- 1Engineering Research Center for Applied Microbiology of Henan Province, Kaifeng, China
- 2School of Pharmaceutical, Henan University, Kaifeng, China
- 3Institute of Microbial Engineering, Laboratory of Bioresource and Applied Microbiology, School of Life Sciences, Henan University, Kaifeng, China
Bacillus cereus 0-9, a Gram-positive endospore-forming bacterium isolated from healthy wheat roots, has biological control capacity against several soil-borne plant diseases of wheat such as sharp eyespot and take-all. The bacterium can produce various biofilms that differ in their architecture and formation mechanisms, possibly for adapting to different environments. The gapB gene, encoding a glyceraldehyde-3-phosphate dehydrogenase (GAPDH), plays a key role in B. cereus 0-9 biofilm formation. We studied the function of GapB and the mechanism of its involvement in regulating B. cereus 0-9 biofilm formation. GapB has GAPDH activities for both NAD+- and NADP+-dependent dehydrogenases and is a key enzyme in gluconeogenesis. Biofilm yield of the ΔgapB strain decreased by 78.5% compared with that of wild-type B. cereus 0-9 in lysogeny broth supplemented with some mineral salts (LBS), and the ΔgapB::gapB mutants were recovered with gapB gene supplementation. Interestingly, supplementing the LBS medium with 0.1–0.5% glycerol restored the biofilm formation capacity of the ΔgapB mutants. Therefore, GapB regulates biofilm formation relative to its function in gluconeogenesis. To illustrate how GapB is involved in regulating biofilm formation through gluconeogenesis, we carried out further research. The results indicate that the GapB regulated the B. cereus 0-9 biofilm formation independently of the exopolysaccharides and regulatory proteins in the typical SinI/R system, likely owing to the release of extracellular DNA in the matrix. Transcriptome analysis showed that the gapB deletion caused changes in the expression levels of only 18 genes, among which, lrgAB was the most significantly increased by 6.17-fold. We confirmed this hypothesis by counting the dead and living cells in the biofilms and found the number of living cells in the biofilm formed by the ΔgapB strain was nearly 7.5 times than that of wild-type B. cereus 0-9. Therefore, we concluded that the GapB is involved in the extracellular DNA release and biofilm formation by regulating the expression or activities of LrgAB. These results provide a new insight into the regulatory mechanism of bacterial biofilm formation and a new foundation for further studying the stress resistance of B. cereus.
Introduction
Microorganisms perform coordinated activities were previously thought to be restricted to multicellular organisms (Song et al., 2014; Yan et al., 2016; Wan et al., 2018). Bacteria exhibit many social activities and display an array of contact-dependent interaction systems, which have evolved to facilitate direct cell-to-cell communication (Li et al., 2018). Biofilm formation, a typical behavior representing part of the bacterial social repertoire, is a constructive survival strategy that enables bacteria to use a greater variety of nutrients, endure rapid environmental changes, and resist multiple adverse threats (Moons et al., 2009). Bacillus cereus produces various biofilms that differ in their architecture and formation mechanisms, possibly to adapt to various environments (Majed et al., 2016).
The complex architecture of a biofilm is maintained by an extracellular matrix of exopolysaccharides, proteins, and DNA (Candela et al., 2019). Extracellular polysaccharide (EPS), produced by a biofilm community, form the microenvironment for cells in the biofilm (Flemming et al., 2007). The EPS matrix is well hydrated and plays various roles, including adhering the biofilm to surfaces, sequestering substances from the environment, and protecting the bacteria from predators (Vilain et al., 2009). Biofilm formation requires a complex regulatory pathway that coordinates gene expression with external environmental conditions and structural components involved in assembling a protective extracellular matrix (Romero, 2013; Vlamakis et al., 2013). Controlling biofilm formation depends on a molecular switch that comprises two transcription repressors and two anti-repressors (Diethmaier et al., 2014). Central to this regulatory switch is the repressor, SinR (Newman et al., 2013), a DNA-binding protein, that represses transcription of operons involved in producing both an exopolysaccharide (Branda et al., 2001) and the protein component of the matrix. SinR activity is controlled by the relative levels of various antagonists, the foremost of which is SinI (Kearns et al., 2005), which causes de-repression by binding to SinR, so that the SinI-SinR complex can no longer bind to DNA (Lewis et al., 1996, 1998). The EPS of P. aeruginosa biofilms contains DNA; therefore, young biofilms can be dislodged using DNase (Whitchurch et al., 2002). Nucleic acid in the EPS matrix of the biofilms has been termed as extracellular DNA and is required for the structural integrity of the biofilms of various Gram-negative and Gram-positive bacteria, including B. cereus (Vilain et al., 2009). Southern blotting revealed that an extracellular DNA from biofilms was similar to chromosomal DNA. At present, B. cereus is not known to take up DNA via natural methods, nor are there any reported indications of a DNA export system in its genome sequence. Thus, it remains unclear how this extracellular DNA is exported and involved in B. cereus biofilm formation.
GAPDH is a key glycolytic enzyme whose primary function is to phosphorylate glyceraldehyde-3-phosphate (G-3-P) to 1,3-diphosphoglycerate during glucose metabolism (Purves et al., 2010). This enzyme is considered as a housekeeping protein that present in nearly all organisms, where it performs the metabolic functions essential for survival (Giménez et al., 2014). Although GAPDH plays an essential role in energy production, it has more functions than those of a classic glycolytic protein. Several studies have described GAPDH as a multifunctional protein in numerous biological processes in pathogens, probiotics, mammals, and plants (Arutyunova et al., 2013). The multifunctionality of intracellular GAPDH has been widely studied in humans; however, these studies are scarce in bacteria. Recent reports on Streptococcus pyogenes and Escherichia coli evidenced that the bacterial GAPDH may also be involved in intracellular processes such as transcriptional regulation, DNA repair, and quorum sensing signaling (Giménez et al., 2014). Recent research reports that the different concentrations of glucose can change the structural characteristics of Candida albicans biofilms (Figueira et al., 2020). And before that, the effect of glycolysis on bacterial biofilm formation has been rarely reported.
Bacillus cereus 0-9, a gram-positive endospore-forming bacterium, isolated from healthy wheat roots, has biological control capacity against several soil-borne plant diseases of wheat such as sharp eyespot and take-all. Biofilm formation is crucial for this bacterium to use variety of nutrients, endure rapid environmental changes, and resist multiple adverse threats. In this work, we uncovered an important role for GapB, one of GAPDHs, in the formation of biofilms in vitro. We previously sequenced and annotated the whole genome of B. cereus 0-9 and found that three gap genes encode GAPDH: gapA, gapB, and gapN. It has been reported that different GAPDH have different functions in glycometabolism (Purves et al., 2010; Romero et al., 2014). For example, GapA is expressed to perform glycolytic function in the presence of glucose, while gluconeogenesis requires GapB to be expressed in the absence of glucose. But the function of GapN is unclear now. The function of GAPDH in glucose metabolism pathways has been studied deeply. However, to our knowledge, the role of this class of enzymes during biofilm formation in B. cereus 0-9 was unnoticed. Furthermore, we also found wild-type B. cereus 0-9 can form architecturally complex colonies and pellicles under laboratory conditions (Zhang et al., 2020a). Studying the genes related to this biofilm formation will enable better understanding the basic characteristics of biocontrol strains. To our knowledge, the function and roles of GAPDH in glucose metabolism pathway has been studied deeply; however, how GAPDH affects B. cereus biofilm formation is unclear. Hence, we studied the biofilm productions of Δgap strains and investigated the mechanism by which GAPDH regulates B. cereus 0-9 biofilm formation in this study. And our results strongly suggest that the molecular basis for GapB-dependent biofilm formation involves control over the autolysis regulator system LrgAB and most likely eDNA release.
Materials and Methods
Strains, Plasmids, Culture Media, and Growth Conditions
The B. cereus 0-9 properties and culture conditions have been reported previously (Zhang et al., 2020b). Both E. coli 116 (pir+) and E. coli GM2163 (dam-) were incubated in lysogeny broth (LB) at 37°C overnight and used for plasmid propagation and demethylation, respectively. The plasmid, pAD (Takara, Dalian, China), containing ampicillin- and erythromycin-resistance genes, was used to develop a new fusion vector, pAD123-Pgal, which was used to generate deletions in B. cereus open reading frames (Arnaud et al., 2004). The plasmid, pMAD (Miaoling, Wuhan, China), containing ampicillin- and erythromycin-resistance genes and a heat-resistant bgaB gene, was used to construct gene deletion mutants. The 19 T-vector (Takara, Dalian, China), containing ampicillin-resistance genes, efficiently clones DNA fragments and was used to generate T-vector cloning. All tested strains and plasmids used in this experiment are listed in Table 1.
Biofilm formed by the tested strains was cultured in LBS and King broth medium supplemented with glucose (KBMS). In LBS medium, per 1,000 ml culture containing 10 g pryptone, 5.0 g yeast extract, 5.0 g NaCl, 1.0 g sodium citrate, 2.0 g (NH3)2SO4, 0.2 g MgSO4·H2O 14 g K2HPO4, 6.0 g KH2PO4, and 1.0 g glucose. In KBMS medium, per 1,000 ml culture containing 10 g pryptone, 10 g acid hydrolyzed casein, 1.5 g K2HPO4, 10 g glycerinum, 1.5 g MgSO4·H2O, and 10 g glucose, pH 7.4. Use of different carbon sources by the tested strains was determined in minimum energy require source (M-eps) medium, with 1,000-ml cultures containing 7.0 g K2HPO4, 3.0 g KH2PO4, 0.1 g MgSO4, 0.01 g CaCl2, 0.001 g FeSO4, 0.1 g NaCl, and 5.0 g acid hydrolyzed casein. Nutrient agar (NA) medium was used to observe the colony morphology of the tested strains with 1,000 ml of distilled water containing 5.0 g beef extract, 10 g peptone, 5.0 g NaCl, and 15 g agar powder.
Construction of a gapB Gene Complementation Vector
To generate the gene complementation strains, pAD123-Pgal, a genetic reverse complementary system, was constructed using the pAD plasmid from the Bacillus Genetic Stock Center (Accession: ECE165; Columbus, OH, Unites States). Supplementary Figure S1 shows the structural map of the pAD123-Pgal vector. A DNA fragment containing the B. cereus 0-9 gapB-coding region and its native promoter was cloned into pAD123-Pgal at the MluI and XhoI sites to construct the recombinant plasmid, pAD123-Pgal-gapB pro, which was used to construct the complementation strains by electric shock conversion. Another vector, pMADchi, was constructed using the plasmid pMAD, with a combination of DNA fragments from upstream and downstream of the chi gene from B. cereus 0-9 inserted at the EcoRI and XhoI sites, and several additional restriction sites were inserted. Supplementary Figure S2 shows the structural map of the pMADchi plasmids. DNA fragment containing gapB gene from B. cereus 0-9 was cloned into pMADchi at the BamHI and XhoI sites, to construct the recombinant plasmid, pMADchi-gapB orf, which were used to construct the complementary strain by allelic exchange. The DNA fragment containing sinI or sinR gene from B. cereus 0-9 was cloned into pMADchi at the XhoI and EcoRI sites, or EcoRI and BamHI sites, respectively, to construct recombinant plasmid pMADchi-sinI and pMADchi-sinR.
Construction of the Gene Mutant Strains and Complementation Experiments
Whole-genome sequencing results (GenBank: CP042874.1) and subsequent gene function annotation showed that the B. cereus 0-9 genome encodes three Gap proteins: GapA (Protein ID: QEF19539.1), GapB (Protein ID: QEF19056.1), and GapN (Protein ID: QEF15645.1). To investigate the functions of Gap in B. cereus 0-9 biofilm formation, we constructed the gap-knockout mutants, ∆gapA, ∆gapB, and ∆gapN by a previously described allelic exchange method (Xu et al., 2014; Zhang et al., 2020b). In detail, two fragments suitable for allelic exchange were created by cloning two BamHI-XhoI DNA fragments containing locus-specific flanking regions into the BamHI site of pMAD. These fragments were created via PCR using the primers in Supplementary Table S1. Ligation of the two fragments led to precise deletion of the respective open reading frame from the start to the stop codons and to generation of an XhoI site at the locus site. The resulting constructs were also used to create ∆sinI, ∆sinR, ∆gapB∆sinI, ∆gapB∆sinR, ∆glk, ∆glk∆gapB, ∆lrgAB, and ∆lrgAB∆gapB mutants of B. cereus 0-9.
The recombinant plasmid, pAD123-Pgal-gapB pro was transformed into the competent cells of ∆gapB mutants to construct the reverse complimentary strain, ∆gapB::gapBpro . The other recombinant vectors, pMADchi-gapB orf, pMADchi-sinR, and pMADchi-sinI were transformed into the competent cells of the B. cereus mutants by electroporation (1700 V, Eporator, Eppendorf) to construct the complemented mutants: ∆gapB::gapB, ∆gapB∆sinR::gapB, ∆gapB∆sinI::gapB, ∆gapB∆sinR::sinR, and ∆gapB∆sinI::sinI. The transformants were cultured on LB plates supplemented with chloramphenicol (20 μg/ml) and incubated at 30°C overnight. The correct complement strain was selected and identified via PCR using the primer pairs, 0-9-pMAD-chi-s/0-9-pMAD-chi-a (Supplementary Table S1). All mutant strains were stored at −80°C and cultivated aerobically in LB broth at 30°C throughout this study.
