- 1State Key Laboratory for Conservation and Utilization of Subtropical Agro-bioresources, Guangdong Province Key Laboratory of Microbial Signals and Disease Control, Integrative Microbiology Research Centre, South China Agricultural University, Guangzhou, China
- 2Guangdong Laboratory for Lingnan Modern Agriculture, Guangzhou, China
Neonicotinoids are derivatives of synthetic nicotinoids with better insecticidal capabilities, including imidacloprid, nitenpyram, acetamiprid, thiacloprid, thiamethoxam, clothianidin, and dinotefuran. These are mainly used to control harmful insects and pests to protect crops. Their main targets are nicotinic acetylcholine receptors. In the past two decades, the environmental residues of neonicotinoids have enormously increased due to large-scale applications. More and more neonicotinoids remain in the environment and pose severe toxicity to humans and animals. An increase in toxicological and hazardous pollution due to the introduction of neonicotinoids into the environment causes problems; thus, the systematic remediation of neonicotinoids is essential and in demand. Various technologies have been developed to remove insecticidal residues from soil and water environments. Compared with non-bioremediation methods, bioremediation is a cost-effective and eco-friendly approach for the treatment of pesticide-polluted environments. Certain neonicotinoid-degrading microorganisms, including Bacillus, Mycobacterium, Pseudoxanthomonas, Rhizobium, Rhodococcus, Actinomycetes, and Stenotrophomonas, have been isolated and characterized. These microbes can degrade neonicotinoids under laboratory and field conditions. The microbial degradation pathways of neonicotinoids and the fate of several metabolites have been investigated in the literature. In addition, the neonicotinoid-degrading enzymes and the correlated genes in organisms have been explored. However, few reviews have focused on the neonicotinoid-degrading microorganisms along with metabolic pathways and degradation mechanisms. Therefore, this review aimed to summarize the microbial degradation and biochemical mechanisms of neonicotinoids. The potentials of neonicotinoid-degrading microbes for the bioremediation of contaminated sites were also discussed.
Introduction
The increasing global demand for food and productive crops has facilitated tremendous growth of the pesticide sector. Neonicotinoid insecticides have been widely used for the protection of crops from a variety of insects and pests, to enhance production (Simon-Delso et al., 2015). These crops include rice, wheat, maize, soybean, cotton, sugar beet, apple, potato, etc. Neonicotinoid insecticides are developed and synthesized based on nicotine structure research with better insecticidal capabilities (Humann-Guilleminot et al., 2019). Neonicotinoids are a kind of neuroactive insecticide. Their insecticidal mechanism involves the action of nicotine acetyl bile on the postsynaptic membrane of insect nicotinic acetylcholine receptors (nAChRs) whereas the surrounding nerves induce excitation leading to paralysis and death (Zhang et al., 2018). There is no cross-resistance to the traditional long-acting insecticide classes due to the user mode of action (MoA). Therefore, neonicotinoids replace those of organochlorine, organophosphorus, chlorinated hydrocarbons, carbamate, pyrethroid insecticides, and several other chemical categories (Jeschke and Nauen, 2008). In the mid-1980s, Bayer contributed to imidacloprid (1-[(6-chloro-3-pyridinyl)-methyl]-N–nitro-2-imidazolidinimine), which was the first neonicotinoid insecticide, and this captured one of the highest shares in the global pesticide market (Peter et al., 2010). Worldwide, neonicotinoids accounted for approximately one-quarter of the pesticide market, and their annual production was about 600,000 tons (Simon-Delso et al., 2015). The broad-spectrum characteristics and high insecticidal activity of imidacloprid led to the development of a series of nicotinic insecticides, including thiamethoxam, clothianidin, dinotefuran, acetamiprid, thiacloprid, and nitenpyram.
Neonicotinoids include three main compounds:
(1) Chloropyridinyl compounds (imidacloprid, nitenpyram, acetamiprid, and thiacloprid),
(2) Chlorothiazolyl compounds (thiamethoxam and clothianidin), and
(3) Tetrahydrofuran compounds (dinotefuran) (Table 1).
Neonicotinoids are commonly used for seed treatment, soil wetting, and foliage sprays to protect crop seedlings from leaf-eating insects (Hussain et al., 2016). Neonicotinoids are water-soluble at concentrations of 184–590.0 mg⋅L–1 at 20°C and pH 7. Therefore, neonicotinoids are absorbed and circulated throughout the plant system to protect against insects (Ellis et al., 2017). Neonicotinoids bind and activate the postsynaptic nAChR of insects, which causes muscle tremors and cell fatigue. The effect of neonicotinoids is significantly stronger on invertebrates than vertebrates as vertebrates have more nAChRs (Taillebois et al., 2018; Zaworra et al., 2018). Neonicotinoids are efficient insecticides with low toxicity but their long-term usage has generated extensive environmental problems (Mariusz et al., 2013). These compounds not only affect the growth of plants and animals but also induce changes in the gene expressions (Mikolić and Karačonji, 2018).
