- 1Key Laboratory of Coastal Environmental Processes and Ecological Remediation, Yantai Institute of Coastal Zone Research, Chinese Academy of Sciences, Yantai, China
- 2College of Resources and Environment, University of Chinese Academy of Sciences, Beijing, China
- 3National Engineering Laboratory for Efficient Utilization of Soil and Fertilizer Resources, College of Resources and Environment, Shandong Agricultural University, Tai’an, China
- 4Center for Ocean Mega-Science, Chinese Academy of Sciences, Qingdao, China
Biofilm formation is important for establishing plants-microbe associations. The role of calcium on biofilm formation has been studied in many bacteria except rhizobia. In this study, we investigated the role of calcium for biofilm formation in Azorhizobium caulindans, which forms nodules in the stem and root of its host plant Sesbania rostrata. We found that calcium is essential for A. caulindans biofilm formation, in addition to the presence of extracellular matrix components, eDNA and proteins. Also, calcium-mediated biofilm formation was tested with chemotaxis, motility, cyclic di-GMP synthesis, and quorum sensing mutants. Finally, calcium was found to promote S. rostrata root colonization of A. caulinodans. In total, these results show that calcium is essential for A. caulindans biofilm formation, and it affects the interaction between A. caulinodans and host plant.
Introduction
Environmental bacteria often form communities on biotic or abiotic surfaces called biofilms. Biofilm formation enables bacteria to survive in harsh environments or build an association with hosts (Tallawi et al., 2017). Biofilm formation can be beneficial to bacteria for several reasons: (1) Biofilm formation can protect bacteria under different stress conditions (Jefferson, 2004), (2) Biofilm formation can promote colonization of many bacteria and can allow bacteria to remain in a nutrient-rich niche (Jefferson, 2004), (3) Biofilm is a stage for the cooperation and communication between bacteria, and it is beneficial for bacteria to resist harsh environment together (Jefferson, 2004), and (4) Biofilm formation can generate niches that provide unique growth advantages. For example, biofilm formation enables free-living nitrogen-fixing rhizobacteria to fix nitrogen under aerobic conditions (Wang et al., 2017).
In biofilms, bacteria are coated with hydrated extracellular polymeric substances, including polysaccharides, proteins, nucleic acids, and lipids (Flemming and Wingender, 2010). The extracellular matrix provides a three-dimensional frame to stabilize the structure of biofilm, regulates the adhesion of bacteria to biotic or abiotic surface, and accommodates extracellular enzymes to digest biopolymers (Flemming and Wingender, 2010). Bacteria generate the extracellular matrix, and the components of the matrix changes depending on the bacterial species and growth conditions (Flemming and Wingender, 2010; Tallawi et al., 2017). In recent years, more and more extracellular matrix components from different bacteria have been characterized. Based on the extracellular matrix components, biofilm can be classified into two major classes: polysaccharides-predominant or polypeptides-predominant (Tallawi et al., 2017). In addition to polysaccharides and proteins, many bacteria also contain additional components, such as extracellular DNA (eDNA) and fimbriae in biofilm (Tallawi et al., 2017).
The development of both major classes of biofilm, represented by Pseudomonas aeruginosa (Ma et al., 2009) and Staphylococcus aureus (Hobley et al., 2015), occurs in similar sequential processes, including the initiation of biofilm formation, adhesion and maturation, and biofilm dispersal (Vlamakis et al., 2013). At the initial stage of biofilm formation, flagella enable bacteria to move in a relatively long-range surface and further increase the likelihood of approaching and adhering to surface (Karatan and Watnick, 2009; Belas, 2014). The flagellar mechanosensing of surfaces switches bacteria from a motile to a sessile lifestyle, inducing biofilm formation (Belas, 2014). The initial adherence is reversible, but once bacterial cells adhere to a surface irreversibly, the function of flagella and flagellar synthesis genes is inhibited (Zamorano-Sánchez et al., 2019). During the adhesion and maturation stages of biofilm formation, a signaling molecule, cyclic dimeric guanosine monophosphate (c-di-GMP) is involved in the regulation of motility and biofilm formation (Yang et al., 2018). The concentration of intracellular c-di-GMP correlates with the transition from a motile to a biofilm lifestyle. For example, in P. aeruginosa, high c-di-GMP levels inhibit motility and enhance biofilm formation (Simm et al., 2004). Diguanylate cyclases (DGCs) catalyze a reaction that generates c-di-GMP from two molecules of GTP. In contrast, phosphodiesterases (PDEs) catalyze the breakdown reaction of c-di-GMP. The detachment and dispersal of cells from biofilm occurs when the size of biofilm grows to a cell density threshold where cells no longer have access to nutrients (Karatan and Watnick, 2009). Cell-cell communication is also involved in biofilm formation. Quorum sensing is a density-dependent system of cell-cell communication by the release of chemical signals called autoinducers (Mukherjee and Bassler, 2019). For example, the biofilm of Vibrio cholerae is promoted at low cell density (Hammer and Bassler, 2003), while quorum sensing promotes the biofilm of Pseudomonas aeruginosa at high cell density (Davies et al., 1998).
The biofilm formation is also affected by many nutritional and environmental conditions. For some bacteria, such as Sinorhizobium meliloti (Rinaudi et al., 2006) and Dickeya zeae (Huang et al., 2019), nutrient sources enhance biofilm formation, while extreme conditions such as extreme temperature or pH prevents biofilm formation. But for others, such as in Azorhizobium caulinodans, lack of nutrient sources increases biofilm formation (Jiang et al., 2016; Liu et al., 2018b). In addition to nutrient and physical factors like pH and temperature, biofilm formation also can be affected by multivalent cations, including Ca2+, Cu2+, Mg2+, and Fe3+. Calcium (Ca2+) can affect biofilm formation positively or negatively in many bacteria, such as P. aeruginosa (Sarkisova et al., 2005) and Vibrio cholerae (Bilecen and Yildiz, 2009), using diverse mechanisms. For example, calcium can cause extensive formation of ordered helices of polysaccharides (Sutherland, 2001) or function as a small-molecule signal (Tischler et al., 2018).
Azorhizobium caulinodans is a symbiont of a tropical legume plant Sesbania rostrata (Dreyfus et al., 1988). A. caulinodans cannot only fix atmospheric nitrogen with S. rostrata in a symbiotic state (Dreyfus and Dommergues, 1981), but also can fix nitrogen in a free-living state (Dreyfus et al., 1983) or in association with non-legume plants, such as rice and wheat (Liu et al., 2017a). During the establishment of rhizobia-plant associations, biofilm formation on plant surfaces plays an important role (Yaryura et al., 2008; Xu et al., 2018). However, the process of biofilm formation of A. caulinodans and the role of biofilm formation on its early symbiosis with S. rostrata are less studied.
In this study, we used tryptone and yeast extract (TY) rich medium instead of L3 minimal medium to form biofilms, and found calcium is an essential factor for A. caulinodans biofilm formation. We not only determined the Ca2+-mediated biofilm of A. caulinodans is an eDNA predominant type, but also determined the roles of chemotaxis, motility, c-di-GMP, and quorum sensing on different stages of Ca2+-mediated biofilm formation and development. Finally, the positive effect of Ca2 + on the establishment of A. caulinodans- S. rostrata associations was also characterized.
Results
Altering A. caulinodans Biofilm Growth Condition Reveals a Crucial Role for Calcium
Previously, we used the L3 minimal medium, which uses 10 mM sodium lactate and 10 mM ammonium chloride as sole carbon and nitrogen sources, to explore the biofilm formation of A. caulinodans (Liu et al., 2018a). It is easy to remove ammonium chloride from L3 medium and construct a nitrogen free environment for A. caulinodans to fix nitrogen, thus L3 minimal medium is better to study the physiological variance of A. caulinodans under nitrogen fixation and nitrogen-replete conditions. A. caulinodans, however, can only form a weak biofilm when grown in L3 medium for 3–5 days. Interestingly, we found that A. caulinodans forms a thicker and broader biofilm in TY medium compared to L3 medium after 3–5 days (Figure 1A). Because A. caulinodans cells grow better in L3 medium than in TY medium (Supplementary Figure S1), we suspected that there might be potential signals in TY medium promoting the biofilm formation of A. caulinodans.