Determination of Biofilm Formation
Solid surface-associated biofilm formation was estimated using a crystal violet staining method with some modifications (Zhang et al., 2020a). A single colony of B. cereus 0-9 and its transformants was inoculated into 5 ml of LB medium and incubated at 30°C overnight. Three milliliter of LBS medium inoculated by 30 μl of the overnight culture was added into the glass tubes with a diameter of 0.7 cm. And then, the tubes were incubated statically at 30°C for 3 days. The cultures were carefully removed from the tubes letting the pellicle slowly adhere to the tube wall. After gently wash, the pellicle and tube wall twice with deionized water. The remaining cells and matrices in each tube were stained with 3.5 ml of 0.1% (w/v) crystal violet solution for 20 min at 25°C. After washing three times with distilled water, the crystal violet attached to the biofilm was solubilized in 3.5 ml 10% (w/v) sodium dodecyl sulfate. Next, 200 μl of the solution was quantified by measuring the absorbance at 570 nm (Bio Tek, Winooski, VT, United States). The experiment was repeated five times per strain.
Construction Expression Vectors and Inducible Expression of GapB
The gapB gene was amplified using a wild-type B. cereus 0-9 template and subcloned into the expression vector, pET28a, before transformation into E. coli BL21 (DE3). Recombinant GapB was expressed in E. coli BL21 (DE3) strains as previously reported (Zhang et al., 2020a). Briefly, E. coli BL21 (DE3) harboring pET28a-gapB, growing exponentially in a culture, was induced using 100 μM isopropyl-β-D-thiogalactopyranoside for 4 h at 25°C. Using E. coli BL21 (DE3) harboring pET28a vector as control. The cells were harvested by centrifugation and lysed by sonication. After lysis, the crude extracts were centrifuged at 10,000 rpm for at least 30 min. GapB-His6 was purified by nickel-immobilized metal affinity chromatography using a His-Trap column (GE Healthcare). The bound protein molecules were eluted with imidazole before pooling, concentrating, and loading onto a Superdex 200 gel filtration column. The GapB-His6 purity was estimated at >90% via SDS-PAGE.
Determination of GADPH Dehydrogenase Activity
Enzymatic activity assays for GapB were performed as the previous method of Purves et al. (2010) with some modifications. In detail, 5 μg of purified GapB was added to 1 ml of buffer (pH 7.5) containing 50 mM Na2HPO4, 5 mM EDTA, 20 mM glyceraldehyde-3-phosphate (G-3-P), and either 10 mM NAD+ or 2 mM NADP+. The sample mixtures were incubated in a 37°C water bath for 30 min. The GapB protein after thermal inactivation was used as the negative control. NAD(P)H production was measured at 340 nm, and an average reading from three wells was recorded for each sample. Each assay was repeated three times on separate days; the results were averaged and are presented alongside the standard error of the data. Values of p values were derived using Student’s t-test.
Growth Curve Measurement
The growth curves of the B. cereus strains in LBS, KBMS, and M-eps media were determined as follows. A single colony was inoculated into 5 ml of LB medium and grown overnight at 37°C with shaking. Next, 1 ml of overnight culture medium was centrifuged to collect the bacteria, washed three times with sterile water, and then resuspended with different volumes of sterile water to make the OD600 value of the tested strains consistent to be 0.6. The suspensions were inoculated into the tested medium at 1:100. The inoculated mediums were grown in the microplates equipped by Bioscreen C (Oy Growth Curves Ab Ltd., Finland) at 37°C statically with oscillating horizontally at 30 min intervals. OD600 value of each micropore was measured every 30 min. The growth curves of the tested strains were recorded automatically.
Complex Colony Formation Assays
To analyze the colony architecture, we used the method previously reported by Diethmaier et al. (2014) with slight modifications. Bacillus cereus strains were precultured in LB medium to an optical density of 0.6–0.8 at 600 nm (OD600), and then 1.0 ml of the culture was pelleted and resuspended in 100 μl of sterile supernatant. Approximately 2 μl of this cell suspension was then spotted onto NA plates and incubated at 30°C for 2–3 days. The colonies were photographed using a Digital Single Lens Reflex (Canon EOS 6D, Beijing, China).
Real-Time Quantitative Reverse Transcription-PCR
Real-time quantitative PCR (qRT-PCR) was performed by using a Cycler instrument (BioRad, Hercules, California, USA) and a Fast Quant RT Kit (Tianjin, China). Bacillus cereus 0-9 and its mutants were grown in LBS medium to an OD600 of 0.8–1.0 and then harvested. The total RNA was extracted as described previously (Gao et al., 2017). The cDNAs were synthesized using the Fast Quant RT Kitper, according to the manufacturer’s instructions. The GoTaq qPCR Master Mixkit (Tianjin, China) was used for the PCR, according to the manufacturer’s recommended protocol using the primers in Supplementary Table S1. The 16s rRNA was used as endogenous controls and the relative expression of test genes were calculated by the −ΔΔCT value.
Determination of Extracellular Polysaccharide
After culture for 3 days, 1.0 g biofilms formed by test strains were picked for the determination of EPS content. The biofilms were mixed with 200 μl sterile water and shaken thoroughly to disperse all the aggregates, and then the EPS content in the supernatant of heavy suspension was determined according to previous report (Asadi et al., 2020) with some modifications. For detail, equal volume of 1 M NaOH was added to the supernatant and incubated at 60°C for 1 h. The mixture was centrifuged (10 min and12,000 rpm), and the polysaccharides content in the supernatant was determined by the phenol-sulfuric acid method. Glucose was used as the standard (Masuko et al., 2005). The absorbance was measured using a plate reader at 490 nm (Bio Tek, Winooski, VT, United States), and the averages of the polysaccharide content, measured in triplicate trials, were reported. The supernatant without adding NaOH was used as blank control.
Determination of Extracellular DNA Content in the Biofilms
Extracellular DNA content in the biofilms was determined using Qubit® dsDNA BR Assay Kits (Thermal Fisher, Shanghai, China). Next, 0.2 g (wet weight) of biofilm from the tested strains was placed into an Eppendorf tube and 200 μl of sterile water was added, and it then oscillated sufficiently to suspend the bacteria cells. The cells were removed by centrifugation at 8,000xg for 5 min. The kits included concentrated assay reagent, dilution buffer, and prediluted DNA standards. The reagent was diluted using the provided buffer, then 20 μl of the liquid supernatant was added and incubated for 2 min, and the concentration of the double-stranded DNA was read using the Qubit® 4 Fluorometer according to the manufacturer’s instructions.
Transcriptome Analysis
ΔgapB and B. cereus 0-9 strains were cultured at 30°C, 220 rpm, in LBS medium. When the tested strains grew to the stable period (Culturing for 24 h), the bacterial cells were harvested. The bacteria were quickly frozen in liquid nitrogen, stored in dry ice, and sent to the GENEWIZ Company (Tianjin, China) for chain-specific prokaryotic transcriptome sequencing. The experimental process included RNA extraction, ribosomal RNA removal, library construction and detection, sequencing cluster generation, and on-machine sequencing. Three libraries were prepared by the cDNA from wild-type B. cereus 0-9 and ΔgapB strain, respectively. Illumina HiSeq sequencing was performed after the qualified libraries were mixed according to the target data volume. Software Bcl2fastq (V2.17.1.14) was used for image base recognition of the original image data of sequencing results. To obtain the original sequencing data (Pass Filter Data), preliminary quality analysis was used as follows. During sequencing, based on the distribution of mass fraction of base position of sample, select analysis results with a quality score value greater than 30 for each Read. For another, Illumina has built-in software based on the mass of the first 25 bases per sequence fragment, then, decide whether the read is retained or discarded. Read count data obtained from gene expression level analysis were used for the input data of gene differential expression. DESeq2 (V1.6.3) of Bioconductor software was used to analyze the genetic differences in this study.
Live Cell Counts in the Biofilms
Approximately 0.2 g (wet weight) of the biofilms from the tested strains were placed into Eppendorf tubes and suspended in 100 μl of sterile water. After thoroughly blowing and dispersing, the bacteria were collected via centrifugation, washed 2–3 times with deionized water, and then suspended with 100 μl deionized water. The number of living cells in the suspension was determined using the viable count method [colony-forming units (CFU)/ml]. The living and dead cells in the suspension were stained with the LIVE/DEAD BacLight Bacterial Viability Kit (Invitrogen, Carlsbad, California, USA) per the manufacturer’s instructions, then 5 μl of stained suspension was dropped on a glass slide, and the ratio of living/dead cells was counted under a fluorescence microscope (Nicon, Tokyo, Japan). Images of the living cells (green fluorescence) and dead cells (red fluorescence) were recorded and combined using the assay software.
Statistical Evaluations
The vegetative growth curves of the wild-type and mutant strains were generated by plotting the average outcomes (OD600) of three experiments per strain. The differences in biofilm formation were analyzed via one-way analysis of variance followed by Tukey’s pairwise post hoc comparisons.
Results
GapB Is Involved in Regulating Bacillus cereus 0-9 Biofilm Formation
Bacillus cereus 0-9 generated biofilm while culturing in the post-stabilization period in both LBS and KBMS media. The overall impression of biofilms formed by B. cereus 0-9 and its Δgap mutants were visually record and shown in Figure 1. Biofilm formation of the SL1001 (ΔgapA) and SL1003 (ΔgapN) mutants could form a normal biofilm structure of B. cereus 0-9, but that of SL1002 (ΔgapB) mutant was noticeably different in LBS medium. The biofilm formation can take on two forms simultaneously, in the air-liquid (pellicle) and solid-liquid interface, both of which are considered to be crucial biofilms of B. cereus 0-9. ΔgapB mutant only form a small amount of biofilm at the solid-liquid interface, instead of forming pellicle in the air-liquid interface (Figure 1A). However, the growth curve of the ΔgapB mutant did not significantly differ from that of the wild-type (Figure 2). This suggests that the difference in biofilm formation is not due to the difference in growth capacity. The yields of biofilms were quantitatively determined by crystal violet staining, and the results show that ΔgapB mutant biofilm yield decreased by 78.5% compared with that of wild-type B. cereus 0-9 (Figure 1C). To clarify the relationship between the gapB gene and the biofilm phenotype, we constructed two complementary strains of SL7120 (∆gapB/gapBpro ) and SL7110 (∆gapB::gapBorf ), which supplemented with gapB via pAD123-Pgal-gapB pro and pMADchi-gapB orf, respectively, consequently recovering all biofilm yields of the complementary mutants (Figure 1B). Thus, gapB may be closely related to B. cereus 0-9 biofilm formation.
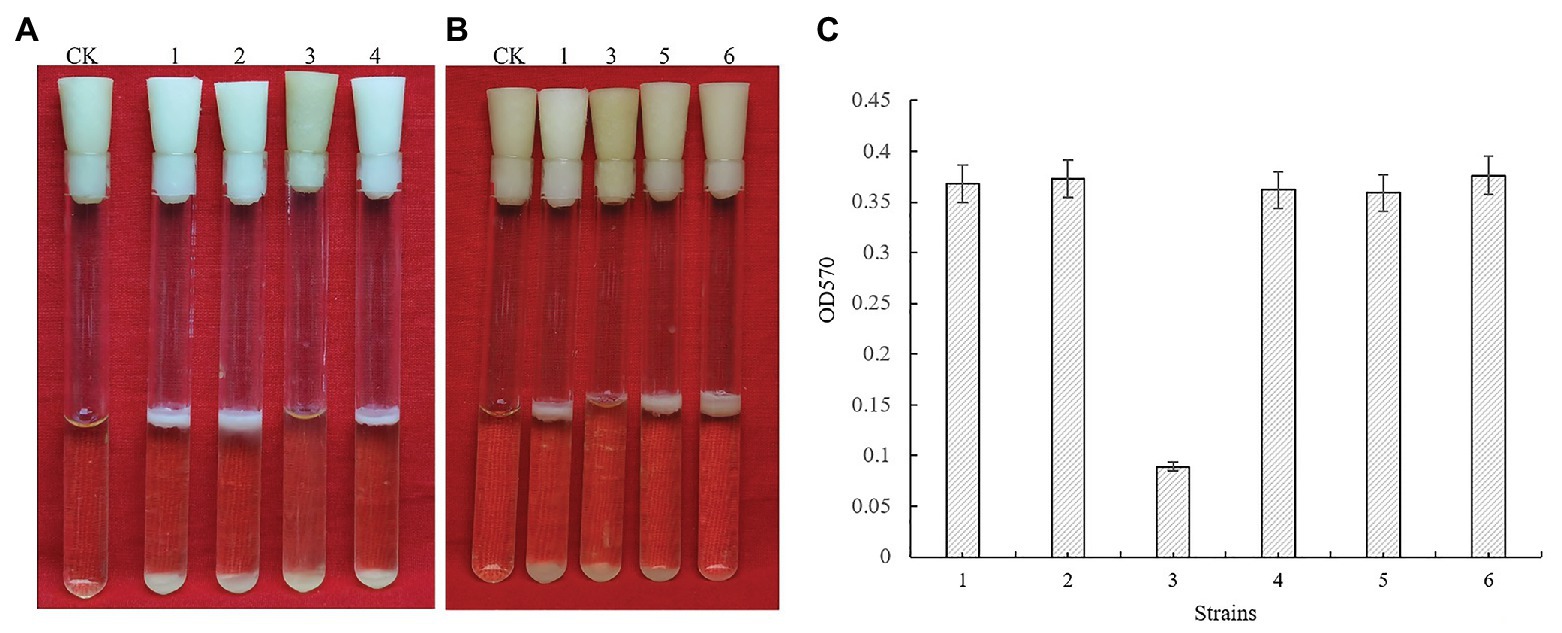
Figure 1. GapB is required for biofilm formation in Bacillus cereus 0-9 when it was grown under static conditions in LBS medium. (A) The biofilms formed by the three gap genes. (B) The biofilms formed by ΔgapB and its complementary mutants. (C) The biofilm production of test strains when they were cultured in LBS medium. Using uninoculated blank medium as control (CK), biofilm formation of the wild-type B. cereus 0-9 (1), ΔgapA (2), ΔgapB (3), ΔgapN (4), ∆gapB/gapB pro (5), and ΔgapB::gapB orf (6) strains were imaged after 3 days of growth. Pictures are representative of five experiments.
