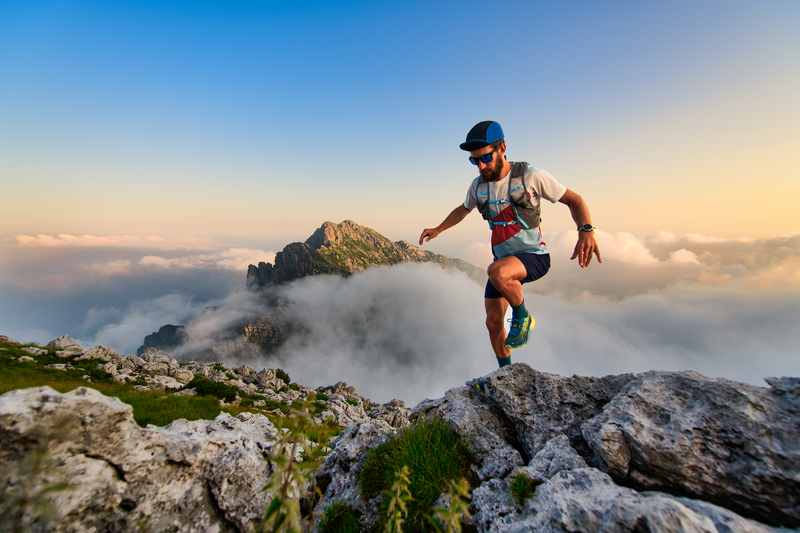
95% of researchers rate our articles as excellent or good
Learn more about the work of our research integrity team to safeguard the quality of each article we publish.
Find out more
ORIGINAL RESEARCH article
Front. Microbiol. , 18 September 2020
Sec. Microbiotechnology
Volume 11 - 2020 | https://doi.org/10.3389/fmicb.2020.586601
This article is part of the Research Topic Bioconversion and Biorefinery of C1 Compounds View all 16 articles
Cyanobacteria, the largest phylum of prokaryotes, perform oxygenic photosynthesis and are regarded as the ancestors of the plant chloroplast and the purveyors of the oxygen and biomass that shaped the biosphere. Nowadays, cyanobacteria are attracting a growing interest in being able to use solar energy, H2O, CO2 and minerals to produce biotechnologically interesting chemicals. This often requires the introduction and expression of heterologous genes encoding the enzymes that are not present in natural cyanobacteria. However, only a handful of model strains with a well-established genetic system are being studied so far, leaving the vast biodiversity of cyanobacteria poorly understood and exploited. In this study, we focused on the robust unicellular cyanobacterium Cyanothece PCC 7425 that has many interesting attributes, such as large cell size; capacity to fix atmospheric nitrogen (under anaerobiosis) and to grow not only on nitrate but also on urea (a frequent pollutant) as the sole nitrogen source; capacity to form CO2-sequestrating intracellular calcium carbonate granules and to produce various biotechnologically interesting products. We demonstrate for the first time that RSF1010-derived plasmid vectors can be used for promoter analysis, as well as constitutive or temperature-controlled overproduction of proteins and analysis of their sub-cellular localization in Cyanothece PCC 7425. These findings are important because no gene manipulation system had been developed for Cyanothece PCC 7425, yet, handicapping its potential to serve as a model host. Furthermore, using this toolbox, we engineered Cyanothece PCC 7425 to produce the high-value terpene, limonene which has applications in biofuels, bioplastics, cosmetics, food and pharmaceutical industries. This is the first report of the engineering of a Cyanothece strain for the production of a chemical and the first demonstration that terpene can be produced by an engineered cyanobacterium growing on urea as the sole nitrogen source.
Cyanobacteria, the oldest and largest phylum of prokaryotes that perform the plant-like photosynthesis (Schirrmeister et al., 2015), are regarded as the ancestors of the plant chloroplast (Ponce-Toledo et al., 2017) and the purveyors of the oxygen that shaped our biosphere (Hamilton et al., 2016). Contemporary cyanobacteria still capture a vast quantity of solar energy to assimilate huge amounts of CO2 (Dai et al., 2018) and nitrogen (nitrate, ammonium or urea) (Singh et al., 2016; Veaudor et al., 2019) to produce an enormous biomass that sustain most life forms on our planet. In colonizing most waters (fresh, brackish and marine) and soils (even deserts) biotopes, cyanobacteria have developed as widely diverse organisms. Their genomes vary in size (1.44–12.07 Mb) and organization (presence/absence of plasmids and linear chromosomes in addition to their circular chromosome) (Cassier-Chauvat et al., 2016). Furthermore, cyanobacteria display different cell sizes (1–10 μm) and morphologies, ranging from unicellular (cylindrical or spherical) (Koksharova and Wolk, 2002; Mazouni et al., 2004) to complex multi-cellular (filamentous) species (Cassier-Chauvat and Chauvat, 2014; Montgomery, 2015) capable to differentiate specialized cells for nitrogen fixation (Herrero et al., 2016) or survival to harsh environments (Chauvat et al., 1982; Legrand et al., 2019). Thus, cyanobacteria are interesting models to study how cells divide and pass their morphology on to their progeny (Cassier-Chauvat and Chauvat, 2014). Collectively, the disparities of the genome size, cell morphology and metabolism of cyanobacteria should prompt us to analyze a larger number of evolutionary-distant models to better understand and distinguish the common and species-specific aspects of cyanobacteria (Cassier-Chauvat and Chauvat, 2018).
Besides their great interest for basic science (Cassier-Chauvat and Chauvat, 2018), cyanobacteria are also regarded as promising cell factories for the production of chemicals for human health (Cassier-Chauvat et al., 2017; Demay et al., 2019) and industries (Cassier-Chauvat and Chauvat, 2018; Knoot et al., 2018). They capture solar energy at high efficiencies (3–9%) (Ducat et al., 2011) to fix a huge amount of carbon from atmospheric CO2 (about 25 gigatons annually) into a huge energy-dense biomass (Jansson and Northen, 2010), and they tolerate high CO2-containing (≥50%) industrial gas (Ducat et al., 2011). So far, only a handful of model strains with a well-established genetic have been engineered, such as Synechocystis PCC 6803, Synechococcus PCC 7942, or Synechococcus PCC 7002, leading to a weak and often transient production (Knoot et al., 2018; Lin and Pakrasi, 2019). Thus, the influence of the large biodiversity of cyanobacteria on the efficiency of the photosynthetic production of chemicals, has been overlooked.
For all the above-mentioned reasons we think that the time has come to enlarge the panel of the model cyanobacteria to better study and exploit their biodiversity for basic and applied research purposes.
In this study, we focused our attention on the poorly studied unicellular cyanobacterium Cyanothece PCC 7425 (also designated as Cyanothece ATCC 29141), which was isolated in 1972 from a rice paddy in Senegal (Rippka et al., 1979), because it has numerous attractive properties. Cyanothece PCC 7425 has larger cells (about 3–4 μm) (Porta et al., 2000; Bandyopadhyay et al., 2011) than the well-studied models Synechococcus PCC 7942 (cylindrical shape, 1.5 μm × 0.5 μm, Koksharova and Wolk, 2002) and Synechocystis PCC 6803 (1.5 μm in diameter, Mazouni et al., 2004). This feature should facilitate the analysis of the localization of proteins involved in assembly and distribution of the CO2-fixing carboxysomes and cell division, which are so far mainly studied in Synechocystis PCC 6803 and Synechococcus PCC 7942 (Cassier-Chauvat and Chauvat, 2014; Sommer et al., 2019; Sun et al., 2019; MacCready et al., 2020). Unlike these models, Cyanothece PCC 7425 can fix atmospheric nitrogen in anaerobiosis (Bandyopadhyay et al., 2011). It is also able to form intracellular CO2-sequestrating calcium carbonate granules, an interesting but as yet poorly studied particularity (Blondeau et al., 2018). Cyanothece PCC 7425 can also synthesize various biotechnologically interesting products, such as: (i) cyanophycin, the nitrogen-rich polymer of arginine and aspartate (Klemke et al., 2016); (ii) cyanobactins, a family of cyclic peptides (Houssen et al., 2012); (iii) alkane, sucrose and polyhydroxyalkanoates (biodegradable bioplastics) (Porta et al., 2000; Bandyopadhyay et al., 2011).
