- 1Department of Interdisciplinary Health Sciences, The University of Texas at El Paso, El Paso, TX, United States
- 2Biology Department, El Paso Community College, El Paso, TX, United States
- 3Biological Sciences Department, The University of Texas at El Paso, El Paso, TX, United States
- 4Clinical Laboratory Science Program/Department of Public Health Sciences, The University of Texas at El Paso, El Paso, TX, United States
Calcium ions (Ca2+) play a pivotal role in eukaryote cell signaling and regulate many physiological functions. Although a similar role for Ca2+ in prokaryotes has been difficult to demonstrate, there is increasing evidence for Ca2+ as a cell regulator in bacteria. The purpose of this study was to investigate Ca2+ signaling and the effect of Ca2+ on the Staphylococcus aureus multidrug resistant efflux pump LmrS. We hypothesized that antibiotics act by increasing Ca2+ concentrations, which in turn enhance the efflux activity of LmrS. These Ca2+ transients were measured by luminometry in response to various antibiotics by using the photoprotein aequorin reconstituted within live bacterial cells. Efflux associated with LmrS was measured by the increase in fluorescence due to the loss of ethidium bromide (EtBr) from both S. aureus cells and from E. coli cells in which the lmrs gene of S. aureus was expressed. We found that addition of antibiotics to cells generated unique cytosolic Ca2+ transients and that addition of CaCl2 to cells enhanced EtBr efflux whereas addition of Ca2+ chelators or efflux pump inhibitors significantly decreased EtBr efflux from cells. We conclude that antibiotics induce a Ca2+ mediated response through transients in cytosolic Ca2+, which then stimulates LmrS efflux pump.
Introduction
Calcium ions (Ca2+) are recognized as key messengers and regulators in nearly all cellular functions of eukaryotic cells (Clapham, 1995; Campbell, 2015, 2018; Carafoli and Krebs, 2016). Ca2+ signaling is well understood in mammals and regulates a wide variety of processes ranging from cell fertilization to apoptosis (Rajagopal and Murugavel, 2017; Moretti et al., 2019). Cells respond to environmental stimuli by transient changes in intracellular free Ca2+ concentration [Ca2+]i, which is utilized by cells to transmit information. Physiological responses also depend on the magnitude, speed and spatiotemporal patterns of the Ca2+ signal (Berridge et al., 2003; Zampese and Pizzo, 2012).
In contrast to eukaryotes where the molecular mechanisms for changes in [Ca2+]i are well understood, in prokaryotes much work needs to be done. However, there is growing evidence that Ca2+ also plays a regulatory role in prokaryotes (Broder et al., 2016; Fishman et al., 2018; Moretti et al., 2019; King et al., 2020). Ca2+ ions are involved in numerous bacterial cellular processes including: chemotaxis, transport mechanisms, cell differentiation, virulence, gene expression and others (Fishman et al., 2018; Moretti et al., 2019; Takahashi and Kopriva, 2019; for reviews see Domínguez, 2004; Domínguez et al., 2015). In addition, various Ca2+-binding proteins have been identified, which possess calcium binding motifs (Zhou et al., 2013; Sarkisova et al., 2014; Domínguez et al., 2015). Similar to eukaryotes, cytosolic Ca2+ homeostasis has been demonstrated in various bacteria and cytosolic Ca2+ transients occur in response to stimuli (Torrecilla et al., 2000; Domínguez et al., 2011; Guragain et al., 2013; Zhou et al., 2013; Sarkisova et al., 2014; Bruni et al., 2017; González-Pleiter et al., 2017). During infection and inflammation processes, levels of Ca2+ fluctuate significantly, which impact host-pathogen interactions (Van Nhieu et al., 2003; Barrán-Berdón et al., 2011; Broder et al., 2016). However, the role of Ca2+ in bacterial pathogenesis is limited and warrants further investigation.
Staphylococcus aureus is a versatile pathogen that can cause a wide variety of infections and is able to survive in various environments (Dastgheyb et al., 2015; Li et al., 2015; Onyango and Alreshidi, 2018). The unique adaptability of S. aureus makes this organism one of the most problematic bacterial pathogens worldwide (Dastgheyb et al., 2015; Hassan et al., 2015; Kobayashi et al., 2015; Onyango and Alreshidi, 2018). The survival strategies of this organism are diverse and include the ability to replicate in phagosomes, the production of a number of virulence factors such hemolysins, immune evasion factors and resistance to cationic antimicrobial peptides, all of which lead to survival in host cells (Fraunholz and Sinha, 2012; Kobayashi et al., 2015). It is notable that Ca2+ plays an important role in S. aureus cell adhesion, modulation of biofilm architecture, modulation of α-hemolysin, and autophagy (Thomas et al., 1993; Arrizubieta et al., 2004; Eichstaedt et al., 2009; Fraunholz and Sinha, 2012).
Staphylococcus aureus can develop resistance to numerous antimicrobial compounds, including antibiotics and biocides (Esposito et al., 2011; Santos Costa et al., 2013; Conceição et al., 2016; Jang, 2016; Foster, 2017). Although the resistance developed by S. aureus strains may be due to different resistance mechanisms, multi-drug resistant efflux pumps (MDREP) play a major role in mediating cross-resistance to antibiotics and biocides (Conceição et al., 2016; Sapula and Brown, 2016; Foster, 2017). In prokaryotes, there are seven classes of MDREP identified: the major facilitator superfamily (MSF), ATP binding cassette (ABC) superfamily, multidrug and toxin extrusion (MATE) family, resistance nodulation division (RND), the small multidrug resistant (SMR) family, the proteobacterial antimicrobial compound efflux (PACE) family, and the p-aminobenzoyl-glutamate transporter (AbgT) family (Webber and Piddock, 2003; Hassan et al., 2013; Santos Costa et al., 2013; Jang, 2016; Schindler and Kaatz, 2016; Chitsaz and Brown, 2017). These transport proteins extrude a wide variety of toxic compounds to the exterior of the cell. Since these transporters have a broad range of substrates, high activity of these pumps can result in the efflux of multiple antibiotics, disinfectants, detergents, dyes, and biocides (Piddock, 2006). Furthermore, efflux pumps play a role in host colonization, virulence and adaptive responses that contribute to resistance during infection (Piddock, 2006; Alcalde-Rico et al., 2016; Jang, 2016; Du et al., 2018). To date, more than twenty putative efflux pumps have been identified in the S. aureus genome but few have been characterized. Overexpression of these pumps is proposed to contribute to antibiotic resistance and possibly enhances survival in different environments (Santos Costa et al., 2013; Schindler et al., 2015; Jang, 2016; Sapula and Brown, 2016).
Bacteria respond to environmental stimuli (oxidative stress, cold and heat, changes in pH, salinity and osmotic stress including antimicrobials) by changes in intracellular calcium ion, [Ca2+]i, (Knight et al., 1991; Herbaud et al., 1998; Torrecilla et al., 2000, 2001; Naseem et al., 2009; González-Pleiter et al., 2017). Since changes in [Ca2+]i are linked to gene expression (Naseem et al., 2009; Domínguez et al., 2011) and virulence (Sarkisova et al., 2005; Broder et al., 2016; Moretti et al., 2019; King et al., 2020), we hypothesize that when S. aureus cells are exposed to antibiotics or other drugs, they respond by spiking [Ca2+]i, which in turn stimulates efflux pump activity. We tested this hypothesis on one of the multi-drug resistant pump proteins of S. aureus, the LmrS, by looking at changes in activity of excretion of EtBr from cells upon varying intracellular Ca2+ concentrations and by measuring whether the addition of antibiotics to S. aureus cells could increase their [Ca2+]i concentrations. Using the photoprotein aequorin as a reporter, we demonstrate for the first time, cytosolic free Ca2+ concentration changes in S. aureus cells in response to stimuli and rates of influx and efflux in live cells. We conclude that cytosolic Ca2+ concentration is carefully controlled in S. aureus, antibiotics induce a unique response mediated by cytosolic Ca2+ and Ca2+ ions enhance the efflux of EtBr in the MDREP LmrS.
Materials and Methods
Bacterial Strains and Culture Media
Staphylococcus aureus ATCC 25923 and clinical isolate EBSA54 were grown in Brain Heart Infusion (BHI) broth (Remel, Thermo Fisher Scientific, Lenexa, KS, United States). E. coli strain JM109 containing the expression vector pMMB66EH with the apoaequorin coding sequence was used to conjugate S. aureus cells. E. coli cells were a gift from Dr. Anthony K. Campbell (Cardiff University, United Kingdom). E. coli cells were grown in Luria-Bertani (LB) broth (Becton Dickinson DifcoTM, Sparks, MD, United States) with carbenicillin (100 μg/ml) (Sigma-Aldrich, St. Louis, MO, United States). Confirmation of S. aureus after conjugation was done by culture in Mannitol Salt Agar (MSA) (Becton Dickinson DifcoTM, Sparks, MD, United States) and testing for coagulase production (plasma rabbit with EDTA, Becton Dickinson BBLTM) and latex agglutination (ProlexTM Pro-Lab Diagnostics, Richmond Hill, ON, Canada). Mueller-Hinton broth (Oxoid Ltd., Basingstoke, Hampshire, United Kingdom) was used to determine the Minimum inhibitory concentration (MIC) assays. The vector pRMC2 was purchased from Addgene to clone the amplified lmrS gene. DH5α E. coli cells were obtained from Promega, Madison, WI, United States).
Chemicals and Biochemicals
Ethidium bromide (EtBr), ethylene glycol-bis (β-aminoethyl ether)-N,N,N′,N′-tetraacetic acid (EGTA), ethylenediaminetetra acetic acid (EDTA), isopropyl-β-D-thiogalactoside (IPTG), 4-(2-hydroxyethyl)-1-piperazineethanesulfonic acid (HEPES) coelenterazine, carbenicillin, CaCl2, Carbonyl cyanide m-chloro phenylhydrazone (CCCP), Calmidazolium (CDZ), Verapamil, coelenterazine, Trifluoperazine (TPZ), Chlorpromazine (CPZ), Phosphate Buffer Solution (PBS), Triton X-100, Tris-Acetate EDTA buffer (TAE), and aequorin oligonucleotides were all purchased from Sigma-Aldrich (St. Louis, MO, United States). Taq polymerase master mix for PCR (Promega, Madison, WI, United States), agarose molecular grade and 100 bp molecular ruler were obtained from Bio-Rad, Hercules, CA, United States). Kanamycin, Gentamicin, Streptomycin, Vancomycin, Ciprofloxacin, and Erythromycin (St. Louis, MO, United States). BamHI and HindIII (Rowley, MA, United States), T4 ligase (Rowley, MA, United States).
