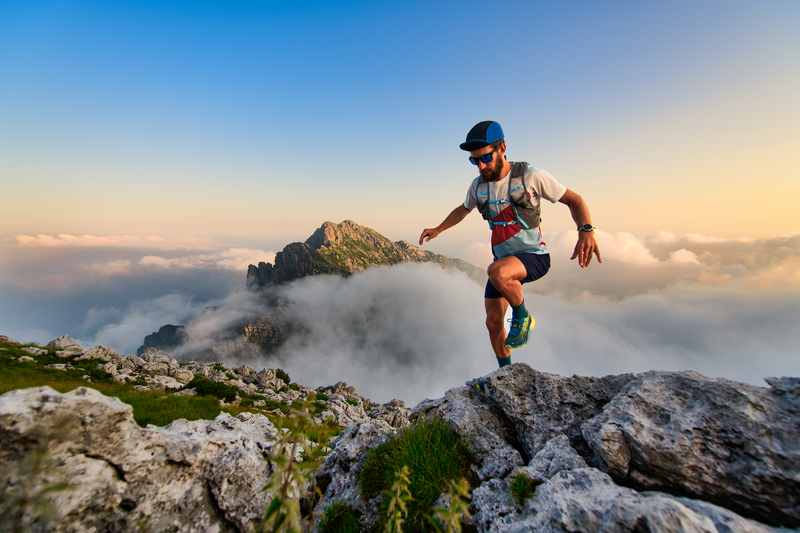
95% of researchers rate our articles as excellent or good
Learn more about the work of our research integrity team to safeguard the quality of each article we publish.
Find out more
MINI REVIEW article
Front. Microbiol. , 27 October 2020
Sec. Microbial Immunology
Volume 11 - 2020 | https://doi.org/10.3389/fmicb.2020.575586
Most antibiotics are produced by soil microbes and typically interfere with macromolecular synthesis processes as their antibacterial mechanism of action. These natural products are often large and suffer from poor chemical tractability. Here, we discuss discovery, mechanism of action, and the therapeutic potentials of an unusual antibiotic, indole propionic acid (IPA). IPA is produced by the human gut microbiota. The molecule is small, chemically tractable, and targets amino acid biosynthesis. IPA is active against a broad spectrum of mycobacteria, including drug resistant Mycobacterium tuberculosis and non-tuberculous mycobacteria (NTM). Interestingly, the microbiota-produced metabolite is detectable in the serum of healthy individuals, tuberculosis (TB) patients, and several animal models. Thus, the microbiota in our gut may influence susceptibility to mycobacterial diseases. If a gut-lung microbiome axis can be demonstrated, IPA may have potential as a biomarker of disease progression, and development of microbiota-based therapies could be explored. In addition to its antimycobacterial activity, the molecule displays anti-inflammatory and antioxidant properties. This raises the possibility that IPA has therapeutic potential as both antibiotic and add-on host-directed drug for the treatment of TB in patient populations where disease morbidity and mortality is driven by excessive inflammation and tissue damage, such as TB-associated immune reconstitution inflammatory syndrome, TB-meningitis, and TB-diabetes.
To identify chemical starting points for the discovery of new drugs against resistant tuberculosis (TB) and lung disease caused by non-tuberculous mycobacteria (NTM), we recently screened a library of rule-of-3 (R03) compliant compounds for whole cell actives (Negatu et al., 2018). R03 compliant compounds are “fragment”-sized (i.e., molecular weight <300 g/mol), have a cLogP of ≤3, and the number of hydrogen bond donors and acceptors is ≤3 (Jhoti et al., 2013). Due to their small size and the resulting limited molecular interaction surface, R03 compliant compounds are expected to show poor on-target activity. However, R03 compounds constitute attractive starting points for lead optimization (Jhoti et al., 2013; Gopal and Dick, 2014). Typically, R03 libraries are employed for structure-based drug discovery approaches in which hits are either grown or connected to generate high affinity binders (Jhoti et al., 2013). It is interesting to note that several anti-TB drugs, such as pyrazinamide and isoniazid, are fragment-sized drugs (Jhoti et al., 2013; Riccardi and Pasca, 2014). The physicochemical properties associated with their size makes these small drugs effective penetrators of lung lesions associated with mycobacterial diseases. Thus, fragment-sized TB drugs effectively reach all mycobacterial populations sequestered in various lesion compartments (Prideaux et al., 2015). This lesion-pharmacokinetic property contributes to the remarkable clinical efficacy of pyrazinamide, despite the drug’s moderate in vitro potency (Dartois, 2014; Prideaux et al., 2015).
Screening the R03 library resulted in the identification of indole propionic acid (IPA) as a new antimycobacterial (Negatu et al., 2018). The compound showed anti-TB (including multi-drug resistant Mycobacterium tuberculosis) and anti-NTM (including Mycobacterium avium) activity in vitro. Using a mouse model of TB infection, we demonstrated in vivo efficacy (Negatu et al., 2018). IPA showed no activity against Gram-negative or -positive bacteria, and thus appears to display selective but broad spectrum antimycobacterial activity (Negatu et al., 2018, 2019).
Interestingly, IPA is a metabolite produced by gut bacteria (Dodd et al., 2017). Recently, several IPA producing gut-dwelling clostridia and Peptostreptococcus anaerobius were identified, and the biosynthetic pathway of IPA production from tryptophan was elucidated (Dodd et al., 2017). Thus, IPA is a microbial metabolite that inhibits growth of other microbes and therefore represents an antibiotic in the classical sense (Negatu et al., 2018). Our clinical antibacterial arsenal is composed to a large extent of natural products or their semi-synthetic derivatives. These antibiotics are mostly derived from soil bacteria, often Streptomyces (Clardy et al., 2009; Genilloud, 2017). They are usually large and of complex chemistry, with the associated pharmacokinetic and synthetic chemistry issues, presenting many challenges for medicinal chemists (Leeson and Springthorpe, 2007). IPA is an unusual antibiotic as it is produced by gut bacteria rather than soil bacteria. Furthermore, IPA is small, and the indole-based scaffold is chemically tractable.
In vitro potency and in vivo efficacy of IPA are moderate (Negatu et al., 2018). Its mechanism of action was determined by our group to enable rational, target-based optimization. IPA is a deamination analog of tryptophan, and thus structurally similar to this aromatic amino acid. Tryptophan biosynthesis is an essential pathway in mycobacteria in vitro as well as in vivo (Zhang et al., 2013; Wellington et al., 2017). The pathway is regulated via a negative feedback loop in which the end product tryptophan acts as allosteric inhibitor of the first committed enzymatic step of the pathway catalyzed by anthranilate synthase TrpE (Bashiri et al., 2015). Thus, we hypothesized that IPA may mimic tryptophan as allosteric inhibitor and block tryptophan synthesis. Through structural modeling followed by metabolic, genetic, and biochemical analyses, we demonstrated that IPA indeed blocks tryptophan biosynthesis by binding to the allosteric tryptophan binding site of TrpE, shutting down the enzyme’s activity (Figure 1; Negatu et al., 2019). Thus, IPA acts by decoupling a bacterial regulatory feedback mechanism. The antibiotic mimics tryptophan as allosteric inhibitor, thereby switching off production of this aromatic amino acid regardless of intracellular tryptophan levels. The identification of the molecular target of IPA provides the platform for rational chemical optimization in which semisynthetic analogs with increased potency can be designed to develop a new class of broad-spectrum antimycobacterials.