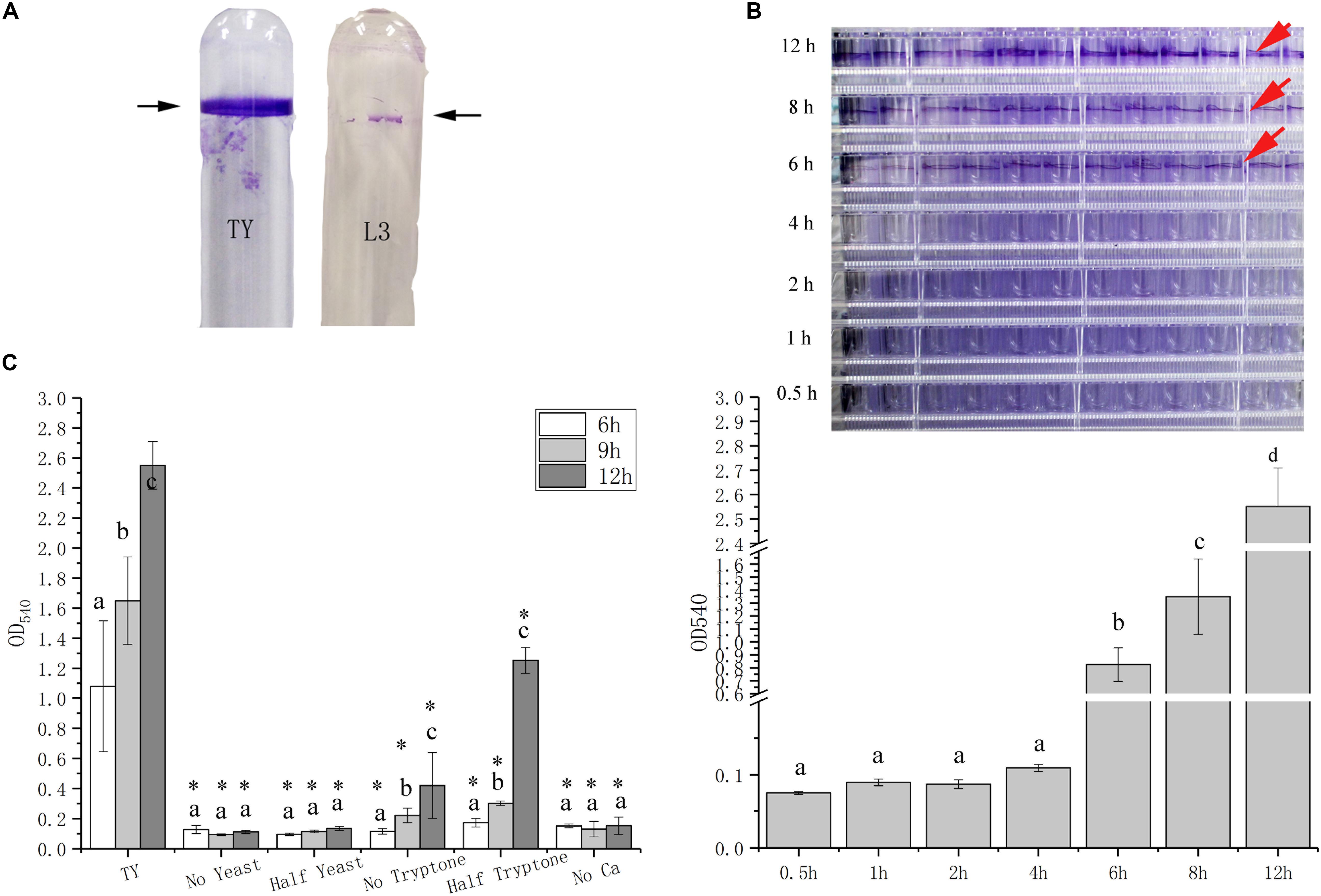
Figure 1. Biofilm formation of Azorhizobium caulinodans at various timepoints with different media components. (A) Biofilm formation of A. caulinodans with TY or L3 medium. Representative images of A. caulinodans biofilm on the wall of glass tubes after culturing for 5 days. (B) A. caulinodans biofilm formation in TY medium from 0.5 to 12 h. Images on the top are representative biofilms on the wall of 96-well plates. (C) Biofilm formation of A. caulinodans after 6, 9, and 12 h, when different components were removed from TY medium. Values are shown as the means and standard deviations from at least three independent experiments. The same letter above the error bars indicates not statistically different with results after culturing for 0.5 h (B) or under same conditions (C) by a Duncan’s test. Asterisks (∗) means P < 0.05 vs. the results after same time with TY medium by a Student’s t test.
To better investigate the biofilm formation of A. caulinodans with TY medium, we first tried to determine the kinetics of biofilm formation. The biofilm biomass of A. caulinodans was assessed by crystal violet (CV) staining assay over 12 h. Although earlier biofilm experiments assessed at biofilm formation after 3–5 days in L3 media, A. caulinodans can form biofilm after 6 h in TY media (Figure 1B). Thus, all the work of this study was performed in TY medium within 6–12 h. To determine if specific signals within TY media promote biofilm formation, we tested the ability of A. caulinodans to form biofilm in TY media that lack either some or all portions of tryptone, yeast extract, and calcium. The results showed that biofilm formation is dependent on calcium and yeast extract (Figure 1C). Because the absence of yeast extract in TY media significantly affected the growth of A. caulinodans (Supplementary Figure S2), we only focused on the role of calcium on biofilm formation in further work.
Determining How Various Concentrations of Calcium Alter Characteristics of A. caulinodans Biofilm
To further explore the role of calcium on biofilm formation, we measured the biofilm biomass with various concentrations of calcium from 0 to 900 mM, which is lower or higher than the concentration of calcium, 6 mM, in standard TY medium. The biofilm biomass is positively correlated with the calcium concentration in the media and the highest level of biofilm formation was reached at 300 mM (Figure 2A). Though the biofilm biomass was high when 900 mM calcium was added, the control group without bacteria bacteria at this calcium concentration showed a false biomass increase up to OD540 of 0.6 (Figure 2A). In addition, the growth of A. caulinodans cells was inhibited when the concentration of calcium reached 60 mM calcium (Supplementary Figure S1). Therefore, calcium promotes biofilm formation, and high concentration of calcium impairs the growth of A. caulinodans.
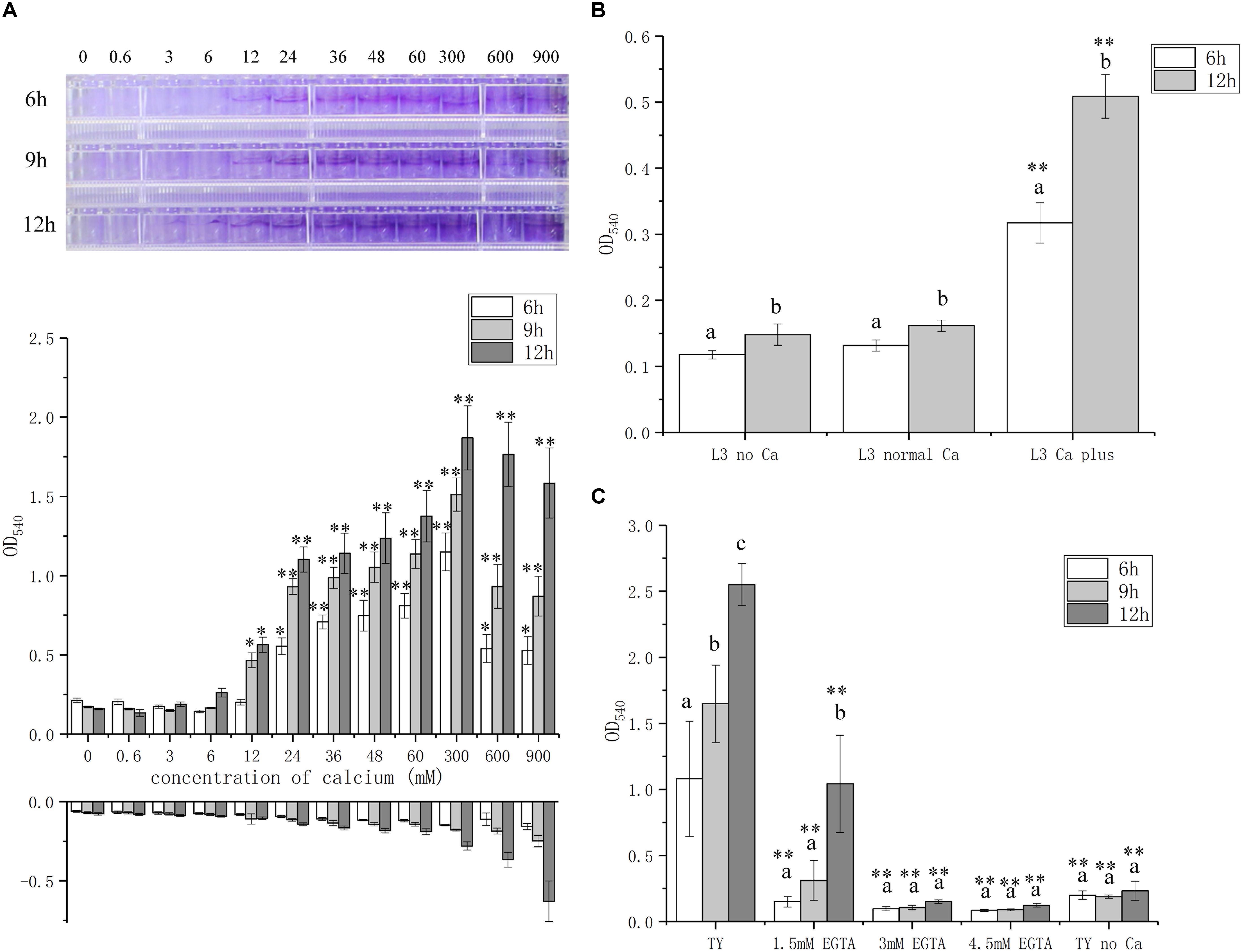
Figure 2. Biofilm formation of A. caulinodans with different concentrations of calcium and calcium chelator. (A) Calcium dose (0 to 900 mM) response of A. caulinodans biofilm formation with TY medium after 6, 9, and 12 h. Below the x axis means control without adding bacteria. Images on the top show the biofilm formed on the wall of 96-well plates. (B) Biofilm of A. caulinodans with different concentrations of calcium on L3 minimal medium after 6 and 12 h. (C) Biofilm for A. caulinodans with TY adding different concentration of calcium chelator, EGTA. Values are shown as the means and standard deviations from at least three independent experiments. The same letter above the error bars indicates not statistically different with results under same treatment by a Duncan’s test. Asterisks (∗) and (∗∗) mean P < 0.05 and P < 0.01 vs. the results after culturing same time with 0 mM calcium (A) or TY medium (C) by a Student’s t test.
Because TY medium is complex, other potential signal molecules may be present in tryptone or yeast extract that could confound the effect of calcium in biofilm formation. To exclude these possible confounding variables, we decided to test the effect of calcium on biofilm formation using L3 medium, containing sodium lactate and ammonium chloride as the sole carbon and nitrogen source, respectively. When 6 mM calcium was supplemented in the L3 medium, the biofilm formation increased from OD540 of 0.1 to 0.4 and 0.5 after 6 and 12 h, respectively (Figure 2B).