Cytosolic Free Ca2+ Measurements: Construction and Expression of Apoaequorin in S. aureus
Staphylococcus aureus cells were transformed by conjugation using E. coli cells containing the apoaequorin coding sequence (apoaequorin is the protein without its prosthetic group, coelenterazine). Conjugation was done according to the protocols for tri-parental mating from The Samuel Miller Lab (2019) Conjugating plasmids into bacteria http://miller-lab.net/Miller Lab/protocols/bacterial-genetics/conjugating-plasmids-into-bac teria/with modifications. E. coli and S. aureus were grown in 250 mL triple-baffled flasks containing 50 ml LB (Becton Dickinson DifcoTM) broth with 100 μg/mL carbenicillin (Sigma-Aldrich) and BHI broth (Remel Thermo Fisher) respectively, at 37°C, in a rotatory shaker at 220 rpm overnight. After 18–20 h of incubation, E. coli and S. aureus cultures were adjusted to an OD600 of 0.5. A 1:5 dilution (100 μL of E. coli and 400 μL S. aureus cells) of the bacterial cultures were incubated in 10 mL of BHI with 100 μg/mL carbenicillin and incubated for 3 h at 37°C (220 rpm). The culture was dotted (25 μl) onto BHI agar plates containing 100 μg/mL carbenicillin (Remel Thermo Fisher; Sigma-Aldrich) and incubated for 48–72 h at 37°C. Transformed S. aureus cells were isolated by streaking onto MSA (Becton Dickinson DifcoTM) for 48–72 h at 37°C. Individual bacterial colonies that fermented mannitol were selected and further tested for coagulase (Becton Dickinson BBLTM) and anti-S. aureus latex agglutination (ProlexTM Pro-Lab Diagnostics). The presence of the plasmid containing the apoaequorin coding sequence was confirmed by PCR (Bio-Rad iCycler Thermal Cycler, Bio-Rad, Hercules, CA, United States). The oligonucleotides, AQ440LICS: Forward: AAGGAGGAAGCAGGTATGGTCAAGCTTACATCAGACTTC GAC and AQ440LICS-CAS Reverse: GACACGCACGAGG TTTAGGGGACAGCTCCACCGTAG were used for DNA amplification (Domínguez et al., 2011) under the following parameters: Initial denaturation 95°C for 4.0 min, 30 cycles of 95° denaturation for 30 s, annealing at 50°C for 30 s, extension at 72°C for 1 min, and final extension for 10 min at 72°C. The aequorin protein was utilized to monitor the amount of intracellular free Ca2+. Aequorin is a protein that luminescence (λmax = 469 nm) when it binds to Ca2. The Ca2+ concentration of the cytosol was measured at rest (basal levels) and after adding increasing concentrations of CaCl2 to the culture media. The luminescence produced is directly proportional to the concentration of free Ca2+ within the cytosol.
Expression and Reconstitution of Aequorin in S. aureus Cells
The transformed S. aureus were grown in BHI-carbenicillin broth overnight at 37° (220 rpm). Cells were diluted 1:100 in BHI-carbenicillin broth and incubated (same conditions) until the culture reached an OD600 of 0.3. The aequorin gene was induced by adding IPTG at 1 mM final concentration and re-incubated for additional 2 h. After 2 h, cells were washed twice with 20 mL of ice cold HEPES buffer (25 mM HEPES, 1 mM MgCl2, and 125 mM NaCl, pH 7.0) or at the respective experimental pHs (5, 7, and 9) and pelleted by centrifugation at 4500 rpm for 5 min (Beckman Coulter Allegra, rotor C0650). Bacterial cells were re-suspended in 1 mL of HEPES and 2.5 μM of coelenterazine and incubated in the dark for 1 h at room temperature. After incubation, cells were washed twice first in 20 mL HEPES buffer and resuspended in 1 mL of HEPES. The buffer was adjusted to an OD600 0.4 at the appropriate pH (according to the experiments performed) and stored on ice for subsequent readings or 4°C overnight for stabilization. To address possible contamination from trace amounts of Ca2+ and other divalent cations, 0.05 mM of EGTA was added to the HEPES media. All glassware and labware were washed with 0.05 mM of EGTA to rinse off trace levels of calcium from the surfaces.
Detection and Quantification of Intracellular Ca2+
Chemiluminescence was measured using a digital Luminometer GloMax 3000 (Promega) equipped with two dispensers allowing the reading on 96-well Microfluor microtiter plates (Thermo Fisher Scientific). Measurements were done in triplicates on 100 μL aequorin-loaded cells once every 10 s for 60 s to determine resting cytosolic free Ca2+ levels. Cells were then injected with CaCl2 to a final concentration of 1.0 mM CaCl2 and chemiluminescence was monitored for an additional 300–500 s according to the appropriate experimental pHs 5, 7, and 9. At the end of each experiment the remaining amount of aequorin was determined by adding equal volumes of discharge buffer (100 mM of CaCl2, 5.0% v/v Triton X-100) as described by Domínguez et al. (2011) to determine the total light output. The total chemiluminescence represented by the available aequorin was used to calculate the concentration of cytosolic Ca2+. To determine the possible effect of pH on aequorin activity, cell lysates of S. aureus, expressing apoaequorin, were reconstituted with coelenterazine as described before. Aliquots of 100 μl were treated with each chemical using the same concentrations as those used in the treatments (different pHs). After each treatment, aliquots were taken to the luminometer and aequorin was completely discharged by adding an equal volume of 100 mM CaCl2 and the discharge buffer to determine the total light output. To rule out the possibility that the luminescence obtained would be due to aequorin being released into the medium by lysed cells or lysis of cells due to addition of calcium, light was measured after addition of Ca2+ to the medium in which reconstituted cells were present after removing the cells by centrifugation. We also checked for cell lysis by microscopy. Negative control used for these experiments were cells without the apoaequorin plasmid. Relative light units were converted to μM Ca2+ concentrations utilizing a matrix created by Dr. Anthony Campbell [pCa = 0.612(−logk) + 3.745 where k is the rate constant for decay of chemiluminescence (s−1)] (Jones et al., 1999).
Antibiotic Signaling Studies
The antibiotics used for the signaling experiments included: erythromycin, gentamicin, kanamycin, vancomycin, streptomycin, and ciprofloxacin. To determine the MIC for each antibiotic, S. aureus cells containing the aequorin plasmid were grown overnight in BHI then subcultured into Mueller-Hinton and grown to an OD600 0.8. The culture was then adjusted to a 0.5 McFarland standard (aprox. 108 CFU/mL) in 5 mL. The MIC was determined using MicroScan autoSCAN-4 automated system using MIC panels for Gram positive bacteria PBCP20. To measure antibiotic stimulus-response, S. aureus cells were grown as stated above. S. aureus cells containing the aequorin were loaded into a microtiter plate and basal intracellular Ca2+ was monitored for 10 s followed by injection of each antibiotic to a final concentration of 0.5 μg/mL in a final volume of 100 μl. Intracellular Ca2+ was then monitored for an additional 250 s. We used a standard concentration for all antibiotics to detect unique responses of cytosolic Ca2+ transients elicited by each antibiotic. This allowed for elimination of concentration as a variable affecting cytosolic responses.
Efflux Experiments: Effect of Ca2+ and Ca2+ Inhibitors in S. aureus
Efflux pump activity was measured indirectly and directly in S. aureus cells. In the indirect method bacterial cells were pre-incubated in (EtBr) and Ca2+ or a Ca2+ inhibitor before the beginning of the assay. Fluorescence was monitored after incubation for 20 min. Fluorescence increases as the EtBr accumulates within the cells until it reaches a steady state of efflux/accumulation. In the direct method, bacterial cells were incubated with dye in the presence of an inhibitor such as the proton decoupler CCCP, then washed to remove excess dye and inhibitor. Fluorescence was measured for 5 min after which 1 mM of Ca2+ was injected into the media. Efflux was then measured for an additional 20 min. The purpose of this is to observe the rate of efflux of EtBr that is inside the cells and to record the change in slope as they respond to the addition of external Ca2+.
Indirect measurements. To determine the effect of Ca2+ in the efflux systems of S. aureus, EtBr was used as a substrate, and cells were treated with various concentrations of CaCl2, Ca2+-chelators, and Ca2+-dependent inhibitors. The MIC for EtBr was done using the broth dilution method and as recommended by the Clinical Laboratory Standards Institute. A final concentration of 2.5 mg/L EtBr was used for both direct and indirect efflux experiments. Bacterial strains were cultured overnight in BHI broth at 37°C in a Gyromax rotatory shaker (Amerex Instruments) at 220 rpm. Overnight cultures (1:100) were transferred into 250 ml triple-baffled flasks containing 50 mL fresh BHI broth and grown to reach an OD600 of 0.8. Bacterial cells were harvested by centrifugation at 5000 rpm for 5 min (Beckman Coulter Allegra, rotor C0650). Cells were washed three times in Phosphate buffer solution PBS (137 mM NaCl, 10 mM phosphate, 2.7 mM KCl) adjusting to the different experimental pHs 5.0, 7.0, and 8.0 (pH for efflux experiments was adjusted at 8.0). Bacterial cell concentration was adjusted to an OD600 of 0.6 for each pH. Experimental procedures for EtBr efflux were done following the protocols of Couto et al. (2008), Martins et al. (2011) with modifications. Briefly, fluorescence of EtBr was measured over time to observe the decrease in fluorescence as the cells excreted EtBr. Cells were incubated in 2.5 mg/L EtBr. Bacterial cells in aliquots of 100 μL were placed in 96 microtiter plates and efflux pump activity of EtBr was measured by fluorescence at 585 nm in 96 microtiter fluorescent-based plates (Thermo Fischer Scientific) using a GloMax 3000 Fluorometer (Promega). To assess the effect of Ca2+ on efflux, media calcium levels were manipulated by addition of CaCl2 (Sigma-Aldrich) to a final concentration of 1.0 and 5.0 mM. Lack of calcium was also investigated by the addition of Ca2+-chelators, EGTA, and EDTA (Thermo Fisher), which were added at final concentrations of 5 and 10 mM. To investigate Ca2+-dependent transport inhibitors, the phenothiazines, CPZ and TFP (Sigma-Aldrich), were added to final concentrations of 0.1 μM and 30 μM, respectively. The Ca2+ channel blocker, verapamil (Sigma-Aldrich) was added at 30 μM. Efflux assays were conducted at the different pHs, 5.0, 7.0, and 8.0. Glucose (0.4%) was used as control for the contribution of metabolic energy to efflux. CCCP (Sigma-Aldrich), a proton uncoupler was used to a final concentration 50 μM to illustrate proton motive force disruption.
Direct measurements. For direct efflux experiments, the cells were grown and cultured as described before. Briefly, the cells were grown overnight in BHI broth at 37°C. The cells were subsequently washed and pelleted twice in PBS and adjusted to a final OD A600 of 0.6 and incubated in EtBr at 2.5 mg/L and the indicated Ca2+ inhibitor. The cells were then pelleted by centrifugation and the supernatant containing excess EtBr and treatment was removed. The cells were then resuspended in PBS at the appropriate pH. 100 μl were loaded into a 96 microtiter plate and fluorescence was monitored for 5 min at 585 nm to establish basal levels. After 5 min, CaCl2 was added to a final concentration of 1 mM and fluorescence was monitored for an additional 20 min.
To investigate if Ca2+ had the ability to restore efflux activity inhibited by EDTA, and alkaline pH of 8, S. aureus cells were grown overnight in BHI following the methods of Martins et al., 2011. The cells were sub-cultured in a 1:100 ratio and grown for an additional 2 h. The cells were then washed and pelleted twice and re-suspended in PBS at pH 8. The OD A600 was adjusted to 0.6 and EDTA and EtBr were added to a final concentration of 5 mM and 2.5 mg/L, respectively. Fluorescence was then measured at 585 nm for 20 min. After 20 min the experiment was stopped and cells were treated with one of the following: CPZ (0.1 μM), CPZ + CaCl2 (0.1 μM, 1 mM), and CaCl2 (1 mM) in a final volume of 100 μl, loaded to a 96 well microtiter plate. Fluorometry was resumed for additional 20 min.
Cloning of lmrS Gene
To investigate further the effects of Ca2+ on an individual S. aureus efflux pump, the lmrS gene was amplified and cloned into the expression vector pRMC2 (Corrigan and Foster, 2009) using the primers designed by Floyd et al., 2010. Forward: GCAAGCTTATGGCTAAAGTTGAATTAACAAC and Reverse: GCGGATCCTTAAAATTTCCTTCTATTACTTT and transformed into E. coli cells strain DH5-α. The following thermocycler parameters were used: 95°C 1 min, 35 cycles of 95°C 30 s, 51°C for 1 min followed by 1 min of extension at 72°C and a final extension step for 5 min at 72°C. Direct efflux activity of both E. coli-lmrS and E. coli DH5α was measured as previously described with an EtBr concentration of 1 mg/L. Cells not incubated in EtBr were also used as a control. Briefly, the cells were incubated for 30 min in EtBr and inhibitor. The cells were subsequently pelleted by centrifugation, washed twice and resuspended in PBS buffer. Fluorescence was then measured as described above. After 5 min cells were injected with 1 mM of CaCl2. Fluorescence was then monitored for an additional 20 min.