Decades of extensive use of neonicotinoids have resulted in a universal phenomenon in the environment, posing a dangerous threat to humans and ecosystems (Casida, 2017; Humann-Guilleminot et al., 2019). These insecticides are considered a key factor in diminishing pollinating insects, especially honeybees (Friedli et al., 2020). Neonicotinoids can be adhered easily to the surface of plants, animals, and human beings, and do not degrade easily into the environment (Hladik et al., 2018). Due to the toxic and hazardous effects to bees, the outdoor use of neonicotinoids is banned in European Union member states.
Usually, both biotic and abiotic factors, including chemicals, sunlight, and microbial agents, promote the degradation of native and foreign organisms in the soil (Chen et al., 2015; Birolli et al., 2018; Yang et al., 2018; Zhan et al., 2018a; Bhatt et al., 2020c; Zhang et al., 2020). Microbial degradation is often used to transform synthetic chemicals into inorganic products (Chen et al., 2011, 2013; Arora et al., 2017; Cycoñ et al., 2017; Huang et al., 2019; Feng et al., 2020a). Uncontrollable photocatalytic conditions are the major disadvantage of non-biological degradation and thus microbial degradation emerges as a better alternative (Zhang et al., 2018). It is usually insufficient for analytical procedures to report the concentrations as most countries generally lack the environmental monitoring data systematically for neonicotinoids.
Individual neonicotinoid concentrations from the water monitoring literature demonstrated average surface water concentrations of 0.13 μg⋅L–1 (n = 19 studies) and a peak surface water concentration of 0.63 μg⋅L–1 (n = 27 studies) (Christy et al., 2015). The fate of neonicotinoid insecticides in the soil environment is greatly decided by the microbial metabolisms. However, bacteria with a complete set of genes required for complete mineralization have not been found yet. Soil microbial degradation processes of imidacloprid, acetamiprid, and thiacloprid have been understood; however, little has been reported regarding the microbial degradation of clothianidin, dinotefuran, thiamethoxam, and nitenpyram (Mulligan et al., 2016).
The microbial degradation of neonicotinoids is considered to be the most efficient and environmentally friendly in situ repair pathway (Hamada et al., 2019). The use of potential and degradative microorganisms, which can grow and survive under high-stress concentrations of insecticides, offers a possible opportunity for the remediation of toxic pollutants and hazardous wastes from contaminated environments (Chen et al., 2014; Rana et al., 2015; Xiao et al., 2015; Zhan et al., 2018b; Bhatt et al., 2020a).
A variety of neonicotinoid-degrading microorganisms have been isolated and identified. These microbes include Bacillus, Mycobacterium, Pseudoxanthomonas, Rhizobium, Rhodococcus, Actinomycetes, and Stenotrophomonas (Dankyi et al., 2018; Zhang et al., 2018; Hamada et al., 2019). The bioremediation potential of neonicotinoid-contaminated water/soil environments using several different microbes has been investigated. However, the detailed knowledge about specific degradative enzymes and genes still needs to be explored. To date, only a few studies have focused on the enzymatic and genetic basis of neonicotinoid-degrading microorganisms to evolve better pathways for sustainable degradation. Hence, in this review article, the microbial degradation pathways of neonicotinoids were summarized, and the molecular biology and genetic pattern of neonicotinoid-degrading microbes were also discussed.
Toxicity of Neonicotinoids
At present, neonicotinoids have developed into one of the most widely used insecticides all over the world and have attracted a great deal of attention for their cumulative toxic effects and drug resistance (Bartlett et al., 2019; Li et al., 2019; Wang et al., 2019a,b,c). It is mainly used to control mealworm planthopper, leafhopper, aphid, psylla thrips, leaf beetle, leaf miner, leaf beetle, beetle, termite, red fire ant, cockroach, fly, nematode, and other pests (Calvo-Agudo et al., 2019). Compared to the original pesticide, several neonicotinoid metabolites possess stronger toxicity and durability (Hamada et al., 2019). Only about 10% of the applied insecticide reaches target organisms, and the remaining 90% is distributed in the environment where it can adversely affect non-target organisms and ecosystems (Lin et al., 2020). Therefore, the bioavailability of pesticides in the soil environment is an important factor affecting soil microbial population.