To further confirm the role of calcium in biofilm formation, various concentrations of Ca2+ chelator EGTA were added in TY medium. A weaker biofilm was formed when 1.5 mM EGTA was added compared to that without EGTA (Figure 2C). When 3 or 4.5 mM EGTA was added, there was no significant biofilm formation compared with TY medium without calcium (Figure 2C). These results strongly suggest that calcium is essential for the biofilm formation of A. caulinodans. To test whether the role of calcium on biofilm formation is common for divalent ion, we used Mg2+ to supplement into TY medium instead of Ca2+. In contrast to the strength of the effect of calcium on biofilm formation, Mg2+ did not promote, but inhibit, the biofilm formation of A. caulinodans (Supplementary Figure S3). This result indicates that the promoting role on A. caulinodans biofilm formation is exclusively calcium-dependent.
Ca2+-Mediated A. caulinodans Biofilms Are Dependent on the Presence of eDNA
When we detected the biomass of biofilm under various concentrations of calcium with TY medium, we found that if the bacteria were washed with sterilized PBS before being used to form biofilm, a fairly higher concentration of calcium was required to trigger biofilm formation, in comparison with unwashed bacteria (Supplementary Figure S4). These results indicate the presence of extracellular matrix might be important for the promoting role of calcium on biofilm formation.
EPS is the main component of biofilm matrix for some bacteria (Tallawi et al., 2017). It has been reported that calcium promotes biofilm formation by inducing the expression of polysaccharide and cellulose synthesis genes (Tischler et al., 2018). In most cases, in the absence of exopolysaccharide synthesis and export, bacteria can adhere to surfaces but are unable to form multilayer biofilms (Karatan and Watnick, 2009). To study the role of EPS on the Ca2+-mediated biofilm formation, we measured the biofilm biomass of Δazc_1831 and Δoac mutant strains, which delete one key gene or whole genes in cluster encoding EPS synthesis proteins (Sun et al., 2020). No matter at the 6 h or 12 h, however, there are no significant differences of biofilm biomass between Δoac and wild type (Figure 3A).
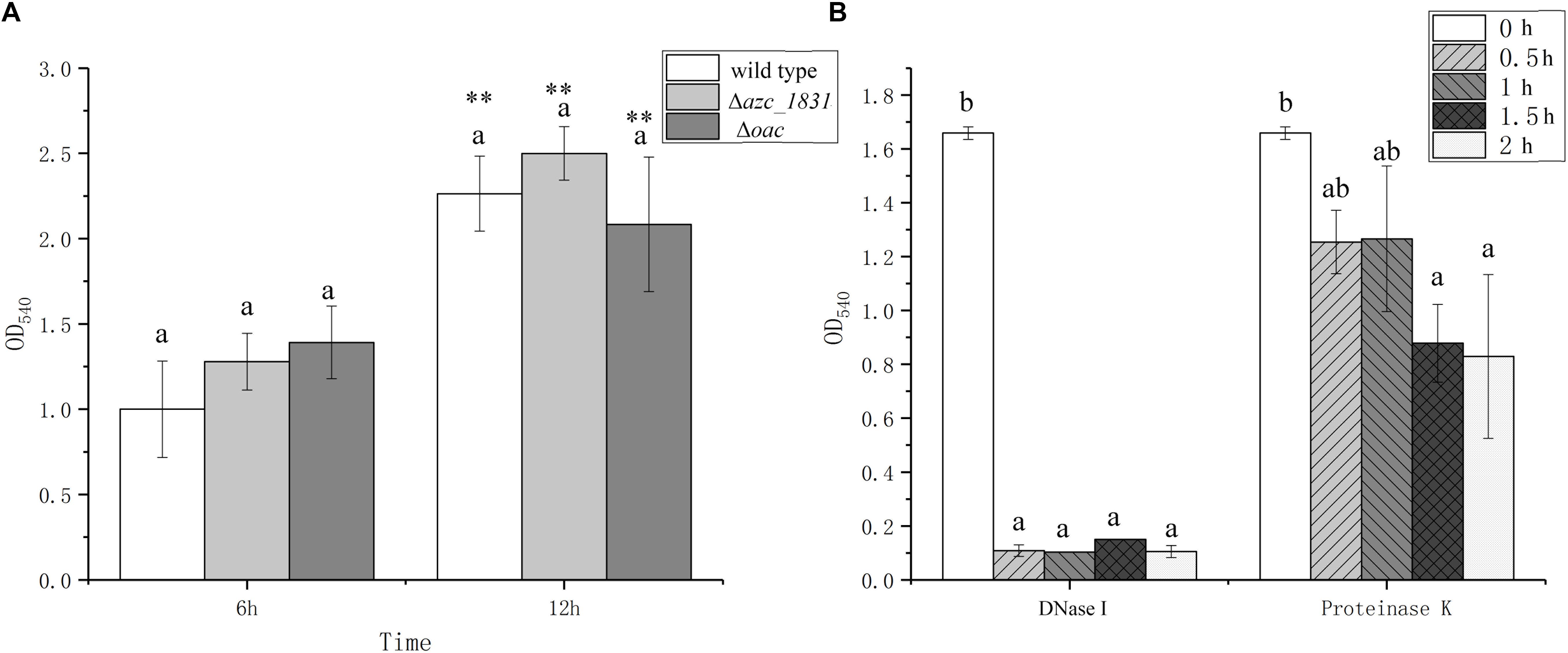
Figure 3. The roles of exopolysaccharides, eDNA, and extra cellular proteins on biofilm formation of A. caulinodans. (A) Biofilm formation of wild type, Δazc_1831 and Δoac after 6 and 12 h with TY medium. (B) After 9 h, the biofilm biomass of wild type after adding DNase or Proteinase K from 0 to 2 h. Values are shown as the means and standard deviations from at least three independent experiments. The same letter above the error bars indicates not statistically different with results after culturing for same time (A) or same treatment (B) by a Turkey HSD’s test. Asterisks (∗∗) means P < 0.01 vs. the wild type after culturing for same time by a Student’s t test.
In addition to EPS, protein and eDNA are two other important biofilm matrix in some bacterial species (Karygianni et al., 2020). When biofilm was formed with TY medium after 9 h, we added DNase or Proteinase K into the formed biofilm and tracked the changes of biofilm biomass after 0.5, 1, and 2 h. Addition of DNase can make the formed biofilm collapse quickly within 0.5 h, while adding Proteinase K only cause the biofilm biomass decrease slowly (Figure 3B). These results showed that the calcium-mediated biofilm of A. caulinodans is dependent on the presence of eDNA.
Chemotaxis and Motility Are Required for Calcium-Mediated Biofilm Formation at Different Stages
The relationship between chemotaxis and biofilm formation has been reported in many bacteria (Reinhardt and Bardy, 2018), and numerous studies suggest that transcription of chemotactic genes are activated during biofilm initiation (Karatan and Watnick, 2009). A. caulinodans has one core chemotactic pathway, including CheA, CheW, CheY1, CheZ, CheB, and CheR, which is similar to Escherichia coli (Sourjik and Wingreen, 2012), and 43 chemoreceptors. Additionally, A. caulinodans has a unique chemotaxis response regulator, CheY2, which functions as a phosphate sink (unsubmitted manuscript). To determine whether chemotaxis plays a role in A. caulinodans calcium-mediated biofilm formation, the biofilm biomass of different chemotaxis mutants including ΔcheA, ΔcheA-R (deletion of cheA, cheY2, cheW, cheB, and cheR operon), ΔcheZ, ΔcheY1, and ΔcheY2 were measured after 6, 9, and 12 h with standard TY medium. Biofilm formation at 6 or 12 h, except ΔcheZ and ΔcheY2, the biomass of ΔcheA, ΔcheA-R, ΔcheY1, and ΔcheY2 all showed significantly decrease compared to wild type (Figure 4A), indicating the chemotaxis plays an important role on these stages of biofilm. Two chemoreceptor mutants, Δazc_0821 and Δazc_0660, were also employed to investigate the role of chemoreceptors on the calcium-mediated biofilm formation. The two chemoreceptors AZC_0821 (also termed as TlpH) and AZC_0660 (also termed as TlpA1) have broad-range ligands including organic acids and amino acids (Liu et al., 2017b; Liu et al., 2019b,c). At 6 or 12 h, they both showed a significant decrease of biofilm biomass compared to wild type (Figure 4A).
