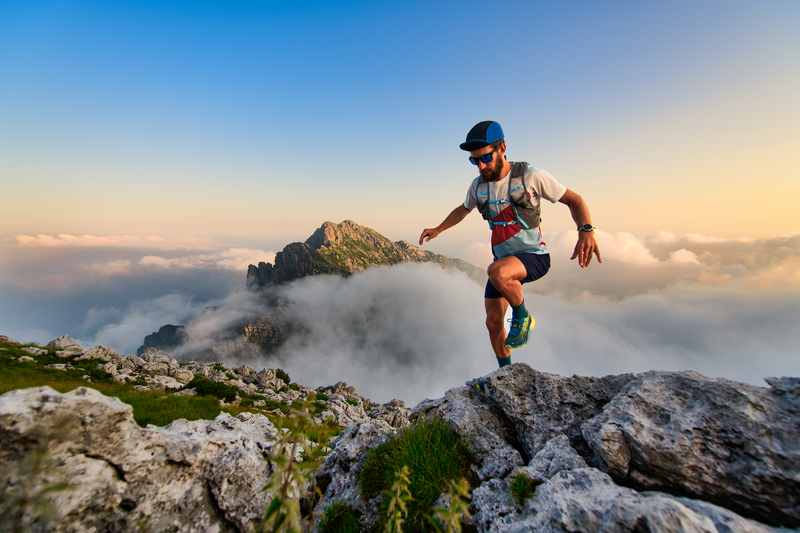
95% of researchers rate our articles as excellent or good
Learn more about the work of our research integrity team to safeguard the quality of each article we publish.
Find out more
ORIGINAL RESEARCH article
Front. Microbiol. , 26 June 2020
Sec. Microbial Physiology and Metabolism
Volume 11 - 2020 | https://doi.org/10.3389/fmicb.2020.01268
This article is part of the Research Topic What Is known and What Remains To Be Discovered About Bacterial Outer Membrane Vesicles View all 18 articles
Outer membrane vesicles (OMVs), produced by nonpathogenic Gram-negative bacteria, have potentially useful biotechnological applications in extraterrestrial extreme environments. However, their biological effects under the impact of various stressors have to be elucidated for safety reasons. In the spaceflight experiment, model biofilm kombucha microbial community (KMC) samples, in which Komagataeibacter intermedius was a dominant community-member, were exposed under simulated Martian factors (i.e., pressure, atmosphere, and UV-illumination) outside the International Space Station (ISS) for 1.5 years. In this study, we have determined that OMVs from post-flight K. intermedius displayed changes in membrane composition, depending on the location of the samples and some other factors. Membrane lipids such as sterols, fatty acids (FAs), and phospholipids (PLs) were modulated under the Mars-like stressors, and saturated FAs, as well as both short-chain saturated and trans FAs, appeared in the membranes of OMVs shed by both post-UV-illuminated and “dark” bacteria. The relative content of zwitterionic and anionic PLs changed, producing a change in surface properties of outer membranes, thereby resulting in a loss of interaction capability with polynucleotides. The changed composition of membranes promoted a bigger OMV size, which correlated with changes of OMV fitness. Biochemical characterization of the membrane-associated enzymes revealed an increase in their activity (DNAse, dehydrogenase) compared to wild type. Other functional membrane-associated capabilities of OMVs (e.g., proton accumulation, interaction with linear DNA, or synaptosomes) were also altered after exposure to the spaceflight stressors. Despite alterations in membranes, vesicles did not acquire endotoxicity, cytotoxicity, and neurotoxicity. Altogether, our results show that OMVs, originating from rationally selected nonpathogenic Gram-negative bacteria, can be considered as candidates in the design of postbiotics or edible mucosal vaccines for in situ production in extreme environment. Furthermore, these OMVs could also be used as promising delivery vectors for applications in Astromedicine.
Different populations of outer membrane vesicles (OMVs) produced by Gram-negative bacteria are naturally enriched with lipopolysaccharides (LPSs), nucleic acids, lipids, and proteins. Such contents endow vesicles with distinct capabilities for interacting with surrounding biopolymers, bacteriophages, cells, or organisms (Schwechheimer and Kuehn, 2015; Toyofuku et al., 2019). OMVs of Gram-negative bacteria have several functions related to intercellular communications and signaling events (Ahmadi Badi et al., 2017) that include transfer of enzymes and antimicrobials (Schulz et al., 2018), immunomodulation (Cañas et al., 2018; Ahmadi Badi et al., 2019; Ladinsky et al., 2019; Molina-Tijeras et al., 2019), contribution to intestinal homeostasis (Patten et al., 2017; Cecil et al., 2019), and epigenetic processes (Celluzzi and Masotti, 2016; Vdovikova et al., 2018). Multifunctionality of OMVs endow bacteria the capability to withstand changed or harsh environment, and this phenomenon has attracted research interests in elucidating adaptation mechanisms under stress-related conditions. McBroom and Kuehn (2007) showed that vesiculation is an independent stress response that mobilizes bacterial populations for survival via alterations in their cell membranes that enhance the export of damaged polymer products under environmental stress.
Spaceflight-associated and simulated Mars-like stressors such as radiation, changed gravity and atmosphere, and temperature fluctuations can be challenging for the survival of microorganisms, and a complex mixture of these extraterrestrial factors promotes global genomic, proteomic, and secondary metabolomic changes that result in impaired cellular processes and functions, affecting cell growth, cell morphology and development, virulence and resistance, and biofilm formation (Huang et al., 2018; Zea et al., 2018; de Vera et al., 2019; Romsdahl et al., 2019). Microorganisms evolve different response and adaptation mechanisms to extreme environments; however, many of them are still unknown. Biological effects of OMVs shed by nonpathogenic Gram-negative bacteria under stressful conditions remain to be elucidated from the point of view of their practical application in extraterrestrial settlements. Under space exploration, microorganisms can produce many special secondary metabolites that could be fabricated (Camere and Karana, 2018) and used as medicine for both humans and animals (e.g., antibiotics, vaccines; Huang et al., 2018). An issue of practical significance for such extracellular vesicles (EVs) is the evaluation of possible risks in terms of safety of modified vesicles with alternated functionality under the effects of various stressors.
A growing body of preclinical and clinical evidence supports the concept of bidirectional microbiota-gut-brain interactions (Mohajeri et al., 2018; Toribio-Mateas, 2018; Mohajeri, 2019), in which OMVs are active players (Choi et al., 2019; Ladinsky et al., 2019). In this context, the maintenance of healthy gut microbiota during the interplanetary journeys, e.g., to Mars, will be a challenge in the near future. During a spaceflight, the lifestyle (diet, low physical activities, and antibiotic usage, etc.) of crew-members, sterility of spaceship confined systems, and environmental stresses (radiation, changed gravity, etc.) result in microbiome alterations, mainly, in species richness, without any significant effects onto the species diversity (Iosim et al., 2019; Voorhies et al., 2019). On the other hand, spaceflight and associated stressors promote changes in gut bacterial physiology (Nickerson et al., 2004; Wilson et al., 2007; Morrison et al., 2019) that could potentially lead to an impaired immunity (Guéguinou et al., 2009; Crucian et al., 2015; Cervantes and Hong, 2016) and predisposition to illnesses among astronauts (Smith, 2018). This concern is heightened by the reported increase in virulence of pathogens in microgravity conditions, as well as a potentially increased risk of bacterial and viral infections (Mehta et al., 2014). Mitigating the adverse effects on astronauts’ health has been difficult and mostly included palliative care, which involves an integrated approach or some medications (Iosim et al., 2019). In the future, during the spaceflight, astronauts may be given cellular therapy for improving their response to spaceflight and helping to ameliorate known hazards (e.g., radiation; Iosim et al., 2019; Schäfer, 2019). While the safety of cell therapy application under extreme conditions is yet to be proven, noncellular EV-based therapy can serve as a safe substitute. The restoration of normal microbial community during a spaceflight may be facilitated by mucosal vaccination, using OMVs of safe rationally selected bacteria or microbiome-targeted probiotics (Sakai et al., 2018; Gorreja, 2019). Protective mucosal immune responses are most effectively induced by mucosal immunization through oral, nasal, rectal, vaginal routes, or by injection (Neutra and Kozlowski, 2006). In spite of significant shifts, occurring in immune function pathways under spaceflight, vaccination is quite possible and T-cell response is expected to be adequate (Iosim et al., 2019). OMVs could also be potentially employed for treating stress-induced depression of crew members during spaceflight (Choi et al., 2019) or amelioration of metabolic dysfunctions (Ashrafian et al., 2019).
In the Biology and Mars Experiment (BIOMEX; de Vera et al., 2019), living kombucha microbial community (KMC; bacterial and yeast species, inhabiting the cellulose-based pellicle film) was exposed outside the International Space Station (ISS) under simulated Mars-like stressors with the purpose of finding out the extent of survival of the KMC-members, as well as to assess the safety of the returned microbial samples and their nanostructures. An increased pool of EVs, produced by the surviving post-flight KMC-members, was discovered and has been published recently (Podolich et al., 2019). The purpose of this study was to characterize the vesicleome of a key KMC bacterium – Komagataeibacter intermedius – exposed to space/Mars-like stressors. Additionally, we aimed to assess whether the populations of OMVs shed by the recovered bacterial strains show any changes in their fitness, and whether these alterations compromise in anyway the safety of the K. intermedius OMVs compared to the KMC metavesicleome (all the membrane vesicle populations shed by many individual microorganisms, KMC-members).