To assess the genetic, structural, and functional biodiversity of the microbial community in neonicotinoid (imidacloprid) treated soils various biotechnological and molecular approaches, including the use of phospholipid fatty acid (PLFA) profiles, denaturing gradient gel electrophoresis (DGGE), and community level physiological profiles (CLPP), have been performed. Among these techniques, PLFA profiles are very useful to give the information about the shifting of microbial community structure and decrease in the total biomass of the microbial community after imidacloprid treatment (Cycoñ and Piotrowska-Seget, 2016). Toxicological studies of neonicotinoids have been conducted on flying organisms, aquatic organisms, terrestrial organisms, and human beings.
This paper summarized the representative studies of the last 3 years (Table 2) and concluded that high levels of neonicotinoid pesticides harmfully affected non-target biological bees during pollen harvesting and flight (Zaworra et al., 2018; Colin et al., 2019). Moreover, long-term exposure to neonicotinoid pesticides led to weight loss, impaired flight, and phototropism. The accumulation of neonicotinoids was demonstrated to severely affect their longevity, pollination, and learning ability, and suppress gene expression (Ellis et al., 2017; Tosi et al., 2017; Tosi and Nieh, 2017; Christen et al., 2018; Manjon et al., 2018; Li et al., 2019). Other non-target organisms may also be seriously affected by these pesticides. The European Siberian carabid Platynus assimilis exercised less after a short period of hyperactivity due to the neonicotinoid pesticide (Tooming et al., 2017). The survival and growth of the mayfly were significantly reduced by sub-lethal concentrations of neonicotinoid pesticides (Bartlett et al., 2018).
Neonicotinoids pose a significant toxicological impact on fish and other aquatic microorganisms (van Gestel et al., 2017; Caron-Beaudoin et al., 2018; Vignet et al., 2019). These were also reported to induce genotoxicity and reduce the immune system in aquatic organisms (Hong et al., 2018). The exposure of fish to neonicotinoids led to increased gene expression and protein levels; whereas, AChE activity decreased (Selçuk et al., 2018; Tian et al., 2018). van Gestel et al. (2017) reported that high concentrations of imidacloprid and thiacloprid caused toxicity for up to three generations of Folsomia candida. The toxicity of neonicotinoids also significantly reduced the number of microorganisms and the abundance of phytoplankton and zooplanktons (Sumon et al., 2018).
Neonicotinoid pesticides can affect land animals by direct contact or food chain transmission. Mollusks and earthworms are more prone to these pesticides as their body surface comes in direct contact with a large area of soil. The oxidation and destruction of lipids, proteins, and nucleic acids in earthworms in response to large amounts of dinotefuran has been well reported (Liu et al., 2017). Qi et al. (2018) have further explained the damage of the epidermis, gut, and neurochord in earthworms by cycloxaprid along with effected catalase and superoxide dismutase enzymes activities.
A variety of neonicotinoid pesticides are known to produce carcinogenic and neurotoxic effects in lizards by damaging the liver and interfering in the growth hormone/insulin-like growth factor (GH/IGF) pathway (Wang et al., 2018, 2019a,b,c, 2020). Thiamethoxam and clothianidin also interfere with the endocrine system (Wang et al., 2019a, c), can increase the thalamic and hippocampal regions of mice, and pose toxic effects on the embryos of mice and rabbits (Babeíová et al., 2017; Hirano et al., 2018).
Şenyildiz et al. (2018) reported that neonicotinoid insecticides might also induce cytotoxicity and DNA damage in mammalian cells. The exposure of human neuroblastoma (SH-SY5Y) as well as human hepatocellular carcinoma (HepG2) cells to neonicotinoid pesticides brings about damage of the SHSY-5Y cells and alteration of the HepG2 cells (Şenyildiz et al., 2018; Bivehed et al., 2019). The exposure of Hs578t cells to environmental concentrations of neonicotinoid results in the inhibition of I.4 promoter activity and aromatase catalytic activity (Caron-Beaudoin et al., 2018). Neonicotinoid pesticides also cause reproductive toxicity in humans by increasing the estrone and estradiol production and strongly inhibiting estriol production (Caron-Beaudoin et al., 2017; Han et al., 2018).
Neonicotinoid-Degrading Microorganisms
Due to their characteristics of high applicability, ease of operation, and low cost, microbial degradation is acceptable in practical applications for the degradation of large amounts of neonicotinoids that remain in the environment (Shaikh et al., 2014; Jean-Marc et al., 2019). As one of the potential applications in the degradation of pollutants, cell immobilization (CI) is an effective method characterized by limiting cells to a defined region while maintaining their metabolic, catabolic, and catalytic activity. Immobilized cells can degrade toxic substances more efficiently than free cells (Conde-Avila et al., 2020). Under the condition of conventional bacteriological media and harsh environmental conditions, most bacteria cannot be cultured and enter into a viable but non-culturable (VBNC) state (Ramamurthy et al., 2014; Fida et al., 2017; Su et al., 2018, 2019). A VBNC state could be used to improve the biodegradation of neonicotinoids. There are two categories of bacterial biodegradation: (a) pure bacterial culture biodegradation and (b) microbial co-degradation (Table 3).
