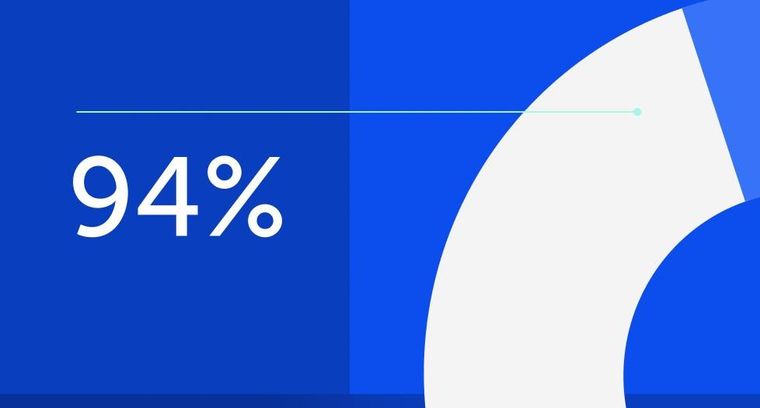
94% of researchers rate our articles as excellent or good
Learn more about the work of our research integrity team to safeguard the quality of each article we publish.
Find out more
ORIGINAL RESEARCH article
Front. Microbiol., 24 June 2020
Sec. Infectious Agents and Disease
Volume 11 - 2020 | https://doi.org/10.3389/fmicb.2020.01270
This article is part of the Research TopicMolecular Adaptations of Vibrionaceae to Changing EnvironmentsView all 14 articles
The biofloc system is a relatively new aquaculture technology that offers practical solution to maintain culture water quality by recycling nutrients and improves the health status and resistance of shrimps against microbial infection, yet the mode of action involved remains unclear. This study aimed to unravel the underlying mechanism behind the protective effect of a biofloc system using Litopenaeus vannamei and acute hepatopancreatic necrosis disease (AHPND)-causing Vibrio parahaemolyticus M0904 strain as a host-pathogen model. The results showed that a biofloc system maintained at a C/N ratio of 15, improves the water quality and contributes to the nutrition of cultured animals as bioflocs might serve as an additional protein source. Furthermore, the study demonstrated that the biofloc system enhances the survival of L. vannamei upon challenge with a V. parahaemolyticus AHPND strain. Remarkably, the results highlight that in the biofloc system, AHPND-causing V. parahaemolyticus possibly switch from free-living virulent planktonic phenotype to a non-virulent biofilm phenotype, as demonstrated by a decreased transcription of flagella-related motility genes (flaA, CheR, and fliS), Pir toxin (PirBVP), and AHPND plasmid genes (ORF14) and increased expression of the phenotype switching marker AlkPhoX gene in both in vitro and in vivo conditions. Taken together, results suggest that biofloc steer phenotype switching, contributing to the decreased virulence of V. parahaemolyticus AHPND strain toward shrimp postlarvae. This information reinforces our understanding about AHPND in a biofloc setting and opens the possibility to combat AHPND not only by trying to eliminate the AHPND-causing V. parahaemolyticus from the system but rather to steer the phenotypic switch.
Shrimp aquaculture is one of the fastest growing high-protein animal food producing sectors in the world (FAO, 2014; Kumar et al., 2016; Roy et al., 2018). However, disease outbreaks, causing production loss in shrimp aquaculture, have moved to the forefront in recent years. Particularly in Asia and North America, acute hepatopancreatic necrosis disease (AHPND) has resulted in collective losses exceeding an estimated US $43 billion (China, Malaysia, Thailand, Vietnam, and Mexico; Leung and Bates, 2013; Shinn et al., 2018; Kumar V. et al., 2018; Roy et al., 2019). Multiple factors might be at the basis of AHPND. The presence of virulent V. parahaemolyticus (VPAHPND) is the primary factor (Kumar and Bossier, 2018, 2019; Roy et al., 2020). However, a compromised health status of the cultured animals in combination with suboptimal environmental conditions most likely facilitates AHPND outbreaks, resulting in high mortalities of juvenile shrimp and often entire loss of stocks within 30 days of stocking (Liu and Chen, 2004; Sajali et al., 2019; Kumar et al., 2019b). Therefore, disease prevention and control measures should not only focus on maintaining a biosecure environment but should be based on an integral approach ensuring among others adequate nutrition and good health of the aquatic animals and maintenance of optimum water quality.
Growing shrimp in a biofloc system can be a promising alternative strategy to improve environmental conditions and health status of cultured animals. The basic principle of the biofloc system is to recycle waste nutrients, in particular, inorganic nitrogen resulting from uneaten feed and feces into microbial biomass, by steering the C/N ratio of the water through the modification of carbohydrate content in feed or by addition of a carbon source in the water (Avnimelech, 1999; Crab et al., 2012; Ekasari et al., 2014; Kumar V.S. et al., 2018). Biofloc systems have been reported to increase feed utilization, growth, and survival. Biofloc can also act as immunostimulants to enhance the shrimp innate immune system and provide protection against microbial pathogens including AHPND-causing V. parahaemolyticus (Crab et al., 2010a; Kim et al., 2014; Bossier and Ekasari, 2017; Sajali et al., 2019). In fact, bioflocs as such can decrease the impact of AHPND V. parahaemolyticus challenge; however, the protective potential depends on operational parameters of the biofloc system (Hostins et al., 2019). In this context, there is an urgent need to systematically unravel the protective mechanisms of a biofloc environment and develop a biofloc system that can control AHPND infection in shrimp aquaculture.
In the previous studies, it was found that the M0904 AHPND strain contains a pVA1 plasmid that harbor the PirABVP toxin genes and encodes for the binary toxins named PirAVP and PirBVP. The binary PirABVP toxin are the primary virulence factor of V. parahaemolyticus that mediate AHPND etiology and mortality in shrimps (Kumar V. et al., 2018; Kumar et al., 2019a, b; Roy et al., 2019). However, in the subsequent study it was shown that environmental conditions can change the V. parahaemolyticus M0904 strain phenotype, displaying, among others, strongly down-regulated expression of flagella-related motility flagellin (flaA), chemotaxis protein (CheR), flagellin specific chaperone (fliS), and virulence related genes (Pir toxin, PirBVP, and AHPND plasmid coding gene, ORF-14), while the expression of phenotype switching marker, alkaline phosphatase PhoX (AlkPhoX) gene, was significantly increased (Kumar et al., 2020). In addition, the phenotype switching resulted in decreased virulence of AHPND-causing V. parahaemolyticus strain toward two crustacean larvae, namely Artemia and Macrobrachium.
Based on observations on the one hand of V. parahaemolyticus phenotype switching in altered environmental conditions (Kumar et al., 2020) and results of Hostins et al. (2019) on the other hand on protective potential of biofloc system against AHPND-causing V. parahaemolyticus, it is hypothesized that a biofloc environment is involved in setting the phenotypic status of AHPND-causing V. parahaemolyticus under both in vitro and in vivo conditions, altering its virulence. This hypothesis was verified using a biofloc-based L. vannamei and V. parahaemolyticus model. The findings demonstrate that apart from improving water quality and growth performance of shrimp, the described biofloc system indeed steers phenotype switching in the V. parahaemolyticus AHPND strain and this effect leads to a decreased in vitro and in vivo virulence and an improved survival of L. vannamei.