Dry organo-mineral samples, containing embedded KMC pellicle fragments, were placed in three positions (top, middle, and bottom) in carrier C2 in tray 2, which has a three-level architecture, each one hosting four kombucha samples (along with samples of other experiments) and maintained under a simulated Mars atmosphere (95.55% CO2, 2.70% N2, 1.60% Ar, 0.15% O2, and ~370 ppm H2O) and a pressure of 980 Pa (Supplementary Figure S1). The top level (tKMCs) samples were additionally exposed to a solar UV flux cutoff by optical filters to wavelengths of >200 nm as prevalent on the Martian surface, whereas the middle (mKMC) and bottom (bKMC) levels were kept in darkness (protected from UV radiation); however, in Mars-like atmosphere and pressure for reference. Returned KMC samples were cultivated for reactivation in a filter-sterilized black tea, as previously described (Podolich et al., 2017).
Kombucha culture of ecotype IMBG-1 was grown in filter-sterilized sugared (7%) black tea infusions at 28°C, for 3–5 days. The previously re-isolated strains of K. intermedius IMBG180 from initial KMC (iKMC), 183 (bKMC), 184 (mKMC), and 185 (tKMC; Podolich et al., 2019) were cultured in HS medium (Hestrin and Schramm, 1954) at 28°C, for 3 days. Bacillus subtilis IMBG132, Escherichia coli S-17 (obtained from Prof. A. Puehler, Germany), and Pseudomonas aeruginosa IMBG188 (the collection of Institute of Molecular Biology and Genetics, Kyiv, Ukraine) were cultured in LB medium (Miller, 1972) at 28°C, overnight for antimicrobial assays. E. coli DH5α (pTZ19R; Thermo Fisher Scientific, Lithuania) was grown overnight in LB using ampicillin (100 mg ml−1).
Reactivated kombucha planktonic culture (at the stage when the pellicle appeared) or K. intermedius cultures were centrifuged at 17,000 rpm for 20 min at 4°C. The supernatants were collected and further ultra-centrifuged at 100,000 × g for 1 h at 4°C (Beckman Instruments Inc., L8M, rotor 55.2 Ti). The resulting pellets were resuspended in sterile filtered (0.10 μ pore size filter; Minisart, Sartorius, Germany) phosphate-buffered saline (PBS). Before visualization, fresh vesicle preparations were filtered (0.20 μ). The isolated vesicle preparations were estimated by the microvolume protein content determination method using NanoDrop ND-1000 spectrophotometer (NanoDrop Technologies, Wilmington, DE). Visualization of isolated vesicles was performed using transmission electron microscopy (TEM) and scanning electron microscopy, and the relative size of vesicles was estimated by dynamic light scattering (DLS), as previously described (Podolich et al., 2019). Zeta potential determination was performed on a Zetasizer Nano S (Malvern, UK) at 25°C. Colloids were irradiated with helium-neon laser with λ = 633 nm, and the scattered light was recorded at an angle of 173°.
In this study, we adopted the following scientific community terms: EVs for vesicles produced by KMC-members (bacteria and yeasts) and OMV for Gram-negative bacterium K. intermedius.
DNAse I (final concentration of 1 μg/μl, Thermo Fisher Scientific, Lithuania) was added to the OMV samples and incubated at 37°C, for 30 min. A 50 mM EDTA solution was used for inactivation of DNAse I at 65°C, for 10 min. Total DNA from K. intermedius OMVs was isolated with the PowerSoil DNA Isolation Kit (MO BIO Laboratories, USA).
Total RNA from the OMVs was isolated with an RNA Clean & Concentrator kit (Zymo Research Corp., USA). In separate experiments before RNA extraction, the OMVs were treated with RNase A (final concentration of 1 μg/μl, Thermo Fisher Scientific, Lithuania) for 30 min, at 37°C and frozen for 15 min, at −20°C, to stop RNase before vesicle lysis. RNA integrity was validated by the microchip electrophoresis system (MCE-202/MultiNA SHIMADZU, Japan).
Total lipids were extracted from vesicle samples as recommended (Bligh and Dyer, 1959; Palmer, 1971). The lipid extracts were separated by thin-layer chromatography on standard plates, using a solvent system of hexane: diethyl ether:glacial acetic acid (85:15:1, by vol.). The total phospholipid (PL) content was determined, as previously described (Vaskovsky et al., 1975). The Carlo Erba HRGC 5300 gas chromatograph (Italy) with flame ionization detector equipped with a glass packed column (length: 3.5 m, internal diameter: 3.0 mm) completed with 10% SP-2300 phase (Silar 5CP) on Chromosorb W/HP was used to separate and identify FA methyl esters. The temperature was programmed from 140 to 250°C, at 2°C/min with a final hold. The Agilent Technologies 7890A gas chromatograph (USA) with SP-2560 column (100 m × 0.25 mm, df 0.20 μm, Germany) was used to separate and identify cis/trans isomers of unsaturated FA methyl esters. The temperature was programmed from 140 to 240°C, at 4°C/min with a final hold. Individual FAs in samples were identified on the basis of their retention time compared to appropriate commercially available standards (Sigma-Aldrich, USA; Serva, Germany). Results were expressed as percentage of total FAs. All the experiments were repeated three times.
The interaction of the OMVs with plasmid pTZ19R DNA EcoRI-linearized form (Fermentas, Lithuania) was indicated by a slower electrophoretic mobility of their complexes in the agarose gel, as described in Podolich et al. (2019).
The vesicle suspensions (0.1 mg ml−1 final protein concentration) in the PBS supplemented with 2 mM CaCl2 were pre-incubated for 10 min, at 37°C, and then transferred to a stirred cuvette thermostat at 37°C. The recording was initiated from the time of the addition of acridine orange to the final concentration of 5 μM. Fluorescence changes were measured using a Hitachi MPF-4 spectrofluorometer at the excitation and emission wavelengths of 490 and 530 nm, respectively.
Тhe MTT [a tetrazolium salt, 3-(4,5-dimethyl-thiazoyl-2-yl)-2,5-diphenyltetrazolium bromide] assay (Mosmann, 1983) was used with some modifications. In brief, a sample of 0.1 ml of OMVs (4 mg ml−1 in protein) in PBS was incubated with 10 μl of 2 mg ml−1 MTT solution (in PBS) for 2.5 h, at 37°C. After incubation, the samples were centrifuged at 8,000 × g for 2 min. The formazan pellet was dissolved in 10 μl dimethyl sulfoxide (DMSO). Optical absorbance of the converted dye was measured at 570 nm. The molar extinction coefficient for MTT-formazan in DMSO, needed to calculate its content in the samples, was 1.35 × 104 M−1 cm−1. The results were converted into mM of produced formazan mg−1 protein min−1.
Antimicrobial effects of both OMVs and KMC EV preparations on model living bacteria were determined by the spot test method, using minimal vesicle concentration that generated clear halos on a bacterial lawn. The grown lawn of target cells (B. subtilis, E. coli, P. aeruginosa) was coated with 20 μl of vesicle preparation (2 mg ml−1 of the total protein). The agar plates were incubated at 30°C, overnight. Antimicrobial activity of vesicles was indicated by the appearance of clear zones at the site of vesicle sample application. A sterile PBS was used as a negative control.
Plasmid pTZ19R DNA from E. coli DH5α was isolated with the innuPREP Plasmid Mini Kit 2.0 (Analytik Jena AG, Germany). OMVs (5 mg ml−1) were suspended in PBS (pH 7.4), filtered (0.20 μ), and added to 300 ng of plasmid pTZ19R DNA (Fermentas, Lithuania), and a final reaction mixture (20 ml) was incubated for 16 h at 37°C in the DEPC-treated water. After incubation, the samples were separated by electrophoresis in 1.2% agarose gel in Tris acetate-EDTA buffer (pH 7.0). The stained gels were visualized under the UV-light.
Murine embryonic fibroblast (MEF) cells were obtained from muscles of the buttocks of a 17-day murine embryo, as described in Jozefczuk et al. (2012) and cultured in DMЕМ-F12 (Biowest, USA) supplemented with the Biowest Antibiotic-Antimycotic solution (2.0 μl/ml). Human colon carcinoma cells COLO 205 (Sigma-Aldrich, USA) were cultured in RPMI 1640 (Sigma-Aldrich, USA) with heat-inactivated fetal bovine serum (10%), 2 mМ ʟ-glutamine, and gentamicin (40 μg/ml) added. Cultures were incubated at 37°C in a humidified atmosphere of 5% CO2/95% air in 100 ml flasks.
Isolated OMVs were labeled with DiO (3,3′-dioctadecyloxacarbocyanine perchlorate; Thermo Fisher Scientific, USA), a fluorescent lipophilic stain, with 20 μg/ml. Nuclei were stained with 0.4 μg/ml DAPI (4′,6-diamidine-2′-phenylindole dihydrochloride; Sigma-Aldrich, USA), and imaging was performed using LEICA DM1000 microscope (100× objective lens).
