- 1Department of Plant and Microbial Biology, University of Zurich, Zurich, Switzerland
- 2Department of Life Sciences, University of Trieste, Trieste, Italy
Paraburkholderia phymatum is a rhizobial strain that belongs to the beta-proteobacteria, a group known to form efficient nitrogen-fixing symbioses within root nodules of several legumes, including the agriculturally important common bean. The establishment of the symbiosis requires the exchange of rhizobial and plant signals such as lipochitooligosaccharides (Nod factors), polysaccharides, and flavonoids. Inspection of the genome of the competitive rhizobium P. phymatum revealed the presence of several polysaccharide biosynthetic gene clusters. In this study, we demonstrate that bceN, a gene encoding a GDP-D-mannose 4,6-dehydratase, which is involved in the production of the exopolysaccharide cepacian, an important component of biofilms produced by closely related opportunistic pathogens of the Burkholderia cepacia complex (Bcc), is required for efficient plant colonization. Wild-type P. phymatum was shown to produce cepacian while a bceN mutant did not. Additionally, the bceN mutant produced a significantly lower amount of biofilm and formed less root nodules compared to the wild-type strain with Phaseolus vulgaris as host plant. Finally, expression of the operon containing bceN was induced by the presence of germinated P. vulgaris seeds under nitrogen limiting conditions suggesting a role of this polysaccharide in the establishment of this ecologically important symbiosis.
Introduction
Rhizobia are phylogenetically diverse soil bacteria, which possess the ability to infect the roots of certain legumes and induce nodules on roots or stems. The nodules represent specialized root organs in which rhizobia fix atmospheric nitrogen (N2) into ammonia, thereby giving legumes a pronounced growth advantage in nitrogen (N) deprived soils (Masson-Boivin et al., 2009; Oldroyd et al., 2011; Udvardi and Poole, 2013). Until recently, rhizobia were thought to belong exclusively to the alpha-subclass of proteobacteria (alpha-rhizobia, isolated first in 1888 by Beijerinck). This situation changed in 2001, when two genera belonging to the beta-proteobacteria (Burkholderia and Cupriavidus) were discovered to form nitrogen-fixing root nodules (beta-rhizobia) (Chen et al., 2001; Moulin et al., 2001; Bontemps et al., 2010). For the legume nodulating strains that belong to the environmental clade of the genus Burkholderia, the new genus Paraburkholderia has been recently proposed (Sawana et al., 2014; Beukes et al., 2017). Paraburkholderia strains have originally been isolated from South American mimosoid and South African papillionoid legumes (Chen et al., 2003, 2005; Elliott et al., 2007; dos Reis et al., 2010; Mishra et al., 2012; Bournaud et al., 2013; Lemaire et al., 2015, 2016). The diversity of mimosoid-nodulating Paraburkholderia is high and includes species such as P. phymatum, which is promiscuous and highly competitive against other alpha- and beta-rhizobial strains for nodulating several mimosoid and papilionoid legumes of major agricultural importance including the common bean (Phaseolus vulgaris) (Elliott et al., 2009; Talbi et al., 2010; Melkonian et al., 2014; Moulin et al., 2014; Lardi et al., 2017a). The establishment of a rhizobia-legume nitrogen-fixing symbiosis is a highly complex and regulated process involving the exchange of a series of specific bacterial and plant signals. Among these signals, bacterial lipochitooligosaccharides (Nod factors) and surface polysaccharides as well as flavonoids secreted by plant roots play crucial roles (Masson-Boivin et al., 2009; Downie, 2010). Rhizobia produce a large variety of surface polysaccharides such as exopolysaccharides, outer membrane-localized lipopolysaccharide (LPS), capsule polysaccharide (CPS), K-antigen polysaccharide (KPS), cyclic beta-glucans, and glucomannan. LPS as well as exopolysaccharides, KPS, and cyclic glucans are important for the interaction with the host plant (Breedveld and Miller, 1994; Cheng and Walker, 1998; Niehaus and Becker, 1998; Pellock et al., 2000; Fraysse et al., 2003; Laus et al., 2006; Skorupska et al., 2006; Jones et al., 2007; Marczak et al., 2017). Exopolysaccharides are secreted into the root environment and are generally only weakly associated with the bacterial surface. They are species- or strain-specific and have heterogeneous structures containing different monosaccharides (D-glucose, D-galactose, D-mannose, L-rhamnose, D-glucuronic acid, and D-galacturonic acid) that are linked in a linear or branched structure. The degree of polymerization, the type of glycosidic bonds as well as the variety of the non-carbohydrate modifications (e.g., acetyl, pyruvyl, or succinyl groups) also greatly contribute to the diversity of bacterial exopolysaccharide matrices. Alpha-rhizobial exopolysaccharide molecules have been shown to be important for the development of an effective symbiosis (Leigh et al., 1985; Djordjevic et al., 1987; Battisti et al., 1992; Urzainqui and Walker, 1992; Gonzalez et al., 1996; Pellock et al., 2000). Exopolysaccharide-deficient mutants of rhizobia that infect plants producing indeterminate-type nodules (e.g., Rhizobium leguminosarum and Sinorhizobium meliloti) were compromised in their ability to induce root hair curling and to form infection-threads (Leigh et al., 1985; Cheng and Walker, 1998; Niehaus and Becker, 1998; Pellock et al., 2000). In addition, exopolysaccharides represent the major component of the biofilm matrix and are thus important for bacterial attachment to surfaces, protection against environmental stresses and antimicrobial compounds, and evasion of host defense responses (Santos et al., 2001; Downie, 2010; Ferreira et al., 2010, 2011; Geddes et al., 2014; Maroti et al., 2015; Arnold et al., 2018). Some polysaccharides have also been shown to suppress plant defense responses and to be involved in plant signaling by binding to specific membrane-spanning receptor-like proteins with significant similarity to Nod factor receptor 1 (NFR1) (Kawaharada et al., 2015). In beta-rhizobia such as the Paraburkholderia strains, nothing is known about the requirement of specific polysaccharides for the establishment of a successful symbiosis with legumes. However, it was shown that several Burkholderia and Paraburkholderia species, including P. phymatum, produce the polysaccharide cepacian (CEP) (Ferreira et al., 2010). In Burkholderia strains of the Burkholderia cepacia complex (Bcc), this is the main exopolysaccharide produced (Cescutti et al., 2000; Conway et al., 2004; Ferreira et al., 2007; Cuzzi et al., 2014). In this group of opportunistic pathogens that can cause lung infections in cystic fibrosis (CF), immunocompromised or granulomatous disease patients, CEP was associated with bacterial persistence in the lung (Conway et al., 2004; Cunha et al., 2004; Sousa et al., 2007; Zlosnik et al., 2011; Ferreira et al., 2019) and shown to act as a protective barrier against antibiotics and other stresses such as oxidative stress, desiccation, and metal ion stress (Bylund et al., 2006; Benincasa et al., 2009; Ferreira et al., 2010, 2011; Cuzzi et al., 2012). Importantly, many of these potentially pathogenic Bcc strains, which produce CEP, are also naturally associated with plants (Coenye and Vandamme, 2003; Eberl and Vandamme, 2016). B. cenocepacia for example was originally described as a pathogen of onions and inhabits the soil and rhizosphere of several plants (Burkholder, 1950; LiPuma et al., 2002; Ramette et al., 2005; Dalmastri et al., 2007; Lee and Chan, 2007).
The structure of CEP has been elucidated; it consists of the five monosaccharides D-glucose, D-mannose, D-rhamnose, D-glucuronic acid, and D-galactose in a 1:1:1:1:3 ratio and is modified in the side chains with acetyl groups (Cerantola et al., 1999; Cescutti et al., 2000, 2010). The first step in CEP biosynthesis is the formation of sugar-nucleotide precursors, which are assembled by the action of several glycosyltranferases into a heptasaccharide repeating unit. CEP is acetylated during the repeating unit process at the inner membrane and the number of substitution seems to be strain dependent (Cescutti et al., 2010). The lipid carrier-linked heptasaccharide repeating units are exported across the inner membrane and polymerized in the periplasm by the Wzy-dependent pathway that involves a transport protein called flippase and polysaccharide polymerase, respectively (Moreira et al., 2003; Ferreira et al., 2010).
In Bcc members and other animal and plant pathogenic non-Bcc isolates, CEP is synthetized by two gene clusters (bce-I and bce-II, for B. cepacia exopolysaccharide), which are separated by 130 to 314 kb (Ferreira et al., 2010). Interestingly, in the genome of environmental strains previously belonging to the Burkholderia genus (e.g., P. phymatum STM815, P. xenovorans LB400, P. phytofirmans PsJN, P. graminis C4D1M, and Caballeronia glathei DSM 50014), the CEP genes are not separated, but are organized as a continuous gene cluster (Moreira et al., 2003; Ferreira et al., 2010, 2019).
In this work, we demonstrate for the first time a role of CEP in the symbiotic interaction of a Paraburkholderia strain with its host plant. Through mutagenesis, the bceN gene of P. phymatum, which encodes a protein with GDP-D-mannose 4,6-dehydratase enzyme activity (Sousa et al., 2013), was shown to be important for CEP production and biofilm formation. Moreover, common bean plants infected with a P. phymatum bceN mutant produced fewer nodules on the roots but otherwise performed nitrogen fixation as efficiently as nodules infected with the wild type, suggesting a role of CEP in root attachment, i.e., the first step of establishing a symbiosis. Finally, we show here that expression of the operon containing bceN is induced under nitrogen-limiting conditions and in the presence of germinated seeds or root exudates.
Materials and Methods
Bacterial Strains, Plasmids, and Growth Conditions
The bacterial strains, plasmids, and primers employed in this work are listed in Supplementary Table S1. Escherichia coli and P. phymatum strains were routinely maintained on Luria-Bertani (LB) (Miller, 1972) and ABS (AB-minimal medium with 15 mM sodium succinate as carbon source) (15.13 mM (NH4)2SO4, 42.25 mM Na2HPO4, 22 mM KH2PO4, 51.33 mM NaCl, 2 mM MgCl2, 0.1 mM CaCl2, 3 μM FeCl3, 15 mM succinate, and 1.5% agar) plate, respectively. E. coli cells were cultivated under aerobic conditions in liquid LB medium, whereas P. phymatum cells were cultivated in the modified LB-NaCl (LB medium without salt: 10 g tryptone and 5 g yeast extract per liter). Where applicable, the following antibiotic concentrations were used: chloramphenicol (20 μg/ml for E. coli and 80 μg/ml for P. phymatum), kanamycin (25 μg/ml for E. coli and 50 μg/ml for P. phymatum). The variations of AB-minimal liquid medium used in this study are ABS (see above), (A)BS that is ABS without nitrogen source (15.13 mM Na2SO4, 42.25 mM Na2HPO4, 22 mM KH2PO4, 51.33 mM NaCl, 2 mM MgCl2, 0.1 mM CaCl2, 3 μM FeCl3, and 15 mM succinate), and (A)B that is ABS without nitrogen and carbon source (15.13 mM Na2SO4, 42.25 mM Na2HPO4, 22 mM KH2PO4, 51.33 mM NaCl, 2 mM MgCl2, 0.1 mM CaCl2, and 3 μM FeCl3). In nitrogen limited conditions, (A)BS medium was supplemented with 0.3 mM NH4Cl. To measure biofilm production, the cells were grown in nitrogen limited (A)BM medium, which contains 10 mM mannitol as carbon source.