The experiments were carried out at the Laboratory of Aquaculture & Artemia Reference Center, Ghent University, Belgium. Six glass aquaria tanks (39 cm × 21 cm × 25 cm) filled with 20 L seawater (30 g l–1 salinity) were used as the experimental culture units. During the entire culture period, the photoperiod was maintained with a regime of 12 h light and 12 h darkness, and the water temperature was maintained at 27.5–28.5°C in a controlled temperature room. The Pacific white shrimp postlarvae (Litopenaeus vannamei) obtained from Shrimp Improvement Systems (SIS, Miami, United States), previously conditioned and acclimatized to the experimental condition for 1 week, at an initial average body weight of 0.31 ± 0.02 g, were randomly distributed in the tanks at a density of 30 shrimps/tank (366 shrimps m–2). Four times daily, a commercial pellet diet containing 35% crude protein and 10% crude fat (CreveTec Grower 2, Belgium) was provided for 28 days to all the tanks. The feeding levels were determined at 7% on wet body weight per day and the daily feed amount was adjusted to the biomass in the tanks.
For biofloc development, the biofloc starter culture, e.g., water sample (100 ml) from an established biofilter system, were collected and added to 100 l seawater (30 g l–1 salinity). Subsequently, the carbon source [D(+)-glucose, Merckmillipore, Belgium] was added continuously throughout the day to provide an estimated daily input C/N ratio of 15 [based on the previous work from our laboratory by Hostins et al. (2019), demonstrated to provide protection against AHPND at C/N ratio of 15], to induce the biofloc formation (Crab et al., 2010a, b; Hostins et al., 2019). The C/N ratio was calculated based on the following assumptions: the commercial feed contains 35–40% protein; 16% of protein is nitrogen (N); approximately 75% of ingested N is excreted by the shrimp and 50% of glucose is carbon.
The amount of glucose (g) added to maintain C/N ratio of 15 were calculated with the following formula
Glucose (g): Feed input (g) × 0.35 (protein content) × 0.16 (N in feed) × 0.75 (N excreted) × 15 × 2
After 2 days, the biofloc water (BFW) was distributed in treatment tanks and the control tanks were filled with seawater (30 g l–1 salinity), in three replicates (Supplementary Figure S1; De Schryver et al., 2008; Hostins et al., 2019). Afterwards, during 28-days culture period, glucose (carbon source) was added daily 2 h after feeding at an estimated C/N ratio of 15 to maintain homogeneous biofloc in the treatment group. The culture water in the control group was exchanged daily @ 60% with seawater (30 g l–1 salinity); in the treatment group seawater was regularly added only to make up for the water loss due to evaporation.
The dissolved oxygen, temperature, pH, and salinity were daily measured in situ using a portable multiparameter photometer (Hanna instruments, Belgium), pH meter (VWR, Belgium), and refractometer (VWR, Belgium). The dissolved inorganic nitrogen, i.e., nitrite nitrogen (NO2–-N) and nitrate nitrogen (NO3–-N), were determined every 2 days using a multiparameter photometer (Hanna instruments, Belgium) according to the manufacturer instructions. On every second day, the BFW samples were filtered using 0.6-μm glass fiber micro-filters (GF-6, Macherey-Nagel, Düren, Germany) and were monitored for total suspended solids (TSS; APHA, 2005). The weight difference of the dried samples before and after ignition was taken for calculation of volatile suspended solids (VSS). The biofloc volume was also measured every second day after 15–20 min of sedimentation using Imhoff cones. The Kjeldahl nitrogen (Kj-N) and total ammonia nitrogen (TAN; NH4+-N + NH3) were measured according to standard methods (Crab et al., 2007). The difference between Kj-N and TAN was used to calculate the protein content of the biofloc by multiplying the organic nitrogen content by 6.25 (Jauncey, 1982; Crab et al., 2010a).
The growth performance of L. vannamei cultured in either biofloc system or clear water system was analyzed at the end of the experimental period. The wet weight of shrimps from the treatment and control groups were measured and performance was assessed using the following formula:
Weight gain (%) = (Final weight – Initial weight)/Initial weight × 100
Weekly weight gain (g wk–1) = (Final weight – Initial weight)/week
SGR (specific growth rate; %) = (ln average final weight – ln average initial weight)/number of culture days × 100
FCR (feed conversion ratio) = Total dry feed intake (g)/wet weight gain
To determine the protective effect of bioflocs in L. vannamei postlarvae, a challenge assay was performed in four separate groups (Figure 1). Briefly, the shrimp postlarvae cultured in either the biofloc system or the clear water system for 28 days were collected and transferred to 2 L plastic containers. In the first and second group, 30 shrimp postlarvae from clear water control group were collected and group of 5 postlarvae/replicate were transferred to 2 L plastic container filled with either 1 L clear seawater (SW; 30 g l–1 salinity) or 1 L BFW. Similarly, in the third and fourth group, the 30 shrimp postlarvae collected from biofloc system were transferred to 2 L plastic container and filled with either 1 L SW or BFW. All the containers provided with aeration was maintained at 27.5–28.5°C in a controlled temperature room. Subsequently, it was verified whether the addition of BFW could protect the postlarvae against subsequent challenge, by immersion, with V. parahaemolyticus M0904 strain (BCCM accession number – LMG P-31518) at 106 cells/ml. The day before challenge assay, 50 μl of V. parahaemolyticus M0904 strain stock culture was inoculated into 20 ml sterile marine broth in 50 ml erlenmeyer and grown overnight at 28°C under constant agitation (120 rpm). The survival of shrimp postlarvae was scored at 12, 24, 36, and 48 h after the addition of the pathogen. The postlarvae that were not challenged with V. parahaemolyticus were maintained as negative controls. Each group in the treatment and control were maintained in triplicate.
Figure 1. Schematic of Litopenaeus vannamei postlarvae challenge assay grown in control clear water and biofloc water and challenged with V. parahaemolyticus M0904 strain.
The water from either biofloc system or the clear water system, collected in separate 2 L plastic containers in triplicates, were supplemented with the V. parahaemolyticus M0904 strain @ 106 cells/ml and further incubated at 28°C. After 12 and 24 h of incubation, the microbial cells were harvested through centrifugation (4000×g for 15 min), and total RNA was extracted with Qiagen RNeasy Plus Mini Kit (Cat No. 74136) according to the manufacturer instructions. The RNA quality and quantity were measured on NanoDrop spectrophotometer (ThermoFisher Scientific, Belgium) and RNA samples with A260/A280 ratios > 2.0 and A260/A230 ratios > 1.5 were used for the analysis. The RNA integrity was checked by agarose gel electrophoresis and the RNA samples were stored in −80°C for subsequent use.