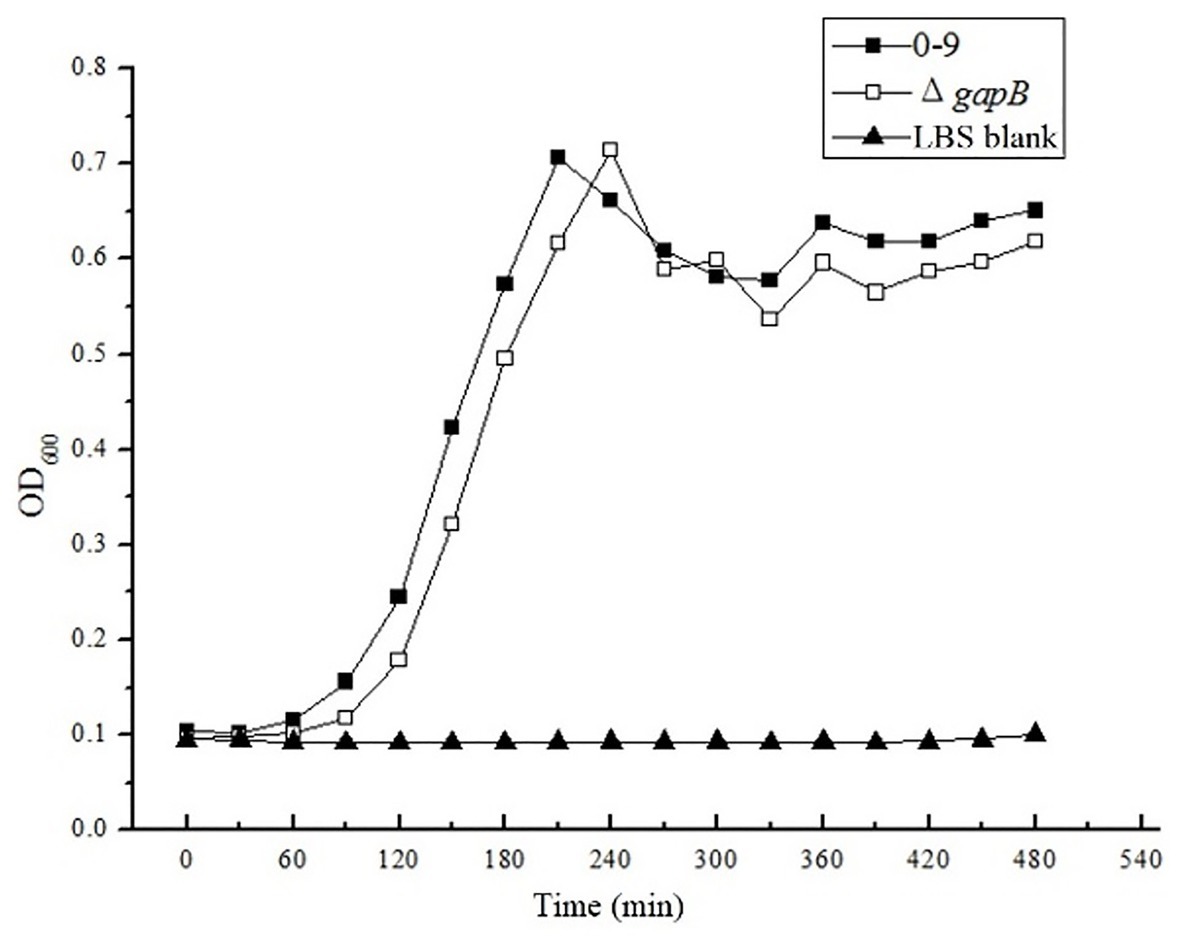
Figure 2. The OD600 value were measured in LBS medium. There is no significantly difference between the growth curve of Bacillus cereus 0-9 and ΔgapB.
Inducible Expression of GapB and Its Enzymatic Activities
GapB can be expressed in E. coli BL21 (DE3). The electrophoresis results of the protein expression profile of E. coli BL21 harboring the pET28a-gapB plasmid was shown in Supplementary Figure S3. GapB weighed 42 kDa, which is the same as the gene annotation. There is no band in the protein expression profile of E. coli BL21 harboring the pET28a plasmid, which indicates that the pET28a plasmid has no effect on the GapB expression. GapB was then purified using a cadmium column, and the high-purity protein was used to subsequently determine enzyme activities. Figure 3 shows the Gap protein enzyme activity in the presence of either NAD+ or NADP+ as cofactors. The B. cereus 0-9 Gap proteins had strong dehydrogenase activities, regardless of whether NAD+ or NADP+ was the cofactor, but the NAD+-dependent activity was higher.
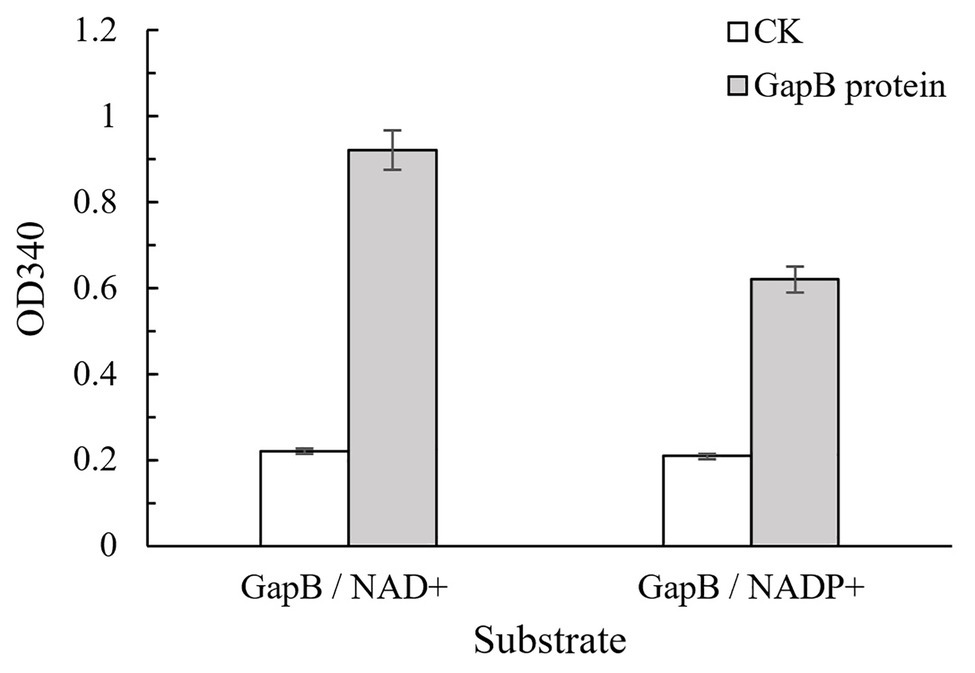
Figure 3. GapB protein has enzyme activities with either NAD+ or NADP+ as a cofactor. GapB protein after thermal inactivation was used as the negative control (CK). The NAD(P)H production was measured at 340 nm, and an average reading from three wells was recorded for each sample.
Different Carbon Sources Affect the Growth Curves of B. cereus 0-9 and ΔgapB
To determine the function of GapB in secondary carbon metabolism, we tested the ability of the ΔgapB mutant to use a range of secondary carbon sources. The secondary carbon sources included glucose, the source of glycolysis; pyruvic acid, a key intermediate in the citric acid cycle; and glycerol, which can enter both the glycolytic and gluconeogenic pathways through conversion to glucose-3-phosphatase. Figure 4 shows the growth curve of ΔgapB and its complementary mutants in M-eps medium with different carbon sources. Our results revealed where various carbon sources enter either glycolysis or gluconeogenesis. Wild-type B. cereus 0-9 grows well in M-eps medium, but ΔgapB does not. When the gapB gene was complemented, the growth curve of the ΔgapB::gapB mutants completely recovered to that of wild strain (Figure 4A). Adding glucose acid to the M-eps medium also recovered the ΔgapB growth curve, only with slightly weaker in the stable period (Figure 4B). Adding glycerol to the medium completely recovered the growth ability of the ΔgapB strain (Figure 4C). Supplementing pyruvic acid in the M-eps medium partly recovered the ΔgapB proliferation, and the growth ability was significantly lower than that of wild-type B. cereus 0-9 (Figure 4D). Thus, we concluded that the role of GapB in the glycolysis pathway may play a key role in gluconeogenesis.
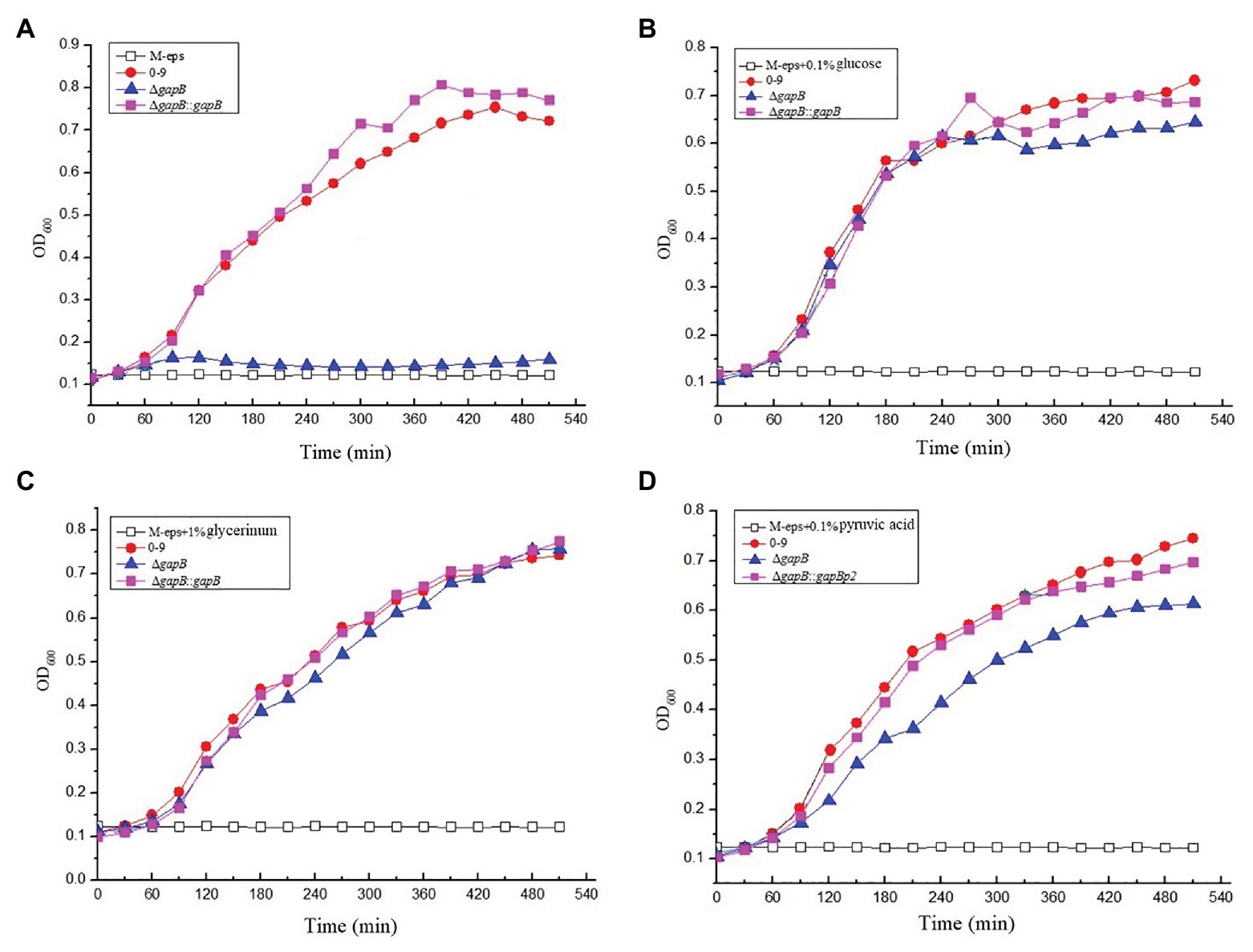
Figure 4. The growth curve of ΔgapB and its complementary mutants were measured in M-eps medium with deferent carbon sources of (A) M-eps, (B) M-eps with 1% glucose, (C) M-eps with 1% glycerinum, and (D) M-eps with 1% pyruvic acid. The average of three parallel measurements was taken for each reading.
