- 1Kent Fungal Group, School of Biosciences, University of Kent, Kent, United Kingdom
- 2East Kent Hospitals, University NHS Foundation Trust, Kent, United Kingdom
- 3Laboratoire National de Santé, Dudelange, Luxembourg
Indwelling silicone valves called voice prostheses (VPs) are the gold standard for speech rehabilitation in patients with laryngeal cancer following total laryngectomy. Reported VP lifespans amongst these patients are highly variable but when devices fail patients experience loss of voice and an increase risk of chest infection. Early failure of VP is a current clinical concern that is associated with regular hospital visits, reduced quality of life and associated medical cost. Poly-microbial biofilms comprised of both bacterial and fungal microorganisms readily colonize VPs and are linked to loss of device performance and its early failure in addition to providing a reservoir for potential infection. Our detailed analysis of poly-microbial biofilm composition on 159 early failing VPs from 48 total laryngectomy patients confirmed Candida albicans as the predominant fungal species and Staphylococcus aureus as the most common bacterial colonizer within our patient cohort. Using a combination of microbiological analysis, patient data and a high-throughput antifungal test assay mimicking in vivo conditions we established an evidence based precision antifungal treatment approach to VP management. Our approach has allowed us to implement a personalized VP management pathway, which increases device in situ lifespan by an average of 270%. Our study represents a significant step forward in both our understanding of the cause of VP failure and a new effective treatment pathway that offers tangible benefit to patients.
Introduction
Head and neck cancer is the sixth most common cancer worldwide and the eighth most common cancer in the United Kingdom with 650,000 new cases (12,000 in the United Kingdom) and 350,000 deaths (4,000 in the United Kingdom) attributed to it each year worldwide (Leonhard and Schneider-Stickler, 2015; Cancer Research UK, 2018). Laryngeal malignancy is the most frequent head and neck cancer, making up 26.2% of newly diagnosed male cases and 13.1% of female cases in the United Kingdom (Cancer Research UK, 2018). Laryngeal malignancy is treated with radiotherapy if it is diagnosed at an early stage, however, at later stages treatment involves surgery often in conjunction with radiotherapy (Brook, 2013). The majority of head and neck cancers are diagnosed at an advanced stage; for example 62% of new diagnoses in Northern Ireland are reported to be at Stage III or Stage IV (Stage IV alone accounts for 45%) (Cancer Research UK, 2018). Late diagnosis means that many cases of laryngeal cancer have to undergo a partial or total laryngectomy. It has been estimated that there are currently 50,000–60,000 laryngectomees living in the United States (Brook, 2013).
Following a laryngectomy, the patient will no longer be able to form speech on their own. The gold standard for speech rehabilitation is tracheesophageal speech which requires the use of a small silicone valve known as a voice prosthesis (VP) (Bunting, 2004). Voice prostheses are susceptible to colonization by microbial biofilms (Busscher et al., 1997a). Such contamination may be enhanced by the environment of the esophagus, e.g., foods, liquids (including saliva), humidity and constant temperature (Holmes et al., 2014; Talpaert et al., 2015), and device failure has been attributed to such microbial growth as it can prevent valve closure (Leunisse et al., 2001; Oosterhof et al., 2005) and leakage of esophageal contents into the trachea. A loss of valve functionality leads to poor speech and requires replacement of the device (Ticac et al., 2010; Leonhard and Schneider-Stickler, 2015). Moreover, the microbial colonization may act as a reservoir of potential pathogens which, subsequent to aspiration, may lead to life-threatening infections such as pneumonia (Delank and Scheuermann, 2008). The mean VP lifespan is highly variable across different studies and has historically been reported as being between 120 and 200 days (Schafer et al., 2001; Sayed et al., 2012). However, a recent large-scale study which analyzed the lifespan of 3648 VPs reported an average of 86 days (Lewin et al., 2017). A number of strategies have been proposed to increase VP lifespan, such as the use of magnets to support the closing of the valve (Hilgers et al., 2003) and the incorporation of compounds, for example silver oxide, within the VP material to prevent microbial colonization (Hilgers et al., 2009). VPs containing magnets such as the Provox ActiValve have been shown to have longer in situ lifespans than other models (Kress et al., 2014; Lewin et al., 2017). However, overall, the levels of success with these approaches has been variable and importantly the average VP device lifespan has not significantly increased over the last 10 years (Lewin et al., 2017).
Co-colonization of VPs by bacterial and fungal species within poly-microbial biofilms is commonly reported. However, it is not clear to what extent yeast and bacterial biofilm colonization contributes to early VP failure, nor how effective their prevention may be in extending device lifespan. Medically, biofilms are of particular importance because it is thought that a significant percentage of human microbial infections include biofilm formation (Costerton et al., 1999; Donlan, 2001a, b). Furthermore, cells within biofilms can be up to 1000x more resistant to antimicrobial treatment than their planktonic counterparts (Chandra et al., 2001; Donlan and Costerton, 2002). The exact microbial composition on any particular VPs is influenced by several factors including patient diet, lifestyle and the voice prosthesis management regime to which they subscribe (Talpaert et al., 2015).
Streptococcal species such as Streptococcus mitis and Streptococcus sobrinus, Staphylococcal species such as Staphylococcus aureus (Neu et al., 1994), and Pseudomonas aeruginosa (Sayed et al., 2012) are among the major bacterial microorganisms frequently isolated from VPs biofilms. Candida albicans is reported as the most prevalent fungal colonizer of a VP surface (Bauters et al., 2002; Buijssen et al., 2012), it is a commensal yeast that is found on the mucosal surfaces of the oral cavity, gastrointestinal tract and genitourinary tract of most healthy individuals (Berman and Sudbery, 2002; Ganguly and Mitchell, 2011). C. albicans is a dimorphic fungus and biofilm formation of this organism involves a switch from growing in the typical budding yeast form to a filamentous hyphal form.
Here we characterize the microflora found on early failing voice prosthesis from 48 patients within Kent (South East United Kingdom) over a 5-year period (2011–2016). We observed that multi-species biofilms form in most cases, with C. albicans and S. aureus being the most prevalent. We demonstrate that the high CO2 environment experienced in the airway promotes C. albicans biofilm formation on VPs, providing an explanation for its prevalence on these devices. To determine whether yeast colonization acts as a driver for early VP failure we devised an evidence based strategy to manage contamination using a newly developed high-throughput assay that mimics in situ conditions in combination with drug sensitivity data. We report results from a 20 patient study that applies our principles of precision antimicrobial assessment that verifies antifungal treatment as a highly effective approach to VP lifespan extension. The study documented voice prosthesis lifespan pre- and post-management pathway implementation and statistical analyses confirmed a significant increase in VP lifespan within the patient cohort. Our guidelines represent a significant advance in both our understanding of the underlying cause of VP failure and a new effective method to increase VP lifespan by an average of 2.7-fold.
Materials and Methods
Patient Cohort and Voice Prostheses
Biofilms from a total of 159 early failing VPs from 48 patients (41 males and 7 females; mean age = 69.5 years, range 35–90 years) were analyzed for microorganism composition over a 5-year period (2011–2016). All prostheses were removed from patients attending one of the three main acute hospital sites within East Kent, United Kingdom: William Harvey Hospital, Ashford; Canterbury Hospital, Canterbury; Queen Elizabeth the Queen Mother Hospital, Margate. 38 of these patients had multiple voice prosthesis failures and were followed as part of a Candida management guideline impact study, which documented the voice prosthesis lifespans before and after implementation of a set of treatment guidelines designed to limit fungal growth. Treatment guidelines for the treatment of VPs with anti-fungal drugs were approved by the East Kent Hospital University Foundation Trust (EKHUFT) Voice Prosthesis Infection Management Multi-disciplinary team (MDT), EKHUFT Antimicrobial Stewardship Group, EKHUFT Drugs and Therapeutics Committee, EKHUFT ENT Audit Group, EKHUFT Adult Speech and Language Therapy Service, East Kent Prescribing Group. This study was approved by the EKHUFT Research and Innovation Department (2018/GAP/20) in accordance with the Department of Health’s Research Governance Framework for Health and Social Care and EKHUFT Research and Innovation policy. All patient data was anonymised prior to use in this study.
Please contact the corresponding authors for information if you wish to start using the Candida Management clinical guidelines for voice prosthesis management used in this study.
Microorganism Isolation and Identification
To determine which microorganisms were present, a failed voice prosthesis was removed from the patient and sealed in a sterile bag. Upon receipt at the microbiology laboratory, the voice prosthesis was added to 2 ml saline solution along with glass beads and vortexed at 2500 rpm for 30 s. The resulting suspension was plated on chromogenic agar to facilitate identification of bacterial species. 50 μl of the suspension was also plated on Sabouraud Dextrose Agar (SDA) plates (Oxoid, CM0041) containing 100 μg/ml chloramphenicol (Oxoid, SR0078) to promote the growth of fungal species while inhibiting bacterial growth. Chromogenic agar and SDA plates were incubated for 48 h at 37°C. Bacterial colonies were then picked from the chromogenic plates and fungal colonies were picked from the SDA plates, species were identified using matrix assisted laser desorption ionization time-of-flight mass spectrometry (MALDI-ToF).
MALDI-ToF Mass Spectrometry Identification of Microorganisms
MALDI-ToF mass spectrometry was used to identify species isolated from failed VPs within this study. Fungal and bacterial colonies were picked and applied to a steel MALDI target plate before being overlaid with an α-cyano-4-hydroxycinnamic acid (4-HCCA) matrix (Sigma-Aldrich, 70990) (Gobom et al., 2001). MALDI-ToF mass spectrometry was performed using a Bruker MALDI Biotyper (Bruker) as per the manufacturer’s instructions. The resulting mass spectra were compared to a reference database for identification using the MBT Compass and MBT Explorer Software along with the MBT Compass Library which comprises approximately 2750 species from 471 microorganism genera.
Antifungal Sensitivity Testing
Antifungal sensitivity testing was performed using the FungitestTM commercial testing kit (BioRad, 60780) as per the manufacturer’s instructions. Isolates were assigned sensitive, intermediate, or resistant based upon the European Committee on Antimicrobial Sensitivity Testing (EUCAST) breakpoint recommendations for each antifungal and/or species (European Committee on Antimicrobial Susceptibility Testing, 2018).
Antibacterial Sensitivity Testing
Antibacterial sensitivity testing was carried out to determine the minimum inhibitory concentrations (MICs) of clinically commonly used antibiotics. Isolates were assigned sensitive, intermediate, or resistant based upon the EUCAST breakpoint recommendations for each antibiotic and/or species (European Committee on Antimicrobial Susceptibility Testing, 2019).
Scanning Electron Microscopy (SEM) of Voices Prosthesis Surfaces
Segments were taken from the valve and flange of a Provox Vega voice prosthesis and mounted onto 12.5 mm aluminum SEM specimen stubs (Agar Scientific, AGG301) with superglue. The surfaces were imaged at ambient temperature with a Hitachi S-3400N scanning electron microscope, using the variable pressure scanning electron microscopy (VP-SEM) mode with a chamber pressure of 30 Pa and accelerating voltage of 10 kV. The backscattered electron (BSE) detector in conjunction with the energy dispersive X-ray spectroscopy (EDS) detector was used throughout with a working distance of 10 mm. The acquisition software was Oxford Instruments INCA and images were exported directly from this.
Atomic Force Microscopy (AFM) of Voice Prosthesis Surfaces
Segments were taken from the valve and flange of a Provox Vega voice prosthesis and mounted onto 15 mm AFM specimen disks (Agar Scientific, F7003) with superglue. These surfaces were imaged at ambient temperature using a Bruker Multimode 8 scanning probe microscope with a Nanoscope V controller, using the ScanAsyst peak-force tapping mode with a 50 μm × 50 μm scan area and a 700 nm scan height. SCANASYST-AIR (Bruker) silicon nitride cantilevers (tip height of 2.5–8.0 μm, nominal tip radius of 2 nm) with a nominal spring constant of 0.4 N/m and a resonance frequency of 70 kHz were used throughout. The image acquisition software was Nanoscope 8.15 R3sr5 and the processing software was Nanoscope Analysis.
Candida Strains and Growth Media
Candida strains (Supplementary Table S3) were routinely grown at 30°C in yeast peptone dextrose (YPD) media (2% peptone (BD Bacto), 2% D-glucose (Fisher Scientific), 1% yeast extract (BD Bacto). For the biofilm growth assays, Candida biofilms were grown at 37°C in RPMI-1640 media (Sigma-Aldrich, R8755) supplemented with 80 μg/ml uridine (Sigma-Aldrich, U3750). Candida strains were maintained in YPD + 20% glycerol at −80°C for long-term storage and revived at 30°C on YPD + 2% Technical Agar (Oxoid) plates.