The bacterial RNA was extracted from challenged L. vannamei postlarvae as described previously (Ruwandeepika et al., 2015). The Pacific white shrimp cultured for 28 days in bioflocs water were challenged with AHPND causing V. parahaemolyticus M0904 strain (@ 106 cells/ml) in new seawater (BFW to SW) or in their respective bioflocs water (BFW to BFW). Similarly, the shrimp cultured in seawater were challenged with AHPND causing V. parahaemolyticus in new seawater (SW to SW) or in bioflocs water (SW to BFW). After 12 and 24 h of incubation, the shrimp were washed with sterile seawater and the hepatopancreas of 3 individual shrimp/group were separately dissected, following a standard protocol of hepatopancreas collection (Cervellione et al., 2017a, b), frozen immediately in liquid nitrogen, and after that they were stored at -80°C until RNA extraction. Before RNA extraction, the postlarval hepatopancreas were homogenized using eppendorf grinder (Mini-Beadbeater, BioSpec Products, United States) under aseptic conditions. Afterwards, the suspension was centrifuged (4000 × g for 5 min) and supernatant was collected to avoid the clogging of the RNA extraction columns during the subsequent RNA extraction. Afterward, the extraction of total RNA was performed as described above for the RNA extraction from in vitro BFW.
Reverse transcription was done with the RevertAidTM H Minus First Strand Complementary deoxyribonucleic acid (cDNA) Synthesis Kit (Thermo Fisher Scientific, Belgium) according to the manufacturer’s guidelines. Briefly, 1 μg total RNA, and 1 μl random hexamer primer solution was mixed first. Then, 8 μl of reaction mixture containing 4 μl of 5× reaction buffer (250 mM Tris–HCl pH 8.3, 250 mM KCl, 20 mM MgCl2, and 50 mM DTT), 2 μl of 0.01 mol–1 dNTP mix, 20 units of ribonuclease inhibitor, and 200 units of RevertAidTM H minus M-MuLV reverse transcriptase was added. The reaction mixture was incubated for 5 min at 25°C followed by 60 min at 42°C. The reaction was terminated by heating at 70°C for 5 min and then cooled to 4°C. cDNA samples were checked by PCR and stored at −20°C for further use.
The expressions of 3 flagella-related motility genes, including regulators, structural, and chemotaxis genes, 2 VPAHPND plasmid related virulent genes including toxin and coding genes of virulent plasmid and AlkPhoX gene, marker gene that indicates the presence of non-virulent AHPND V. parahaemolyticus phenotype, were measured by RT-qPCR with pair of specific primers using StepOnePlus Real-time PCR systems (Applied Biosystems; Supplementary Table S1; Han et al., 2015; Yang and Defoirdt, 2015; Kumar et al., 2020). The Ct values from the two reference genes rpoA (RNA polymerase A submit) and toxR mRNA, used as the internal control, were subjected to geomean and expression of the genes was calculated relative to the rpoA and toxR mRNA levels. The amplification was performed in a total volume of 20 μl, containing 10 μl of 2× Maxima SYBR Green/ROX qPCR Master Mix (Thermo Fisher Scientific), 1 μl of cDNA (50 ng), 8 μl of nuclease free water, and 0.5 μl of each specific primer. Master mixes were prepared for each biological replicate of the sample in triplicate and RT-qPCR for target and reference genes was performed with a four-step amplification protocol: initial denaturation (10 min at 95°C); 40 cycles of amplification and quantification (15 s at 95°C, 30 s at 60°C, and 30 s at 72°C); melting curve (55–95°C) with a heating rate of 0.10°C/s and a continuous fluorescence measurement, and cooling (4°C). A negative control reaction was included for each primer set by omitting template cDNA. The comparative CT method (2-ΔΔCt method) following Livak and Schmittgen (2001) was used to analyze the expression level of the target genes and verified by Pfaffl relative standard curve method (Pfaffl, 2002). The Log transformed 2^ΔΔCT value were subjected to a t-test, and the P values smaller than 0.05 were considered statistically significant.
Water quality and growth parameter data were arcsin transformed to satisfy normality and homoscedasticity requirements as necessary. The data were then subjected to one-way analysis of variances (ANOVA) followed by Duncan’s multiple range test using statistical software statistical package for the social sciences version 24.0. P values ≤ 0.01 were considered significant. Survival data of L. vannamei were subjected to logistic regression analysis using GenStat 16 (VSN International, Hemel Hempstead, United Kingdom) to determine significant differences between the control and treatment. Gene expressions results were represented as fold expression relative to the geometrical mean of two internal control genes (toxR and rpoA). The expression level in the control clear SW group (+V. parahaemolyticus) was regarded as 1.0 and thereby the expression ratio of the treatment biofloc (BFW) group (+V. parahaemolyticus) was expressed in relation to the SW group in the in vitro biofloc experiment. Moreover, in the in vivo experiment, the expression level in the SW to SW group was regarded as 1.0 and thereby the expression of the SW to BFW, BFW to SW, and BFW to BFW was expressed in relation to the SW to SW group. Statistical analysis for the significant differences in the expression levels between the control and treatment groups was performed with single-tailed Student’s t-tests using the log transformed data.
In the first experiment, the water quality of biofloc system was monitored every week for a period of 28 days and was compared with that in the clear water system (Table 1). Significantly lower values of DO and pH were recorded in BFW as compared to the clear water system. The dissolved inorganic nitrogen concentration (NH4+-N and NO2–-N) was significantly lower in BFW as compared to clear water system (Table 1). However, higher values of NO3-N were observed in BFW as compared with clear water system in all sampling weeks. The TSS and VSS values were recorded significantly higher in biofloc group as compared to clear water control group. A crude protein content between 28–43% was observed in biofloc group during the sampling weeks (Table 1). These results indicate that in a biofloc system maintained at a calculated C/N ratio of 15, water quality is improved.
Next, to determine whether the rearing of L. vannamei in biofloc system improves the nutrition and growth of the cultured animals, the growth parameters of shrimp after 28 days of culture period were analyzed. The results showed that growth performance of shrimp reared in biofloc system was significantly higher as compared to those of shrimp cultured in clear water system (Table 2). Final body weight, weight gain, weekly weight gain, and specific growth rate of shrimp reared in the biofloc system were significantly increased as compared to those cultured in clear water system. Additionally, the biofloc maintained at C/N ratio of 15 resulted in significantly lower food conversion ratio (FCR) as compared to the control (Table 2). These results suggest that biofloc is an effective method to improve culture environment and nutrition of the cultured shrimp and the beneficial effect of biofloc is paralleled with improved water quality and growth performance of shrimp in biofloc system (Ekasari et al., 2014; Luis-villaseñor et al., 2016; Bossier and Ekasari, 2017; Lee et al., 2017).
Next, the potential role of BFW in conveying protection in shrimp against AHPND was evaluated. It was verified by four different approaches as demonstrated in Figure 2. The results showed that L. vannamei postlarvae reared for 28 days in biofloc system display significantly higher survival (group 2, 3, and 4) compared to the clear seawater shrimp postlarvae (group 1). In fact, the protective effect against V. parahaemolyticus for biofloc reared shrimp even lasted when transferred to clear water (group 3; Figure 2). Additionally, the results also showed that L. vannamei reared in clear seawater system but challenged in the presence of flocs are also protected (Figure 2), indicating that the protective effect can be conveyed by bioflocs as such.