Figure 1. Possible link between gut microbiota and mycobacterial lung disease. Left: indole propionic acid (IPA) is produced by gut bacteria from tryptophan and enters the blood stream. Right: IPA inhibits mycobacterial tryptophan biosynthesis by targeting anthranilate synthase TrpE, mimicking tryptophan as allosteric inhibitor. Mtb, Mycobacterium tuberculosis.
Blocking an amino acid biosynthetic pathway is a novel antibiotic mechanism. Most antibiotics, including synthetic antibacterials, target macromolecular synthesis processes such as protein (e.g., streptomycin), RNA (e.g., rifamycins), or peptidoglycan synthesis (e.g., beta-lactams; Clardy et al., 2009). Only few synthetic antibacterials interfere with bacterial metabolism. A classic example is trimethoprim, a dihydrofolate reductase inhibitor, blocking folate biosynthesis (Hitchings, 1973). A recent example is pyrazinamide, an aspartate decarboxylase degrader, blocking coenzyme A biosynthesis in M. tuberculosis (Gopal et al., 2017, 2020).
IPA is well-known as a metabolite produced by human gut bacteria (Young et al., 1980). It can be detected systemically in blood, and thus reaches all major organs after migrating from the gut to the bloodstream (Young et al., 1980; Wikoff et al., 2009). Recent observations suggest that the gut microbiota may affect TB progression by modulating the host immune response (Wood et al., 2017; Naidoo et al., 2019). The discovery of IPA’s antimycobacterial activity may provide the missing functional link between gut bacteria and mycobacterial lung disease (Figure 1). Analyses of serum of healthy volunteers show large (up to 1,000-fold) person-to-person variability (Wikoff et al., 2009; unpublished observations of the authors). IPA can be detected in the serum of animal models, which are used to study mycobacterial lung disease, such as mice and rabbits (Wikoff et al., 2009; Kennedy et al., 2018; unpublished observations by the authors). Hence, we have the models available to study a possible IPA-related microbiota effect on mycobacterial lung diseases. Furthermore, studies can be carried out with human cohorts to determine whether there is a correlation between disease susceptibility or disease progression and IPA levels in the serum. If these studies do show correlations, IPA may be useful as biomarker (Naidoo et al., 2019).
These studies may open avenues for the development of microbiota-based therapies (Reardon, 2014; Mimee et al., 2016; Suez and Elinav, 2017) using IPA producing strains as probiotics, possibly supplemented with high content tryptophan (the substrate for IPA production; Dodd et al., 2017) foods. In addition to using natural IPA producers, generation of recombinant probiotics could be considered by incorporating the gene cluster that converts tryptophan to IPA (Mathipa and Thantsha, 2017). Increased levels of endogenously produced IPA may complement standard, exogenously supplied antimycobacterials.
In addition to targeting the pathogen directly via inhibiting tryptophan biosynthesis, increased IPA levels may also act indirectly on the disease via host directed mechanism, as discussed in the next sections.
Before the discovery of IPA’s antibiotic activity, the molecule and other tryptophan metabolites were shown to have immune modulatory properties (Gao et al., 2018; Nicolas and Chang, 2019). Tryptophan metabolites are potent inducers of the Aryl hydrocarbon Receptor (AhR), a central player in the regulation of both innate and adaptive immune responses (Stockinger et al., 2014; Gao et al., 2018; Gutierrez-Vazquez and Quintana, 2018; Nicolas and Chang, 2019). AhR is a ligand-activated transcription factor localized in the cytoplasm of human cells in complex with other proteins (Figure 2A; Gutierrez-Vazquez and Quintana, 2018). Upon activation by tryptophan metabolites, AhR translocates into the cell nucleus and binds its partner, the AhR nuclear translocator (ARNT), to regulate expression of various immune response genes (Gutierrez-Vazquez and Quintana, 2018; Roager and Licht, 2018). These include genes involved in the suppression of hyper-inflammation, including Type I interferons, transforming growth factor β, and interleukin 10 (Figure 2A; Benson and Shepherd, 2011; Rothhammer et al., 2016; Yisireyili et al., 2017). Excess inflammation is one of the key factors contributing to pathogenesis of TB (Kaufmann and Dorhoi, 2013; Roca and Ramakrishnan, 2013). Thus, IPA could be explored as host-directed therapy to treat mycobacterial diseases (Kroesen et al., 2017; Palucci and Delogu, 2018). Importantly, activated AhR enhances the production of the interleukin 22, which stimulates the production of antimicrobial peptides and is also involved in inhibiting intra-macrophage growth of mycobacteria through increasing phagolysosomal fusion (Dhiman et al., 2009; Zelante et al., 2013; Memari et al., 2015; Shen and Chen, 2018). In addition, this cytokine stimulates the production of Th17 T cells, which produce interleukin 17, a protective cytokine against mycobacterial infection (Umemura et al., 2007; Scriba et al., 2008; Veldhoen et al., 2008; Shen and Chen, 2018). In line with this, double knock-out AhR−/− mice are hyper-susceptible to M. tuberculosis, confirming the involvement of AhR in the control of mycobacterial infections (Moura-Alves et al., 2014). Recently, it has been shown that several anti-TB drugs bind to AhR, altering host defense mechanisms such as phagocytosis, and influencing the TB treatment response in a mouse model (Puyskens et al., 2019). These observations suggest that IPA may have potential in controlling mycobacterial disease by dampening excess inflammation and enhancing killing of mycobacteria. In the next section, we discuss three mycobacterial diseases, TB-meningitis, TB-HIV, and TB-diabetes for which the IPA treatment may be of relevance.
Figure 2. Aryl hydrocarbon receptor (AhR) signaling pathway and tryptophan-indoleamine 2,3-dioxygenase (IDO)-AhR axis. (A) AhR (red) is localized in the cytosol of human cells in complex with other proteins. Upon activation by ligands (e.g., tryptophan metabolites), AhR is released from the complex and binds its partner, the AhR nuclear translocator (ARNT) in the nucleus. The complex of AhR and ARNT binds upstream of target genes and enhances the expression of anti-inflammatory (green) and “antimycobacterial” cytokines (orange). AIP, AhR-interacting protein; p23, chaperone; HSP90, 90kDa heat shock protein. (B) Tryptophan-IDO-AhR axis in inflammation.
