change thresholds. We added this gene to Table 1. We also searched each of the differentially expressed genes in the UniProtKB (UniProt Consortium, 2019) and KEGG databases to assign functional roles. The subcellular localization was predicted using the sequence analysis tools at UniProtKB (SignalP), PSORTb 3.0 (Yu et al., 2010) and CELLO v.2.5 (Yu et al., 2004). Predictions about the domain organization of each protein and identification of metal-binding motifs used the Pfam 33.1 (El-Gebali et al., 2019) tool available at the UniProtKB database. The curated RNAseq data (Supplementary Data 1) has been deposited in the Gene Expression Omnibus (GEO) functional genomics data repository2 under accession number GSE157146. We also identified in the RNAseq data genes differentially regulated in G. sulfurreducens under FeII intoxication, which were calculated as the ratio between Fe excess and homeostatic transcript abundance from microarray data reported elsewhere (Embree et al., 2014). The transcriptomic data comparisons used to generate a heatmap of CoII versus FeII intoxication (Figure 4C) are provided as a Supplementary Data 2.
Transmission Electron Microscopy (TEM) and X-Ray Energy-Dispersive Spectroscopy (EDS)
Mid-log phase cells from untreated or treated (0 or 250 μM CoCl2 supplementation, respectively) cultures were fixed with 2.5% glutaraldehyde before deposition for 5 min on Formvar-coated grids (150 square-mesh Ni, Electron Microscopy Sciences). After three washes with ddH2O (30 s each), we side-blotted the excess liquid and stored the samples at room temperature until ready for TEM examination. The cells were unstained to prevent stain and mineral artifacts during examination with a JEOL 1400 Flash 120kV transmission electron microscope. For EDS elemental analyses, we deposited CoII-stressed cells on a PELCO NetMeshTM copper grid coated with a lacey formvar/carbon support film and examined the samples with a JEM-2200FS ultra-high resolution TEM instrument equipped with an EDS detector. To minimize interference during EDS detection, we collected energy spectra from cells exposed in the holes of the support film and, as controls, from similar areas within the grid that had no cells. We used the two primary X-ray signatures of Co around 0.776 keV (Lα peak) and 6.924 keV (Kα peak) to compare the average intensity of the cell-associated CoII to the cell-free controls. Because the detection limit of the EDS system is 0.001 keV, we averaged the counts detected at 0.76, 0.77, and 0.78 keV to calculate the intensity of the Lα peak and of 6.91, 6.92, and 6.93 keV for the Kα peak. The peak intensities from cells and cell-free areas on the grid were statistically analyzed with the unpaired, unequal variance t-test function of the Excel software.
Colorimetric Detection of CoII
We developed a colorimetric assay for the detection of CoII in culture supernatant fluids based on the color response of the metal after complexation with 2-β-mercaptoethanol (BME). The reducing agent, BME, replaces the water molecules in the cobalt hexaaqua complex (Co[H2O]6)2+, turning the solution brown and permitting the spectrophotometric detection of CoII at 475 nm. Prior to the assay, we grew G. sulfurreducens in DBAF medium at 30°C with or without 250 μM CoCl2 supplementation, collected 200-μl samples periodically, and recovered the culture supernatant fluids after centrifugation at 20,000 rcf for 3 min. To initiate the complexation reaction, we added 10 μl of BME (from a freshly prepared 100 mM aqueous stock) to 190 μl of supernatant sample. After mixing the solution by aspiration with a pipette, we incubated the reactions at 30°C for 20 min to reach their maximum color response and measured the absorbance at 475 nm against CoCl2 standards (0 to 500 μM CoCl2) prepared in DBAF medium. We calculated the amount of CoII removed by the cells in the cultures as the difference between the initial and final concentration of the metal in the culture supernatant fluids.
Biofilm Assay
Biofilm formation in stationary phase cultures was measured with a crystal violet assay (Merritt et al., 2005). Briefly, we poured out the liquid culture (∼ 10 ml) once the cells reached stationary phase, gently rinsed the tubes with ddH2O and added 1 ml of 0.1% crystal violet to stain the biomass of biofilms formed at the bottom of the tube. After 15 min, we poured out the crystal violet solution, rinsed the tubes with ddH2O and left them to dry overnight. We used 1 ml of 30% acetic acid to solubilize the biomass-associated crystal violet for 15 min and measured its absorbance at 550 nm to estimate the biofilm biomass.