In vitro Biofilm Growth Assays
To investigate fungal biofilm formation and its treatment on VPs a high-throughput assay was developed. C. albicans biofilms were grown on a PDMS silicone elastomer (Provincial Rubber, S1). The silicone was cut into 1 cm2 squares and placed in clips in a modified 24-well plate lid (Academic Centre for Dentistry Amsterdam, AAA-model) so they could be suspended in media within a sterile 24-well plate (Greiner Bio-one, CELLSTAR, 662160). Silicone squares were incubated in 1 ml 50% donor bovine serum (DBS) (Gibco, 16030074) for 30 min at 30°C, then washed twice with 1 ml Phosphate-Buffered Saline (PBS – 137 mM NaCl, 2.7 mM KCl, 10 mM Na2HPO4, 1.8 mM KH2PO4, pH 7.4) to remove excess DBS. Candida strains were inoculated into a test tube containing 5 ml YPD media and placed in a 30°C orbital shaking incubator with constant shaking at 180 rpm for 18 h. OD600 measurements were taken and a volume of overnight culture corresponding to OD600 = 1.0 (3 × 107 CFU/ml) was removed. These cells were pelleted by centrifugation at 4000 rpm for 5 min at which point the supernatant was discarded. The resultant pellet was re-suspended in 5 ml PBS to wash and centrifuged again at 4000 rpm for 5 min. The PBS supernatant was discarded and the pellet re-suspended in fresh PBS (at an OD600 of 1.0). The OD600 1.0 standard cell suspension was added to wells (1 ml per well) in a pre-sterilized 24-well plate and the lid with the silicone squares attached was placed on top so the silicone squares protruded into the cell suspension. These plates were then incubated at 37°C (in either 0.03% CO2 or 5% CO2) without shaking for 90 min to allow cell attachment to the silicone. After the attachment phase, the silicone squares were washed twice with 1 ml PBS to remove any unattached cells and transferred to 1 ml RPMI-1640 media (Sigma-Aldrich, R8755). They were then incubated at 37°C (in either 0.03% CO2 or 5% CO2) without shaking for up to 48 h to allow biofilm maturation. Growth of biofilms on the surface of a sterile VP was conducted using the same protocol.
Biofilm Quantification via XTT Assay
Biofilm growth was quantified using an XTT assay (Kuhn et al., 2002). Biofilms were washed twice with 1 ml PBS to remove any planktonic cells before proceeding to quantification. After washing, the biofilms were transferred to a new pre-sterilized 24-well plate (Greiner Bio-one, CELLSTAR, 662160) containing 30 μg/ml XTT labeling reagent (Roche, 11465015001) and incubated at 37°C for 4 h. After incubation, the biofilms were removed from the 24-well plate and the absorbance of the remaining XTT labeling reagent was measured at 492 nm using a BMG LABTECH FLUOstar Omega plate reader machine.
Antifungal Treatment of Biofilms
Biofilms were seeded on silicone elastomer sections as described above and grown in RPMI-1640 for 24 h at 37°C. The biofilms were then transferred to fresh RPMI-1640 media containing an antifungal, either; Fluconazole, Miconazole, or Nystatin at indicated concentrations. Fluconazole (Santa Cruz Biotechnology, sc-205698) was made as a 50 mg/ml stock solution in ethanol and diluted in RPMI-1640 final concentrations of 128 and 32 μg/ml. Miconazole (Santa Cruz Biotechnology, sc-205753) was made as a 50 mg/ml stock solution in DMSO and also diluted in RPMI-1640 to final concentrations of 32 and 128 μg/ml. Nystatin (Santa Cruz Biotechnology, sc-212431) was made as a 5 mg/ml stock solution in DMSO and diluted in RPMI-1640 to final concentrations of 2 and 8 μg/ml. Drug vehicle controls (0.25% ethanol for Fluconazole, 0.25% DMSO for Miconazole, and 0.15% DMSO for Nystatin) were used to ensure the solvents were not affecting biofilm growth. The biofilms matured in the RPMI-1640 media containing the select antifungal for a further 24 h at 37°C in both 0.03 and 5% CO2 before proceeding to quantification via the XTT assay. Experiments were performed in biological and technical triplicate.
Mixed Species Biofilm Competition Assays
Biofilms were set up as described previously, except that for mixed biofilms C. albicans and C. parapsilosis clinical isolate overnight cultures were each counted and adjusted to 1.5 × 107 CFU/ml to give a 3 × 107 CFU/ml overall inoculum (equivalent to OD600 of 1.0) for the 90 min attachment phase. After attachment, the silicone squares were washed twice with 1 ml PBS to remove any unattached cells and transferred to 1 ml RPMI-1640 media (Sigma-Aldrich, R8755). They were then incubated at 37°C in both 0.03% CO2 or 5% CO2 without shaking for 48 h to allow biofilm maturation before proceeding to quantification via the XTT assay. Experiment was performed in biological and technical triplicate.
Biofilm Composition Analysis via Chromogenic Agar
Silicone squares with biofilms on them were dropped into 2 ml PBS and vortexed for 10 s at 2500 rpm to release the biofilm cells from the surface. The resulting biofilm cell suspension was plated on Candida Ident Agar (Fluka Analytical, 94382) in triplicate (200 μl per plate). The chromogenic agar plates were incubated at 30°C for 48 h, at which point photographs of the plates were taken. C. albicans colonies appeared green and C. parapsilosis colonies stayed white on the Candida Ident Agar plates.
Analysis of Voice Prosthesis Lifespans
Voice prosthesis lifespans at the Speech and Language Therapy Centre at the Kent and Canterbury Hospital were investigated over the course of 8 years (2010–2018). In total, 38 patients had their voice prosthesis lifespan documented. However, 18 patients were removed from further analysis due to a lack of adherence to the guidelines, moving out of the hospital catchment area resulting in incomplete data entries, or simply not enough VPs failures/changes in the time period. The voice prosthesis lifespans of the remaining 20 patients, before and after the implementation of our treatment pathway, were analyzed using the Online Application for Survival Analysis 2 (OASIS 2) platform (Kim et al., 2016). In total, 143 VPs before and 176 after implementation of the pathway were included in this study. Kaplan–Meier survival curves were plotted and log-rank tests performed to statistically compare device lifespans before and after the treatment pathway. Wilcoxon Signed-Rank tests were also carried out to determine if the pathway effect was significant when taking into account the inherent differences in device lifespan from patient to patient. For all analyses, the significance level (α) was 0.05.
Results
Analysis of Microorganisms Found on Early Failing Voice Prostheses
Staphylococcus aureus was the most frequently isolated bacterial species (Figure 1B), being found on multiple early failing VP (Figures 1B,C). This finding is in line with previous studies that also identified S. aureus as the prevalent bacterial organism found within VP biofilms (Neu et al., 1994; van Weissenbruch et al., 1997). However, S. aureus was one of several Staphylococcal species identified, the others being identified at lower frequencies included Staphylococcus epidermidis and Staphylococcus schleiferi (Figures 1B,C). We also identified the S. aureus strain methicillin-resistant S. aureus (MRSA) on 5 VPs and counted these separately from the rest of the S. aureus isolates (Figures 1B,C). P. aeruginosa was the second most frequent bacterial species found on early failing VPs (Figures 1B,C). Previous studies have also identified P. aeruginosa as a prevalent bacterial species found within VP biofilms (Sayed et al., 2012). Several Streptococcus species were also identified, but at a low frequency, including Streptococcus milleri, Streptococcus mitis, and Streptococcus intermedius (Supplementary Table S1).
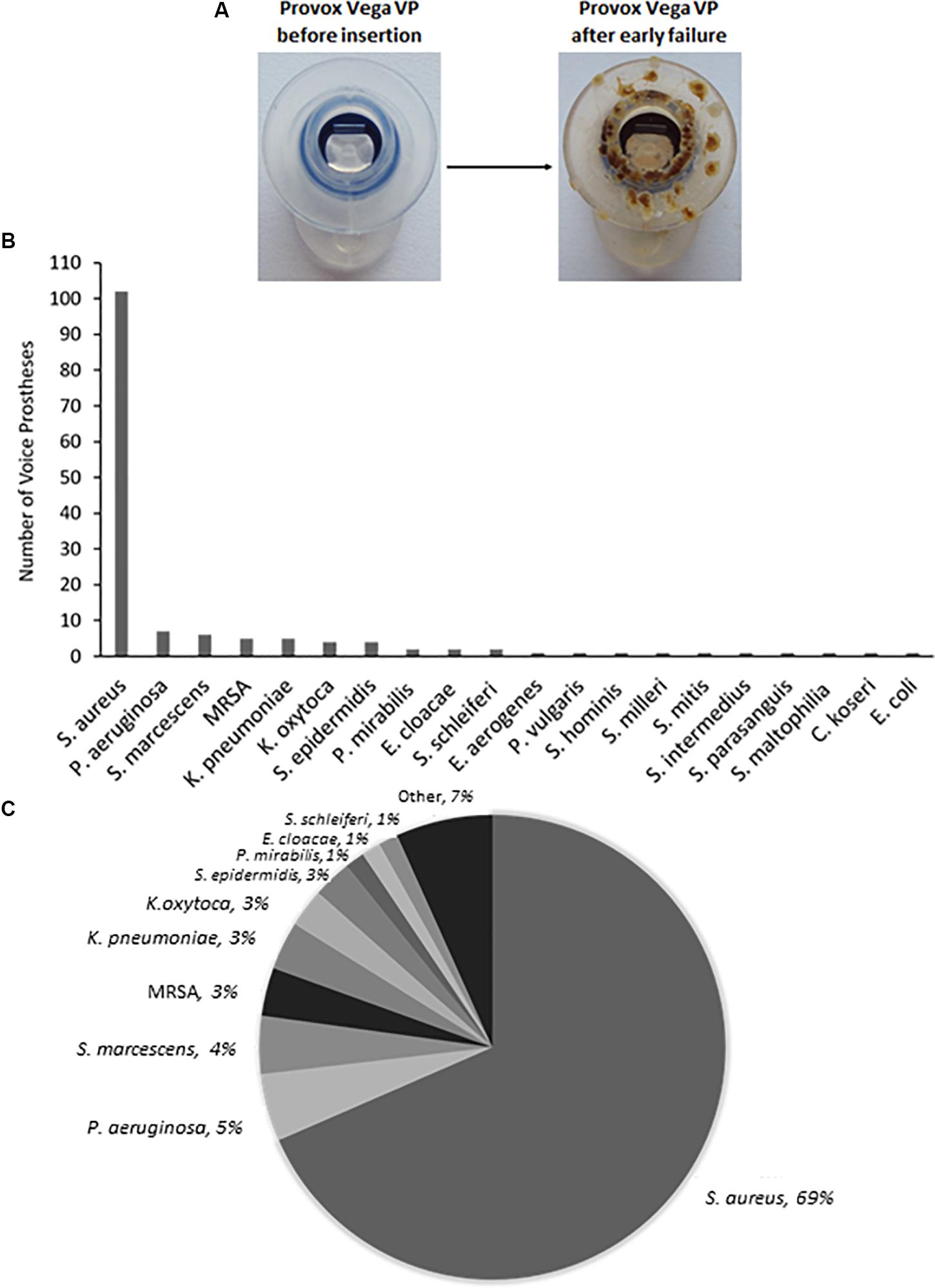
Figure 1. The range and distribution of bacterial species found on voice prostheses within this study. (A) An example of a failed voice prosthesis removed from a patient. Note the ring of colonization on the prosthesis hood around the valve mechanism. (B) Bar graph representation of absolute numbers of times each bacterial species was isolated from 159 early failing VPs. (C) Pie chart representation of percentage of total bacteria isolated from 159 early failing VPs.
Yeast species were isolated from the vast majority of the 159 early failing VPs; 107 (67.3%) were colonized by a single yeast species, 37 (23.3%) were colonized by multiple yeast species, and just 15 (9.4%) showed no yeast species presence. In total, 178 yeast isolates were identified and 168 (94.4%) of these were Candida species, the remaining 10 fungal isolates were made up of Saccharomyces cerevisiae (8) and Pichia manshurica (2). S. cerevisiae and P. manshurica isolates were normally found alongside Candida species (62.5 and 100% respectively). The most frequently isolated yeast species was C. albicans (Figures 2A,B). This is in line with previous studies which identified C. albicans as the yeast species most commonly found on VPs (Everaert et al., 1997; Bauters et al., 2002; Buijssen et al., 2012). The non-albicans Candida isolates constituted 44.4% of all fungal isolates, with Candida glabrata being the most common of these (Supplementary Table S2).