Expression Level of pgm in the ΔgapB Mutant Determined via qRT-PCR
The pgm gene encodes allosteric glucose-6-phosphatase, which catalyzes glucose-6-phosphate to glucose-1-phosphate, an irreversible catalytic reaction in the gluconeogenic pathway (Goto et al., 2016). To confirm the function of GapB in B. cereus 0-9 gluconeogenesis, we measured the transcription level of pgm, which is related to the allostery of phosphorylated glucose in gluconeogenesis, via qRT-PCR. When cultured for 6 h in LBS medium, the transcription level of pgm in the ΔgapB mutant did not significantly differ (p > 0.5) from that of wild-type B. cereus 0-9. However, incubating ΔgapB to a stable level for 12 h, downregulated its pgm transcription by 32-fold, indicating that deleting gapB blocked the gluconeogenic pathway in B. cereus 0-9 and inhibited the Pgm protein activity, thus affecting glucose-1-phosphate synthesis. These results demonstrated that the GapB is a key factor controlling gluconeogenesis in B. cereus 0-9.
Different Carbon Sources Affect the ΔgapB Biofilm Yield
Figure 5 shows the biofilm formation of ΔgapB and its complementary mutants in LBS medium with different carbon sources. Adding glucose or pyruvate to LBS did not recover the ΔgapB biofilm formation (Figures 5A,C), but the growth curve of ΔgapB in LBS did not significantly differ from B. cereus 0-9 (p < 0.05; Figure 2). Glycerinum supplementation recovered the ΔgapB biofilm formation to the same level as that of wild-type B. cereus 0-9 for concentrations ranging from 0.1–0.5% (Figure 5B). Thus, we concluded that the B. cereus 0-9 biofilm formation requires sufficient glucose-1-phosphatase, and gapB deletion blocking gluconeogenesis, which leads to abnormal biofilm formation. Supplementing glycerol in the medium enabled gluconeogenesis via the glycerol branch, which circumvented the limitation of the gapB deletion. This is consistent with the findings for S. aureus reported by Purves et al. (2010).
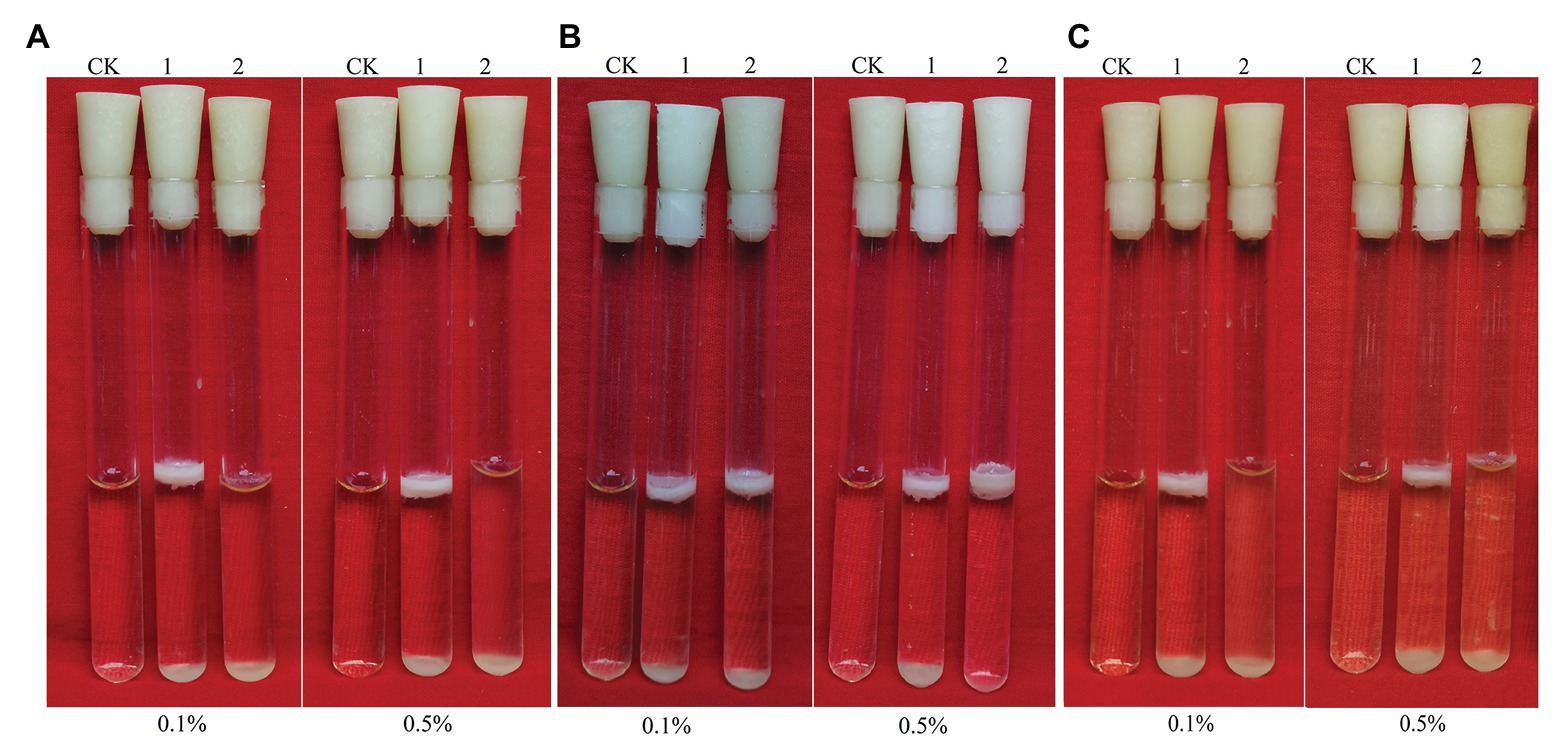
Figure 5. Biofilms of Bacillus cereus 0-9 (1) and ΔgapB (2) formed in LBS medium with different carbon sources of (A) glucose, (B) glycerinum, and (C) pyruvic acid, using uninoculated blank medium as control (CK). Biofilms were imaged after 3 days of growth. Pictures are representative of five experiments.
Effects of glk and gapB Gene Deletion on B. cereus 0-9 Biofilm Formation
The glk gene encodes glycerol kinase, which catalyzes the phosphorylation of glycerin to glycerin-3-phosphate, which is then dehydrogenated to dihydroxyacetone phosphate and converted to glyceraldehyde triphosphate to participate in glycometabolism or gluconeogenesis (Park et al., 2000). Thus, deleting glk would block the glycerol branch in the gluconeogenic pathway. To determine the function of GapB in B. cereus 0-9, we studied the biofilm formation and colony morphology of SL1004 (Δglk), ΔgapB, SL2003 (ΔglkΔgapB), and wild-type B. cereus 0-9. Our experimental results show that only glk gene deletion alone would not affect the B. cereus 0-9 biofilm formation. When gapB gene was deleted, the biofilm formation of B. cereus 0-9 was repressed. Supplementing glycerinum in the medium completely recovered the biofilm formation of ΔgapB, but the double gene mutant, ΔglkΔgapB, still could not restore the biofilm formation abilities (Figure 6). When the gluconeogenesis was blocked by deletion of gapB gene, glycerol was transferred into the gluconeogenic pathway via dihydroxyacetone phosphate to supplement the function of GapB. However, the glk gene deletion can block glycerin into the glycometabolism, so the double knockout strain of ΔglkΔgapB cannot restore the gluconeogenesis, even in the presence of glycerol. Therefore, these results indicated that the gluconeogenesis plays an important role in the regulation of the biofilm formation of B. cereus 0-9, and the GapB is a key enzyme in the control of glucogenesis, so it is crucial for the formation of biofilms. Further, we determined the colony morphology on solid NA plates with or without 1% glycerinum (Figure 7). The Δglk colony morphology did not noticeably differ from the that of wild-type B. cereus 0-9, regardless of whether the plate contained 1% glycerinum. After knocking out gapB, the colonies became smaller, their folds became less uniform, and most of the surrounding areas became smooth and flat (Figure 7A). Supplementing 1% glycerinum in the plates restored the ΔgapB colony morphology, but the glk and gapB double mutants did not recover the colony morphology on the same plates (Figure 7B). This is consistent with the results for the biofilm formed by these tested strains in liquid LBS medium. Hence, we considered that the GapB is also critical to B. cereus 0-9 colony morphology and may be an important factor in biofilm formation on the surfaces of solid substrates. Therefore, we concluded that the GapB influenced B. cereus 0-9 biofilm formation by regulating gluconeogenesis.
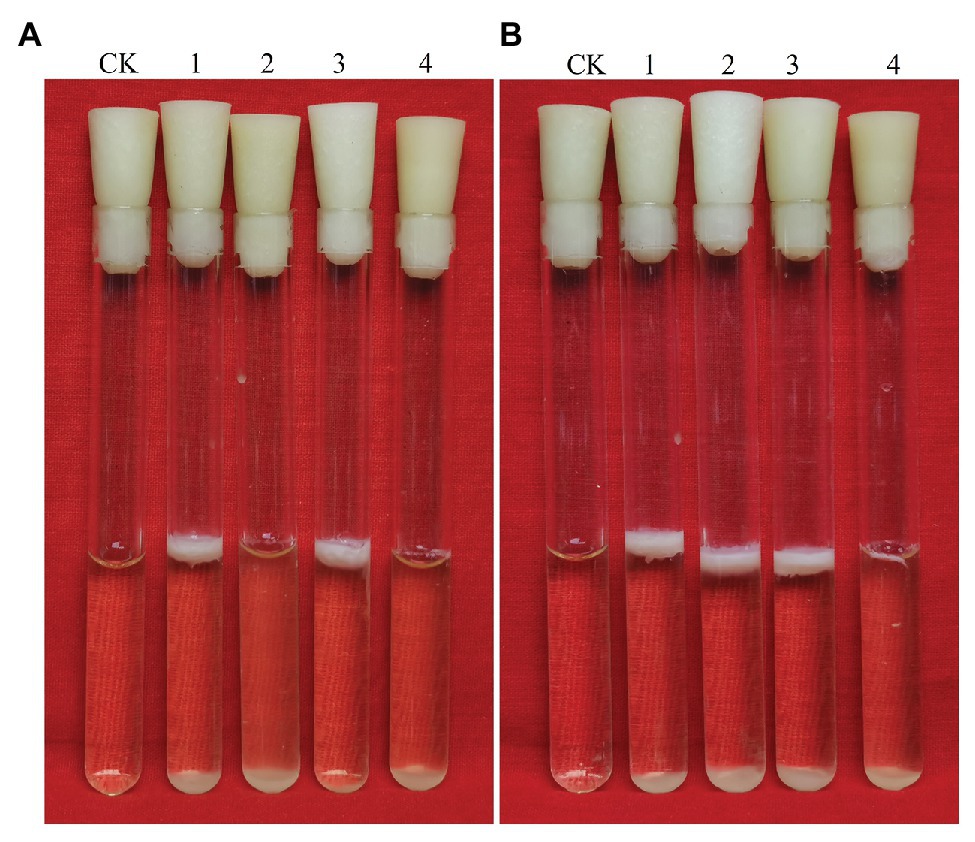
Figure 6. Biofilms of Bacillus cereus 0-9 (1), ΔgapB (2), Δglk (3), and ΔglkΔgapB (4) mutants in LBS medium with different carbon sources of (A) glucose and (B) glycerinum were imaged after 3 days of growth, using uninoculated blank medium as control (CK). Pictures are representative of five experiments.
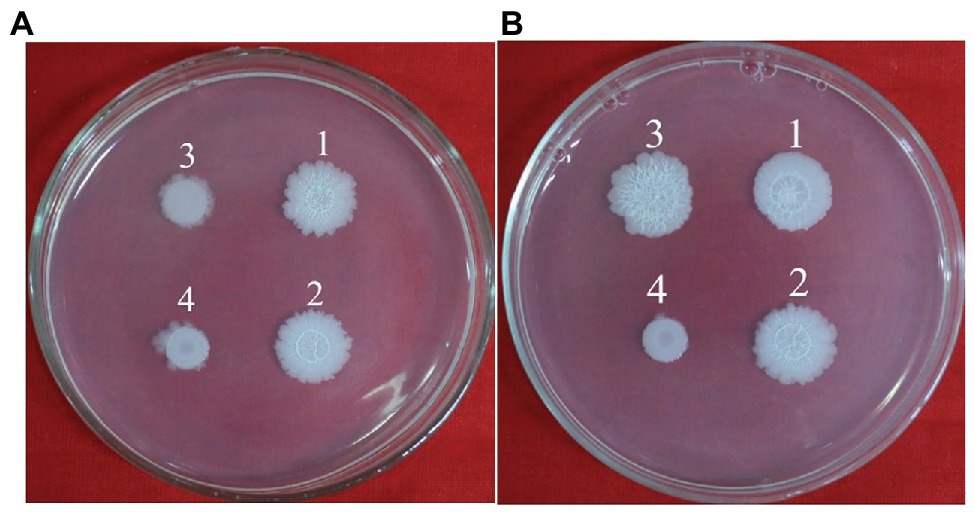
Figure 7. Colony morphology of B. cereus 0-9 (1), Δglk (2), ΔgapB (3) and ΔglkΔgapB (4) strains were determined in NA medium with 1% glycerinum (B), using NA medium without glycerinum as control (A). Pictures are representative of three experiments.