Data Availability Statement
The datasets presented in this study can be found in online repositories. The names of the repository/repositories and accession number(s) can be found in the article/Supplementary Material.
Author Contributions
GR conceived and designed the study, with contributions from HD, MT, and KK to parts of the experimental design. GR, HD, and MT performed the genomic analyses. HD and MT performed the growth studies and cobalt assays. MT carried out the RNAseq experiments and GR and HD interpreted the results. HD performed the microscopy analyses, cobalt challenge, and biofilm assays. GR wrote the first draft of the manuscript. All authors contributed to manuscript revision and read and approved the submitted version.
Conflict of Interest
The authors declare that the research was conducted in the absence of any commercial or financial relationships that could be construed as a potential conflict of interest.
Supplementary Material
The Supplementary Material for this article can be found online at: https://www.frontiersin.org/articles/10.3389/fmicb.2020.600463/full#supplementary-material
Footnotes
References
Aklujkar, M., Coppi, M. V., Leang, C., Kim, B. C., Chavan, M. A., Perpetua, L. A., et al. (2013). Proteins involved in electron transfer to Fe(III) and Mn(IV) oxides by Geobacter sulfurreducens and Geobacter uraniireducens. Microbiology 159, 515–535. doi: 10.1099/mic.0.064089-0
Anzaldi, L. L., and Skaar, E. P. (2010). Overcoming the heme paradox: heme toxicity and tolerance in bacterial pathogens. Infect. Immun. 78, 4977–4989. doi: 10.1128/iai.00613-10
Arnesano, F., Banci, L., Benvenuti, M., Bertini, I., Calderone, V., Mangani, S., et al. (2003). The evolutionarily conserved trimeric structure of CutA1 proteins suggests a role in signal transduction. J. Biol. Chem. 278, 45999–46006. doi: 10.1074/jbc.M304398200
Backes, C. A., Mclaren, R. G., Rate, A. W., and Swift, R. S. (1995). Kinetics of cadmium and cobalt desorption from iron and manganese oxides. Soil Sci. Soc. Am. J. 59, 778–785. doi: 10.2136/sssaj1995.03615995005900030021x
Bagai, I., Rensing, C., Blackburn, N. J., and Mcevoy, M. M. (2008). Direct metal transfer between periplasmic proteins identifies a bacterial copper chaperone. Biochemistry 47, 11408–11414. doi: 10.1021/bi801638m
Barras, F., and Fontecave, M. (2011). Cobalt stress in Escherichia coli and Salmonella enterica: molecular bases for toxicity and resistance. Metallomics 3, 1130–1134. doi: 10.1039/c1mt00099c
Borisov, V. B., Gennis, R. B., Hemp, J., and Verkhovsky, M. I. (2011). The cytochrome bd respiratory oxygen reductases. Biochim. Biophys. Acta 1807, 1398–1413. doi: 10.1016/j.bbabio.2011.06.016
Brenner, S., and Horne, R. W. (1959). A negative staining method for high resolution electron microscopy of viruses. Biochim. Biophys. Acta 34, 103–110. doi: 10.1016/0006-3002(59)90237-9
Buccella, D., Lim, M. H., and Morrow, J. R. (2019). Metals in biology: from metallomics to trafficking. Inorg. Chem. 58, 13505–13508. doi: 10.1021/acs.inorgchem.9b02965
Burkhardt, E.-M., Meißner, S., Merten, D., Büchel, G., and Küsel, K. (2009). Heavy metal retention and microbial activities in geochemical barriers formed in glacial sediments subjacent to a former uranium mining leaching heap. Geochemistry 69, 21–34. doi: 10.1016/j.chemer.2008.12.003
Chandrangsu, P., Rensing, C., and Helmann, J. D. (2017). Metal homeostasis and resistance in bacteria. Nat. Rev. Microbiol. 15, 338–350. doi: 10.1038/nrmicro.2017.15