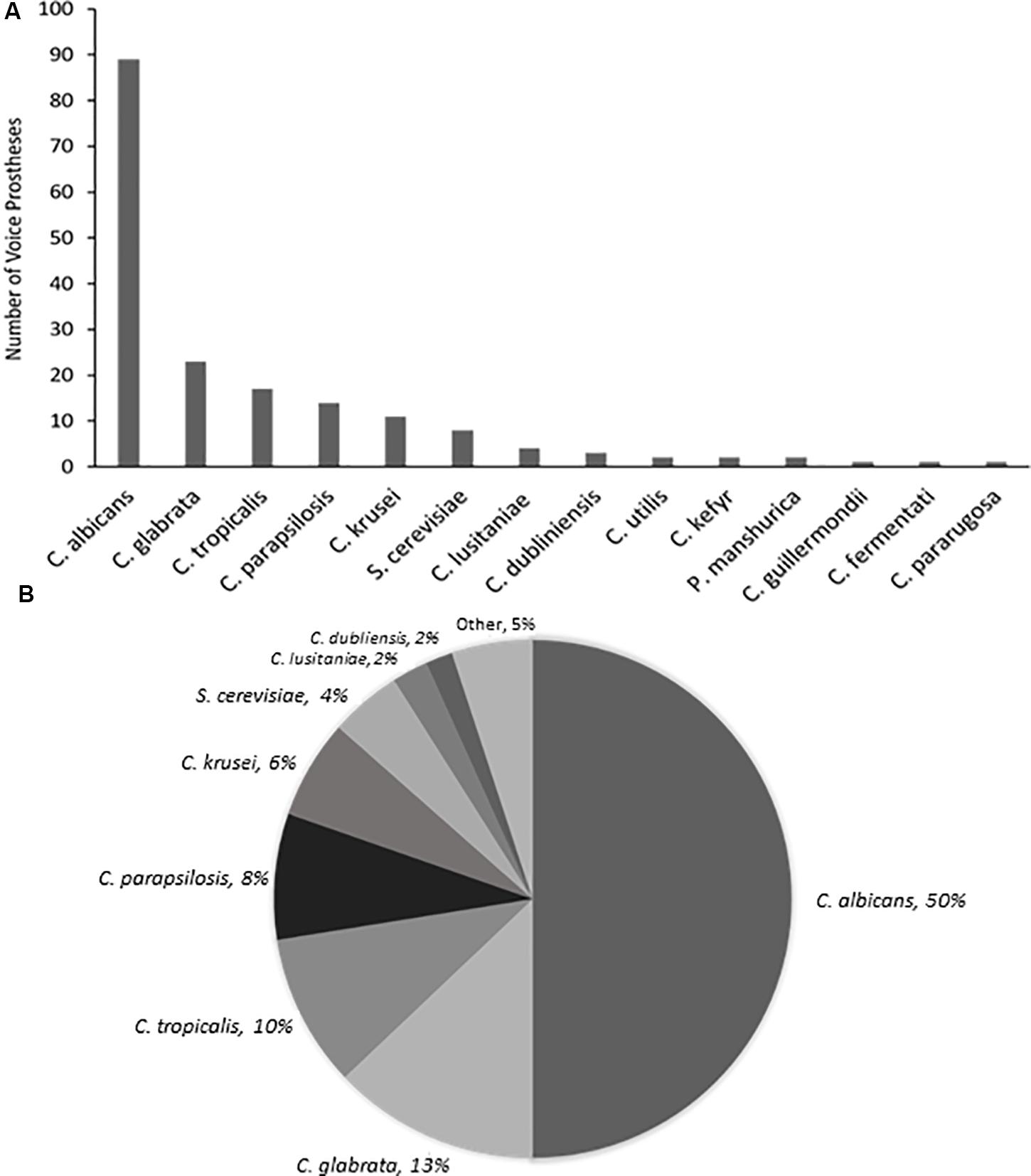
Figure 2. The range and distribution of fungal species found on early failing VP. (A) Bar graph representation of absolute numbers of times each yeast species was isolated from 159 early failing VPs. (B) Pie chart representation of percentage of total yeast isolated from 159 early failing VPs.
The same bacterial and fungal species were frequently isolated from sequential VPs removed from the same patient implicating a common pattern of biofilm establishment. In total, 38 of the 48 patients had more than one voice prosthesis failure during the course of the study. 30 of these (78.9%) had the same bacterial species present on at least 2 VPs while 29 (76.3%) had the same fungal species on at least 2 VPs. 25 of the 29 patients which had reoccurring fungal species also had the same bacterial species on at least 2 VPs. The most commonly reoccurring fungal species was C. albicans, being found on multiple VPs within 18 patients.
It is worth noting that fungal and bacterial species were often found together on early failing VPs, 134 (84.3%) had at least one species of each present. The two most numerous species in this study, S. aureus and C. albicans, were the most commonly co-isolated species, being found together on 60 (37.7%) VPs. It was very rare for only fungal species to be present; only 10 (6.3%) VPs harbored solely fungal species and intriguingly all of these were either the first or second VP failure of their respective patients. The significance of this, if any, is yet to be determined. The two most commonly co-isolated fungal species were C. albicans and C. glabrata which were found together on 17 (10.7%) VPs.
It is of importance to highlight that the most prevalent voice prosthesis colonizing organisms within our patient cohort correlate with previous findings. This suggests that an effective treatment plan designed to tackle biofilm formation on VP in our cohort may have wide-reaching applicability.
Factors Promoting Microbial Colonization of Voice Prostheses
Our routine examination of early failing VPs often suggested concentration of microbial growth on certain regions of the device surface (Figure 1A). We investigated surface topography using atomic force and scanning electron microscopy as roughness has been shown to be an important driver of colonization (Radford et al., 1998; Nevzatoğlu et al., 2007). The flange (Figure 3A) of the device exhibited a significantly rougher topography than the valve which appeared smooth using these high resolution techniques (Figures 3B,C). The roughness of the flange was particularly evident in the AFM 3D plot of its surface (Figure 3D). In line with previous studies, this may suggest that the flange, specifically the valve-flange interface, provides a more likely site for initial attachment (Leunisse et al., 2001). This proposition is also consistent with our observations that failed VPs often exhibit heavy colonization on the flange, particularly at the rougher inner edge where the flange interfaces with the valve (Figures 1A, 3Bii).
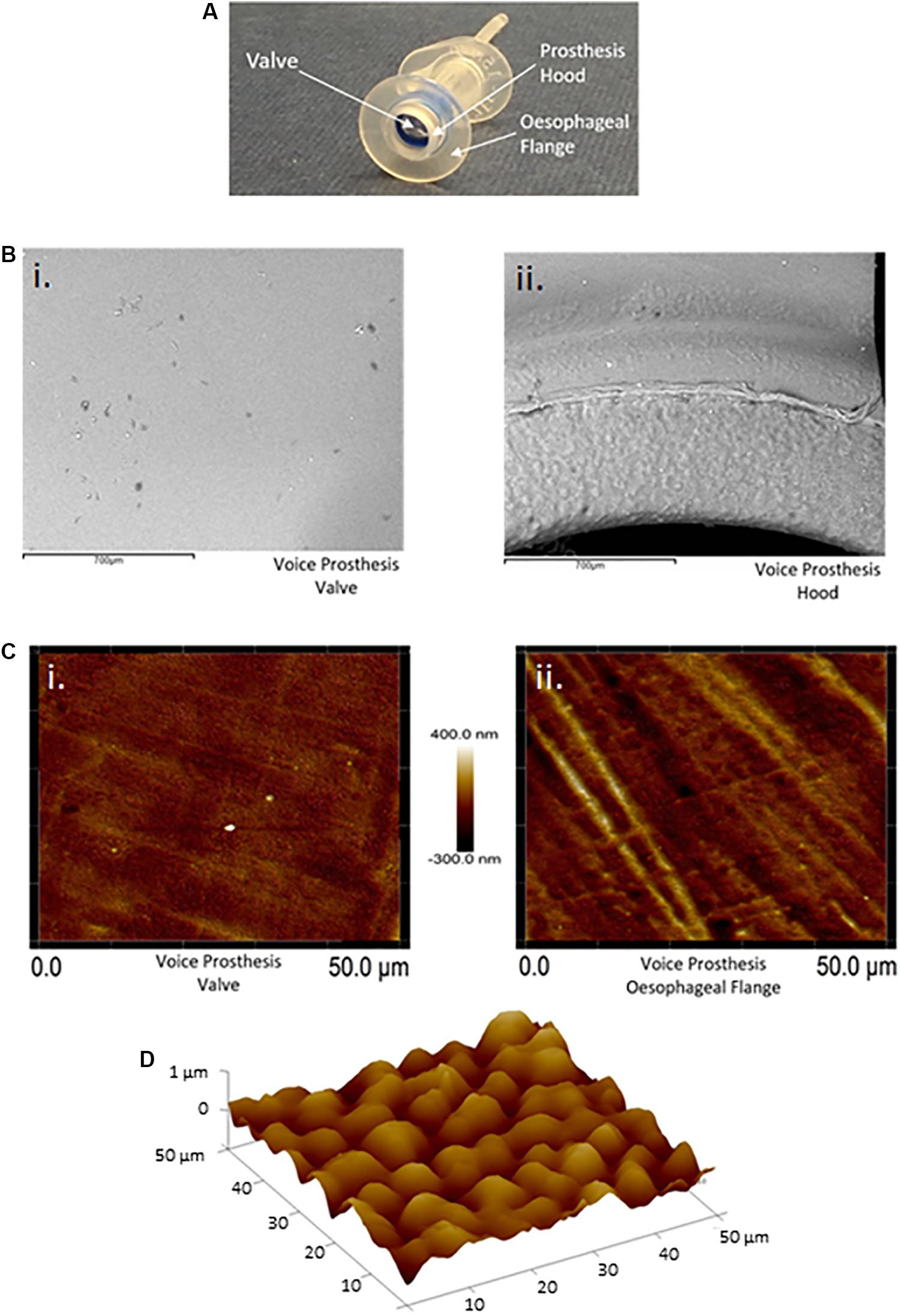
Figure 3. Atomic force and scanning electron microscopy surface topography of the valve and esophageal flange of the Provox Vega voice prosthesis. (A) The Provox Vega voice prosthesis. (B) SEM images of the valve and inner-side of the prosthesis hood were taken at x120 magnification. The scale bars represent 700 μm. (C) AFM images of the valve and esophageal flange were taken of 50 μm × 50 μm surface areas with a scan height of 700 nm. (D) AFM 3D plot of a 50 μm × 50 μm area of the esophageal flange. Z-axis is 0–1 μm. Several images were taken and representative examples are presented.
Due to the high CO2 levels (∼5%) experienced in the airway as a result of exhaled breath (Tsoukias et al., 1998), we contemplated whether CO2 could be exerting an effect on C. albicans biofilm formation in the voice prosthesis scenario. Indeed, when seeded onto a voice prosthetic surface, C. albicans has increased biofilm growth in 5% CO2 after 24 h than in atmospheric air (Figure 4). Constant exposure of the valve mechanism and parts of the esophageal flange to exhaled air may therefore contribute to the prevalence of C. albicans colonization upon VPs.