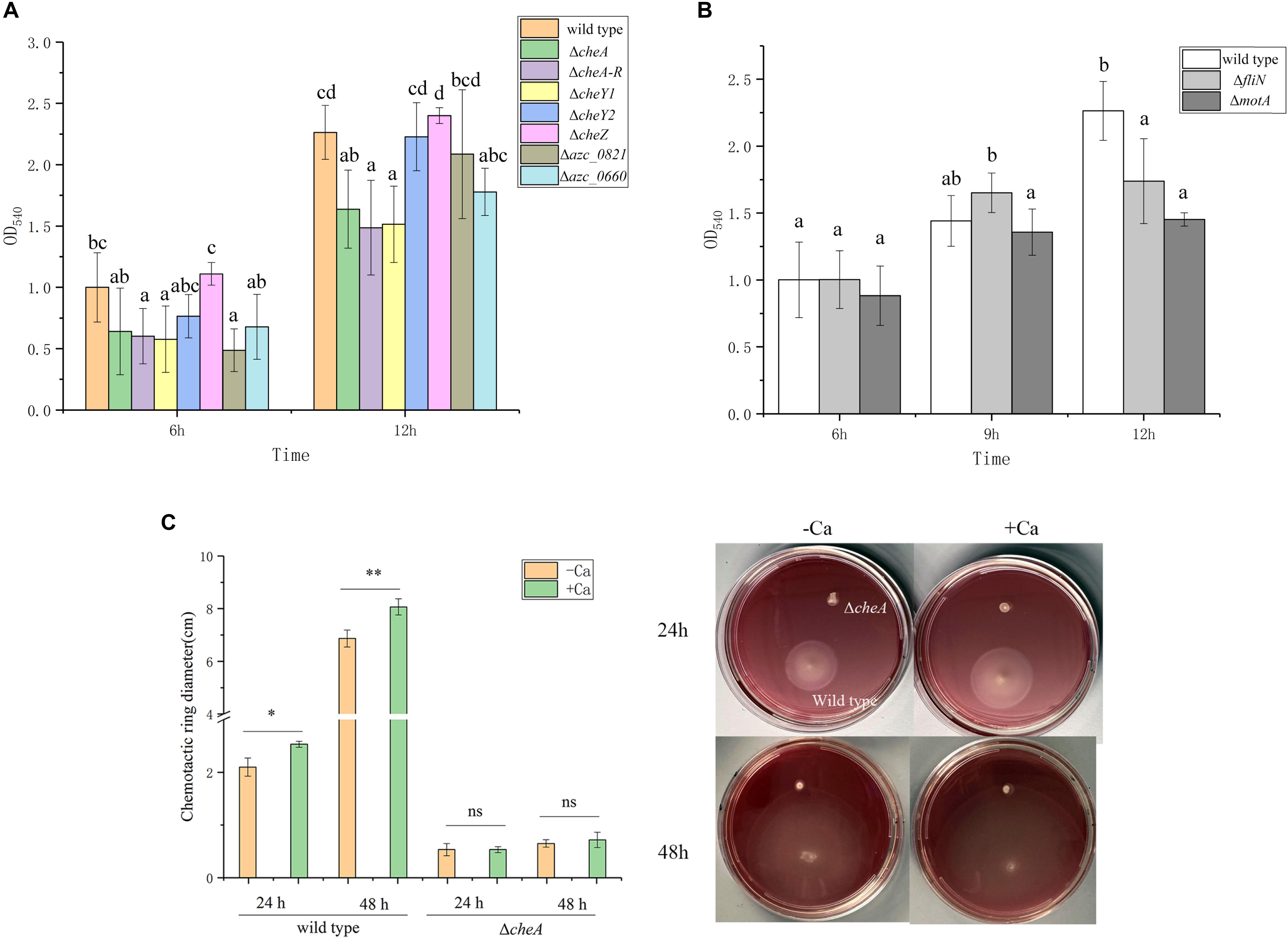
Figure 4. Biofilm formation of wild type and different chemotaxis and motility defective mutants. (A) Biofilm formation of chemotaxis defective mutants ΔcheA, ΔcheA-R, ΔcheZ, ΔcheY1, ΔcheY2, Δazc_0821, and Δazc_0660 with TY medium after 6 and 12 h. (B) Two motility defective mutants ΔfliN and ΔmotA form biofilm with TY medium after 6, 9, and 12 h. The same letter above the error bars indicates not statistically different with results under same culturing time by a Duncan’s test. (C) Swimming behavior of A. caulinodans wild type and ΔcheA on soft-agar plates with or without 6 mM calcium. Asterisks (∗P < 0.05; ∗∗P < 0.01) show a significant difference between conditions according to a t-test. Values are shown as the means and standard deviations from at least three independent experiments.
For many bacteria, the initiation of biofilm formation is dependent on flagellar motility (Jung et al., 2019). In addition to regulating swimming motility, the existence of flagellar filaments has been shown to help establish a robust biofilm (Hathroubi et al., 2018). To investigate the role of flagella and motility on the calcium-mediated biofilm, ΔfliN and ΔmotA, two non-flagellated and non-motile mutants (Shen et al., 2018), were tested for biofilm formation. The biofilm biomass of both flagellar mutants was not significantly different from wild type at 6 h; however, the two mutants showed significant decrease of biofilm biomass after 12 h with standard TY medium (Figure 4B).
Considering the role of chemotaxis and motility on the calcium-mediated biofilm, we wonder what about the role of calcium on motility and chemotaxis of A. caulinodans? When we compared the swimming behavior of A. caulinodans in the presence and absence of 6 mM calcium using TY soft-agar plate assay. The result shows that calcium promotes the chemotactic motility of A. caulinodans significantly (Figure 4C). The non-chemotactic mutant ΔcheA was used as a control.
Cyclic di-GMP and Quorum Sensing Proteins Are Involved in the Calcium-Mediated Biofilm Formation
Cyclic di-GMP is a conserved intracellular signal molecule that regulates motility, biofilm formation, and virulence in several species of bacteria (Zamorano-Sánchez et al., 2019). It has been reported that c-di-GMP can induce a calcium-binding protein CabA, which is essential for biofilm formation in Vibrio vulnificus (Park et al., 2015). In A. caulinodans, AZC_0308 (also termed as Chp1) is a c-di-GMP phosphodiesterase, and deletion of azc_0308 causes an increase of intracellular c-di-GMP level (Sun et al., 2020). AZC_2412 is a diguanylate cyclase, and the intracellular level of c-di-GMP decreases when azc_2412 is deleted (Yang et al., 2019). To investigate the role of c-di-GMP on calcium-mediated biofilm formation, Δazc_0308 and Δazc_2412 were tested for biofilm formation in standard TY medium. The Δazc_0308 showed an increase of biofilm biomass after 9 h compared to wild type, while Δazc_2412 showed a significant decrease of biofilm biomass compared to wild type after 6 or 12 h (Figure 5A). Interestingly, the biofilm biomass of wild type and Δazc_2412 were similar at 9 h (Figure 5A).
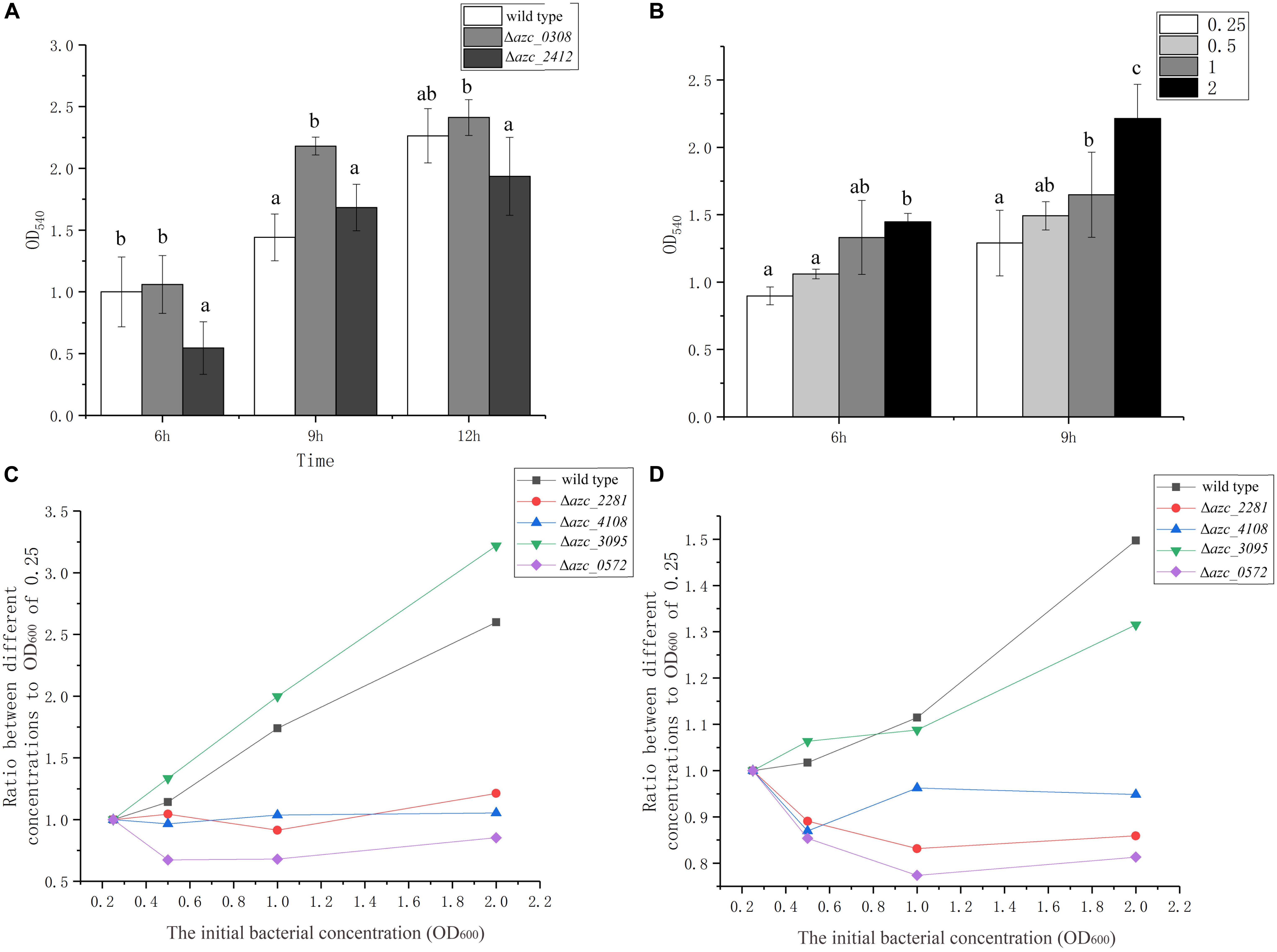
Figure 5. The role of c-di-GMP and quorum sensing on biofilm formation. (A) Biofilm formation of c-di-GMP increased and decreased mutants, Δazc_0308 and Δazc_2412 after 6, 9, and 12 h. (B) Biofilm formation of wild type with different initial bacterial concentrations from OD600 of 0.25 to 2.5 after 6 and 9 h. (C,D) The relationship between the biofilm biomass and initial bacterial concentration for different quorum sensing luxR-like gene mutants, Δazc_0572, Δazc_2281, Δazc_3095, Δazc_4108 after 6 h (C) and 9 h (D). Values are shown as the means and standard deviations from at least three independent experiments. The same letter above the error bars indicates not statistically different with results under same culturing time by a Duncan’s test.