Cologgi, D. L., Lampa-Pastirk, S., Speers, A. M., Kelly, S. D., and Reguera, G. (2011). Extracellular reduction of uranium via Geobacter conductive pili as a protective cellular mechanism. Proc. Natl. Acad. Sci. U.S.A. 108, 15248–15252. doi: 10.1073/pnas.1108616108
Cologgi, D. L., Speers, A. M., Bullard, B. A., Kelly, S. D., and Reguera, G. (2014). Enhanced uranium immobilization and reduction by Geobacter sulfurreducens biofil. Appl. Environ. Microbiol. 80, 6638–6646. doi: 10.1128/AEM.02289-14
Cosert, K. M., and Reguera, G. (2019). Voltammetric study of conductive planar assemblies of Geobacter nanowire pilins unmasks their ability to bind and mineralize divalent cobalt. J. Ind. Microbiol. Biotechnol. 46, 1239–1249. doi: 10.1007/s10295-019-02167-5
Cracan, V., and Banerjee, R. (2013). Cobalt and corrinoid transport and biochemistry. Met. Ions Life Sci. 12, 333–374. doi: 10.1007/978-94-007-5561-1_10
Crowther, D. L., Dillard, J. G., and Murray, J. W. (1983). The mechanisms of Co(II) oxidation on synthetic birnessite. Geochim. Cosmochim. Acta 47, 1399–1403. doi: 10.1016/0016-7037(83)90298-3
Dailey, H. A., Dailey, T. A., Gerdes, S., Jahn, D., Jahn, M., Brian, M. R., et al. (2017). Prokaryotic heme biosynthesis: multiple pathways to a common essential product. Microbiol. Mol. Biol. Rev. 81:e00048-16. doi: 10.1128/MMBR.00048-16
D’Argenio, D. A., Calfee, M. W., Rainey, P. B., and Pesci, E. C. (2002). Autolysis and autoaggregation in Pseudomonas aeruginosa colony morphology mutants. J. Bacteriol. 184, 6481–6489. doi: 10.1128/jb.184.23.6481-6489.2002
De, N., Pirruccello, M., Krasteva, P. V., Bae, N., Raghavan, R. V., and Sondermann, H. (2008). Phosphorylation-independent regulation of the diguanylate cyclase WspR. PLoS Biol. 6:e67. doi: 10.1371/journal.pbio.0060067
El-Gebali, S., Mistry, J., Bateman, A., Eddy, S. R., Luciani, A., Potter, S. C., et al. (2019). The Pfam protein families database in 2019. Nucleic Acids Res. 47, D427–D432. doi: 10.1093/nar/gky995
Embree, M., Qiu, Y., Shieu, W., Nagarajan, H., O’neil, R., Lovley, D., et al. (2014). The iron stimulon and Fur regulon of Geobacter sulfurreducens and their role in energy metabolism. Appl. Environ. Microbiol. 80, 2918–2927. doi: 10.1128/aem.03916-13
Epshtein, V., Kamarthapu, V., Mcgary, K., Svetlov, V., Ueberheide, B., Proshkin, S., et al. (2014). UvrD facilitates DNA repair by pulling RNA polymerase backwards. Nature 505, 372–377. doi: 10.1038/nature12928
Eser, M., Masip, L., Kadokura, H., Georgiou, G., and Beckwith, J. (2009). Disulfide bond formation by exported glutaredoxin indicates glutathione’s presence in the E. coli periplasm. Proc. Natl. Acad. Sci. U.S.A. 106, 1572–1577. doi: 10.1073/pnas.0812596106
Everse, J., and Hsia, N. (1997). The toxicities of native and modified hemoglobins. Free Radic. Biol. Med. 22, 1075–1099. doi: 10.1016/S0891-5849(96)00499-6
Ezraty, B., Gennaris, A., Barras, F., and Collet, J. F. (2017). Oxidative stress, protein damage and repair in bacteria. Nat. Rev. Microbiol. 15, 385–396. doi: 10.1038/nrmicro.2017.26
Feliciano, G. T., Steidl, R. J., and Reguera, G. (2015). Structural and functional insights into the conductive pili of Geobacter sulfurreducens revealed in molecular dynamics simulations. Phys. Chem. Chem. Phys. 17, 22217–22226. doi: 10.1039/C5CP03432A
Fong, S. T., Camakaris, J., and Lee, B. T. (1995). Molecular genetics of a chromosomal locus involved in copper tolerance in Escherichia coli K-12. Mol. Microbiol. 15, 1127–1137. doi: 10.1111/j.1365-2958.1995.tb02286.x