Figure 2. Effect of Biofloc on the survival of Litopenaeus vannamei postlarvae after 12, 24, 36, and 48 h post challenge with V. parahaemolyticus M0904 strain. The Pacific white shrimp cultured for 28 days in bioflocs water were challenged with AHPND causing V. parahaemolyticus (@ 106 cells/ml) in new seawater (BFW to SW) or in their respective bioflocs water (BFW to BFW). Similarly, the shrimp cultured in seawater were challenged with AHPND causing V. parahaemolyticus in new seawater (SW to SW) or in bioflocs water (SW to BFW). The postlarvae that were not challenged with V. parahaemolyticus served as negative controls. Error bars represent the standard error of three replicates and asterisks represents significant difference between the SW to SW with SW to BFW, BFW to SW, and BFW to BFW *(P < 0.05), **(P < 0.01), ***(P < 0.001), and ****(P < 0.0001).
To investigate the effect of biofloc system further, in vitro temporal expression of virulence-related genes, i.e., flagella-related motility genes, including polar flaA, CheR, polar fliS gene, and VPAHPND plasmid related virulent genes, including toxin (PirBVP) and coding genes of the virulent plasmid (ORF14), were investigated from control clear SW (+V. parahaemolyticus) and treatment BFW (+V. parahaemolyticus). As for the flagella-related motility genes, there was a significant decrease in the expression of flaA, CheR, and flis genes in the BFW as compared to the SW group at any of the time point tested (Figures 3A–C). In addition, the transcript level of PirBVP and ORF14 was also markedly decreased in the BFW when compared with the SW group at 12 and 24 h post treatment (Figures 3D,E).
Figure 3. Expression of flagella-related motility genes: (A) flaA (polar flagellin), (B) flis (polar flagellin specific chaperone), (C) cheR (chemotaxis protein); virulent AHPND plasmid genes: (D) ORF14, (E) PirBVP, and a marker gene that indicate presence of non-virulent AHPND V. parahaemolyticus phenotype: (F) AlkPhoX (alkaline phosphatase PhoX) from in vitro experiment. The expression level in the control clear water group (+ V. parahaemolyticus) was regarded as 1.0 and thereby the expression ratio of the biofloc group (+ V. parahaemolyticus) was expressed in relation to the control SW group (+ V. parahaemolyticus). The results are the mean ± SE (n = 3) and are presented relative to V. parahaemolyticus toxR and rpoA mRNA. Asterisks represents significant difference between the control SW group and biofloc group (+ V. parahaemolyticus) ∗(P < 0.05), ∗∗(P < 0.01), ∗∗∗(P < 0.001), ****(P < 0.0001), and *****(P < 0.00001).
Next, the differential expression of the phenotype switching marker AlkPhoX gene was determined from SW and BFW. Interestingly, as shown in Figure 3F, the mRNA transcript levels of AlkPhoX gene in the BFW increased significantly as compared to SW group (+V. parahaemolyticus) at 12 and 24 h post treatment. This observation suggests that the biofloc environment indeed facilitates phenotypic switching in V. parahaemolyticus leading to a decreased transcription of virulence related genes and increased expression of phenotype switching marker gene, AlkPhoX gene (Kumar et al., 2020).
To study further the transcriptional modifications occurring in biofloc system, the in vivo temporal expression of flagella-related motility genes, VPAHPND plasmid related virulent genes and phenotype switching marker gene of V. parahaemolyticus AHPND strain from control shrimp (SW group) in clear seawater (SW to SW; +V. parahaemolyticus) were investigated and compared with control shrimp in biofloc water (SW to BFW; +V. parahaemolyticus) and biofloc shrimp in either BFW to SW or BFW to BFW (+V. parahaemolyticus). Similar to the in vitro experiment, the transcription of flagella-related motility genes, i.e., flaA, CheR, and flis genes and VPAHPND plasmid related virulent genes, PirBVP and ORF14, was significantly decreased in L. vannamei at 12 and 24 h post exposure (+V. parahaemolyticus) in BFW to SW or BFW to BFW (+V. parahaemolyticus) as compared to the SW to SW group (Figures 4A–E). In addition (Figure 4), there was a downregulation of flaA, CheR, flis, PirBVP, and ORF14 gene in SW to BFW group (+V. parahaemolyticus) when compared with the SW to SW group (+V. parahaemolyticus).
Figure 4. Expression of flagella-related motility genes: (A) flaA (polar flagellin), (B) flis (polar flagellin specific chaperone), (C) cheR (chemotaxis protein); virulent AHPND plasmid genes: (D) ORF14, (E) PirBVP, and marker gene that indicate presence of non-virulent AHPND V. parahaemolyticus phenotype: (F) AlkPhoX (alkaline phosphatase PhoX) from shrimp in vivo experiment. The gene expression level in Pacific white shrimp cultured in seawater and subsequently challenged with AHPND-causing V. parahaemolyticus in new seawater (SW to SW) was regarded as 1.0. Thereby the expression of SW to BFW, BFW to SW, and BFW to BFW was expressed in relation to the SW to SW. The results are the mean ± SE (n = 3) and are presented relative to V. parahaemolyticus toxR and rpoA mRNA. Asterisks represents significant difference between the SW to SW with SW to BFW, BFW to SW, and BFW to BFW *(P < 0.05), **(P < 0.01), ***(P < 0.001), ****(P < 0.0001), and *****(P < 0.00001).
To further substantiate that biofloc system induces phenotypic switching and increase the expression of the switching marker gene, the transcription of AlkPhoX gene was examined in shrimp reared under biofloc or clear water conditions. Interestingly, increased expression level of AlkPhoX gene was observed at 12 and 24 h post exposure (+V. parahaemolyticus) in BFW to BFW group as compared to the SW to BFW, BFW to SW, and SW to SW groups (Figure 4F). In contrast, downregulation of AlkPhoX gene was recorded in BFW to SW group after 24 h post challenge (+V. parahaemolyticus). Taken together, our in vivo assay strongly suggests that the biofloc system indeed plays an essential role in phenotype switching, mediating virulence of V. parahaemolyticus in L. vannamei postlarvae.
Acute hepatopancreatic necrosis disease, a newly emergent farmed penaeid shrimp bacterial disease originally known as early mortality syndrome (EMS), has been particularly devastating in the cultivation of shrimp, causing massive mortality (up to 100%) within 20–30 days of post-larvae stocking (Tran et al., 2013; Lee et al., 2015; Dong et al., 2017; Kumar V. et al., 2018; Kumar et al., 2019a, b; Roy et al., 2019). However, the AHPND V. parahaemolyticus strain, under differential flow conditions (low fluid shear stress), switches phenotype causing a major shift in the protein secretome, e.g., AlkPhoX is produced instead of PirAVP/PirBVP toxins. This phenotype is also less virulent to shrimp species (Kumar et al., 2020). On the other hand it has been shown that bioflocs stimulates the innate immunity and decrease the impact of AHPND V. parahaemolyticus challenge in shrimp (Hostins et al., 2019). In combination, these observations suggest that the protective mechanisms of biofloc might be at least, in part, related to the specific biofloc environment that potentially regulates the virulence of AHPND V. parahaemolyticus. Here, a possible scenario is considered that biofloc environment is involved in mediating the phenotypic status of AHPND-causing V. parahaemolyticus. In addition, it is assumed that biofloc induced phenotype switching modulates the in vitro and in vivo virulence of AHPND strain.