MIC of the E. coli-lmrS and E. coli DH5α
Both the wild-type and E. coli-lmrS were grown in LB broth and then subcultured into Mueller-Hinton broth and grown to an OD600 0.8. The culture was then adjusted to a 0.5 McFarland standard (aprox. 108 CFU/mL) The MICs for the antibiotics tested were determined using the MicroScan autoSCAN-4 automated system using PBCP34 panels.
Statistical Methods
The Kruskal–Wallis test was utilized to determine the differences in Ca2+ treatments (CaCl2 0.5, 1.0, and 5.0 mM), Ca2+ inhibitor treatments (phenothiazines, verapamil, and Ca2+ chelators) compared to controls in fluorescent assays. The same test was used to determine significant differences in intracellular Ca2+ levels at concentrations: 0.5 mM, 1.0 mM, and 5.0 mM. To determine significant differences of cytosolic Ca2+ at each pH (5.0, 7.0, and 8.0) a one sample t-test and Wilcoxon test was conducted. Both the efflux and cytosolic free Ca2+ experiments were analyzed using the statistical and graphics software, Graph Pad 8.0.
Results
S. aureus Cells Maintain Ca2+ Homeostasis
The assumption that Ca2+ acts as a messenger in prokaryotes is based on the observation that environmental signals induce changes in the level of cytosolic free Ca2+. Therefore, measurement of [Ca2+]i is essential in establishing that Ca2+ might serve as an intracellular signal (Jones et al., 1999; Torrecilla et al., 2000; Campbell, 2015). Based on this premise, we examined the ability of S. aureus to maintain Ca2+ homeostasis and the response of [Ca2+]i to environmental changes such as the presence of antibiotics and pH shifts. We used recombinant S. aureus cells constitutively over-expressing the photoprotein aequorin to measure cytosolic free Ca2+. Increasing concentrations of external CaCl2 (as described in “Materials and Methods”) showed an increase in luminescence directly proportional to the amount of CaCl2 injected followed by a rapid decline within seconds (Figure 1A). Conversely, cells treated with Ca2+ chelators, such as EGTA showed a sharp decline in cytosolic [Ca2+]i as evidenced by low luminescence (all these results were statistically significant) (Figure 1B). These results demonstrate that S. aureus maintains cytosolic Ca2+ concentrations in the μM range in the presence of CaCl2, in the culture broth of 0.5–5 mM, which is consistent with other studies (Jones et al., 1999; Torrecilla et al., 2000, 2001; Domínguez et al., 2011; Guragain et al., 2016). The effect of pH was also examined since S. aureus MFS and MATE transporter families have been shown to use electrochemical gradients and proton motive force as the driving force for extrusion of toxic compounds (toxic compound/H+) (Santos Costa et al., 2013; Jang, 2016). External CaCl2 was added to a final concentration of 1 mM and the pH was adjusted to pH 5, 7 or 9 (as described in “Materials and Methods”) and luminescence was measured as before. A rapid cytosolic Ca2+ transient was observed after injection of 1 mM CaCl2 with a fast decline to basal levels. However, cultures maintained at differing pH values had differing cytosolic Ca2+ showing different amplitudes (peaks) (Figure 1C). At pH 9, free cytosolic Ca2+ rose to 23 μM whereas at pH 7 it was 17 μM and at pH 5 it was 9.5 μM. These results imply that H+ is competing for Ca2+ influx, perhaps directly at the Ca2+ site of the transporter or perhaps by some other mechanism. When EGTA (5 mM) was added after the injection of 1 mM CaCl2 the removal of Ca2+ caused a sharp decline to basal levels (Figure 1D). S. aureus cells without the aequorin plasmid were used as a negative control. Our results indicated that S. aureus maintains cytosolic Ca2+ in the micro-molar range in the presence of 0.5–5 mM external CaCl2. These findings are the first intracellular Ca2+ measurements reported in S. aureus.
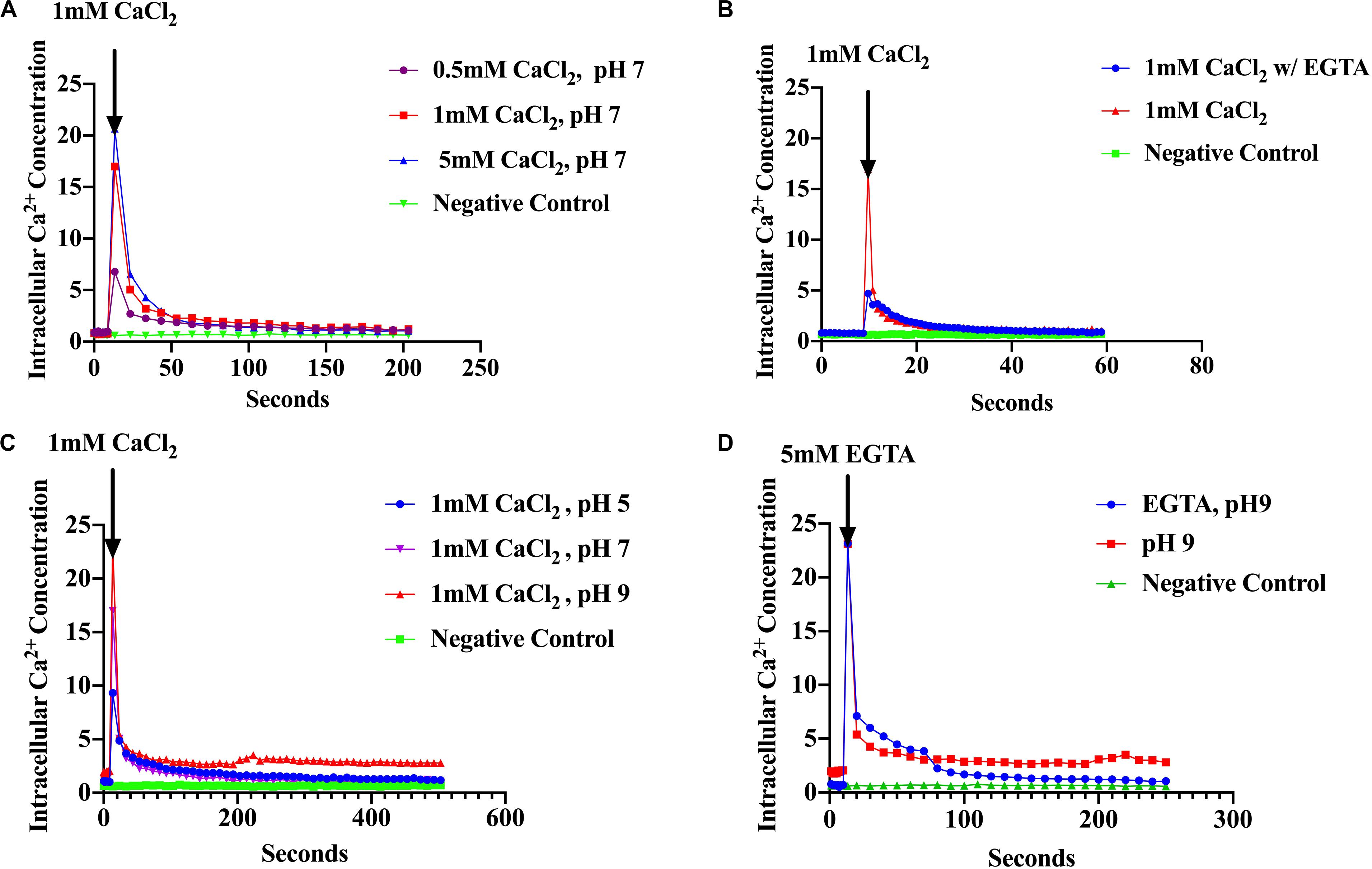
Figure 1. S. aureus cells maintain Ca2+ homeostasis. (A) Cytosolic Ca2+ transient in response to various CaCl2 concentrations: 0.5, 1.0, and 5.0 mM (B) Cytosolic Ca2+ transients after addition of 1 mM CaCl2 and the Ca2+ chelator EGTA (C) Cytosolic Ca2+ transients in response to different pH 5, 7, and 9 (D) Cytosolic Ca2+ transients at pH 9 and addition of EGTA. S. aureus cells without aequorin were used as the negative controls. The results represent the means of three independent measurements. Significant peak differences were observed in response to pH as well as Ca2+ levels. A student t was conducted to evaluate treatments (p < 0.0001).
Unique Ca2+ Signals in Response to Antibiotics in S. aureus Cells
The immediate response to changes in environmental conditions is crucial for organisms to adapt and survive. Bacteria sense environmental conditions through transients in cytosolic Ca2+ (Herbaud et al., 1998; Torrecilla et al., 2000; Naseem et al., 2009). Similar to eukaryotic cells, cytosolic Ca2+ transients are very dynamic and vary in shape, amplitude, speed, and spatiotemporal patterns. Several studies showed that exposure to antibiotics have been linked to gene expression including genes related to virulence, biofilm formation, and transporters (Nichols et al., 2011; Romero et al., 2011; Du et al., 2018). Here we investigated the stimulus-response of S. aureus cells in sensing the presence of various antibiotics. S. aureus cells expressing the photoprotein aequorin were loaded into microtiter plates and basal [Ca2+]i was measured for 10 s followed by injection of selected antibiotics (as indicated in the “Materials and Methods” section). Antibiotics used in this study included: erythromycin, gentamicin, kanamycin, vancomycin, streptomycin, and ciprofloxacin. Concentrations of antibiotics were adjusted according to a standard concentration described in “Materials and Methods” section. All antibiotics tested elicited a rapid increase in cytosolic Ca2+ (Figure 2). The [Ca2+]i response to the antibiotic was very rapid but varied dramatically in amplitude, shape, oscillation pattern, and duration (Table 1) as a function of the antibiotic used. The amplitude of the transient ranged from 12.8 μM Ca2+ for erythromycin to 3.4 μM Ca2+ for vancomycin. The transient shape differed considerably, some showing oscillations and/or a second peak before reaching basal levels. These results indicate that there is a Ca2+ mediated signal response to antibiotics in S. aureus and that cells have the ability to differentiate among types of antibiotics, which may trigger further physiological reactions such as gene expression, for cellular adaptation. It is possible that the [Ca2+]i response might provide information as to the cells potential to become drug resistant.