MEF or COLO 205 cells were harvested by centrifugation and suspended at lg5 cells/ml in complete medium. Fifty-microliter aliquots of the cell suspension were transferred in duplicate to the 96-well microtiter plate, containing diluted samples (total volume, 100 μl). As a positive control, amitozyn (1 mg ml−1; Hermant et al., 2013) kindly provided by Dr. A. Potopalsky (IMBG, Kyiv) was used, and as a negative control, PBS was used. Cytotoxicity test was performed using the MTT reduction assay (Mosmann, 1983). The optical density of each well was measured with a microplate spectrophotometer (Multiscan Tirertek MMC 340, USA) equipped with a 540 nm filter.
Biological activity of OMV-associated LPS was quantified by the end-point chromogenic Limulus amebocyte lysate (LAL) assay, using Pierce™ LAL Chromogenic Endotoxin Quantitation Kit (Thermo Fisher Scientific, USA). The optical density of each sample was measured at 405 nm by NanoDrop ND-1000 spectrophotometer (NanoDrop Technologies, USA). Endotoxin concentration in test samples was calculated using the E. coli Endotoxin Standard (011:B4) for calibration.
Experiments on neurotoxicity risk assessment have been performed at the neurochemical level of the nervous system organization and involved an analysis of radiolabeled glutamate transport characteristics, which is a key excitatory neurotransmitter in the central nervous system, using synaptosomes (according to Guidelines for Neurotoxicity Risk Assessment of US Environmental Protection Agency, 1998, based on paragraph 3. Hazard Characterization: 3.1.3.4. In Vitro Data in Neurotoxicology; US Environmental Protection Agency, 1998). Synaptosomes’ preparation from a rat brain and measurements of the ambient level of ʟ-[14C] glutamate in the preparations of nerve terminals were conducted, as previously described (Borisova et al., 2016). Briefly, the synaptosomal suspensions (125 μl, 0.5 mg of protein/ml) were pre-incubated at 37°C, for 10 min, and the OMVs (70 μg of protein/ml) were added and incubated for 7 min, following sedimentation (10,000 g, 20 s). The release value was measured in the aliquots of the supernatants (100 μl) and in the pellets by liquid scintillation counting with scintillation cocktail (1.5 ml). The results were expressed in nMol of ʟ-[14C] glutamate/mg of protein.
Pregnant ICR mice (body weight 19–23 g) and Wistar rats (body weight 100–120 g) were maintained in special animal facilities at the Institute of Molecular Biology and Genetics and the Palladin Institute of Biochemistry of NAS in Ukraine, respectively. Animals were housed in quiet, temperature-controlled rooms (22–23°C) with a 12 h light:12 h dark cycle (lights on between 08:00 and 20:00 h). The animals were provided with purified water and dry food pellets ad libitum. The experimental procedures were carried out according to the standard ethical guidelines (European Community Guidelines on the Care and Use of Laboratory Animals 86/609/EEC) and approved by Institutes’ Ethics Committees.
Results were expressed as mean ± standard deviation (SD) of independent experiments performed in triplicate. The difference between groups was compared by two-tailed Student’s t-test. The differences were considered to be significant when p ≤ 0.05.
Cellulose-synthesizing K. intermedius constitutes more than 90% of prokaryotic microbes of the kombucha community (Podolich et al., 2019), and it is a carrier of LPS without O-antigen–endotoxin–integrated into the outer membrane (Barja et al., 2016). Previously, K. intermedius were re-isolated from the returned and reactivated space-exposed samples and identified using the 16S rDNA as a phylogenetic marker (Podolich et al., 2019). These strains were used in this study.
Bacterial OMV samples analyzed by DLS displayed larger average sizes (IMBG185: 70.31 ± 0.5 nm; IMBG184: 78.33 ± 1.3 nm; IMBG183: 66.41 ± 0.2 nm; Supplementary Figure S2), compared to OMVs from ground-based bacteria (IMBG180: 65.81 ± 0.3 nm). The DLS data were in agreement with the data obtained from the SEM and TEM micrographs of OMVs (Figures 1A–F). Furthermore, vesicles originating from the isolates of K. intermedius had partially deformed membranes as shown on both the scanning electron microscopy and TEM micrographs in Figures 1B,E.
Figure 1. Outer membrane vesicles (OMVs) of the key kombucha microbial community (KMC)-member Komagataeibacter intermedius recovered from the KMC samples exposed to the space/Mars-like stressors outside the International Space Station (ISS) and their interaction with a linear plasmid DNA. Scanning electron micrographs of OMVs of reference K. intermedius IMBG180 (A); OMVs of K. intermedius IMBG185 from KMC exposed at the top level of the carrier on the exposure platform (tKMC) (B); OMVs of K. intermedius IMBG183 from KMC exposed at the bottom level of the carrier on the exposure platform (bKMC) (C); transmission electron micrographs of OMVs with one‐ and double-bilayer membranes (indicated with an arrow) derived from initial reference K. intermedius IMBG180 (D); IMBG185, in the inset, outer-inner membrane vesicle is shown (E); IMBG183 (F). Scale bars are 50–100 nm. (G) Effect of interaction of the EcoRI-linearized plasmid pTZ19R (Fermentas, Lithuania) with OMVs of K. intermedius from the initial kombucha microbial culture (KMC) (I) and KMCs exposed to Mars-like conditions, in LEO: a top level (II), a bottom level (III), and a middle level (IV). Lane C is the reference EcoRI-linearized plasmid pTZ19R. Lanes 1–6 are various concentrations of OMVs (1.25–0.25 mg ml−1).
Gram-negative bacterium K. intermedius IMBG180 releases conventional one-bilayer OMVs and, to a lesser extent, outer-inner membrane vesicles (characterized by the protrusion of both outer and plasma membranes; Figure 1D). The same pattern was visible in vesicles produced by K. intermedius strains recovered from KMCs exposed to space/Mars-like stressors (inset of Figure 1E). In OMVs, only the periplasm is entrapped while in more complex vesicles, with a double-bilayer structure, cytoplasmic content can also be entrapped, including such cellular components as DNA, RNAs, enzymes, and other metabolites. Exogenous DNA was detected in all OMV preparations, including OMVs from a reference strain, by staining with the fluorogenic DNA-binding dye DAPI (data not shown). When intact OMVs were treated with DNAse I, the DNA in the OMV preparations was digested by the enzyme. It indicates that DNA molecules were located on the vesicle surface. Although the presence of a small size RNA (42 nt) was detected with the Agilent 2100 Bioanalyzer (Thermo Scientific; data not shown), the isolation of RNA from OMVs after the treatment with RNase A did not yield detectable material. Post-stress K. intermedius OMVs had a negative charge; however, their zeta potential changed from −5.5 (OMVs/iKMC) to −1.5 mV (OMVs/tKMC).
Changes in the post-stress K. intermedius OMVs’ membrane surface charge can be reflected in the OMV interactions with biopolymers, e.g., polynucleotides. Electrophoresis mobility shift assay (EMSA) is a rapid and sensitive method to detect nucleic acid complexation with polymers, e.g., membrane proteins (Garner and Revzin, 1986). The electrophoretic patterns of the linearized plasmid pTZ19R DNA showed the concentration-dependent formation of molecular complexes with OMVs from non-exposed strain, which slowed their movement in the gel, and did not exhibit the mobility shifts after incubation with vesicles originated from K. intermedius isolated from the exposed KMCs (Figure 1G).
As OMVs are derived from outer membranes via budding, they carry information about their composition. By studying the membrane lipid profiles in post-stress K. intermedius OMVs and comparing them with the profile characterizing non-extreme conditions, we aimed to determine the response of bacterial lipids to simulated Martian stressors. Compositional analysis of membrane lipids revealed that vesicles of all K. intermedius strains consisted mostly of the following classes of lipid molecules: PLs, triglycerides, FAs, and sterols. Similarly, across all the strains, several minor lipid components of the OMVs such as 1,3-diglycerides, sterol ethers, and monoglycerides were also revealed (Figures 2A,B).
Figure 2. Classes of lipids of OMVs of bacterial monocultures Komagataeibacter intermedius (A,B); alterations in the lipid content in OMVs of K. intermedius strains recovered from post-spaceflight kombucha microbial cultures (KMC) (C,D). (A) Representative thin layer chromatography separation of lipids isolated from OMVs of K. intermedius IMBG185 recovered from the top level of the exposure platform (on the left); on the right–lipid markers (M): 1 – phospholipids (PLs); 2 – sterols; 3 – 1,3-diglycerides; 4 – free fatty acids; 5 – triglycerides; 6 – sterol ethers. (B) Lipids of OMVs of initial K. intermedius IMBG180 (on the left); on the right–markers (M). (C) Content of total lipids in OMVs of K. intermedius strains of post-spaceflight KMCs. (D) PLs of OMVs of K. intermedius strains of post-spaceflight KMCs. (E) Content of fatty acids in OMVs of K. intermedius strains of post-spaceflight KMCs. Three replicates were used in the experiments on lipid content measurements. Data were shown as mean ± SD (n = 3). *p ≤ 0.05; **p ≤ 0.01.
The total yield of lipids (in milligram of protein) in the OMVs of K. intermedius re-isolated from all the tKMC, bKMC, and mKMC space samples increased, compared with the reference (Figure 2C). After the spaceflight experiment, the relative content of zwitterionic phosphatidylethanolamine, as well as anionic PL phosphatidic acid decreased while other anionic PLs like phosphatidylserine and lysine-phosphatidylcholine increased (Figure 2D and Supplementary Table S1), probably producing a change in surface properties of outer membranes, including their charge.