Biofloc technology offers interesting perspectives of high-density culture with the possibility to maintain good water quality with minimal or no water exchange by nutrient recycling, in particular, nitrogen into microbial biomass that can be used in situ by the cultured animals (Avnimelech, 1999; Kuhn et al., 2009; Fatimah et al., 2019). In the present study, the water quality characteristics in the biofloc group were significantly improved and found to be within the optimal range required for growth and improved immunity of L. vannamei (Wickins, 1976; Cohen et al., 2005; Mishra et al., 2008). TAN (NH4+-N + NH3) and nitrite (NO2–N) concentration were significantly reduced in the biofloc system after 7, 14, 21, and 28 days culture period as compared to the control (Table 1). In addition, the biofloc system significantly improved the growth performance and L. vannamei tolerance against the AHPND-causing V. parahaemolyticus strain (Table 2 and Figure 2). The biofloc system is generally formed by aggregation of microorganism, microalgae, organic particles, or solids from uneaten feeds (Cohen et al., 2005; Wasielesky et al., 2006; Azim and Little, 2008; De Schryver et al., 2008; Ju et al., 2008; Trichet, 2010; Cardona et al., 2015; Lee et al., 2017). Also, for shrimp in a biofloc system, flocs consumed may constitute up to 29% of the daily feed intake (Burford et al., 2003). Therefore, the consumed biofloc might nutritionally modulate the shrimp health status resulting in improved growth performance and increased protection of L. vannamei against V. parahaemolyticus AHPND strain.
Shear stress or flow behavior is an important factor modulating the growth of bacteria either in a free-living planktonic form or in a matrix enclosed communities (e.g., a biofilm; Crabbé et al., 2008; Giese et al., 2014; Mancilla et al., 2015; Dingemans et al., 2016; Kumar et al., 2020). Hence, to gain insight into the V. parahaemolyticus phenotype status in biofloc system, we used qPCR technique to assess the in vitro temporal expression of flagella-related motility genes and VPAHPND plasmid related virulent genes. The results indicated that expression of polar flaA, CheR, and polar fliS gene was significantly decreased in biofloc group (+V. parahaemolyticus) when compared with the control SW group (+V. parahaemolyticus; Figures 3A–C). Since there exist a correlation between flagellar motility and virulence of bacterial pathogens, the temporal downregulation of flagellar motility genes in V. parahaemolyticus suggests a decrease in its virulence in biofloc condition (Watnick, 2000; Hawkins et al., 2017; Zhang et al., 2018). In addition, the transcription of pVA1 plasmid-bound genes, i.e., PirBVP (responsible for PirBVP toxin), and ORF-14 (copy number of the pVA1 plasmid) which mediate the virulence of V. parahaemolyticus was also significantly downregulated in the biofloc group (+V. parahaemolyticus) as compared with the control SW group (+V. parahaemolyticus; Figures 3D,E). In contrast, the in vitro temporal expression of AlkPhoX gene in the biofloc group (+V. parahaemolyticus) was significantly increased at 12 and 24 h as compared to the control SW group (+V. parahaemolyticus; Figure 3F). The results therefore suggest that V. parahaemolyticus in biofloc system switch to non-virulent phenotype, as deduced from the increased expression of phenotype switching marker gene, AlkPhoX gene, with a simultaneous decrease of the expression of flagella-related motility and other virulent genes. This expression profile has previously been shown to be associated with the non-virulent phenotypic status of this V. parahaemolyticus AHPND strain (M0904 strain; Kumar et al., 2020).
The hepatopancreas is considered the primary target tissue of AHPND bacteria, as affected shrimp show an abnormal hepatopancreas (small, shrunken, or black coloration) and histopathological lesions on gross examination (Eduardo and Mohan, 2012; Lightner et al., 2013), apparently caused by in situ PirAVP/PirBVP toxin production. Hence, L. vannamei hepatopancreas was used to study the in vivo effect of biofloc supplementation on the phenotype status and virulence of AHPND bacteria by measurement of flagella-related motility genes and VPAHPND plasmid related virulent genes expression after 12 and 24 h post AHPND-causing V. parahaemolyticus challenge. In the control clear SW group, V. parahaemolyticus produce AHPND-causing Pir toxin in the hepatopancreas, and hence appear to adopt the planktonic mode of growth (Figures 4A–E; Nunan et al., 2014; Khimmakthong and Sukkarun, 2017). However, in the treatment biofloc group, the expression of motility and Pir toxin genes in the hepatopancreas were significantly downregulated, and increased production of AlkPhoX was recorded (Figure 4F). The results allow to develop the following hypothesis. When AHPND-causing V. parahaemolyticus are added into biofloc system, the bacterium might get settled in flocs and possibly switch into the biofilm phenotype (in vitro upregulation of AlkPhoX gene, found in earlier results). Subsequently, after consumption of flocs, the AHPND bacteria enters the host. Additionally, the production of AlkPhoX in hepatopancreas of biofloc treatment shrimps suggests that under biofloc consumption conditions V. parahaemolyticus might remain in the biofilm phenotype and is not switching back to the planktonic status. It is, however, not clear why AHPND Vibrio inside the host don’t switch back to the free-living planktonic form and produce PirAVP/PirBVP toxins, like it seems to happen when AHPND symptoms are apparent. This needs further investigations. Taking together, it can be suggested that biofloc indeed induces phenotype switching in V. parahaemolyticus in both in vitro and in vivo conditions, which results in increased resistance of L. vannamei against V. parahaemolyticus AHPND strain. Moreover, the aggregating bioflocs are rich in microbially bioactive components (so called MAMPs) which potentially improve the immune response resulting in better growth performance and improved resistance against pathogenic microbial infections (Cardona et al., 2015; Anand et al., 2017; Lee et al., 2017). For instance, the expression of immune related genes, i.e., prophenoloxidase, serine protease, prophenoloxidase activating enzyme, complement system and lysozyme, were reported to be increased significantly in L. vannamei cultured in a biofloc system leading to an increased resistance against Vibrio harveyi (Kim et al., 2014; Liu et al., 2017). In another study, Xu and Pan (2013) found that shrimp grown in biofloc environment has higher total haemocyte count, phagocytic activity, and total antioxidant activity as compared to non-biofloc control shrimp (Xu and Pan, 2013). Hence, further studies on the mechanisms by which bioflocs interfere with both host nutrition and immunological response on the one hand and expression of virulence genes in V. parahaemolyticus on the other hand will help to better understand how biofloc system enhance survival of L. vannamei upon V. parahaemolyticus AHPND strain challenge.
In conclusion, the findings presented here provide new insight on the protective action of biofloc system against V. parahaemolyticus AHPND strain. Our results imply that the protective effect of biofloc system is at least partially caused by steering the phenotype switching, where AHPND-causing V. parahaemolyticus might switch to a non-virulent matrix-enclosed biofilm phenotype. Furthermore, the biofloc-induced phenotype switching seems to be responsible for regulating the expression of AHPND bacteria flagella-related motility genes and Pir toxins and AHPND plasmid genes in both in vitro and in vivo conditions. The ability of the biofloc system to boost the water quality, growth performance, and resistance of L. vannamei against V. parahaemolyticus AHPND strain makes it a potent aquaculture technology that will be valuable to prevent microbial infection including AHPND and increase the shrimp production with high-density and minimal or no water exchange culture.