Furthermore, the genome of Cyanothece PCC 7425 (5.82 Mb) in being much larger than those of the well-studied models Synechocystis PCC 6803 (3.95 Mb), Synechococcus PCC 7002 (3.40 Mb) and Synechococcus PCC 7942 (2.75 Mb), should teach us new lessons about cyanobacteria. For example, Cyanothece PCC 7425 has the genes encoding the two (anti-oxidant) super-oxide dismutases SodA (Mn-dependent) and SodB (Fe-dependent), whereas Synechocystis PCC 6803, Synechococcus PCC 7002 and Synechococcus PCC 7942 only have SodB (Veaudor et al., 2019). Similarly, Cyanothece PCC 7425 encodes the anti-oxidant glutathione reductase enzyme that is missing in both Synechocystis PCC 6803 (Marteyn et al., 2009) and Synechococcus PCC 7002 (Narainsamy et al., 2013). Cyanothece PCC 7425 has two radA DNA-repair genes whereas all three model cyanobacteria have a single-copy radA gene (Cassier-Chauvat et al., 2016). Also interestingly, Cyanothece PCC 7425 possesses the full panoply of genes coding for urea uptake and catabolism, whereas Synechococcus PCC 7942 has no such genes, and Synechocystis PCC 6803 and Synechococcus PCC 7002 have no genes for the ureolytic enzymes urea carboxylase and allophanate hydrolase (Veaudor et al., 2019).
So far, only two attempts to manipulate a Cyanothece strain have been reported. First, a single-stranded spectinomycin resistance DNA-cassette, which could be introduced (by electro-transformation) and integrated into the genome of Cyanothece PCC 7822 cells, yielded no spectinomycin resistant clones when tested with Cyanothece PCC 7425 (Min and Sherman, 2010). More recently, Liberton et al. (2019) using DNA methylases to protect the incoming DNA from the Cyanothece ATCC 51142 restriction enzymes, could insert a kanamycin resistance cassette into the glycogen-catabolism gene glgX, generating a glycogen-rich mutant. However, this technique was not tested with Cyanothece PCC 7425. Thus, no gene manipulation system is yet available for Cyanothece PCC 7425, hampering its otherwise interesting potential to serve as a model host.
In this work, we first improved the classical BG-11 mineral medium cyanobacteria (Stanier et al., 1971) for better growth of Cyanothece PCC 7425, which appeared capable to grow not only on nitrate, the usual nitrogen source for cyanobacteria cultivated in the laboratory, but also on urea a frequent pollutant. Then, we developed a simple and efficient protocol for the conjugative transfer to Cyanothece PCC 7425 of the plasmid vectors derived from the broad-host-range plasmid RSF1010 that we previously constructed for gene manipulation in Synechocystis PCC 6803 and Synechococcus PCC 7942 (Marraccini et al., 1993; Mermet-Bouvier and Chauvat, 1994; Mazouni et al., 2004). We showed that these vectors replicate autonomously in Cyanothece PCC 7425 where they can be used for facile (i) promoter analysis, (ii) high-level, constitutive or temperature-controlled, protein productions, and (iii) analysis of sub-cellular localization of proteins. Finally, using this genetic toolbox we engineered a Cyanothece PCC 7425 strain for the stable photosynthetic production of limonene. This high-value terpene serves in cosmetics and food industries (Jongedijk et al., 2016), and it can be used as a fuel additive (Tracy et al., 2009; Chuck and Donnelly, 2014). This is the first report of the engineering of a Cyanothece strain for the photosynthetic production of a chemical, and the first demonstration that a terpene can be produced by an engineered cyanobacterium growing on urea as the sole nitrogen source. This suggests that it could be important in the future to couple chemicals productions with water treatment to reduce the costs (Veaudor et al., 2019).
As Cyanothece PCC 7425 has been poorly studied so far, we first analyzed the influence of usually important parameters, such as light fluence, temperature and mineral availability, on its photoautotrophic growth, Cyanothece PCC 7425 appeared to grow well (Figure 1A) under the conditions defined as standard for Synechocystis PCC 6803, e.g., under white light 2000 lux (25.0 μE.m–2.s–1), at 30°C, in the MM mineral medium (Domain et al., 2004). As Cyanothece PCC 7425 possesses the full panoply of genes encoding the uptake and catabolism of urea that frequently occurs in natural waters (Veaudor et al., 2019), we have tested its capability to grow on urea as the sole nitrogen source. The results showed that cells grew well on urea up to 2 mM, whereas higher urea concentrations reduced the duration of healthy growth and production of biomass (Supplementary Figure S1). After 7–10 days of cultivation on urea, Cyanothece PCC 7425 can turn yellowish, as previously observed in the phylogenetically distant cyanobacteria Anabaena cylindrica, Synechococcus PCC 7002 (Sakamoto et al., 1998) and Synechocystis PCC 6803 growing on urea as the sole nitrogen source (Veaudor et al., 2019). Again as observed in Synechocystis PCC 6803 (Veaudor et al., 2018), once installed the chlorosis process decreased the cell viability measured by plating assays on standard growth medium (it contains nitrate, not urea). Interestingly, Cyanothece PCC 7425 grew up to increasing cell densities in response to increasing urea quantities, which needed to be supplied not all at once, but as small successive sub-doses along cell growth (Supplementary Figure S1). Also interestingly, the maximal growth (biomass production) of Cyanothece PCC 7425 was greatly improved by supplementing the MM with both 9.52 mM NaHCO3 and 2.92 mM CaCl2 (Figure 1A), in agreement with the previous finding that Cyanothece PCC 7425 forms intracellular calcium carbonate granules (De Wever et al., 2019).
Figure 1. Influence of various conditions on the growth of Cyanothece PCC 7425. (A) Typical photoautotrophic growth of Cyanothece PCC 7425 at 30°C, 2000 lux (25.0 μE.m–2.s–1) in liquid mineral medium (MM) or MM supplemented with 9.52 mM NaHCO3 and 2.92 mM CaCl2 (MMCaC). (B) Influence of the addition of vitamin B12 (4 μg.L–1). (C) Influence of various light intensities on cell growth at 30°C in liquid MMCaC. (D) Influence of various temperatures on cell growth in liquid MMCaC under 2000 lux. Error bars represent standard deviation from three biological replicates.
Using the presently improved growth medium, hereafter designated as MMCaC, we found that the growth of Cyanothece PCC 7425 (doubling time about 24 h) is (i) similar under light intensities ranging from 1500 to 3500 lux (18.75–43.75 μE.m–2.s–1) (Figure 1C); (ii) not stimulated by the addition of vitamin B12 that can be beneficial or essential to cyanobacterial life (Figure 1B); and (iii) slightly improved or decreased by increasing the temperature to 34 or 39°C, respectively (Figure 1D).
Three decades ago, we contributed to the development of the genetics of the cyanobacteria Synechocystis PCC 6803 and Synechococcus PCC 7942, in using the broad-host-range plasmid RSF1010 for the construction of pSB2A, the first promoter-probe vector (Marraccini et al., 1993), and pFC1, the first conditional expression vector (Mermet-Bouvier and Chauvat, 1994). These vectors could be transferred by conjugation (or electroporation) from Escherichia coli, to these model cyanobacteria, where they stably replicate autonomously, though they contain no cyanobacterial origin of DNA replication (Mermet-Bouvier et al., 1993). Like RSF1010, pSB2A and pFC1 are not self-transmissible. They must be first introduced (by standard transformation) into an E. coli strain that already contains the self-transmissible RP4 plasmid, which cannot replicate in cyanobacteria, but encodes in trans the functions promoting the transfer of pSB2A or pFC1 to cyanobacteria. The resulting E. coli donor cells possessing both RP4 and pSB2A, or pFC1, are co-incubated with the cyanobacterial recipient cells in liquid medium. Then, the mixture is plated on solid mineral medium for selecting the cyanobacterial conjugants based on their antibiotic resistance encoded by pSB2A or pFC1 (Mermet-Bouvier et al., 1993).