The SinI/R System Is Involved in Bacillus cereus 0-9 Biofilm Formation
To determine whether the SinI/R system is involved in B. cereus 0-9 biofilm formation, we constructed FPU1051I (ΔsinI) and SL1052R (ΔsinR) mutants and measured their biofilm yields (Figure 8). The biofilm formation ability of ΔsinI was decreased by 66.30% compared with that of wild-type B. cereus 0-9. Conversely, the biofilm formation ability of the ΔsinR mutants was increased by 63.32%; therefore, the SinI/R system also exists in B. cereus 0-9. The repressor SinR is central to the regulatory switch in biofilm formation in B. subtilis (Diethmaier et al., 2014), whose function and mechanism in regulating biofilm formation has been clearly revealed (Chu et al., 2006; Newman et al., 2013). To examine the mechanism of the SinI/R system in regulating B. cereus 0-9 biofilm formation, we measured the transcription levels of several biofilm formation-related genes via qRT-PCR. Culturing ΔsinR for 4 h upregulated the transcription levels of tasA, calY, and sipW by 147.03-, 630.35-, and 445.72-fold, respectively, compared with that of wild-type B. cereus 0-9 (Supplementary Table S2). These results indicated that the mechanism by which the SinI/R system regulates biofilm formation in B. cereus 0-9 may the same as that of B. subtilis.
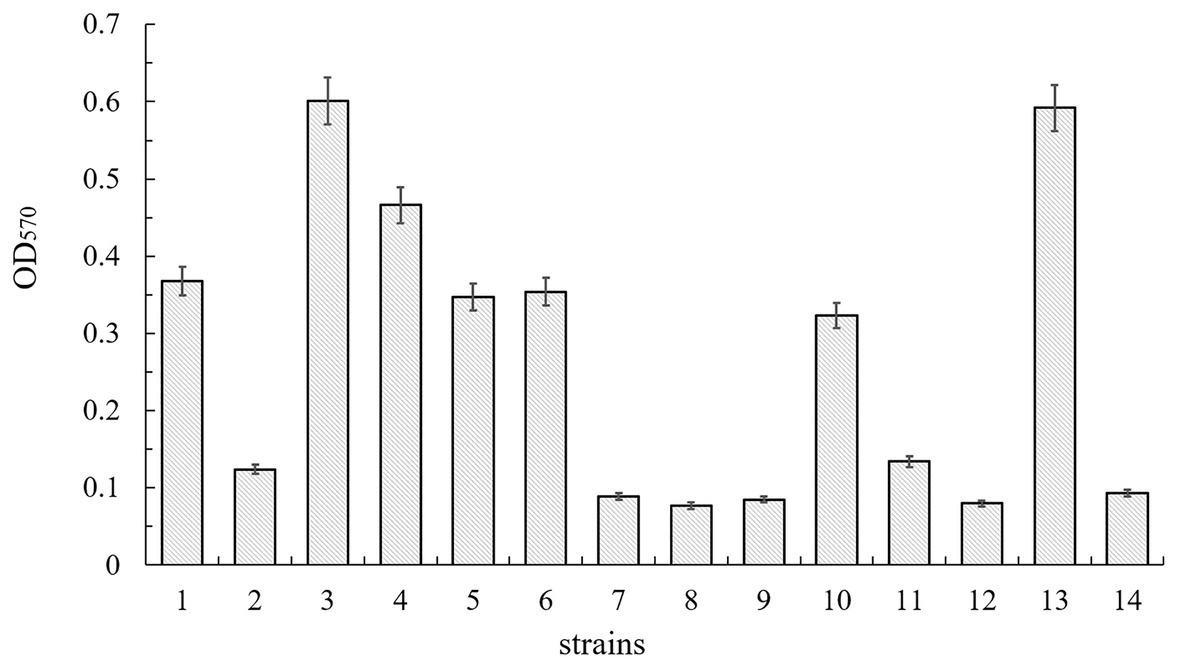
Figure 8. Biofilm production of B. cereus 0-9 (1), ΔsinI (2), ΔsinR (3), ΔsinIΔsinR (4), ΔsinI::sinI (5), ΔsinR::sinR (6), ΔgapB (7), ΔgapBΔsinI (8), ΔgapBΔsinR (9), ΔgapB::gapB (10), ΔgapBΔsinI::gapB (11), ΔgapBΔsinI::sinI (12), ΔgapBΔsinR::gapB (13), and ΔgapBΔsinR::sinR (14) were measured by crystal violet staining. The average of three parallel measurements was taken for each reading.
GapB Do Not Affect Biofilm Formation Through the SinI/R System
To determine whether GapB-mediated inhibition of biofilm formation depends on the presence or absence of SinI/R, the biofilm yield was measured using the ΔgapB, SL2001 (ΔgapBΔsinI), and SL2002 (ΔgapBΔsinR) gene mutants and their complementary strains. Figure 8 shows the relative biofilm formation ratios by the experimental strains. Compared with that of B. cereus 0-9, the biofilm formation ability of the ΔgapB mutant was decreased by 75.82%. The biofilm yield of the double-knockout strains, ΔgapBΔsinI and ΔgapBΔsinR, were decreased by 79.08 and 76.90%, respectively. Among the complementary mutants, the biofilm yields of SL7251I (ΔgapBΔsinI::sinI) and SL7252R (ΔgapBΔsinR::sinR) were also decreased by 78.26 and 74.73%, respectively. Conversely, the biofilm yield of SL7212 (ΔgapBΔsinR::gapB) was increased by 60.87%, which was the same as that of ΔsinR, and the biofilm yield of SL7211 (ΔgapBΔsinI::gapB) was decreased by 63.59%, which was the same as that of ΔsinI. Thus, we concluded that once gapB is lost, wild-type B. cereus 0-9 can no longer form biofilm normally, regardless of whether sinI and sinR are present. In other words, gapB deletion does not affect the SinI/R system function in B. cereus 0-9. And it is possible that the absence of gapB is dominant over the absence of sinR under this experimental condition.
GapB expression in mutant strains without sinR was detected by western blotting (Supplementary Figure S4). GapB was not expressed in the ΔgapB or ΔgapBΔsinR mutants but was expressed normally in the ΔsinR mutant, which is similar to that of wild-type B. cereus 0-9, indicating that the sinR does not affect GapB expression.
EPS and Extracellular DNA Content in Biofilms
The polysaccharides content in the biofilms were determined by the phenol-sulfuric acid method. According to our experiment results, the EPS contents in the biofilms of B. cereus 0-9 and ΔgapB mutant were 45.64 and 46.10 mg/g (WW), respectively (Supplementary Table S3). This suggests that the gapB gene deletion will not cause changes in the production of exopolysaccharides in B. cereus 0-9 biofilm. Therefore, it is very possible that the biofilm defect of ΔgapB was irrelated to the production of exopolysaccharides.
Table 2 shows the detection results for extracellular DNA in the biofilms. The extracellular DNA content in the biofilms formed by ΔgapB was 0.099 μg/mg, while that of wild-type B. cereus 0-9 reached 1.677 μg/mg. Thus, gapB deletion reduced the amount of extracellular DNA released. Additionally, the DNA contents of the biofilms formed by ΔgapB/gapBpro and ΔgapB::gapBorf , in which gapB gene was complemented, were recovered to the levels of wild-type B. cereus 0-9. This indicated that the change in biofilm yield due to gapB deletion was positively correlated with the extracellular DNA content in the biofilms. Vilain et al. (2009) reported that the B. cereus requires extracellular DNA to form biofilms. Therefore, we concluded that the GapB likely regulates biofilm formation of B. cereus 0-9 by controlling the release of extracellular DNA.
Deletion of gapB Induces Upregulation of the Autolysis Regulator LrgAB in the Stable Phase
Cell grown to stationary phase are the source for biofilm formation in the solid-liquid interface in static conditions. We hypothesized that the differences in the ability to form biofilms between the wild-type B. cereus 0-9 and its ΔgapB mutants, stem from changes in their transcriptomic profile at the stationary growth phase. Our results showed that only 18 genes differed significantly after knocking out gapB, among which, six genes were upregulated, and 12 genes were downregulated (Table 3). The gene with the most significantly different expression level was the operon lrgAB (containing lrgA and lrgB). LrgAB was previously reported to be a regulatory system closely related to autolysis of S. aureus NCTC8325 (Chu et al., 2013), and it negatively regulates extracellular murein hydrolase activity; thus, it can inhibit the autolysis of S. aureus (Groicher et al., 2000). LrgAB likely plays a similar role in B. cereus 0-9, which explains why less extracellular DNA exists in the ΔgapB mutant biofilm than in that of wild-type B. cereus 0-9.
Dead and Living Cells in Biofilms
Dead and living bacterial cells in biofilms were observed using a fluorescence microimaging system (Figure 9). Dead cells (red) in the biofilm of wild-type B. cereus 0-9 constituted nearly half the total cells in the suspension, but the ΔgapB mutant contained only a few dead cells. To confirm the difference between the dead and living cells in the biofilm, we determined the number of living bacteria per unit wet weight of the biofilms by the viable count method, and the results were shown in Table 4. The “colony number” in Table 4 means 100 μl of bacterial suspension of biofilm cells was diluted to 10−8, then, 100 μl of this diluent was coated on a counting plate, and the number of bacterial colonies was calculated after culturing for 24 h. The viable count was 24.65 × 109 CFU per gram (wet weight) in the B. cereus 0-9 biofilms. However, the viable count reached 181.88 × 109 CFU per gram in the ΔgapB biofilms, which was nearly 7.5 times than that of B. cereus 0-9. This result is consistent with that of the transcriptome analysis, in which lrgAB expression was the most upregulated in the ΔgapB mutant. Therefore, it is very possible that the GapB was involved in extracellular DNA release and biofilm formation by regulating the expression of LrgAB.
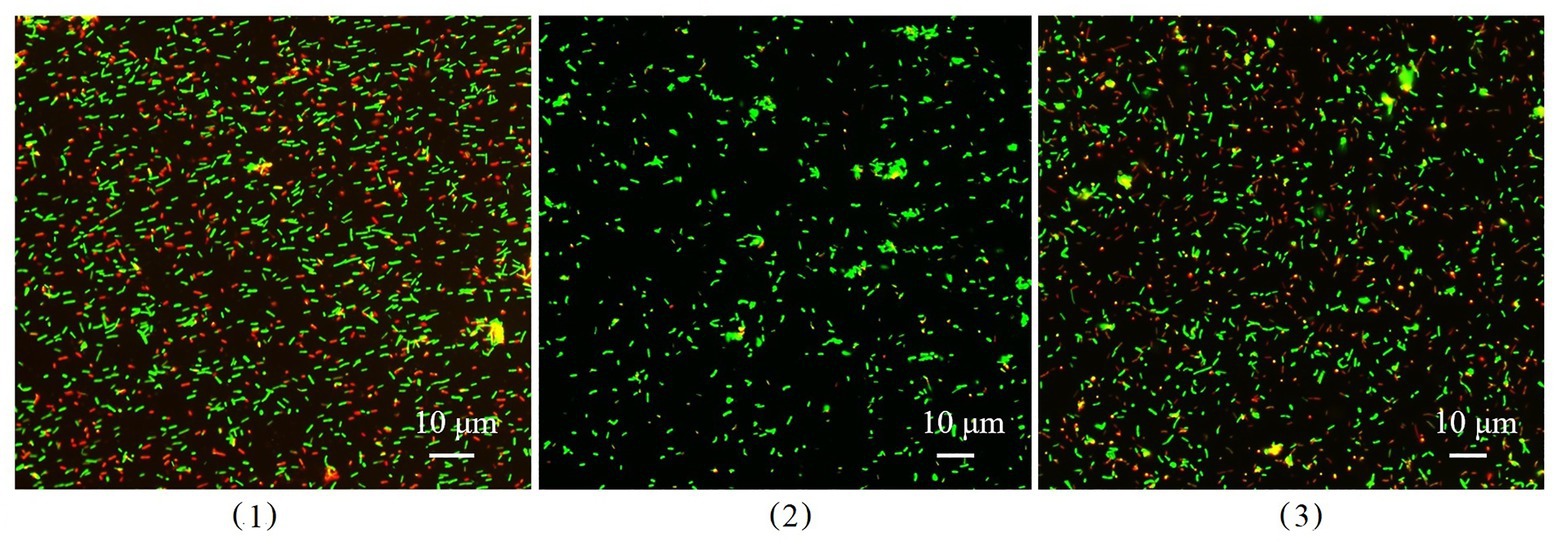
Figure 9. The image of dead and living bacterial cells in biofilms of Bacillus cereus 0-9 (1), ΔgapB (2), and ΔgapB::gapB (3) observed by fluorescence microscopy. Green fluorescence indicates the live bacterial cells and red ones are the dead cells.