The raw data supporting the conclusions of this article will be made available by the authors, without undue reservation, to any qualified researcher.
VK and PB conceived and designed the experiments. VK performed the experiments and drafted the figures and manuscript. VK, MW, and TL made the laboratory analysis, statistics, and interpreted data. VK, MW, and PB reviewed and edited the manuscript. All the authors approved the final manuscript.
The authors declare that the research was conducted in the absence of any commercial or financial relationships that could be construed as a potential conflict of interest.
We thank Jorg De Smyter, Lab of Aquaculture & Artemia Reference Center, Ghent University for his technical support in Litopenaeus vannamei culture. Additionally, we also thank Brecht Stechele and Manh Hoang Nghia, Lab of Aquaculture & Artemia Reference Center, Ghent University, for their support in biofloc experiment. VK is supported by Netaji Subhas International Doctoral Fellowship from Indian Council of Agricultural Research (ICAR), New Delhi.
The Supplementary Material for this article can be found online at: https://www.frontiersin.org/articles/10.3389/fmicb.2020.01270/full#supplementary-material
Anand, P. S. S., Kumar, S., Sundaray, J. K., and Sinha, A. (2017). Dietary biofloc supplementation in black tiger shrimp, Penaeus monodon: effects on immunity, antioxidant and metabolic enzyme activities. Aquac. Res. 48, 4512–4523. doi: 10.1111/are.13276
APHA (2005). Standard Methods for the Examination of the Water and Waste Water. Washington, DC: American Public Health Association.
Avnimelech, Y. (1999). Carbon/nitrogen ratio as a control element in aquaculture systems. Aquaculture 176, 227–235. doi: 10.1016/s0044-8486(99)00085-x
Azim, M. E., and Little, D. C. (2008). The biofloc technology (BFT) in indoor tanks: water quality, biofloc composition, and growth and welfare of Nile tilapia (Oreochromis niloticus). Aquaculture 283, 29–35. doi: 10.1016/j.aquaculture.2008.06.036
Bossier, P., and Ekasari, J. (2017). Biofloc technology application in aquaculture to support sustainable development goals. Microb. Biotechnol. 10, 1012–1016. doi: 10.1111/1751-7915.12836
Burford, M. A., Thompson, P. J., Bauman, H., and Pearson, D. C. (2003). Microbial communities affect water quality, shrimp performance at belize aquaculture. Glob. Aquac. Advoc. 6, 64–65.
Cardona, E., Saulnier, D., Lorgeoux, B., Chim, L., and Gueguen, Y. (2015). Rearing effect of biofloc on antioxidant and antimicrobial transcriptional response in Litopenaeus stylirostris shrimp facing an experimental sub-lethal hydrogen peroxide stress. Fish Shellf. Immunol. 45, 933–939. doi: 10.1016/j.fsi.2015.05.041
Cervellione, F., McGurk, C., Berger Eriksen, T., and Van den Broeck, W. (2017a). Use of computer-assisted image analysis for semi-quantitative histology of the hepatopancreas in whiteleg shrimp Penaeus vannamei (Boone). J. Fish Dis. 40, 1223–1234. doi: 10.1111/jfd.12599
Cervellione, F., McGurk, C., and Van Den Broeck, W. (2017b). Effect of starvation and refeeding on the ultrastructure of the perigastric organ (hepatopancreas) in the whiteleg shrimp Litopenaeus vannamei (Boone, 1931) (Decapoda: Caridea: Penaeidae). J. Crustac. Biol. 37, 693–700. doi: 10.1093/jcbiol/rux085
Cohen, J. M., Samocha, T. M., Fox, J. M., Gandy, R. L., and Lawrence, A. L. (2005). Characterization of water quality factors during intensive raceway production of juvenile Litopenaeus vannamei using limited discharge and biosecure management tools. Aquac. Eng. 32, 425–442. doi: 10.1016/j.aquaeng.2004.09.005
Crab, R., Avnimelech, Y., and Defoirdt, T. (2007). Nitrogen removal techniques in aquaculture for a sustainable production. Aquaculture 270, 1–14. doi: 10.1016/j.aquaculture.2007.05.006
Crab, R., Chielens, B., Wille, M., Bossier, P., and Verstraete, W. (2010a). The effect of different carbon sources on the nutritional value of bioflocs, a feed for Macrobrachium rosenbergii postlarvae. Aquac. Res. 41, 559–567. doi: 10.1111/j.1365-2109.2009.02353.x
Crab, R., Lambert, A., Defoirdt, T., Bossier, P., and Verstraete, W. (2010b). The application of bioflocs technology to protect brine shrimp (Artemia franciscana) from pathogenic Vibrio harveyi. J. Appl. Microbiol. 109, 1643–1649. doi: 10.1111/j.1365-2672.2010.04791.x
Crab, R., Defoirdt, T., Bossier, P., and Verstraete, W. (2012). Biofloc technology in aquaculture: beneficial effects and future challenges. Aquaculture 356-357, 351–356. doi: 10.1016/j.aquaculture.2012.04.046
Crabbé, A., De Boever, P., Van Houdt, R., Moors, H., Mergeay, M., and Cornelis, P. (2008). Use of the rotating wall vessel technology to study the effect of shear stress on growth behaviour of Pseudomonas aeruginosa PA01. Environ. Microbiol. 10, 2098–2110. doi: 10.1111/j.1462-2920.2008.01631.x
De Schryver, P., Crab, R., Defoirdt, T., Boon, N., and Verstraete, W. (2008). The basics of bio-flocs technology: the added value for aquaculture. Aquaculture 277, 125–137. doi: 10.1016/j.aquaculture.2008.02.019
Dingemans, J., Monsieurs, P., Yu, S.-H., Crabbé, A., Förstner, K., Malfroot, A., et al. (2016). Effect of Shear stress on Pseudomonas aeruginosa Isolated from the cyctic fibrosis lung. mBio 7, 1–16. doi: 10.1128/mBio.00813-16.Editor
Dong, X., Bi, D., Wang, H., Zou, P., Xie, G., Wan, X., et al. (2017). pirABvp-Bearing Vibrio parahaemolyticus and Vibrio campbellii pathogens isolated from the Same AHPND-affected pond possess highly similar pathogenic plasmids. Front. Microbiol. 8:1859. doi: 10.3389/fmicb.2017.01859
Eduardo, M. L., and Mohan, C. V. (2012). Emerging Threat In The Asian Shrimp Industry: Early Mortality Syndrome (EMS)/Acute Hepatopancreatic Necrosis Syndrome (AHPNS). Available online at: http://www.fhs-afs.net/pdf/FHS(AFS)_Newsletter_10_2012_Final.pdf (accessed June 21, 2019).