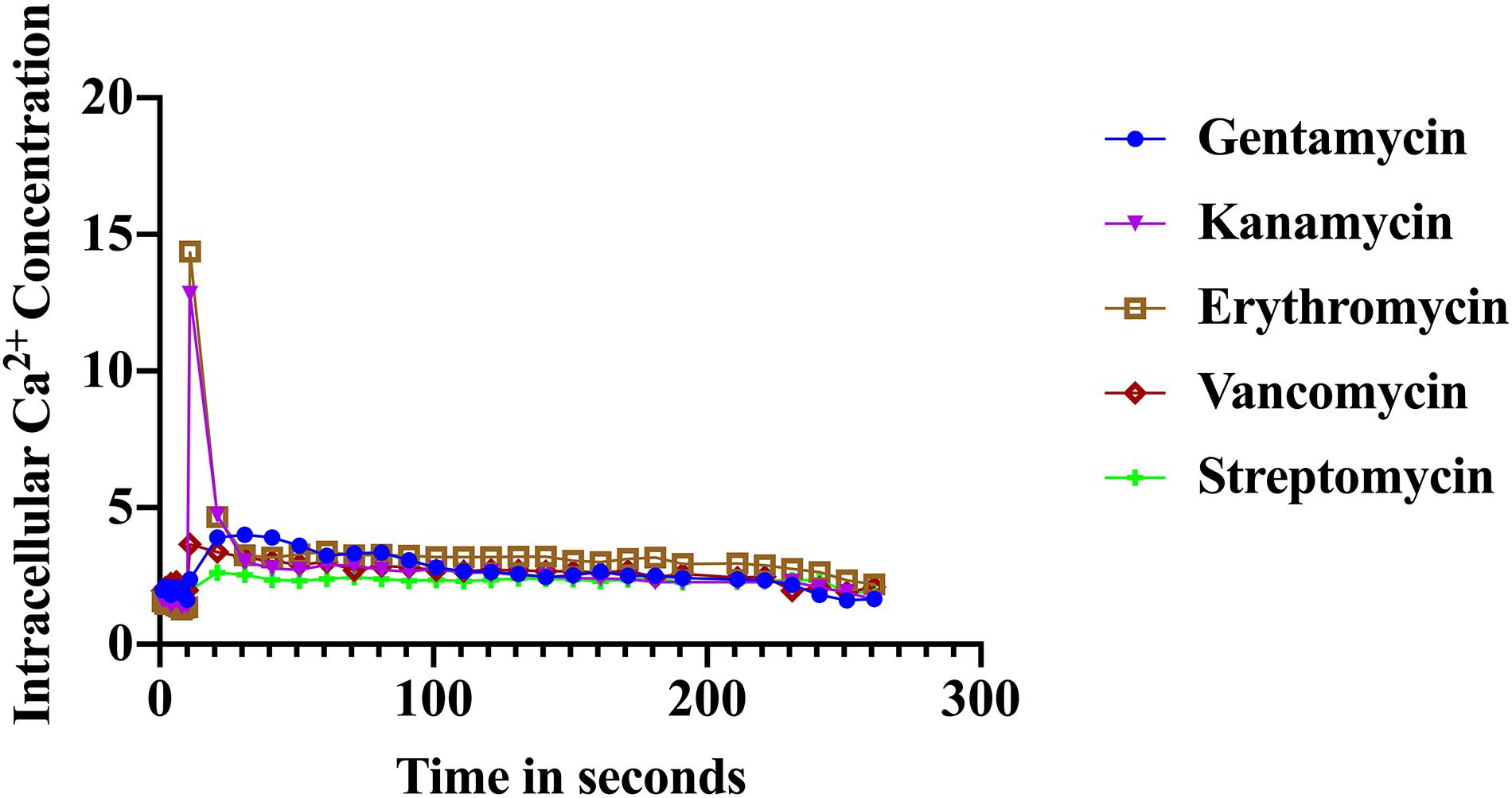
Figure 2. Calcium transients in S. aureus cells induced in response to various antibiotics. The results represent the means of three separate determinations.
Effect of Ca2+ and Ca2+ Inhibitors in S. aureus Efflux of EtBr
Measurements of EtBr accumulation were done to assess efflux activity in S. aureus cells by fluorescence. The premise is that the higher the efflux, the lower the concentration of EtBr accumulated within bacterial cells resulting in lower fluorescence. Conversely, accumulation of EtBr within cells is indicated by higher fluorescence. Efflux pump activity was measured directly and indirectly in S. aureus cells. In the direct method bacterial cells were pre-incubated with EtBr in the presence of an inhibitor of the Ca2+ or pump energy source at the appropriate pH, then subsequently washed and fluorescence was measured. In the indirect assays fluorescence was recorded after the pre-incubation period with EtBr and the treatment.
To evaluate the effect of Ca2+ on the efflux of EtBr, cells were incubated in various concentrations of CaCl2 and EtBr, with or without 0.4% glucose. The addition of glucose was used as a control to distinguish the effects of Ca2+ from that of metabolic energy. In addition, samples were incubated in various conditions with one of the following, CaCl2, Ca2+-chelator EGTA and the phenothiazines, chlorpromazine (CPZ) and trifluoperazine (TFP), which are known to inhibit efflux pump activity in various pathogens including S. aureus (Naseem et al., 2008; Koul et al., 2009; Martins et al., 2011; Pule et al., 2016). The phenothiazines are known to bind to Ca2+-binding proteins and to target prokaryotic cell membranes (Kaatz et al., 2003; Amaral et al., 2010). These drugs affect Ca2+ related processes such as Ca2+ binding to transporters and Ca2+ dependent enzymes involved in ATP hydrolysis thus inhibiting Ca2+ influx and as a consequence, the Ca2+ signal. The Ca2+ channel blocker, verapamil, which inhibits Ca2+ influx/efflux, and the calmodulin inhibitor calmidazolium (CDZ), which is involved in modulation of pumps in eukaryotes, were also used in this study (Couto et al., 2008; Bishai et al., 2013). Efflux activity was monitored at pH 7 according to the protocols of Martins et al., 2011 with modifications. As shown in (Figures 3A–D) addition of EGTA, TFP, Ver, and CDZ showed significant increase in the relative fluorescence (RF), indicating accumulation of the EtBr compared to CaCl2 treated cells and control cells (cells without EtBr and cells with EtBr no treatment), which showed low fluorescence. Assays in panel A were conducted at slightly higher (pH 7.6) than panels B-D, showing a slight effect in efflux. A Kruskal–Wallis statistical analysis was used to determine significant differences. Each of the inhibitor treatment was significantly different as compared to 1 mM of Ca2+ and to the positive control where the cells were incubated in EtBr alone (data not shown). Each treatment represents the average of three replicates with the mean and ±SD (p-value < 0.001). These results show that Ca2+ ions have the ability to enhance efflux in S. aureus cells. Since proton availability and metabolic energy affect efflux, measurements were also done at different pHs. At pH 5 (Figure 4A) where H+ is higher, efflux activity is more efficient compared to efflux at pH 8 where proton availability is lower (Figure 4B).
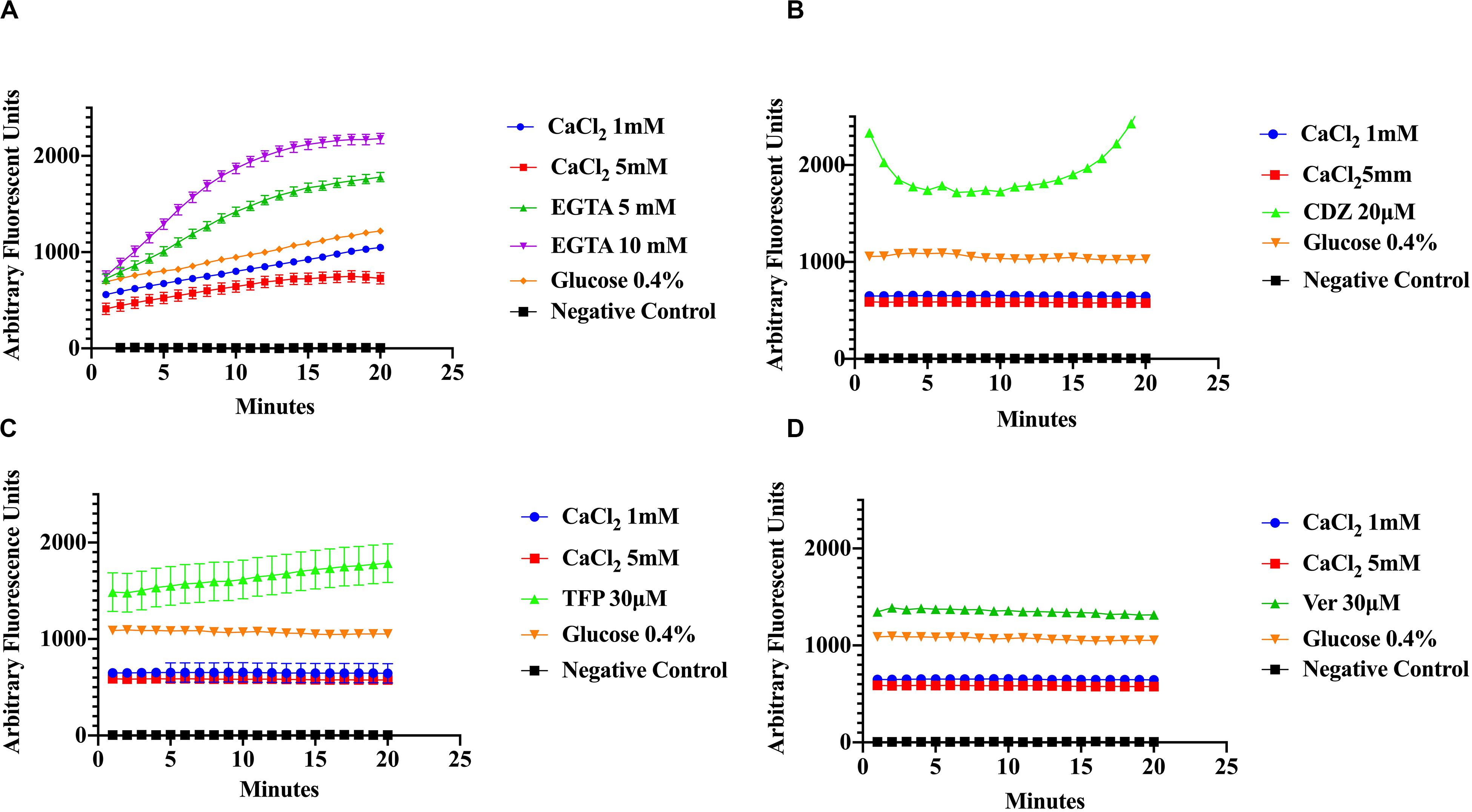
Figure 3. Ethidium bromide accumulation in response to calcium and calcium inhibitors at pH 7. S. aureus cells were preincubated in the presence of Ca2+ (1 mM and 5 mM), Ca2+ chelator EGTA (5, 10 mM), the phenothiazine trifluoperazine (TFP 30 μM), the Ca2+-channel blocker verapamil (Ver 30 μM), and the Ca2+-Calmodulin inhibitor Calmidazolium (CDZ 20 μM). The results show that calcium enhances the efflux of Ethidium Bromide in S. aureus (A) effect of CaCl2, EGTA, and glucose (4%) on ethidium bromide accumulation at pH 7.6 on S. aureus. (B) The effect of CaCl2, CDZ, and glucose on ethidium bromide accumulation at pH 7 on MRS A cells. (C) Effect of CaCl2, TFP and glucose on ethidium bromide accumulation (pH 7). (D) The effects of Ver, CaCl2, and glucose on EtBr efflux (pH 7). The results represent the average of three replicates at each time point. Significant differences were seen between calcium, inhibitor treatments and controls (p < 0.0001) Kruskal–Wallis, non-parametric.