Construction of a P. phymatum bceN Mutant and Reporter Strains
Chromosomal DNA of P. phymatum STM815 was isolated by using GenEluteTM Bacterial Genomic DNA Kit (Sigma-Aldrich, St. Louis, MO, United States). Plasmid DNA from E. coli strains was obtained by using the QIAprep Spin Miniprep Kit (Qiagen, Hilden, Germany). To generate a bceN mutant, an internal fragment of Bphy_1069 was PCR amplified with primers Bphy1069_IM_F_EcoRI and Bphy1069_IM_R_XbaI, cloned into a pSHAFT2 plasmid between the EcoRI and XbaI sites. The plasmid pSHAFT-bceN was then transferred into the wild-type P. phymatum to generate the Bphy_1069 insertion mutant (bceN-IM). Genomic integration of the plasmid was confirmed with PCR using primers Bphy1069_veri_F and pSHAFT_F. The coding sequence of Bphy_1069 was produced by PCR with primers Bphy_1069_comp_F_EcoRI and Bphy_1069_comp_R_BamHI and cloned into pBBR1MCS-2 between the EcoRI and BamHI sites. The constructed plasmid pBBR1MCS2-bceN was transferred into bceN-IM to complement the mutant strain, resulting in the complemented strain (bceN-COMP). In bceN-COMP, bceN expression is driven by the lacZ promoter. We also transferred the vector pBBR1MCS-2 into bceN-IM as an empty vector control strain of bceN-COMP (bceN-pBBR). In order to quantify the expression of the CEP encoding genes, a promoter fusion strain was constructed. The promoter region of the bceOVN operon was cloned using primers bceOVNpro_F_XbaI and bceOVNpro_R_EcoRI into a pPROBE-NT vector between the XbaI and EcoRI sites. The resulting plasmid and the empty vector pPROBE-NT were transconjugated into wild-type P. phymatum to create the promoter reporter (WT-pPROBE-bceOVN) and the negative control strain (WT-pPROBE), respectively. The correct sequence of the clones was confirmed at Microsynth AG (Balgach, St. Gallen, Switzerland).
Phenotypical Analysis
Paraburkholderia phymatum strains (WT, bceN-IM and bceN-COMP) were grown aerobically in liquid LB-NaCl and ABS media (three independent cultures; 50 ml liquid in 250 ml Erlenmeyer flasks at 30°C with shaking at 220 rpm). To quantify transcription of the bceN operon, the GFP expression of the promoter reporter was recorded in a 96-well plate (Falcon, Corning, United States) by a TECAN plate reader (TECAN Infinite M200 PRO, Tecan Trading AG, Switzerland). Cells of strain WT-pPROBE-bceOVN together with the negative control WT-pPROBE were washed twice with (A)B medium and the OD600 was normalized to 0.2 in four different media: ABS, (A)BS supplemented with 0.3 mM NH4Cl, in the presence or absence of bean root exudates. In the media containing root exudates, half the volume of the medium was replaced by freshly prepared root exudate solution (final concentration 50% root exudates). Each strain was tested with three independent biological clones in technical duplicates. The cells were added into a 96-well plate, which was then incubated at 30°C for 48 h. OD600 and GFP were measured by TECAN plate reader.
Polysaccharide production was assayed as previously described (Lardi et al., 2017b). Three independent clones of each strain were tested on three media containing 0.06% yeast extract, 1.5% Agar, and 1% of one of three different carbon sources (mannitol, glucose, and galactose). The plates were incubated at 30°C for three days before analyzing the results. Biofilm assays were carried out as previously described (Huber et al., 2001) with slight modifications. The cells were grown in nitrogen limited (A)BM medium. The plate was incubated at 30°C for 5 days before OD550 (growth) and OD570 (crystal violet) measurement.
Exopolysaccharides Extraction and Nuclear Magnetic Resonance (NMR) Analysis
Paraburkholderia phymatum wild-type and mutant strains were cultivated in the modified LB-NaCl liquid medium. Cells were washed in liquid YEM (0.06% yeast extract, 1% mannitol) twice and spread on 9 cm YEM agar plate at the density of OD600 0.1 cells per plate. The plates were incubated at 30°C for 4 days. The bacterial lawn was collected with 0.9% NaCl (about 3 mL per dish), gently stirred at 10°C for 2 h, centrifuged at 22,400 × g at 4°C for 30 min to separate the cells from the supernatant, which was subsequently precipitated with four volumes of cold ethanol. The precipitated material was recovered by centrifugation at 1,900 × g at 4°C for 30 min and subsequently dried to eliminate the alcohol. The samples were dissolved in 0.1 M NaCl and dialyzed first against 0.1 M NaCl and then against water. Polysaccharides were recovered by lyophilization. 1H nuclear magnetic resonance (NMR) spectra were recorded on a 500 MHz VARIAN spectrometer. Polysaccharides (3.7 mg of exopolysaccharide produced by the wild-type strain, 2.6 mg by bceN-IM and 2.3 mg bceN-COMP) were exchanged two times with 99.9% D2O by lyophilization and subsequently dissolved in 0.6 mL of 99.96% D2O. 1H NMR spectra were recorded at 50°C. Polysaccharides (5.1 mg of exopolysaccharides produced by the wild-type strain, 4.2 mg by bceN-IM strain, and 4.7 mg by bceN-COMP) were dissolved in 4 mL of water (16 h with stirring) and sonicated using a Branson sonifier equipped with a microtip at 2.8 Å, in order to decrease their molecular masses. The samples were cooled in an ice bath and sonicated using 10 bursts of 1 min each, separated by 1 min intervals. In order to de-acetylate the exopolysaccharide, a solution of NaOH was added to each exopolysaccharide solution to a final NaOH concentration of 0.01 M. The reaction was let to proceed 5 h under a N2 flow with stirring, followed by extensive dialysis against water. Afterward, the exopolysaccharide solutions were taken to pH 6.6 and recovered by lyophilization. They were exchanged with 99.9% D2O as described above, subsequently dissolved in 0.6 mL of 99.96% D2O and subjected to 1H NMR spectroscopy at 70°C.
Plant Infection Test, Root Attachment Assay, and Production of Root Exudates
Common bean seedlings (Phaseolus vulgaris, cv. Negro Jamapa) were surface sterilized as previously described (Talbi et al., 2010). Seeds were subsequently placed on 0.8% agar plates and incubated in the dark at 28°C. After 40 h, germinated seedlings were planted into autoclaved yogurt-jars containing vermiculite (VTT-Group, Muttenz, Switzerland) and 170 ml diluted Jensen medium (Hahn and Hennecke, 1984). The bacterial cells of each strain were grown in LB-NaCl liquid medium overnight (15 h) and washed twice in A(B) medium. Then the OD600 of each strain was adjusted to 0.025. A total of 1 ml of normalized cells (about 107 cells) were directly inoculated on to each germinated seedling. Important symbiotic properties (nodule number, nodule dry weight, and nitrogenase activity of bacteroids) were determined as described previously (Göttfert et al., 1990). Importantly, nitrogenase activity was normalized with nodule dry weight. The plants were grown with the following parameters: temperature: 22°C at night and 25°C during the day; light: approximately 16 h (200 μM intensity); humidity: 60%. The plants were harvested 21 days post infection (dpi).
In the root attachment assay, germinated beans and bacterial cells to be tested were prepared in the same way as for the plant infection tests. Each bean root with length between 1 and 2.5 cm was submerged in 1 ml bacterial inoculum for 4 h. To remove the loosely attached bacteria, individual beans were briefly rinsed with water and then twice washed in 20 ml PBS-S buffer (130 mM NaCl, 7 mM Na2HPO4, 3 mM NaH2PO4, pH 7.0, 0.02% Silwet L-77) with vigorous agitation at 180 rpm for 20 min (Bulgarelli et al., 2012). Afterward, each root was carefully cut off and homogenized with one 7 mm glass bead in 500 μl (A)B medium by a bead mill (TissueLyser II, QIAGEN). The homogenate was then diluted serially and plated on LB-NaCl agar plates. The number of attached cells was determined by colony forming units.
Root exudates were collected in a sterile manner by the following procedure. Bean seeds were germinated as in the plant infection tests. A sterile loose cotton ball was placed on the surface of 30 ml sterile dH2O in a 50 ml standing syringe with a stopper. Eight germinated seeds were carefully placed on the cotton ball with roots soaking into the water. Seedlings were incubating at 28°C for 4 days. Root exudate water solution was collected and immediately used in the experiments.
The induction of promoter GFP fusion reporters by germinated bean seeds were visualized on soft agar plates. To do so, bean seeds were surface sterilized and germinated, the reporter strain was cultivated and washed in the same way as in the plant infection tests. The cells were added into the melted soft agar (0.8%) media ABS and (A)BS with 0.3 mM NH4Cl to reach a final OD600 = 0.1 at approximately 37°C and was immediately poured into a 9-cm petri-dish. Before the plate had solidified, one germinated bean was placed on top of a soft agar medium plate with the root sticking into the medium. The images of the plates were taken every 24 h using a custom built fluorescence imaging device (Infinity 3 camera, Lumenera, Canada). The excitation/emission wavelength of GFP is 490 nm/510 nm.
Bioinformatics and Statistical Analyses
The statistical analyses of biofilm formation, symbiotic properties of bean nodules, root attachment assay, and GFP induction of promoter fusions were performed by GraphPad Prism 7.00 using unpaired t-tests (p-value: ****<0.0001, ∗∗∗<0.001, ∗∗<0.01, and ∗<0.05). For bce cluster analysis (Supplementary Figure S4), the DNA sequence from P. phymatum STM815 bce-I cluster (position: 1183179–1199877) and bce-II cluster (1201886–1215931) was searched with blast-2.9.0 + against a self-made blast database. The blast database contained all DNA sequences of the representing genomes on NCBI from the 16S rRNA gene sequences used to create Figure 1 in the published review (Eberl and Vandamme, 2016). For the following five strains B. arboris R-24201, B. seminalis R24196, B. tuberum STM678, B. unamae MTI-641, and B. silvatlantica SRMrh-20 no reference genome was available on NCBI. The percent of identity was above 72% for each blast result.
Results
Genetic Organization of the bce Gene Cluster in P. phymatum
Previous work has identified the CEP biosynthetic gene cluster in P. phymatum (Ferreira et al., 2010). The products of this gene cluster containing 22 genes showed high amino acid identity (from 61 to 85%) with enzymes required for the biosynthesis of the polysaccharide CEP in the opportunistic pathogen Bcc strains B. cenocepacia H111 and B. cenocepacia J2315 (Figure 1). In strains H111, J2315 and other Bcc members, the CEP genes are located in two separated clusters (bce-I and bce-II). In contrast, the genomic organization in P. phymatum STM815 differs in that the respective genes were found adjacent to each other forming one continuous cluster (Figure 1) (Ferreira et al., 2010): genes of the bce-I cluster (bceABCDEFGHIJK, Bphy_1056–Bphy_1066) are followed by the bce-II cluster (bceNVOPQRST, Bphy_1069–Bphy_1077). While the gene products BceA (Bphy_1056), BceC (Bphy_1058), BceN (Bphy_1069), and BceT (Bphy_1077) are predicted to be involved in sugar-nucleotide biosynthesis, BceB (Bphy_1057), BceG (Bphy_1062), BceH (Bphy_1063), BceJ (Bphy_1065), BceK (Bphy_1066), BceO (Bphy_1071), BceR (Bphy_1074), and BceS (Bphy_1075) are predicted to play a role in heptasaccharide repeat-unit assembly, whereas BceD (Bphy_1059), BceE (Bphy_1060), BceF (Bphy_1061), BceI (Bphy_1064), and BceQ (Bphy_1073) are needed for polymerization and export. Bphy_1070 (bceV) codes for a putative lipase, which is missing in the bce cluster of pathogenic Burkholderia strains and a subgroup of Paraburkholderia strains such as P. tropica, P. unamae, and P. mimosarum. The P. phymatum bce cluster also contains genes encoding proteins of unknown function, such as Bphy_1072 (bceP), Bphy_1076, and Bphy_1067-68. The gene bceP is the first in a putative operon (bcePQR) and the corresponding protein contains a six-bladed beta-propeller domain that can also be found in TolB proteins. The two genes Bphy_1067-68 are located in a putative operon downstream of bceK (Figure 1) and are only present in the phylogenetically closely related Paraburkholderia strains P. caribensis, P. hospita, and P. terrae. Bphy_1067 displays homology to transcriptional regulators of the xenobiotic response element (XRE) family that have a helix-turn-helix DNA-binding motif similar to that of the CI repressor and the Cro proteins of λ bacteriophage. Bphy_1068 codes for a hipA (toxin) domain-containing protein and is often co-occurring with Bphy_1067 in the genomes of other Paraburkholderia strains.