TB-meningitis, an inflammation of meninges resulting from M. tuberculosis infection of the brain and spinal cord, mainly affects children and immuno-compromised patients (Wilkinson et al., 2017). It is a devastating disease with limited treatment options and unfavorable treatment outcomes (Chiang et al., 2014; Graham and Donald, 2014; Davis et al., 2018; Nguyen et al., 2019). Neurological damage is the most relevant sequelae of TB-meningitis (Thwaites et al., 2000; Rock et al., 2008; Chiang et al., 2014). Inflammation-mediated damage has been recognized as the major contributor to the irreversible neurological pathology as well as high mortality (Thwaites et al., 2004; Rock et al., 2008; Be et al., 2009; Thuong and Thwaites, 2017; Rohlwink et al., 2019). Hence, administration of anti-inflammatory drugs, usually corticosteroids, along with antimycobacterials is common practice (Thwaites et al., 2004; Prasad et al., 2016; Schutz et al., 2018). IPA is not only detectable in human serum but also in cerebrospinal fluid, showing its ability to cross the brain-blood barrier (Young et al., 1980). Interestingly, IPA has been shown to have neuroprotective effects (Chyan et al., 1999; Bendheim et al., 2002; Hwang et al., 2009) and is in early clinical development under the name of VP20629 for Friedreich’s ataxia, a neurodegenerative disease.1 Similarly, tryptophan metabolites prevented Aβ peptide-induced neurodegeneration via AhR activation (Platten et al., 2019; Maitre et al., 2020). An observational cohort study identified an association between low tryptophan concentration in cerebrospinal fluid and survival of TB-meningitis patients (van Laarhoven et al., 2018). Tryptophan is known to be catabolized by indoleamine 2,3-dioxygenase (IDO) upon inflammation (Kueck et al., 2018) as well as in response to tuberculosis infection (Gautam et al., 2018). The major product of this conversion, kynurenine, is an anti-inflammatory metabolite known to suppress central nervous system (CNS) inflammation via AhR activation (Cervenka et al., 2017; Crunkhorn, 2018; Figure 2B), consistent with the association of low tryptophan in the cerebrospinal fluid with improved survival (van Laarhoven et al., 2018). Likewise, it has been shown that IPA activates AhR and suppresses CNS inflammation (Rothhammer et al., 2016), and thus may also be beneficial in TB-meningitis, where mortality is driven by immunopathology (Graham and Donald, 2014; Davis et al., 2019).
High levels of the antioxidant glutathione have also been associated with the positive treatment outcomes among patients with TB-meningitis (Kalita et al., 2019). In this context, it is interesting to note that IPA is not only an antibiotic and an immune-modulator, but also a potent antioxidant that detoxifies reactive oxygen species, such as hydroxyl radicals (Karbownik et al., 2001, 2006; Hwang et al., 2009). IPA does not undergo autoxidation in the presence of transition metals making the molecule superior to most naturally occurring and synthetic antioxidants (Chyan et al., 1999). Thus, IPA could have additional positive effects on treatment outcome and irreversible neurological damage of TB-meningitis via its antioxidant properties (Wilkinson et al., 2017; Jain et al., 2018). Considering IPA’s brain pharmacokinetic, antimycobacterial, anti-inflammatory, and antioxidant properties, the molecule may find utility in the management of TB-meningitis (Wilkinson et al., 2017; Davis et al., 2018; Jain et al., 2018). Animal models are available to explore this therapeutic option (Tsenova et al., 2006; Tucker et al., 2016; Zhan et al., 2017).
TB-HIV is a dominant co-morbidity that accounted for 251,000 deaths in 2018 (WHO, 2019). Tuberculosis-associated immune reconstitution inflammatory syndrome (TB-IRIS) is an excessive inflammatory response among TB patients initiating anti-retroviral therapy (ART) leading to T-cell restoration (Lai et al., 2015). The condition is associated with increased proinflammatory cytokines, such as interferon γ and TNF-α (Lai et al., 2015; Walker et al., 2018). In a randomized control trial, the anti-inflammatory drug prednisone reduced the incidence of tuberculosis-associated IRIS (Meintjes et al., 2018). In a recent multi-cohort study, increased catabolism of tryptophan to kynurenine was associated with active TB compared to healthy subjects, and gradually reverted to baseline during the course of successful treatment (Collins et al., 2020). Increased kynurenine/tryptophan was associated with significant increases in IDO transcripts, suggesting that tryptophan catabolism is mediated by induction of IDO. The authors suggested IDO mediated tryptophan catabolism as a biomarker of TB disease progression as well as host-directed therapy (Collins et al., 2020). Accordingly, the early treatment with corticosteroids was effective in reducing the incidence of TB-IRIS and symptom severity (Sereti, 2020), partly due to increased IDO expression (Maneechotesuwan et al., 2008). Whereas the anti-inflammatory activity of IDO may hamper immune control of M. tuberculosis replication due to decreased proliferation of M. tuberculosis antigen-specific T cells (Mehra et al., 2013; Gautam et al., 2018), it may also be beneficial under conditions where mortality is driven by excessive inflammation, such as TB-IRIS. Since IPA phenocopies the anti-inflammatory effect of kynurenine via AhR (Rothhammer et al., 2016) and exhibits antimycobacterial properties, IPA could modulate the Trp-IDO-AhR axis to influence infection outcome to the benefit of the host in TB-IRIS management (Figure 2B).
TB-diabetes co-morbidity is one of the major challenges in the management of TB, particularly considering the fast-growing incidence of diabetes in developing countries and the huge burden of TB (Dooley and Chaisson, 2009; Jiménez-Corona et al., 2013; Zheng et al., 2017). In a series of reports, IPA has been suggested to play a protective role against Type II diabetes, the major type of diabetes associated with TB (de Mello et al., 2017; Abildgaard et al., 2018; Tuomainen et al., 2018). The likelihood of developing diabetes has been shown to be reduced among individuals who have higher levels of IPA in their blood (de Mello et al., 2017). IPA has also been shown to reduce fasting glucose levels in rats (Abildgaard et al., 2018). Furthermore, a positive correlation of dietary fiber intake, insulin secretion, and IPA concentration in the blood was found, while IPA levels were negatively associated with inflammation markers (Kumar et al., 2013; Tuomainen et al., 2018). Consistent with these findings, the proportion of some of the IPA-producing gut bacteria is reduced among diabetes Type II patients (Larsen et al., 2010; Aw and Fukuda, 2018). Interestingly, a meta-analysis showed that the antihyperglycemic drug metformin, which also displays anti-inflammatory properties, has benefits in both prevention and treatment outcomes of TB patients with diabetes (Cameron et al., 2016; Zhang and He, 2020). Paradoxically, serum AhR ligand activity was higher in subjects with Type II diabetes, correlated with parameters of insulin resistance, and was a risk factor for diabetic nephropathy (Kim et al., 2013; Roh et al., 2015; Lee et al., 2020). The authors attributed AhR ligand activity to persistent organic pollutants such as tetrachlorodibenzo-para-dioxin, a dioxin with the highest known binding affinity to AhR (Mandal, 2005), although a causal relationship was not established. It has been proposed that activation of AhR by organic pollutants influences glucose tolerance via regulation of PPAR-α (Wang et al., 2011). Whether IPA-mediated AhR signaling influences glucose metabolism via the same pathways as environmental toxicants remain to be determined. Animal models are available to explore the antimycobacterial, anti-inflammatory, and anti-diabetic effects of IPA, and its potential to improve the clinical management of TB-diabetes (Martens et al., 2007; Srinivasan and Ramarao, 2007; Vallerskog et al., 2010).