Ekasari, J., Hanif, M., Surawidjaja, E. H., Nuryati, S., Schryver, P., and Bossier, P. (2014). Immune response and disease resistance of shrimp fed biofloc grown on different carbon sources. Fish Shellfish Immunol. 41, 332–339. doi: 10.1016/j.fsi.2014.09.004
Fatimah, N., Pande, G. S. J., Natrah, F. M. I., Meritha, W. W., Widanarni, L., Sucipto, A., et al. (2019). The role of microbial quorum sensing on the characteristics and functionality of bioflocs in aquaculture systems. Aquaculture 504, 420–426. doi: 10.1016/j.aquaculture.2019.02.022
Giese, H., Klockner, W., Pena, C., Galindo, E., Lotter, S., Wetzel, K., et al. (2014). Effective shear rates in shake flasks. Chem. Eng. Sci. 118, 102–113. doi: 10.1016/j.ces.2014.07.037
Han, J. E., Tang, K. F. J., Tran, L. H., and Lightner, D. V. (2015). Photorhabdus insect-related (Pir) toxin-like genes in a plasmid of Vibrio parahaemolyticus, the causative agent of acute hepatopancreatic necrosis disease (AHPND) of shrimp. Dis. Aquat. Organ. 113, 33–40. doi: 10.3354/dao02830
Hawkins, J. P., Geddes, B. A., and Oresnik, I. J. (2017). Common dyes used to determine bacterial polysaccharides on agar are affected by medium acidification. Can. J. Microbiol. Common 63, 559–562. doi: 10.1139/cjm-2016-0743
Hostins, B., Wasielesky, W., Decamp, O., Bossier, P., and De Schryver, P. (2019). Managing input C/N ratio to reduce the risk of Acute hepatopancreatic necrosis disease (AHPND) outbreaks in biofloc systems - a laboratory study. Aquaculture 508, 60–65. doi: 10.1016/j.aquaculture.2019.04.055
Jauncey, K. (1982). The effects of varying dietary protein level on the growth, food conversion, protein utilization and body composition of juvenile tilapias. Aquaculture 27, 43–54. doi: 10.1016/0044-8486(82)90108-9
Ju, Z. Y., Forster, I., Conquest, L., and Dominy, W. (2008). Enhanced growth effects on shrimp (Litopenaeus vannamei) from inclusion of whole shrimp floc or floc fractions to a formulated diet. Aquac. Nutr. 14, 533–543. doi: 10.1111/j.1365-2095.2007.00559.x
Khimmakthong, U., and Sukkarun, P. (2017). The spread of Vibrio parahaemolyticus in tissues of the Pacific white shrimp Litopenaeus vannamei analyzed by PCR and histopathology. Microb. Pathog. 113, 107–112. doi: 10.1016/j.micpath.2017.10.028
Kim, S., Pang, Z., Seo, H., Cho, Y., Samocha, T., and Jang, I. (2014). Effect of bioflocs on growth and immune activity of Pacific white shrimp, Litopenaeus vannamei postlarvae. Aquac. Res. 45, 362–371. doi: 10.1111/are.12319
Kuhn, D. D., Boardman, G. D., Lawrence, A. L., Marsh, L., and Flick, G. J. (2009). Microbial floc meal as a replacement ingredient for fish meal and soybean protein in shrimp feed. Aquaculture 296, 51–57. doi: 10.1016/j.aquaculture.2009.07.025
Kumar, V., Baruah, K., Nguyen, D. V., Smagghe, G., Vossen, E., and Bossier, P. (2018). Phloroglucinol mediated Hsp70 production in crustaceans: protection against Vibrio parahaemolyticus in Artemia franciscana and Macrobrachium rosenbergii. Front. Immunol. 9:1091. doi: 10.3389/fimmu.2018.01091
Kumar, V. S., Pandey, P. K., Anand, T., Bhuvaneswari, G. R., Dhinakaran, A., and Kumar, S. (2018). Biofloc improves water, effluent quality and growth parameters of Penaeus vannamei in an intensive culture system. J. Environ. Manag. 215, 206–215. doi: 10.1016/j.jenvman.2018.03.015
Kumar, V., Bels, L., De Couck, L., Baruah, K., Bossier, P., Broeck, W., et al. (2019a). PirABVP Toxin binds to epithelial cells of the digestive tract and produce pathognomonic AHPND lesions in germ-free brine shrimp. Toxins 11:717. doi: 10.3390/toxins11120717
Kumar, V., Viet, D., Baruah, K., and Bossier, P. (2019b). Probing the mechanism of VP AHPND extracellular proteins toxicity purified from Vibrio parahaemolyticus AHPND strain in germ-free Artemia test system. Aquaculture 504, 414–419. doi: 10.1016/j.aquaculture.2019.02.029
Kumar, V., and Bossier, P. (2018). Importance of plant - derived compounds and/or natural products in aquaculture. Aquafeed 10, 28–31.
Kumar, V., and Bossier, P. (2019). Novel plant-based compounds could be useful in protecting shrimp species against AHPND Vibrio parahaemolyticus. J. Inl. Fish. Soc. India 51, 03–05.
Kumar, V., Roy, S., Baruah, K., Van Haver, D., Impens, F., and Bossier, P. (2020). Environmental conditions steer phenotypic switching in AHPND-causing Vibrio parahaemolyticus, affecting PirABVP toxin production. Environ. Microbiol. 1–19. doi: 10.1111/1462-2920.14903
Kumar, V., Roy, S., Meena, D. K., and Sarkar, U. K. (2016). Application of probiotics in shrimp aquaculture: importance, mechanisms of action, and methods of administration. Rev. Fish. Sci. Aquac. 24:841. doi: 10.1080/23308249.2016.1193841
Lee, C., Kim, S., Lim, S., and Lee, K. (2017). Supplemental effects of biofloc powder on growth performance, innate immunity, and disease resistance of Pacific white shrimp Litopenaeus vannamei. Fish. Aquat. Sci. 20, 1–7. doi: 10.1186/s41240-017-0059-7
Lee, C.-T., Chen, I.-T., Yang, Y.-T., Ko, T.-P., Huang, Y.-T., Huang, J.-Y., et al. (2015). The opportunistic marine pathogen Vibrio parahaemolyticus becomes virulent by acquiring a plasmid that expresses a deadly toxin. Proc. Natl. Acad. Sci. U.S.A. 112, 10798–10803. doi: 10.1073/pnas.1503129112
Leung, T. L. F., and Bates, A. E. (2013). More rapid and severe disease outbreaks for aquaculture at the tropics: implications for food security. J. Appl. Ecol. 50, 215–222. doi: 10.1111/1365-2644.12017
Lightner, D. V., Redman, R. M., Pantoja, C. R., Noble, B. L., Nunan, L. M., Tran, L., et al. (2013). “Documentation of an emerging disease (early mortality syndrome) in SE Asia & Mexico Spread of EMS/AHPND in East and SE Asia,” in Proceedings of the Department of Veterinary Science Microbiology School Animal Comp. Biomedical Science University of Arizona, Tucson, AZ.