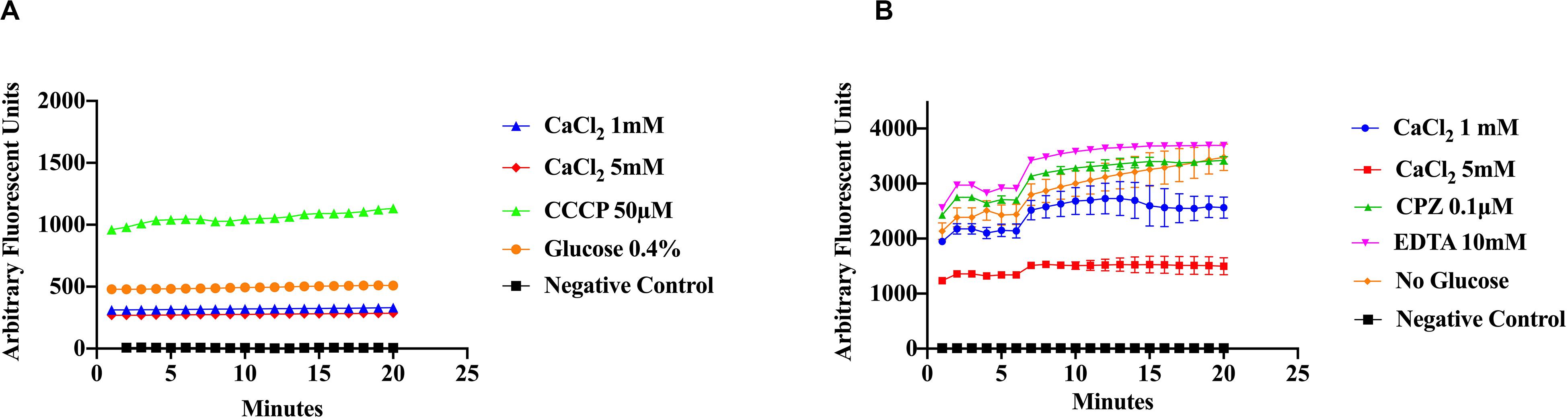
Figure 4. Ethidium bromide accumulation in response to CaCl2, Ca2+-inhibitors and a proton uncoupler at pH 5 and pH8. Efflux activity of S. aureus cells was measured under different pH conditions, calcium and inhibitors. (A) S. aureus cells were preincubated in the presence of two Ca2+ concentrations (1 mM and 5 mM), glucose (0.4%), and CCCP (50 μM) was added to disrupt the electrochemical gradient (pH 5). (B) EtBr efflux measured at pH 8, with CaCl2 (1 mM and 5 mM), 0.4% glucose, the phenothiazine CPZ (0.1 μ), and EDTA (10 mM). The results represent an average of three replicates. Significant differences were found between the treatments using a non-parametric Kruskal–Wallis test (p < 0.0001).
As shown previously (Figure 3), addition of CPZ, EDTA or EGTA resulted in increased EtBr accumulation in S. aureus cells. Here we investigated whether the addition of CaCl2 could restore efflux activity suppressed by the Ca2+ antagonist CPZ (0.1 μM) and the Ca2+ chelator EDTA. We also examined the effect of adding both CPZ and CaCl2 together on efflux of EtBr. Our results showed that addition of 1 mM CaCl2 decreased the accumulation of EtBr caused by the CPZ. However, when CPZ + CaCl2 were added the decrease in fluorescence was less pronounced (Figure 5). These results imply that Ca2+ has the ability to restore efflux activity in S. aureus cells. These findings are consistent with those reported in E. coli (Martins et al., 2011).
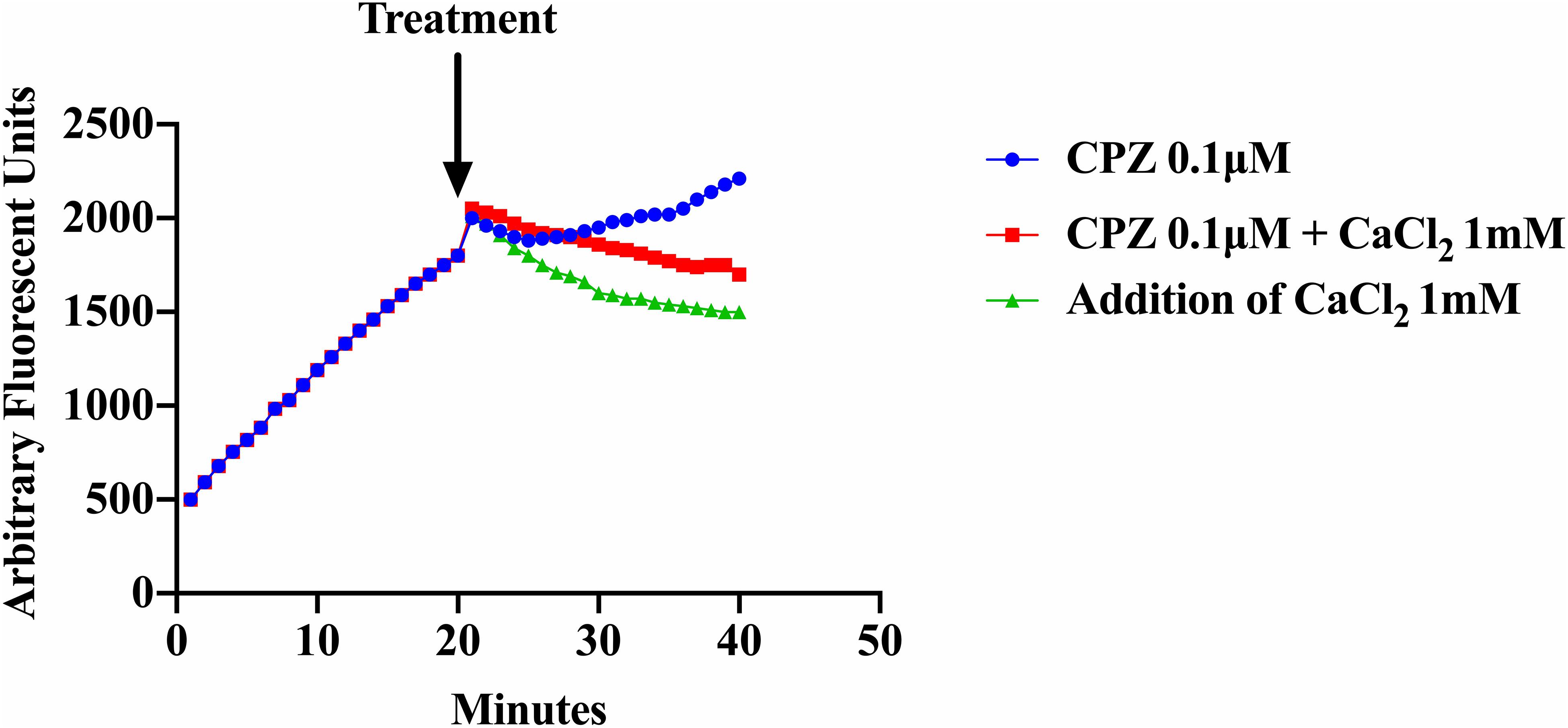
Figure 5. Effect of CPZ, CPZ + CaCl2, and CaCl2 on the accumulation of EtBr in S. aureus cells. Cells were incubated with EtBr and EDTA at a pH of 8 and fluorescence was measured at 585 nm for 40 min. Measurements were halted briefly after 20 min and three treatments were added to a final concentration of 0.1 μM of CPZ, 0.1 μM CPZ + 1 mM CaCl2, and 1 mM CaCl2 and to a final volume of 100 μl and added to a 96 well microtiter plate. Fluorescence measurements were then resumed for an additional 20 min. The addition of CPZ resulted in further EtBr accumulation (increased fluorescence) whereas the addition of CPZ + CaCl2 reduced accumulation of EtBr compared to CPZ alone. The addition of 1 mM CaCl2 resulted in the highest increase of efflux (decreased fluorescence) of EtBr. These results represent the average of three independent biological replicates. The results suggest that calcium restored efflux activity in an environment where protons are lost to high alkalinity and metals are chelated from solution.
Calcium Enhances Efflux of Ethidium Bromide (EtBr) by LmrS
In order to evaluate the effect of Ca2+ in the efflux of EtBr more directly, we cloned the S. aureus multi-drug resistant pump lmrS gene into E. coli cells using the pRMC2 vector (as described in “Materials and Methods” section). The LmrS (lincomycin resistance protein of S. aureus) belongs to the MFS superfamily, which transport diverse molecules across the membrane using an electrochemical gradient. The lmrS gene was identified by Floyd et al. (2010). The lmrS gene was amplified and cloned as described in “Materials and Methods.” To investigate if recombinant E. coli cells (DH5α-lmrS) have acquired resistance through the incorporation and overexpression of the lmrS gene, we evaluated the susceptibility of the recombinant E. coli cells to various antibiotics by determining the MIC using the micro broth dilution method (“Materials and Methods”). The results indicate that the E. coli cells carrying the lmrS gene were resistant to all antibiotic tested in contrast to E. coli cells without the lmrS gene (Table 2).
Differences of EtBr Efflux Between E. coli DH5α Cloned LmrS (DH5α-LmrS)
Efflux of EtBr was assessed by direct EtBr assays (“Materials and Methods”). Recombinant E. coli cells (DH5α-lmrS) were incubated in EtBr (2.5 mg/L). Cells were pelleted and washed twice with PBS and fluorescence was monitored for 5 min at 585 nm. After 5 min 1 mM CaCl2 was added and efflux was monitored for an additional 30 min. E. coli cells without LmrS were also measured as control. Significant differences in efflux (Kruskal–Wallis test) were observed between recombinant E. coli cells (DH5α-lmrS) and E. coli cells (DH5α) without the lmrS pump (Figure 6). The expression of lmrS significantly increases efflux as indicated by the lower fluorescence values.
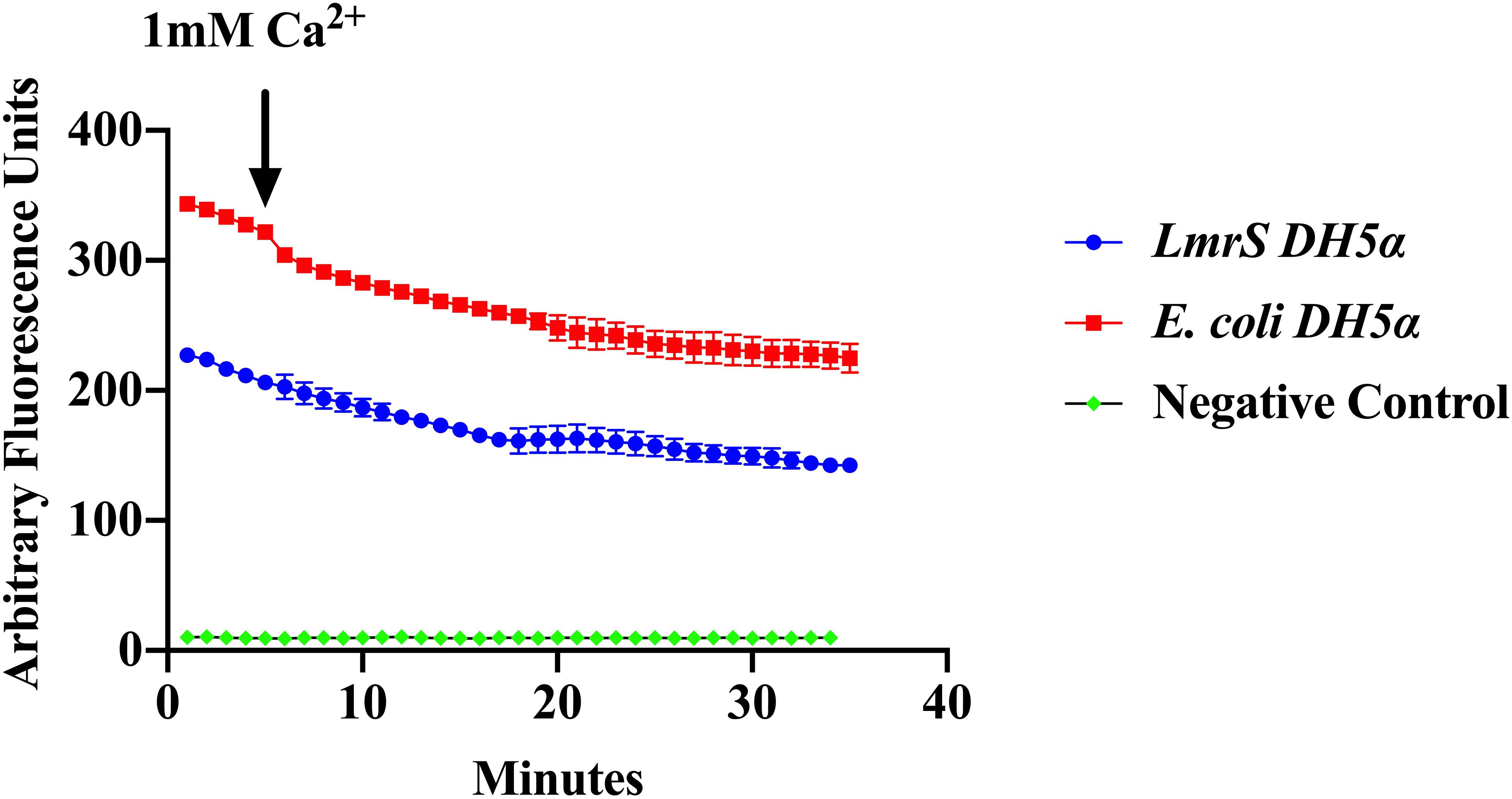
Figure 6. Ethidium bromide efflux measurements in E. coli DH5α cells carrying the lmrS gene. Direct measurements of EtBr assays in E. coli cells carrying the lmrS gene and cells without lmrS gene (control) were conducted as described in “Materials and Methods.” The results show that the addition of 1 mM CaCl2 enhances efflux of EtBr in the cloned LmrS, indicated by the lower fluorescence values as well as cells without the cloned lmrS gene. Control cells contained no EtBr. Results show the mean of three independent biological replicates. A Kruskal–Wallis test was utilized to detect significant differences in efflux between the E. coli DH5α wild-type and the E. coli recombinant strain (p < 0.0001).