The discovery that the gut microbiota metabolite IPA has antimycobacterial, anti-inflammatory, and antioxidant activity has a multitude of implications. In the context of drug discovery, this molecule presents an attractive chemical starting point for medicinal chemistry campaigns to generate more potent semi-synthetic IPA analogs, paving the way for the development of a novel broad spectrum antimycobacterial for the treatment of both TB and NTM lung disease. In the context of human microbiome and disease, IPA may present a functional link between the gut microbiota and host susceptibility to mycobacterial diseases. If confirmed, circulating IPA levels could turn out to be a useful prognostic marker and microbiota-based treatment strategies could be explored. Further analyses of IPAs immune modulatory and antioxidant effects in mycobacterial diseases may reveal adjunctive host-directed therapy opportunities, particularly in TB-meningitis and TB-diabetes patient populations. Lastly, the fact that this new antibiotic is a microbiota metabolite suggests that human microbiome-related metabolites may present an underappreciated source for the discovery of novel antibiotics (Donia and Fischbach, 2015; Milshteyn et al., 2018).
DN, MG, VD, and TD: writing – original draft preparation, review, and editing. TD: funding acquisition. All authors have read and agreed to the published version of the manuscript.
This research was funded by the National Institute of Allergy and Infectious Diseases of the National Institutes of Health under Award Number R01AI132374. The content is solely the responsibility of the authors and does not necessarily represent the official views of the National Institutes of Health.
The authors declare that the research was conducted in the absence of any commercial or financial relationships that could be construed as a potential conflict of interest.
IPA, Indole propionic acid; TB, Tuberculosis; NTM, Non-tuberculous mycobacteria; R03, Rule-of-3; AhR, Aryl hydrocarbon receptor.
Abildgaard, A., Elfving, B., Hokland, M., Wegener, G., and Lund, S. (2018). The microbial metabolite indole-3-propionic acid improves glucose metabolism in rats, but does not affect behaviour. Arch. Physiol. Biochem. 124, 306–312. doi: 10.1080/13813455.2017.1398262
Aw, W., and Fukuda, S. (2018). Understanding the role of the gut ecosystem in diabetes mellitus. J. Diabetes Investig. 9, 5–12. doi: 10.1111/jdi.12673
Bashiri, G., Johnston, J. M., Evans, G. L., Bulloch, E. M., Goldstone, D. C., Jirgis, E. N., et al. (2015). Structure and inhibition of subunit I of the anthranilate synthase complex of Mycobacterium tuberculosis and expression of the active complex. Acta Crystallogr. D Biol. Crystallogr. 71, 2297–2308. doi: 10.1107/s1399004715017216
Be, N. A., Kim, K. S., Bishai, W. R., and Jain, S. K. (2009). Pathogenesis of central nervous system tuberculosis. Curr. Mol. 9, 94–99. doi: 10.2174/156652409787581655
Bendheim, P. E., Poeggeler, B., Neria, E., Ziv, V., Pappolla, M. A., and Chain, D. G. (2002). Development of indole-3-propionic acid (OXIGON) for Alzheimer’s disease. J. Mol. Neurosci. 19, 213–217. doi: 10.1007/s12031-002-0036-0
Benson, J. M., and Shepherd, D. M. (2011). Dietary ligands of the aryl hydrocarbon receptor induce anti-inflammatory and immunoregulatory effects on murine dendritic cells. Toxicol. Sci. 124, 327–338. doi: 10.1093/toxsci/kfr249
Cameron, A. R., Morrison, V. L., Levin, D., Mohan, M., Forteath, C., Beall, C., et al. (2016). Anti-inflammatory effects of metformin irrespective of diabetes status. Circ. Res. 119, 652–665. doi: 10.1161/CIRCRESAHA.116.308445
Cervenka, I., Agudelo, L. Z., and Ruas, J. L. (2017). Kynurenines: tryptophan’s metabolites in exercise, inflammation, and mental health. Science 357:eaaf9794. doi: 10.1126/science.aaf9794
Chiang, S. S., Khan, F. A., Milstein, M. B., Tolman, A. W., Benedetti, A., Starke, J. R., et al. (2014). Treatment outcomes of childhood tuberculous meningitis: a systematic review and meta-analysis. Lancet Infect. Dis. 14, 947–957. doi: 10.1016/s1473-3099(14)70852-7
Chyan, Y. J., Poeggeler, B., Omar, R. A., Chain, D. G., Frangione, B., Ghiso, J., et al. (1999). Potent neuroprotective properties against the Alzheimer beta-amyloid by an endogenous melatonin-related indole structure, indole-3-propionic acid. J. Biol. Chem. 274, 21937–21942. doi: 10.1074/jbc.274.31.21937
Clardy, J., Fischbach, M. A., and Currie, C. R. (2009). The natural history of antibiotics. Curr. Biol. 19, R437–R441. doi: 10.1016/j.cub.2009.04.001
Collins, J. M., Siddiqa, A., Jones, D. P., Liu, K., Kempker, R. R., Nizam, A., et al. (2020). Tryptophan catabolism reflects disease activity in human tuberculosis. JCI Insight 5:e137131. doi: 10.1172/jci.insight.137131
Crunkhorn, S. (2018). Autoimmune disease: aryl hydrocarbon receptor suppresses inflammation. Nat. Rev. Drug Discov. 17:470. doi: 10.1038/nrd.2018.107
Dartois, V. (2014). The path of anti-tuberculosis drugs: from blood to lesions to mycobacterial cells. Nat. Rev. Microbiol. 12, 159–167. doi: 10.1038/nrmicro3200
Davis, A., Meintjes, G., and Wilkinson, R. J. (2018). Treatment of tuberculous meningitis and its complications in adults. Curr. Treat. Options Neurol. 20:5. doi: 10.1007/s11940-018-0490-9
Davis, A. G., Rohlwink, U. K., Proust, A., Figaji, A. A., and Wilkinson, R. J. (2019). The pathogenesis of tuberculous meningitis. J. Leukoc. Biol. 105, 267–280. doi: 10.1002/jlb.Mr0318-102r