Quorum sensing can increase or decrease the intracellular levels of c-di-GMP level and regulate virulence and biofilm formation of bacteria (Karatan and Watnick, 2009; Papenfort and Bassler, 2016). Because the role of quorum sensing is dependent on the cell density, thus we determined the biofilm formation with different initial cell concentrations from OD600 of 0.25 to 2 after 6 or 9 h with standard TY medium. There is a positive correlation between the initial cell density of biofilm biomass of A. caulinodans (Figure 5B), indicating cell density may be involved in the calcium-mediated biofilm formation.
In gram-negative bacteria, LuxR-type receptors are employed to detect the autoinducers of quorum sensing (Papenfort and Bassler, 2016). Previously, we showed that one LuxR-type receptor AZC_0572 (also termed as AclR1) negatively regulates the biofilm formation of A. caulinodans with L3 minimal medium (Liu et al., 2019a). In addition to AZC_0572, there are eight other LuxR-type receptors in A. caulinodans, containing two functional domains of LuxR-type receptors, including a ligand-binding domain and a DNA-binding domain (Smith et al., 2006). In this study, the roles of four of them, AZC_0572, AZC_2281, AZC_3095, and AZC_4108, on the calcium-mediated biofilm were investigated. Except Δazc_3095, the positive correlation between cell density and biofilm formation was disrupted in Δazc_0572, Δazc_2281, and Δazc_4108 (Figures 5C,D). This result further indicates the calcium-mediated biofilm is regulated by quorum sensing.
The Role of Calcium on Colonization of S. rostrata
For many bacteria, such as Bacillus amyloliquefaciens, Enterococcus faecalis, and Vibrio fischeri, the successful colonization of a host is influenced by their ability to attach and form a biofilm (Yaryura et al., 2008; Tischler et al., 2018; Xu et al., 2018; Willett et al., 2019). For P. syringae pv. Tomato DC3000, its association with host can be promoted by adding calcium (Fishman et al., 2018). These reports prompted us to determine the role of calcium on the Sesbania colonization of A. caulinodans. To test the role of calcium on the colonization of A. caulinodans, two kinds of mediums (L3 and TY) with or without calcium were used in a S. rostrata root colonization assay. In L3 medium, there is no significant differences on the colonization of A. caulinodans with or without calcium (0.36 or 0 mM) (Figure 6). In TY medium, the colonization of A. caulinodans was reduced significantly when calcium was removed from TY medium (Figure 6).
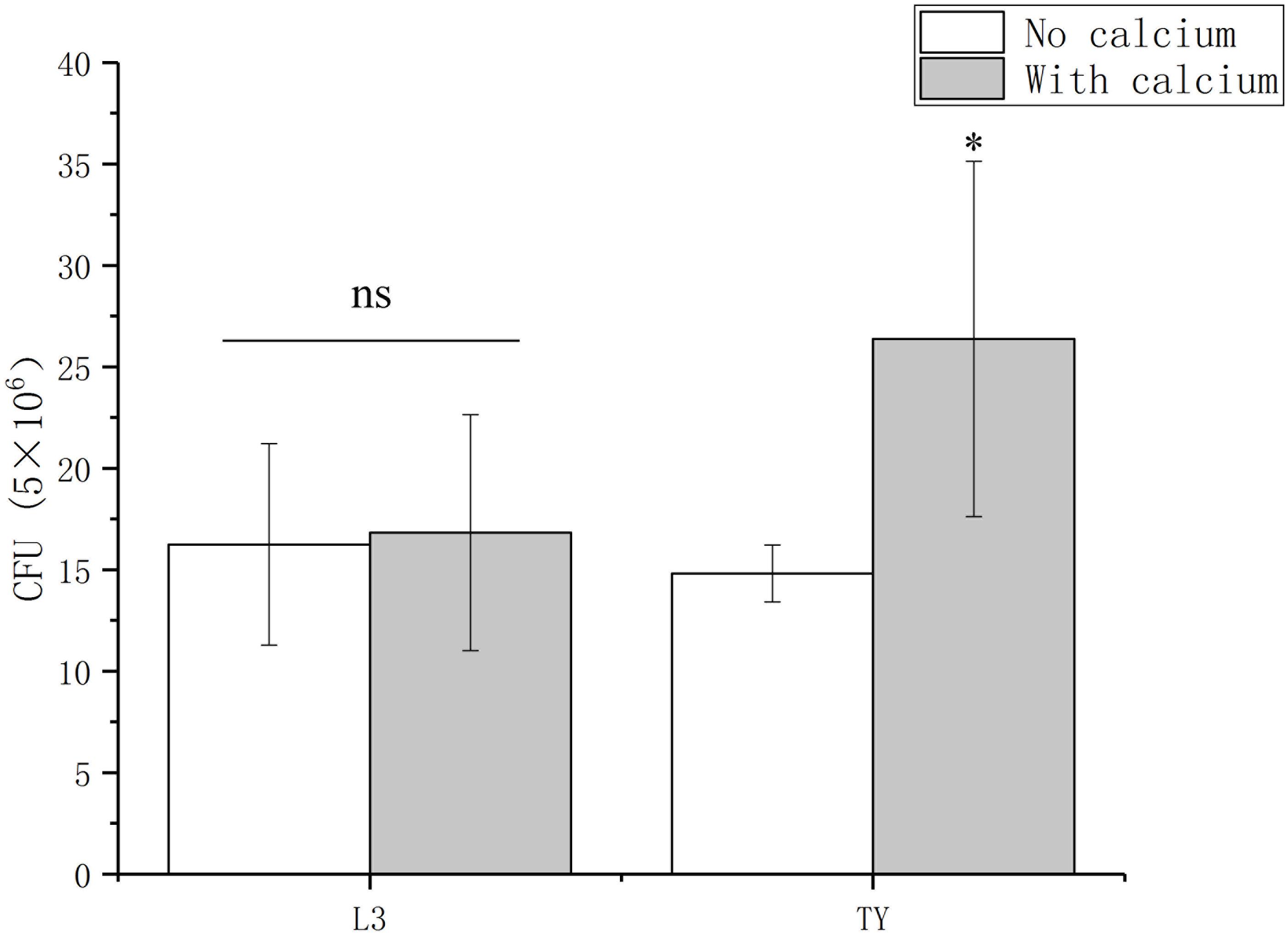
Figure 6. Colonization of wild type on S. rostrata roots with L3 or TY medium including calcium or not. The concentration of calcium in L3 and TY mediums is 0.36 and 6 mM, respectively. Values are shown as the means and standard deviations from at least three independent experiments. Asterisks (∗) means p < 0.05 vs. each medium without calcium by a Student’s t test.
Discussion
Biofilm formation is important for the establishment of rhizobacteria and legume plants associations (Scharf et al., 2016). The present work revealed that calcium plays a significant role in A. caulindans biofilm formation and A. caulindans colonization of S. rostrata. Also, we found that extracellular protein and eDNA are involved in the calcium-mediated biofilm formation. Additionally, we identified the effects of chemotaxis, motility, cyclic di-GMP, and quorum sensing on the calcium-mediated biofilm formation.
When yeast extract was removed from TY medium, A. caulinodans did not form biofilm, indicating the biofilm formation of A. caulinodans is correlated with the nutritional conditions. In addition to A. caulinodans, the biofilm formation of many other bacteria also depends on nutritional conditions. For example, Bacillus subtilis cannot form biofilm in mineral media (Voberkova et al., 2016), and nutrients are important for the biofilm formation of Sinorhizobium meliloti (Rinaudi et al., 2006).
When calcium was removed from or the calcium chelator, EGTA, was added into TY medium, both of them resulted in no biofilm formation, indicating that calcium is essential for the biofilm formation of A. caulinodans. Why calcium is essential for the biofilm formation of A. caulinodans? Calcium could promote the biofilm formation using several strategies. First, calcium can cross-link EPS. Some EPS in gram-negative bacteria have uronic acids, which contribute to the association with Ca2+ and enhance the mechanical stability of biofilm (Rinaudi et al., 2006; Flemming and Wingender, 2010). Second, calcium forms a bridge between eDNA. Calcium, as a divalent cation, could link different bacterial surfaces, which are negatively charged. It has been shown that eDNA at the cell surface can be bound to calcium and medicate bacterial aggregation in many bacteria such as Haemophilus influenzae (Das et al., 2017) and Xylella fastidiosa (Chen et al., 2017). Third, calcium can interact with biofilm-associated proteins. Biofilm-associated surface proteins are conserved in many bacteria, including Pseudomonas putida, Salmonella enteritidis, and Pseudomonas fluorescens, and they are important for the biofilm formation in early stage (Lasa and Penades, 2006). In Salmonella, biofilm-associated protein, BapA, is an important structural component for biofilm formation, and calcium was found to be important for proper protein folding (Guttula et al., 2019). In addition, biofilm-associated surface proteins are important for the maintenance of biofilm (McCall et al., 2019). Fourth, calcium can function as a signal and induce the expression of genes involved in different pathways to mediate biofilm formation. For example, in Vibrio vulnificus, in the presence of calcium, a LysR-type regulator, IamR, is induced and then affects pilus production and biofilm formation (Pu et al., 2020). In Vibrio fischeri and Pseudomonas syringae pv. tomato DC3000, calcium induces the biofilm formation and infection via two-component system (Cruz et al., 2012; Tischler et al., 2018). Calcium also can mediate the expression of cabABC operon, encoding a system to secret a calcium-binding matrix protein CabA, which is required for biofilm and rugose colony formation in V. fischeri (Park et al., 2015). In this study, exopolysaccharide mutants showed a similar phenotype as wild type on biofilm formation, indicating EPS does not influence the biofilm formation of A. caulinodans. However, another two main matrix components, eDNA and matrix proteins, especially eDNA, are involved in the biofilm formation and maintenance, because addition of DNase results in dispersal of biofilm. When we tested the biofilm biomass with different concentration with calcium from 0.6 to 900 mM, the maximum biofilm formation was observed with 300 mM calcium (Figure 2A), though the cells viability were decreased significantly at concentrations above 60 mM (Supplementary Figure S2). One possibility is that the effect of calcium on biofilm formation is modulated at least partially by cell death and lysis, releasing DNA into the medium. Then the enhanced amounts of eDNA might promote the calcium-mediated biofilm formation. Another possibility is that high osmolality caused by higher concentration of calcium may signal biofilm formation in A. caulinodans. These hypotheses need to be further studied via quantifying the amounts of eDNA of biofilm under different conditions.