The free FA content of OMVs isolated from the K. intermedius/tKMC increased significantly, compared to the untreated control (Figure 2A). The quantity of total saturated FAs had a 1.2–2 fold (p < 0.05) increase while unsaturated FAs (mainly monounsaturated) decreased 7.5 fold (p < 0.05) in OMVs from K. intermedius/tKMC, in contrast to wild type K. intermedius OMVs/iKMC. The amount of unsaturated FAs–polyenes–was found to have increased by 1.2–1.3 fold (p < 0.05) in OMVs of re-isolates from mKMC and bKMC as compared to preparations of OMVs from the reference strain (Figure 2E and Supplementary Table S2). At the same time, it was established that the UV-illumination caused the appearance of short-chain saturated FAs (С6:0–С11:0) in the OMVs/tKMC and OMVs/mKMC and trans FAs – elaidic (18:1w9) and linolelaidic (18:2w6) – in the OMVs/tKMC.
Structure deviations in cell/OMV membranes may lead to changes in membrane permeability, and we assessed a proton gradient formation in OMVs of post-flight K. intermedius strains. A pH-sensitive fluorescent dye, acridine orange, is commonly used to detect acidification in the whole cells, isolated lysosomes, endocytotic granules, and synaptic vesicles (Zoccarato et al., 1999; Borisova, 2014). Acridine orange is easily permeable via the membranes as the unprotonated amine, and thereafter, it converts itself to the protonated form and is concentrated in the acidic compartments, where this dye dimerizes, changing the optical features (Zoccarato et al., 1999). As shown in Figure 3A, an addition of acridine orange dye to OMVs/K. intermedius/iKMC caused a partial quenching of the fluorescence signal due to an accumulation of the dye inside of the vesicles that indicated a definite vesicle acidification. In contrast, a treatment of OMVs/K. intermedius/tKMCs with acridine orange did not change the fluorescence intensity of the dye. OMVs of K. intermedius from other locations – medium and bottom levels – exhibited some capability to form proton gradient; however, it was weaker compared to K. intermedius OMVs from initial KMC.
Figure 3. Functionality of OMVs of K. intermedius, isolated from post-flight KMCs (A,B,D,E) and extracellular vesicles (EVs) isolated from the post-flight KMC samples (C). (A) OMVs acidification measured using a pH-sensitive fluorescent dye acridine orange. Typical recording of acidification of OMVs from K. intermedius isolated from initial KMC and KMCs located at the top, middle, and bottom levels of a carrier mounted outside the ISS. (B) Dehydrogenase activity of OMVs of K. intermedius re-isolated from different KMCs. Data were shown as mean ± SD (n = 3), *p ≤ 0.05; **p ≤ 0.01; ***p ≤ 0.001. (C) The image of Bacillus subtilis lawn spotted with EVs. Clear zones are seen in spots of EVs from initial KMC (4) and EVs from bottom-located KMC (2). No visible changes seen after spotting with EVs of top‐ (1) and middle-located KMC (3). (D) Inhibitory activity of OMVs from K. intermedius in regard to B. subtilis. (E) Electrophoregram of plasmid pTZ19R after incubation with OMVs of K. intermedius. M–DNA ladder (ThermoFisher Scientific GeneRuler DNA Ladder Mix). 1 – control DNA (without incubation); 2 – control DNA (incubation, 37°С); 3 – control DNA (+PBS, incubation, 37°С); 4 – OMVs of K. intermedius from initial KMC; 5–7 – OMVs of K. intermedius from bottom-, middle-, and top-located KMCs, incubated with DNA at 37°С.
Membrane leaflet is a harbor of bioactive molecules, including enzymes, and alterations in membrane lipid composition may lead to changes in its activities or its deprivation. We selected a set of membrane-associated enzymatic activities for OMVs/EVs (dehydrogenases, DNAse, hydrolyses) to study their activities associated with vesicles from exposed microorganisms.
Dehydrogenases are enzymes belonging to a group of oxidoreductases that oxidize the substrate by transferring protons and electrons through a chain of intermediate electron carriers [usually, Flavin adenine dinucleotide (FAD)‐ or pyrroloquinoline quinone (PQQ)-dependent in Gram-negative bacteria] to a final electron acceptor (Adachi et al., 2007). These activities have increased in all OMVs from post-exposed strains of K. intermedius: 1.6-fold (from tKMC), 3-fold (from mKMC), and 8.6-fold (from bKMC), when compared to a control sample from wt K. intermedius (Figure 3B).
Antimicrobial compounds and bacteriolytic enzymes, including autolysins carried by bacterial OMVs, are suggested to exert an inhibitory or killing effect on co-existing bacteria or fungi (Vasilyeva et al., 2013; Schulz et al., 2018). EVs from initial KMC had a weak lytic action on the B. subtilis lawn in the form of a translucent lysis zone (Figure 3C). A stronger lytic effect was seen as a clear visible zone of lysis at the site of application by EVs/bKMC. No clear spots were produced by EVs from tKMC and mKMC, or even a negative control. Nonetheless, in the case of Gram-negative bacterial species E. coli and P. aeruginosa, which have different membrane structures as compared to Gram-positive bacilli, the results were the opposite: EVs of KMCs supported growth of these cultures (data not shown) and probably were used as nutrients. The antimicrobial activity in relation to Gram-positive bacilli was less expressed in K. intermedius OMVs from exposed bacteria compared to EVs/bKMC and the wt OMVs (Figure 3D).
Nucleases perform different roles to fit bacterial lifestyle (Al-Wahaibi et al., 2019; Pressler et al., 2019). Extracellular nucleases are known as enzymes to accommodate invading bacteria in hosts via the formation of a set of diverse oligonucleotides with immunomodulatory properties (Takahashi et al., 2006) or help evade the innate immune response (Jhelum et al., 2018). Single-stranded specific deoxyribonuclease activity was detected on a formation of open-circular and linear plasmid DNA forms from a supercoiled form of pTZ19R by K. intermedius OMVs (Figure 3E, wells 5–7). Conversely, control OMVs had lower activity and formed only an open-circular form of the plasmid (Figure 3E, well 4).
As exposed Komagataeibacter possess LPS in their outer membrane, known as endotoxin, and as both this bacteria and KMC are promising probiotics, we examined their safety after a spaceflight, using vesicles as their representatives. First, OMVs were labeled with lipophilic dye DiO and introduced into MEF to show the vesicles interaction with murine cells. The cell interior of MEF showed the fluorescence of DiO labeled OMVs of both wt and space-exposed (UVC-illuminated) K. intermedius (Figure 4A, panels 1 and 3). Exposure of MEF cells to total vesicle preparation isolated just after revival of returned tKMC had a small inhibitory effect (15%) on the cell growth at concentration of 50 ng ml−1 (Figure 4C) compared to vesicles of iKMC. Nevertheless, this effect did not reach a threshold level (50%) and did not cause cytotoxicity in MEF. Co-incubation of the OMVs with cell culture COLO 205 did not exhibit cytotoxicity of the OMVs in both spaceflight-related and control OMVs (Figure 4D).
Figure 4. A biosafety assessment of EVs after exposure of kombucha multimicrobial culture (KMC) under space/Mars-like stressors outboard the ISS and OMVs of K. intermedius, isolated from post-flight KMCs. (A) A micrograph of OMVs stained with the lipophilic dye DiO visible in murine fibroblasts. Fibroblast nuclei were stained with DAPI. (1, 2) – OMVs of K. intermedius from initial KMC; (3, 4) – OMVs of K. intermedius from top-located KMC (tKMC). Scale bar, 10 μm. (B) The environment level of ʟ-[14C] glutamate in the preparations of rat brain nerve terminals after co-cultivation with EVs from post-flight KMCs. (C) Survival rate of murine embryo fibroblasts after co-cultivation with different concentrations of OMVs from K. intermedius isolated from tKMC. (D) Survival rate of colorectal carcinoma cells COLO 205 after co-cultivation with different concentrations of OMVs/K. intermedius, isolated from KMCs exposed outboard the ISS. (E) Endotoxin activity of EVs/KMCs detected with the Limulus amebocyte lysate (LAL) assay compared to standard endotoxin activity from Escherichia coli. (F) Endotoxin activity of OMVs/K. intermedius detected with the LAL assay. Data were shown as mean ± SD (n = 3), *p ≤ 0.05; **p ≤ 0.01; ***p ≤ 0.001.
EVs isolated from iKMC, representing the LPS-bearing fraction of Gram-negative bacteria (including K. intermedius), displayed 50-fold lower endotoxin activity level in the LAL assay compared to E. coli, as an endotoxin standard (Figure 4E). Levels of endotoxin activity in metavesicleomes from tKMC and mKMC were 18.4‐ and 5-fold higher, respectively, compared to the iKMC. Nonetheless, their levels were 21‐ and 6-fold lower, compared to E. coli. Endotoxin activity of OMVs of bacteria isolated from tKMC and mKMC exhibited a 2-fold increase compared to the OMVs from K. intermedius/iKMC, and it was less than 0.01% of the E. coli activity (Figure 4F). The level of endotoxin activity of OMVs/K. intermedius/tKMC was practically the same as in vesicles of the iKMC.
The ambient glutamate level between episodes of exocytosis is a crucial characteristic of synaptic neurotransmission and depends on numerous individual presynaptic parameters (Borisova, 2019). The total nanovesicle pool of revived post-flight KMCs did not increase the ambient level of ʟ-[14C] glutamate in the synaptosome suspensions in the rat brain nerve terminals, i.e., it did not acquire neuromodulation. The EVs of tKMC even decreased the ʟ-[14C] glutamate content, but EVs from KMC specimens, exposed at the middle and bottom levels, did not differ from the EVs of iKMC (Figure 4B).