In the present study, the pSB2A and pFC1 plasmids were used to test whether RSF1010-derivatives can be transferred by conjugation from E. coli to Cyanothece PCC 7425, and whether they can stably replicate autonomously in this cyanobacterium as observed in Synechocystis PCC 6803 and Synechococcus PCC 7942 (Mermet-Bouvier et al., 1993). For this purpose, a simpler and faster efficient protocol was developed that bypassed the introduction of pSB2A or pFC1 into the RP4-harboring E. coli strain that normally precedes and enables their conjugative transfer to cyanobacteria. Instead, the cyanobacterial recipient strain was co-incubated, on plate (not in liquid medium) in order to favor cell-cell interaction, with the two E. coli strains, one harboring RP4 and the other one pSB2A or pFC1. Also interestingly, similar high frequencies of conjugation, about 5.10–4 per cyanobacterial cell, were obtained when pSB2A or pFC1 were propagated in commonly used (recA KO) strains of E. coli, such as MC1061, TOP10 or XL1-Blue.
In this section we used pSB2A, the first promoter-probe-vector originally developed for Synechocystis PCC 6803 and Synechococcus PCC 7942 (Marraccini et al., 1993; Dutheil et al., 2012). pSB2A possesses a multiple cloning site for cloning any studied promoter in front of the promoter-less chloramphenicol acetyl transferase (cat) reporter gene. When expressed, for example by the strong E. coli tac promoter we cloned in pSB2A, yielding pSB2T (Supplementary Table S1), cat directs the production of the CAT reporter enzyme. The activity of this enzyme can be easily monitored by a spectrophotometric assay (Ferino and Chauvat, 1989) and confers the resistance to chloramphenicol (Marraccini et al., 1993; Dutheil et al., 2012) and references therein.
The presently reported conjugation protocol was used to introduce the SmR/SpR plasmids pSB2A and pSB2T in Cyanothece PCC 7425. Among the large number of SmR/SpR conjugants obtained, two independent clones were collected, re-streaked on SmR/SpR plates and analyzed by PCR using relevant oligonucleotides primers (Supplementary Table S2). The results showed that pSB2A and pSB2T replicate stably in Cyanothece PCC 7425 (Supplementary Figure S2). Then, we measured the cat activities of the Cyanothece PCC 7425 reporter strains propagating pSB2A or pSB2T. As expected, a strong CAT activity was observed in Cyanothece PCC 7425 cells propagating pSB2T (Figure 2A). In contrast, no CAT activities were detected in Cyanothece PCC 7425 WT cells, which have no cat gene, and pSB2A-propagating cells, where the cat reporter gene is not expressed. Collectively, these results showed that the replicative promoter-probe vector pSB2A can be used for promoter analyses in Cyanothece PCC 7425.
Figure 2. Validation of the pSB2A and pFC1 plasmid vectors for promoter analysis and temperature-controlled protein production in Cyanothece PCC 7425, respectively. (A) Chloramphenicol acyl transferase (CAT) activities of Cyanothece PCC 7425 cells propagating either the promoter probe vector pSB2A, which harbors the promoter-less cat reporter gene, or its pSB2T derivative, which expresses the cat gene from the strong E. coli tac promoter. (B) β-galactosidase activities of Cyanothece PCC 7425 cells propagating either the temperature-controlled expression vector pFC1 or its pMB13 derivative harboring the lacZ protein coding sequence. All activities are the mean values of three measurements performed on two different cellular extracts.
In this section, we used pFC1, the first conditional expression vector for cyanobacteria, originally developed for Synechocystis PCC 6803 and Synechococcus PCC 7942 (Mermet-Bouvier and Chauvat, 1994). pFC1 harbors the lambda-phage cI857 gene encoding the temperature-sensitive repressor that tightly controls the activity of the otherwise strong pR promoter located behind cI857 (in opposite direction) (Mermet-Bouvier and Chauvat, 1994). The pR promoter is followed by a canonical ribosome-binding site (RBS, 5′-AGGA-3′) and a correctly spaced ATG initiation codon embedded within the unique NdeI restriction site (5′-CATATG-3′) for easy cloning and strong conditional expression of the studied protein-coding sequences (Sakr et al., 2013; Ortega-Ramos et al., 2014) and references therein.
The SmR/SpR plasmids pFC1, and its pPMB13 derivative for temperature-controlled expression of the E. coli lacZ gene encoding the beta-galactosidase reporter enzyme (Mermet-Bouvier and Chauvat, 1994), were introduced by conjugation (see above) in Cyanothece PCC 7425. Among the large number of SmR/SpR conjugant clones obtained, two independent clones were collected and re-streaked on SmR/SpR plates, prior to PCR analyses that showed that pFC1 and pPMB13 replicate stably in Cyanothece PCC 7425 (Supplementary Figure S3). Then, we measured the beta-galactosidase activities of the Cyanothece PCC 7425 reporter strains propagating pFC1 or pPMB13. No beta-galactosidase activities were detected in cells propagating pFC1, which lacks lacZ. A weak beta-galactosidase activity was observed in cells propagating pPMB13 grown at 30°C where lacZ expression is mostly blocked by the temperature-sensitive repressor CI857 produced by pPMB13. Finally, the transfer of pPMB13 reporter cells to higher temperatures increased lacZ expression, proportionally to the growth temperature, i.e., moderately at 34°C and massively at 39°C, as expected (Figure 2B). These results showed that the autonomously replicating plasmid vector pFC1 can be used for temperature-regulated protein production in Cyanothece PCC 7425, as was observed in Synechocystis PCC 6803 for many endogenous proteins (Sakr et al., 2013; Ortega-Ramos et al., 2014) and references therein. This system for tight control of (strong) gene expression is very interesting when one wants to produce chemicals that are toxic and prevent cell growth or generates mutations that decrease the production to escape cell death (Cassier-Chauvat et al., 2016). Using such a tightly controlled production system it is possible to first grow the engineered cyanobacterium up to a large cell population, before triggering the production of the toxic product which should thus be more efficient (Cassier-Chauvat et al., 2016).
In a few model cyanobacteria, such as Synechocystis PCC 6803 and Synechococcus PCC 7942, translational fusion of studied proteins to the green-fluorescent reporter protein (GFP) proved useful to analyze the sub-cellular localization of proteins involved in cell division (Cassier-Chauvat and Chauvat, 2014) or the biogenesis of the CO2-fixing carboxysome microcompartment (Cameron et al., 2013).
To test whether the GFP reporter protein can be employed to study protein localization in Cyanothece PCC 7425, we tried to conjugate it with our previously constructed plasmids pSB2T-ftsZ-gfp, pSB2T-gfp-ftn6 and pSB2T-gfp-sepF that produce the Synechocystis PCC 6803 cytokinetic proteins FtsZ, SepF, and Ftn6 translationally fused to GFP (Mazouni et al., 2004; Marbouty et al., 2009). All attempts were unsuccessful, suggesting that these fusion proteins impair the crucial cytokinetic process of Cyanothece PCC 7425.
Consequently, we tried to introduce in Cyanothece PCC 7425 the presently constructed pSB2T-derived plasmids pSB2T-ccmk1tsbp1-gfp and pSB2T-mafS6803-gfp which encode GFP fusions with the presumptive carboxysome protein CcmK1 (Tll0946) of the cyanobacterium Thermosynechococcus elongatus BP1 (Cameron et al., 2013) and the Synechocystis PCC 6803 presumptive cytokinetic protein (Sll0905) Maf (Hamoen, 2011), respectively (Supplementary Figure S2). pSB2T-ccmk1tsbp1-gfp was constructed by cloning downstream of the tac promoter of pSB2T opened at its unique HpaI restriction site, an EcoRV restriction fragment containing the ccmk1tsbp1-gfp fusion gene (synthetized by Genecust; Supplementary Figure S4). Similarly, pSB2T-mafS6803-gfp harbors the mafS6803-gfp fusion gene downstream of its tac promoter (Supplementary Figures S2, S5).