Chemotaxis and flagellum-based motility are important for biofilm formation in many bacteria (Holscher et al., 2015). The result in this work further indicates that the calcium-mediated biofilm can be regulated by chemotaxis signaling in A. caulinodans in early and late stages. There is a positive correlation between aggregation and biofilm formation (Alexandre, 2015). We have shown that ΔcheZ is easier to form aggregates than wild type (Liu et al., 2018b). Consistent with previous results, there are significant differences between ΔcheZ and wild type or other chemotaxis mutants on the calcium-mediated biofilm formation. This result indicates that the role of CheZ on cellular processes other than chemotaxis might cause the phenotype of the mutant on biofilm formation. The ΔcheY2 shows a similar phenotype as ΔcheZ, which functions as phosphate sink to terminate chemotactic signal transduction and might be involved in other cellular processes, such as aggregation. One possibility for the opposite roles of ΔcheZ and ΔcheY2 on biofilm to that of the other mutants is the different swimming bias between them. Because lack of cheZ or cheY2 will increase the clockwise rotation of flagella, while deletion of cheY1 or cheA will increase the counter-clockwise rotation of flagella. The differences of flagellar rotation between them may influence the attachment of cells to plant root or 96-well surface.
There are two reasons for the negative role of two chemoreceptors AZC_0821 and AZC_0660 on biofilm formation. First, chemotaxis toward ligands in TY medium, which might be sensed by AZC_0821 and AZC_0660, is important for the calcium-mediated biofilm. In Pseudomonas fluorescens, the ligand citrate can bind to the Cache domain of GcbC to mediate biofilm formation (Giacalone et al., 2018). Second, calcium might function as a chemotaxis ligand, and AZC_0821 and AZC_0660 might be involved in the sensing of calcium signals directly.
Flagella-based motility is required for many bacteria in the initial stage of biofilm formation (Guttenplan and Kearns, 2013). However, flagella-based motility is not involved in the early stage of calcium-mediated biofilm formation in A. caulinodans. Interestingly, the absence of flagella decreases the biofilm biomass after 12 h. These results are consistent with the role of flagella in Helicobacter pylori biofilm formation, which plays a structural role in stabilizing biofilm (Hathroubi et al., 2018). According to some reports, calcium can enhance twitching motility in Xylella fastidiosa (Cruz et al., 2012, 2014), and promote swarming behavior of Vibrio parahaemolyticus (Gode-Potratz et al., 2010). Here, we further showed that calcium can also increase flagellar motility of A. caulinodans. Considering that FliN and MotA only play roles on the late stage of biofilm formation, these results indicate that flagellar motility is required for calcium-depended biofilm maintenance, but not initiation.
There are numerous studies about the role of c-di-GMP and quorum sensing on biofilm formation (Papenfort and Bassler, 2016). In this work, we found that increasing or decreasing the intracellular level of c-di-GMP, Δazc_0308 or Δazc_2412, only affects the rate of biofilm development but not the maturation of biofilm. Because there are 37 GGDEF/EAL domain-containing proteins in A. caulinodans, and the role of Δazc_0308 or Δazc_2412 might be masked by other redundant proteins (Sun et al., 2019). Nine LuxR-like proteins were annotated in Microbe online website1. In this work, four of them were deleted separately, however, only three of them AZC_572, AZC_2281, and AZC_4108 showed a concentration-dependent role on biofilm formation. AZC_3095 might not play a role in the biofilm formation or its role is masked by other LuxR-like proteins. What needs to be pointed out is that, in this study, we only tested the biofilm formation of LuxR paralog mutants, and the direct role of quorum sensing on biofilm formation needs to be further studied.
The A. caulinodans colonization of S. rostrata is related to the attachment, biofilm formation and colonization. Calcium can promote the biofilm formation via bridging the negatively charged groups between the surfaces of plant and bacteria (Rinaudi et al., 2006). It is not surprising that the colonization of A. caulinodans can be enhanced. No differences of colonization using L3 medium with or without calcium indicate that there might be a concentration threshold of calcium to promote biofilm formation and colonization. In this study, however, we cannot exclude the possibility that the calcium in TY or L3 media might affect different plant physiology or growth of plant roots was limited within 4 h. In addition, the calcium concentrations vary with the soil types, and clayey soil has much higher calcium than sandy soil (Bonomelli et al., 2019), whether the promoting effect of calcium on the biofilm formation also applies to different soil types and rhizobacteria? These questions need to be answered using numerous studies in the future.
Taken together, these results from this work expand our horizon with regard to calcium in the biofilm formation and colonization of A. caulinodans. The mechanism of inducing biofilm through calcium remains unknown, and potential biofilm associated proteins, which are involved in the calcium sensing or binding, need to be further studied.
Materials and Methods
Strains and Media
Azorhizobium caulinodans ORS571 wild type, its derivative mutants, and all plasmids used in this work are listed in Table 1. Tryptone-yeast extract (TY) medium and L3 minimal medium and their modified medium were used to culture A. caulinodans wild type and its derivative mutants. Calcium chloride was used as the source of calcium ranged from 0.6 to 900 mM in TY medium and from 0.36 to 6 mM in L3 medium. E. coli and derivative strains were grown in Luria-Bertani medium.
Molecular Methods and Strain Construction
To construct motA knock-out in this study, upstream and downstream fragments (around 500–800 bp) of the motA gene were amplified with cognate primers listed in Table 2. Two amplicons and allelic exchange vector, pCM351 (Marx and Lidstrom, 2002) were digested with cognate restriction enzymes and then they were purified and linked together with T4 DNA ligase. The resulting plasmid with upstream and downstream homology arms of motA was introduced into wild-type cells with the help of a helper pRK2013 (Ditta et al., 1980) using triparental conjugation. Correct mutant was selected using antibiotic gentamicin and verified by PCR and sequencing. For the construction of other mutants including Δazc_3095, Δazc_2281, and Δazc_4108, the same method was used as above.
Biofilm Formation Assay
Glass tubes and 96-well plates were used to form biofilm. The initial concentration of cells was adjusted to OD600 of 2.5. Three milliliter and 200 microliter cell cultures were added into glass tubes and 96-well plates, respectively. Cells in glass tubes were incubated for 3–5 days at 37°C. For the cells in 96-well plates, cells were incubated from 0.5 to 12 h. After incubation, the glass tubes or 96-well plates were washed gently with sterile PBS and then adding 3 milliliters or 300 microliters 0.1% w/v Crystal violet in glass tubes or wells. The crystal violet was removed gently after incubating for 20 min at room temperature, and PBS was used to wash tubes or wells. Representative results of them were taken photos, and then 200 microliters of 30% acetic acid was added into each well. The OD540 of each well was determined by microplate reader (Tecan Infinite M200) after being transferred into a new 96-well plate.
DNase and Proteinase K Assay
Biofilm was formed using method as above. After incubating for 9 h, the biofilm was used to determine the role of DNase and Proteinase K on the biofilm disperse. DNase I (50 μg/ml) and Proteinase K (200 μg/ml) were added into each well at 37° from 0.5 to 2 h, before being stained by crystal violet.
Soft-Agar Plate Assay
Azorhizobium caulinodans wild type and derivative strains were cultured overnight with TY media. The overnight cultures were collected and washed with PBS at least two times. The cell suspension was then adjusted to an OD600 of 0.6 with PBS. Five microliter suspension were dropped into 0.3% soft-agar plates. The 0.3% soft-agar with or without 6 mM calcium (CaCl2) were poured 12 h before being used. The plates with cells were transferred to incubator at 37°. The diameter of chemotactic ring on each plate was recorded after culturing for 24 and 48 h.
Colonization Assay
Sulfuric acid was used to sterilize the surface of S. rostrata seed, and then induced uniform germination. The sulfuric acid was removed after incubating 30 min, and sterile water was used to wash the seed at least five times. After washing, the seeds were immersed into sterile water and incubated in the dark condition for 48 h at 37°C. Overnight cultured cells were adjusted to OD600 of 0.01 using L3 medium or TY medium with or without calcium. Germinated seeds were soaked into cell suspension with different mediums for 4 h. Then, the surface of seedings was washed at least five times with sterile water. The washed seedlings were vortexed completely, and bacteria were reisolated from the surface of seedlings. After serial dilutions, 20 microliters of cells were plated on TY solid plates with antibiotics.
Statistically Analysis
Differences among the treatments were statistically analyzed using the Statistical Package for the Social Sciences (version 20.0; SPSS Inc.). Student t test, Duncan test, and Turkey HSD test assuming equal variances (P < 0.05 or 0.01) were used to determine significant differences between treatments.
Data Availability Statement
The raw data supporting the conclusions of this article will be made available by the authors, without undue reservation.
Author Contributions
XL, KZ, and ZX conceived and designed the experiments, analyzed the data, prepared the figures and tables, and wrote the manuscript. XL, KZ, YL, DZ, and DW carried out the experiments. All authors approved the submitted manuscript for publication.
Funding
This work was financed by the NSFC-Shandong Joint Fund Key Projects (U1806206), National Key Research and Development Program (2019YFD1002702), and National Natural Science Foundation of China (31870020).
Conflict of Interest
The authors declare that the research was conducted in the absence of any commercial or financial relationships that could be construed as a potential conflict of interest.
The reviewer LM declared a shared affiliation with the authors to the handling editor at time of review.
Acknowledgments
We thank Karen Ottemann, Kevin Johnson, Shuai Hu, and the two reviewers for helpful comments on the manuscript.