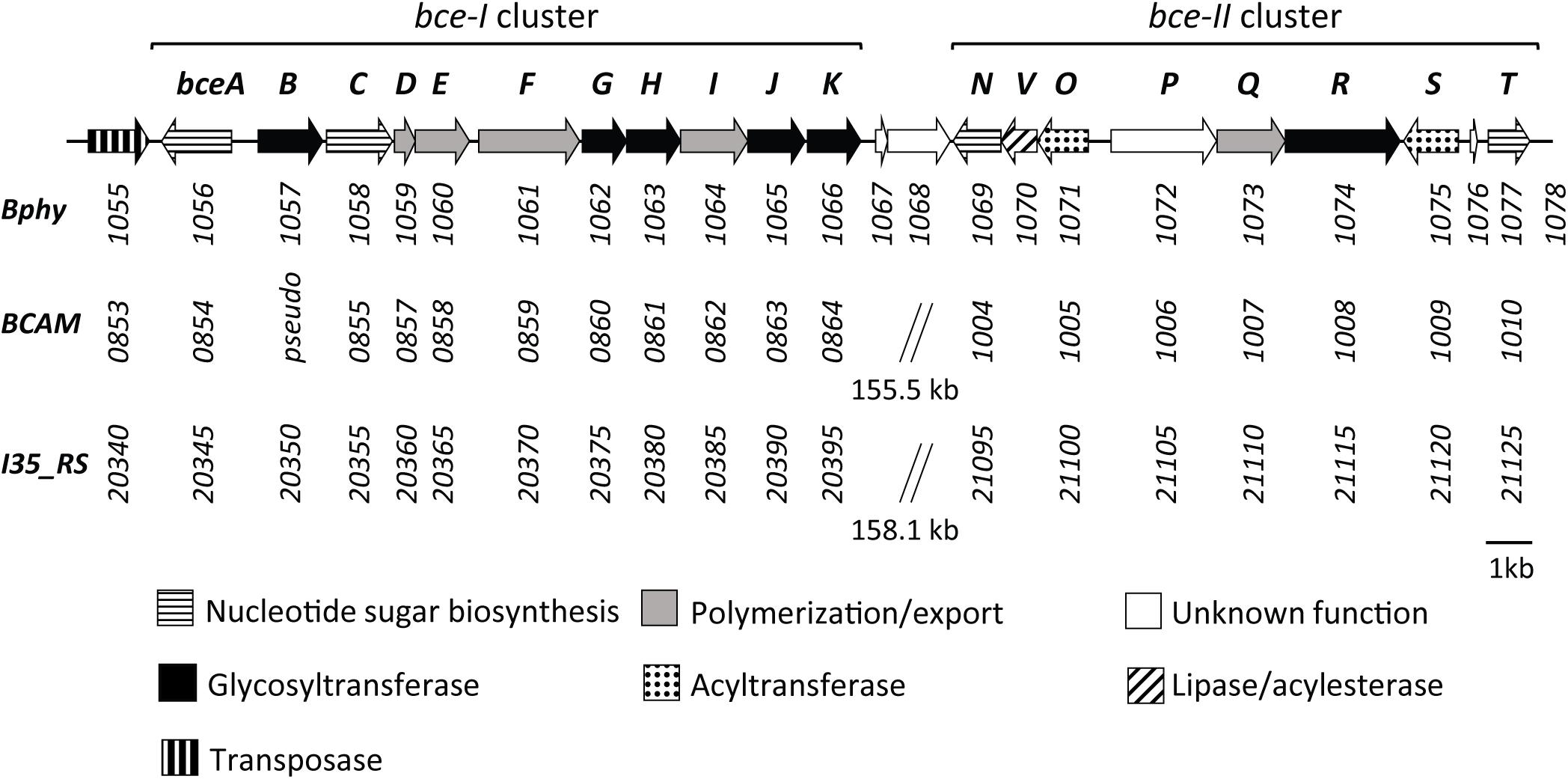
Figure 1. Genetic organization of the bce gene clusters in P. phymatum STM815 (Bphy) in comparison with B. cenocepacia J2315 (BCAM), and B. cenocepacia H111 (I35_RS). Gene numbers are listed under each gene indicating a sequential arrangement of genes in two bce clusters (bce-I and bce-II), which are either continuous or separated. Functional categorization adapted from Ferreira et al. (2010).
A comparison with the bce genes present in strain J2315 and other Bcc strains revealed that two genes, bceM and bceU were missing in the P. phymatum STM815 bce cluster. In J2315 and other Bcc strains, these two genes are located upstream (bceM) and downstream (bceU) of the bce-II cluster. BceM codes for a GDP-6-deoxy-D-lyxo-4-hexulose reductase (also called RMD) and bceU for a membrane protein involved in the acylation of CEP.
Interestingly, upstream of the first gene of the bce cluster we identified a gene (Bphy_1055) potentially coding for a transposase A-like protein (Figure 1), which could be involved in the acquisition or transfer of this gene cluster between Burkholderia strains.
Mutation of bceN Reduces Exopolysaccharides and Biofilm Production in P. phymatum
BceN (Bphy_1069) is the last gene of the bceOVN operon and codes for a GDP-D-mannose 4,6-dehydratase with 82% identity to the previously characterized BceN of Bcc strains H111 and J2315 (Sousa et al., 2013). This enzyme catalyzes the conversion of GDP-D-mannose into the sugar nucleotide GDP-4-keto-6-deoxy-D-mannose, which is the precursor of GDP-D-rhamnose, one of the sugar nucleotides of CEP. Interestingly, two additional bceN paralogs have been found in two other potential exopolysaccharides clusters in P. phymatum genome (Bphy_6734 and Bphy_2471). Since GDP-D-rhamnose is one important sugar nucleotide required for CEP synthesis, the bceN gene of P. phymatum was inactivated by the insertion of a suicide plasmid (see section “Materials and Methods”). The bceN mutant strain (called bceN-IM) was complemented by introducing a pBBR1MCS-2 plasmid carrying the bceN gene (bceN-COMP). While the bceN mutant did not show a growth defect under aerobic conditions in rich (LB without salt) and minimal ABS media, the growth of the complemented strain was slightly slower compared to the wild-type but reached the same final OD600 after 11 h of incubation in rich medium (Supplementary Figure S1).
In order to evaluate the contribution of bceN to exopolysaccharide synthesis, mutant and complemented strain were grown on plates containing different carbon sources (glucose, galactose and the sugar alcohol mannitol) (Figure 2). In contrast to the wild-type and the complemented strain, the appearance of the bceN mutant was non-mucoid on all carbon sources tested, suggesting that BceN plays an important role in exopolysaccharide production. The amount of exopolysaccharide produced in the wild-type strain did not seem to vary depending on the carbon sources (Figure 2).
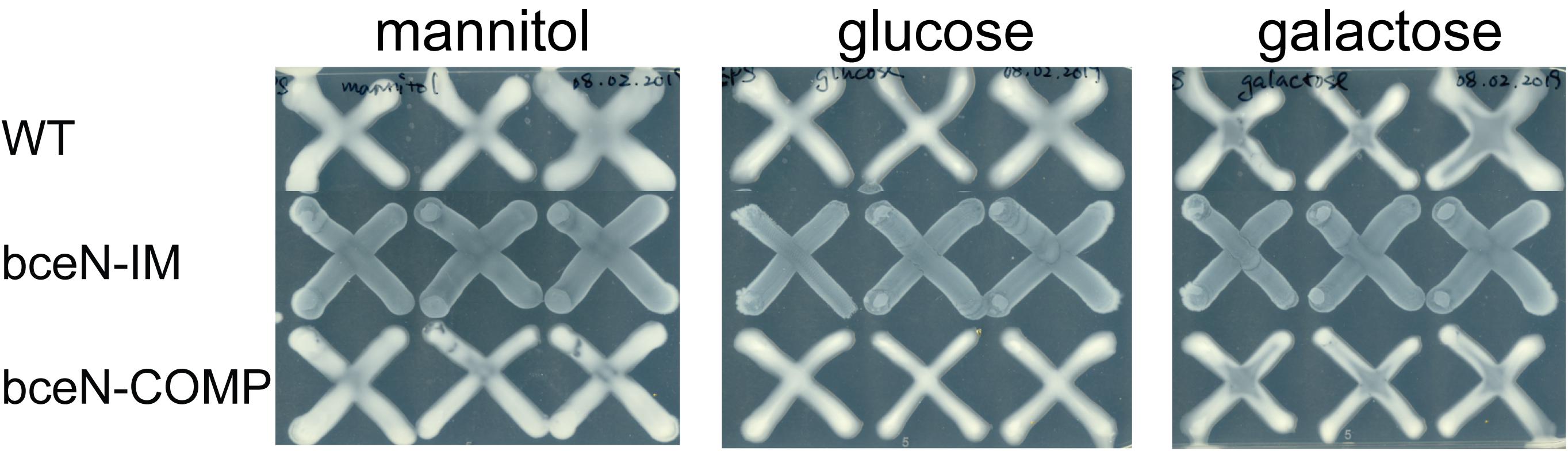
Figure 2. Exopolysaccharide production of P. phymatum wild-type (WT), bceN mutant (bceN-IM), and complemented (bceN-COMP) strains assayed on plates containing 0.06% yeast extract and 1% of the indicated carbon source. Three independent biological replicates were tested per strain. Plates were incubated at 30°C for 3 days.
Since exopolysaccharides are key components of the biofilm matrix in numerous bacteria, the capacity of four P. phymatum strains (wild-type, bceN-IM, bceN-COMP, and bceN-pBBR) to form biofilm was assessed in 96-well polystyrene plates using the crystal violet method. The cells were grown in minimal medium (A)BM with mannitol as carbon source under nitrogen limiting condition and incubated aerobically for 5 days at 30°C. Under this growth condition, all strains grew to a similar optical density (OD550). Although the P. phymatum wild-type strain produced only a relatively low amount of biofilm, a statistically significant difference was observed with the bceN mutant that produced approximately two-fold less biofilm as compared to the wild-type (Figure 3), suggesting an important contribution of this BceN-dependent exopolysaccharide in biofilm formation. However, in the complemented mutant strain (bceN-COMP), which harbors an intact bceN gene in a plasmid construct, biofilm production was only partially restored, while the mutant strain containing the empty vector (bceN-pBBR) formed even less biofilm as the mutant strain (Figure 3).