Franklin, N. M., Stauber, J. L., Apte, S. C., and Lim, R. P. (2002). Effect of initial cell density on the bioavailability and toxicity of copper in microalgal bioassays. Environ. Toxicol. Chem. 21, 742–751. doi: 10.1897/1551-50282002021<0742:eoicdo<2.0.co;2
Girvan, H. M., and Munro, A. W. (2013). Heme sensor proteins. J. Biol. Chem. 288, 13194–13203. doi: 10.1074/jbc.R112.422642
Gorelsky, S. I., Basumallick, L., Vura-Weis, J., Sarangi, R., Hodgson, K. O., Hedman, B., et al. (2005). Spectroscopic and DFT investigation of [M{HB(3,5-iPr2pz)3}(SC6F5)] (M = Mn, Fe, Co, Ni, Cu, and Zn) model complexes:? Periodic trends in metal-thiolate bonding. Inorg. Chem. 44, 4947–4960. doi: 10.1021/ic050371m
Grass, G., Otto, M., Fricke, B., Haney, C. J., Rensing, C., Nies, D. H., et al. (2005). FieF (YiiP) from Escherichia coli mediates decreased cellular accumulation of iron and relieves iron stress. Arch. Microbiol. 183, 9–18. doi: 10.1007/s00203-004-0739-4
Haft, D. H., Paulsen, I. T., Ward, N., and Selengut, J. D. (2006). Exopolysaccharide-associated protein sorting in environmental organisms: the PEP-CTERM/EpsH system. Application of a novel phylogenetic profiling heuristic. BMC Biol. 4:29. doi: 10.1186/1741-7007-4-29
Haft, D. H., Payne, S. H., and Selengut, J. D. (2012). Archaeosortases and exosortases are widely distributed systems linking membrane transit with posttranslational modification. J. Bacteriol. 194, 36–48. doi: 10.1128/JB.06026-11
Hatahet, F., Boyd, D., and Beckwith, J. (2014). Disulfide bond formation in prokaryotes: history, diversity and design. Biochim. Biophys. Acta 1844, 1402–1414. doi: 10.1016/j.bbapap.2014.02.014
Hau, H. H., Gilbert, A., Coursolle, D., and Gralnick, J. A. (2008). Mechanism and consequences of anaerobic respiration of cobalt by Shewanella oneidensis strain MR-1. Appl. Environ. Microbiol. 74, 6880–6886. doi: 10.1128/AEM.00840-08
Hazra, A. B., Han, A. W., Mehta, A. P., Mok, K. C., Osadchiy, V., Begley, T. P., et al. (2015). Anaerobic biosynthesis of the lower ligand of vitamin B12. Proc. Natl. Acad. Sci. U.S.A. 112, 10792–10797. doi: 10.1073/pnas.1509132112
Hickman, J. W., Tifrea, D. F., and Harwood, C. S. (2005). A chemosensory system that regulates biofilm formation through modulation of cyclic diguanylate levels. Proc. Natl. Acad. Sci. U.S.A. 102, 14422–14427. doi: 10.1073/pnas.0507170102
Hill, H. A. O., and Sadler, P. J. (2016). Bringing inorganic chemistry to life with inspiration from R. J. P. Williams. J. Biol. Inorg. Chem. 21, 5–12. doi: 10.1007/s00775-016-1333-3
Hiniker, A., Collet, J. F., and Bardwell, J. C. (2005). Copper stress causes an in vivo requirement for the Escherichia coli disulfide isomerase DsbC. J. Biol. Chem. 280, 33785–33791. doi: 10.1074/jbc.M505742200
Hu, H., Lin, H., Zheng, W., Rao, B., Feng, X., Liang, L., et al. (2013). Mercury reduction and cell-surface adsorption by Geobacter sulfurreducens PCA. Environ. Sci. Technol. 47, 10922–10930. doi: 10.1021/es400527m
Huang, J., and Zhang, H. (2020). Redox reactions of iron and manganese oxides in complex systems. Front. Environ. Sci. Eng. 14:76. doi: 10.1007/s11783-020-1255-8
Huergo, L. F., Chandra, G., and Merrick, M. (2013). PII signal transduction proteins: nitrogen regulation and beyond. FEMS Microbiol. Rev. 37, 251–283. doi: 10.1111/j.1574-6976.2012.00351.x
Imlay, J. A. (2008). Cellular defenses against superoxide and hydrogen peroxide. Annu. Rev. Biochem. 77, 755–776. doi: 10.1146/annurev.biochem.77.061606.161055