Effect of Ca2+ Chelator EGTA and CPZ in Cloned LmrS
The effect of the Ca2+ chelator EGTA and the phenothiazine chlorpromazine (CPZ) were also evaluated as described in “Materials and Methods.” The cells were pre-incubated in the Ca2+ chelator, EGTA (Figure 7A) and the inhibitor, CPZ (Figure 7B) which resulted in an increased accumulation and higher fluorescence in both the wild-type and recombinant E. coli cells when compared to Figure 6 where no Ca2+ inhibitors or chelators were added. A Kruskal–Wallis analysis indicated significant efflux differences between the recombinant and wild-type E. coli cells (Figure 7). The ability of the recombinant E. coli cells to efflux EtBr more efficiently was indicated by the significant decrease in fluorescence as compared to the wild-type E. coli. After 5 min, 1 mM of Ca2+ was injected into the media and a decrease in fluorescence was observed in both the recombinant and wild-type E. coli cells indicating an enhancing effect on efflux.
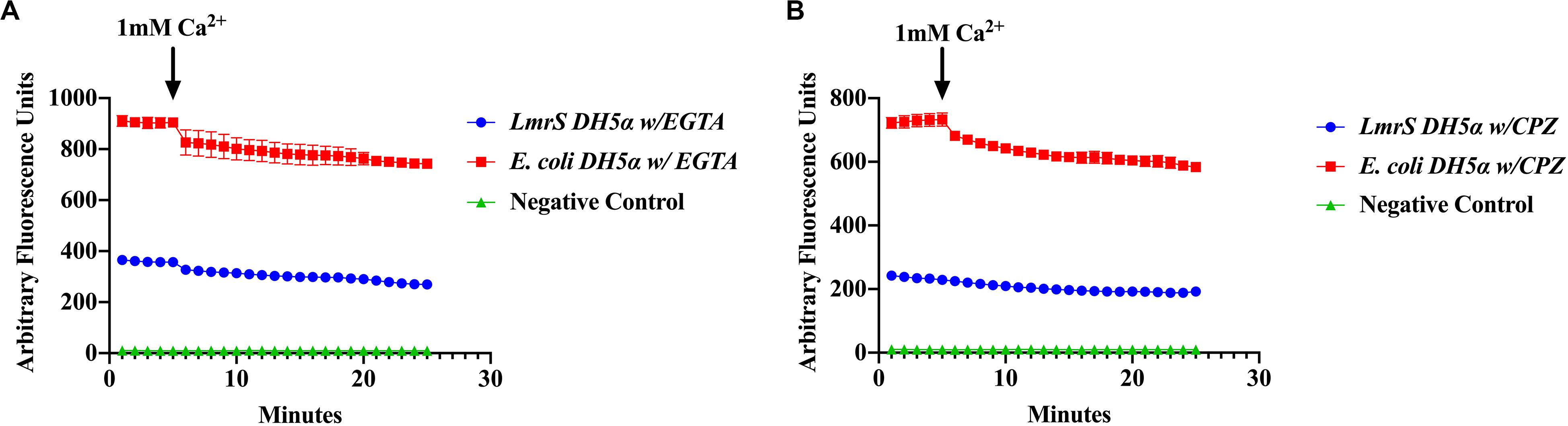
Figure 7. The calcium chelator EGTA (A) and the phenothiazine chlorpromazine (CPZ) (B) inhibit efflux of EtBr by LmrS. Recombinant E. coli cells and E. coli cells without the LmrS gene (used as control) were assayed by direct EtBr assay as described in the “Materials and Methods” section. The results represent the mean of at least three biological replicates. Each time point represents the average mean ± SD. A Kruskal–Wallis test was utilized to detect significant differences between the Ca2+ inhibitor treatments and without inhibitors. Significant differences were observed in both the E. coli DH5α and the recombinant E. coli strain as well as significant differences in efflux between the strains (p < 0.0001).
Discussion
The results presented in this study demonstrate that S. aureus cells maintain a tight control of cytosolic [Ca2+]i, which is a pre-requisite for its cell signaling. We also present evidence that various antibiotics induce Ca2+ mediated responses exhibiting unique and diverse spatial and temporal patterns. Moreover, here we demonstrate for the first time, that extrusion of EtBr by the multidrug transporter LmrS is enhanced by Ca2+.
Measurements of intracellular Ca2+ have been documented in several genera of bacteria but here we report for the first time, cytosolic Ca2+ measurements in an important pathogen, S. aureus (Domínguez, 2004; Domínguez et al., 2011). In agreement with previous studies, our results show that S. aureus maintains [Ca2+]i in the micromolar range in the presence of 0.5–5 mM external CaCl2. S. aureus cells responded rapidly to increases in the external Ca2+ concentration, while addition of the Ca2+ ion chelator EGTA produced a sharp decline. The maintenance of the low cytosolic Ca2+ is not only required by all cell types to protect them from the toxic effects of high cytosolic Ca2+ but also, to be able to use Ca2+ as a cellular signal. Any increase in cytosolic Ca2+ due to the transmission of the signal, must disappear quickly in order for the next signal to occur (Carafoli, 1987; Torrecilla et al., 2000).
The observation that Ca2+ transients increased significantly as pH values increased (from 5, to 7 to 9) suggests that S. aureus cells sense pH environmental conditions through changes in cytosolic Ca2+. These signals are essential for the organism to adapt and survive under wide variety of conditions, in the human host, during infection and colonization and in different environments. pH plays an important role in skin colonization, wound healing and immune cell chemotaxis. Moreover, the phagosome-lysosome environment is highly acidic. S. aureus must have sensor systems in response to the acidic conditions to adjust gene expression for survival (Venditri et al., 2003; Martins et al., 2008). Most Ca2+ exchangers in prokaryotes utilize Ca2+/H+ and Ca/Na+ gradients to transport Ca2+ into the cell via an electrogenic mechanism (Domínguez et al., 2015). The short Ca2+ transient (influx) developed at pH 5 (9.5 μM Ca2+) suggests proton competition for translocation of Ca2+ into the cell compared to pH 9 (23 μM Ca2+). These findings are in agreement with those presented by Naseem et al. (2008) in E. coli where a pH of 5 resulted in lowest luminescence. Our Ca2+ efflux results, however, differ slightly to those reported by Naseem et al. (2008). While Ca2+ decline was fast for the three pHs, at pH 9, Ca2+ levels did not reached basal levels compared to pH 5 and 7. It is interesting to note that pH triggers gene expression in various bacteria (Weinrick et al., 2004; Perez and Groisman, 2007; Martins et al., 2009; Serra-Cardona et al., 2015) including genes involved in cell envelope structure, ion transporters and multidrug transporters (Tucker et al., 2002; Weinrick et al., 2004; Hayes et al., 2006; Truong-Bolduc et al., 2011). However, the regulatory pathways by which bacteria sense and respond to pH stimuli have not been elucidated. The work presented here is of significance as it strongly suggests a role of Ca2+ in the regulation of genes encoding bacterial transporters or other proteins affecting efflux pumps in the response to antibiotics.
Exposure of different types of antibiotics to S. aureus-aequorin cells elicited immediate [Ca2+]i transients with unique properties for each antibiotic suggesting that S. aureus cells have the ability to sense and distinguish different classes of antibiotics. Interestingly, antibiotics within the same group such as gentamicin and kanamycin (aminoglycosides) showed different transients. Rapid increases in cytosolic Ca2+ in response to environmental pollutants and antibiotics have also been reported in Saccharomyces cerevisiae (Ruta et al., 2014; Farcasanu et al., 2018) and in the Cyanobacteria Anabaena, which also triggered specific Ca2+ signatures (Barrán-Berdón et al., 2011; González-Pleiter et al., 2017).
As previously stated, Ca2+ plays a pivotal role in many metabolic pathways including transport systems and are essential for signaling. The antimicrobial properties of the phenothiazines have been described since 1930 and 1940 (Grimsey and Piddock, 2019). The ways by which phenothiazines exert their antimicrobial effect include, damaging cell membranes, inhibiting Ca2+-dependent processes, disrupting cation-dependent transporters, acting as antagonist of Ca2+-binding proteins and in other ways (Grimsey and Piddock, 2019). Therefore, we investigated the effect of Ca2+ on the efflux of EtBr first by analyzing the effect of various divalent metals (Supplementary Material). CaCl2 showed a significantly greater effect on EtBr efflux compared to KCl2, MgCl2, and ZnCl2. Further evidence of the effect of Ca2+ on EtBr efflux was investigated by the addition of Ca2+ chelators, EGTA (higher specificity for Ca2+ binding) and EDTA, various efflux pump and Ca2+ binding proteins inhibitors such as the phenothiazines CPZ and TFP, which all had a strong inhibitory effect on efflux and accumulation of EtBr. The reversal of efflux inhibition by addition of Ca2+, indicated by the levels of fluorescence, showed the significant role that Ca2+ play in S. aureus efflux systems. These results are consistent with previous studies (Kaatz et al., 2003; Martins et al., 2009, 2011) and may have an impact in the development of novel inhibitors to combat antibiotic resistance.
Efflux transport systems are required for S. aureus survival in a wide range of environmental conditions, including high and low pH systems (Truong-Bolduc et al., 2011; Dastgheyb et al., 2015; Rippke et al., 2018). The efflux of EtBr was measured at different pHs (5, 7, and 9). The efflux of EtBr showed significant differences between the pHs and when glucose was added. In general, protons contributed to the ability of S. aureus to efflux EtBr. These results agree with those reported by Couto et al. (2008), Martins et al. (2011), which demonstrated differences in efflux due to pH and showed that proton-motive force is a significant contributor to efflux in E. coli. This was also observed in the present study as significant accumulation of EtBr was observed when CCCP, a proton un-coupler, was added at pH 5.
Cloning of the lmrS gene into E. coli conferred resistance to all antibiotics tested as compared to E. coli cells without the gene and efflux of EtBr was higher in E. coli cells carrying the lmrS gene than control cells. The effects of Ca2+ enhancing efflux of EtBr were corroborated in E. coli-lmrS by addition of Ca2+ and Ca2+ inhibitors consistent with our findings in S. aureus cells.
In conclusion these findings show that Ca2+homeostasis is maintained in S. aureus cells, that antibiotics induce a unique Ca2+ mediated response through transients in cytosolic Ca2+ and that the LmrS efflux pump is enhanced by Ca2+. Certainly, new therapeutic approaches are urgently needed to reduce the demand for antibiotics and to combat antimicrobial resistance. These studies suggest that further analysis of the effect of Ca2+ on efflux pump regulators, elucidation of their mechanism of action and control of their gene expression might lead to the development of novel efflux pump inhibitors, which could mitigate the antimicrobial resistance crisis.