These plasmids, along with the previously constructed pSB2T-GFP plasmid encoding the unfused GFP (Mazouni et al., 2004), were transferred by conjugation to Cyanothece PCC 7425 and also to Synechocystis PCC 6803 as a control strain. Similar high-numbers of conjugant clones were obtained in all cases. PCR analyses showed that all three plasmids appeared to propagate stably in Synechocystis PCC 6803 and Cyanothece PCC 7425 (Supplementary Figure S2). In both hosts, the GFP protein alone displayed no particular localization, as previously observed in Synechocystis PCC 6803 (Mazouni et al., 2004). In contrast, the CcmK1tsbp1-GFP protein appeared to localize inside the cells of both Synechocystis PCC 6803 and Cyanothece PCC 7425 (Figures 3A,B), likely in their carboxysome as observed with the similar CcmK1S7942-GFP fusion protein in Synechococcus PCC 7942 (Cameron et al., 2013). The localization of the MafS6803-GFP fusion protein was also similar in both Synechocystis PCC 6803 and Cyanothece PCC 7425, but this protein was accumulated in one to two spots at the inner periphery of the cells (Figures 3C,D), unlike the CcmK1tsbp1-GFP protein. Collectively these data show that RSF1010-derived plasmids and the fluorescent GFP reporter protein are useful tools to study protein localization in the interesting cyanobacterium Cyanothece PCC 7425 that has larger cells than the models Synechocystis PCC 6803 and Synechococcus PCC 7942 classically used for analyzing the sub-cellular localization of proteins.
Figure 3. Localization of the fusion proteins Ccmk1tsbp1-GFP and MafS6803-GFP in Cyanothece PCC 7425 and Synechocystis PCC 6803. Fluorescence images (scale bars, 500 nm) of Cyanothece PCC 7425 and Synechocystis PCC 6803 reporter cells propagating the plasmids pSB2T-ccmk1tsbp1-gfp (A,B) or pSB2T-mafS6803-gfp (C,D), respectively.
Then we tested whether Cyanothece PCC 7425 can be used for the photosynthetic production of high-value chemicals, such as limonene (C10H16). This volatile terpene, naturally produced by plants, has applications in biofuels (Tracy et al., 2009; Chuck and Donnelly, 2014), bioplastics, cosmetic and pharmaceutical industries (Jongedijk et al., 2016). We employed the limonene synthase from Mentha spicata because it efficiently transforms the geranyl diphosphate metabolite produced by the NAD(P)H-dependent MEP pathway, into limonene of high purity (Colby et al., 1993). Furthermore, this plant enzyme worked well in the model cyanobacteria Synechococcus PCC 7002 (Davies et al., 2014), Synechococcus PCC 7942 (Wang et al., 2016) and Synechocystis PCC 6803 (Lin et al., 2017). Like previous workers, we removed the first 168 bp encoding the chloroplast targeting sequence from the Mentha spicata limonene synthase gene (ls) and we adapted its coding sequence to the cyanobacterial usage of codon. We chose the codon usage of Synechocystis PCC 6803, because it is similar to that of Cyanothece PCC 7425 and proteins efficiently produced in Synechocystis PCC 6803 are also well produced in Cyanothece PCC 7425 (Figures 2, 3). The ls nucleotide sequence was synthesized by the Eurofins company as a DNA segment flanked by NdeI and EcoRI restriction sites at its 5′- and 3′-ends, respectively. After cleavage with both NdeI and EcoRI, the ls gene was cloned downstream of the strong λpR promoter of the pFC1-derivative pC plasmid for high-level constitutive gene expression (Veaudor et al., 2018), which was opened with the same enzymes (Supplementary Figures S6, S7).
The resulting pC-LS plasmid (Supplementary Figure S6) was transferred by conjugation to Cyanothece PCC 7425. Two independent SmR/SpR clones were selected and analyzed by PCR and DNA sequencing (Supplementary Figure S3), which showed that pC-LS replicates stably in Cyanothece PCC 7425. As expected, the presence of the pC-LS plasmid did not adversely affect the photoautotrophic growth of Cyanothece PCC 7425 (Figures 4A,B), irrespectively of the number of sub-cultivation (regular verifications were performed during 12 months). Because of the volatility of limonene, a dodecane overlay was applied on cultures to collect limonene in the organic layer. As observed with Synechococcus PCC 7002 (Davies et al., 2014) and Synechocystis PCC 6803 (Lin et al., 2017), the dodecane overlay had little influence on the growth of Cyanothece PCC 7425 (Figures 4A,B), and the C12 chain length of dodecane (C12H26) allowed chromatographic separation from the C10 length of limonene (C10H16) (Supplementary Figure S8).
Figure 4. Influence of the pC-LS plasmid expressing the limonene synthase gene on the photoautotrophic growth of Cyanothece PCC 7425, in absence or presence of a dodecane overlay. (A) Typical photoautotrophic growth of the Cyanothece PCC 7425 wild-type strain (WT) and its derivative propagating the pC plasmid, at 30°C, 2000 lux (25.0 μE.m–2.s–1) in liquid MMCaC mineral medium, in the presence or absence of a 20% (vol/vol) dodecane overlay. (B) Typical photoautotrophic growth in the same conditions of Cyanothece PCC 7425 strains propagating pC or its pC-LS derivative for high-level constitutive expression of the limonene synthase gene. Error bars represent standard deviation from three biological replicates.
The rate of limonene biosynthesis during the growth phase of the Cyanothece PCC 7425 strains propagating the pC-LS plasmid or the (empty) pC vector, were measured over a 21-days period of cultures with a 20% (v/v) dodecane overlay (Figure 5A). GC-MS analysis of dodecane overlay samples from the pC-LS propagating strain showed a prominent peak with a similar retention time to pure commercial standards of S-(-)-limonene (6.90 min). This peak, which was not observed in the case of the negative control strain propagating the (empty) pC vector, displays a similar pattern than the S-(-)-limonene reference spectra from the NIST Mass Spectral Library (Supplementary Figure S8).
Figure 5. Influence of various growth conditions on the production of limonene by the engineered strain of Cyanothece PCC 7425. Cells growing in MMCaC at 30°C under a 1500 lux light (18.75 μE.m–2.s–1) were inoculated at the initial cell density of 4 × 106 cells mL–1 (OD750 nm = 0.15) and further grown under the indicated conditions. Limonene production was monitored at the indicated time intervals. (A) Cells were grown for 21 days under above indicated conditions in the presence of a 20% (vol/vol) dodecane overlay. (B) Cells were sub-cultured in standard conditions (absence of dodecane) for variable periods (a few weeks to 9 months) prior to assay limonene production as described above during 14 days periods. (C) Cells were grown for 14 days in MMCaC at 30°C under the indicated light intensities: 1500 lux (18.75 μE.m–2.s–1), 2500 lux (31.25 μE.m–2.s–1) or 3500 lux (42 μE.m–2.s–1) in the presence of a 20% (vol/vol) dodecane (dod) overlay prior to test limonene production. (D) Cells were grown at 30°C or 34°C in MMCaC under a 1500 lux light (18.75 μE.m–2.s–1) and a 20% (vol/vol) dodecane overlay. Typical growth (E) and limonene production (F) of cells incubated at 30°C under 1500 lux (18.75 μE.m–2.s–1) in a nitrogen-free MMCaC medium (MMCaC0N), which was supplemented at times 0, 4, and 8 days with the same amounts of nitrogen. They were provided as successive additions of either 2 mM nitrate (NO3; up to 8 mM final) or 1 mM urea [CO(NH2)2; up to 4 mM final]. The dodecane overlay was added either at the beginning of the experiment (dodecane 0 h) or after 72 h of growth. In all cases, error bars represent standard deviation from three biological replicates. The hooks ⊏ indicate a significant difference between the two compared experiments (t-test, P < 0.05; symbolized by *).