Supplementary Material
The Supplementary Material for this article can be found online at: https://www.frontiersin.org/articles/10.3389/fmicb.2020.563367/full#supplementary-material
FIGURE S1 | Growth curve of wild type with different modified TY and L3 medium. Values are shown as the means and standard deviations from at least three independent experiments.
FIGURE S2 | Growth curve of wild type with different concentrations of calcium from 0 to 900 mM. Values are shown as the means and standard deviations from at least three independent experiments.
FIGURE S3 | Biofilm biomass of wild type with different concentrations of magnesium instead of calcium after 6, 9, and 12 h. Values are shown as the means and standard deviations from at least three independent experiments.
FIGURE S4 | Biofilm formation of wild type with different washing treatment. (A) Before testing the role of calcium on biofilm formation of A. caulinodans, bacterial cells cultured with TY medium were washed three times with TY no calcium medium. (B) Bacterial cells were cultured with TY medium without calcium, and then they were used to test the role of calcium on biofilm formation directly. Images at the top means the representative results of them. Values are shown as the means and standard deviations from at least three independent experiments.
Footnotes
References
Alexandre, G. (2015). Chemotaxis control of transient cell aggregation. J. Bacteriol. 197, 3230–3237. doi: 10.1128/jb.00121-15
Belas, R. (2014). Biofilms, flagella, and mechanosensing of surfaces by bacteria. Trends Microbiol. 22, 517–527. doi: 10.1016/j.tim.2014.05.002
Bilecen, K., and Yildiz, F. H. (2009). Identification of a calcium-controlled negative regulatory system affecting Vibrio cholerae biofilm formation. Environ. Microbiol. 11, 2015–2029. doi: 10.1111/j.1462-2920.2009.01923.x
Bonomelli, C., Gil, P. M., and Schaffer, B. (2019). Effect of soil type on calcium absorption and partitioning in young avocado (Persea americana Mill.) trees. Agronomy Basel 9:11.
Chen, H. Y., Kandel, P. P., Cruz, L. F., Cobine, P. A., and De La Fuente, L. (2017). The major outer membrane protein MopB is required for twitching movement and affects biofilm formation and virulence in two Xylella fastidiosa strains. Mol. Plant Microbe Interact. 30, 896–905. doi: 10.1094/mpmi-07-17-0161-r
Cruz, L. F., Cobine, P. A., and De La Fuente, L. (2012). Calcium increases Xylella fastidiosa surface attachment, biofilm formation, and twitching motility. Appl. Environ. Microbiol. 78, 1321–1331. doi: 10.1128/aem.06501-11
Cruz, L. F., Parker, J. K., Cobine, P. A., and De La Fuente, L. (2014). Calcium-enhanced twitching motility in Xylella fastidiosa is linked to a single PilY1 homolog. Appl. Environ. Microbiol. 80, 7176–7185. doi: 10.1128/aem.02153-14
Das, J., Mokrzan, E., Lakhani, V., Rosas, L., Jurcisek, J. A., Ray, W. C., et al. (2017). Extracellular DNA and Type IV pilus expression regulate the structure and kinetics of biofilm formation by Nontypeable Haemophilus influenzae. mBio 8:e01466-17.
Davies, D. G., Parsek, M. R., Pearson, J. P., Iglewski, B. H., Costerton, J. W., and Greenberg, E. P. (1998). The involvement of cell-to-cell signals in the development of a bacterial biofilm. Science 280, 295–298. doi: 10.1126/science.280.5361.295
Ditta, G., Stanfield, S., Corbin, D., and Helinski, D. R. (1980). Broad host range DNA cloning system for gram-negative bacteria: construction of a gene bank of Rhizobium meliloti. Proc. Natl. Acad. Sci. U.S.A. 77, 7347–7351. doi: 10.1073/pnas.77.12.7347
Dreyfus, B., Garcia, J.-L., and Gillis, M. (1988). Characterization of Azorhizobium caulinodans gen. nov., sp. nov., a stem-nodulating nitrogen-fixing bacterium isolated from Sesbania rostrata. Int. J. Syst. Evol. Microbiol. 38, 89–98. doi: 10.1099/00207713-38-1-89
Dreyfus, B. L., and Dommergues, Y. R. (1981). nitrogen-fixing nodules induced by Rhizobium on the stem of the tropical legume Sesbania rostrata. FEMS Microbiol. Lett. 10, 313–317. doi: 10.1111/j.1574-6968.1981.tb06262.x
Dreyfus, B. L., Elmerich, C., and Dommergues, Y. R. (1983). Free-living Rhizobium strain able to grow on N2 as the sole nitrogen source. Appl. Environ. Microbiol. 45, 711–713. doi: 10.1128/aem.45.2.711-713.1983
Fishman, M. R., Zhang, J., Bronstein, P. A., Stodghill, P., and Filiatrault, M. J. (2018). Ca2+-induced two-component system CvsSR regulates the Type III secretion system and the extracytoplasmic function sigma factor AlgU in Pseudomonas syringae pv. tomato DC3000. Journal of Bacteriology 200: e00538-17.
Giacalone, D., Smith, T. J., Collins, A. J., Sondermann, H., Koziol, L. J., and O’toole, G. A. (2018). Ligand-mediated biofilm formation via enhanced physical interaction between a diguanylate cyclase and its receptor. mBio 9:e01254-18.
Gode-Potratz, C. J., Chodur, D. M., and Mccarter, L. L. (2010). Calcium and iron regulate swarming and type III secretion in Vibrio parahaemolyticus. J. Bacteriol. 192, 6025–6038. doi: 10.1128/jb.00654-10
Guttenplan, S. B., and Kearns, D. B. (2013). Regulation of flagellar motility during biofilm formation. FEMS Microbiol. Rev. 37, 849–871. doi: 10.1111/1574-6976.12018
Guttula, D., Yao, M., Baker, K., Yang, L., Goult, B. T., Doyle, P. S., et al. (2019). Calcium-mediated protein folding and stabilization of Salmonella biofilm-associated protein A. J. Mol. Biol. 431, 433–443. doi: 10.1016/j.jmb.2018.11.014
Hammer, B. K., and Bassler, B. L. (2003). Quorum sensing controls biofilm formation in Vibrio cholerae. Mol. Microbiol. 50, 101–104. doi: 10.1046/j.1365-2958.2003.03688.x
Hathroubi, S., Zerebinski, J., and Ottemann, K. M. (2018). Helicobacter pylori biofilm involves a multigene stress-biased response, including a structural role for flagella. mBio 9:e01973-18.
Hobley, L., Harkins, C., Macphee, C. E., and Stanley-Wall, N. R. (2015). Giving structure to the biofilm matrix: an overview of individual strategies and emerging common themes. FEMS Microbiol. Rev. 39, 649–669. doi: 10.1093/femsre/fuv015
Holscher, T., Bartels, B., Lin, Y. C., Gallegos-Monterrosa, R., Price-Whelan, A., Kolter, R., et al. (2015). Motility, chemotaxis and aerotaxis contribute to competitiveness during bacterial pellicle biofilm development. J. Mol. Biol. 427, 3695–3708. doi: 10.1016/j.jmb.2015.06.014
Huang, N., Pu, X., Zhang, J., Shen, H., Yang, Q., Wang, Z., et al. (2019). In vitro formation of Dickeya zeae MS1 biofilm. Curr. Microbiol. 76, 100–107. doi: 10.1007/s00284-018-1593-y
Jefferson, K. K. (2004). What drives bacteria to produce a biofilm? FEMS Microbiol. Lett. 236, 163–173. doi: 10.1111/j.1574-6968.2004.tb09643.x
Jiang, N., Liu, W., Li, Y., Wu, H., Zhang, Z., Alexandre, G., et al. (2016). A chemotaxis receptor modulates nodulation during the Azorhizobium caulinodans-Sesbania rostrata symbiosis. Appl. Environ. Microbiol. 82, 3174–3184. doi: 10.1128/aem.00230-16
Jung, Y.-C., Lee, M.-A., and Lee, K.-H. (2019). Role of flagellin-homologous proteins in biofilm formation by pathogenic Vibrio species. mBio 10:e01793-19.
Karatan, E., and Watnick, P. (2009). Signals, regulatory networks, and materials that build and break bacterial biofilms. Microbiol. Mol. Biol. Rev. 73, 310–347. doi: 10.1128/mmbr.00041-08
Karygianni, L., Ren, Z., Koo, H., and Thurnheer, T. (2020). Biofilm matrixome: extracellular components in structured microbial communities. Trends Microbiol. 28, 668–681. doi: 10.1016/j.tim.2020.03.016
Lasa, I., and Penades, J. R. (2006). Bap: a family of surface proteins involved in biofilm formation. Res. Microbiol. 157, 99–107. doi: 10.1016/j.resmic.2005.11.003
Liu, H., Wang, X., Qi, H., Wang, Q., Chen, Y., Li, Q., et al. (2017a). The infection and impact of Azorhizobium caulinodans ORS571 on wheat (Triticum aestivum L.). PLoS One 12:e0187947. doi: 10.1371/journal.pone.0187947
Liu, W., Li, Y., Bai, X., Wu, H., Bian, L., and Hu, X. (2019a). LuxR-type regulator AclR1 of Azorhizobium caulinodans regulates cyclic di-GMP and numerous phenotypes in free-living and symbiotic states. Mol. Plant Microbe Interact. 33, 528–538. doi: 10.1094/mpmi-10-19-0306-r
Liu, W., Sun, Y., Shen, R., Dang, X., Liu, X., Sui, F., et al. (2018a). A chemotaxis-like pathway of Azorhizobium caulinodans controls flagella-driven motility, which regulates biofilm formation, exopolysaccharide biosynthesis, and competitive nodulation. Mol. Plant Microbe Interact. 31, 737–749. doi: 10.1094/mpmi-12-17-0290-r
Liu, W., Yang, J., Sun, Y., Liu, X., Li, Y., Zhang, Z., et al. (2017b). Azorhizobium caulinodans transmembrane chemoreceptor TlpA1 involved in host colonization and nodulation on roots and stems. Front. Microbiol. 8:1327. doi: 10.3389/fmicb.2017.01327
Liu, X., Liu, W., Sun, Y., Xia, C., Elmerich, C., and Xie, Z. (2018b). A cheZ-Like gene in Azorhizobium caulinodans is a key gene in the control of chemotaxis and colonization of the host plant. Appl. Environ. Microbiol. 84:e01827-17.