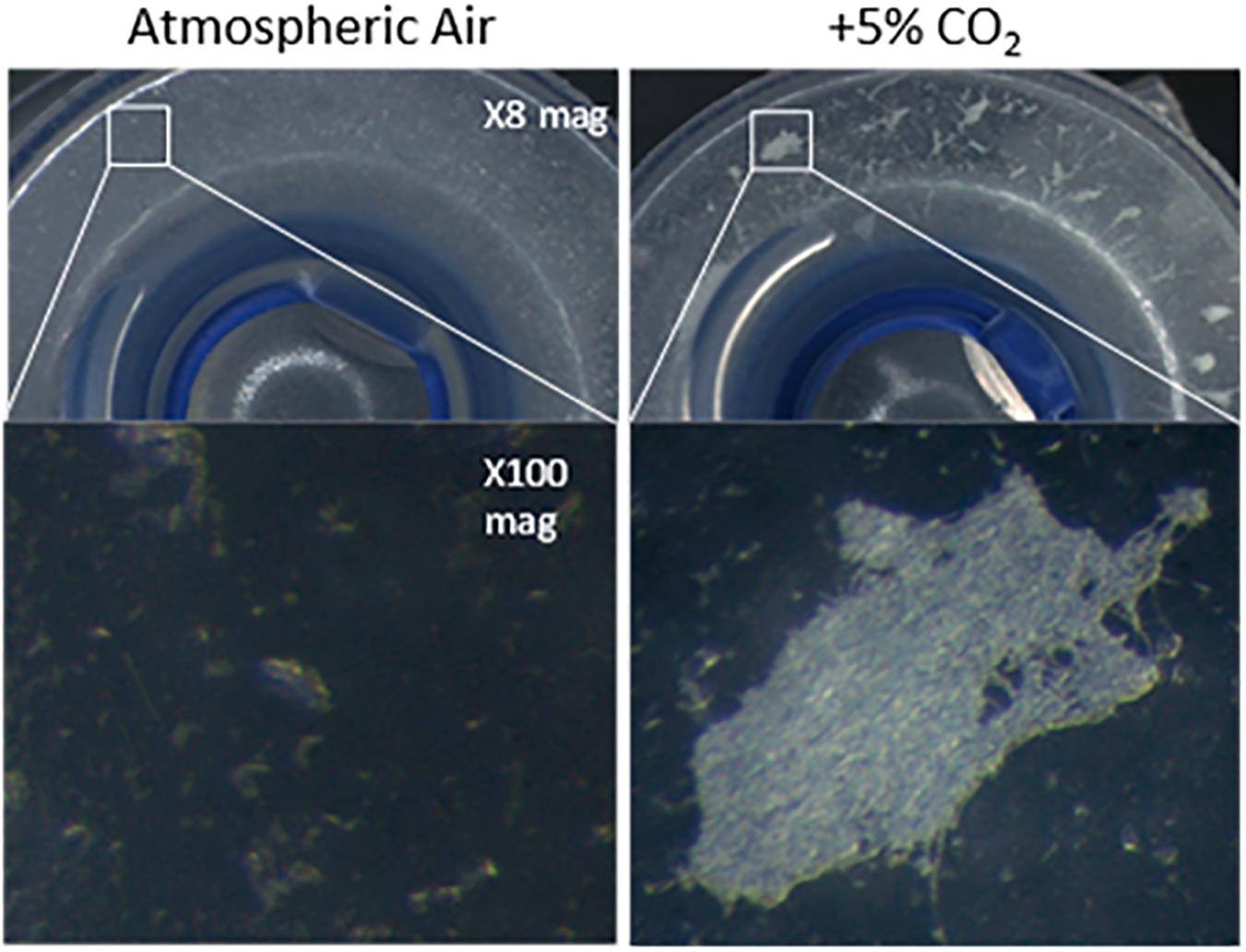
Figure 4. Candida albicans biofilm forming on the flange of a voice prosthesis in vitro in both atmospheric air and elevated CO2 conditions. C. albicans biofilm formation was assessed on a Provox Vega VP at 37°C under atmospheric air and elevated CO2 conditions that mimic exhaled breath. Images were collected after 24 h at x8 and x100 magnification. The experiment was repeated three times and representative images are presented.
C. parapsilosis Does Not Have a Competitive Advantage Over C. albicans in Biofilm Establishment
We observed that Candida parapsilosis was rarely co-isolated with another Candida species. Of the 14 VPs which harbored C. parapsilosis, only 1 also had another Candida species present. This is a stark contrast to other Candida species, such as C. glabrata which was found on 23 VPs, 19 of which also had additional Candida species. This led us to investigate whether C. parapsilosis exhibited a competitive advantage over C. albicans with regards to biofilm growth. A C. parapsilosis clinical isolate found as the sole yeast species on a failed VP was selected for this investigation. Biofilms were seeded using equal cell numbers of the C. albicans and C. parapsilosis clinical isolates either alone or in combination and incubated for 48 h to mature. Overall biofilm growth was analyzed by measuring the metabolic activity of the biofilms using the XTT colorimetric assay (Kuhn et al., 2002) (Figure 5). The proportions of C. albicans and C. parapsilosis cells were then analyzed by removing biofilms from the silicone surface and plating the resulting cell suspension on chromogenic agar (Supplementary Figure S1). Strikingly, while the C. albicans G-3065 clinical isolate was able to form a robust biofilm on the silicone surface, the C. parapsilosis G10402 clinical isolate was not (Figure 5). Moreover, when these two clinical isolates were seeded together, the resultant biofilm was composed primarily of C. albicans cells (Supplementary Figure S1). In addition, while C. albicans biofilms were significantly increased when grown in the presence of increased CO2 this was not observed for C. parapsilosis. These data suggest that the identification of C. parapsilosis in isolation on failed VPs is unlikely to arise from a competitive advantage over C. albicans.
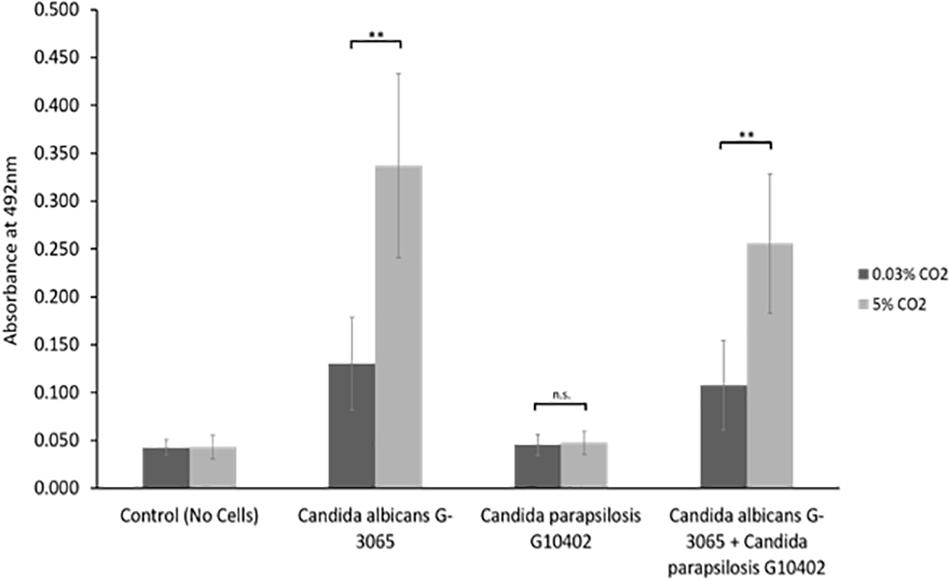
Figure 5. In vitro biofilm assay using C. albicans and C. parapsilosis early failing VP clinical isolates. Biofilms were seeded and grown for 48 h to mature, the resulting biofilms were quantified using the XTT assay which produces a red product that can be measured spectrophotometrically at 492 nm (higher cell number = higher absorbance). The C. albicans G-3065 clinical isolate makes bigger biofilms than the C. parapsilosis G10402 clinical isolate, and this biofilm formation is influenced by CO2 concentration. The graph represents three independent experiments each containing triplicates, error bars denote Standard Deviation. Paired two-tail t-tests were carried out: *p < 0.05, **p < 0.01, ***p < 0.001, n.s. = not significant.
Antimicrobial Sensitivity of Clinical Isolates
The sensitivities of the five most common bacterial species found on early failing VP to an example antibiotic from the five major antibiotic classes (Coates et al., 2011) are reported (Table 1). Only 10 (8.5%) of the most common bacterial isolates tested for sensitivity against the β-lactam antibiotic amoxicillin were judged to be sensitive, and these were all S. aureus isolates. All of the P. aeruginosa, S. marcescens, MRSA, and K. pneumoniae isolates tested for amoxicillin sensitivity were resistant. 76 (74.5%) were deemed to be sensitive to the macrolide erythromycin, with a significant number of S. aureus isolates being found to be resistant. The majority of the common bacterial isolates were sensitive to the fluoroquinolone Ciprofloxacin with 111 (93.3%) being judged as sensitive, however, all of the MRSA isolates tested were resistant to Ciprofloxacin. Likewise, a high number of isolates were sensitive to Tetracycline (104–87.4%), but this antibiotic did not exhibit good activity against the P. aeruginosa and S. marcescens isolates with 5 (83.3%) of both species being resistant. Finally, 115 (96.6%) of the common bacterial isolates tested were sensitive to the aminoglycoside Gentamicin, this was in fact the most effective antibiotic tested in this study (Table 1).
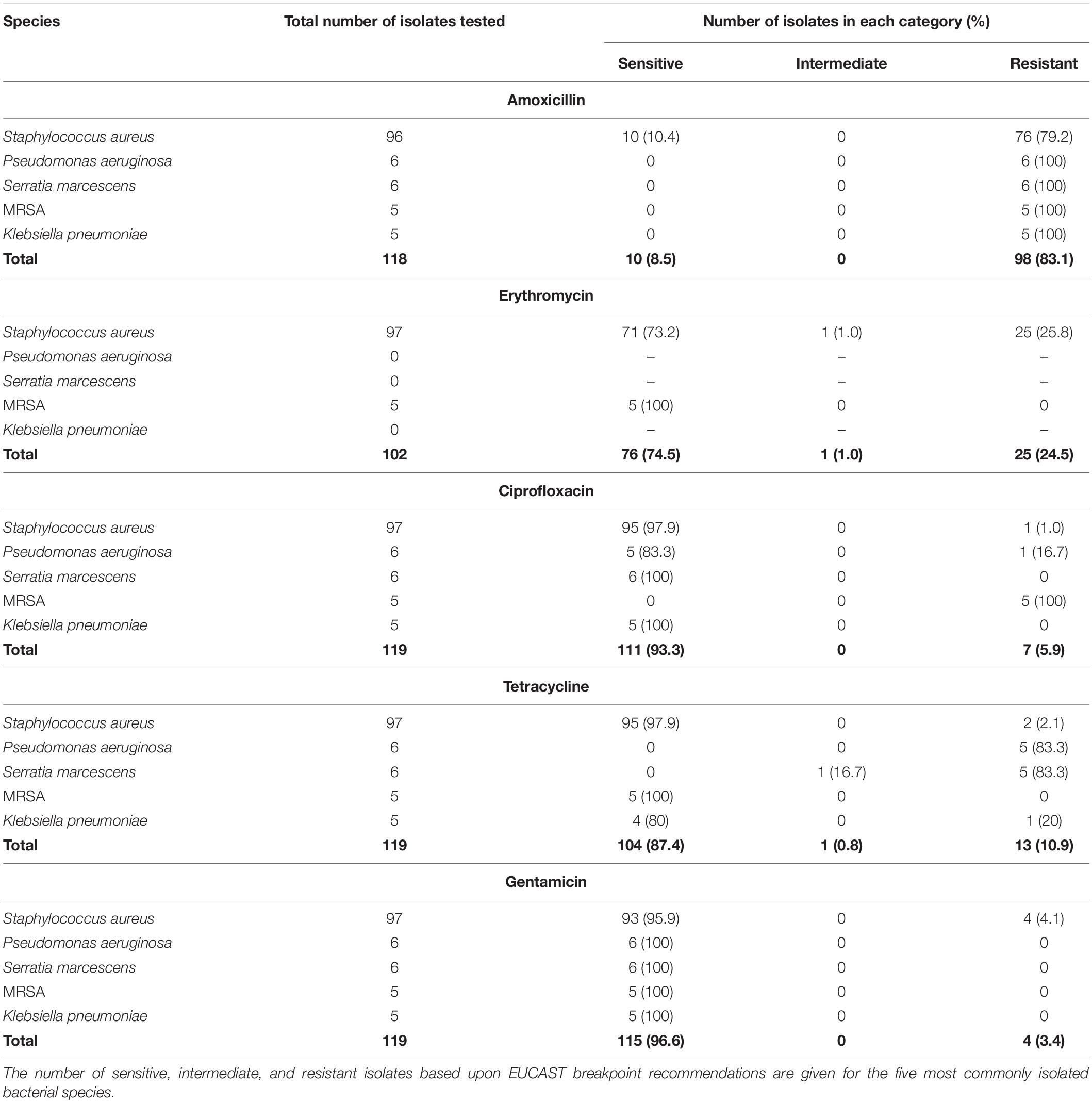
Table 1. Antibiotic sensitivity of the five most common bacterial species isolated from early failing VPs.