The overall limonene production, about 0.6–1.0 mg/L, was unaffected by the duration of sub-cultivation under photoautotrophic conditions (up to 9 months) of the engineered Cyanothece PCC 7425 strain, prior to limonene assay (Figures 5A,B). This test, rarely presented in articles reporting the engineering of cyanobacteria for chemical production (Knoot et al., 2018; Lin and Pakrasi, 2019), showed that the Cyanothece PCC 7425 limonene producer is genetically stable. This finding is interesting since it is known that cyanobacteria engineered for chemical production can be genetically unstable (for a review see Cassier-Chauvat et al., 2016). The level of limonene production by the engineered Cyanothece PCC 7425 strain was slightly increased by either decreasing the light down to 1500 lux or shifting the temperature up to 34°C (Figures 5C,D). In every cases it was similar to what was reported for Synechococcus PCC 7942 (Wang et al., 2016), but lower (about fivefold) than what was reported for the very-well known model cyanobacteria Synechococcus PCC 7002 (Davies et al., 2014) and Synechocystis PCC 6803 (Lin et al., 2017). However, it is very difficult to meaningfully compare limonene production from different cyanobacteria growing under various conditions in different laboratories. Future work will be required to attempt at increasing limonene production by the engineered Cyanothece PCC 7425. This will involve finding better conditions for cell growth and limonene collection, as well as effective metabolic engineering strategies.
As we have shown above, Cyanothece PCC 7425 can grow not only on nitrate, the usual nitrogen source of cyanobacteria, but also on urea, a frequent and abundant pollutant (Veaudor et al., 2019). Growth on urea occurs when it is supplied not all at once, but as small successive sub-doses along cell growth (Supplementary Figure S1). Consequently, we compared the level of limonene production by our engineered strain of Cyanothece PCC 7425 growing either on nitrate or urea added in similar small quantities along cell growth. As compared to cells growing on nitrate, cells growing on urea can be affected by the dodecane overlay used for limonene trapping (Figure 5E). Both cell growth and limonene production are reduced when the dodecane overlay is applied at the beginning of the culture (when the cell density is very low) (Figures 5E,F). The negative influence of dodecane on urea-growing cells is reduced when it is applied after 72 h of growth (when the cell density is higher). Such urea-growing cells produced limonene up to about 50% of the quantity produced by cells growing on nitrate. Future work will be required to find good conditions for the photosynthetic production of limonene by Cyanothece PCC 7425 growing either on urea. This objective is important in the future view of using cyanobacteria for the photosynthetic production of chemicals coupled with water treatment to reduce the costs (Veaudor et al., 2019).
We have developed a genetic toolbox for the robust unicellular cyanobacterium Cyanothece PCC 7425. We first showed that the addition of extra calcium and carbonate to the BG-11 mineral medium, classically used to grow cyanobacteria (Stanier et al., 1971), improved the growth of Cyanothece PCC 7425. Then, we showed that Cyanothece PCC 7425 can grow, not only on nitrate the standard nitrogen source of cyanobacteria, but also on urea a frequent and abundant pollutant (Veaudor et al., 2019). Then, we developed a simple and efficient protocol for the conjugative transfer to Cyanothece PCC 7425 of plasmid vectors derived from the broad-host-range plasmid RSF1010. We previously constructed these vectors for (i) promoter analysis (Marraccini et al., 1993), (ii) constitutive or temperature-controlled protein productions (Mermet-Bouvier and Chauvat, 1994), and (iii) analysis of sub-cellular localization of proteins (Mazouni et al., 2004; Marbouty et al., 2009) in the model cyanobacteria Synechocystis PCC 6803 and Synechococcus PCC 7942. As expected, these autonomously replicating plasmid vectors appeared to work well in Cyanothece PCC 7425.
To emphasize the interest of this genetic toolbox for gene manipulation in Cyanothece PCC 7425, we used it to engineer a strain for the photosynthetic production of the high-value chemical limonene. Limonene, a 10-carbons volatile terpene (C10H16) normally produced by plants, is commercially used in cosmetics and food industries (Jongedijk et al., 2016), and it can be used as an additive to diesel (Tracy et al., 2009) or jet-fuel (Chuck and Donnelly, 2014; Lin et al., 2017). Finally, we have also shown that our engineered strain of Cyanothece PCC 7425 is genetically stable and it can produce limonene in calcium-rich water using either nitrate or urea as the sole nitrogen source. This is the first report of the engineering of a Cyanothece strain for the photosynthetic production of a chemical. It is also the first demonstration that a terpene can be produced by an engineered cyanobacterium growing on urea as the sole nitrogen source. This finding is interesting, because it could be important in the future to couple chemicals productions with wastewater treatment to reduce the costs (Veaudor et al., 2019).
Cyanothece PCC 7425 and Synechocystis PCC 6803 were grown at 30°C, in the MM mineral medium that corresponds to BG-11 (Stanier et al., 1971) enriched with 3.78 mM Na2CO3 (Domain et al., 2004), under continuous agitation (140 rpm, Infors rotary shaker) and cool white light. The intensities were, respectively 1500–2000 lux (18.75–25.00 μE.m–2.s–1) for Cyanothece PCC 7425 and 2500 lux (31.25 μE.m–2.s–1) for Synechocystis PCC 6803. Both of them were also grown on MM solidified with 10% Bacto Agar (Difco). For some experiments performed with Cyanothece PCC 7425, MM was supplemented with 9.52 mM NaHCO3 and 2.92 mM CaCl2 (hereafter designated as MMCaC) and/or nitrate was replaced by urea (1–4 mM), as indicated. In these latter cases, nitrate-grown cells were washed twice with nitrate-free medium before resuspension in urea-containing medium.
Escherichia coli strains MC1061 (Casadaban and Cohen, 1980), TOP10 (Invitrogen) or XL1-Blue (Agilent) were used for gene manipulations and/or conjugative transfer (CM404, Mermet-Bouvier et al., 1993) of RSF1010-derived replicative plasmids to Cyanothece PCC 7425 and Synechocystis PCC 6803 (CM404) (Supplementary Table S1). They were grown on LB medium at 30°C (CM404 and all cells harboring pFC1 derivatives) or 37°C (all other strains). Antibiotic selections were as follows for E. coli: chloramphenicol (Cm) 34 μg.mL–1, kanamycin (Km) 50 μg.mL–1, streptomycin (Sm) 25 μg.mL–1 and spectinomycin (Sp) 75 μg.mL–1; for Cyanothece PCC 7425: Cm 10 μg.mL–1, Km 25 μg.mL–1, Sm 2 μg.mL–1, and Sp 10 μg.mL–1; for Synechocystis PCC 6803: Km 50 μg.mL–1, Sp 5 μg.mL–1, and Sm 5 μg.mL–1.
All plasmids were transferred from E. coli to cyanobacterial cells by trans-conjugation (Mermet-Bouvier et al., 1993), using a simplified and more efficient procedure involving a triparental mating and the co-incubation of E. coli and recipient cyanobacterial cells on solid medium. Five mL overnight-grown cultures of E. coli CM404 harboring the self-transferable mobilization vector (pRK2013, a derivative of RP4, Mermet-Bouvier et al., 1993) and a 5 mL overnight grown culture of E. coli strains harboring one of the studied pSB2A- or pFC1-derivative plasmids were washed twice with LB and resuspended separately into 800 μL LB (final concentration of 1.3 × 109 cells.mL–1). Hundred μL Cyanothece PCC 7425 mid-log phase culture (about 1.25 × 107 cells) were mixed with 30 μL of a CM404 E. coli culture and 30 μL of a culture of an E. coli strain harboring a studied plasmid. Then, 30 μL aliquots of the E. coli and cyanobacterial mixture were spotted onto non-selective MM agar plates that were incubated at 30°C for 72 h under light (1500 lux, 18.75 μE.m–2.s–1). Then, 4 spots were resuspended into 200 μL of fresh MM medium. One 50 μL-aliquot was serially diluted in and plated on fresh MM to calculate the number of Cyanothece PCC 7425 cells (colony-forming units), while the other three 50 μL-aliquots were plated onto MM containing the selective antibiotics and incubated for 10 days under standard conditions to select the conjugant clones, and calculate the frequency of trans-conjugation. A negative control was realized by spreading onto antibiotic-containing MM plates Cyanothece PCC 7425 cells that were not co-incubated with E. coli cells to detect possible spontaneous antibiotic resistant mutant cells. Antibiotics resistant conjugant clones were re-streaked onto selective plates, prior to analyzing their plasmid content through PCR and DNA sequencing (Eurofins Genomics, see Supplementary Table S2 for sequencing primers).