Within the BIOMEX project, different extraterrestrial stressors subjected to different sample carrier layers had a distinct mode of action, and some of them were lethal to KMC-members, resulting in changes in the microbial community structure (Podolich et al., 2019). A key community member, the Gram-negative bacterium K. intermedius, has survived under harsh Mars-like radiation, atmosphere, and low gravity within the returned KMC samples. OMVs mirrored, to some extent, changes in their outer membrane structure, as well as membrane-associated activities of the K. intermedius re-isolates. An increase in both K. intermedius OMVs size and their number resulted from microbial adaptation to extraterrestrial stressors, apparently via cellular envelope remodeling, which is known from other Gram-negative bacteria exposed to stressful environmental or induced conditions (Sabra et al., 2003; Gerritzen et al., 2019; Klimentova et al., 2019). The increase in envelope thickness and production rate of OMVs with respect to the ground-based reference was previously demonstrated in the spaceflight samples of E. coli under microgravity (Zea et al., 2017).
The core lipids of K. intermedius, which serve as the framework for fully mature membrane lipids, are PLs, triglycerides, FAs, and sterols. Typical membrane parameters adversely affected by environmental challenges are permeability and fluidity, which, in turn, affect the function and mobility of membrane proteins, diffusion of nutrients, and energy sources (Rowlett et al., 2017; Siliakus et al., 2017; Jasim et al., 2018). In our study, the most striking changes were recorded in the membranes of OMVs from the UV-exposed K. intermedius, compared to the OMVs from K. intermedius/iKMC, and this correlated with increased synthesis of lipids, especially, PLs, free FAs, and sterols, which could cause alteration in membrane fluidity. Shortening of FA chain lengths occurred, probably by degrading the unfavorable membrane PLs that retained adequate membrane fluidity during the bacterial growth. Detected imbalance in saturated and unsaturated FAs and increased membrane condensation in cells/OMVs of K. intermedius/tKMC is an adaptive response to stressors that allow bacteria to limit metabolism, save energy, and survive under harsh conditions of Mars-like UV, simulated pressure, and atmosphere. Deformed membranes of the OMVs (tKMC), as shown in both the scanning electron microscopy and TEM micrographs, can be explained by membrane saturation leading to its rigidity. Additional strategies were also adopted by cells, such as maintenance of a changed membrane potential and development of a chemiosmotic barrier to protons. The polar PL head groups could also play a significant role in the maintenance of membrane fluidity and permeability. The membrane PLs diversity allows for different non-covalent forces, i.e., van der Waals, electrostatic, solvation (hydration and hydrophobic), steric, and entropic. Changes in the PL pattern led to alteration of the membrane surface properties, including charge, and this was reflected in the OMV interactions with biopolymers. For instance, modified membranes of vesicles could lose the capability to interact and form complexes with polynucleotides, in contrast to the reference OMVs, which interacted with plasmid DNA, resulting in the formation of supramolecular complexes or aggregates. Our data on the membrane lipid composition alterations are consistent with the previously reported membrane lipid modifications, including PL content, occurring under other stressful conditions in closely related acetic acid bacteria (Trcˇek et al., 2007; Ogawa et al., 2010) or other bacteria (Pei et al., 2019).
The capability to form proton gradient of post-flight OMVs was weaker, as compared to the OMVs of initial untreated strain. The bacterial membrane controls the traffic of various molecules into and out of the cell and also participates in signal transduction and chemical processing of incoming molecular species. The outer membrane contains porins involved in the influx of various compounds in bacteria (Masi et al., 2019), and membrane H+-ATPase, which performs secondary active transport processes across the membrane, energizing the membrane and forming an electrochemical gradient of protons (Turina et al., 2006). K. intermedius OMVs from KMC, exposed at upper unprotected level of the sample carrier, had very low capacity to build the proton gradient. It should be noted that UV can act as a trigger for modulation of membrane structure modification, leading to either loss of implicated proteins or their suppression (Ghorbal et al., 2019). To sum up, Mars-like illumination, gravity, and atmosphere influenced outer membrane alterations, and UV, as a stressful factor, was the most pronounced for K. intermedius. Usually, UV is detrimental for microorganisms and even fatal, especially when UV-C induces bacterial death by targeting DNA (Dai et al., 2012). Bacterial exposure to sublethal UV doses leads to membrane PL bilayer rearrangement and pore formation (Smith et al., 2009), inducing changes in the amounts and composition of FAs (Khefacha et al., 2015) and affecting LPSs (Chourabi et al., 2017). These modifications caused by UV lead to altered membrane permeability (Santos et al., 2013). Under stressful conditions, bacterial adaptation, regarded as a stress response to the physicochemical change, occurs and aids in the survival of bacterial cell populations via physiological membrane adaptations (Siliakus et al., 2017).
Most of the structural changes occur in the membranes of unprotected microorganisms exposed to UV similar to Martian surface, translated in proton translocation, and interacted with synaptosomes. Other complex stressors affected exposed bacteria that were protected from UV. For example, the appearance of short-chain saturated FAs in membranes of bacteria/OMVs from middle level of exposure has been recorded. Comparative genomics of wild-type and post-stress K. intermedius revealed a reduction of genome in the UV-irradiated returned bacteria (manuscript in preparation). We hypothesize, that such changes may be related to multiple deletions.
In this study, we observed increased DNAse activity in OMVs of post-stress K. imtermedius from all levels of exposure (top, middle, and bottom) compared to ground reference. This phenomenon can be attributed to modifications in membrane structure, more specifically, in PL pattern, and, consequently, membrane charge. As OMVs of reference K. intermedius probably may change the topological form of the plasmid linear DNA, e.g., as under the influence of topoisomerase, leading to knotting or catenation of DNA (Portugal and Rodríguez-Campos, 1996), OMVs of post-stress bacteria did not change the spatial architecture of linear DNA and had target DNA more accessible. Enhanced DNAse activities observed in OMVs from post-spaceflight re-isolates correlated with a poor biofilm production in parental bacteria, when the activity of such nucleases was demonstrated to balance the extent of biofilm formation, and this is congruent with the previously published results by Sharma and Singh (2018). Nucleases also contribute to the lower bacterial transformation capability of different bacteria, preventing horizontal gene transfer (Binnenkade et al., 2018; Jhelum et al., 2018). The nuclease-associated OMVs of the dominant KMC K. intermedius can be represented as safeguards, keeping the essential genome stability in this microecosystem. This is the first step toward the understanding of enzymatic properties of DNAses associated with OMVs, affecting K. intermedius survival, genome stability, and cellulose-synthetic fitness in the planktonic and pellicle environment of kombucha multi-species community.
We also observed increased dehydrogenase(s) activity in the order top > middle > bottom of reference OMV samples. In acetic acid bacteria of the Komagataeibacter genus, membrane-bound dehydrogenases are mainly represented by PQQ-dependent alcohol dehydrogenase, and the glucose dehydrogenase that contains PQQ as the prosthetic group (Matsutani and Yakushi, 2018). PQQ-dependent dehydrogenases catalyze the oxidation of non-phosphorylated substrates and can be beneficial for detoxifying cellular toxic compounds. In some organisms, they are induced under energy stress, providing an auxiliary energy generation pathway (Tribelli et al., 2015). In other organisms, they play a role in maintaining the transmembrane proton gradient (Liu et al., 2018). In our study, the increase in total DH-activities of post-spaceflight K. intermedius OMVs probably mirrors the compensatory mechanism of impaired proton gradient formation: the less proton gradient is, the higher membrane-associated DH-activities are.
Nanovesicle preparations derived from wild ecotype of KMC showed a killing activity toward B. subtilis, in a context of known KMC antimicrobial properties (Chakravorty et al., 2019), probably due to the hydrolytic enzyme(s), destroying the cell wall of Gram-positive bacterium. On the other hand, total vesicles from UV-illuminated tKMC lost this activity. Notably, EVs from “dark” bKMC did not change antimicrobial activity toward bacilli. OMVs isolated from K. intermedius did not exhibit a lytic activity; however, inhibited the growth of bacilli while OMVs isolated from K. intermedius/tKMC exhibited lower inhibitory activity toward B. subtilis.
In order to summarize the results on enzymatic activities associated with K. intermedius OMVs, we suppose that, as long as membrane-associated enzymes used in this study belong to the integral outer membrane protein types (e.g., DNAse belong to the β-barrel superfamily of phospholipase D; Nelson and Frohman, 2015) and penetrate the peripheral regions of the lipid bilayer, an activation of their biological activity depends on the impact of stressors that can perturb the membrane. Alterations in cell membrane result in the promotion of conformational changes within the enzyme structural domains and in their activity (Johnson and Cornell, 1999). Alternatively, changes in the outer membrane structure may prevent enzyme integration and result in a loss of appropriate function.