The sensitivities of the five most frequently isolated fungal species in this study to Fluconazole, Miconazole and Nystatin, three commonly used antifungals in the clinical setting, are reported (Table 2). Of the 168 Candida isolates, 155 were tested for Fluconazole sensitivity and 139 of these were found to be sensitive (89.7%). Moreover, 123 of the Candida isolates were tested for Miconazole sensitivity and 107 were sensitive (87.0%). Finally, 129 of the Candida isolates were tested for Nystatin sensitivity and 126 of these were found to be sensitive (97.7%). Nystatin therefore appeared to be the most effective antifungal with regards to inhibition of growth of Candida species obtained from early failing VPs in this patient cohort.
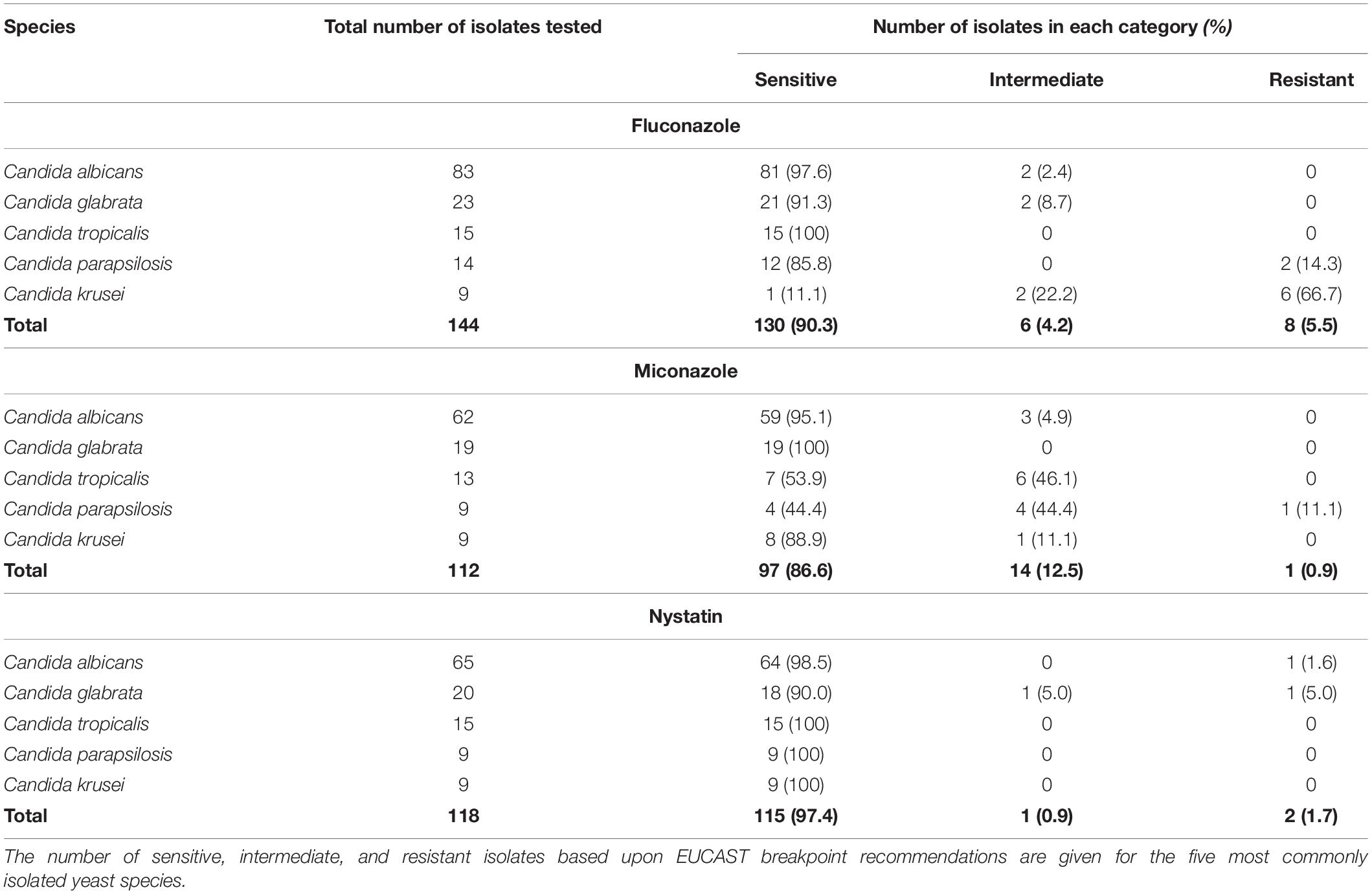
Table 2. Antifungal sensitivity of the five most common Candida species isolated from early failing VPs.
Antifungal Sensitivity of C. albicans Clinical Isolates Within Biofilms
The antifungal drugs Nystatin, Miconazole, and Fluconazole are commonly used as agents to treat fungal colonization of the ear, nose, and throat. We therefore tested their efficacy against C. albicans biofilms grown from isolates recovered from early failing VPs. The clinical isolates displayed higher resistances to Fluconazole and Miconazole compared to Nystatin when growing as biofilms (Table 3). Moreover, this azole resistance was significantly increased in biofilms grown in high CO2 environments (Supplementary Figure S2). For instance, G-8424 biofilms grown in 0.03% CO2 had an average decrease in XTT activity of 59% relative to the untreated control upon treatment with 32 μg/ml Fluconazole (p < 0.001), whereas there was no significant decrease in relative XTT activity in the 5% CO2 G-8424 biofilms (Table 3 and Supplementary Figure S2). This was also true for the 128 μg/ml Miconazole treatments in all three isolates (Table 3 and Supplementary Figure S3). Furthermore, despite the fact that 0.03 and 5% CO2 G-1625 biofilms both displayed significant decreases in relative XTT activity upon Fluconazole treatment, the relative XTT activity was still significantly higher in the biofilms grown in 5% CO2 (Supplementary Figure S2). Nystatin sensitivities were generally independent of CO2 concentration (Figure 6). The G-3065 and G-8424 clinical isolate biofilms were more resistant to Fluconazole and Miconazole than the G-1625 isolate, however, all isolate biofilms exhibited good sensitivity to Nystatin (Table 3). Based on these data, we conclude that Nystatin is the most potent against C. albicans biofilms on silicone surfaces and also offers the greatest protection against the CO2 biofilm activation. However, Fluconazole and Miconazole are still both efficacious against some isolates. This approach, which extended current drug susceptibility testing practice to better represent in vivo biofilm growth conditions, was crucial in our decision to make use of Nystatin as the lead compound in the development of antifungal treatment guidelines.
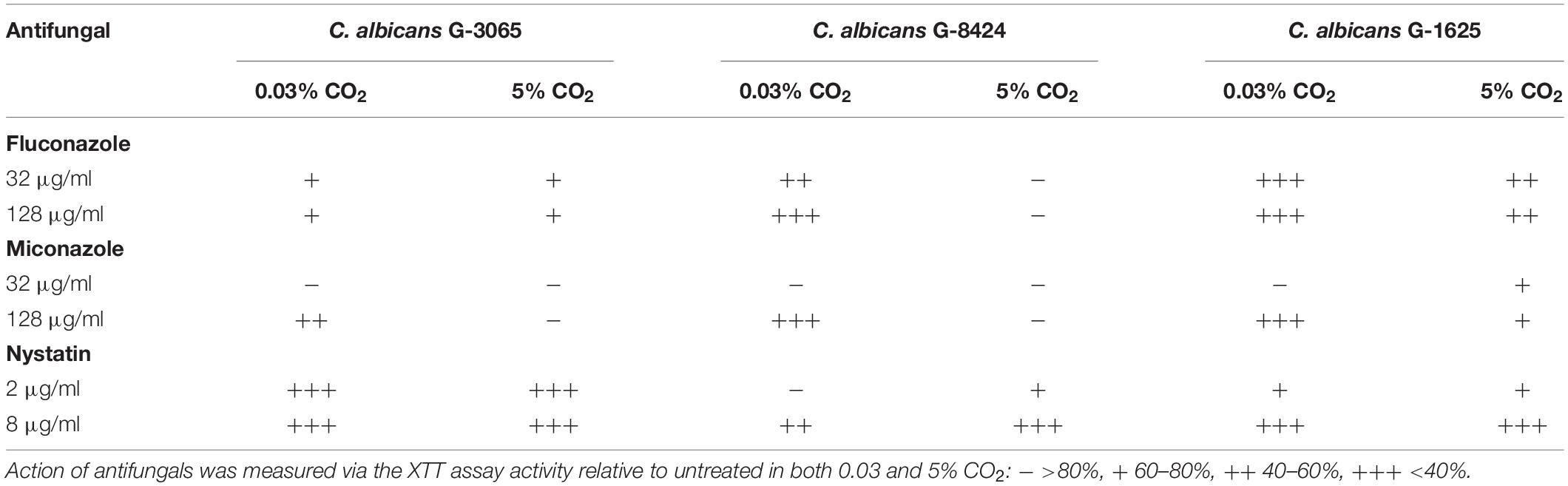
Table 3. Antifungal sensitivity of biofilms of three C. albicans clinical isolates from early failing VPs.
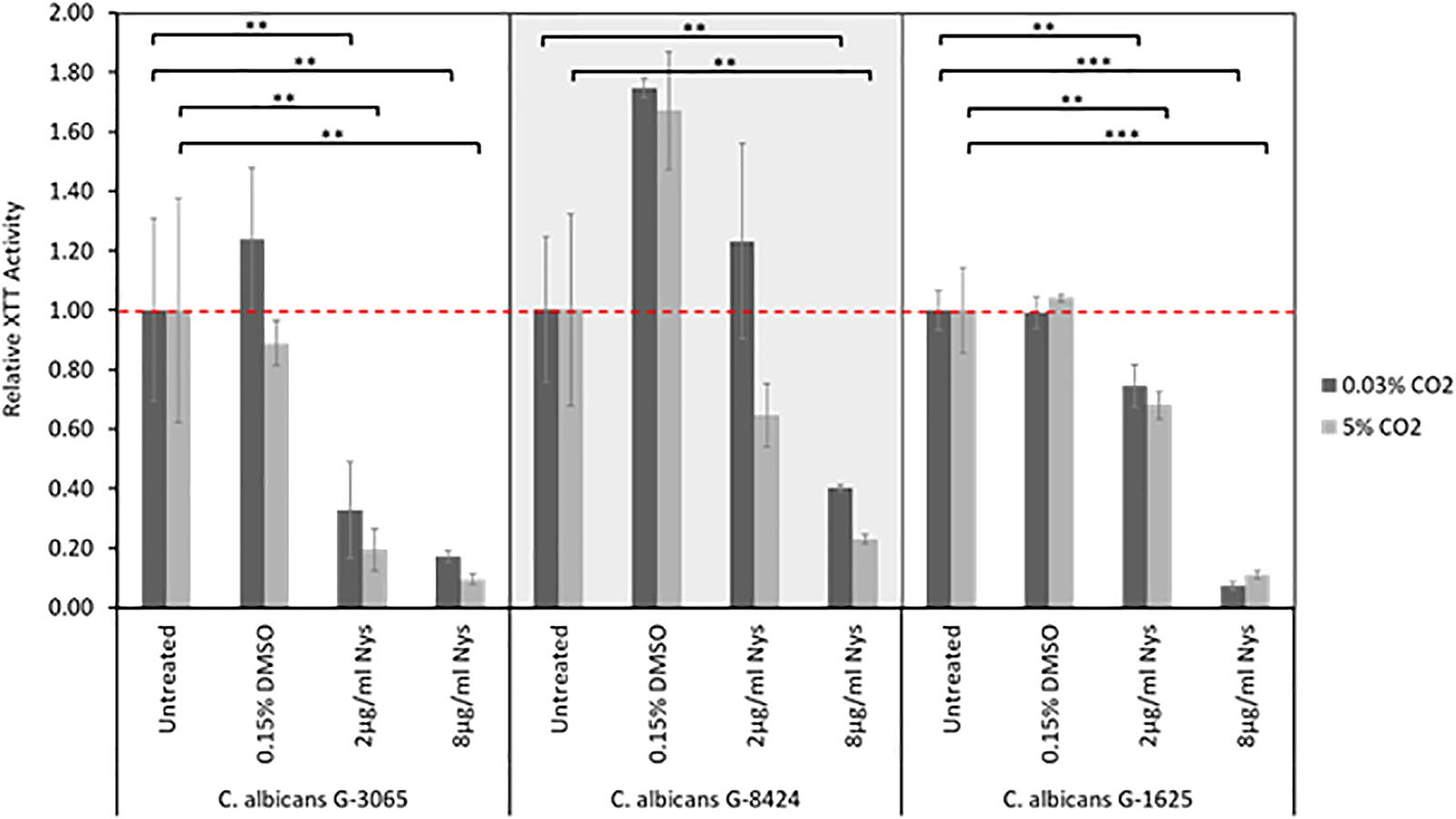
Figure 6. Nystatin sensitivities of C. albicans clinical isolate biofilms. Biofilms were seeded and grown for 24 h before addition of Nystatin, they were then grown for a further 24 h before quantification using the XTT assay. The relative XTT activity is presented with the 0.03% CO2 biofilms being normalized to the 0.03% CO2 untreated control and the 5% CO2 biofilms being normalized to the 5% CO2 untreated control. This prevents the general higher growth of 5% CO2 biofilms impacting the analysis. Graphs represent three independent experiments each containing triplicates, error bars denote Standard Deviation. Two-way ANOVAs followed by a Tukey test for multiple comparisons were carried out: *p < 0.05, **p < 0.01, ***p < 0.001. Stars directly above the bars indicate a significant difference to untreated in the same CO2 environment.