de Mello, V. D., Paananen, J., Lindstrom, J., Lankinen, M. A., Shi, L., Kuusisto, J., et al. (2017). Indolepropionic acid and novel lipid metabolites are associated with a lower risk of type 2 diabetes in the finnish diabetes prevention study. Sci. Rep. 7:46337. doi: 10.1038/srep46337
Dhiman, R., Indramohan, M., Barnes, P. F., Nayak, R. C., Paidipally, P., Rao, L. V., et al. (2009). IL-22 produced by human NK cells inhibits growth of Mycobacterium tuberculosis by enhancing phagolysosomal fusion. J. Immunol. 183, 6639–6645. doi: 10.4049/jimmunol.0902587
Dodd, D., Spitzer, M. H., Van Treuren, W., Merrill, B. D., Hryckowian, A. J., Higginbottom, S. K., et al. (2017). A gut bacterial pathway metabolizes aromatic amino acids into nine circulating metabolites. Nature 551, 648–652. doi: 10.1038/nature24661
Donia, M. S., and Fischbach, M. A. (2015). Human microbiota. Small molecules from the human microbiota. Science 349:1254766. doi: 10.1126/science.1254766
Dooley, K. E., and Chaisson, R. E. (2009). Tuberculosis and diabetes mellitus: convergence of two epidemics. Lancet Infect. Dis. 9, 737–746. doi: 10.1016/s1473-3099(09)70282-8
Gao, J., Xu, K., Liu, H., Liu, G., Bai, M., Peng, C., et al. (2018). Impact of the gut microbiota on intestinal immunity mediated by tryptophan metabolism. Front. Cell. Infect. Microbiol. 8:13. doi: 10.3389/fcimb.2018.00013
Gautam, U. S., Foreman, T. W., Bucsan, A. N., Veatch, A. V., Alvarez, X., Adekambi, T., et al. (2018). In vivo inhibition of tryptophan catabolism reorganizes the tuberculoma and augments immune-mediated control of Mycobacterium tuberculosis. Proc. Natl. Acad. Sci. U. S. A. 115, E62–E71. doi: 10.1073/pnas.1711373114
Genilloud, O. (2017). Actinomycetes: still a source of novel antibiotics. Nat. Prod. Rep. 34, 1203–1232. doi: 10.1039/c7np00026j
Gopal, P., and Dick, T. (2014). Reactive dirty fragments: implications for tuberculosis drug discovery. Curr. Opin. Microbiol. 21, 7–12. doi: 10.1016/j.mib.2014.06.015
Gopal, P., Nartey, W., Ragunathan, P., Sarathy, J., Kaya, F., Yee, M., et al. (2017). Pyrazinoic acid inhibits mycobacterial coenzyme a biosynthesis by binding to aspartate decarboxylase panD. ACS Infect. Dis. 3, 807–819. doi: 10.1021/acsinfecdis.7b00079
Gopal, P., Sarathy, J. P., Yee, M., Ragunathan, P., Shin, J., Bhushan, S., et al. (2020). Pyrazinamide triggers degradation of its target aspartate decarboxylase. Nat. Commun. 11:1661. doi: 10.1038/s41467-020-15516-1
Graham, S. M., and Donald, P. R. (2014). Death and disability: the outcomes of tuberculous meningitis. Lancet Infect. Dis. 14, 902–904. doi: 10.1016/s1473-3099(14)70872-2
Gutierrez-Vazquez, C., and Quintana, F. J. (2018). Regulation of the immune response by the aryl hydrocarbon receptor. Immunity 48, 19–33. doi: 10.1016/j.immuni.2017.12.012
Hitchings, G. H. (1973). Mechanism of action of trimethoprim-sulfamethoxazole—I. J. Infect. 128, S433–S436. doi: 10.1093/infdis/128.Supplement_3.S433
Hwang, I. K., Yoo, K. Y., Li, H., Park, O. K., Lee, C. H., Choi, J. H., et al. (2009). Indole-3-propionic acid attenuates neuronal damage and oxidative stress in the ischemic hippocampus. J. Neurosci. Res. 87, 2126–2137. doi: 10.1002/jnr.22030
Jain, S. K., Tobin, D. M., Tucker, E. W., Venketaraman, V., Ordonez, A. A., Jayashankar, L., et al. (2018). Tuberculous meningitis: a roadmap for advancing basic and translational research. Nat. Immunol. 19, 521–525. doi: 10.1038/s41590-018-0119-x
Jhoti, H., Williams, G., Rees, D. C., and Murray, C. W. (2013). The ‘rule of three’ for fragment-based drug discovery: where are we now? Nat. Rev. Drug Discov. 12, 644–645. doi: 10.1038/nrd3926-c1
Jiménez-Corona, M. E., Cruz-hervert, L. P., García-garcía, L., Ferreyra-reyes, L., Delgado-sánchez, G., Bobadilla-del-valle, M., et al. (2013). Association of diabetes and tuberculosis: impact on treatment and post-treatment outcomes. J. Thorax 68, 214–220. doi: 10.1136/thoraxjnl-2012-201756
Kalita, J., Misra, U. K., and Dubey, A. K. (2019). Role of oxidative stress in Tuberculous meningitis: a clinico-radiological correlation. J. Mol. Neurosci. 68, 287–294. doi: 10.1007/s12031-019-01304-0
Karbownik, M., Reiter, R. J., Garcia, J. J., Cabrera, J., Burkhardt, S., Osuna, C., et al. (2001). Indole-3-propionic acid, a melatonin-related molecule, protects hepatic microsomal membranes from iron-induced oxidative damage: relevance to cancer reduction. J. Cell. Biochem. 81, 507–513. doi: 10.1002/1097-4644(20010601)81:3<507::aid-jcb1064>3.0.co;2-m
Karbownik, M., Stasiak, M., Zygmunt, A., Zasada, K., and Lewinski, A. (2006). Protective effects of melatonin and indole-3-propionic acid against lipid peroxidation, caused by potassium bromate in the rat kidney. Cell Biochem. Funct. 24, 483–489. doi: 10.1002/cbf.1321
Kaufmann, S. H., and Dorhoi, A. (2013). Inflammation in tuberculosis: interactions, imbalances and interventions. Curr. Opin. Immunol. 25, 441–449. doi: 10.1016/j.coi.2013.05.005
Kennedy, E. A., King, K. Y., and Baldridge, M. T. (2018). Mouse microbiota models: comparing germ-free mice and antibiotics treatment as tools for modifying gut bacteria. Front. Physiol. 9:1534. doi: 10.3389/fphys.2018.01534
Kim, J. T., Kim, S. S., Jun, D. W., Hwang, Y. H., Park, W. -H., Pak, Y. K., et al. (2013). Serum arylhydrocarbon receptor transactivating activity is elevated in type 2 diabetic patients with diabetic nephropathy. J. Diabetes Investig. 4, 483–491. doi: 10.1111/jdi.12081
Kroesen, V. M., Gröschel, M. I., Martinson, N., Zumla, A., Maeurer, M., van der Werf, T. S., et al. (2017). Non-steroidal anti-inflammatory drugs as host-directed therapy for tuberculosis: a systematic review. Front. Immunol. 8:772. doi: 10.3389/fimmu.2017.00772