Changes in the K. intermedius OMV membrane structure were vital for its communications not only with alien bacteria, but also with mammalian cell cultures. In this study, internalized OMVs interacted with MEF cells and human colorectal carcinoma cells but these communications did not result in acquisition of cytotoxicity. Despite occurring at low concentrations, the OMVs inhibited both murine and human cell proliferation. The metavesicleome of post-flight KMCs did not increase the ambient level of ʟ-[14C] glutamate in the synaptosome suspensions in the rat brain nerve terminals, i.e., it did not acquire neuromodulation, and the vesicles of tKMC even decreased the ʟ-[14C] glutamate content. Biological activity of the post-flight K. intermedius OMV-associated LPS was slightly increased; however, it was almost identical to the wt KMC/EVs LPS-activity. To sum up, we can conclude that, despite alterations in membranes after the impact of extraterrestrial and simulated stressors outside the ISS, K. intermedius OMVs did not acquire endotoxicity, cytotoxicity, and neuromodulation. Therefore, OMVs, originating from carefully selected nonpathogenic Gram-negative bacteria, can be considered as potential candidates in the design of postbiotics or edible mucosal vaccines for in situ production in extreme environments, besides being promising delivery vectors for applications in Astromedicine.
All datasets generated for this study are included in the article/Supplementary Material.
This study was carried out in accordance with the standard ethical guidelines (European Community Guidelines on the Care and Use of Laboratory Animals 86/609/EEC) and approved by the Ethics Committees of the Institute of Molecular Biology and Genetics and the Palladin Institute of Biochemistry of NAS in Ukraine.
OP, J-PV, and NK conceived and designed the experiments. NK, GZ, DB, and PG were responsible for drafting the article. IZ, OK, IO, OR, LP, LZ, TH, MG, VK, MS, and SS were involved in performing the experiments and TB, HK, DB, AG-N, VA, MK, and NK analyzed the data. All authors reviewed and approved the final version of the manuscript.
This study was supported by National Academy of Sciences of Ukraine (grant 47/2018).
MK, VK, and MS were employed by NanoMedTech LLC, Kyiv.
The remaining authors declare that the research was conducted in the absence of any commercial or financial relationships that could be construed as a potential conflict of interest.
Authors thank Ms. Rosa Suleimanova, Dr. V.M. Klimashevsky, Ms. Natalia Andryushyna, and Ms. Natalia Melnychuk for their excellent technical assistance.
The Supplementary Material for this article can be found online at: https://www.frontiersin.org/articles/10.3389/fmicb.2020.01268/full#supplementary-material.
Adachi, O., Ano, Y., Toyama, H., and Matsushita, K. (2007). “Biooxidation with PQQ‐ and FAD-dependent dehydrogenases” in Modern biooxidation: Enzymes, reactions and applications. eds. R. D. Schmid and V. B. Urlacher (Weinheim, Germany: WILEY-VCH Verlag GmbH & Co. KGaA), 1–41.
Ahmadi Badi, S., Khatami, S. H., Irani, S. H., and Siadat, S. D. (2019). Induction effects of Bacteroides fragilis derived outer membrane vesicles on toll like receptor 2, toll like receptor 4 genes expression and cytokines concentration in human intestinal epithelial cells. Cell J. 21, 57–61. doi: 10.22074/cellj.2019.5750
Ahmadi Badi, S., Moshiri, A., Fateh, A., Rahimi Jamnani, F., Sarshar, M., Vaziri, F., et al. (2017). Microbiota-derived extracellular vesicles as new systemic regulators. Front. Microbiol. 8:1610. doi: 10.3389/fmicb.2017.01610
Al-Wahaibi, A. S. M., Lapinska, E., Rajarajan, N., Dobretsov, S., Upstill-Goddard, R., and Burgess, J. G. (2019). Secretion of DNases by marine bacteria: a culture based and bioinformatics approach. Front. Microbiol. 10:969. doi: 10.3389/fmicb.2019.00969
Ashrafian, F., Shahriary, A., Behrouzi, A., Moradi, H. R., Keshavarz Azizi Raftar, S., Lari, A., et al. (2019). Akkermansia muciniphila-derived extracellular vesicles as a mucosal delivery vector for amelioration of obesity in mice. Front. Microbiol. 10:2155. doi: 10.3389/fmicb.2019.02155
Barja, F., Andrés-Barrao, C., Ortega Pérez, R., Cabello, E. M., and Chappuis, M. L. (2016). “Physiology of Komagataeibacter spp. during acetic acid fermentation” in Acetic acid bacteria. eds. K. Matsushita, H. Toyama, N. Tonouchi, and A. Okamoto-Kainuma (Tokyo: Springer).
Binnenkade, L., Kreienbaum, M., and Thormann, K. M. (2018). Characterization of ExeM, an extracellular nuclease of Shewanella oneidensis MR-1. Front. Microbiol. 9:1761. doi: 10.3389/fmicb.2018.01761
Bligh, E. G., and Dyer, W. J. (1959). A rapid method of total lipid extraction and purification. Can. J. Biochem. Physiol. 37, 911–917. doi: 10.1139/o59-099
Borisova, T. (2014). “The neurotoxic effects of heavy metals: alterations in acidification of synaptic vesicles and glutamate transport in brain nerve terminals” in Horizons in neuroscience research. Vol. 14. eds. A. Costa and E. Villalba (Hauppauge, NY: Nova Science Publisher, Inc.), 89–112.
Borisova, T. (2019). Perspective express assessment of neurotoxicity of particles of planetary and interstellar dust. NPJ Microgravity 5:2. doi: 10.1038/s41526-019-0062-7
Borisova, T., Borysov, A., Pastukhov, A., and Krisanova, N. (2016). Dynamic gradient of glutamate across the membrane: glutamate/aspartate-induced changes in the ambient level of ʟ-[14C] glutamate and d-[3H] aspartate in rat brain nerve terminals. Cell. Mol. Neurobiol. 36, 1229–1240. doi: 10.1007/s10571-015-0321-4
Camere, S., and Karana, E. (2018). Fabricating materials from living organisms: an emerging design practice. J. Clean. Prod. 186, 570–584. doi: 10.1016/j.jclepro.2018.03.081
Cañas, M. A., Fábrega, M. J., Giménez, R., Badia, J., and Baldomà, L. (2018). Outer membrane vesicles from probiotic and commensal Escherichia coli activate NOD1-mediated immune responses in intestinal epithelial cells. Front. Microbiol. 9:498. doi: 10.3389/fmicb.2018.00498
Cecil, J. D., Sirisaengtaksin, N., O’Brien-Simpson, N. M., and Krachler, A. M. (2019). Outer membrane vesicle-host cell interactions. Microbiol. Spectr. 7, 1–18. doi: 10.1128/microbiolspec.PSIB-0001-2018
Celluzzi, A., and Masotti, A. (2016). How our other genome controls our epigenome. Trends Microbiol. 24, 777–787. doi: 10.1016/j.tim.2016.05.005
Cervantes, J. L., and Hong, B. -L. (2016). Dysbiosis and immune dysregulation in outer space. Int. Rev. Immunol. 35, 67–82. doi: 10.3109/08830185.2015.1027821
Chakravorty, S., Bhattacharya, S., Bhattacharya, D., Sarkar, S., and Gachhui, R. (2019). “Kombucha: a promising functional beverage prepared from tea” in Non-alcoholic beverages, volume 6: The science of beverages. eds. A. M. Grumezescu and A. M. Holban (Woodhead Publishing, Elsevier Inc.), 285–327. doi: 10.1016/B978-0-12-815270-6.00010-4
Choi, J., Kim, Y. K., and Han, P. L. (2019). Vesicles derived from Lactobacillus plantarum increase BDNF expression in cultured hippocampal neurons and produce antidepressant-like effects in mice. Exp. Neurobiol. 28, 158–171. doi: 10.5607/en.2019.28.2.158
Chourabi, K., Campoy, S., Rodriguez, J. A., Kloula, S., Landoulsi, A., and Chatti, A. (2017). UV-C adaptation of Shigella: morphological, outer membrane proteins, secreted proteins, and lipopolysaccharides effects. Curr. Microbiol. 74:1261. doi: 10.1007/s00284-017-1311-1