Data Availability Statement
The raw data supporting the conclusions of this article will be made available by the authors, without undue reservation.
Ethics Statement
This study was approved by The University of Texas at El Paso (UTEP) Institutional Biosafety Committee and all protocols were done according to the rules and regulations of the Environmental and Safety Office at UTEP. Project # 1104408-4.
Author Contributions
DD and AN: conceptualization and design. DD: administration and coordination. AN, NM, and AS: experimental work. AN and DD: data analysis and interpretation, writing, and editing. All authors contributed to the article and approved the submitted version.
Funding
This project was supported in part by the National Institute of General Medical Sciences of the National Institutes of Health under award numbers R25GM123928. The content is solely the responsibility of the authors and does not necessarily represent the official views of the National Institutes of Health.
Conflict of Interest
The authors declare that the research was conducted in the absence of any commercial or financial relationships that could be construed as a potential conflict of interest.
Acknowledgments
We like to express our gratitude to Jim H. Hageman, Ph.D. for his valuable comments and suggestions.
Supplementary Material
The Supplementary Material for this article can be found online at: https://www.frontiersin.org/articles/10.3389/fmicb.2020.573388/full#supplementary-material
References
Alcalde-Rico, M., Hernando-Amado, S., Blanco, P., and Martínez, J. L. (2016). Multidrug efflux pumps at the crossroad between antibiotic resistance and bacterial virulence. Front. Microbiol. 7:1483. doi: 10.3389/fmicb.2016.01483
Amaral, L., Martins, A., Molnar, J., Kristiansen, J. E., Martins, M., Viveiros, M., et al. (2010). Phenothiazines, bacterial efflux pumps and targeting the macrophage for enhanced killing of intracellular XDRTB. In Vivo 24, 409–424.
Arrizubieta, M. J., Toledo-Arana, A., Amorena, B., Penadés, J. R., and Lasa, I. (2004). Calcium inhibits bap-dependent multicellular behavior in Staphylococcus aureus. J. Bacteriol. 186, 7490–7498. doi: 10.1128/JB.186.22.7490-7498.2004
Asmat, T. M., Tenenbaum, T., Jonsson, A. B., Schwerk, C., and Schroten, H. (2014). Impact of calcium signaling during infection of Neisseria meningitidis to human brain microvascular endothelial cells. PLoS One 9:e114474. doi: 10.1371/journal.pone.0114474
Barrán-Berdón, A. L., Rodea-Palomares, I., Leganés, F., and Fernández-Piñas, F. (2011). Free Ca2 + as an early intracellular biomarker of exposure of cyanobacteria to environmental pollution. Anal. Bioanal. Chem. 400, 1015–1029. doi: 10.1007/s00216-010-4209-3
Berridge, M. J., Bootman, M. D., and Roderick, H. L. (2003). Calcium signalling: dynamics, homeostasis and remodelling. Nat. Rev. Mol. Cell Biol. 4, 517–529. doi: 10.1038/nrm1155
Bishai, W. R., Diarra, B., Maiga, M., Cohen, K. A., Winglee, K., and Gupta, S. (2013). Efflux inhibition with verapamil potentiates bedaquiline in Mycobacterium tuberculosis. Antimicrob. Agents Chemother. 58, 574–576. doi: 10.1128/aac.01462-13
Broder, U. N., Jaeger, T., and Jenal, U. (2016). LadS is a calcium-responsive kinase that induces acute-to-chronic virulence switch in Pseudomonas aeruginosa. Nat. Microbiol. 2:16184. doi: 10.1038/nmicrobiol.2016.184
Bruni, G. N., Weekley, R. A., Dodd, B. J. T., and Kralj, J. M. (2017). Voltage-gated calcium flux mediates Escherichia coli mechanosensation. Proc. Natl. Acad. Sci. U.S.A. 114, 9445–9450. doi: 10.1073/pnas.1703084114
Carafoli, E. (1987). Intracellular calcium homeostasis. Annu. Rev. Biochem. 56, 395–433. doi: 10.1146/annurev.bi.56.070187.002143
Carafoli, E., and Krebs, J. (2016). Why calcium? How calcium became the best communicator. J. Biol. Chem. 291, 20849–20857. doi: 10.1074/jbc.R116.735894
Chitsaz, M., and Brown, M. H. (2017). The role played by drug efflux pumps in bacterial multidrug resistance. Essays Biochem. 61, 127–139. doi: 10.1042/EBC20160064
Conceição, T., Coelho, C., De Lencastre, H., and Aires-De-Sousa, M. (2016). High prevalence of biocide resistance determinants in Staphylococcus aureus isolates from three African countries. Antimicrob. Agents Chemother. 60, 678–681. doi: 10.1128/AAC.02140-15
Corrigan, R. M., and Foster, T. J. (2009). An improved tetracycline-inducible expression vector for Staphylococcus aureus. Plasmid 61, 126–129. doi: 10.1016/j.plasmid.2008.10.001
Couto, I., Costa, S. S., Viveiros, M., Martins, M., and Amaral, L. (2008). Efflux-mediated response of Staphylococcus aureus exposed to ethidium bromide. J. Antimicrob. Chemother. 62, 504–513. doi: 10.1093/jac/dkn217
Dastgheyb, S. S., Villaruz, A. E., Le, K. Y., Tan, V. Y., Duong, A. C., Chatterjee, S. S., et al. (2015). Role of phenol-soluble modulins in formation of Staphylococcus aureus biofilms in synovial fluid. Infect. Immun. 83, 2966–2975. doi: 10.1128/IAI.00394-15
Domínguez, D. C. (2004). Calcium signalling in bacteria. Mol. Microbiol. 54, 291–297. doi: 10.1111/j.1365-2958.2004.04276.x
Domínguez, D. C., Lopes, R., Holland, I. B., and Campbell, A. K. (2011). Proteome analysis of B. subtilis in response to calcium. J. Analyt. Bioanalyt. Tech. 6, 1–9. doi: 10.4172/2155-9872.s6-001
Domínguez, D. C., Guragain, M., and Patrauchan, M. (2015). Calcium binding proteins and calcium signaling in prokaryotes. Cell Calcium 57, 151–165. doi: 10.1016/j.ceca.2014.12.006
Du, D., Wang-Kan, X., Neuberger, A., van Veen, H. W., Pos, K. M., Piddock, L. J. V., et al. (2018). Multidrug efflux pumps: structure, function and regulation. Nat. Rev. Microbiol. 16, 523–539. doi: 10.1038/s41579-018-0048-6
Eichstaedt, S., Gäbler, K., Below, S., Müller, C., Kohler, C., Engelmann, S., et al. (2009). Effects of Staphylococcus aureus-hemolysin A on calcium signalling in immortalized human airway epithelial cells. Cell Calcium 45, 165–176. doi: 10.1016/j.ceca.2008.09.001
Esposito, S., Garau, J., David, M. Z., Peters, G., Lina, G., Gould, I. M., et al. (2011). New insights into meticillin-resistant Staphylococcus aureus (MRSA) pathogenesis, treatment and resistance. Int. J. Antimicrob. Agents 39, 96–104. doi: 10.1016/j.ijantimicag.2011.09.028
Farcasanu, I. C., Popa, C.-V., and Ruta, L. L. (2018). “Calcium and cell response to heavy metals: can yeast provide an answer?,” in Calcium and Signal Transduction, eds J. N. Buchholz and E. J. Behringer (Rijeka: IntechOpen). doi: 10.5772/intechopen.78941
Fishman, M. R., Giglio, K., Fay, D., and Filiatrault, M. J. (2018). Physiological and genetic characterization of calcium phosphate precipitation by Pseudomonas species. Sci. Rep. 8:10156. doi: 10.1038/s41598-018-28525-4
Floyd, J. L., Smith, K. P., Kumar, S. H., Floyd, J. T., and Varela, M. F. (2010). LmrS is a multidrug efflux pump of the major facilitator superfamily from Staphylococcus aureus. Antimicrob. Agents Chemother. 54, 5406–5412. doi: 10.1128/AAC.00580-10
Foster, T. J. (2017). Antibiotic resistance in Staphylococcus aureus. Current status and future prospects. FEMS Microbiol. Rev. 41, 430–449. doi: 10.1093/femsre/fux007
Fraunholz, M., and Sinha, B. (2012). Intracellular Staphylococcus aureus: live-in and let die. Front. Cell. Infect. Microbiol. 2:43. doi: 10.3389/fcimb.2012.00043
González-Pleiter, M., Leganés, F., and Fernández-Piñas, F. (2017). Intracellular free Ca2 + signals antibiotic exposure in cyanobacteria. RSC Adv. 7, 35385–35393. doi: 10.1039/c7ra03001k
Grimsey, E. M., and Piddock, L. J. V. (2019). Do phenothiazines possess antimicrobial and efflux inhibitory properties? FEMS Microbiol. Rev. 43, 577–590. doi: 10.1093/femsre/fuz017
Guragain, M., Campbell, A., and Patrauchan, M. (2016). Measurement of intracellular calcium concentration in Pseudomonas aeruginosa. Bio-protocol 6:e2041. doi: 10.21769/bioprotoc.2041
Guragain, M., Lenaburg, D. L., Moore, F. S., Reutlinger, I., and Patrauchan, M. A. (2013). Calcium homeostasis in Pseudomonas aeruginosa requires multiple transporters and modulates swarming motility. Cell Calcium 54, 350–361. doi: 10.1016/j.ceca.2013.08.004
Hassan, K., Elbourne, L., Li, L., Hewawasam Gamage, H., Liu, Q., Jackson, S., et al. (2015). An ace up their sleeve: a transcriptomic approach exposes the AceI efflux protein of Acinetobacter baumannii and reveals the drug efflux potential hidden in many microbial pathogens. Front. Microbiol. 6:333. doi: 10.3389/fmicb.2015.00333
Hassan, K. A., Jackson, S. M., Penesyan, A., Patching, S. G., Tetu, S. G., Eijkelkamp, B. A., et al. (2013). Transcriptomic and biochemical analyses identify a family of chlorhexidine efflux proteins. Proc. Natl. Acad. Sci. U.S.A. 110, 20254–20259. doi: 10.1073/pnas.1317052110
Hayes, E. T., Wilks, J. C., Sanfilippo, P., Yohannes, E., Tate, D. P., Jones, B. D., et al. (2006). Oxygen limitation modulates pH regulation of catabolism and hydrogenases, multidrug transporters, and envelope composition in Escherichia coli K-12. BMC Microbiol. 6:89. doi: 10.1186/1471-2180-6-89
Herbaud, M. L., Guiseppi, A., Denizot, F., Haiech, J., and Kilhoffer, M. C. (1998). Calcium signalling in Bacillus subtilis. Biochim. Biophys. Acta 1448, 212–226. doi: 10.1016/S0167-4889(98)00145-1
Jang, S. (2016). Multidrug efflux pumps in Staphylococcus aureus and their clinical. J. Microbiol. 54, 1–8. doi: 10.1007/s12275-016-5159-z
Jones, H. E., Holland, I. B., Baker, H. L., and Campbell, A. K. (1999). Slow changes in cytosolic free Ca2 + in Escherichia Coli highlight two putative influx mechanisms in response to changes in extracellular calcium. Cell Calcium 25, 265–274. doi: 10.1054/ceca.1999.0028
Kaatz, G. W., Moudgal, V. V., Seo, S. M., Kristiansen, E., and Kristiansen, J. E. (2003). Phenothiazines and thioxanthenes inhibit multidrug efflux pump activity in Staphylococcus aureus phenothiazines and thioxanthenes inhibit multidrug efflux pump activity in Staphylococcus aureus. Antimicrob. Agents Chemother. 47, 719–726. doi: 10.1128/AAC.47.2.719
King, M. M., Kayastha, B. B., Franklin, M. J., and Patrauchan, M. A. (2020). Calcium regulation of bacterial virulence. Adv. Exp. Med. Biol. 1131, 827–855. doi: 10.1007/978-3-030-12457-1_33
Knight, M. R., Campbell, A. K., Smith, S. M., and Trewavas, A. J. (1991). Transgenic plant aequorin reports the effects of touch and cold-shock and elicitors on cytoplasmic calcium. Nature 352, 524–526. doi: 10.1038/352524a0
Kobayashi, S. D., Malachowa, N., and Deleo, F. R. (2015). Pathogenesis of Staphylococcus aureus abscesses. Am. J. Pathol. 185, 1518–1527. doi: 10.1016/j.ajpath.2014.11.030
Koul, S., Somayajulu, A., Advani, M. J., and Reddy, H. (2009). A novel calcium binding protein in mycobacterium tuberculosis – potential target for trifluoperazine. Indian J. Exp. Biol. 47, 480–488.