CAT assays were done basically as described for Synechocystis PCC 6803 (Ferino and Chauvat, 1989; Marraccini et al., 1993). Cyanothece PCC 7425 strains harboring the pSB2A or pSB2T plasmids were grown in MMCaC at 30°C under 2000 lux (1 × 108 cells.mL–1) washed twice and resuspended into 2 mL of 50 mM Tris-HCl, pH 8.0. Approximately 2.109 cells were broken in a chilled Eaton press (250 Mpa). Cell extracts were centrifuged (14,000 rpm, 4°C, 10 min) to remove cell debris and were either stored at −20°C until assay or used directly. 1–40 μL of cells extracts mixed with 200 μL of reaction solution (Tris HCl 100 mM pH 8, DTNB 0.4 mg.mL–1 and acetyl CoA 0.1 mM) were loaded into a 96-well plate (Greiner bio-one). Five μL of chloramphenicol at 5 mM was automatically added in each well by a microplate reader (ClarioStar; BMG Labtech). Immediately the absorption at 412 nm of the yellow TNB (5′-thio-2-nitrobenzoic acid) product was measured for 3 min at 30°C. Chloramphenicol acyltransferase activity was defined as the number of nanomoles of chloramphenicol acetylated per minute per mg protein that were quantified by the Bradford assay (Bio-Rad) using bovine serum albumin (0–10 μg BSA) as the standard.
β-galactosidase assays were done basically as described for Synechocystis PCC 6803 (Mermet-Bouvier and Chauvat, 1994). Cyanothece PCC 7425 strains harboring the pFCI or pPMB13 plasmids grown at 30°C in MMCaC under 2000 lux (5 × 107 cells.mL–1) were heat-induced by a fourfold dilution into prewarmed growth medium and then incubated for 168 h at the indicated temperatures (30–39°C). Roughly, 2 × 109 cells were washed, resuspended (in 2 mL Tris-HCl 50 mM pH 8.0), broken and centrifuged as described above. Immediately, 2–10 μL of cells extracts mixed with 200 μL of reaction solution (100 mM Na2HPO4/NaH2PO4 buffer pH 7.5, 50 mM β-mercaptoethanol) were loaded into a clear 96-well plate (Greiner bio-one). Then, 40 μL of ONPG (of o-nitrophenol-galactoside) 4 mg.mL–1 in K2HPO4/KH2PO4 buffer, pH 7.5) were automatically added in each well with a microplate reader (ClarioStar; BMG Labtech). The reaction was immediately followed by measuring the absorption at 420 nm of the yellow ONP (o-nitrophenol) product for 3 min at 30°C. β-galactosidase activity was calculated as the number of micromoles ONPG hydrolyzed per minute per mg proteins, which were quantified by the Bradford assay.
Mid-log phase cultures of Synechocystis PCC 6803 and Cyanothece PCC 7425 cells harboring the pSB2TΔKmR-gfp, pSB2T-ccmk1tsbp1-gfp and pSB2T-mafS6803-gfp plasmids were placed in sandwiches consisting of two glass coverslips (22 mm diameter, Paul Marienfeld GmbH & Co. KG) one of which being coated with a Poly-L-lysine (Sigma-Aldrich) monolayer. These coverslip sandwiches were sealed and placed inside a home-made sample holder, which was mounted on a Nikon Ti-U inverted microscope coupled with an iXon ULTRA 897 CCD camera (Andor Technology), equipped with a 100x oil immersion (NA 1.45) microscope objective. Epifluorescence images were recorded using an excitation provided by a plasma light source (HPLS245 Thorlabs, Inc.) and an excitation filter (MF469-35 Thorlabs, Inc.), while for super-resolution laser scanning images a 488 nm laser (OBIS, Coherent) was used as an excitation source.
Chlorophyll and GFP fluorescence were recorded using ET655LP (Chroma Technology Corporation) and MF525/39 (Thorlabs, Inc.) emission filters, respectively.
Cyanothece PCC 7425 engineered for limonene production was grown photoautotrophically (1500–3500 lux) in 125- or 250-mL erlenmeyers respectively containing 25 or 50 mL cell suspensions overlaid with 20% (vol/vol) dodecane (analytical grade, Sigma-Aldrich) to trap limonene during the whole experimental time course. At time intervals, aliquots of 300 μL were harvested from the dodecane overlay. One μL samples were injected into a GC-MS [Trace1300 (GC) + ISQ LT (MS), ThermoScientific] equipped with a TG-5MS column (30 m × 0.25 mm × 0.25 μm) and operated with H2 carrier gas at 1.0 mL.min–1; ionization voltage 70 eV, split ratio of 25:1; transfer line temperature 250°C; ion source temperature 200°C. The oven program was as followed: 50°C (held for 1 min), 50–150°C (10°C min–1), 150–250°C (20°C min–1), and held for 5 min, with a total program of 21 min. Analysis were carried out in the selected ion monitoring mode: m/z = 50–650.
The limonene peak was identified based on its different ion chromatogram and retention time as compared to those of the α-pinene (Supelco 80599, Sigma-Aldrich) used as an internal standard. A standard curve at m/z = 136 was constructed by GC peak integration of serial dilutions of S-limonene (Supelco 62128, Sigma-Aldrich) pure standard in dodecane (Supplementary Figure S9). The limonene concentration in every dodecane sample was determined using this ratio and by taking-into-account the decrease of the dodecane overlay volume after each sample collection.
All datasets generated for this study are included in the article/Supplementary Material.
FC, CC-C, J-FS, and KB conceived the project. FC, CC-C, CC, ED-S, AG, BR, J-FS, and KB conceived the experiments. CC, ED-S, XK, SG, CI, SS, AG, EM, and D-AB performed the experiments. All authors analyzed the data, read and approved the manuscript. FC, CC-C, and CC drafted the manuscript. FC and CC-C wrote the manuscript. FC agreed to serve as the author responsible for contact and ensures communication.
This work was partly supported by the CEA-DRF PTNTE Greenfuels project, the ANR Harley project (ANR-19-CE44-0017-03), and the platform of Biophysics of I2BC supported by French Infrastructure for Integrated Structural Biology (FRISBI) ANR-10-INBS-05-05. ED-S received a post-doctoral fellowship from the CEA (PTNTE project Greenfuels). CC and SG received a Ph.D. fellowship from the CEA (SL-DRF-17-0106) and the MENESR, respectively.
The authors declare that the research was conducted in the absence of any commercial or financial relationships that could be construed as a potential conflict of interest.
We thank Amélie Goudet for help using GC-MS apparatus.
The Supplementary Material for this article can be found online at: https://www.frontiersin.org/articles/10.3389/fmicb.2020.586601/full#supplementary-material
Bandyopadhyay, A., Elvitigala, T., Welsh, E., Stöckel, J., Liberton, M., Min, H., et al. (2011). Novel metabolic attributes of the genus Cyanothece, comprising a group of unicellular nitrogen-fixing cyanobacteria. mBio 2:e00214-11. doi: 10.1128/mBio.00214-11
Blondeau, M., Sachse, M., Boulogne, C., Gillet, C., Guigner, J. M., Skouri-Panet, F., et al. (2018). Amorphous calcium carbonate granules form within an intracellular compartment in calcifying cyanobacteria. Front. Microbiol. 9:1768. doi: 10.3389/fmicb.2018.01768
Cameron, J. C., Wilson, S. C., Bernstein, S. L., and Kerfeld, C. A. (2013). Biogenesis of a bacterial organelle: the carboxysome assembly pathway. Cell 155, 1131–1140. doi: 10.1016/j.cell.2013.10.044
Casadaban, M. J., and Cohen, S. N. (1980). Analysis of gene control signals by DNA fusion and cloning in Escherichia coli. J. Mol. Biol. 138, 179–207. doi: 10.1016/0022-2836(80)90283-1
Cassier-Chauvat, C., and Chauvat, F. (2014). “Cell division in cyanobacteria,” in The Cell Biology of Cyanobacteria, eds E. Flores and A. Herrero (Norfolk: Caister Academic Press).
Cassier-Chauvat, C., and Chauvat, F. (2018). Cyanobacteria: Wonderful Microorganisms for Basic and Applied Research. Hoboken, NJ: John Wiley & Sons Ltd.