Crucian, B., Stowe, R., Mehta, S., Quiriarte, H., Pierson, D., and Sams, C. (2015). Alterations in adaptive immunity persist during long-duration spaceflight. NPJ Microgravity 1:15013. doi: 10.1038/npjmgrav.2015.13
Dai, T., Vrahas, M. S., Murray, C. K., and Hamblin, M. R. (2012). Ultraviolet C irradiation: an alternative antimicrobial approach to localized infections? Expert Rev. Anti-Infect. Ther. 10, 185–195. doi: 10.1586/eri.11.166
de Vera, J. P., Alawi, M., Backhaus, T., Baqué, M., Billi, D., Böttger, U., et al. (2019). Limits of life and the habitability of Mars: the ESA Space Experiment BIOMEX on the ISS. Astrobiology 19, 145–157. doi: 10.1089/ast.2018.1897
Garner, M. M., and Revzin, A. (1986). The use of gel electrophoresis to detect and study nucleic acid-protein interactions. Trends Biochem. Sci. 11, 395–396. doi: 10.1016/0968-0004(86)90149-0
Gerritzen, M. J. H., Martens, D. E., Uittenbogaard, J. P., Uittenbogaard, J., Wijffels, R., and Stork, M. (2019). Sulfate depletion triggers overproduction of phospholipids and the release of outer membrane vesicles by Neisseria meningitidis. Sci. Rep. 9:4716. doi: 10.1038/s41598-019-41233-x
Ghorbal, S. K. B., Chourabi, K., Maalej, L., Ammar, A. B., Ouzari, H. -I., Hassen, A., et al. (2019). Pseudomonas aeruginosa swarmer cells adaptation toward UVc radiations. Front. Microbiol. 10:556. doi: 10.3389/fmicb.2019.00556
Gorreja, F. (2019). Gene expression changes as predictors of the immune-modulatory effects of probiotics: towards a better understanding of strain-disease specific interactions. NFS J. 14, 1–5. doi: 10.1016/j.nfs.2019.02.001
Guéguinou, N., Huin-Schohn, C., Bascove, M., Bueb, J. L., Tschirhart, E., Legrand-Frossi, C., et al. (2009). Could spaceflight-associated immune system weakening preclude the expansion of human presence beyond Earth’s orbit? J. Leukoc. Biol. 86, 1027–1038. doi: 10.1189/jlb.0309167
Hermant, B., Gudrun, A., Potopalsky, A. I., Chroboczek, J., and Tcherniuk, S. O. (2013). Amitozyn impairs chromosome segregation and induces apoptosis via mitotic checkpoint activation. PLoS One 8:e57461. doi: 10.1371/annotation/0b7ab40b-2246-4426-a98d-36b028029c5c
Hestrin, S., and Schramm, M. (1954). Synthesis of cellulose by Acetobacter xylinum. II. Preparation of freeze-dried cells capable of polymerizing glucose to cellulose. Biochem. J. 58, 345–352. doi: 10.1042/bj0580345
Huang, B., Li, D., Huang, Y., and Liu, C. -T. (2018). Effects of spaceflight and simulated microgravity on microbial growth and secondary metabolism. Mil. Med. Res. 5:18. doi: 10.1186/s40779-018-0162-9
Iosim, S., MacKay, M., Westover, C., and Mason, C. E. (2019). Translating current biomedical therapies to astronaut medicine. Precis. Clin. Med., pbz022. doi: 10.1093/pcmedi/pbz022
Jasim, R., Han, M. L., Zhu, Y., Hu, X., Hussein, M. H., Lin, Y. W., et al. (2018). Lipidomic analysis of the outer membrane vesicles from paired polymyxin-susceptible and -resistant Klebsiella pneumoniae clinical isolates. Int. J. Mol. Sci. 19:E2356. doi: 10.3390/ijms19082356
Jhelum, H., Sori, H., and Sehgal, D. (2018). A novel extracellular vesicle associated endodeoxyribonuclease helps Streptococcus pneumoniae evade neutrophil extracellular traps and is required for full virulence. Sci. Rep. 8:7985. doi: 10.1038/s41598-018-25865-z
Johnson, J. E., and Cornell, R. B. (1999). Amphitropic proteins: regulation by reversible membrane interactions (review). Mol. Membr. Biol. 16, 217–235. doi: 10.1080/096876899294544
Jozefczuk, J., Drews, K., and Adjaye, J. (2012). Preparation of mouse embryonic fibroblast cells suitable for culturing human embryonic and induced pluripotent stem cells. J. Vis. Exp. 64:3854. doi: 10.3791/3854
Khefacha, S., Chatti, A., Maalej, L., Gottardi, D., Kloula, S., Vannini, L., et al. (2015). Survival and fatty acid composition of UV-C treated Staphylococcus aureus. Ann. Microbiol. 65:235. doi: 10.1007/s13213-014-0855-6
Klimentova, J., Pavkova, I., Horcickova, L., Bavlovic, J., Kofronova, O., Benada, O., et al. (2019). Francisella tularensis subsp. holarctica releases differentially loaded outer membrane vesicles under various stress conditions. Front. Microbiol. 10:2304. doi: 10.3389/fmicb.2019.02304
Ladinsky, M., Araujo, L., Zhang, X., Veltri, J., Galan-Diez, M., Soualhi, S., et al. (2019). Endocytosis of commensal antigens by intestinal epithelial cells regulates mucosal T cell homeostasis. Science 363:eaat4042. doi: 10.1126/science.aat4042
Liu, Y., Defourny, K. A. Y., Smid, E. J., and Abee, T. (2018). Gram-positive bacterial extracellular vesicles and their impact on health and disease. Front. Microbiol. 9:1502. doi: 10.3389/fmicb.2018.01502
Masi, M., Winterhalter, M., and Pagès, J. M. (2019). Outer membrane porins. Subcell. Biochem. 92, 79–123. doi: 10.1007/978-3-030-18768-2_4
Matsutani, M., and Yakushi, T. (2018). Pyrroloquinoline quinone-dependent dehydrogenases of acetic acid bacteria. Appl. Microbiol. Biotechnol. 102, 9531–9540. doi: 10.1007/s00253-018-9360-3
McBroom, A. J., and Kuehn, M. J. (2007). Release of outer membrane vesicles by Gram-negative bacteria is a novel envelope stress response. Mol. Microbiol. 63, 545–558. doi: 10.1111/j.1365-2958.2006.05522.x
Mehta, S. K., Laudenslager, M. L., Stowe, R. P., Crucian, B. E., Sams, C. F., and Pierson, D. L. (2014). Multiple latent viruses reactivate in astronauts during space shuttle missions. Brain Behav. Immun. 41, 210–217. doi: 10.1016/j.bbi.2014.05.014
Miller, J. H. (1972). Experiments in molecular genetics. New York: Cold Spring Harbour Laboratory Press.
Mohajeri, M. H., Brummer, R. J. M., Rastall, R. A., Weersma, R. K., Harmsen, H. J. M., Faas, M., et al. (2018). The role of the microbiome for human health: from basic science to clinical applications. Eur. J. Nutr. 57, 1–14. doi: 10.1007/s00394-018-1703-4
Molina-Tijeras, J. A., Gálvez, J., and Rodríguez-Cabezas, M. E. (2019). The immunomodulatory properties of extracellular vesicles derived from probiotics: a novel approach for the management of gastrointestinal diseases. Nutrients 11:E1038. doi: 10.3390/nu11051038
Morrison, M. D., Fajardo-Cavazos, P., and Nicholson, W. L. (2019). Comparison of Bacillus subtilis transcriptome profiles from two separate missions to the International Space Station. NPJ Microgravity 5:1. doi: 10.1038/s41526-018-0061-0
Mosmann, T. (1983). Rapid colorimetric assay for cellular growth and survival: application to proliferation and cytotoxicity assays. J. Immunol. Methods 65, 55–63. doi: 10.1016/0022-1759(83)90303-4
Nelson, R. K., and Frohman, M. A. (2015). Physiological and pathophysiological roles for phospholipase D. J. Lipid Res. 56, 2229–2237. doi: 10.1194/jlr.R059220
Neutra, M., and Kozlowski, P. (2006). Mucosal vaccines: the promise and the challenge. Nat. Rev. Immunol. 6, 148–158. doi: 10.1038/nri1777
Nickerson, C. A., Ott, C. M., Wilson, J. W., Ramamurthy, R., and Pierson, D. L. (2004). Microbial responses to microgravity and other low-shear environments. Microbiol. Mol. Biol. Rev. 68, 345–361. doi: 10.1128/MMBR.68.2.345-361.2004
Ogawa, S., Tachimoto, H., and Kaga, T. (2010). Elevation of ceramide in Acetobacter malorum S24 by low pH stress and high temperature stress. J. Biosci. Bioeng. 109, 32–36. doi: 10.1016/j.jbiosc.2009.07.007
Palmer, F. B. (1971). The extraction of acidic phospholipids in organic solvent mixtures containing water. Biochim. Biophys. Acta 231, 134–144.