Johnson, T. A., Stedtfeld, R. D., Wang, Q., Cole, J. R., Hashsham, S. A., Looft, T., et al. (2016). Clusters of antibiotic resistance genes enriched together stay together in swine agriculture. mBio 7:e02214-15. doi: 10.1128/mBio.02214-15
Kamarthapu, V., and Nudler, E. (2015). Rethinking transcription coupled DNA repair. Curr. Opin. Microbiol. 24, 15–20. doi: 10.1016/j.mib.2014.12.005
Kanellis, V. G., and Dos Remedios, C. G. (2018). A review of heavy metal cation binding to deoxyribonucleic acids for the creation of chemical sensors. Biophys. Rev. 10, 1401–1414. doi: 10.1007/s12551-018-0455-y
Karp, P. D., Billington, R., Caspi, R., Fulcher, C. A., Latendresse, M., Kothari, A., et al. (2019). The BioCyc collection of microbial genomes and metabolic pathways. Brief. Bioinform. 20, 1085–1093. doi: 10.1093/bib/bbx085
Kay, J. T., Conklin, M. H., Fuller, C. C., and O’day, P. A. (2001). Processes of nickel and cobalt uptake by a manganese oxide forming sediment in Pinal Creek. Globe Mining District, Arizona. Environ. Sci. Technol. 35, 4719–4725. doi: 10.1021/es010514d
Keon, R. G., Fu, R., and Voordouw, G. (1997). Deletion of two downstream genes alters expression of the hmc operon of Desulfovibrio vulgaris subsp. vulgaris Hildenborough. Arch. Microbiol. 167, 376–383. doi: 10.1007/s002030050458
Kim, E. H., Nies, D. H., Mcevoy, M. M., and Rensing, C. (2011). Switch or funnel: how RND-type transport systems control periplasmic metal homeostasis. J. Bacteriol. 193, 2381–2387. doi: 10.1128/JB.01323-10
Kimber, R. L., Bagshaw, H., Smith, K., Buchanan, D. M., Coker, V. S., Cavet, J. S., et al. (2020). Biomineralization of Cu2S nanoparticles by Geobacter sulfurreducens. Appl. Environ. Microbiol. 86:e00967-20. doi: 10.1128/aem.00967-20
Kolaj-Robin, O., Russell, D., Hayes, K. A., Pembroke, J. T., and Soulimane, T. (2015). Cation diffusion facilitator family: structure and function. FEBS Lett. 589, 1283–1295. doi: 10.1016/j.febslet.2015.04.007
Konovalova, A., and Silhavy, T. J. (2015). Outer membrane lipoprotein biogenesis: lol is not the end. Philos. Trans. R. Soc. Lond. B. Biol. Sci. 370:20150030. doi: 10.1098/rstb.2015.0030
Krupka, K. M., and Serne, R. J. (2002). Geochemical Factors Affecting the Behavior of Antimony, Cobalt, Europium, Technetium, And Uranium In Vadose Sediments. Svanhovd Environmental Centre Report, No. 10379. Washington, DC: Pacific Northwest National Laboratory.
Leonard, S., Gannett, M., Rojanasakul, Y., Schwegler-Berry, D., Castranova, V., Vallyathan, V., et al. (1998). Cobalt-mediated generation of reactive oxygen species and its possible mechanism. J. Inorg. Biochem. 70, 239–244. doi: 10.1016/S0162-0134(98)10022-3
Li, Y. H., Hanna, M. N., Svensater, G., Ellen, R. P., and Cvitkovitch, D. G. (2001). Cell density modulates acid adaptation in Streptococcus mutans: implications for survival in biofilms. J. Bacteriol. 183, 6875–6884. doi: 10.1128/JB.183.23.6875-6884.2001
Lin, H., and Everse, J. (1987). The cytotoxic activity of hematoheme: evidence for two different mechanisms. Anal. Biochem. 161, 323–331. doi: 10.1016/0003-2697(87)90458-1
Ma, X., Yan, Y., Wang, W., Guo, J., and Wang, Y. (2020). Metatranscriptomic analysis of adaptive response of anammox bacteria Candidatus ’Kuenenia stuttgartiensis’ to Zn(II) exposure. Chemosphere 246:125682. doi: 10.1016/j.chemosphere.2019.125682
Majtan, T., Frerman, F. E., and Kraus, J. P. (2011). Effect of cobalt on Escherichia coli metabolism and metalloporphyrin formation. Biometals 24, 335–347. doi: 10.1007/s10534-010-9400-7