Development and Clinical Testing of Antifungal Treatment Guidelines (ATG) to Extend VP Lifespan
Although VP biofilms are likely to be formed from several species we hypothesized that an antifungal approach to reduce colonization may effective in extending device lifespan. The rationale behind this was based on three observations. First, yeast contamination contributes significant biomass which in turn is more likely to impair valve function. Second, the elevated physiological CO2 environment in which a VP sits promotes biofilm growth of the most commonly isolated yeast, C. albicans. Third, since other commonly isolated bacteria, such as S. aureus and P. aeruginosa have been reported to use Candida hyphae as a scaffold to attach to during biofilm formation (Harriott and Noverr, 2009), it may be the case that antifungal treatment also attenuates the bacterial colonization.
The antifungal drugs selected for treatment were chosen based upon the fungal sensitivity data with Nystatin being demonstrated to be the most effective as it had the lowest levels of resistance (Table 2). Previous studies have also identified that most non-albicans Candida species have higher azole MICs (Ribeiro et al., 2001; Singh et al., 2002), whereas all Candida species have low in vitro Nystatin MICs (Nenoff et al., 2016). Moreover, C. albicans sensitivity to Nystatin when in a biofilm appears to be unaffected by CO2 level (Figure 6). Our antifungal voice prosthesis management guidelines involve determining the identity and antimicrobial sensitivities of microorganisms present on a failed VP. Based on the antifungal sensitivities of the colonizing fungal species, a course of antifungals (most commonly Nystatin) is suggested and prescribed for topical application directly on the VP.
To test the effectiveness of our antifungal approach to managing VP data from 38 patients was assessed. VP lifespan data from the patient cohort before and after their placement on the ATG was assessed. Over the course of the study, 18 patients were removed from further analysis because of either; concerns over a lack of adherence to the ATG, moving out of the hospital catchment area resulting in incomplete data entries, or insufficient VP replacement data. This left 20 patients with complete VP lifespan data entries before and after guideline implementation on which to conduct statistical analyses.
Throughout the 8 year course of the study, the lifespan of 319 VPs (143 before and 176 after guidelines) across the 20 patient cohort were analyzed. These 319 VPs represented all the changes for the 20 patients when the removed VP was replaced by the same model of the same size. This ensured there were no external factors such as VP surface/surface area influencing the results. We also included all changes and not just those specifically attributed to the presence of Candida growth to remove bias.
Overall, implementation of the ATG resulted in a significant (p < 0.001) increase in VP lifespan within our patient cohort (Figure 7A). Importantly, this lifespan increase was not dependent on VP manufacturer/model as both the Blom-Singer Classic and the Provox Vega (the two most common VPs in this study) exhibited similar increases in lifespan (Figure 7B). It is also important to highlight that the lifespans of these two models were not significantly different before or after the pathway implementation (Figure 7B).
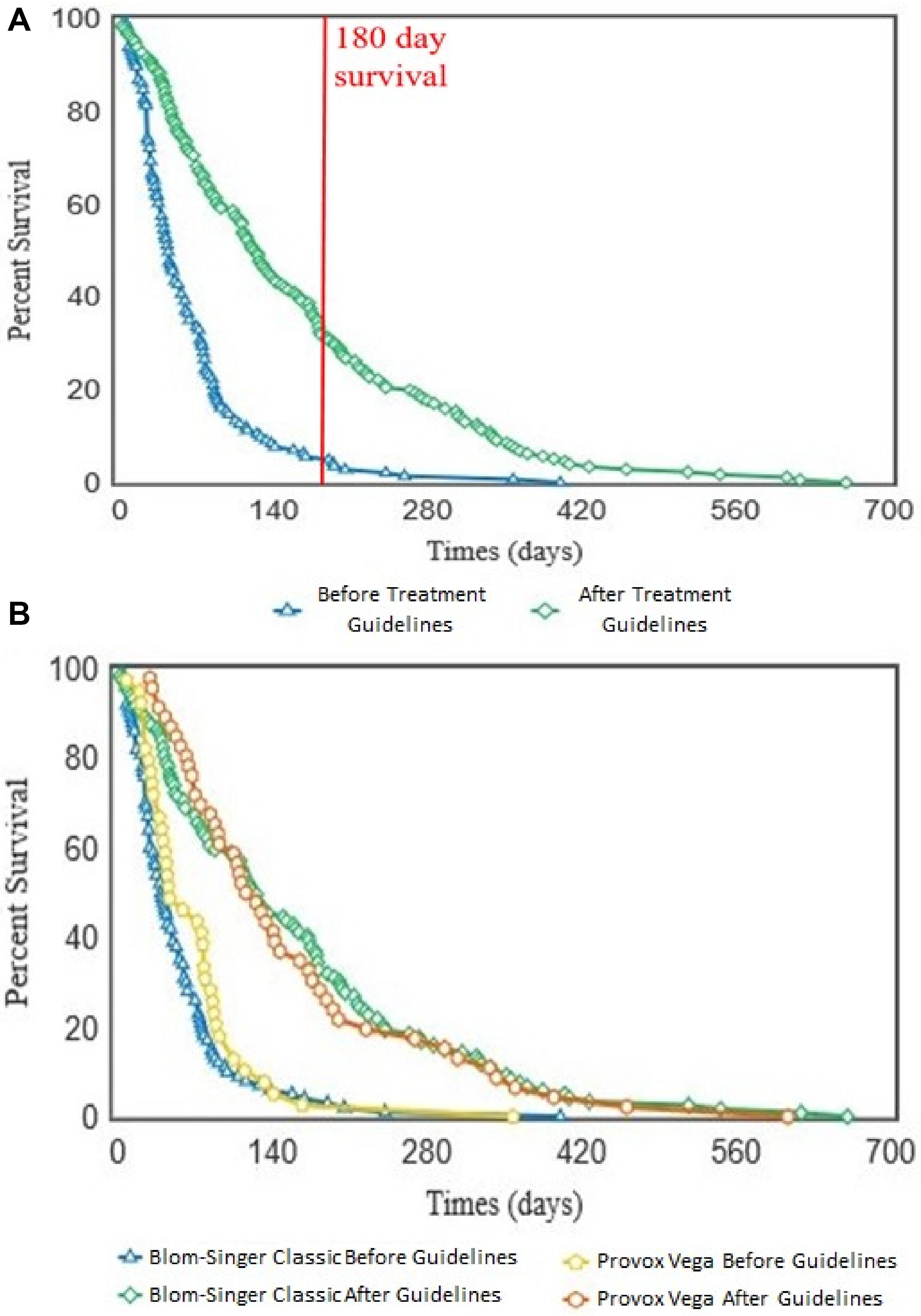
Figure 7. Kaplan–Meier survival curves showing the voice prosthesis in situ lifespans before and after implementation of our antifungal treatment pathway. (A) Device lifespans were documented before and after implementation of the ATG. Kaplan–Meier survival curves were plotted using the OASIS2 online platform and a log-rank test was used to compare the survival curves: p < 0.001. Red line is at 180 days lifespan which we targeted as the ideal lifespan to achieve. (B) After lifespan analysis across all devices was performed, the lifespans were categorized into groups depending on which VP model a patient was using. Kaplan–Meier survival curves were again plotted using the OASIS2 online platform and log-rank tests were used to compare them: Blom-Singer Classic, Before vs. After; p < 0.001. Provox Vega, Before vs. After; p < 0.001.
Although there was variation in average post-pathway VP lifespan increase between patients, the majority of the patients in our cohort enjoyed an overall increase in device lifespan (Figure 8A). Two patients did not exhibit an increase in VP lifespan, instead having a slight decrease of −2.4 and −45.5 days respectively (Figure 8A). However, these two patients had long-lived VPs on average (97.1 and 193.3 days respectively) in comparison with the rest of the cohort prior to the introduction of the new antifungal clinical guidelines. This was particularly evident for patient 16 whose VPs were already lasting longer than the post-pathway mean lifespan across all patients. Thus, the treatment guidelines may be of most benefit to patients who are experiencing early failing VP. The mean VPs lifespan before guideline implementation was 71.9 days and this increased to 192.0 days after implementation of the antifungal management pathway representing an average 2.7-fold increase in lifespan (Figure 8B).
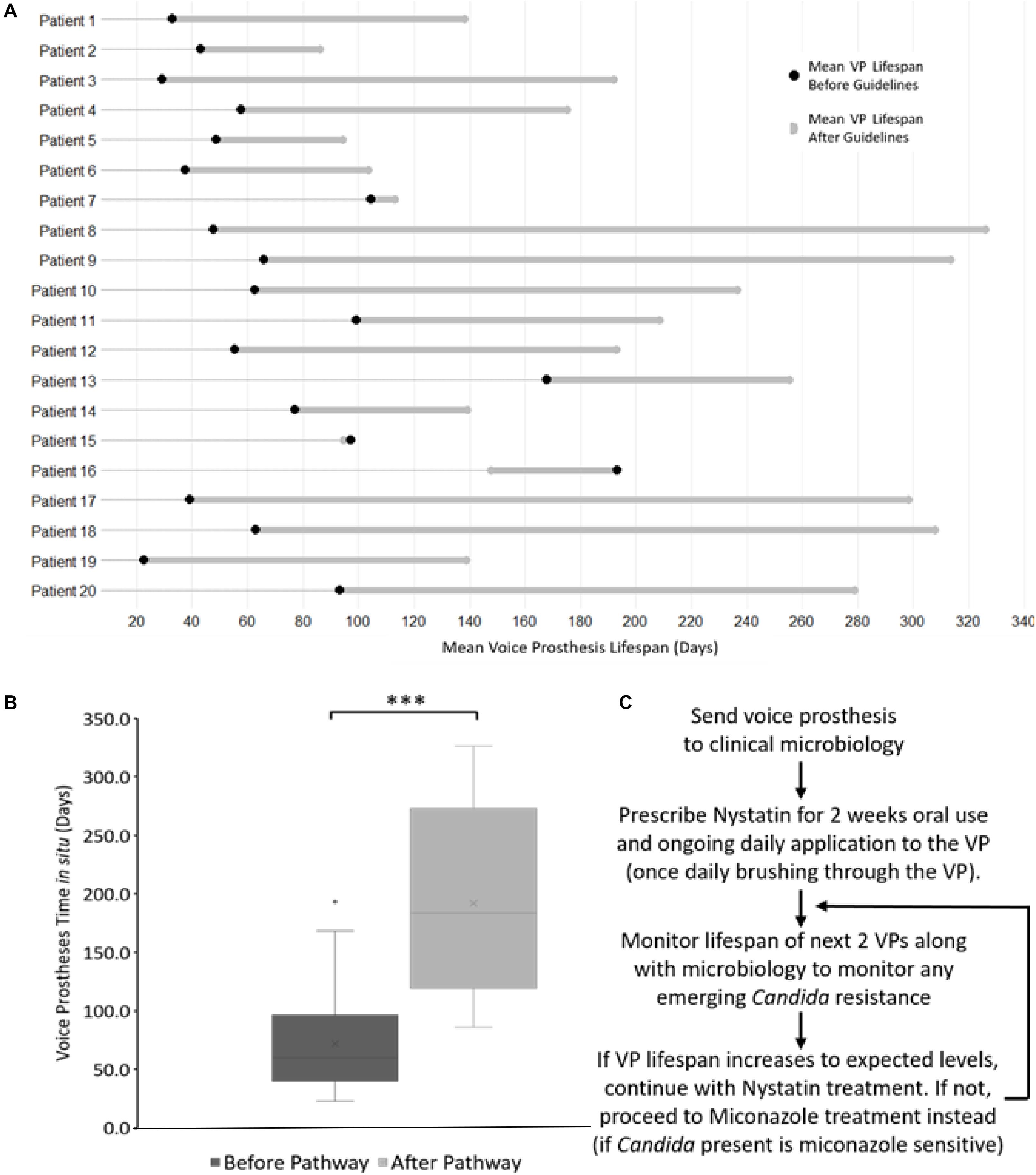
Figure 8. The antifungal treatment guidelines along with its effects on voice prosthesis lifespan within each patient. (A) Dumbbell chart representation of the mean VP lifespan difference after guideline implementation for each of the 20 patients. (B) Box plot representation of VP in situ lifespans before and after guideline implementation, Wilcoxon Signed-Rank test: ***p < 0.001. (C) Summary of the treatment pathway. As of January 2019, this management pathway has been distributed to 34 different speech and language therapy centers (32 in the United Kingdom, 2 internationally) upon request.