Patten, D. A., Hussein, E., Davies, S. P., Humphreys, P. N., and Collett, A. (2017). Commensal-derived OMVs elicit a mild proinflammatory response in intestinal epithelial cells. Microbiology 163, 702–711. doi: 10.1099/mic.0.000468
Pei, H., Wang, C., Wang, Y., Yang, H., and Xie, S. (2019). Distribution of microbial lipids at an acid mine drainage site in China: insights into microbial adaptation to extremely low pH conditions. Org. Geochem. 134, 77–91. doi: 10.1016/j.orggeochem.2019.05.008
Podolich, O., Kukharenko, O., Haidak, A., Zaets, I., Zaika, L., Storozhuk, O., et al. (2019). Multimicrobial kombucha culture tolerates Mars-like conditions simulated on low-earth orbit. Astrobiology 19, 183–196. doi: 10.1089/ast.2017.1746
Podolich, O., Zaets, I., Kukharenko, O., Orlovska, I., Reva, O., Khirunenko, L., et al. (2017). The first space-related study of a kombucha multimicrobial cellulose-forming community: preparatory paboratory experiments. Orig. Life Evol. Biosph. 47, 169–185. doi: 10.1007/s11084-016-9483-4
Portugal, J., and Rodríguez-Campos, A. (1996). T7 RNA polymerase cannot transcribe through a highly knotted DNA template. Nucleic Acids Res. 24, 4890–4894. doi: 10.1093/nar/24.24.4890
Pressler, K., Mitterer, F., Vorkapic, D., Reidl, J., Oberer, M., and Schild, S. (2019). Characterization of Vibrio cholerae’s extracellular nuclease Xds. Front. Microbiol. 10:2057. doi: 10.3389/fmicb.2019.02057
Romsdahl, J., Blachowicz, A., Chiang, A. J., Chiang, Y. M., Masonjones, S., Yaegashi, J., et al. (2019). International Space Station conditions alter genomics, proteomics, and metabolomics in Aspergillus nidulans. Appl. Microbiol. Biotechnol. 103, 1363–1377. doi: 10.1007/s00253-018-9525-0
Rowlett, V. W., Mallampalli, V. K. P. S., Karlstaedt, A., Dowhan, W., Taegtmeyer, H., Margolin, W., et al. (2017). Impact of membrane phospholipid alterations in Escherichia coli on cellular function and bacterial stress adaptation. J. Bacteriol. 199, e00849–e00816. doi: 10.1128/JB.00849-16
Sabra, W., Lunsdorf, H., and Zeng, A. P. (2003). Alterations in the formation of lipopolysaccharide and membrane vesicles on the surface of Pseudomonas aeruginosa PAO1 under oxygen stress conditions. Microbiology 149, 2789–2795. doi: 10.1099/mic.0.26443-0
Sakai, T., Moteki, Y., Takahashi, T., Shida, K., Kiwaki, M., Shimakawa, Y., et al. (2018). Probiotics into outer space: feasibility assessments of encapsulated freeze-dried probiotics during 1 month’s storage on the International Space Station. Sci. Rep. 8:10687. doi: 10.1038/s41598-018-29094-2
Santos, A. L., Moreirinha, C., Lopes, D., Esteves, A. C., Henriques, I., Almeida, A., et al. (2013). Effects of UV radiation on the lipid and proteins of bacteria studied by mid-infrared spectroscopy. Environ. Sci. Technol. 47, 6306–6315. doi: 10.1021/es400660g
Schäfer, R. (2019). Advanced cell therapeutics are changing the clinical landscape: will mesenchymal stromal cells be a part of it? BMC Med. 17:53. doi: 10.1186/s12916-019-1289-6
Schulz, E., Goes, A., Garcia, R., Panter, F., Koch, M., Müller, R., et al. (2018). Biocompatible bacteria-derived vesicles show inherent antimicrobial activity. J. Control. Release 290, 46–55. doi: 10.1016/j.jconrel.2018.09.030
Schwechheimer, C., and Kuehn, M. J. (2015). Outer-membrane vesicles from gram-negative bacteria: biogenesis and functions. Nat. Rev. Microbiol. 13, 605–619. doi: 10.1038/nrmicro3525
Sharma, K., and Singh, A. P. (2018). Antibiofilm effect of DNase against single and mixed species biofilm. Foods 7:E42. doi: 10.3390/foods7030042
Siliakus, M., van der Oost, J., and Kengen, S. (2017). Adaptations of archaeal and bacterial membranes to variations in temperature, pH and pressure. Extremophiles 21, 651–670. doi: 10.1007/s00792-017-0939-x
Smith, J. K. (2018). IL-6 and the dysregulation of immune, bone, muscle, and metabolic homeostasis during spaceflight. NPJ Microgravity 4:24. doi: 10.1038/s41526-018-0057-9
Smith, H. L., Howland, M. C., Szmodis, A. W., Li, Q., Daemen, L. L., Parikh, A. N., et al. (2009). Early stages of oxidative stressinduced membrane permeabilization: a neutron reflectometry study. J. Am. Chem. Soc. 131, 3631–3638. doi: 10.1021/ja807680m
Takahashi, N., Kitazawa, H., Iwabuchi, N., Xiao, J. Z., Miyaji, K., Iwatsuki, K., et al. (2006). Immunostimulatory oligodeoxynucleotide from Bifidobacterium longum suppresses Th2 immune responses in a murine model. Clin. Exp. Immunol. 145, 130–138. doi: 10.1111/j.1365-2249.2006.03111.x
Toribio-Mateas, M. (2018). Harnessing the power of microbiome assessment tools as part of neuroprotective nutrition and lifestyle medicine interventions. Microorganisms 6:E35. doi: 10.3390/microorganisms6020035
Toyofuku, M., Nomura, N., and Eberl, L. (2019). Types and origins of bacterial membrane vesicles. Nat. Rev. Microbiol. 17, 13–24. doi: 10.1038/s41579-018-0112-2
Trcˇek, J., Jerneje, K., and Matsushita, K. (2007). The highly tolerant acetic acid bacterium Gluconacetobacter europaeus adapts to the presence of acetic acid by changes in lipid composition, morphological properties and PQQ-dependent ADH expression. Extremophiles 11, 627–635. doi: 10.1007/s00792-007-0077-y
Tribelli, P. M., Solar Venero, E. C., Ricardi, M. M., Gómez-Lozano, M., Raiger Iustman, L. J., Molin, S., et al. (2015). Novel essential role of ethanol oxidation genes at low temperature revealed by transcriptome analysis in the antarctic bacterium Pseudomonas extremaustralis. PLoS One 10:e0145353. doi: 10.1371/journal.pone.0145353
Turina, P., Rebecchi, A., D’Alessandro, M., Anefors, S., and Melandri, B. A. (2006). Modulation of proton pumping efficiency in bacterial ATP synthases. Biochim. Biophys. Acta 1757, 320–325. doi: 10.1016/j.bbabio.2006.04.018
US Environmental Protection Agency (1998). Guidelines for neurotoxicity risk assessment. Federal Register 63 (93), 26926–26954. EPA/630/R-95/00IF (Washington, DC), 79.
Vasilyeva, N. V., Tsfasman, I. M., Kudryakova, I. V., Suzina, N. E., Shishkova, N. A., Kulaev, I. S., et al. (2013). The role of membrane vesicles in secretion of Lysobacter sp. bacteriolytic enzymes. J. Mol. Microbiol. Biotechnol. 23, 142–151. doi: 10.1159/000346550
Vaskovsky, V. E., Kostetsky, E. Y., and Vasendin, I. M. (1975). A universal reagent for phospholipid analysis. J. Chromatogr. 114, 129–141.
Vdovikova, S., Gilfillan, S., Wang, S., Dongre, M., Wai, S. N., and Hurtado, A. (2018). Modulation of gene transcription and epigenetics of colon carcinoma cells by bacterial membrane vesicles. Sci. Rep. 8:7434. doi: 10.1038/s41598-018-25308-9
Voorhies, A. A., Ott, M., Mehta, S., Pierson, D. L., Crucian, B. E., Feiveson, A., et al. (2019). Study of the impact of long-duration space missions at the International Space Station on the astronaut microbiome. Sci. Rep. 9:9911. doi: 10.1038/s41598-019-46303-8
Wilson, J. W., Ott, C. M., Höner Zu Bentrup, K., Ramamurthy, R., Quick, L., Porwollik, S., et al. (2007). Space flight alters bacterial gene expression and virulence and reveals a role for global regulator Hfq. Proc. Natl. Acad. Sci. U. S. A. 104, 16299–16304. doi: 10.1073/pnas.0707155104
Zea, L., Larsen, M., Estante, F., Qvortrup, K., Moeller, R., Dias de Oliveira, S., et al. (2017). Phenotypic changes exhibited by E. coli cultured in space. Front. Microbiol. 8:1598. doi: 10.3389/fmicb.2017.01598
Zea, L., Nisar, Z., Rubin, P., Cortesao, M., Luo, J., and McBride, S. A. (2018). Design of a spaceflight biofilm experiment. Acta Astronautica 148, 294–300. doi: 10.1016/j.actaastro.2018.04.039
Keywords: outer membrane vesicles, Mars-like stressors, lipids, functionality, biosafety
Citation: Podolich O, Kukharenko O, Zaets I, Orlovska I, Palchykovska L, Zaika L, Sysoliatin S, Zubova G, Reva O, Galkin M, Horid’ko T, Kosiakova H, Borisova T, Kravchenko V, Skoryk M, Kremenskoy M, Ghosh P, Barh D, Góes-Neto A, Azevedo V, de Vera J-P and Kozyrovska N (2020) Fitness of Outer Membrane Vesicles From Komagataeibacter intermedius Is Altered Under the Impact of Simulated Mars-like Stressors Outside the International Space Station. Front. Microbiol. 11:1268. doi: 10.3389/fmicb.2020.01268
Received: 28 January 2020; Accepted: 19 May 2020;
Published: 26 June 2020.
Edited by:
Araceli Contreras-Rodriguez, National Polytechnic Institute of Mexico (IPN), MexicoReviewed by:
Griselda Noemi Moreno, Consejo Nacional de Investigaciones Científicas y Técnicas (CONICET), ArgentinaCopyright © 2020 Podolich, Kukharenko, Zaets, Orlovska, Palchykovska, Zaika, Sysoliatin, Zubova, Reva, Galkin, Horid’ko, Kosiakova, Borisova, Kravchenko, Skoryk, Kremenskoy, Ghosh, Barh, Góes-Neto, Azevedo, de Vera and Kozyrovska. This is an open-access article distributed under the terms of the Creative Commons Attribution License (CC BY). The use, distribution or reproduction in other forums is permitted, provided the original author(s) and the copyright owner(s) are credited and that the original publication in this journal is cited, in accordance with accepted academic practice. No use, distribution or reproduction is permitted which does not comply with these terms.
*Correspondence: Olga Podolich, cG9kb2xvbGdhQHVrci5uZXQ=
Disclaimer: All claims expressed in this article are solely those of the authors and do not necessarily represent those of their affiliated organizations, or those of the publisher, the editors and the reviewers. Any product that may be evaluated in this article or claim that may be made by its manufacturer is not guaranteed or endorsed by the publisher.
Research integrity at Frontiers
Learn more about the work of our research integrity team to safeguard the quality of each article we publish.