Kueck, T., Cassella, E., Holler, J., Kim, B., and Bieniasz, P. D. (2018). The aryl hydrocarbon receptor and interferon gamma generate antiviral states via transcriptional repression. eLife 7:e38867. doi: 10.7554/eLife.38867
Kumar, N. P., Sridhar, R., Banurekha, V. V., Jawahar, M. S., Fay, M. P., Nutman, T. B., et al. (2013). Type 2 diabetes mellitus coincident with pulmonary tuberculosis is associated with heightened systemic type 1, type 17, and other proinflammatory cytokines. Ann. Am. Thorac. Soc. 10, 441–449. doi: 10.1513/AnnalsATS.201305-112OC
Lai, R. P. J., Meintjes, G., Wilkinson, K. A., Graham, C. M., Marais, S., Van der Plas, H., et al. (2015). HIV-tuberculosis-associated immune reconstitution inflammatory syndrome is characterized by toll-like receptor and inflammasome signalling. Nat. Commun. 6:8451. doi: 10.1038/ncomms9451
Larsen, N., Vogensen, F. K., van den Berg, F. W., Nielsen, D. S., Andreasen, A. S., Pedersen, B. K., et al. (2010). Gut microbiota in human adults with type 2 diabetes differs from non-diabetic adults. PLoS One 5:e9085. doi: 10.1371/journal.pone.0009085
Lee, H. K., Park, W. H., Kang, Y. C., Kang, S., Im, S., Park, S., et al. (2020). Serum biomarkers from cell-based assays for AhRL and MIS strongly predicted the future development of diabetes in a large community-based prospective study in Korea. Sci. Rep. 10:6339. doi: 10.1038/s41598-020-62550-6
Leeson, P. D., and Springthorpe, B. (2007). The influence of drug-like concepts on decision-making in medicinal chemistry. Nat. Rev. Drug Discov. 6, 881–890. doi: 10.1038/nrd2445
Maitre, M., Klein, C., Patte-mensah, C., and Mensah-nyagan, A. G. (2020). Tryptophan metabolites modify brain Aβ peptide degradation: a role in Alzheimer’s disease? Prog. Neurobiol. 190:101800. doi: 10.1016/j.pneurobio.2020.101800
Mandal, P. K. (2005). Dioxin: a review of its environmental effects and its aryl hydrocarbon receptor biology. J. Comp. Physiol. B. 175, 221–230. doi: 10.1007/s00360-005-0483-3
Maneechotesuwan, K., Supawita, S., Kasetsinsombat, K., Wongkajornsilp, A., and Barnes, P. J. (2008). Sputum indoleamine-2, 3-dioxygenase activity is increased in asthmatic airways by using inhaled corticosteroids. J. Allergy Clin. Immunol. 121, 43–50. doi: 10.1016/j.jaci.2007.10.011
Martens, G. W., Arikan, M. C., Lee, J., Ren, F., Greiner, D., and Kornfeld, H. (2007). Tuberculosis susceptibility of diabetic mice. Am. J. Respir. Cell Mol. Biol. 37, 518–524. doi: 10.1165/rcmb.2006-0478OC
Mathipa, M. G., and Thantsha, M. S. (2017). Probiotic engineering: towards development of robust probiotic strains with enhanced functional properties and for targeted control of enteric pathogens. Gut Pathog. 9:28. doi: 10.1186/s13099-017-0178-9
Mehra, S., Alvarez, X., Didier, P. J., Doyle, L. A., Blanchard, J. L., Lackner, A. A., et al. (2013). Granuloma correlates of protection against tuberculosis and mechanisms of immune modulation by Mycobacterium tuberculosis. J. Infect. Dis. 207, 1115–1127. doi: 10.1093/infdis/jis778
Meintjes, G., Stek, C., Blumenthal, L., Thienemann, F., Schutz, C., Buyze, J., et al. (2018). Prednisone for the prevention of paradoxical tuberculosis-associated IRIS. N. Engl. J. Med. 379, 1915–1925. doi: 10.1056/NEJMoa1800762
Memari, B., Bouttier, M., Dimitrov, V., Ouellette, M., Behr, M. A., Fritz, J. H., et al. (2015). Engagement of the aryl hydrocarbon receptor in Mycobacterium tuberculosis-infected macrophages has pleiotropic effects on innate immune signaling. J. Immunol. 195, 4479–4491. doi: 10.4049/jimmunol.1501141
Milshteyn, A., Colosimo, D. A., and Brady, S. F. (2018). Accessing bioactive natural products from the human microbiome. Cell Host Microbe 23, 725–736. doi: 10.1016/j.chom.2018.05.013
Mimee, M., Citorik, R. J., and Lu, T. K. (2016). Microbiome therapeutics—advances and challenges. Adv. Drug Deliv. Rev. 105, 44–54. doi: 10.1016/j.addr.2016.04.032
Moura-Alves, P., Fae, K., Houthuys, E., Dorhoi, A., Kreuchwig, A., Furkert, J., et al. (2014). AhR sensing of bacterial pigments regulates antibacterial defence. Nature 512, 387–392. doi: 10.1038/nature13684
Naidoo, C. C., Nyawo, G. R., Wu, B. G., Walzl, G., Warren, R. M., Segal, L. N., et al. (2019). The microbiome and tuberculosis: state of the art, potential applications, and defining the clinical research agenda. Lancet Respir. Med. 7, 892–906. doi: 10.1016/s2213-2600(18)30501-0
Negatu, D. A., Liu, J. J. J., Zimmerman, M., Kaya, F., Dartois, V., Aldrich, C. C., et al. (2018). Whole-cell screen of fragment library identifies gut microbiota metabolite indole propionic acid as antitubercular. Antimicrob. Agents Chemother. 62, e01571–e01617. doi: 10.1128/AAC.01571-17
Negatu, D. A., Yamada, Y., Xi, Y., Go, M. L., Zimmerman, M., Ganapathy, U., et al. (2019). Gut microbiota metabolite indole propionic acid targets tryptophan biosynthesis in Mycobacterium tuberculosis. mBio 10, e02781–e02818. doi: 10.1128/mBio.02781-18
Nguyen, D. T., Agarwal, S., and Graviss, E. A. (2019). Trends of tuberculosis meningitis and associated mortality in Texas, 2010-2017, a large population-based analysis. PLoS One 14:e0212729. doi: 10.1371/journal.pone.0212729
Nicolas, G. R., and Chang, P. V. (2019). Deciphering the chemical lexicon of host-gut microbiota interactions. Trends Pharmacol. Sci. 40, 430–445. doi: 10.1016/j.tips.2019.04.006
Palucci, I., and Delogu, G. (2018). Host directed therapies for tuberculosis: futures strategies for an ancient disease. Chemotherapy 63, 172–180. doi: 10.1159/000490478