LrgAB Is Involved in the Biofilm Formation of Bacillus cereus 0-9
We simultaneously knocked out lrgA and lrgB genes to construct ΔlrgAB and ΔgapBΔlrgAB mutant strains, and the determination results of their biofilm formation states are shown in Figure 10. It shows that the biofilm production of ΔlrgAB is higher than that of wild strains in the LBS medium. The biofilm formation ability of ΔgapBΔlrgAB mutant recovered, but its biofilm production is lower than that of ΔlrgAB mutant. This suggests that the LrgAB is indeed related to the biofilm formation of B. cereus 0-9, and the GapB is very possible to be the upstream regulator of LrgAB. Due to lack of regulatory proteins that maintain the vitality of bacterial cells, ΔlrgAB mutant strain may release a large number of DNA. Thus, a huge accumulation of eDNA making the biofilm production increases. These results may further support our view of “GapB is involved in extracellular DNA release and biofilm formation dependent on regulating the expression or activities of LrgAB.”
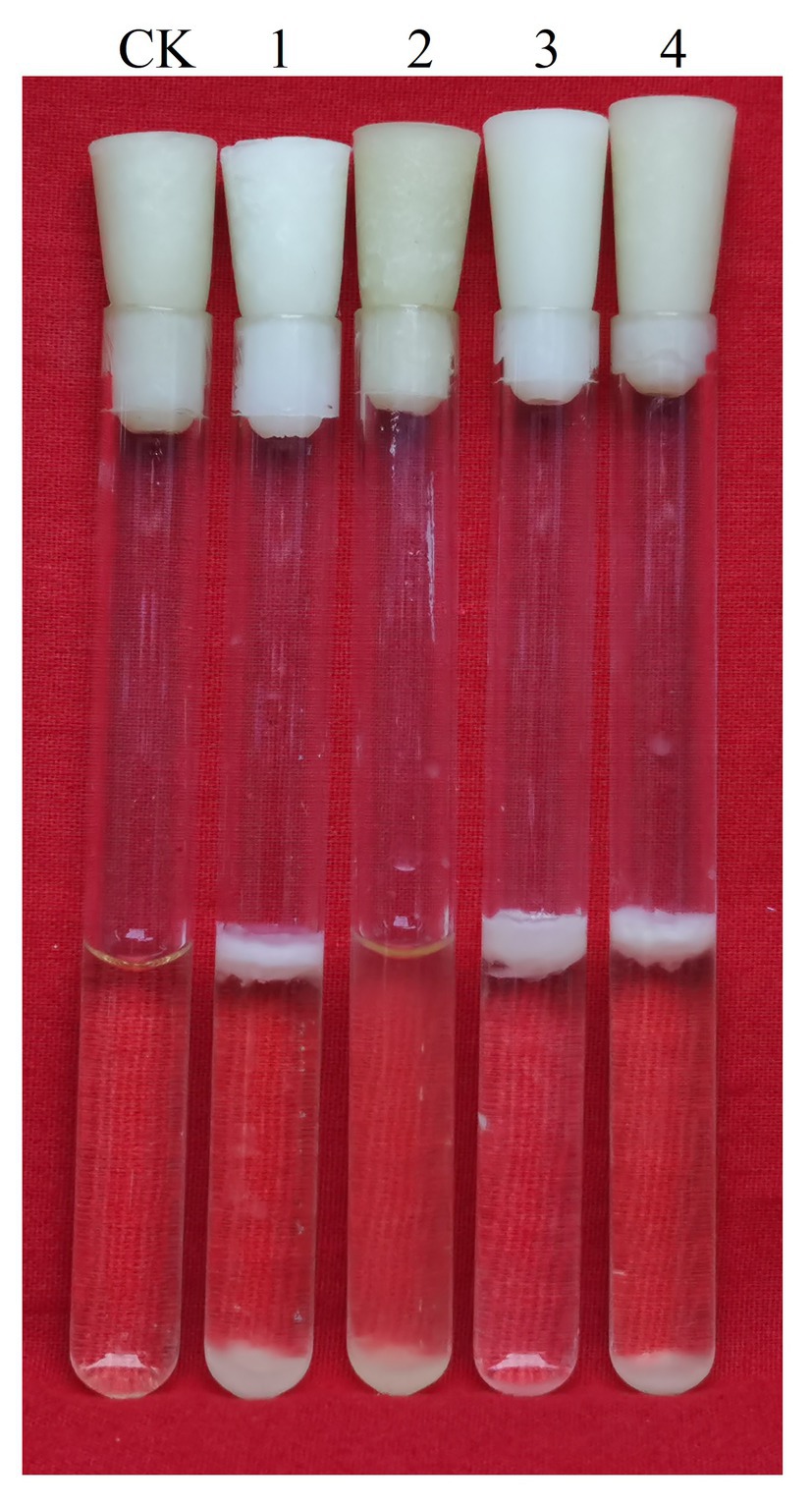
Figure 10. Biofilms of Bacillus cereus 0-9 (1), ΔgapB (2), ΔlrgAB (3), and ΔgapBΔlrgAB (4) mutants in LBS medium were imaged after 3 days of growth, using uninoculated blank medium as control (CK). Pictures are representative of five experiments.
Discussion
Deleting gapB Affected B. cereus 0-9 Biofilm Formation
Bacillus cereus 0-9 is a highly adaptable bacterium that is widely distributed in soil, water, plants, and wheat rhizospheres (Kang et al., 2019). Our previous study showed that the wild-type B. cereus 0-9 isolates can form pellicles on air-liquid interfaces and architecturally complex colonies on solid surfaces (Zhang et al., 2020a). This constructive survival strategy enables bacteria to use a greater variety of nutrients, endure rapid environmental changes, and resist multiple adverse threats (Zhang et al., 2020b). Previous reports showed that the GapA, a glyceraldehyde-3-phosphate dehydrogenase that plays a key role in glycometabolism pathways, is involved in the biofilm formation of S. aureus and B. subtilis (Purves et al., 2010; Romero et al., 2014). In our previous research, we often found that in solid medium with different carbon sources, the bacterial colony folds formed by the same bacteria are significantly different, which is also a form of biofilm existence. It is speculated that some enzymes of glucometabolism pathway may be involved in the formation of bacterial biofilm. GAPDH, as a kind of moonlighting protein, not only plays a role in the process of glucose metabolism, but also has a certain regulatory effect on the pathogenic capacity of bacteria (Giménez et al., 2014). More recent research shows that the glucose effect on Candida albicans biofilm during tissue invasion (Figueira et al., 2020). Therefore, in this study, we aimed to investigate whether GAPDH, a key regulator of glucose metabolism, affects biofilm formation in B. cereus 0-9. Whole-genome sequencing (GenBank: CP042874.1) and subsequent gene function annotation revealed that three genes encode GAPDH in the B. cereus 0-9 genome: gapA, gapB, and gapN. Thus, we knocked out the gap genes and measured the biofilm yields of these gene deletion mutants. We do not know which gap gene may be related to the regulation of biofilm formation. Therefore, mutant strains of each gap gene were constructed separately, and the production of each biofilm was measured under the same conditions. Our results showed that the biofilm yields of the ΔgapB mutant were decreased by 78.5%, but deletion of gapA and gapN did not affect B. cereus 0-9 biofilm formation. Thus, we further studied the function of gapB to illustrate the mechanism by which gapB regulates B. cereus 0-9 biofilm formation.
GapB Is a Glyceraldehyde-3-Phosphate Dehydrogenase and a Key Regulator of Gluconeogenesis
We cloned the gapB gene and expressed GapB protein in E. coli BL21 (DE3). Measuring the dehydrogenase activity of purified GapB showed that the GapB is a glyceraldehyde-3-phosphate dehydrogenase with strong dehydrogenase activities, regardless of whether NAD+ or NADP+ is a cofactor. Bacillus cereus possesses two GAPDH activities, which are NAD+- and NADP+-dependent, catalyzed by two enzymes with distinct coenzyme specificities and different phosphate regulation patterns (Abdelghani et al., 2002). Our results indicated that the GapB in B. cereus 0-9 has both NAD+- and NADP+-dependent dehydrogenase activity. Purves et al. (2010) previously reported that the GapB in S. aureus lacks NAD+-dependent dehydrogenase activity. Thus, although GapB plays an important role in glucose metabolism in different bacterial strains, some functions vary among strains. Giménez et al. (2014) reported that GapB, as a GAPDH, is a moonlighting protein in bacteria, which has unrelated and independent functions.
The Gap protein, a GAPDH, catalyzes the conversion between glyceraldehyde-3-phosphate and 1,3-diphosphate glycerate and is a key enzyme in the glycolytic pathway (Abdelghani et al., 2002). Our results showed that the B. cereus 0-9 grew well in M-eps medium, but ΔgapB could not, unless 1% glucose or glycerol was added. This suggests that the GapB may play an important role in gluconeogenesis, and the loss of anabolic carbon metabolism is most likely due to disruption of the gluconeogenesis pathway. Interestingly, the ΔgapB mutant could grow with glycerol as the primary carbon source, indicating that the GapB must play key roles before this stage in the gluconeogenic pathway for glycerol to recover the growth defect of the ΔgapB mutant. To confirm the function of gapB, we further measured the transcription level of pgm gene, which is related to the allostery of phosphorylated glucose in gluconeogenesis (Goto et al., 2016). Consequently, pgm expression was downregulated 32-fold when gapB was deleted, indicating that deleting gapB blocked the gluconeogenic pathway of B. cereus 0-9, thus inhibiting glucose-1-phosphate synthesis, thereby affecting the synthesis of polysaccharides and other bacterial phenotypes.
GapB Is Closely Related to B. cereus 0-9 Biofilm Formation
Bacillus cereus displays highly diverse lifestyles and ecological niches. It can produce various biofilms that differ in their architecture and formation mechanisms, possibly reflecting an adaptation to various environments (Majed et al., 2016). We determined the biofilm yield of ΔgapB strain and discovered that their biofilm formation ability was dramatically decreased. However, when the gapB gene was complemented, the biofilm formation ability of ΔgapB::gapB mutants could be recovered. Thus, in addition to being a GAPDH, GapB has some environmental stress resistance functions such as biofilm formation.
Interestingly, supplementing the medium with 0.1–0.5% glycerol restored the biofilm formation capacity of the ΔgapB mutants, but glucose and pyruvate did not (Figure 5). Carbon source decomposable metabolite repression (activation) is a conserved regulatory pathway of carbon source metabolism in bacteria, which ensures the orderly utilization of different carbon sources by bacteria and improves the growth rate and environmental adaptability of bacteria (Lorca et al., 2005). In G+ bacteria, including Bacillus, carbon catabolite repression (CCR) functions mainly through carbon decomposition metabolite repressor protein CcpA. In the presence of a preferred carbon source of glucose, fructose-1,6-diphosphate (FBP), as a product of glycolysis intermediate, can stimulate the bifunctional enzyme HprK/P to perform kinase activity, causing the phosphorylation of Hpr protein to produce Hpr (ser46 ~ P). Hpr binds to CcpA to form complexes that bind to CRE-sites and repress or activate transcription of metabolic genes from other carbon sources. After glucose depletion, HprK/P exerts phosphatase activity, resulting in dephosphorylation of Hpr (ser46 ~ P), inability to bind to CcpA to form complexes, and loss of regulatory function (Voort et al., 2008; Tomoyasu et al., 2010). In addition, the other two CcpA-independent carbon repressor proteins, CcpN and CcpC, inhibit gluconeogenesis and TCA cycles in the presence of glucose, ensuring the priority of glycolysis (Voort et al., 2008). Due to the conservative regulatory mechanism found above, glycolysis takes precedence after glucose addition, so the production of biofilms by ΔgapB mutants cannot be restored. The related glycerol metabolism genes glpF (glycerol uptake facilitator) and glpK (glycerol kinase) are strictly regulated by CcpA and can be expressed only when glucose utilization is completed. Therefore, when glucose is exhausted and glycerol is present, glycerol enters the cell under the action of glycerol kinase and glycerol triphosphate dehydrogenase, and is converted into dihydroxyacetone phosphate and glycerol triphosphate simultaneously, entering the glycolysis and gluconeogenesis pathways, restoring the biofilm defect of ΔgapB.