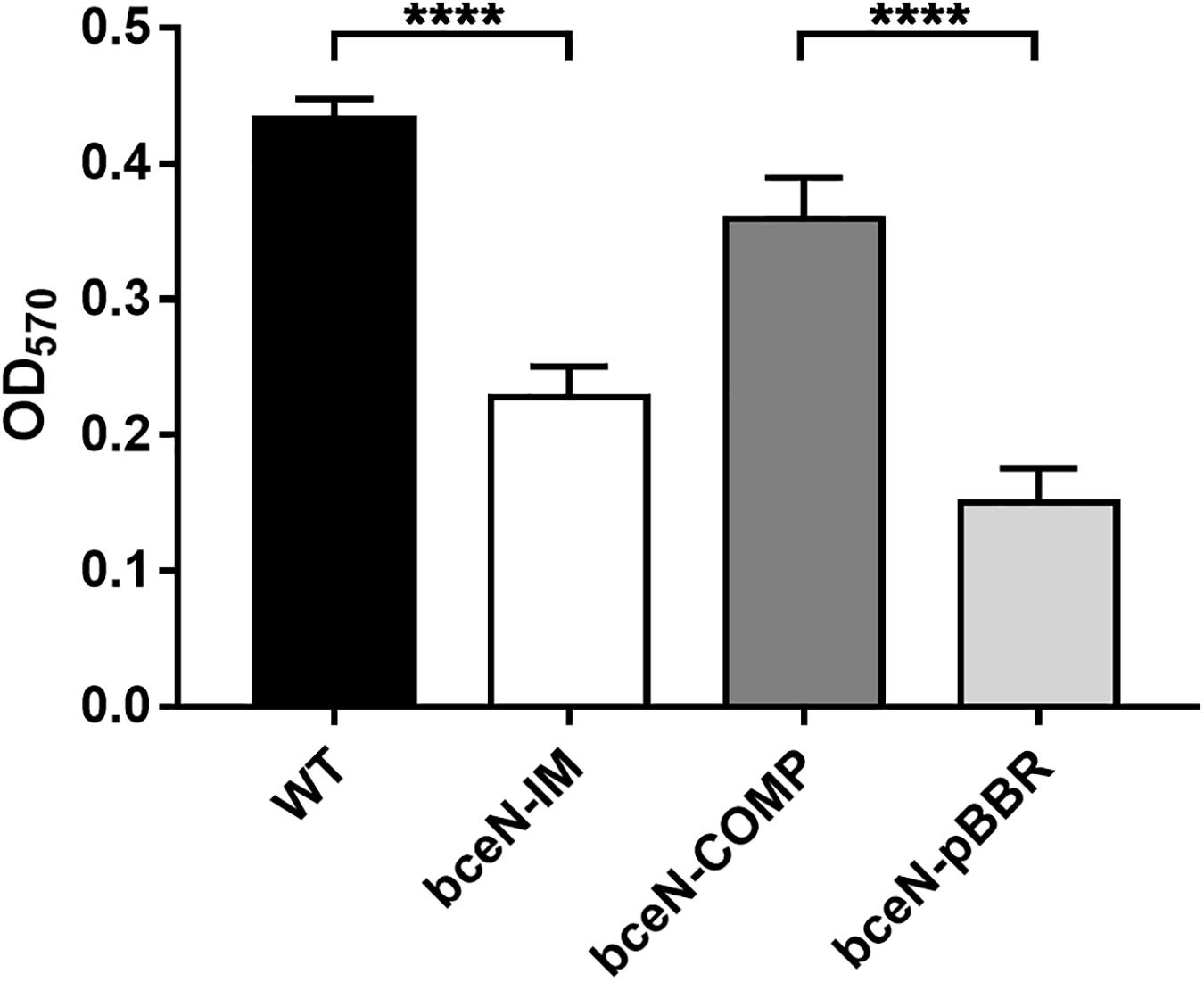
Figure 3. Biofilm production in P. phymatum wild-type and mutant strains. The cells were incubated in nitrogen limited (A)BM minimal medium at 30°C for 5 days before biofilm production (OD570) was quantified. Three independent biological replicates of each strain were assayed. Wild-type (WT) and the complemented (bceN-COMP) strains produced significantly more biofilm than the mutant (bceN-IM) and the mutant containing the empty vector (bceN-pBBR), respectively. Error bars indicate the standard error of the mean (SEM). ****p-value < 0.0001.
P. phymatum Produces the Polysaccharide Cepacian
A previous study from Ferreira et al. (2010) showed by Fourier transform infrared (FTIR) spectroscopy analysis that several Burkholderia strains, among these P. phymatum, produces a polysaccharide with structure similar to CEP’s produced by Bcc strains. In this study, we used NMR spectroscopy to analyze the polysaccharides synthetized by P. phymatum strain STM815 wild-type and mutant strains after 4 days of growth at 30°C on YE-mannitol plates (Cescutti et al., 2010). The polysaccharide was isolated and subjected to 1H NMR spectroscopy at 50°C. The 1H NMR spectrum of P. phymatum strain STM815 wild type (Supplementary Figure S2) showed very broad signals in the anomeric and ring regions of the spectrum, together with resonances attributable to methyl groups of acetyl substituents (at about 2.15 ppm) and 6-deoxy hexoses at 1.24 ppm. Integration of the areas under these peaks gave a ratio of 2.4 acetyl groups for each 6-deoxy hexose. The 1H NMR spectrum was highly similar to that of the polysaccharide CEP (Cescutti et al., 2000). With the aim of getting better resolved 1H NMR spectra, the polysaccharides produced by the three strains were sonicated, to decrease the viscosity of the solutions, de-acetylated and their spectra were recorded at 70°C (Figure 4). The 1H NMR spectrum of the polysaccharide produced by P. phymatum unambiguously identified it with CEP; assignments of resonances in the anomeric region and of the methyl group of rhamnose are reported in Figure 4 in agreement with published data (Cescutti et al., 2000). Comparison of the 1H NMR spectra of P. phymatum wild-type and P. phymatum bceN-COMP exopolysaccharide solutions (Figures 4A,B) showed their complete identity, thus demonstrating that also P. phymatum bceN-COMP strain produced CEP. Moreover, 1H NMR data of the P. phymatum wild type exopolysaccharide (Supplementary Figure S2) prior to deacetylation determined the presence of about 2.4 acetyl groups per repeating unit of CEP. The complemented strain also produced CEP with a comparable degree of acetylation (data not shown). On the contrary, although the 1H NMR spectrum of P. phymatum bceN-IM extract (Figure 4C) is compatible with that of a polysaccharide, it is completely different from the other two spectra, thus establishing that it does not produce CEP and nothing can be said about the structural identity of the polymer resorting only to this experimental data. Extremely low intensity signals belonging to the polymer produced by the bceN-IM strain are also present in the spectra of the other two samples, as indicated by stars in Figure 4. The data obtained suggested that CEP is the main polysaccharide produced by P. phymatum wild type in the experimental conditions used, while inactivation of the bceN gene led to lack of CEP biosynthesis, as expected.
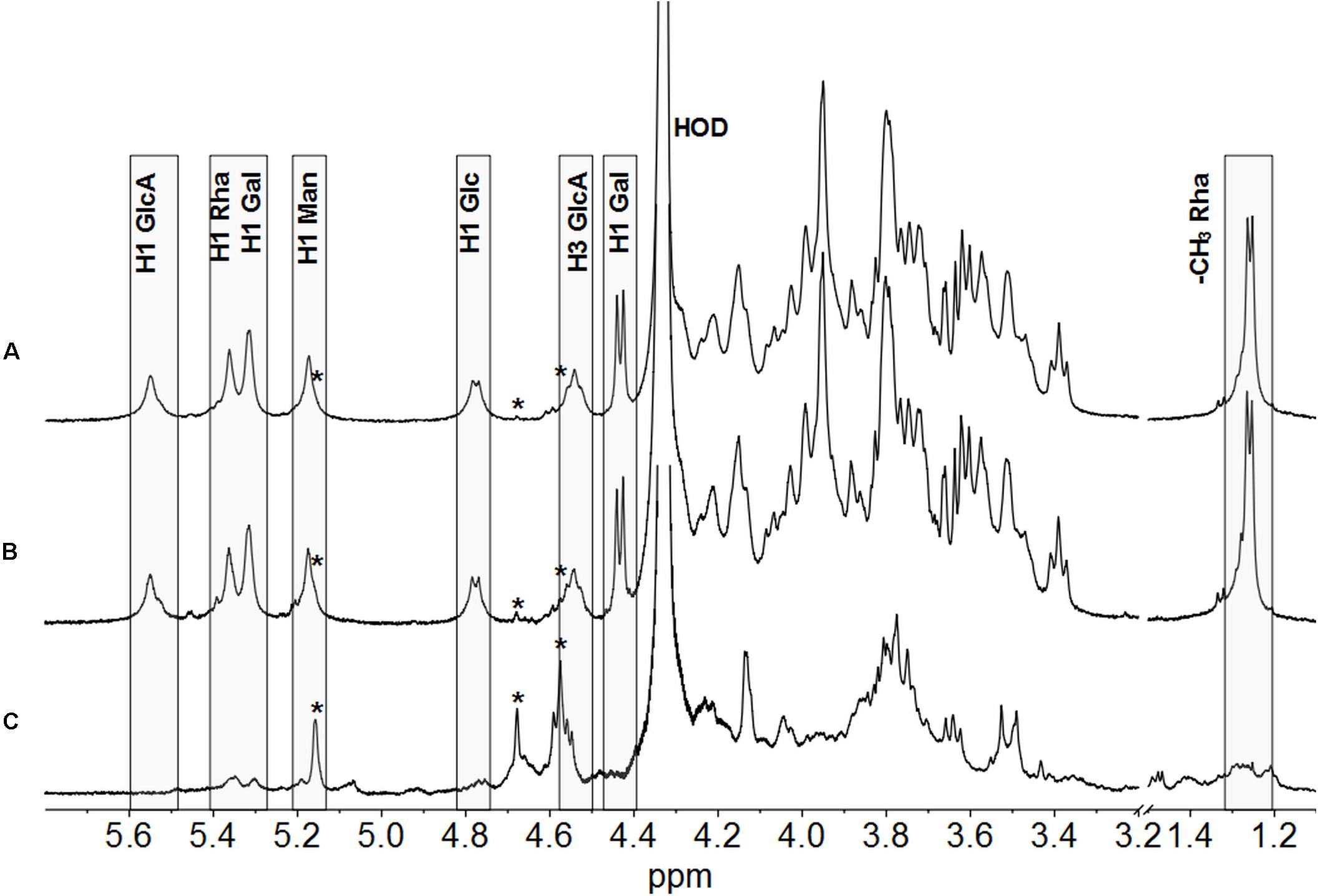
Figure 4. 1H NMR spectra of the polysaccharides produced by P. phymatum wild type (A), a P. phymatum bceN complemented mutant (B), and a P. phymatum bceN mutant (C). Spectra were recorded at 500 MHz and 70°C. Resonances attributed to the anomeric protons of CEP, residual water (HOD), H3 of glucuronic acid, and methyl group of rhamnose are reported. Boxes show diagnostic resonances of cepacian. H1, Anomeric proton; GlcA, Glucuronic acid; Rha, Rhamnose; Gal, Galactose; Man, Mannose; Glc, Glucose. Low intensity signals belonging to the polymer produced by the bceN mutant strain are indicated by stars.
Cepacian Facilitates Plant Root Attachment
To evaluate the potential role of CEP in the interaction of P. phymatum with plants, the symbiotic efficiency of the bceN mutant strain was tested using Phaseolus vulgaris var. Negro Jamapa as host plant. After 3 weeks of incubation in the green-house, several symbiosis-relevant parameters were assessed, i.e., nitrogenase activity, number and weight of nodules. While bean plants infected with the bceN mutant showed wild-type nitrogenase activity, the nodule number was reduced by around 30% (Figure 5). This observed reduction in nodule number could be partially complemented by introducing bceN on a plasmid. In contrast, the dry weight per nodule was similar in plants infected with mutant and wild type. However, nodules occupied with the complemented strain were lighter and displayed a 30% reduced nitrogenase activity as compared to wild-type nodules. This negative effect of the complemented strain in nitrogenase activity and nodules dry weight could be due to a possible overexpression of bceN from the plasmid. Additionally, a root attachment assay showed that after 4 h of incubation, the bceN-IM mutant as well as the empty vector control (bceN-pBBR) were impaired in attaching to germinated P. vulgaris roots relative to the wild type and the complemented strain (Figure 6). These results suggested a potential role of CEP in root attachment, the first step of root infection.