Platten, M., Nollen, E. A. A., Röhrig, U. F., Fallarino, F., and Opitz, C. A. (2019). Tryptophan metabolism as a common therapeutic target in cancer, neurodegeneration and beyond. Nat. Rev. Drug Discov. 18, 379–401. doi: 10.1038/s41573-019-0016-5
Prasad, K., Singh, M. B., and Ryan, H. (2016). Corticosteroids for managing tuberculous meningitis. Cochrane Database Syst. Rev. 4:Cd002244. doi: 10.1002/14651858.CD002244.pub4
Prideaux, B., Via, L. E., Zimmerman, M. D., Eum, S., Sarathy, J., O’Brien, P., et al. (2015). The association between sterilizing activity and drug distribution into tuberculosis lesions. Nat. Med. 21, 1223–1227. doi: 10.1038/nm.3937
Puyskens, A., Stinn, A., van der Vaart, M., Kreuchwig, A., Protze, J., Pei, G., et al. (2019). Aryl hydrocarbon receptor modulation by tuberculosis drugs impairs host defense and treatment outcomes. Cell Host Microbe 27:238.e7–248.e7. doi: 10.1016/j.chom.2019.12.005
Reardon, S. (2014). Microbiome therapy gains market traction. Nature 509, 269–270. doi: 10.1038/509269a
Riccardi, G., and Pasca, M. R. (2014). Trends in discovery of new drugs for tuberculosis therapy. J. Antibiot. 67, 655–659. doi: 10.1038/ja.2014.109
Roager, H. M., and Licht, T. R. (2018). Microbial tryptophan catabolites in health and disease. Nat. Commun. 9:3294. doi: 10.1038/s41467-018-05470-4
Roca, F. J., and Ramakrishnan, L. (2013). TNF dually mediates resistance and susceptibility to mycobacteria via mitochondrial reactive oxygen species. Cell 153, 521–534. doi: 10.1016/j.cell.2013.03.022
Rock, R. B., Olin, M., Baker, C. A., Molitor, T. W., and Peterson, P. K. (2008). Central nervous system tuberculosis: pathogenesis and clinical aspects. Clin. Microbiol. Rev. 21, 243–261. doi: 10.1128/CMR.00042-07
Roh, E., Kwak, S. H., Jung, H. S., Cho, Y. M., Pak, Y. K., Park, K. S., et al. (2015). Serum aryl hydrocarbon receptor ligand activity is associated with insulin resistance and resulting type 2 diabetes. Acta Diabetol. 52, 489–495. doi: 10.1007/s00592-014-0674-z
Rohlwink, U. K., Figaji, A., Wilkinson, K. A., Horswell, S., Sesay, A. K., Deffur, A., et al. (2019). Tuberculous meningitis in children is characterized by compartmentalized immune responses and neural excitotoxicity. Nat. Commun. 10:3767. doi: 10.1038/s41467-019-11783-9
Rothhammer, V., Mascanfroni, I. D., Bunse, L., Takenaka, M. C., Kenison, J. E., Mayo, L., et al. (2016). Type I interferons and microbial metabolites of tryptophan modulate astrocyte activity and central nervous system inflammation via the aryl hydrocarbon receptor. Nat. Med. 22, 586–597. doi: 10.1038/nm.4106
Schutz, C., Davis, A. G., Sossen, B., Lai, R. P., Ntsekhe, M., Harley, Y. X., et al. (2018). Corticosteroids as an adjunct to tuberculosis therapy. Expert Rev. Respir. Med. 12, 881–891. doi: 10.1080/17476348.2018.1515628
Scriba, T. J., Kalsdorf, B., Abrahams, D. A., Isaacs, F., Hofmeister, J., Black, G., et al. (2008). Distinct, specific IL-17- and IL-22-producing CD4+ T cell subsets contribute to the human anti-mycobacterial immune response. J. Immunol. 180, 1962–1970. doi: 10.4049/jimmunol.180.3.1962
Sereti, I. (2020). Immune reconstruction inflammatory syndrome in HIV infection: beyond what meets the eye. Top. Antivir. Med. 27, 106–111.
Shen, H., and Chen, Z. W. (2018). The crucial roles of Th17-related cytokines/signal pathways in M. tuberculosis infection. Cell. Mol. Immunol. 15, 216–225. doi: 10.1038/cmi.2017.128
Srinivasan, K., and Ramarao, P. (2007). Animal models in type 2 diabetes research: an overview. Indian J. Med. Res. 125, 451–472.
Stockinger, B., Di Meglio, P., Gialitakis, M., and Duarte, J. H. (2014). The aryl hydrocarbon receptor: multitasking in the immune system. Annu. Rev. Immunol. 32, 403–432. doi: 10.1146/annurev-immunol-032713-120245
Suez, J., and Elinav, E. (2017). The path towards microbiome-based metabolite treatment. Nat. Microbiol. 2:17075. doi: 10.1038/nmicrobiol.2017.75
Thuong, N. T. T., and Thwaites, G. E. (2017). Treatment-associated inflammatory deterioration in tuberculous meningitis: unpicking the paradox. J. Infect. 215, 665–667. doi: 10.1093/infdis/jiw565
Thwaites, G. E., Bang, N. D., Dung, N. H., Quy, H. T., Oanh, D. T. T., Thoa, N. T. C., et al. (2004). Dexamethasone for the treatment of tuberculous meningitis in adolescents and adults. N. Engl. J. Med. 351, 1741–1751. doi: 10.1056/NEJMoa040573
Thwaites, G., Chau, T. T. H., Mai, N. T. H., Drobniewski, F., McAdam, K., and Farrar, J. (2000). Tuberculous meningitis. J. Neurol. Neurosurg. Psychiatry 68, 289–299. doi: 10.1136/jnnp.68.3.289
Tsenova, L., Harbacheuski, R., Moreira, A. L., Ellison, E., Dalemans, W., Alderson, M. R., et al. (2006). Evaluation of the Mtb72F polyprotein vaccine in a rabbit model of tuberculous meningitis. Infect. Immun. 74, 2392–2401. doi: 10.1128/iai.74.4.2392-2401.2006
Tucker, E. W., Pokkali, S., Zhang, Z., Demarco, V. P., Klunk, M., Smith, E. S., et al. (2016). Microglia activation in a pediatric rabbit model of tuberculous meningitis. Dis. Model. Mech. 9, 1497–1506. doi: 10.1242/dmm.027326
Tuomainen, M., Lindström, J., Lehtonen, M., Auriola, S., Pihlajamäki, J., Peltonen, M., et al. (2018). Associations of serum indolepropionic acid, a gut microbiota metabolite, with type 2 diabetes and low-grade inflammation in high-risk individuals. Nutr. Diabetes 8:35. doi: 10.1038/s41387-018-0046-9