Li, J., Zhao, X., Tian, X., Li, J., Sjollema, J., and Wang, A. (2015). Retention in treated wastewater affects survival and deposition of Staphylococcus aureus and Escherichia coli in sand columns. Appl. Environ. Microbiol. 81, 2199–2205. doi: 10.1128/AEM.03740-14
Martins, A., MacHado, L., Costa, S., Cerca, P., Spengler, G., Viveiros, M., et al. (2011). Role of calcium in the efflux system of Escherichia coli. Int. J. Antimicrob. Agents 37, 410–414. doi: 10.1016/j.ijantimicag.2011.01.010
Martins, A., Spengler, G., Rodrigues, L., Viveiros, M., Ramos, J., Martins, M., et al. (2009). pH modulation of efflux pump activity of multi-drug resistant Escherichia coli: protection during its passage and eventual colonization of the colon. PLoS One 4:e6656. doi: 10.1371/journal.pone.0006656
Martins, M., Viveiros, M., Amaral, L., Costa, S., and Couto, I. (2008). Efflux-mediated response of Staphylococcus aureus exposed to ethidium bromide. J. Antimicrob. Chemother. 62, 504–513. doi: 10.1093/jac/dkn217
Moretti, C., Trabalza, S., Granieri, L., Caballo-Ponce, E., Devescovi, G., Del Pino, A. M., et al. (2019). A Na+/Ca2 + exchanger of the olive pathogen Pseudomonas savastanoi pv. savastanoi is critical for its virulence. Mol. Plant Pathol. 20, 716–730. doi: 10.1111/mpp.12787
Naseem, R., Holland, I. B., Jacq, A., Wann, K. T., and Campbell, A. K. (2008). pH and monovalent cations regulate cytosolic free Ca2 + in E. coli. Biochim. Biophys. Acta 1778, 1415–1422. doi: 10.1016/j.bbamem.2008.02.006
Naseem, R., Wann, K. T., Holland, I. B., and Campbell, A. K. (2009). ATP regulates calcium efflux and growth in E. coli. J. Mol. Biol. 391, 42–56. doi: 10.1016/j.jmb.2009.05.064
Nichols, R. J., Sen, S., Choo, Y. J., Beltrao, P., Zietek, M., Chaba, R., et al. (2011). Phenotypic landscape of a bacterial cell. Cell 144, 143–156. doi: 10.1016/j.cell.2010.11.052
Onyango, L. A., and Alreshidi, M. M. (2018). Adaptive metabolism in staphylococci: survival and persistence in environmental and clinical settings. J. Pathog. 2018:1092632. doi: 10.1155/2018/1092632
Perez, J. C., and Groisman, E. A. (2007). Acid pH activation of the PmrA/PmrB two-component regulatory system of Salmonella enterica. Mol. Microbiol. 63, 283–293. doi: 10.1111/j.1365-2958.2006.05512.x
Piddock, L. J. V. (2006). Multidrug-resistance efflux pumps? not just for resistance. Nat. Rev. Microbiol. 4, 629–636. doi: 10.1038/nrmicro1464
Pule, C. M., Sampson, S. L., Warren, R. M., Black, P. A., van Helden, P. D., Victor, T. C., et al. (2016). Efflux pump inhibitors: targeting mycobacterial efflux systems to enhance TB therapy. J. Antimicrob. Chemother. 71, 17–26. doi: 10.1093/jac/dkv316
Rajagopal, S., and Murugavel, P. (2017). Calcium Signaling: From Physiology to Diseases. Singapore: Springer. doi: 10.1007/978-981-10-5160-9
Rippke, F., Berardesca, E., and Weber, T. M. (2018). PH and microbial infections. Curr. Prob. Dermatol. 54, 87–94. doi: 10.1159/000489522
Romero, D., Traxler, M. F., López, D., and Kolter, R. (2011). Antibiotics as signal molecules. Chem. Rev. 111, 5492–5505. doi: 10.1021/cr2000509
Ruta, L. L., Popa, V. C., Nicolau, I., Danet, A. F., Iordache, V., Neagoe, A. D., et al. (2014). Calcium signaling mediates the response to cadmium toxicity in Saccharomyces cerevisiae cells. FEBS Lett. 588, 3202–3212. doi: 10.1016/j.febslet.2014.07.001
Santos Costa, S., Viveiros, M., Amaral, L., and Couto, I. (2013). Multidrug efflux pumps in Staphylococcus aureus: an update. Open Microbiol J. 7, 59–71. doi: 10.1111/avsc.12262
Sapula, S. A., and Brown, M. H. (2016). “Antimicrobial drug efflux pumps in Staphylococcus aureus,” in Efflux-Mediated Antimicrobial Resistance in Bacteria: Mechanisms, Regulation and Clinical Implications, eds X.-Z. Li, C. A. Elkins, and H. I. Zgurskaya (Cham: Springer International Publishing), 165–195. doi: 10.1007/978-3-319-39658-3_7
Sarkisova, S., Patrauchan, M. A., Berglund, D., Nivens, D. E., and Franklinm, M. J. (2005). Calcium-induced virulence factors associated with the extracellular matrix of mucoid Pseudomonas aeruginosa biofilms. J. Bacteriol. 187, 4327–4337. doi: 10.1128/JB.187.13.4327-4337.2005
Sarkisova, S. A., Lotlikar, S. R., Guragain, M., Kubat, R., Cloud, J., Franklin, M. J., et al. (2014). A Pseudomonas aeruginosa EF-hand protein, EfhP (PA4107), modulates stress responses and virulence at high calcium concentration. PLoS One 9:e98985. doi: 10.1371/journal.pone.0098985
Schindler, B. D., Jacinto, P. L., Buensalido, J. A. L., Seo, S. M., and Kaatz, G. W. (2015). Clonal relatedness is a predictor of spontaneous multidrug efflux pump gene overexpression in Staphylococcus aureus. Int. J. Antimicrob. Agents 45, 464–470. doi: 10.1016/j.ijantimicag.2014.11.007
Schindler, B. D., and Kaatz, G. W. (2016). Multidrug efflux pumps of Gram-positive bacteria. Drug Resist. Updat. 27, 1–13. doi: 10.1016/j.drup.2016.04.003
Serra-Cardona, A., Canadell, D., and Ariño, J. (2015). Coordinate responses to alkaline pH stress in budding yeast. Microb. Cell 2, 182–196. doi: 10.15698/mic2015.06.205
Takahashi, H., and Kopriva, S. (2019). Sulfate transport systems in plants: Functional diversity and molecular mechanisms underlying regulatory coordination. J. Exp. Bot. 70, 4075–4087. doi: 10.1093/jxb/erz132
Thomas, V. L., Sanford, B. A., and Ramsay, M. A. (1993). Calcium- and mucin-binding proteins of staphylococci. J. Gen. Microbiol. 139, 623–629. doi: 10.1099/00221287-139-3-623
Torrecilla, I., Leganés, F., Bonilla, I., and Fernández-Piñas, F. (2000). Use of recombinant aequorin to study calcium homeostasis and monitor calcium transients in response to heat and cold shock in cyanobacteria. Plant Physiol. 123, 161–176. doi: 10.1104/pp.123.1.161
Torrecilla, I., Leganés, F., Bonilla, I., and Fernández-Piñas, F. (2001). Calcium transients in response to salinity and osmotic stress in the nitrogen-fixing cyanobacterium Anabaena sp. PCC7120, expressing cytosolic apoaequorin. Plant Cell Environ. 24, 641–648. doi: 10.1046/j.0016-8025.2001.00708.x
Truong-Bolduc, Q. C., Bolduc, G. R., Okumura, R., Celino, B., Bevis, J., Liao, C. H., et al. (2011). Implication of the NorB efflux pump in the adaptation of Staphylococcus aureus to growth at acid ph and in resistance to moxifloxacin. Antimicrob. Agents Chemother. 55, 3214–3219. doi: 10.1128/AAC.00289-11
Tucker, D. L., Tucker, D. L., Tucker, N., Tucker, N., Conway, T., and Conway, T. (2002). Gene expression profling of the pH response in Escherichia coli. J. Bacteriol. 184, 6551–6558. doi: 10.1128/JB.184.23.6551
Van Nhieu, G. T., Clair, C., Bruzzone, R., Mesnil, M., Sansonetti, P., and Combettes, L. (2003). Connexin-dependent inter-cellular communication increases invasion and dissemination of Shigella in epithelial cells. Nat. Cell Biol. 5, 720–726. doi: 10.1038/ncb1021
Venditri, M., Falcone, M., Micozzi, A., Carfagna, P., Taglietti, F., Serra, P. F., et al. (2003). Staphylococcus aureus bacteremia in patients with hematologic malignancies: a retrospective case-control study. Haematologica 88, 923–930.
Webber, M. A., and Piddock, L. J. V. (2003). The importance of efflux pumps in bacterial antibiotic resistance. J. Antimicrob. Chemother. 51, 9–11. doi: 10.1093/jac/dkg050
Weinrick, B., Dunman, P. M., McAleese, F., Murphy, E., Projan, S. J., Fang, Y., et al. (2004). Effect of mild acid on gene expression in Staphylococcus aureus. J. Bacteriol. 186, 8407–8423. doi: 10.1128/JB.186.24.8407-8423.2004
Zampese, E., and Pizzo, P. (2012). Intracellular organelles in the saga of Ca2 + homeostasis: different molecules for different purposes? Cell. Mol. Life Sci. 69, 1077–1104. doi: 10.1007/s00018-011-0845-9
Keywords: efflux pumps, LmrS, prokaryotic calcium transport, calcium homeostasis, phenothiazines
Citation: Nava AR, Mauricio N, Sanca AJ and Domínguez DC (2020) Evidence of Calcium Signaling and Modulation of the LmrS Multidrug Resistant Efflux Pump Activity by Ca2 + Ions in S. aureus. Front. Microbiol. 11:573388. doi: 10.3389/fmicb.2020.573388
Received: 16 June 2020; Accepted: 14 September 2020;
Published: 22 October 2020.
Edited by:
Mattias Collin, Lund University, SwedenReviewed by:
Govindan Rajamohan, Institute of Microbial Technology (CSIR), IndiaSteven W. Polyak, University of South Australia, Australia
Copyright © 2020 Nava, Mauricio, Sanca and Domínguez. This is an open-access article distributed under the terms of the Creative Commons Attribution License (CC BY). The use, distribution or reproduction in other forums is permitted, provided the original author(s) and the copyright owner(s) are credited and that the original publication in this journal is cited, in accordance with accepted academic practice. No use, distribution or reproduction is permitted which does not comply with these terms.
*Correspondence: Delfina C. Domínguez, ZGVsZmluYUB1dGVwLmVkdQ==