The glk gene encodes glycerol kinase, which catalyzes the phosphorylation of glycerin to glycerin-3-phosphate. Colony morphology assays of Δglk, ΔgapB, and ΔglkΔgapB revealed that the Δglk colony morphology did not noticeably differ from that of wild-type B. cereus 0-9. When gapB was knocked out, the colonies became smaller and the colony folds became less uniform (Figure 7A). Adding 1% glycerinum to the plates restored the ΔgapB colony morphology, but did not restore the double mutant, ΔglkΔgapB, which is on the same plates (Figure 7B). It indicated that the GapB is a key factor of controlling gluconeogenesis in B. cereus 0-9, and glycerol supplementation in the culture medium can remove the restriction induced by deleting gapB. Therefore, we concluded that the gapB is closely related to B. cereus 0-9 biofilm formation and is likely linked by GapB-regulated gluconeogenesis. However, how GapB is involved in regulating biofilm formation is unclear. Exopolysaccharides are important substrates for B. cereus biofilm formation (Majed et al., 2016). GapB, as a key regulator of gluconeogenesis, may regulate glucose-1-phosphate synthesis when carbon sources are scarce, thereby affecting the synthesis of polysaccharides and other bacterial phenotypes. Surprisingly, however, gapB gene deletion caused no change on EPS production of B. cereus 0-9 in LBS medium (Supplementary Table S3). Therefore, the mechanism by which GapB regulates biofilm formation should be further explored.
GapB Did Not Affect Biofilm Formation Through the SinI/R System
Biofilm formation requires a complex regulatory pathway that coordinates gene expression with external environmental conditions and structural components involved in assembling the protective extracellular matrix (Romero, 2013; Vlamakis et al., 2013). The SinI/R system is the most clearly reported system for regulating B. cereus biofilm formation (Newman et al., 2013). SinR is a DNA-binding protein that represses the transcription of operons involved in producing both exopolysaccharides and the protein component of the matrix (Branda et al., 2001; Diethmaier et al., 2014). In B. subtilis and B. cereus, three proteins, TapA, TasA, and SipW, are required for biofilm formation (Caro-Astorga et al., 2015; Candela et al., 2019). SinR represses transcription of the tapA-sipW-tasA operon (Kearns et al., 2005). Gene annotations show that both sinI and sinR exist in the B. cereus 0-9 genome. Our results showed that the biofilm formation ability of ΔsinI was decreased by 66.30%, and that of the ΔsinR mutants was increased by 63.32% compared with that of wild-type B. cereus 0-9. Knocking out sinR upregulated tasA, calY, and sipW transcription levels by 147.03-, 630.35-, and 445.72-fold, respectively (Supplementary Table S2). Therefore, the SinI/R system exists in B. cereus 0-9 and regulates its biofilm formation as previously reported by Newman et al. (2013).
We further constructed a series of double-knockout strains and their complementary mutants and measured their biofilm yields. The mutant strains without gapB showed a similar ability to form biofilms as that of ΔgapB, regardless of whether sinR and sinI were present (Figure 8). When gapB was complemented, ΔgapBΔsinI::gapB showed the same ability to form biofilms as did ΔsinI and as did ΔgapBΔsinR::gapB with ΔsinR. This indicates that the GapB is not involved in regulating the SinI/R system. Furthermore, GapB was expressed in the ΔsinR mutant. Therefore, we concluded that GapB may affect biofilm formation through another regulatory mechanism but not through the SinI/R system.
GapB Affects Biofilm Formation by Regulating Extracellular DNA Production
Bacteria are usually embedded in a self-produced matrix to form biofilm, and the complex architecture of a biofilm is maintained by an extracellular matrix of EPSs, proteins, and eDNA (Flemming and Wingender, 2010; Candela et al., 2019). The function of eDNA in biofilms has attracted much attention in recent years (Okshevsky et al., 2015; Saunders et al., 2020). Bacillus cereus follows this rule, and its matrix contains all three components (Majed et al., 2016). GapB regulates B. cereus 0-9 biofilm formation independent of EPSs and regulatory proteins in the SinI/R system, which is likely related to the release of DNA in the matrix. Exponential-phase cells of B. cereus are reportedly decorated with extracellular DNA, and biofilm formation requires DNA as part of the extracellular polymeric matrix (Vilain et al., 2009). Bacillus cereus can form DNA-containing biofilms on glass surfaces exposed to exponentially growing bacterial populations. Thus, we measured the extracellular DNA content in the biofilms formed by B. cereus 0-9 and its ΔgapB mutant and discovered that deleting gapB decreased the DNA content in the biofilms from 167.7 to 9.9 mg/g, indicating that the change in biofilm yield caused by deleting gapB was positively correlated with the extracellular DNA content in the biofilms. Therefore, GapB is involved in regulating biofilm formation, likely by controlling the release of extracellular DNA. However, whether this extracellular DNA comes from cell lysis remains uncertain.
GapB Participated in Regulating Biofilm Formation Through LrgAB
To explore the mechanism by which GapB is involved in regulating extracellular DNA production in B. cereus 0-9 biofilm, we sequenced the transcriptomes of the ΔgapB mutants. The gene that differed the most significantly between B. cereus 0-9 and ΔgapB was the lrgAB gene, which is annotated as an operon. LrgAB was previously reported to play a key role in autolysis, biofilm formation, glucosyltransferase expression, and oxidative stress tolerance (Ahn et al., 2010). LrgAB negatively regulates extracellular murein hydrolase activity and is a type of regulatory system closely related to the autolysis of S. aureus (Chu et al., 2013). To prove whether the biofilm formation defect of ΔgapB is related to the lysis of bacterial cells in biofilm, we counted the viable bacteria in per unit of wet-weight biofilms and found that the number of living cells in the biofilm formed by ΔgapB mutant was nearly 7.5 times more than that of wild-type B. cereus 0-9 (Table 4). Observing the ratio of dead to living cells in the biofilm using a fluorescence microimaging system confirmed this result (Figure 9). Thus, we concluded that the biofilm formation defect of ΔgapB is related to bacterial cell autophagy and lysis, which is regulated by LrgAB. This conclusion is inconsistent with that of Vilain et al. (2006), who considered the B. cereus biofilm extracellular DNA did not originate primarily through cell lysis; this conclusion was speculative and not directly evidenced. We further knocked out lrgAB gene, and found that the ΔlrgAB can form more biofilms than wild-type B. cereus 0-9 at the same conditions. It means that the deletion of lrgAB inevitably induces bacterial cell autophagy and lysis, so, it leads to more extracellular DNA existing in the biofilms of ΔlrgAB than in that of B. cereus 0-9. The biofilm production of ΔgapBΔlrgAB was recovered to that of B. cereus 0-9, but ΔgapB (Figure 10). Therefore, we concluded that deleting gapB would likely induce the expression of lrgAB to prevent autolysis of the ΔgapB cells, thus reducing the release of extracellular DNA and affecting its biofilm formation. It also indicated that the GapB is located in the upstream of lrgAB, and it is involved in extracellular DNA release and biofilm formation of B. cereus 0-9 by regulating the expression or activities of LrgAB. However, the biofilm production of ΔgapBΔlrgAB is lower than that of ΔlrgAB mutant. It means that the absence of GapB also affects biofilm formation through any mechanisms different from regulation of lrgAB. Transcriptional sequencing results show that in addition to lrgA and lrgB, there are significant differences in the expression levels of other 10 genes, which is also indicating that the GapB is involved in the regulation of various gene expressions, not only lrgAB. At present, there are still many interesting mysteries about GapB, which will attract us to explore it further in our future study.
In addition to its glycolytic activity, GAPDH is implicated in regulation of proliferation, autophagy, maintenance of highly tumorogenic cancer cells, development of apoptosis, and many other processes (Lazarev et al., 2016). However, how GAPDH is linked to bacterial autolysis or modified cell death is unclear. When GAPDH molecules are inactivated by oxidation or S-nitrosylation, they may bind polypeptides, such as Siah1 ubiquitin ligase, and transport them to the nucleus (Hara et al., 2006). Other studies have reported that an extracellular Streptococcus GAPDH can interact with C5, a component of the complement system, which promotes its degradation, in coordination with the bacterial surface protease (Giménez et al., 2014). This strategy allows the pathogenic Streptococcus to escape detection by the host immune system. Cell surface-associated extracellular DNA would provide a selective advantage to B. cereus in its natural environment in the soil (Vilain et al., 2009). Deleting gapB can affect lrgAB expression, which is closely related to autophagy; however, it is unclear how GapB is linked to the autolysis of B. cereus. It is also unclear how GapB regulates programed bacterial cell lysis and releases intracellular nutrients and their DNA through the LrgAB system. We are interested in answering these questions and plan to study them in our future research.
Conclusion
The B. cereus 0-9 genome contains three gap genes that encode three GAPDHs: GapA, GapB, and GapN. Only GapB plays a key role in B. cereus 0-9 biofilm formation. Here, we focused mainly on the function of GapB and the mechanism by which it regulates B. cereus 0-9 biofilm formation. GapB has GAPDH activities of both NAD+- and NADP+-dependent dehydrogenases and may play an important role in gluconeogenesis, which can control the anabolic carbon metabolism, most likely by regulating the gluconeogenesis pathway. The gapB gene is closely related to B. cereus 0-9 biofilm formation and is likely linked by gluconeogenesis. Studies on the mechanism of gluconeogenesis that affects biofilm formation have shown that the GapB is uninvolved in regulating biofilm formation through polysaccharide synthesis. The SinI/R system exists in B. cereus 0-9, but GapB regulates B. cereus 0-9 biofilm formation and does not rely on the regulatory proteins in the sinI/R system. Thus, GapB likely regulates B. cereus 0-9 biofilm formation relative to the release of DNA in the matrix. The transcriptome sequencing results showed that only 18 genes differed significantly after knocking out gapB, among which, the most significant difference between B. cereus 0-9 and ΔgapB was the lrgAB gene, which is annotated as an operon. We counted the viable bacteria per unit of biofilm, and the number of living cells in the biofilm formed by the ΔgapB strain was nearly 7.5 times than that of wild-type B. cereus 0-9. Therefore, we concluded that deleting gapB would likely to prevent autolysis of the ΔgapB cells, thereby reducing the release of extracellular DNA and affecting its biofilm formation. It also indicated that the GapB is located in the upstream of lrgAB, and it is involved in biofilm formation of B. cereus 0-9 by regulating the expression or activities of LrgAB. These results can help further to explain the biocontrol characteristics of B. cereus 0-9. We plan to conduct further research on the mechanism by which GapB regulates extracellular DNA release through the LrgAB system.
Data Availability Statement
The datasets presented in this study can be found in online repositories. The names of the repository/repositories and accession number(s) can be found in the article/ Supplementary Material.
Author Contributions
JZ and LM designed the experiments, carried out the experiment of the mechanism of biofilm formation, determined the dead and living cells in biofilms, drafted the manuscript, and revised the paper. YZ helped to design some experiments, carried out the experiment of quantitative detection of biofilms, and done a lot of photographing and sorting work of experimental pictures. LS constructed the expression vectors and gene knockout strains, and has done a lot of work in pioneering studies such as the phenotypic determination of mutant strains and gene expression level. QL carried out the experiment of determination of double-stranded DNAs in biofilms and collected test data. LZ and FL performed the data analyses and helped the experiment going. GW led the relevant project and helped designed the experiment. All authors contributed to the article and approved the submitted version.
Funding
This study was funded by the National Nature Science Foundation of China (number: 31701831).
Conflict of Interest
The authors declare that the research was conducted in the absence of any commercial or financial relationships that could be construed as a potential conflict of interest.
Acknowledgments
We would like to thank the Microbiology Bioengineering Experimental Center of Henan University for assistance with figures and feedback on an early manuscript draft.
Supplementary Material
The Supplementary Material for this article can be found online at:
https://www.frontiersin.org/articles/10.3389/fmicb.2020.591926/full#supplementary-material
Abbreviations
GAPDH, Glyceraldehyde-3-phosphate dehydrogenase; LB, Luria-Bertani broth; LBS, Luria-Bertani broth supplemented with some mineral salts; KBMS, King broth medium supplemented with glucose; qRT-PCR, Real-time quantitative PCR; EPS, Extracellular polysaccharide; CFU, Colony-forming units.
References
Abdelghani, I., Aurelio, S., and Abdelaziz, S. (2002). A phosphate-stimulated NAD(P)+-dependent glyceraldehyde-3-phosphate dehydrogenase in Bacillus cereus. FEMS Microbiol. Let. 211, 29–35. doi: 10.1016/S0378-1097(02)00639-0
Ahn, S. J., Rice, K. C., Oleas, J., Bayles, K. W., and Burne, R. A. (2010). The Streptococcus mutans Cid and Lrg systems modulate virulence traits in response to multiple environmental signals. Microbiology 156, 3136–3147. doi: 10.1099/mic.0.039586-0
Arnaud, M., Chastanet, A., and Débarbouillé, M. (2004). New vector for efficient allelic replacement in naturally nontransformable, low-GC-content, gram-positive Bacteria. Appl. Environ. Microb. 70, 6887–6891. doi: 10.1128/AEM.70.11.6887-6891.2004
Arutyunova, E. I., Domnina, L. V., Chudinova, A. A., Makshakova, O. N., Arutyuno, D. Y., and Muronetz, V. I. (2013). Localization of non-native D-glyceraldehyde-3-phosphate dehydrogenase in growing and apoptotic HeLa cells. Biochemistry 78, 91–95. doi: 10.1134/S0006297913010112
Asadi, F., Barshan-tashnizi, M., Hatamian-Zarmi, A., Davoodi-Dehaghani, F., and Ebrahimi-Hosseinzadeh, F. (2020). Enhancement of exopolysaccharide production from Ganoderma lucidum using a novel submerged volatile co-culture system. Fungal Biol. doi: 10.1016/j.funbio.2020.09.010 (in press).