Umemura, M., Yahagi, A., Hamada, S., Begum, M. D., Watanabe, H., Kawakami, K., et al. (2007). IL-17-mediated regulation of innate and acquired immune response against pulmonary Mycobacterium bovis bacille Calmette-Guerin infection. J. Immunol. 178, 3786–3796. doi: 10.4049/jimmunol.178.6.3786
Vallerskog, T., Martens, G. W., and Kornfeld, H. (2010). Diabetic mice display a delayed adaptive immune response to Mycobacterium tuberculosis. J. Immunol. 184, 6275–6782. doi: 10.4049/jimmunol.1000304
van Laarhoven, A., Dian, S., Aguirre-gamboa, R., Avila-pacheco, J., Ricano-ponce, I., Ruesen, C., et al. (2018). Cerebral tryptophan metabolism and outcome of tuberculous meningitis: an observational cohort study. Lancet Infect. Dis. 18, 526–535. doi: 10.1016/S1473-3099(18)30053-7
Veldhoen, M., Hirota, K., Westendorf, A. M., Buer, J., Dumoutier, L., Renauld, J. C., et al. (2008). The aryl hydrocarbon receptor links TH17-cell-mediated autoimmunity to environmental toxins. Nature 453, 106–109. doi: 10.1038/nature06881
Walker, N. F., Stek, C., Wasserman, S., Wilkinson, R. J., and Meintjes, G. (2018). The tuberculosis-associated immune reconstitution inflammatory syndrome: recent advances in clinical and pathogenesis research. Curr. Opin. HIV AIDS 13, 512–521. doi: 10.1097/COH.0000000000000502
Wang, C., Xu, C. X., Krager, S. L., Bottum, K. M., Liao, D. F., and Tischkau, S. A. (2011). Aryl hydrocarbon receptor deficiency enhances insulin sensitivity and reduces PPAR-α pathway activity in mice. Environ. Health Perspect. 119, 1739–1744. doi: 10.1289/ehp.1103593
Wellington, S., Nag, P. P., Michalska, K., Johnston, S. E., Jedrzejczak, R. P., Kaushik, V. K., et al. (2017). A small-molecule allosteric inhibitor of Mycobacterium tuberculosis tryptophan synthase. Nat. Chem. Biol. 13, 943–950. doi: 10.1038/nchembio.2420
WHO (2019). Global tuberculosis report 2019. Geneva: World Health Organization Licence: CC BY-NC-SA 3.0 IGO.
Wikoff, W. R., Anfora, A. T., Liu, J., Schultz, P. G., Lesley, S. A., Peters, E. C., et al. (2009). Metabolomics analysis reveals large effects of gut microflora on mammalian blood metabolites. Proc. Natl. Acad. Sci. U. S. A. 106, 3698–3703. doi: 10.1073/pnas.0812874106
Wilkinson, R. J., Rohlwink, U., Misra, U. K., van Crevel, R., Mai, N. T. H., Dooley, K. E., et al. (2017). Tuberculous meningitis. Nat. Rev. Neurol. 13, 581–598. doi: 10.1038/nrneurol.2017.120
Wood, M. R., Yu, E. A., and Mehta, S. (2017). The human microbiome in the fight against tuberculosis. Am. J. Trop. Med. Hyg. 96, 1274–1284. doi: 10.4269/ajtmh.16-0581
Yisireyili, M., Takeshita, K., Saito, S., Murohara, T., and Niwa, T. (2017). Indole-3-propionic acid suppresses indoxyl sulfate-induced expression of fibrotic and inflammatory genes in proximal tubular cells. Nagoya J. Med. Sci. 79, 477–486. doi: 10.18999/nagjms.79.4.477
Young, S. N., Anderson, G. M., Gauthier, S., and Purdy, W. C. (1980). The origin of indoleacetic acid and indolepropionic acid in rat and human cerebrospinal fluid. J. Neurochem. 34, 1087–1092. doi: 10.1111/j.1471-4159.1980.tb09944.x
Zelante, T., Iannitti, R. G., Cunha, C., De Luca, A., Giovannini, G., Pieraccini, G., et al. (2013). Tryptophan catabolites from microbiota engage aryl hydrocarbon receptor and balance mucosal reactivity via interleukin-22. Immunity 39, 372–385. doi: 10.1016/j.immuni.2013.08.003
Zhan, L., Tang, J., Sun, M., and Qin, C. (2017). Animal models for tuberculosis in translational and precision medicine. Front. Microbiol. 8:717. doi: 10.3389/fmicb.2017.00717
Zhang, M., and He, J. Q. (2020). Impacts of metformin on tuberculosis incidence and clinical outcomes in patients with diabetes: a systematic review and meta-analysis. Eur. J. Clin. Pharmacol. 76, 149–159. doi: 10.1007/s00228-019-02786-y
Zhang, Y. J., Reddy, M. C., Ioerger, T. R., Rothchild, A. C., Dartois, V., Schuster, B. M., et al. (2013). Tryptophan biosynthesis protects mycobacteria from CD4 T-cell-mediated killing. Cell 155, 1296–1308. doi: 10.1016/j.cell.2013.10.045
Keywords: antibiotic, gut microbiota, Mycobacterium, tuberculosis, non-tuberculous mycobacteria, host-directed therapy
Citation: Negatu DA, Gengenbacher M, Dartois V and Dick T (2020) Indole Propionic Acid, an Unusual Antibiotic Produced by the Gut Microbiota, With Anti-inflammatory and Antioxidant Properties. Front. Microbiol. 11:575586. doi: 10.3389/fmicb.2020.575586
Received: 25 June 2020; Accepted: 02 October 2020;
Published: 27 October 2020.
Edited by:
Paul Laszlo Bollyky, Stanford University, United StatesReviewed by:
Katalin A. Wilkinson, Francis Crick Institute, United KingdomCopyright © 2020 Negatu, Gengenbacher, Dartois and Dick. This is an open-access article distributed under the terms of the Creative Commons Attribution License (CC BY). The use, distribution or reproduction in other forums is permitted, provided the original author(s) and the copyright owner(s) are credited and that the original publication in this journal is cited, in accordance with accepted academic practice. No use, distribution or reproduction is permitted which does not comply with these terms.
*Correspondence: Thomas Dick, dGhvbWFzLmRpY2tAaG1oLWNkaS5vcmc=
Disclaimer: All claims expressed in this article are solely those of the authors and do not necessarily represent those of their affiliated organizations, or those of the publisher, the editors and the reviewers. Any product that may be evaluated in this article or claim that may be made by its manufacturer is not guaranteed or endorsed by the publisher.
Research integrity at Frontiers
Learn more about the work of our research integrity team to safeguard the quality of each article we publish.