We did not identify any cases of increased drug resistance to antifungal application within the study. However, our guidelines recommend a 6 months (180 days) limit before VP change. This length of time was chosen primarily to ensure that the VP remains structurally sound. Before ATG use only 8 VPs (5.6%) reached this target across all 20 patients, while post-ATG 63 VPs (35.8%) lasted at least 180 days (Figure 7A). It is worth noting that after the treatment guidelines were implemented, several VPs were being routinely changed because they had been in situ for >6 months and not because they had actually failed. The full set of guidelines can be obtained by contacting the authors and are briefly summarized in Figure 8C.
Discussion
The colonization of VP by mixed biofilms has been reported as a potential cause for their early failure. However, a mechanistic understanding as to such biofilms may drive early failure and to what extend bacterial or fungal presence underlies failure is not well-understood. In this study we sought to investigate whether an antifungal treatment regime would provide an effective option for prevention early VP failure. Using a dedicated extraction protocol combined with high-powered microbiology identification we confirmed Candida albicans as the most prevalent fungal microorganism found on early failing VP. Our findings are in-line with previous studies where C. albicans was the most common fungal species isolated from VP (Buijssen et al., 2012). It is however likely that differences in the identification methods used, different patient demographics or lifestyles and different lifestyles or dietary habits will impact upon the microbial colonization of VP. For instance, Chaturvedi et al. (2014) found that C. tropicalis was the most prevalent fungal species colonizing VP within an Indian patient cohort. The high consumption of dairy products such as yogurt and buttermilk in India has been proposed to influence biofilm growth on VPs. For example, the presence of Streptococcus thermophilus and Lactobacillus in yogurt may reduce biofilm formation (Havenaar and Huis In’t Veld, 1992; Busscher et al., 1997b), while the high lactoferrin content (also found in saliva) in buttermilk has antibacterial and antifungal properties against organisms such as C. albicans and Streptococcus mutans (Soukka et al., 1991, 1992; Nikawa et al., 1993).
Our study found S. aureus as the most prevalent bacterial species on failed VPs, present on 64.2% of VPs, and was frequently found in combination with one or more Candida species. This may be expected as S. aureus is a member of the normal oral and perioral microbiota (McCormack et al., 2015). Moreover, it is the third most commonly isolated organism with C. albicans in poly-microbial infections (a significant proportion of which are nosocomial infections) (Klotz et al., 2007). Synergistic relationships between S. aureus and C. albicans have previously been documented. S. aureus is poor at forming biofilms on its own, however, it has been suggested that the hyphal C. albicans cells provide a scaffold on which the bacteria can attach within a poly-microbial biofilm (Harriott and Noverr, 2009). S. aureus has been proposed attach to C. albicans hyphae as they penetrate epithelial layers and this has been proposed as relevant for the invasion of human tissues during infection (Schlecht et al., 2015). Within a mixed biofilm, S. aureus cells have also been shown to become coated in C. albicans matrix material, enhancing its antibiotic resistance (Kong et al., 2016). Furthermore, S. aureus and C. albicans mixed biofilms can withstand higher shear stresses than pure C. albicans biofilms (Lin et al., 2013). Given the high frequency with which these two microorganisms are found together, along with the synergy they have been previously shown to exhibit, their inter-species relationship in with respect to biofilm formation on medical implant devices requires further investigation. However, it is quite plausible that by treating fungal contamination we may also decrease the burden of important bacterial pathogens, such as S. aureus on medical devices such as VPs. The potential impact of this relationship is highlighted by the fact co-infection results in increased mortality in mouse models than either microorganism alone (Carlson, 1982, 1983).
We have demonstrated that the lifespan of VP can be significantly increased by applying a highly effective personalized antimicrobial treatment regime focusing on Candida colonization, without directly treating the bacterial colonization. Initially we hypothesized that as VP failure occurs as a result of valve blockage then tackling the more significant biomass caused by yeast contamination then we may see better results than attempting to treat bacterial growth. However, our data also suggest that the rougher silicone surfaces of the VP and more significantly the high CO2 environment within exhaled breath can promote Candida biofilm establishment. Another important reason for tackling yeast contamination is that C. albicans has been shown to be able to degrading silicone rubber (Buijssen et al., 2012) which may contribute further to device failure. An antifungal approach may also be advantageous because bacteria such as S. aureus and P. aeruginosa have been reported to attach to Candida hyphae during biofilm formation (Harriott and Noverr, 2009), meaning antifungal treatment may also attenuate the bacterial colonization.
Prior to implementation of our VPs management pathway, the average in situ lifespan of VPs within our patient cohort was 71.9 days. This is similar to a recent large-scale study of voice prosthesis lifespans in the U.S. by Lewin et al. (2017) which found an average lifespan of 86 days. While being slightly less than a study by Kress et al. (2014) which found an average lifespan of 108 days. The average device lifespan within our patient cohort increased to 192 days after clinicians started following the management guidelines – a 270% increase. Importantly, the increase in lifespan was not affected by the make or model of VP within the study group, suggesting that this treatment regime may be applicable to a broad range of patients. The actual lifespan of VPs post-pathway may be higher than the 192 days reported here, as we recommended the clinicians at the Kent and Canterbury Hospital Speech and Language Therapy Centre change VPs prophylactically after 6 months (180 days) in situ. This ensures there is no biofilm formation around the TEP as well as no structural deterioration of the device due to mechanical stress and the constant application of antifungals. It should be noted that two of our patients did not experience an increase in VP lifespan while using the antifungal treatment guidelines; such a result may be expected within such a multi-factorial clinical scenario. In both cases the patients exhibited relatively long lived VPs before treatment began, and these serve to highlight the importance of patient monitoring and that fungal colonization may not be the cause of early VP failure in every case.
Conclusion
Candida albicans was the most prevalent fungal species found on failed VPs within our patient group and we have demonstrated that the topical application of antifungals significantly extends voice prosthesis lifespan in the majority of patients. Our anti-fungal treatment guidelines have been fully ratified by the relevant EKHUFT NHS committees and have distributed widely throughout the United Kingdom. Given that several studies have identified similar microorganisms as predominant colonizers of VP it is possible that our ATG protocol may be widely applied and at time of submission it has been distributed to 34 speech and language therapy centers (32 in the United Kingdom and 2 internationally). Given the success of this approach it will be imperative to investigate the use of precision antifungal methodologies to tackle biofilm formation on a wider range of medical devices and tubing as a means to reducing infection, morbidity and mortality associated with their use.
Data Availability Statement
All datasets generated for this study are included in the article/Supplementary Material.
Ethics Statement
The studies involving human participants were reviewed and approved by East Kent Hospital University Foundation Trust (EKHUFT), EKHUFT Antimicrobial Stewardship Group, EKHUFT Drugs and Therapeutics Committee, EKHUFT ENT Audit Group, EKHUFT Adult Speech and Language Therapy Service, East Kent Prescribing Group. Written informed consent for participation was not required for this study in accordance with the national legislation and the institutional requirements.
Author Contributions
This work was made possible through the collaborative efforts of the members of the Candida management multi-disciplinary team, all of whom played important roles and are placed as authors of this manuscript. DP performed data analysis, experimental procedures and was the primary author of this manuscript. CG, FM, AB, VM, and SS were responsible for conception of the study, experimental design, and editing of the manuscript. SS, LW, and CM were responsible for patient data collection. Microorganism identification from early failing VPs and initial antimicrobial sensitivity testing was carried out by MB.
Conflict of Interest
The authors declare that the research was conducted in the absence of any commercial or financial relationships that could be construed as a potential conflict of interest.
Acknowledgments
We acknowledge the contributions of East Kent Hospital University Foundation Trust committees for their role in ratifying the Candida management voice prosthesis pathway guidelines. We are also grateful for the support and positive comments of patients within the study and to other NHS trusts who have implemented the pathway and reported upon its effectiveness. Our gratitude is extended to the Kent Cancer Trust who provided funds to support Mr. DP through his Ph.D. and to SS and LW while patient data was collated.
Supplementary Material
The Supplementary Material for this article can be found online at: https://www.frontiersin.org/articles/10.3389/fmicb.2020.00975/full#supplementary-material
References
Bauters, T. G. M., Moerman, M., Vermeersch, H., and Nelis, H. J. (2002). Colonization of voice prostheses by albicans and non-albicans Candida species. Laryngoscope 112, 708–712. doi: 10.1097/00005537-200204000-00021
Berman, J., and Sudbery, P. E. (2002). Candida albicans: a molecular revolution built on lessons from budding yeast. Nat. Rev. Genet. 3, 918–930. doi: 10.1038/nrg948
Buijssen, K. J. D. A., van der Laan, B. F. A. M., van der Mei, H. C., Atema-Smit, J., van den Huijssen, P., Busscher, H. J., et al. (2012). Composition and architecture of biofilms on used voice prostheses. Head Neck 34, 863–871. doi: 10.1002/hed.21833
Bunting, G. W. (2004). Voice following laryngeal cancer surgery: troubleshooting common problems after tracheoesophageal voice restoration. Otolaryngol. Clin. North Am. 37, 597–612. doi: 10.1016/j.otc.2004.01.007
Busscher, H. J., Geertsema-Doornbusch, G. I., and van der Mei, H. C. (1997a). Adhesion to silicone rubber of yeasts and bacteria isolated from voice prostheses: influence of salivary conditioning films. J. Biomed. Mater. Res. 34, 201–209. doi: 10.1002/(sici)1097-4636(199702)34:2<201::aid-jbm9>3.0.co;2-u
Busscher, H. J., van Hoogmoed, C. G., Geertsema-Doornbusch, G. I., van der Kuijl-Booij, M., and van der Mei, H. C. (1997b). Streptococcus thermophilus and its biosurfactants inhibit adhesion by Candida spp. on silicone rubber. Appl. Environ. Microbiol. 63, 3810–3817.
Cancer Research UK (2018). Head and Neck Cancers Incidence Statistics. Available online at: https://www.cancerresearchuk.org/health-professional/cancer-statistics/statistics-by-cancer-type/head-and-neck-cancers/incidence. (accessed October, 31 2018).
Carlson, E. (1982). Synergistic effect of Candida albicans and Staphylococcus aureus on mouse mortality. Infect. Immun. 38, 921–924.
Carlson, E. (1983). Effect of strain of Staphylococcus aureus on synergism with Candida albicans resulting in mouse mortality and morbidity. Infect. Immun. 42, 285–292.
Chandra, J., Kuhn, D., Mukherjee, P. K., Hoyer, L. L., Mccormick, T., and Ghannoum, M. A. (2001). Biofilm formation by the fungal pathogen Candida albicans: development, architecture, and drug resistance. J. Bacteriol. 183, 5385–5394. doi: 10.1128/jb.183.18.5385-5394.2001
Chaturvedi, P., Sayed, S., Pawar, P., Kelkar, R., Biswas, S., Datta, S., et al. (2014). Microbial colonization of Provox voice prosthesis in the Indian scenario. Indian J. Cancer 51:184. doi: 10.4103/0019-509X.138303
Coates, A. R., Halls, G., and Hu, Y. (2011). Novel classes of antibiotics or more of the same? Br. J. Pharmacol. 163, 184–194. doi: 10.1111/j.1476-5381.2011.01250.x
Costerton, J. W., Stewart, P. S., and Greenberg, E. P. (1999). Bacterial biofilms: a common cause of persistent infections. Science 284, 1318–1322. doi: 10.1126/science.284.5418.1318
Delank, K., and Scheuermann, K. (2008). Practical aspects of voice prosthesis use after laryngectomy. Laryngorhinootologie 87, 160–166. doi: 10.1055/s-2007-995370
Donlan, R. M. (2001a). Biofilms and device-associated infections. Emerg. Infect. Dis. 7, 277–281. doi: 10.3201/eid0702.010226
Donlan, R. M. (2001b). Biofilm formation: a clinically relevant microbiological process. Clin. Infect. Dis. 33, 1387–1392. doi: 10.1086/322972
Donlan, R. M., and Costerton, J. W. (2002). Biofilms: survival mechanisms of clinically relevant microorganisms. Clin. Microbiol. Rev. 15, 167–193. doi: 10.1128/cmr.15.2.167-193.2002
European Committee on Antimicrobial Susceptibility Testing (2018). Clinical Breakpoints for Antifungals. Available online at: http://www.eucast.org/astoffungi/clinicalbreakpointsforantifungals/. (Accessed January 16, 2019).