Cassier-Chauvat, C., Dive, V., and Chauvat, F. (2017). Cyanobacteria: photosynthetic factories combining biodiversity, radiation resistance, and genetics to facilitate drug discovery. Appl. Microbiol. Biotechnol. 101, 1359–1364. doi: 10.1007/s00253-017-8105-z
Cassier-Chauvat, C., Veaudor, T., and Chauvat, F. (2016). Comparative genomics of DNA recombination and repair in cyanobacteria: biotechnological implications. Front. Microbiol. 7:1809. doi: 10.3389/fmicb.2016.01809
Chauvat, F., Corre, B., Herdman, M., and Joset-Espardellier, F. (1982). Energetic and metabolic requirements for the germination of akinetes of the cyanobacterium Nostoc PCC 7524. Arch. Microbiol. 133, 44–49. doi: 10.1007/BF00943768
Chuck, C. J., and Donnelly, J. (2014). The compatibility of potential bioderived fuels with Jet A-1 aviation kerosene. Appl. Energy 118, 83–91. doi: 10.1016/j.apenergy.2013.12.019
Colby, S. M., Alonso, W. R., Katahira, E. J., McGarvey, D. J., and Croteau, R. (1993). 4S-limonene synthase from the oil glands of spearmint (Mentha spicata). cDNA isolation, characterization, and bacterial expression of the catalytically active monoterpene cyclase. J. Biol. Chem. 263, 23016–23024.
Dai, W., Chen, M., Myers, C., Ludtke, S. J., Pettitt, B. M., King, J. A., et al. (2018). Visualizing individual RuBisCO and its assembly into carboxysomes in marine cyanobacteria by cryo-electron tomography. J. Mol. Biol. 430, 4156–4167. doi: 10.1016/j.jmb.2018.08.013
Davies, F. K., Work, V. H., Beliaev, A. S., and Posewitz, M. C. (2014). Engineering limonene and bisabolene production in wild type and a glycogen-deficient mutant of Synechococcus sp. PCC 7002. Front. Bioeng. Biotechnol. 2:21. doi: 10.3389/fbioe.2014.00021
De Wever, A., Benzerara, K., Coutaud, M., Caumes, G., Poinsot, M., Skouri-Panet, F., et al. (2019). Evidence of high Ca uptake by cyanobacteria forming intracellular CaCO3 and impact on their growth. Geobiology 17, 676–690. doi: 10.1111/gbi.12358
Demay, J., Bernard, C., Reinhardt, A., and Marie, B. (2019). Natural products from cyanobacteria: focus on beneficial activities. Mar. Drugs 17:320. doi: 10.3390/md17060320
Domain, F., Houot, L., Chauvat, F., and Cassier-Chauvat, C. (2004). Function and regulation of the cyanobacterial genes lexA, recA and ruvB: LexA is critical to the survival of cells facing inorganic carbon starvation. Mol. Microbiol. 53, 65–80. doi: 10.1111/j.1365-2958.2004.04100.x
Ducat, D. C., Way, J. C., and Silver, P. A. (2011). Engineering cyanobacteria to generate high-value products. Trends Biotechnol. 29, 95–103. doi: 10.1016/j.tibtech.2010.12.003
Dutheil, J., Saenkham, P., Sakr, S., Leplat, C., Ortega-Ramos, M., Bottin, H., et al. (2012). The AbrB2 autorepressor, expressed from an atypical promoter, represses the hydrogenase operon to regulate hydrogen production in Synechocystis strain PCC6803. J. Bacteriol. 194, 5423–5433. doi: 10.1128/JB.00543-12
Ferino, F., and Chauvat, F. (1989). A promoter-probe vector-host system for the cyanobacterium, Synechocystis PCC6803. Gene 84, 257–266. doi: 10.1016/0378-1119(89)90499-X
Hamilton, T. L., Bryant, D. A., and Macalady, J. L. (2016). The role of biology in planetary evolution: cyanobacterial primary production in low-oxygen Proterozoic oceans. Environ. Microbiol. 18, 325–340. doi: 10.1111/1462-2920.13118
Hamoen, L. W. (2011). Cell division blockage: but this time by a surprisingly conserved protein. Mol. Microbiol. 81, 1–3. doi: 10.1111/j.1365-2958.2011.07693.x
Herrero, A., Stavans, J., and Flores, E. (2016). The multicellular nature of filamentous heterocyst-forming cyanobacteria. FEMS Microbiol. Rev. 40, 831–854. doi: 10.1093/femsre/fuw029
Houssen, W. E., Koehnke, J., Zollman, D., Vendome, J., Raab, A., Smith, M. C. M., et al. (2012). The discovery of new cyanobactins from Cyanothece PCC 7425 defines a new signature for processing of patellamides. ChemBioChem 13, 2683–2689. doi: 10.1002/cbic.201200661
Jansson, C., and Northen, T. (2010). Calcifying cyanobacteria-the potential of biomineralization for carbon capture and storage. Curr. Opin. Biotechnol. 21, 365–371. doi: 10.1016/j.copbio.2010.03.017
Jongedijk, E., Cankar, K., Buchhaupt, M., Schrader, J., Bouwmeester, H., and Beekwilder, J. (2016). Biotechnological production of limonene in microorganisms. Appl. Microbiol. Biotechnol. 100, 2927–2938. doi: 10.1007/s00253-016-7337-7
Klemke, F., Nürnberg, D. J., Ziegler, K., Beyer, G., Kahmann, U., Lockau, W., et al. (2016). CphA2 is a novel type of cyanophycin synthetase in N2-fixing cyanobacteria. Microbiology 162, 526–536. doi: 10.1099/mic.0.000241
Knoot, C. J., Ungerer, J., Wangikar, P. P., and Pakrasi, H. B. (2018). Cyanobacteria: Promising biocatalysts for sustainable chemical production. J. Biol. Chem. 293, 5044–5052. doi: 10.1074/jbc.R117.815886
Koksharova, O. A., and Wolk, C. P. (2002). A novel gene that bears a DnaJ motif influences cyanobacterial cell division. J. Bacteriol. 184, 5524–5528. doi: 10.1128/JB.184.19.5524-5528.2002
Legrand, B., Miras, Y., Beauger, A., Dussauze, M., and Latour, D. (2019). Akinetes and ancient DNA reveal toxic cyanobacterial recurrences and their potential for resurrection in a 6700-year-old core from a eutrophic lake. Sci. Total Environ. 687, 1369–1380. doi: 10.1016/j.scitotenv.2019.07.100
Liberton, M., Bandyopadhyay, A., and Pakrasi, H. B. (2019). Enhanced nitrogen fixation in a glgX-deficient strain of Cyanothece sp. strain ATCC 51142, a unicellular nitrogen-fixing cyanobacterium. Appl. Environ. Microbiol. 85:e02887-18. doi: 10.1128/AEM.02887-18
Lin, P. C., and Pakrasi, H. B. (2019). Engineering cyanobacteria for production of terpenoids. Planta 249, 145–154. doi: 10.1007/s00425-018-3047-y
Lin, P.-C., Saha, R., Zhang, F., and Pakrasi, H. B. (2017). Metabolic engineering of the pentose phosphate pathway for enhanced limonene production in the cyanobacterium Synechocystis sp. PCC 6803. Sci. Rep. 7:17503. doi: 10.1038/s41598-017-17831-y
MacCready, J. S., Basalla, J. L., and Vecchiarelli, A. G. (2020). Origin and evolution of carboxysome positioning systems in cyanobacteria. Mol. Biol. Evol. 37, 1434–1451. doi: 10.1093/molbev/msz308
Marbouty, M., Saguez, C., Cassier-Chauvat, C., and Chauvat, F. (2009). Characterization of the FtsZ-interacting septal proteins SepF and Ftn6 in the spherical-celled cyanobacterium Synechocystis strain PCC 6803. J. Bacteriol. 191, 6178–6185. doi: 10.1128/JB.00723-09
Marraccini, P., Bulteau, S., Cassier-Chauvat, C., Mermet-Bouvier, P., and Chauvat, F. (1993). A conjugative plasmid vector for promoter analysis in several cyanobacteria of the genera Synechococcus and Synechocystis. Plant Mol. Biol. 23, 905–909. doi: 10.1007/BF00021546
Marteyn, B., Domain, F., Legrain, P., Chauvat, F., and Cassier-Chauvat, C. (2009). The thioredoxin reductase-glutaredoxins-ferredoxin crossroad pathway for selenate tolerance in Synechocystis PCC6803. Mol. Microbiol. 71, 520–532. doi: 10.1111/j.1365-2958.2008.06550.x