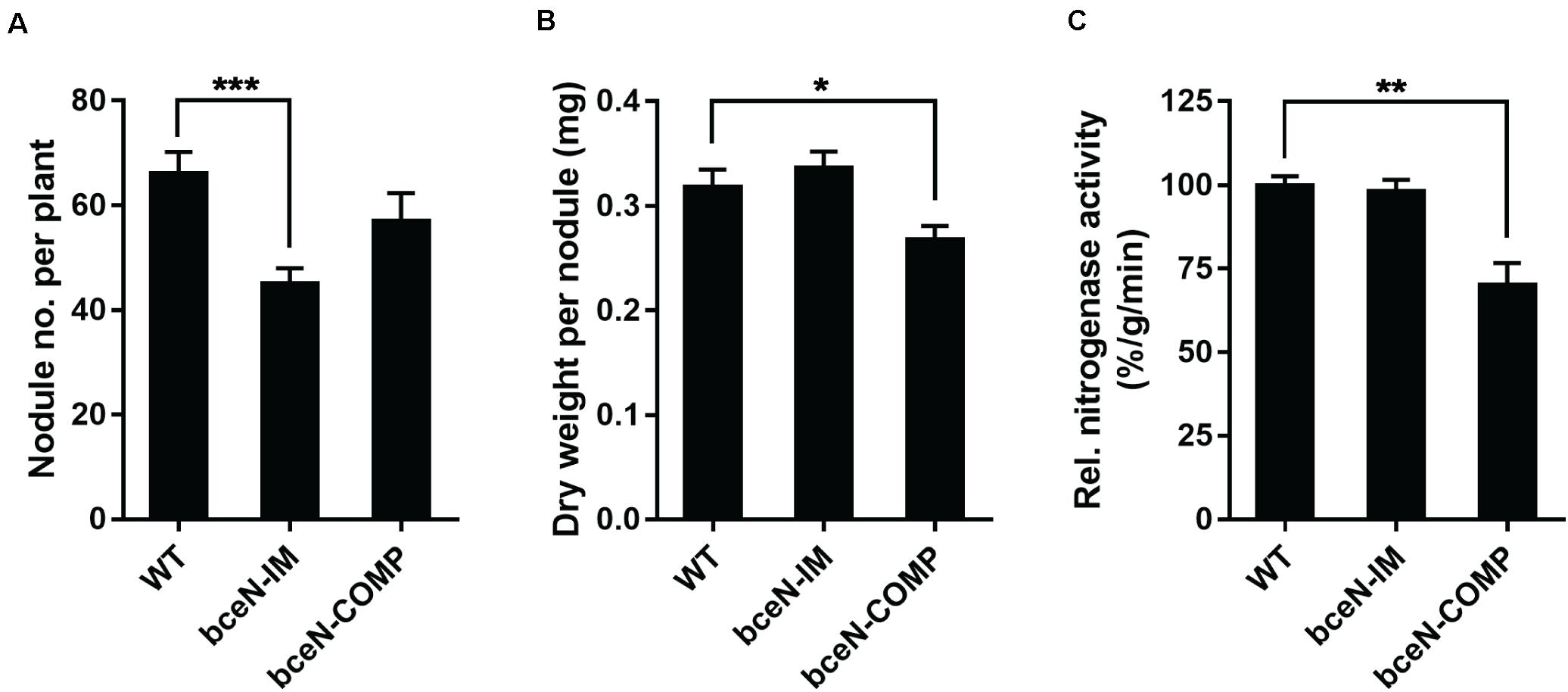
Figure 5. The symbiotic properties of P. vulgaris plants inoculated with P. phymatum wild-type (WT), bceN mutant (bceN-IM), and the complemented (bceN-COMP) strains. Number of nodules per plant (A), dry weight per nodule (B), and relative nitrogenase activity (C) were analyzed 21 dpi. The results are a combination of at least two independent experiments with two biological replicates of each strain, n = 16. Error bars indicate the standard error of the mean (SEM). ***p = 0.0007; *p = 0.0321; **p = 0.006.
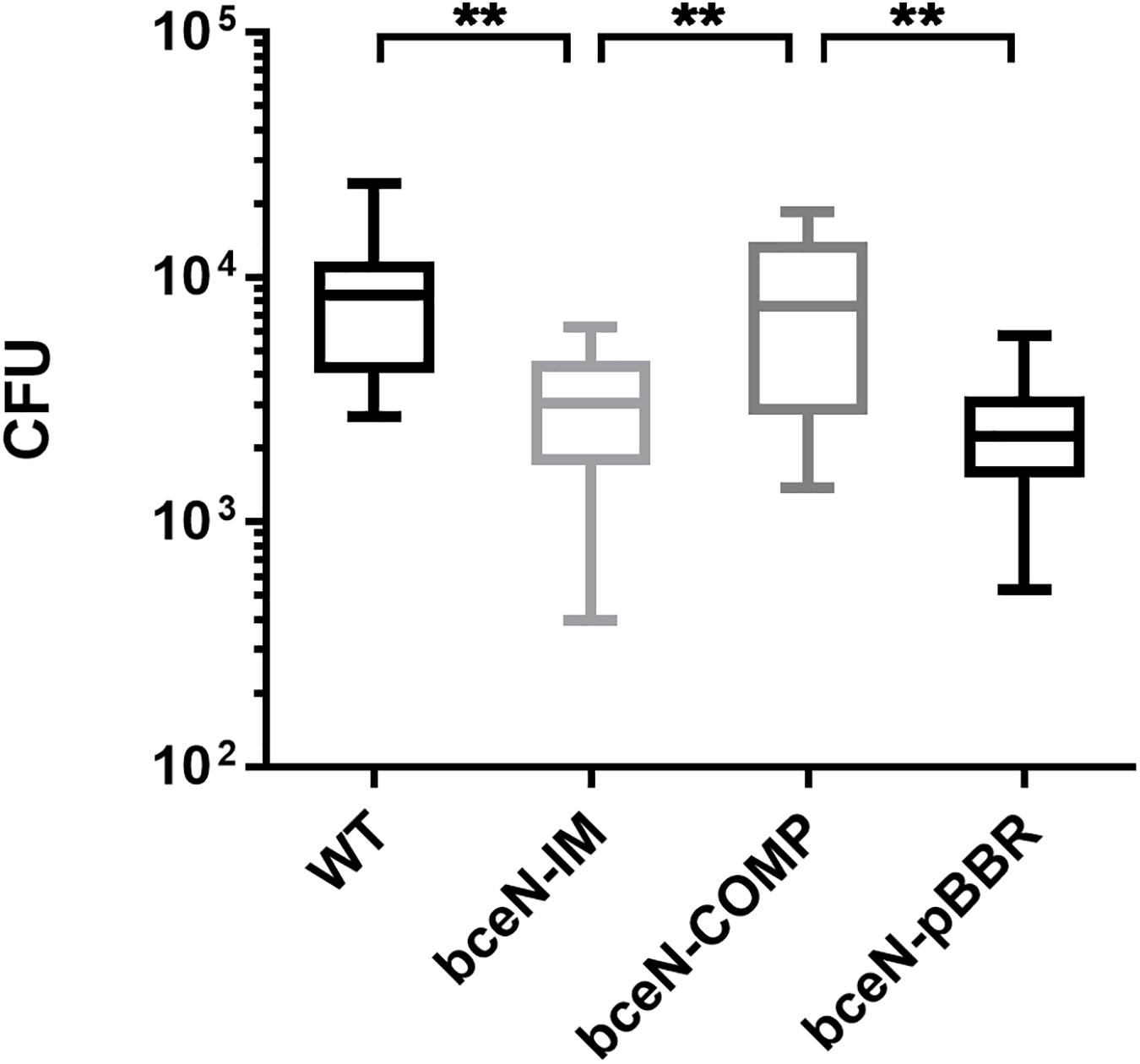
Figure 6. The P. phymatum bceN mutant is less efficient than the wild-type and the complemented strain in attaching to bean roots. Numbers of bacterial cells attached to bean roots after 4-h incubation followed by two stringent washing steps to remove loosely attached cells. Shown are five independent experiments with one biological replicate of each strain, n = 13. CFU, colony forming units. Error bars indicate the standard error of the mean (SEM). WT vs bceN-IM, **p = 0.0024; WT vs bceN-COMP, p = 0.8473; WT vs bceN-pBBR, **p = 0.0020; bceN-IM vs bceN-COMP, **p = 0.0040; bceN-COMP vs bceN-pBBR, **p = 0.0032.
Expression of the bce Cluster Is Induced Under Nitrogen Limitation and by Roots of Germinated Seeds
Polysaccharide biosynthesis is a multi-step and complex process influenced by various environmental conditions such as the presence and amount of different nutrients (carbon, nitrogen, oxygen, and phosphate) (Serrato et al., 2006). Since there is a clear correlation between functional bceN and CEP production, we constructed a reporter strain where the promotor of the operon containing bceN was placed in front of the gene encoding the green fluorescent protein (gfp). GFP expression indicating bceN transcriptional activity was investigated under different conditions including nitrogen limitation, the presence of root exudates obtained from P. vulgaris and the presence of germinated seeds (Figure 7 and Supplementary Figure S3). Interestingly, both the presence of root exudates and nitrogen limiting conditions increased GFP expression by a factor of roughly two after 48 h incubation (Supplementary Figure S3). A maximal four-fold induction of expression was reached when the reporter construct was grown 48 h in nitrogen limiting conditions and in presence of root exudates (Supplementary Figure S3). When we incubated the bce reporter construct on a soft agar plate under nitrogen limiting conditions and in presence of a germinated bean seed, a clear induction of bce expression was observed (Figure 7). This result suggested that in addition to nitrogen limitation an inducing signal is present in the root exudates and/or on the root surface.
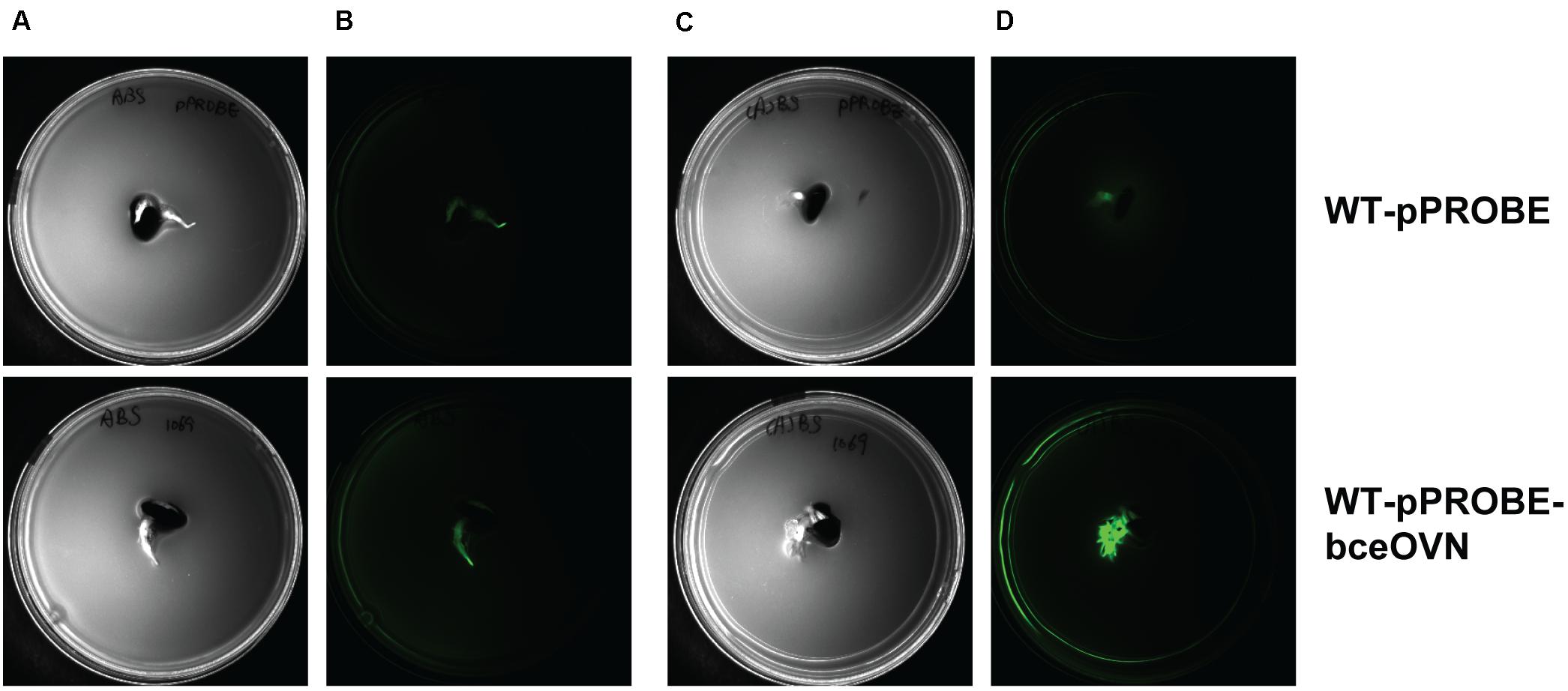
Figure 7. Expression of bceN is induced by the presence of a root of a germinated seed under nitrogen starvation. Images are bottom-up view of soft agar plates in the bright field (A,C) or under a GFP filter with 500 ms exposure (B,D). Bacteria carrying either pPROBE vector without promoter (WT-pPROBE) or GFP driven by the promoter of bceOVN operon (WT-pPROBE-bceOVN) were grown in either ABS minimal medium (A,B) or (A)BS medium with limited nitrogen source (C,D) co-incubated with a root of a germinated seed for 48 h at 30°C. GFP was induced in WT-pPROBE-bceOVN cells located in the proximity of the bean root in nitrogen-limited medium.