European Committee on Antimicrobial Susceptibility Testing (2019). Clinical Breakpoints for Bacteria. Available online at: http://www.eucast.org/clinical_breakpoints/. (Accessed January 16, 2019).
Everaert, E., Mahieu, H. F., Wong Chung, R. P., Verkerke, G. J., van der Mei, H. C., and Busscher, H. J. (1997). A new method for in vivo evaluation of biofilms on surface-modified silicone rubber voice prostheses. Eur. Arch. Otorhinolaryngol. 254, 261–263. doi: 10.1007/bf02905983
Ganguly, S., and Mitchell, A. P. (2011). Mucosal biofilms of Candida albicans. Curr. Opin. Microbiol. 14, 380–385.
Gobom, J., Schuerenberg, M., Mueller, M., Theiss, D., Lehrach, H., and Nordhoff, E. (2001). α-Cyano-4-hydroxycinnamic acid affinity sample preparation. A protocol for MALDI-MS peptide analysis in proteomics. Anal. Chem. 73, 434–438. doi: 10.1021/ac001241s
Harriott, M. M., and Noverr, M. C. (2009). Candida albicans and Staphylococcus aureus form polymicrobial biofilms: effects on antimicrobial resistance. Antimicrob. Agents Chemother. 53, 3914–3922. doi: 10.1128/AAC.00657-09
Havenaar, R., Huis In’t, and Veld, J. H. J. (1992). Probiotics: a general view. Lactic Acid Bacteria 1, 151–170. doi: 10.1007/978-1-4615-3522-5_6
Hilgers, F. J. M., Ackerstaff, A. H., Balm, A., van den Brekel, M. W. M., Tan, I. B., and Persson, J.-O. (2003). A new problem-solving indwelling voice prosthesis, eliminating the need for frequent Candida- and ‘underpressure’-related replacements: provox activalve. Acta Otolaryngol. 123, 972–979. doi: 10.1080/00016480310015371
Hilgers, F. J. M., Ackerstaff, A. H., Jacobi, I., Balm, A., Tan, I. B., and van den Brekel, M. W. M. (2009). Prospective clinical phase II study of two new indwelling voice prostheses (provox vega 22.5 and 20 Fr) and a novel anterograde insertion device (provox smart inserter). Laryngoscope 120, 1135–1143. doi: 10.1002/lary.20925
Holmes, A. R., Rodrigues, E., van der Wielen, P., Lyons, K. M., Haigh, B. J., Wheeler, T. T., et al. (2014). Adherence of Candida albicans to silicone is promoted by the human salivary protein SPLUNC2/PSP/BPIFA2. Mol. Oral Microbiol. 29, 90–98. doi: 10.1111/omi.12048
Kim, S., Lee, D., Lee, H., Kim, D., Son, H. G., Yang, J.-S., et al. (2016). OASIS 2: online application for survival analysis 2 with features for the analysis of maximal lifespan and healthspan in aging research. Oncotarget 7, 56147–56152. doi: 10.18632/oncotarget.11269
Klotz, S. A., Chasin, B. S., Powell, B., Gaur, N. K., and Lipke, P. N. (2007). Polymicrobial bloodstream infections involving Candida species: analysis of patients and review of the literature. Diagn. Microbiol. Infect. Dis. 59, 401–406. doi: 10.1016/j.diagmicrobio.2007.07.001
Kong, E. F., Tsui, C., Kucharíková, S., and Andes, D. (2016). Commensal protection of Staphylococcus aureus against antimicrobials by Candida albicans biofilm matrix. mBio 7:e01365-16. doi: 10.1128/mBio.01365-16
Kress, P., Schäfer, P., Schwerdtfeger, F. P., and Rösler, S. (2014). Are modern voice prostheses better? A lifetime comparison of 749 voice prostheses. Eur. Arch. Oto Rhino Laryngol. 271, 133–140. doi: 10.1007/s00405-013-2611-0
Kuhn, D. M., Chandra, J., Mukherjee, P. K., and Ghannoum, M. A. (2002). Comparison of biofilms formed by Candida albicans and Candida parapsilosis on bioprosthetic surfaces. Infect. Immun. 70, 878–888. doi: 10.1128/iai.70.2.878-888.2002
Leonhard, M., and Schneider-Stickler, B. (2015). Voice prostheses, microbial colonization and biofilm formation. Adv. Exp. Med. Biol. 830, 123–136. doi: 10.1007/978-3-319-11038-7_8
Leunisse, C., Weissenbruch, R., Busscher, H. J., van der Mei, H. C., Dijk, F., and Albers, F. W. J. (2001). Biofilm formation and design features of indwelling silicone rubber tracheoesophageal voice prostheses–an electron microscopical study. J. Biomed. Mater. Res. 58, 556–563. doi: 10.1002/jbm.1054
Lewin, J. S., Baumgart, L. M., Barrow, M. P., and Hutcheson, K. A. (2017). Device life of the tracheoesophageal voice prosthesis revisited. JAMA Otolaryngol. Head Neck Surg. 143, 65–71. doi: 10.1001/jamaoto.2016.2771
Lin, Y. J., Alsad, L., Vogel, F., Koppar, S., Nevarez, L., Auguste, F., et al. (2013). Interactions between Candida albicans and Staphylococcus aureus within mixed species biofilms. Bios 84, 30–39.
McCormack, M. G., Smith, A. J., Akram, A. N., Jackson, M., Robertson, D., Edwards, G., et al. (2015). Staphylococcus aureus and the oral cavity: an overlooked source of carriage and infection? Am. J. Infect. Control 43, 35–37. doi: 10.1016/j.ajic.2014.09.015
Nenoff, P., Krüger, C., Neumeister, C., Schwantes, U., and Koch, D. (2016). In vitro susceptibility testing of yeasts to nystatin – low minimum inhibitory concentrations suggest no indication of in vitro resistance of Candida albicans, Candida species or non-Candida yeast species to nystatin. Clin. Med. Investig. 1, 71–76.
Neu, T., Verkerke, G. V., Herrmann, I. F., Schutte, H. K., Vander Mei, H. C., and Busscher, H. J. (1994). Microflora on explanted silicone rubber voice prostheses: taxonomy, hydrophobicity and electrophoretic mobility. J. Appl. Bacteriol. 76, 521–528. doi: 10.1111/j.1365-2672.1994.tb01111.x
Nevzatoğlu, E. U., Özcan, M., Kulak-Ozkan, Y., and Kadir, T. (2007). Adherence of Candida albicans to denture base acrylics and silicone-based resilient liner materials with different surface finishes. Clin. Oral Investig. 11, 231–236. doi: 10.1007/s00784-007-0106-3
Nikawa, H., Samaranayake, L. P., Tenovuo, J., Pang, K. M., and Hamada, T. (1993). The fungicidal effect of human lactoferrin on Candida albicans and Candida krusei. Arch. Oral Biol. 38, 1057–1063.
Oosterhof, J. J. H., van der Mei, H. C., Busscher, H. J., Free, R. H., Kaper, H. J., van Weissenbruch, R., et al. (2005). In vitro leakage susceptibility of tracheoesophageal shunt prostheses in the absence and presence of a biofilm. J. Biomed. Mater. Res. B Appl. Biomater. 73, 23–28. doi: 10.1002/jbm.b.30422
Radford, D. R., Sweet, S. P., Challacombe, S. J., and Walter, J. D. (1998). Adherence of Candida albicans to denture-base materials with different surface finishes. J. Dent. 26, 577–583. doi: 10.1016/s0300-5712(97)00034-1
Ribeiro, M. A., Dietze, R., Paula, C. R., Da Matta, D. A., and Colombo, A. L. (2001). Susceptibility profile of vaginal yeast isolates from Brazil. Mycopathologia 151, 5–10. doi: 10.1023/a:1010909504071
Sayed, S., Kazi, R., Sengupta, S., Chowdhari, A., and Jagade, M. (2012). Microbial colonization of Blom-Singer indwelling voice prostheses in laryngectomized patients: a perspective from India. Ear Nose Throat J. 91, E19–E22. doi: 10.1177/014556131209100418
Schafer, P., Klutzke, N., and Schwerdtfeger, F. P. (2001). Voice restoration with voice prosthesis after total laryngectomy. Assessment of survival time of 378 Provox-1, Provox-2 and Blom Singer voice prosthesis. Laryngorhinootologie 80, 677–681. doi: 10.1055/s-2001-18276
Schlecht, L. M., Peters, B., Krom, B. P., Freiberg, J. A., Hänsch, G. M., Filler, S. G., et al. (2015). Systemic Staphylococcus aureus infection mediated by Candida albicans hyphal invasion of mucosal tissue. Microbiology 161, 168–181. doi: 10.1099/mic.0.083485-0
Singh, S., Bhargava, P., Sobel, J. D., Boikov, D., and Vazquez, J. A. (2002). Vaginitis due to Candida krusei: epidemiology, clinical aspects, and therapy. Clin. Infect. Dis. 35, 1066–1070. doi: 10.1086/343826
Soukka, T., Lumikari, M., and Tenovuo, J. (1991). Combined inhibitory effect of lactoferrin and lactoperoxidase system on the viability of Streptococcus mutans, serotype c. Scand. J. Dent. Res. 99, 390–396. doi: 10.1111/j.1600-0722.1991.tb01046.x
Soukka, T., Tenovuo, J., Lenander-Lumikari, M., and Fungicidal. (1992). effect of human lactoferrin against Candida albicans. FEMS Microbiol. Lett. 69, 223–228.
Talpaert, M. J., Balfour, A., Stevens, S., Baker, M., Muhlschlegel, F. A., and Gourlay, C. W. (2015). Candida biofilm formation on voice prostheses. J. Med. Microbiol. 64, 199–208.
Ticac, B., Tiæac, R., Rukavina, T., Kesovija, P. G., Pedisiæ, D., Maljevac, B., et al. (2010). Microbial colonization of tracheoesophageal voice prostheses (Provox2) following total laryngectomy. Eur. Arch. Otorhinolaryngol. 267, 1579–1586. doi: 10.1007/s00405-010-1253-8
Tsoukias, N. M., Tannous, Z., Wilson, A. F., and George, S. C. (1998). Single-exhalation profiles of NO and CO2 in humans: effect of dynamically changing flow rate. J. Appl. Physiol. 85, 642–652. doi: 10.1152/jappl.1998.85.2.642
Keywords: Candida, voice prosthesis, laryngectomy, speech therapy, microbiology, antifungal
Citation: Pentland DR, Stevens S, Williams L, Baker M, McCall C, Makarovaite V, Balfour A, Mühlschlegel FA and Gourlay CW (2020) Precision Antifungal Treatment Significantly Extends Voice Prosthesis Lifespan in Patients Following Total Laryngectomy. Front. Microbiol. 11:975. doi: 10.3389/fmicb.2020.00975
Received: 17 December 2019; Accepted: 22 April 2020;
Published: 20 May 2020.
Edited by:
Juliana Campos Junqueira, São Paulo State University, BrazilReviewed by:
Renátó Kovács, University of Debrecen, HungaryPatrícia Pimentel Barros, São Paulo State University, Brazil
Copyright © 2020 Pentland, Stevens, Williams, Baker, McCall, Makarovaite, Balfour, Mühlschlegel and Gourlay. This is an open-access article distributed under the terms of the Creative Commons Attribution License (CC BY). The use, distribution or reproduction in other forums is permitted, provided the original author(s) and the copyright owner(s) are credited and that the original publication in this journal is cited, in accordance with accepted academic practice. No use, distribution or reproduction is permitted which does not comply with these terms.
*Correspondence: Campbell W. Gourlay, Qy5XLkdvdXJsYXlAa2VudC5hYy51aw==