Makarova, K. S., and Koonin, E. V. (2015). Annotation and classification of CRISPR-Cas systems. Methods Mol. Biol. 1311, 47–75. doi: 10.1007/978-1-4939-2687-9_4
Merritt, J. H., Kadouri, D. E., and O’toole, G. A. (2005). Growing and analyzing static biofilms. Curr. Protoc. Microbiol Chapter 1:Unit1B.1. doi: 10.1002/9780471729259.mc01b01s00
Moore, S. J., and Warren, M. J. (2012). The anaerobic biosynthesis of vitamin B12. Biochem. Soc. Trans. 40, 581–586. doi: 10.1042/bst20120066
Munkelt, D., Grass, G., and Nies, D. H. (2004). The chromosomally encoded cation diffusion facilitator proteins DmeF and FieF from Wautersia metallidurans CH34 are transporters of broad metal specificity. J. Bacteriol. 186:8036. doi: 10.1128/JB.186.23.8036-8043.2004
Nies, D. H. (1999). Microbial heavy-metal resistance. Appl. Microbiol. Biotechnol. 51, 730–750. doi: 10.1007/s002530051457
Nikaido, H. (2003). Molecular basis of bacterial outer membrane permeability revisited. Microbiol. Mol. Biol. Rev. 67, 593–656. doi: 10.1128/mmbr.67.4.593-656.2003
Nikaido, H., and Takatsuka, Y. (2009). Mechanisms of RND multidrug efflux pumps. Biochim. Biophys. Acta 1794, 769–781. doi: 10.1016/j.bbapap.2008.10.004
Outten, C. E., and O’Halloran, T. V. (2001). Femtomolar sensitivity of metalloregulatory proteins controlling zinc homeostasis. Science 292, 2488–2492. doi: 10.1126/science.1060331
Pak, J. E., Ekende, E. N., Kifle, E. G., O’connell, J. D. III, De Angelis, F., Tessema, M. B., et al. (2013). Structures of intermediate transport states of ZneA, a Zn(II)/proton antiporter. Proc. Natl. Acad. Sci. U.S.A. 110, 18484–18489. doi: 10.1073/pnas.1318705110
Pettigrew, G. W., Echalier, A., and Pauleta, S. R. (2006). Structure and mechanism in the bacterial dihaem cytochrome c peroxidases. J. Inorg. Biochem. 100, 551–567. doi: 10.1016/j.jinorgbio.2005.12.008
Poole, L. B., and Nelson, K. J. (2016). Distribution and features of the six classes of peroxiredoxins. Mol. Cells 39, 53–59. doi: 10.14348/molcells.2016.2330
Qian, X., Reguera, G., Mester, T., and Lovley, D. R. (2007). Evidence that OmcB and OmpB of Geobacter sulfurreducens are outer membrane surface proteins. FEMS Microbiol. Lett. 277, 21–27. doi: 10.1111/j.1574-6968.2007.00915.x
Rajagopalan, S., Teter, S. J., Zwart, P. H., Brennan, R. G., Phillips, K. J., and Kiley, P. J. (2013). Studies of IscR reveal a unique mechanism for metal-dependent regulation of DNA binding specificity. Nat. Struct. Mol. Biol. 20, 740–747. doi: 10.1038/nsmb.2568
Reguera, G. (2018). Harnessing the power of microbial nanowires. Microb. Biotechnol. 11, 979–994. doi: 10.1111/1751-7915.13280
Reguera, G., and Kashefi, K. (2019). The electrifying physiology of Geobacter bacteria, 30 years on. Adv. Microb. Physiol. 74, 1–96. doi: 10.1016/bs.ampbs.2019.02.007
Reguera, G., Mccarthy, K. D., Mehta, T., Nicoll, J. S., Tuominen, M. T., and Lovley, D. R. (2005). Extracellular electron transfer via microbial nanowires. Nature 435, 1098–1101. doi: 10.1038/nature03661
Rehm, B. H. (2010). Bacterial polymers: biosynthesis, modifications and applications. Nat. Rev. Microbiol. 8, 578–592. doi: 10.1038/nrmicro2354
Rodionov, D. A., Hebbeln, P., Gelfand, M. S., and Eitinger, T. (2006). Comparative and functional genomic analysis of prokaryotic nickel and cobalt uptake transporters: evidence for a novel group of ATP-binding cassette transporters. J. Bacteriol. 188, 317–327. doi: 10.1128/JB.188.1.317-327.2006