Mazouni, K., Domain, F., Cassier-Chauvat, C., and Chauvat, F. (2004). Molecular analysis of the key cytokinetic components of cyanobacteria: FtsZ, ZipN and MinCDE. Mol. Microbiol. 52, 1145–1158. doi: 10.1111/j.1365-2958.2004.04042.x
Mermet-Bouvier, P., Cassier-Chauvat, C., Marraccini, P., and Chauvat, F. (1993). Transfer and replication of RSF1010-derived plasmids in several cyanobacteria of the genera Synechocystis and Synechococcus. Curr. Microbiol. 27, 323–327. doi: 10.1007/BF01568955
Mermet-Bouvier, P., and Chauvat, F. (1994). A conditional expression vector for the cyanobacteria Synechocystis sp. strains PCC6803 and PCC6714 or Synechococcus sp. strains PCC7942 and PCC6301. Curr. Microbiol. 28, 145–148. doi: 10.1007/BF01571055
Min, H., and Sherman, L. A. (2010). Genetic transformation and mutagenesis via single-stranded DNA in the unicellular, diazotrophic cyanobacteria of the genus Cyanothece. Appl. Environ. Microbiol. 16, 7641–7645. doi: 10.1128/AEM.01456-10
Montgomery, B. L. (2015). Light-dependent governance of cell shape dimensions in cyanobacteria. Front. Microbiol. 6:514. doi: 10.3389/fmicb.2015.00514
Narainsamy, K., Marteyn, B., Sakr, S., Cassier-Chauvat, C., and Chauvat, F. (2013). “Genomics of the Pleïotropic Glutathione System in Cyanobacteria,” in Advances in Botanical Research, ed. J. A. Callow (Amsterdam: Elsevier), 157–188. doi: 10.1016/b978-0-12-394313-2.00005-6
Ortega-Ramos, M., Jittawuttipoka, T., Saenkham, P., Czarnecka-Kwasiborski, A., Bottin, H., Cassier-Chauvat, C., et al. (2014). Engineering Synechocystis PCC6803 for hydrogen production: influence on the tolerance to oxidative and sugar stresses. PLoS One 9:e0089372. doi: 10.1371/journal.pone.0089372
Ponce-Toledo, R. I., Deschamps, P., López-García, P., Zivanovic, Y., Benzerara, K., and Moreira, D. (2017). An early-branching freshwater cyanobacterium at the origin of plastids. Curr. Biol. 27, 386–391. doi: 10.1016/j.cub.2016.11.056
Porta, D., Rippka, R., and Hernández-Mariné, M. (2000). Unusual ultrastructural features in three strains of Cyanothece (cyanobacteria). Arch. Microbiol. 173, 154–163. doi: 10.1007/s002039900126
Rippka, R., Deruelles, J., Waterbury, J. B., Herdman, M., and Stanier, R. Y. (1979). Generic assignments, strain histories and properties of pure cultures of cyano- bacteria. J. Gen. Microbiol. 11, 1–61. doi: 10.1099/00221287-111-1-1
Sakamoto, T., Delgaizo, V. B., and Bryant, D. A. (1998). Growth on urea can trigger death and peroxidation of the cyanobacterium Synechococcus sp. strain PCC 7002. Appl. Environ. Microbiol. 64, 2361–2366. doi: 10.1128/AEM.64.7.2361-2366.1998
Sakr, S., Dutheil, J., Saenkham, P., Bottin, H., Leplat, C., Ortega-Ramos, M., et al. (2013). The activity of the Synechocystis PCC6803 AbrB2 regulator of hydrogen production can be post-translationally controlled through glutathionylation. Int. J. Hydrogen Energy 38, 13547–13555. doi: 10.1016/j.ijhydene.2013.07.124
Schirrmeister, B. E., Gugger, M., and Donoghue, P. C. J. (2015). Cyanobacteria and the great oxidation event: evidence from genes and fossils. Palaeontology 58, 769–785. doi: 10.1111/pala.12178
Singh, J. S., Kumar, A., Rai, A. N., and Singh, D. P. (2016). Cyanobacteria: a precious bio-resource in agriculture, ecosystem, and environmental sustainability. Front. Microbiol. 7:529. doi: 10.3389/fmicb.2016.00529
Sommer, M., Sutter, M., Gupta, S., Kirst, H., Turmo, A., Lechno-Yossef, S., et al. (2019). Heterohexamers formed by CcmK3 and CcmK4 increase the complexity of beta carboxysome shells. Plant Physiol. 179, 156–167. doi: 10.1104/pp.18.01190
Stanier, R. Y., Kunisawa, R., Mandel, M., and Cohen-Bazire, G. (1971). Purification and properties of unicellular blue-green algae (order Chroococcales). Bacteriol. Rev. 35, 171–205. doi: 10.1128/mmbr.35.2.171-205.1971
Sun, Y., Wollman, A. J. M., Huang, F., Leake, M. C., and Liu, L. N. (2019). Single-organelle quantification reveals stoichiometric and structural variability of carboxysomes dependent on the environment. Plant Cell 31, 1648–1664. doi: 10.1105/tpc.18.00787
Tracy, N. I., Chen, D., Crunkleton, D. W., and Price, G. L. (2009). Hydrogenated monoterpenes as diesel fuel additives. Fuel 88, 2238–2240. doi: 10.1016/j.fuel.2009.02.002
Veaudor, T., Cassier-Chauvat, C., and Chauvat, F. (2018). Overproduction of the cyanobacterial hydrogenase and selection of a mutant thriving on urea, as a possible step towards the future production of hydrogen coupled with water treatment. PLoS One 13:e0198836. doi: 10.1371/journal.pone.0198836
Veaudor, T., Cassier-Chauvat, C., and Chauvat, F. (2019). Genomics of urea transport and catabolism in cyanobacteria: biotechnological implications. Front. Microbiol. 10:2052. doi: 10.3389/fmicb.2019.02052
Keywords: conjugation, RSF1010 derivative plasmids, promoter probe vector, temperature-controlled expression vector, sub-cellular localization, growth on urea, terpene production
Citation: Chenebault C, Diaz-Santos E, Kammerscheit X, Görgen S, Ilioaia C, Streckaite S, Gall A, Robert B, Marcon E, Buisson D-A, Benzerara K, Sassi J-F, Cassier-Chauvat C and Chauvat F (2020) A Genetic Toolbox for the New Model Cyanobacterium Cyanothece PCC 7425: A Case Study for the Photosynthetic Production of Limonene. Front. Microbiol. 11:586601. doi: 10.3389/fmicb.2020.586601
Received: 23 July 2020; Accepted: 31 August 2020;
Published: 18 September 2020.
Edited by:
Guodong Luan, Qingdao Institute of Bioenergy and Bioprocess Technology (CAS), ChinaCopyright © 2020 Chenebault, Diaz-Santos, Kammerscheit, Görgen, Ilioaia, Streckaite, Gall, Robert, Marcon, Buisson, Benzerara, Sassi, Cassier-Chauvat and Chauvat. This is an open-access article distributed under the terms of the Creative Commons Attribution License (CC BY). The use, distribution or reproduction in other forums is permitted, provided the original author(s) and the copyright owner(s) are credited and that the original publication in this journal is cited, in accordance with accepted academic practice. No use, distribution or reproduction is permitted which does not comply with these terms.
*Correspondence: Corinne Cassier-Chauvat, Y29yaW5uZS5jYXNzaWVyLWNoYXV2YXRAY2VhLmZy; Franck Chauvat, ZnJhbmNrLmNoYXV2YXRAY2VhLmZy
Disclaimer: All claims expressed in this article are solely those of the authors and do not necessarily represent those of their affiliated organizations, or those of the publisher, the editors and the reviewers. Any product that may be evaluated in this article or claim that may be made by its manufacturer is not guaranteed or endorsed by the publisher.
Research integrity at Frontiers
Learn more about the work of our research integrity team to safeguard the quality of each article we publish.