Liu, X., Xie, Z., Wang, Y., Sun, Y., Dang, X., and Sun, H. (2019b). A dual role of amino acids from Sesbania rostrata seed exudates in the chemotaxis response of Azorhizobium caulinodans ORS571. Mol. Plant Microbe Interact. 32, 1134–1147. doi: 10.1094/mpmi-03-19-0059-r
Liu, X., Zhang, K., Liu, Y., Xie, Z., and Zhang, C. (2019c). Oxalic acid from Sesbania rostrata seed exudates mediates the chemotactic response of Azorhizobium caulinodans ORS571 using multiple strategies. Front. Microbiol. 10:2727. doi: 10.3389/fmicb.2019.02727
Ma, L., Conover, M., Lu, H., Parsek, M. R., Bayles, K., and Wozniak, D. J. (2009). Assembly and development of the Pseudomonas aeruginosa biofilm matrix. PLoS Pathog. 5:e1000354. doi: 10.1371/journal.ppat.1000354
Marx, C. J., and Lidstrom, M. E. (2002). Broad-host-range cre-lox system for antibiotic marker recycling in gram-negative bacteria. Biotechniques 33, 1062–1067. doi: 10.2144/02335rr01
McCall, A. D., Pathirana, R. U., Prabhakar, A., Cullen, P. J., and Edgerton, M. (2019). Candida albicans biofilm development is governed by cooperative attachment and adhesion maintenance proteins. NPJ Biofilms Microbiomes 5:21.
Mukherjee, S., and Bassler, B. L. (2019). Bacterial quorum sensing in complex and dynamically changing environments. Nat. Rev. Microbiol. 17, 371–382. doi: 10.1038/s41579-019-0186-5
Papenfort, K., and Bassler, B. L. (2016). Quorum sensing signal–response systems in Gram-negative bacteria. Nat. Rev. Microbiol. 14, 576–588. doi: 10.1038/nrmicro.2016.89
Park, J. H., Jo, Y., Jang, S. Y., Kwon, H., Irie, Y., Parsek, M. R., et al. (2015). The cabABC operon essential for biofilm and rugose colony development in Vibrio vulnificus. PLoS Pathog. 11:e1005192. doi: 10.1371/journal.ppat.1005192
Pu, M., Storms, E., Chodur, D. M., and Rowe-Magnus, D. A. (2020). Calcium-dependent site-switching regulates expression of the atypical iam pilus locus in Vibrio vulnificus. Environ. Microbiol. doi: 10.1111/1462-2920.14763
Reinhardt, J. M., and Bardy, S. L. (2018). Partitioning protein ParP directly links chemotaxis to biofilm dispersal in Pseudomonas aeruginosa. bioRxiv [Preprint]. doi: 10.1101/330878
Rinaudi, L., Fujishige, N. A., Hirsch, A. M., Banchio, E., Zorreguieta, A., and Giordano, W. (2006). Effects of nutritional and environmental conditions on Sinorhizobium meliloti biofilm formation. Res. Microbiol. 157, 867–875. doi: 10.1016/j.resmic.2006.06.002
Sarkisova, S., Patrauchan, M. A., Berglund, D., Nivens, D. E., and Franklin, M. J. (2005). Calcium-induced virulence factors associated with the extracellular matrix of mucoid Pseudomonas aeruginosa biofilms. J. Bacteriol. 187, 4327–4337. doi: 10.1128/jb.187.13.4327-4337.2005
Scharf, B. E., Hynes, M. F., and Alexandre, G. M. (2016). Chemotaxis signaling systems in model beneficial plant-bacteria associations. Plant Mol. Biol. 90, 549–559. doi: 10.1007/s11103-016-0432-4
Shen, R., Liu, W., Sun, Y., Li, R., and Xie, Z. (2018). Function analysis of flagellar genes fliN and FliM in Azorhizobium caulinodans ORS571. Acta Microbiol. Sin. 58, 882–892.
Simm, R., Morr, M., Kader, A., Nimtz, M., and Romling, U. (2004). GGDEF and EAL domains inversely regulate cyclic di-GMP levels and transition from sessility to motility. Mol. Microbiol. 53, 1123–1134. doi: 10.1111/j.1365-2958.2004.04206.x
Smith, D., Wang, J. H., Swatton, J. E., Davenport, P., Price, B., Mikkelsen, H., et al. (2006). Variations on a theme: diverse N-acyl homoserine lactone-mediated quorum sensing mechanisms in gram-negative bacteria. Sci. Prog. 89, 167–211. doi: 10.3184/003685006783238335
Sourjik, V., and Wingreen, N. S. (2012). Responding to chemical gradients: bacterial chemotaxis. Curr. Opin. Cell Biol. 24, 262–268. doi: 10.1016/j.ceb.2011.11.008
Sun, Y., Liu, Y., Liu, X., Dang, X., Dong, X., and Xie, Z. (2020). Azorhizobium caulinodans c-di-GMP phosphodiesterase Chp1 involved in motility, EPS production, and nodulation of the host plant. Appl. Microbiol. Biotechnol. 104, 2715–2729. doi: 10.1007/s00253-020-10404-6
Sun, Y., Xie, Z., Liu, W., and Guo, H. (2019). Prediction and functional analysis of GGDEF/EAL domain-containing proteins in Azorhizobium caulinodans ORS571. Acta Microbiol. Sin. 59, 2000–2012.
Sutherland, I. (2001). Biofilm exopolysaccharides: a strong and sticky framework. Microbiology 147, 3–9. doi: 10.1099/00221287-147-1-3
Tallawi, M., Opitz, M., and Lieleg, O. (2017). Modulation of the mechanical properties of bacterial biofilms in response to environmental challenges. Biomater. Sci. 5, 887–900. doi: 10.1039/c6bm00832a
Tischler, A. H., Lie, L., Thompson, C. M., and Visick, K. L. (2018). Discovery of calcium as a biofilm-promoting signal for Vibrio fischeri reveals new phenotypes and underlying regulatory complexity. J. Bacteriol. 200:18.
Vlamakis, H., Chai, Y., Beauregard, P., Losick, R., and Kolter, R. (2013). Sticking together: building a biofilm the Bacillus subtilis way. Nat. Rev. Microbiol. 11, 157–168. doi: 10.1038/nrmicro2960
Voberkova, S., Hermanova, S., Hrubanova, K., and Krzyzanek, V. (2016). Biofilm formation and extracellular polymeric substances (EPS) production by Bacillus subtilis depending on nutritional conditions in the presence of polyester film. Folia Microbiol. 61, 91–100. doi: 10.1007/s12223-015-0406-y
Wang, D., Xu, A., Elmerich, C., and Ma, L. Z. (2017). Biofilm formation enables free-living nitrogen-fixing rhizobacteria to fix nitrogen under aerobic conditions. ISME J. 11, 1602–1613. doi: 10.1038/ismej.2017.30
Willett, J. L. E., Ji, M. M., and Dunny, G. M. (2019). Exploiting biofilm phenotypes for functional characterization of hypothetical genes in Enterococcus faecalis. NPJ Biofilms Microbiomes 5:23.
Xu, Z., Zhang, H., Sun, X., Liu, Y., Yan, W., Xun, W., et al. (2018). Bacillus velezensis wall teichoic acids is required for biofilm formation and root colonization. Appl. Environ. Microbiol. 85:e02116-18.
Yang, X., Shen, R., Sun, Y., Li, R., and Xie, Z. (2019). Functional characterization of diguanylate cyclase metabolism-related gene in Azorhizobium caulinodans ORS571. Acta Microbiol. Sin. 59, 1500–1511.
Yang, Y., Li, Y., Gao, T., Zhang, Y., and Wang, Q. (2018). C-di-GMP turnover influences motility and biofilm formation in Bacillus amyloliquefaciens PG12. Res. Microbiol. 169, 205–213. doi: 10.1016/j.resmic.2018.04.009
Yaryura, P. M., Leon, M., Correa, O. S., Kerber, N. L., Pucheu, N. L., and Garcia, A. F. (2008). Assessment of the role of chemotaxis and biofilm formation as requirements for colonization of roots and seeds of soybean plants by Bacillus amyloliquefaciens BNM339. Curr. Microbiol. 56, 625–632. doi: 10.1007/s00284-008-9137-5
Keywords: Azorhizobium caulinodans, calcium, biofilm, chemotaxis, quorum sensing, eDNA
Citation: Liu X, Zhang K, Liu Y, Zou D, Wang D and Xie Z (2020) Effects of Calcium and Signal Sensing Systems on Azorhizobium caulinodans Biofilm Formation and Host Colonization. Front. Microbiol. 11:563367. doi: 10.3389/fmicb.2020.563367
Received: 26 June 2020; Accepted: 25 August 2020;
Published: 16 September 2020.
Edited by:
Jacob George Malone, John Innes Centre, United KingdomReviewed by:
Gladys Alexandre, The University of Tennessee, Knoxville, United StatesLuyan Zulie Ma, Institute of Microbiology, Chinese Academy of Sciences, China
Copyright © 2020 Liu, Zhang, Liu, Zou, Wang and Xie. This is an open-access article distributed under the terms of the Creative Commons Attribution License (CC BY). The use, distribution or reproduction in other forums is permitted, provided the original author(s) and the copyright owner(s) are credited and that the original publication in this journal is cited, in accordance with accepted academic practice. No use, distribution or reproduction is permitted which does not comply with these terms.
*Correspondence: Zhihong Xie, zhxie@yic.ac.cn
†These authors have contributed equally to this work