Discussion
Rhizobial surface polysaccharides represent the interface between bacteria and roots in the soil and are therefore important traits for the establishment of a successful rhizobial symbiosis (Niehaus and Becker, 1998; Fraysse et al., 2003). Rhizobial polysaccharides are chemically diverse and species- and strain-specific. Several fast-growing rhizobia such as S. meliloti and R. leguminosarum synthetize exopolysaccharides containing octasaccharide repeating units of mainly glucose. The well-studied S. meliloti produces two types of exopolysaccharides, succinoglycan (exo/exs gene cluster), and galactoglucan (wge/wga gene cluster) (Gonzalez et al., 1996; Cheng and Walker, 1998; Pellock et al., 2000; Arnold et al., 2018).
In the recently discovered beta-rhizobia, nothing is known about the identity and the role of polysaccharides for persistence in soil and for symbiosis with legumes. Inspection of the complete genome of our model strain P. phymatum STM815 (Moulin et al., 2014) suggested the presence of nine potential polysaccharide biosynthetic gene clusters. However, none of these nine clusters displayed sequence similarity to the classical exo/exs and wge/wga clusters found in alpha-rhizobia, suggesting that beta-rhizobia use another exopolysaccharide to form biofilms on the plant root surface. In silico analysis of P. phymatum gene cluster (Bphy_1056–Bphy_1077) suggested that it is involved in the biosynthesis of CEP, a branched acetylated heptasaccharide also produced by many strains of the Burkholderia sensu latu, which are opportunistic pathogens (Conway et al., 2004; Cescutti et al., 2010; Ferreira et al., 2010; Hallack et al., 2010; Cuzzi et al., 2012). The role of CEP and particularly the associated switch to mucoid colonies in Burkholderia virulence is controversially discussed in the literature (Govan et al., 1993; Conway et al., 2004; Zlosnik et al., 2008, 2011; Silva et al., 2018). Recent data suggest that CEP is important for chronic infections and appears to be rather counter-productive for acute virulent infections (Silva et al., 2018). The fact that the B. cenocepacia ET12 epidemic CF isolates J2315, K56-2, and BC7 have lost their ability to produce CEP due to an 11 bp deletion in bceB (Moreira et al., 2003; Bartholdson et al., 2008) also suggests that CEP may not be required for acute infections caused by pathogenic Burkholderia strains.
In this study, we show for the first time a functional role of CEP for bacterial colonization of plants, which are the natural host not only for Paraburkholderia but also for several pathogenic Burkholderia strains (Coenye and Vandamme, 2003; Eberl and Vandamme, 2016). A P. phymatum bceN mutant did not produce CEP and was affected in biofilm formation. Moreover, when inoculated on common bean, the mutant led to the formation of a reduced number of root nodules (roughly 30% lower) and was affected in root attachment, suggesting that CEP increases the efficiency of P. phymatum to interact with its host plant. In contrast to the situation in Bcc strains and other Burkholderia sensu stricto, where the bce-I and bce-II clusters are separated by hundreds of kilobase pairs (Ferreira et al., 2010), the bce genes in P. phymatum STM815 are organized in one continuous gene cluster. Like in P. phymatum, other plant associated Paraburkholderia and Caballeronia strains previously belonging to the Burkholderia genus (P. graminis C4D1M, P. xenovorans LB400, P. phytofirmans PsJN, P. hospita DSM 17164, P. sprentiae WSM5005, several P. caribensis strains, and C. glathei DSM 50014) also all harbor the bce genes in one single genomic locus (Ferreira et al., 2010) (Supplementary Figure S4).
A complex regulatory network controls CEP biosynthesis in response to changing levels of nutrients such as nitrogen, carbon and phosphate. We previously showed that in P. phymatum STM815 as well as in the opportunistic pathogen B. cenocepacia H111, the expression of the bce genes is significantly up-regulated in nitrogen limiting conditions (Lardi et al., 2015, 2017a). Moreover, in H111 bce expressions depends on the alternative sigma factor σ54 (RpoN) and on its activator NtrC (Lardi et al., 2015; Liu et al., 2017). In H111, a σ54 consensus sequence has been found in the bce-I and bce-II promoter regions suggesting that σ54 directly regulates bce expression. Cell-density dependent quorum sensing (QS) systems, which use LuxR-type transcriptional regulators and homoserine lactone signals (AHLs), were reported to regulate exopolysaccharide synthesis in different bacteria including S. meliloti (Gurich and Gonzalez, 2009). However, Coutinho et al. (2013) reported that in P. phymatum the BraRI AHL-dependent QS system represses expression of the bce-I cluster. Another important regulator of CEP biosynthesis is the response regulator OmpR. In the Bcc strain B. multivorans, OmpR was shown to be important for the mucoid to non-mucoid switch (Silva et al., 2018). Interestingly, several Bcc non-mucoid isolates produce large amounts of the polysaccharide CEP when tissue of the natural host plant onion was provided as nutrient source (Bartholdson et al., 2008). The plant compounds responsible for this induction of CEP biosynthesis resulted to be primarily sugar alcohols such as mannitol. Although we did not observe an activation of polysaccharide synthesis by mannitol in P. phymatum (Figure 2), the fact that plant metabolites induced CEP synthesis in Bcc strains, which also associate with plants, suggests that CEP may be primarily used for plant interaction rather than being a virulence factor in the pathogenic Burkholderia lineage.
In this study, we used a promoter fusion to elucidate activation of bceN expression under different growth conditions that mimic the natural soil environment of these bacteria (nitrogen limitation and presence of root exudates). We could observe a maximal activation of expression (four-fold after 48 h of incubation) when the cells were nitrogen-limited and incubated in presence of root exudates as well as on agar plates in presence of common bean roots suggesting that P. phymatum induces CEP synthesis in the often nitrogen limited rhizosphere. We speculate that the roots or its exudates present a signal, which induces bce expression. Interestingly, in the plant-growth promoting strain Bacillus subtilis, biofilm formation, an important feature for root colonization, was triggered by the plant polysaccharides arabinogalactan, pectin, and xylan. In addition, these plant polysaccharides were shown to be used as substrates for the synthesis of matrix polysaccharides of B. subtilis biofilms (Beauregard et al., 2013). Further studies are required to identify the signal(s) in the root exudates that induce CEP production. Whether CEP contributes to P. phymatum’s success in competitively colonizing different host plants and whether this represents a general feature for all plant-associated Burkholderia strains remain important open questions.
Data Availability Statement
The raw data supporting the conclusions of this article will be made available by the authors, without undue reservation.
Author Contributions
YL, PC, and GP conceived and designed the experiments. YL, SH, BB, and PC performed the experiments. YL, SH, LE, PC, and GP analyzed the data. YL, LE, PC, and GP wrote the manuscript. All authors contributed to the article and approved the submitted version.
Funding
This work was supported by the Swiss National Science Foundation (Grant 31003A_179322 to GP).
Conflict of Interest
The authors declare that the research was conducted in the absence of any commercial or financial relationships that could be construed as a potential conflict of interest.
Acknowledgments
We thank Prof. Eulogio Bedmar (ZAIDIN, Granada) for kindly providing the P. vulgaris Negro Jamapa seeds. We acknowledge Hans-Martin Fischer (ETH Zürich) for providing SILWET, Karl Huwiler for help in the green-house, and Lawrence Och for graphic assistance.
Supplementary Material
The Supplementary Material for this article can be found online at: https://www.frontiersin.org/articles/10.3389/fmicb.2020.01600/full#supplementary-material
FIGURE S1 | Aerobic growth of P. phymatum WT, bceN-IM, and bceN-COMP in liquid LB-NaCl (A) and ABS (B) media. Data of three biological replicates of each strain were combined.
FIGURE S2 | 1H NMR spectrum of the polysaccharide produced by P. phymatum recorded at 500 MHz and 50°C. Main assignments are reported. The structure of CEP repeating unit is reported.
FIGURE S3 | Expression of bceN is induced under nitrogen limiting growth conditions and in presence of root exudates after 24 and 48 h incubation. P. phymatum promoter reporter (WT-pPROBE-bceOVN) cells were incubated in four different minimal media: ABS, AB minimal medium with succinate as carbon source; (A)BS, ABS with limited nitrogen source; ABS + RE, ABS with root exudates; (A)BS + RE, (A)BS with root exudates and under nitrogen limitation. The GFP expression was normalized by cell density OD600. Statistical analysis was carried out on (A)BS + RE sample group in comparison with other conditions to show statistically significant induction of GFP expression in this group. Error bars indicate the standard error of the mean (SEM). ****p < 0.0001, ∗∗∗p < 0.001, and ∗∗p < 0.01.
FIGURE S4 | Organization of bce-I and bce-II clusters in selected Paraburkholderia and Burkholderia sensu stricto strains (figure modified from Eberl and Vandamme, 2016, phylogenetic tree based on 16S rRNA).
TABLE S1 | Bacterial strains, plasmids, and oligonucleotides used in this study.
References
Arnold, M. F. F., Penterman, J., Shabab, M., Chen, E. J., and Walker, G. C. (2018). Important late-stage symbiotic role of the Sinorhizobium meliloti exopolysaccharide succinoglycan. J. Bacteriol. 200:e00665-17.