Rollefson, J. B., Stephen, C. S., Tien, M., and Bond, D. R. (2011). Identification of an extracellular polysaccharide network essential for cytochrome anchoring and biofilm formation in Geobacter sulfurreducens. J. Bacteriol. 193, 1023–1033. doi: 10.1128/JB.01092-10
Sant’Anna, F. H., Trentini, D. B., De Souto Weber, S., Cecagno, R., Da Silva, S. C., and Schrank, I. S. (2009). The PII superfamily revised: a novel group and evolutionary insights. J. Mol. Evol. 68, 322–336. doi: 10.1007/s00239-009-9209-6
Schmidt, T., and Schlegel, H. G. (1989). Nickel and cobalt resistance of various bacteria isolated from soil and highly polluted domestic and industrial wastes. FEMS Microbiol. Lett. 62, 315–328. doi: 10.1016/0378-1097(89)90014-1
Schulze, R. J., and Zuckert, W. R. (2006). Borrelia burgdorferi lipoproteins are secreted to the outer surface by default. Mol. Microbiol. 59, 1473–1484. doi: 10.1111/j.1365-2958.2006.05039.x
Schwartz, C. J., Giel, J. L., Patschkowski, T., Luther, C., Ruzicka, F. J., Beinert, H., et al. (2001). IscR, an Fe-S cluster-containing transcription factor, represses expression of Escherichia coli genes encoding Fe-S cluster assembly proteins. Proc. Natl. Acad. Sci. U.S.A. 98, 14895–14900. doi: 10.1073/pnas.251550898
Seth, E. C., and Taga, M. E. (2014). Nutrient cross-feeding in the microbial world. Front. Microbiol. 5:350. doi: 10.3389/fmicb.2014.00350
Shelton, A. N., Seth, E. C., Mok, K. C., Han, A. W., Jackson, S. N., Haft, D. R., et al. (2019). Uneven distribution of cobamide biosynthesis and dependence in bacteria predicted by comparative genomics. ISME J. 13, 789–804. doi: 10.1038/s41396-018-0304-9
Shi, L., Dong, H., Reguera, G., Beyenal, H., Lu, A., Liu, J., et al. (2016). Extracellular electron transfer mechanisms between microorganisms and minerals. Nat. Rev. Microbiol. 14, 651–662. doi: 10.1038/nrmicro.2016.93
Smith, A. T., Barupala, D., Stemmler, T. L., and Rosenzweig, A. C. (2015). A new metal binding domain involved in cadmium, cobalt and zinc transport. Nat. Chem. Biol. 11, 678–684. doi: 10.1038/nchembio.1863
Speers, A. M., and Reguera, G. (2012). Electron donors supporting growth and electroactivity of Geobacter sulfurreducens anode biofilms. Appl. Environ. Microbiol. 78, 437–444. doi: 10.1128/aem.06782-11
Speers, A. M., Schindler, B. D., Hwang, J., Genc, A., and Reguera, G. (2016). Genetic identification of a PilT motor in Geobacter sulfurreducens reveals a role for pilus retraction in extracellular electron transfer. Front. Microbiol. 7:1578. doi: 10.3389/fmicb.2016.01578
Steidl, R., Lampa-Pastirk, S., and Reguera, G. (2016). Mechanistic stratification in electroactive biofilms of Geobacter sulfurreducens mediated by pilus nanowires. Nat. Commun. 7:12217. doi: 10.1038/ncomms12217
Sundaresan, R., Parameshwaran, H. P., Yogesha, S. D., Keilbarth, M. W., and Rajan, R. (2017). RNA-independent DNA cleavage activities of Cas9 and Cas12a. Cell Rep. 21, 3728–3739. doi: 10.1016/j.celrep.2017.11.100
Thorgersen, M. P., and Downs, D. M. (2007). Cobalt targets multiple metabolic processes in Salmonella enterica. J. Bacteriol. 189, 7774–7781. doi: 10.1128/jb.00962-07
Toledano, M. B., and Huang, B. (2016). Microbial 2-Cys peroxiredoxins: insights into their complex physiological roles. Mol. Cells 39, 31–39. doi: 10.14348/molcells.2016.2326
Trumpower, B. L. (1990). Cytochrome bc1 complexes of microorganisms. Microbiol. Rev. 54, 101–129. doi: 10.1128/mmbr.54.2.101-129.1990
Ueki, T., and Lovley, D. R. (2010). Genome-wide gene regulation of biosynthesis and energy generation by a novel transcriptional repressor in Geobacter species. Nucleic Acids Res. 38, 810–821. doi: 10.1093/nar/gkp1085