Liu, C. H., and Chen, J. C. (2004). Effect of ammonia on the immune response of white shrimp Litopenaeus vannamei and its susceptibility to Vibrio alginolyticus. Fish Shellf. Immunol. 16, 321–334. doi: 10.1016/S1050-4648(03)00113-X
Liu, G., Zhu, S., Liu, D., Guo, X., and Ye, Z. (2017). Effects of stocking density of the white shrimp Litopenaeus vannamei (Boone) on immunities, antioxidant status, and resistance against Vibrio harveyi in a biofloc system. Fish Shellf. Immunol. 67, 19–26. doi: 10.1016/j.fsi.2017.05.038
Livak, K. J., and Schmittgen, T. D. (2001). Analysis of relative gene expression data using real-time quantitative PCR and the 2-ΔΔCT method. Methods 25, 402–408. doi: 10.1006/meth.2001.1262
Luis-villaseñor, I. E., Voltolina, D., Audelo-naranjo, J. M., Pacheco-marges, M. R., Herrera-espericueta, V. E., and Romero-beltrán, E. (2016). Effects of biofloc promotion on water quality, growth, biomass yield and heterotrophic community in Litopenaeus vannamei (Boone, 1931) experimental intensive culture. Ital. J. Anim. Sci. 14:3726. doi: 10.4081/ijas.2015.3726
Mancilla, E., Palacios-Morales, C. A., Córdova-Aguilar, M. S., Trujillo-Roldán, M. A., Ascanio, G., and Zenit, R. (2015). A hydrodynamic description of the flow behavior in shaken flasks. Biochem. Eng. J. 99, 61–66. doi: 10.1016/j.bej.2015.03.003
Mishra, J. K., Samocha, T. M., Patnaik, S., Speed, M., Gandy, R. L., and Ali, A. M. (2008). Performance of an intensive nursery system for the Pacific white shrimp, Litopenaeus vannamei, under limited discharge condition. Aquac. Eng. 38, 2–15. doi: 10.1016/j.aquaeng.2007.10.003
Nunan, L., Lightner, D., Pantoja, C., and Gomez-Jimenez, S. (2014). Detection of acute hepatopancreatic necrosis disease (AHPND) in Mexico. Dis. Aquat. Organ. 111, 81–86. doi: 10.3354/dao02776
Pfaffl, M. W. (2002). Relative expression software tool (REST(C)) for group-wise comparison and statistical analysis of relative expression results in real-time PCR. Nucleic Acids Res. 30:36. doi: 10.1093/nar/30.9.e36
Roy, S., Bossier, P., Norouzitallab, P., and Vanrompay, D. (2020). Trained immunity and perspectives for shrimp aquaculture. Rev. Aquac. 1–20. doi: 10.1111/raq.12438
Roy, S., Kumar, V., Bossier, P., Norouzitallab, P., and Vanrompay, D. (2019). Phloroglucinol treatment induces transgenerational epigenetic inherited resistance against Vibrio infections and thermal stress in a brine shrimp (Artemia franciscana) model. Front. Immunol. 10:2745. doi: 10.3389/fimmu.2019.02745
Roy, S., Kumar, V., Mitra, A., Manna, R. K., Suresh, V. R., and Homechaudhuri, S. (2018). Amylase and protease activity in shrimps and prawn of sundarbans, west bengal, india. Indian J. Geo Mar. Sci. 47, 53–59.
Ruwandeepika, H. A. D., Karunasagar, I., Bossier, P., and Defoirdt, T. (2015). Expression and quorum sensing regulation of type III secretion system genes of Vibrio harveyi during infection of gnotobiotic brine shrimp. PLoS One 10:e0143935. doi: 10.1371/journal.pone.0143935
Sajali, U. S. B. A., Atkinson, N. L., Desbois, A. P., Little, D. C., Murray, F. J., and Shinn, A. P. (2019). Prophylactic properties of bio fl oc- or Nile tilapia-conditioned water against Vibrio parahaemolyticus infection of whiteleg shrimp (Penaeus vannamei). Aquaculture 498, 496–502. doi: 10.1016/j.aquaculture.2018.09.002
Shinn, A. P., Pratoomyot, J., Griffiths, D., Jiravanichpaisal, J., and Briggs, M. (2018). Asian shrimp production and the economic costs of disease. Asian Fish. Sci. J. 31S, 29–58.
Tran, L., Nunan, L., Redman, R. M., Mohney, L. L., Pantoja, C. R., Fitzsimmons, K., et al. (2013). Determination of the infectious nature of the agent of acute hepatopancreatic necrosis syndrome affecting penaeid shrimp. Dis. Aquat. Organ. 105, 45–55. doi: 10.3354/dao02621
Trichet, V. V. (2010). Nutrition and immunity: an update. Aquac. Res. 41, 356–372. doi: 10.1111/j.1365-2109.2009.02374.x
Wasielesky, W., Atwood, H., Stokes, A., and Browdy, C. L. (2006). Effect of natural production in a zero exchange suspended microbial floc based super-intensive culture system for white shrimp Litopenaeus vannamei. Aquaculture 258, 396–403. doi: 10.1016/j.aquaculture.2006.04.030
Watnick, P. (2000). Biofilm, city of microbes. J. Bacteriol. 182, 2675–2679. doi: 10.1128/jb.182.10.2675-2679.2000
Wickins, J. F. (1976). The tolerance of warm-water prawns to recirculated water. Aquaculture 9, 19–37. doi: 10.1016/0044-8486(76)90045-4
Xu, W. J., and Pan, L. Q. (2013). Enhancement of immune response and antioxidant status of Litopenaeus vannamei juvenile in biofloc-based culture tanks manipulating high C/N ratio of feed input. Aquaculture 412-413, 117–124. doi: 10.1016/j.aquaculture.2013.07.017
Yang, Q., and Defoirdt, T. (2015). Quorum sensing positively regulates flagellar motility in pathogenic Vibrio harveyi. Environ. Microbiol. 17, 960–968. doi: 10.1111/1462-2920.12420
Keywords: biofloc system, Litopenaeus vannamei, Vibrio parahaemolyticus, AHPND, phenotype switching
Citation: Kumar V, Wille M, Lourenço TM and Bossier P (2020) Biofloc-Based Enhanced Survival of Litopenaeus vannamei Upon AHPND-Causing Vibrio parahaemolyticus Challenge Is Partially Mediated by Reduced Expression of Its Virulence Genes. Front. Microbiol. 11:1270. doi: 10.3389/fmicb.2020.01270
Received: 14 January 2020; Accepted: 19 May 2020;
Published: 24 June 2020.
Edited by:
Jennifer M. Ritchie, University of Surrey, United KingdomReviewed by:
Chu-Fang Lo, National Cheng Kung University, TaiwanCopyright © 2020 Kumar, Wille, Lourenço and Bossier. This is an open-access article distributed under the terms of the Creative Commons Attribution License (CC BY). The use, distribution or reproduction in other forums is permitted, provided the original author(s) and the copyright owner(s) are credited and that the original publication in this journal is cited, in accordance with accepted academic practice. No use, distribution or reproduction is permitted which does not comply with these terms.
*Correspondence: Vikash, VmlrYXNoLkt1bWFyQHVnZW50LmJl
Disclaimer: All claims expressed in this article are solely those of the authors and do not necessarily represent those of their affiliated organizations, or those of the publisher, the editors and the reviewers. Any product that may be evaluated in this article or claim that may be made by its manufacturer is not guaranteed or endorsed by the publisher.
Research integrity at Frontiers
Learn more about the work of our research integrity team to safeguard the quality of each article we publish.