Bartholdson, S. J., Brown, A. R., Mewburn, B. R., Clarke, D. J., Fry, S. C., Campopiano, D. J., et al. (2008). Plant host and sugar alcohol induced exopolysaccharide biosynthesis in the Burkholderia cepacia complex. Microbiology 154(Pt 8), 2513–2521. doi: 10.1099/mic.0.2008/019216-0
Battisti, L., Lara, J. C., and Leigh, J. A. (1992). Specific oligosaccharide form of the Rhizobium meliloti exopolysaccharide promotes nodule invasion in alfalfa. Proc. Natl. Acad. Sci. U.S.A. 89, 5625–5629. doi: 10.1073/pnas.89.12.5625
Beauregard, P. B., Chai, Y., Vlamakis, H., Losick, R., and Kolter, R. (2013). Bacillus subtilis biofilm induction by plant polysaccharides. Proc. Natl. Acad. Sci. U.S.A. 110, E1621–E1630. doi: 10.1073/pnas.1218984110
Benincasa, M., Mattiuzzo, M., Herasimenka, Y., Cescutti, P., Rizzo, R., and Gennaro, R. (2009). Activity of antimicrobial peptides in the presence of polysaccharides produced by pulmonary pathogens. J. Pept. Sci. 15, 595–600. doi: 10.1002/psc.1142
Beukes, C. W., Palmer, M., Manyaka, P., Chan, W. Y., Avontuur, J. R., van Zyl, E., et al. (2017). Genome data provides high support for generic boundaries in Burkholderia sensu lato. Front. Microbiol. 8:1154. doi: 10.3389/fmicb.2017.01154
Bontemps, C., Elliott, G. N., Simon, M. F., Dos Reis, F. B. D., Gross, E., Lawton, R. C., et al. (2010). Burkholderia species are ancient symbionts of legumes. Mol. Ecol. 19, 44–52. doi: 10.1111/j.1365-294X.2009.04458.x
Bournaud, C., de Faria, S. M., dos Santos, J. M., Tisseyre, P., Silva, M., Chaintreuil, C., et al. (2013). Burkholderia species are the most common and preferred nodulating symbionts of the Piptadenia group (tribe Mimoseae). PLoS One 8:e63478. doi: 10.1371/journal.pone.0063478
Breedveld, M. W., and Miller, K. J. (1994). Cyclic beta-glucans of members of the family Rhizobiaceae. Microbiol. Rev. 58, 145–161. doi: 10.1128/mmbr.58.2.145-161.1994
Bulgarelli, D., Rott, M., Schlaeppi, K., Ver Loren van Themaat, E., Ahmadinejad, N., Assenza, F., et al. (2012). Revealing structure and assembly cues for Arabidopsis root-inhabiting bacterial microbiota. Nature 488, 91–95. doi: 10.1038/nature11336
Bylund, J., Burgess, L. A., Cescutti, P., Ernst, R. K., and Speert, D. P. (2006). Exopolysaccharides from Burkholderia cenocepacia inhibit neutrophil chemotaxis and scavenge reactive oxygen species. J. Biol. Chem. 281, 2526–2532. doi: 10.1074/jbc.m510692200
Cerantola, S., Lemassu-Jacquier, A., and Montrozier, H. (1999). Structural elucidation of a novel exopolysaccharide produced by a mucoid clinical isolate of Burkholderia cepacia. Characterization of a trisubstituted glucuronic acid residue in a heptasaccharide repeating unit. Eur. J. Biochem. 260, 373–383. doi: 10.1046/j.1432-1327.1999.00171.x
Cescutti, P., Bosco, M., Picotti, F., Impallomeni, G., Leitão, J. H., Richau, J. A., et al. (2000). Structural study of the exopolysaccharide produced by a clinical isolate of Burkholderia cepacia. Biochem. Biophys. Res. Commun. 273, 1088–1094. doi: 10.1006/bbrc.2000.3059
Cescutti, P., Foschiatti, M., Furlanis, L., Lagatolla, C., and Rizzo, R. (2010). Isolation and characterisation of the biological repeating unit of cepacian, the exopolysaccharide produced by bacteria of the Burkholderia cepacia complex. Carbohydr. Res. 345, 1455–1460. doi: 10.1016/j.carres.2010.03.029
Chen, W. M., de Faria, S. M., Straliotto, R., Pitard, R. M., Simoes-Araujo, J. L., Chou, J. H., et al. (2005). Proof that Burkholderia strains form effective symbioses with legumes: a study of novel Mimosa-nodulating strains from South America. Appl. Environ. Microbiol. 71, 7461–7471. doi: 10.1128/aem.71.11.7461-7471.2005
Chen, W. M., Laevens, S., Lee, T. M., Coenye, T., De Vos, P., Mergeay, M., et al. (2001). Ralstonia taiwanensis sp. nov., isolated from root nodules of Mimosa species and sputum of a cystic fibrosis patient. Int. J. Syst. Evol. Microbiol. 51(Pt 5), 1729–1735. doi: 10.1099/00207713-51-5-1729
Chen, W. M., Moulin, L., Bontemps, C., Vandamme, P., Bena, G., and Boivin-Masson, C. (2003). Legume symbiotic nitrogen fixation by beta-proteobacteria is widespread in nature. J. Bacteriol. 185, 7266–7272. doi: 10.1128/jb.185.24.7266-7272.2003
Cheng, H. P., and Walker, G. C. (1998). Succinoglycan is required for initiation and elongation of infection threads during nodulation of alfalfa by Rhizobium meliloti. J. Bacteriol. 180, 5183–5191. doi: 10.1128/jb.180.19.5183-5191.1998
Coenye, T., and Vandamme, P. (2003). Diversity and significance of Burkholderia species occupying diverse ecological niches. Environ. Microbiol. 5, 719–729. doi: 10.1046/j.1462-2920.2003.00471.x
Conway, B. A., Chu, K. K., Bylund, J., Altman, E., and Speert, D. P. (2004). Production of exopolysaccharide by Burkholderia cenocepacia results in altered cell-surface interactions and altered bacterial clearance in mice. J. Infect Dis. 190, 957–966. doi: 10.1086/423141
Coutinho, B. G., Mitter, B., Talbi, C., Sessitsch, A., Bedmar, E. J., Halliday, N., et al. (2013). Regulon studies and in planta role of the BraI/R quorum-sensing system in the plant-beneficial Burkholderia cluster. Appl. Environ. Microbiol. 79, 4421–4432. doi: 10.1128/AEM.00635-13
Cunha, M. V., Sousa, S. A., Leitão, J. H., Moreira, L. M., Videira, P. A., and Sá-Correia, I. (2004). Studies on the involvement of the exopolysaccharide produced by cystic fibrosis-associated isolates of the Burkholderia cepacia complex in biofilm formation and in persistence of respiratory infections. J. Clin. Microbiol. 42, 3052–3058. doi: 10.1128/JCM.42.7.3052-3058.2004
Cuzzi, B., Cescutti, P., Furlanis, L., Lagatolla, C., Sturiale, L., Garozzo, D., et al. (2012). Investigation of bacterial resistance to the immune system response: cepacian depolymerisation by reactive oxygen species. Innate Immun. 18, 661–671. doi: 10.1177/1753425911435954
Cuzzi, B., Herasimenka, Y., Silipo, A., Lanzetta, R., Liut, G., Rizzo, R., et al. (2014). Versatility of the Burkholderia cepacia complex for the biosynthesis of exopolysaccharides: a comparative structural investigation. PLoS One 9:e94372. doi: 10.1371/journal.pone.0094372
Dalmastri, C., Baldwin, A., Tabacchioni, S., Bevivino, A., Mahenthiralingam, E., Chiarini, L., et al. (2007). Investigating Burkholderia cepacia complex populations recovered from Italian maize rhizosphere by multilocus sequence typing. Environ. Microbiol. 9, 1632–1639. doi: 10.1111/j.1462-2920.2007.01273.x
Djordjevic, S. P., Chen, H., Batley, M., Redmond, J. W., and Rolfe, B. G. (1987). Nitrogen fixation ability of exopolysaccharide synthesis mutants of Rhizobium sp. strain NGR234 and Rhizobium trifolii is restored by the addition of homologous exopolysaccharides. J. Bacteriol. 169, 53–60. doi: 10.1128/jb.169.1.53-60.1987
dos Reis, F. B. Jr., Simon, M. F., Gross, E., Boddey, R. M., Elliott, G. N., Neto, N. E., et al. (2010). Nodulation and nitrogen fixation by Mimosa spp. in the Cerrado and Caatinga biomes of Brazil. New Phytol. 186, 934–946. doi: 10.1111/j.1469-8137.2010.03267
Downie, J. A. (2010). The roles of extracellular proteins, polysaccharides and signals in the interactions of rhizobia with legume roots. FEMS Microbiol. Rev. 34, 150–170. doi: 10.1111/j.1574-6976.2009.00205.x
Eberl, L., and Vandamme, P. (2016). Members of the genus Burkholderia: good and bad guys. F1000Res 5:F1000FacultyRev-1007. doi: 10.12688/f1000research.8221.1
Elliott, G. N., Chen, W. M., Chou, J. H., Wang, H. C., Sheu, S. Y., Perin, L., et al. (2007). Burkholderia phymatum is a highly effective nitrogen-fixing symbiont of Mimosa spp. and fixes nitrogen ex planta. New Phytol. 173, 168–180. doi: 10.1111/j.1469-8137.2006.01894.x
Elliott, G. N., Chou, J. H., Chen, W. M., Bloemberg, G. V., Bontemps, C., Martinez-Romero, E., et al. (2009). Burkholderia spp. are the most competitive symbionts of Mimosa, particularly under N-limited conditions. Environ. Microbiol. 11, 762–778. doi: 10.1111/j.1462-2920.2008.01799.x
Ferreira, A. S., Leitão, J. H., Silva, I. N., Pinheiro, P. F., Sousa, S. A., Ramos, C. G., et al. (2010). Distribution of cepacian biosynthesis genes among environmental and clinical Burkholderia strains and role of cepacian exopolysaccharide in resistance to stress conditions. Appl. Environ. Microbiol. 76, 441–450. doi: 10.1128/AEM.01828-09
Ferreira, A. S., Leitão, J. H., Sousa, S. A., Cosme, A. M., Sá-Correia, I., and Moreira, L. M. (2007). Functional analysis of Burkholderia cepacia genes bceD and bceF, encoding a phosphotyrosine phosphatase and a tyrosine autokinase, respectively: role in exopolysaccharide biosynthesis and biofilm formation. Appl. Environ. Microbiol. 73, 524–534. doi: 10.1128/aem.01450-06
Ferreira, A. S., Silva, I. N., Oliveira, V. H., Cunha, R., and Moreira, L. M. (2011). Insights into the role of extracellular polysaccharides in Burkholderia adaptation to different environments. Front. Cell. Infect. Microbiol. 1:16. doi: 10.3389/fcimb.2011.00016
Ferreira, M. R., Gomes, S. C., and Moreira, L. M. (2019). Mucoid switch in Burkholderia cepacia complex bacteria: triggers, molecular mechanisms and implications in pathogenesis. Adv. Appl. Microbiol. 107, 113–140. doi: 10.1016/bs.aambs.2019.03.001
Fraysse, N., Couderc, F., and Poinsot, V. (2003). Surface polysaccharide involvement in establishing the rhizobium-legume symbiosis. Eur. J. Biochem. 270, 1365–1380. doi: 10.1046/j.1432-1033.2003.03492.x
Geddes, B. A., Gonzalez, J. E., and Oresnik, I. J. (2014). Exopolysaccharide production in response to medium acidification is correlated with an increase in competition for nodule occupancy. Mol. Plant Microbe Interact. 27, 1307–1317. doi: 10.1094/MPMI-06-14-0168-R
Gonzalez, J. E., York, G. M., and Walker, G. C. (1996). Rhizobium meliloti exopolysaccharides: synthesis and symbiotic function. Gene 179, 141–146. doi: 10.1016/s0378-1119(96)00322-8
Göttfert, M., Hitz, S., and Hennecke, H. (1990). Identification of nodS and nodU, two inducible genes inserted between the Bradyrhizobium japonicum nodYABC and nodIJ genes. Mol. Plant Microbe Interact. 3, 308–316.
Govan, J. R., Brown, P. H., Maddison, J., Doherty, C. J., Nelson, J. W., Dodd, M., et al. (1993). Evidence for transmission of Pseudomonas cepacia by social contact in cystic fibrosis. Lancet 342, 15–19. doi: 10.1016/0140-6736(93)91881-l
Gurich, N., and Gonzalez, J. E. (2009). Role of quorum sensing in Sinorhizobium meliloti-Alfalfa symbiosis. J. Bacteriol. 191, 4372–4382. doi: 10.1128/JB.00376-09
Hahn, M., and Hennecke, H. (1984). Localized mutagenesis in Rhizobium japonicum. Mol. Gen. Genet. 193, 46–52. doi: 10.1007/Bf00327412
Hallack, L. F., Passos, D. S., Mattos, K. A., Agrellos, O. A., Jones, C., Mendonça-Previato, L., et al. (2010). Structural elucidation of the repeat unit in highly branched acidic exopolysaccharides produced by nitrogen fixing Burkholderia. Glycobiology 20, 338–347. doi: 10.1093/glycob/cwp181
Huber, B., Riedel, K., Hentzer, M., Heydorn, A., Götschlich, A., Givskov, M., et al. (2001). The cep quorum-sensing system of Burkholderia cepacia H111 controls biofilm formation and swarming motility. Microbiology 147(Pt 9), 2517–2528. doi: 10.1099/00221287-147-9-2517
Jones, K. M., Kobayashi, H., Davies, B. W., Taga, M. E., and Walker, G. C. (2007). How rhizobial symbionts invade plants: the Sinorhizobium-Medicago model. Nat. Rev. Microbiol. 5, 619–633. doi: 10.1038/nrmicro1705
Kawaharada, Y., Kelly, S., Nielsen, M. W., Hjuler, C. T., Gysel, K., Muszynski, A., et al. (2015). Receptor-mediated exopolysaccharide perception controls bacterial infection. Nature 523, 308–312. doi: 10.1038/nature14611
Lardi, M., Aguilar, C., Pedrioli, A., Omasits, U., Suppiger, A., Carcamo-Oyarce, G., et al. (2015). σ54-dependent response to nitrogen limitation and virulence in Burkholderia cenocepacia strain H111. Appl. Environ. Microbiol. 81, 4077–4089. doi: 10.1128/AEM.00694-15
Lardi, M., de Campos, S. B., Purtschert, G., Eberl, L., and Pessi, G. (2017a). Competition experiments for legume infection identify Burkholderia phymatum as a highly competitive beta-Rhizobium. Front. Microbiol. 8:1527. doi: 10.3389/fmicb.2017.01527
Lardi, M., Liu, Y., Purtschert, G., Bolzan de Campos, S., and Pessi, G. (2017b). Transcriptome analysis of Paraburkholderia phymatum under nitrogen starvation and during symbiosis with Phaseolus vulgaris. Genes (Basel) 8:E389.