Branda, S. S., Gonzalez-Pastor, J. E., Ben-Yehuda, S., Losick, R., and Kolter, R. (2001). Fruiting body formation by Bacillus subtilis. Proc. Natl. Acad. Sci. U.S.A 98, 11621–11626. doi: 10.1073/pnas.191384198
Candela, T., Fagerlund, A., Buisson, C., et al. (2019). CalY is a major virulence factor and a biofilm matrix protein. Mol. Microbiol. 111, 1416–1429. doi: 10.1111/mmi.14184
Caro-Astorga, J., Pérez-García, A., De Vicente, A., and Romero, D. (2015). A genomic region involved in the formation of adhesin fibers in Bacillus cereus biofilms. Front. Microbiol. 5:745. doi: 10.3389/fmicb.2014.00745
Chu, F., Kearns, D. B., Branda, S. S., Kolter, R., and Losick, R. (2006). Targets of the master regulator of biofilm formation in Bacillus subtilis. Mol. Microbiol. 59, 1216–1228. doi: 10.1111/j.1365-2958.2005.05019.x
Chu, X., Xia, R., He, N., and Fang, Y. (2013). Role of rot in bacterial autolysis regulation of Staphylococcus aureus NCTC8325. Res. Microbiol. 164, 695–700. doi: 10.1016/j.resmic.2013.06.001
Diethmaier, C., Newman, J. A., Akos, T. K., et al. (2014). The YmdB phosphodiesterase is a global regulator of late adaptive responses in Bacillus subtilis. J. Bacteriol. 196, 265–275. doi: 10.1128/JB.00826-13
Figueira, D. M. L., Filho, A. P. R., Silva, W. J., Cury, A. A. D. B., and Ruiz, K. G. S. (2020). Glucose effect on Candida albicans biofilm during tissue invasion. Arch. Oral Biol. 117:104728. doi: 10.1016/j.archoralbio.2020.104728
Flemming, H. C., Neu, T. R., and Wozniak, D. J. (2007). The EPS matrix, the “house of biofilm cells.” J. Bacteriol. 189, 7945–7947. doi: 10.1128/JB.00858-07
Flemming, H. C., and Wingender, J. (2010). The biofilm matrix. Nat. Rev. Microbiol. 8, 623–633. doi: 10.1038/nrmicro2415
Gao, T., Li, Y., Ding, M., Chai, Y., and Wang, Q. (2017). The phosphotransferase system gene ptsI in Bacillus cereus regulates expression of sodA2 and contributes to colonization of wheat roots. Res. Microbiol. 168, 524–535. doi: 10.1016/j.resmic.2017.04.003
Giménez, R., Aguilera, L., Ferreira, E., Baldomà, J. A. L., and Badia, J. (2014). Glyceraldehyde-3-phosphate dehydrogenase as a moonlighting protein in bacteria. Res. Signpost. 37/661, 165–180. ISBN: 978-81-308-0554-2
Goto, L. S., Alexandrino, A. V., Pereira, C. M., Martins, C. S., Pereira, H. M., Brandao-Neto, J., et al. (2016). Structural and functional characterization of the phosphoglucomutase from Xanthomonas citri subsp. citri. Biochim. Biophys. Acta 1864, 1658–1666. doi: 10.1016/j.bbapap.2016.08.014
Groicher, K. H., Firek, B. A., Fujimoto, D. F., and Bayles, K. W. (2000). The Staphylococcus aureus lrgab operon modulates murein hydrolase activity and penicillin tolerance. J. Bacteriol. 182:1794. doi: 10.1128/JB.182.7.1794-1801.2000
Hara, M. R., Cascio, M. B., and Sawa, A. (2006). GAPDH as a sensor of NO stress. Biochim. Biophys. Acta 17, 502–509. doi: 10.1016/j.bbadis.2006.01.012
Kang, X., Wang, L., Guo, Y., et al. (2019). A comparative transcriptomic and proteomic analysis of hexaploid wheat’s responses to colonization by Bacillus velezensis and Gaeumannomyces graminis, both separately and combined. Mol. Plant-Microbe Interact. 32, 1336–1347. doi: 10.1094/MPMI-03-19-0066-R
Kearns, D. B., Chu, F., Branda, S. S., Kolter, R., and Losick, R. (2005). A master regulator for biofilm formation by Bacillus subtilis. Mol. Microbiol. 55, 739–749. doi: 10.1111/j.1365-2958.2004.04440.x
Lazarev, V. F., Nikotina, A. D., Semenyuk, P. I., Evstafyeva, D. B., and Margulis, B. A. (2016). Small molecules preventing GAPDH aggregation are therapeutically applicable in cell and rat models of oxidative stress. Free Radic. Biol. Med. 92, 29–38. doi: 10.1016/j.freeradbiomed.2015.12.025
Lewis, R. J., Brannigan, J. A., Offen, W. A., Smith, I., and Wilkinson, A. J. (1998). An evolutionary link between sporulation and prophage induction in the structure of a repressor: anti-repressor complex. J. Mol. Biol. 283, 907–912. doi: 10.1006/jmbi.1998.2163
Lewis, R. J., Brannigan, J. A., Smith, I., and Wilkinson, A. J. (1996). Crystallisation of the Bacillus subtilis sporulation inhibitor SinR, complexed with its antagonist, SinI. FEBS Lett. 378, 98–100. doi: 10.1016/0014-5793(95)01432-2
Li, M., Zhao, J., Tang, N., Sun, H., and Huang, J. (2018). Horizontal gene transfer from bacteria and plants to the arbuscular mycorrhizal fungus Rhizophagus irregularis. Front. Plant Sci. 9:701. doi: 10.3389/fpls.2018.00701
Lorca, G., Chung, Y. J., Barabote, R. D., Weyler, W., Schilling, C. H., and Saier, M. H. (2005). Catabolite repression and activation in Bacillus subtilis: dependency on CcpA, HPr, and HprK. J. Bacteriol. 187, 7826–7839. doi: 10.1128/JB.187.22.7826-7839.2005
Majed, R., Faille, C., Kallassy, M., and Gohar, M. (2016). Bacillus cereus biofilms-same, only different. Front. Microbiol. 7:1054. doi: 10.3389/fmicb.2016.01054
Masuko, T., Minami, A., Iwasaki, N., Majima, T., Nishimura, S. I., and Lee, Y. C. (2005). Carbohydrate analysis by a phenol-sulfuric acid method in microplate format. Anal. Biochem. 339, 69–72. doi: 10.1016/j.ab.2004.12.001
Moons, P., Chris, M., and Abram, A. (2009). Bacterial interactions in biofilms. Crit. Rev. Microbiol. 35, 157–168. doi: 10.1080/10408410902809431
Newman, J. A., Rodrigues, C., and Lewis, R. J. (2013). Molecular basis of the activity of SinR protein, the master regulator of biofilm formation in Bacillus subtilis. J. Biol. Chem. 288, 10766–10778. doi: 10.4161/cib.25658
Okshevsky, M., Regina, V. R., and Meyer, R. L. (2015). Extracellular DNA as a target for biofilm control. Curr. Opin. Biotechnol. 33, 73–80. doi: 10.1016/j.copbio.2014.12.002
Park, S. Y., Kim, H. K., Yoo, S. K., Oh, T. K., and Lee, J. K. (2000). Characterization of glk, a gene coding for glucose kinase of Corynebacterium glutamicum. FEMS Microbiol. Let. 188, 209–215. doi: 10.1016/s0378-1097(00)00238-x
Purves, J., Cockayne, A., Moody, P. C. E., and Morrissey, J. A. (2010). Comparison of the regulation, metabolic functions, and roles in virulence of the glyceraldehyde-3-phosphate dehydrogenase homologues gapA and gapB in Staphylococcus aureus. Infect. Immun. 78, 5223–5232. doi: 10.1128/IAI.00762-10
Romero, D. (2013). Bacterial determinants of the social behavior of Bacillus subtilis. Res. Microbiol. 164, 788–798. doi: 10.1016/j.resmic.2013.06.004
Romero, D., Vlamakis, H., Losick, R., and Kolter, R. (2014). Functional analysis of the accessory protein TapA in Bacillus subtilis amyloid fiber assembly. J. Bacteriol. 196, 1505–1513. doi: 10.1128/JB.01363-13
Saunders, S. H., Tse, E. C. M., Yates, M. D., Otero, F. J., Trammell, S. A., Stemp, E. D. A., et al. (2020). Extracellular DNA promotes efficient extracellular electron transfer by pyocyanin in Pseudomonas aeruginosa biofilms. Cell 182, 919–932. doi: 10.1016/j.cell.2020.07.006
Song, Y., Miao, Y., and Song, C. P. (2014). Behind the scenes, the roles of reactive oxygen species in guard cells. New Phytol. 201, 1121–1140. doi: 10.1111/nph.12565
Tomoyasu, T., Tabata, A., Hiroshima, R., Imaki, H., Masuda, S., Whiley, R. A., et al. (2010). Role of catabolite control protein a in the regulation of intermedilysin production by Streptococcus intermedius. Infect. Immun. 78, 4012–4021. doi: 10.1128/IAI.00113-10
Vilain, S., Luo, Y., Hildreth, M. B., and Brozel, V. S. (2006). Analysis of the life cycle of the soil saprophyte Bacillus cereus in liquid soil extract and in soil. Appl. Environ. Microbiol. 72, 4970–4977. doi: 10.1128/AEM.03076-05
Vilain, S., Pretorius, J. M., Theron, J., and Brozel, V. S. (2009). DNA as an adhesin: Bacillus cereus requires extracellular DNA to form biofilms. Appl. Environ. Microbiol. 75, 2861–2868. doi: 10.1128/AEM.01317-08
Vlamakis, H., Chai, Y., Beauregard, P., Losick, R., and Kolter, R. (2013). Sticking together: building a biofilm the Bacillus subtilis way. Nat. Rev. Microbiol. 11, 157–168. doi: 10.1038/nrmicro2960
Voort, M., Kuipers, O. P., Buist, G., Vos, W. M., and Abee, T. (2008). Assessment of CcpA-mediated catabolite control of gene expression in Bacillus cereus ATCC 14579. BMC Microbiol. 8:62. doi: 10.1186/1471-2180-8-62
Wan, T., Liu, Z. M., Li, L. F., et al. (2018). A genome for gnetophytes and early evolution of seed plants. Nat. Plants 4, 82–89. doi: 10.1038/s41477-017-0097-2
Whitchurch, C. B., Tolker-Nielsen, T., Ragas, P. C., and Mattick, J. S. (2002). Extracellular DNA required for bacterial biofilm formation. Science 295:1487. doi: 10.1126/science.295.5559.1487
Xu, Y., Chen, M., Zhang, Y., Wang, M., Wang, Y., Huang, Q., et al. (2014). The phosphotransferase system gene ptsI in the endophytic bacterium Bacillus cereus is required for biofilm formation, colonization, and biocontrol against wheat sharp eyespot. FEMS Microbiol. Let. 354, 142–152. doi: 10.1111/1574-6968.12438
Yan, M., Wang, X., Deng, J., Wang, L., Cui, Z., Deng, J., et al. (2016). DNA methylation and cerebellar development, the regulation of notch and Shh pathway. Ital. J. Zool. 83, 34–42. doi: 10.1080/11250003.2015.1126651
Zhang, J., Wang, H., Huang, Q., Zhang, Y., Zhao, L., Liu, F., et al. (2020a). Four superoxide dismutases of Bacillus cereus 0-9 are non-redundant and perform different functions in diverse living conditions. World J. Microbiol. Biotechnol. 36:12. doi: 10.1007/s11274-019-2786-7
Keywords: biofilm, glyceraldehyde-3-phosphate dehydrogenase, extracellular DNA, gluconeogenesis, GapB
Citation: Zhang J, Meng L, Zhang Y, Sang L, Liu Q, Zhao L, Liu F and Wang G (2020) GapB Is Involved in Biofilm Formation Dependent on LrgAB but Not the SinI/R System in Bacillus cereus 0-9. Front. Microbiol. 11:591926. doi: 10.3389/fmicb.2020.591926
Edited by:
Ilana Kolodkin-Gal, Weizmann Institute of Science, IsraelReviewed by:
David Salvador Zamorano Sanchez, National Autonomous University of Mexico, MexicoYang Wu, Fudan University, China
Copyright © 2020 Zhang, Meng, Zhang, Sang, Liu, Zhao, Liu and Wang. This is an open-access article distributed under the terms of the Creative Commons Attribution License (CC BY). The use, distribution or reproduction in other forums is permitted, provided the original author(s) and the copyright owner(s) are credited and that the original publication in this journal is cited, in accordance with accepted academic practice. No use, distribution or reproduction is permitted which does not comply with these terms.
*Correspondence: Gang Wang, Z3dhbmdiaW9AaGVudS5lZHUuY24=
†These authors share first authorship