UniProt Consortium (2019). UniProt: a worldwide hub of protein knowledge. Nucleic Acids Res. 47, D506–D515. doi: 10.1093/nar/gky1049
Valko, M., Morris, H., and Cronin, M. T. (2005). Metals, toxicity and oxidative stress. Curr. Med. Chem. 12, 1161–1208. doi: 10.2174/0929867053764635
Waldron, K. J., and Robinson, N. J. (2009). How do bacterial cells ensure that metalloproteins get the correct metal? Nat. Rev. Microbiol. 7, 25–35. doi: 10.1038/nrmicro2057
Weber, F.-A., Voegelin, A., Kaegi, R., and Kretzschmar, R. (2009). Contaminant mobilization by metallic copper and metal sulphide colloids in flooded soil. Nature Geosci. 2, 267–271. doi: 10.1038/ngeo476
Westra, E. R., Semenova, E., Datsenko, K. A., Jackson, R. N., Wiedenheft, B., Severinov, K., et al. (2013). Type I-E CRISPR-Cas systems discriminate target from non-target DNA through base pairing-Iindependent PAM recognition. PLoS Genetics 9:e1003742. doi: 10.1371/journal.pgen.1003742
Yan, J., Ritalahti, K. M., Wagner, D. D., and Löffler, F. E. (2012). Unexpected specificity of interspecies cobamide transfer from Geobacter spp. to organohalide-respiring Dehalococcoides mccartyi strains. Appl. Environ. Microbiol. 78, 6630–6636. doi: 10.1128/aem.01535-12
Yu, C. S., Lin, C. J., and Hwang, J. K. (2004). Predicting subcellular localization of proteins for Gram-negative bacteria by support vector machines based on n-peptide compositions. Protein Sci. 13, 1402–1406. doi: 10.1110/ps.03479604
Yu, N. Y., Wagner, J. R., Laird, M. R., Melli, G., Rey, S., Lo, R., et al. (2010). PSORTb 3.0: improved protein subcellular localization prediction with refined localization subcategories and predictive capabilities for all prokaryotes. Bioinformatics 26, 1608–1615. doi: 10.1093/bioinformatics/btq249
Zähringer, F., Lacanna, E., Jenal, U., Schirmer, T., and Boehm, A. (2013). Structure and signaling mechanism of a zinc-sensory diguanylate cyclase. Structure 21, 1149–1157. doi: 10.1016/j.str.2013.04.026
Zhang, Y., Rodionov, D. A., Gelfand, M. S., and Gladyshev, V. N. (2009). Comparative genomic analyses of nickel, cobalt and vitamin B12 utilization. BMC Genomics 10:78. doi: 10.1186/1471-2164-10-78
Keywords: cobalt detoxification, metal homeostasis, stress response, extracellular electron transfer, cytochromes, biomineralization
Citation: Dulay H, Tabares M, Kashefi K and Reguera G (2020) Cobalt Resistance via Detoxification and Mineralization in the Iron-Reducing Bacterium Geobacter sulfurreducens. Front. Microbiol. 11:600463. doi: 10.3389/fmicb.2020.600463
Received: 30 August 2020; Accepted: 03 November 2020;
Published: 26 November 2020.
Edited by:
Jennifer Glass, Georgia Institute of Technology, United StatesReviewed by:
Eric Roden, University of Wisconsin-Madison, United StatesArpita Bose, Washington University in St. Louis, United States
Copyright © 2020 Dulay, Tabares, Kashefi and Reguera. This is an open-access article distributed under the terms of the Creative Commons Attribution License (CC BY). The use, distribution or reproduction in other forums is permitted, provided the original author(s) and the copyright owner(s) are credited and that the original publication in this journal is cited, in accordance with accepted academic practice. No use, distribution or reproduction is permitted which does not comply with these terms.
*Correspondence: Gemma Reguera, cmVndWVyYUBtc3UuZWR1
†These authors have contributed equally to this work