Laus, M. C., Logman, T. J., Lamers, G. E., Van Brussel, A. A., Carlson, R. W., and Kijne, J. W. (2006). A novel polar surface polysaccharide from Rhizobium leguminosarum binds host plant lectin. Mol. Microbiol. 59, 1704–1713. doi: 10.1111/j.1365-2958.2006.05057.x
Lee, Y. A., and Chan, C. W. (2007). Molecular typing and presence of genetic markers among strains of Banana finger-tip rot pathogen, Burkholderia cenocepacia, in Taiwan. Phytopathology 97, 195–201. doi: 10.1094/PHYTO-97-2-0195
Leigh, J. A., Signer, E. R., and Walker, G. C. (1985). Exopolysaccharide-deficient mutants of Rhizobium meliloti that form ineffective nodules. Proc. Natl. Acad. Sci. U.S.A. 82, 6231–6235. doi: 10.1073/pnas.82.18.6231
Lemaire, B., Chimphango, S. B., Stirton, C., Rafudeen, S., Honnay, O., Smets, E., et al. (2016). Biogeographical patterns of legume-nodulating Burkholderia spp.: from African fynbos to continental scales. Appl. Environ. Microbiol. 82, 5099–5115. doi: 10.1128/AEM.00591-16
Lemaire, B., Dlodlo, O., Chimphango, S., Stirton, C., Schrire, B., Boatwright, J. S., et al. (2015). Symbiotic diversity, specificity and distribution of rhizobia in native legumes of the Core Cape Subregion (South Africa). FEMS Microbiol. Ecol. 91, 1–17. doi: 10.1093/femsec/fiu024
LiPuma, J. J., Spilker, T., Coenye, T., and Gonzalez, C. F. (2002). An epidemic Burkholderia cepacia complex strain identified in soil. Lancet 359, 2002–2003. doi: 10.1016/s0140-6736(02)08836-0
Liu, Y., Lardi, M., Pedrioli, A., Eberl, L., and Pessi, G. (2017). NtrC-dependent control of exopolysaccharide synthesis and motility in Burkholderia cenocepacia H111. PLoS One 12:e0180362. doi: 10.1371/journal.pone.0180362
Marczak, M., Mazur, A., Koper, P., Zebracki, K., and Skorupska, A. (2017). Synthesis of rhizobial exopolysaccharides and their importance for symbiosis with legume plants. Genes (Basel) 8:E360. doi: 10.3390/genes8120360
Maroti, G., Downie, J. A., and Kondorosi, E. (2015). Plant cysteine-rich peptides that inhibit pathogen growth and control rhizobial differentiation in legume nodules. Curr. Opin. Plant Biol. 26, 57–63. doi: 10.1016/j.pbi.2015.05.031
Masson-Boivin, C., Giraud, E., Perret, X., and Batut, J. (2009). Establishing nitrogen-fixing symbiosis with legumes: how many rhizobium recipes? Trends Microbiol. 17, 458–466. doi: 10.1016/j.tim.2009.07.004
Melkonian, R., Moulin, L., Bena, G., Tisseyre, P., Chaintreuil, C., Heulin, K., et al. (2014). The geographical patterns of symbiont diversity in the invasive legume Mimosa pudica can be explained by the competitiveness of its symbionts and by the host genotype. Environ. Microbiol. 16, 2099–2111. doi: 10.1111/1462-2920.12286
Miller, J. H. (1972). Experiments in Molecular Genetics. New York, NY: Cold Spring Harbor Laboratory.
Mishra, R. P., Tisseyre, P., Melkonian, R., Chaintreuil, C., Miche, L., Klonowska, A., et al. (2012). Genetic diversity of Mimosa pudica rhizobial symbionts in soils of French Guiana: investigating the origin and diversity of Burkholderia phymatum and other beta-rhizobia. FEMS Microbiol. Ecol. 79, 487–503. doi: 10.1111/j.1574-6941.2011.01235.x
Moreira, L. M., Videira, P. A., Sousa, S. A., Leitão, J. H., Cunha, M. V., and Sá-Correia, I. (2003). Identification and physical organization of the gene cluster involved in the biosynthesis of Burkholderia cepacia complex exopolysaccharide. Biochem. Biophys. Res. Commun. 312, 323–333. doi: 10.1016/j.bbrc.2003.10.118
Moulin, L., Klonowska, A., Caroline, B., Booth, K., Vriezen, J. A., Melkonian, R., et al. (2014). Complete Genome sequence of Burkholderia phymatum STM815(T), a broad host range and efficient nitrogen-fixing symbiont of Mimosa species. Stand Genomic Sci. 9, 763–774. doi: 10.4056/sigs.4861021
Moulin, L., Munive, A., Dreyfus, B., and Boivin-Masson, C. (2001). Nodulation of legumes by members of the beta-subclass of Proteobacteria. Nature 411, 948–950. doi: 10.1038/35082070
Niehaus, K., and Becker, A. (1998). The role of microbial surface polysaccharides in the Rhizobium-legume interaction. Subcell Biochem. 29, 73–116. doi: 10.1007/978-1-4899-1707-2_3
Oldroyd, G. E., Murray, J. D., Poole, P. S., and Downie, J. A. (2011). The rules of engagement in the legume-rhizobial symbiosis. Annu. Rev. Genet. 45, 119–144. doi: 10.1146/annurev-genet-110410-132549
Pellock, B. J., Cheng, H. P., and Walker, G. C. (2000). Alfalfa root nodule invasion efficiency is dependent on Sinorhizobium meliloti polysaccharides. J. Bacteriol. 182, 4310–4318. doi: 10.1128/jb.182.15.4310-4318.2000
Ramette, A., LiPuma, J. J., and Tiedje, J. M. (2005). Species abundance and diversity of Burkholderia cepacia complex in the environment. Appl. Environ. Microbiol. 71, 1193–1201. doi: 10.1128/aem.71.3.1193-1201.2005
Santos, R., Herouart, D., Sigaud, S., Touati, D., and Puppo, A. (2001). Oxidative burst in alfalfa-Sinorhizobium meliloti symbiotic interaction. Mol. Plant Microbe Interact. 14, 86–89. doi: 10.1094/MPMI.2001.14.1.86
Sawana, A., Adeolu, M., and Gupta, R. S. (2014). Molecular signatures and phylogenomic analysis of the genus Burkholderia: proposal for division of this genus into the emended genus Burkholderia containing pathogenic organisms and a new genus Paraburkholderia gen. nov harboring environmental species. Front. Genet. 5:429. doi: 10.3389/fgene.2014.00429
Serrato, R. V., Sassaki, G. L., Cruz, L. M., Pedrosa, F. O., Gorin, P. A., and Iacomini, M. (2006). Culture conditions for the production of an acidic exopolysaccharide by the nitrogen-fixing bacterium Burkholderia tropica. Can. J. Microbiol. 52, 489–493. doi: 10.1139/w05-155
Silva, I. N., Pessoa, F. D., Ramires, M. J., Santos, M. R., Becker, J. D., Cooper, V. S., et al. (2018). The OmpR regulator of Burkholderia multivorans controls mucoid-to-nonmucoid transition and other cell envelope properties associated with persistence in the cystic fibrosis lung. J. Bacteriol. 200, e216–e218.
Skorupska, A., Janczarek, M., Marczak, M., Mazur, A., and Krol, J. (2006). Rhizobial exopolysaccharides: genetic control and symbiotic functions. Microb Cell Fact 5:7.
Sousa, S. A., Feliciano, J. R., Pinheiro, P. F., and Leitão, J. H. (2013). Biochemical and functional studies on the Burkholderia cepacia complex bceN gene, encoding a GDP-D-mannose 4,6-dehydratase. PLoS One 8:e56902. doi: 10.1371/journal.pone.0056902
Sousa, S. A., Ulrich, M., Bragonzi, A., Burke, M., Worlitzsch, D., Leitão, J. H., et al. (2007). Virulence of Burkholderia cepacia complex strains in gp91phox-/- mice. Cell Microbiol. 9, 2817–2825. doi: 10.1111/j.1462-5822.2007.00998.x
Talbi, C., Delgado, M. J., Girard, L., Ramirez-Trujillo, A., Caballero-Mellado, J., and Bedmar, E. J. (2010). Burkholderia phymatum strains capable of nodulating Phaseolus vulgaris are present in Moroccan soils. Appl. Environ. Microbiol. 76, 4587–4591. doi: 10.1128/AEM.02886-09
Udvardi, M., and Poole, P. S. (2013). Transport and metabolism in legume-rhizobia symbioses. Annu. Rev. Plant Biol. 64, 781–805. doi: 10.1146/annurev-arplant-050312-120235
Urzainqui, A., and Walker, G. C. (1992). Exogenous suppression of the symbiotic deficiencies of Rhizobium meliloti exo mutants. J. Bacteriol. 174, 3403–3406. doi: 10.1128/jb.174.10.3403-3406.1992
Zlosnik, J. E., Costa, P. S., Brant, R., Mori, P. Y., Hird, T. J., Fraenkel, M. C., et al. (2011). Mucoid and nonmucoid Burkholderia cepacia complex bacteria in cystic fibrosis infections. Am. J. Respir. Crit. Care Med. 183, 67–72. doi: 10.1164/rccm.201002-0203OC
Keywords: Rhizobium, legume, nodulation, Burkholderia cepacia exopolysaccharide (bce) cluster, biofilm, nitrogen limitation
Citation: Liu Y, Bellich B, Hug S, Eberl L, Cescutti P and Pessi G (2020) The Exopolysaccharide Cepacian Plays a Role in the Establishment of the Paraburkholderia phymatum – Phaseolus vulgaris Symbiosis. Front. Microbiol. 11:1600. doi: 10.3389/fmicb.2020.01600
Received: 09 April 2020; Accepted: 18 June 2020;
Published: 16 July 2020.
Edited by:
Brigitte Mauch-Mani, Université de Neuchâtel, SwitzerlandReviewed by:
Manojkumar Arthikala, National Autonomous University of Mexico, MexicoMaria Jose Soto, Estación Experimental de Zaidín (CSIC), Spain
Copyright © 2020 Liu, Bellich, Hug, Eberl, Cescutti and Pessi. This is an open-access article distributed under the terms of the Creative Commons Attribution License (CC BY). The use, distribution or reproduction in other forums is permitted, provided the original author(s) and the copyright owner(s) are credited and that the original publication in this journal is cited, in accordance with accepted academic practice. No use, distribution or reproduction is permitted which does not comply with these terms.
*Correspondence: Gabriella Pessi, Z2FicmllbGxhLnBlc3NpQGJvdGluc3QudXpoLmNo