Pure bacterial biodegradation uses pesticides as the only source of nitrogen or carbon needed for growth, whereas co-metabolic biodegradation requires more nutrition sources, in addition to pesticides. According to the chemical structure of pesticides and the specific environmental conditions to reduce the decomposition activity of microorganisms, neonatal metabolites may vary greatly (Hussain et al., 2016). A clear understanding of the degradation kinetics is important for further enhancement of the degradation (Wang et al., 2013b). Rhizobacterial inoculants have been mainly used for controlling plants and biology. Recently, it was reported that the inoculation of plants with specific plant-growth-promoting rhizobacteria (PGPR) strains enhanced the absorption of ingredients (N, P, K, Fe, Zn, and Mg), heavy metals (Cd, Ni, and Pb), and pesticides. However, research on the relationship between plant growth promotion by PGPR inoculants and enhanced absorption is being further studied (Myresiotis et al., 2015).
Imidacloprid
Imidacloprid, (1-[(6-chloro-3-pyridinyl)-methyl]-N-nitro-2-imidazolidinimine), is a kind of super-efficient neonicotinoid with colorless crystals and a faint smell. It is a broad-spectrum, widely used, and has comparatively lower toxicity and residues. Imidacloprid is relatively safe for humans and animals, and it has not been reported with pest resistance. It is mainly used against sucking pests of rice, wheat, and cotton crops including aphids, leafhoppers, thrips, whitefly, potato beetle, and straw fly.
Imidacloprid blocks the central nervous conduction in insects and induces paralysis leading to death. The decrease of the bioavailability of microbial-degradable pesticides led to the extension of the half-life of imidacloprid and its metabolites in soil (Anhalt et al., 2007). The report on microbial degradation of imidacloprid by Leifsonia sp. strain PC-21 was isolated from contaminated sites by an enrichment cultivation technique that degraded 70% of imidacloprid within 14 days and formed desnitro and urea metabolites (Anhalt et al., 2007). Leifsonia strain PC-21 was also reported to degrade about half of 25 mg⋅L–1 imidacloprid in tryptic soy broth containing 1 g⋅L–1 succinate and D-glucose at 27°C in 3 weeks (Phugare et al., 2013).
6-Chloronicotinic acid (6-CNA), olefinic cyclic nitroguanidine, cyclic urea, cyclic guanidine, nitroso, and nitro derivatives are major imidacloprid metabolites, which have been detected in soil and water samples (Kandil et al., 2015; Wang et al., 2016; Seifrtova et al., 2017). In the same way, Pseudomonas sp. 1G can also convert imidacloprid into denitrification products and urea metabolites (Pandey et al., 2009). Stenotrophomonas maltophilia induces the hydroxylation of imidacloprid to generate 5-hydroxyl imidacloprid, which has a stronger insecticidal activity than the parent compound (Dai et al., 2006).
The Bradyrhizobiaceae strain SG-6C can utilize 6-CNA as the sole carbon source and a 1% (v/v) seed culture of this strain completely degraded 20 mg⋅L–1 of 6-CNA (0.1 mM) within 152 h (Shettigar et al., 2012). Phugare et al. (2013) reported that the Klebsiella pneumoniae strain BCH1 degraded 78% imidacloprid within 7 days at 30°C. Bacillus, Bacillus brevis, Pseudomonas sp. F1, Bacillus subtilis and Rhizobia degraded 25%–45% initial imidacloprid (25 g⋅L–1) in carbon limited minimal salt medium (MSM) within 25 days (Sabourmoghaddam et al., 2015).
In addition to pure cultures, mixed cultures of native soil bacteria (Bacillus aerophilus and Bacillus alkalinitrilicus) have also been reported for the remediation of imidacloprid-contaminated soils (Sharma et al., 2014; Akoijam and Singh, 2015). As a sole source of carbon and energy, 46.5% of 0.5 mM imidacloprid in a minimal medium was degraded by Pseudomonas sp. RPT 52 at more than 40 h. Rhizospheric microorganisms are potent degraders of environmental contaminants due to their unique resistance properties. Pseudoxanthomonas indica isolated from rhizospheric soil was reported with the fastest imidacloprid biodegradation rate (2.17 μg⋅mL–1⋅h–1) in liquid culture and soil slurry as compared to other bacterial strains (Ma et al., 2014).
According to the latest research, Guo et al. (2020) isolated an Oligotrophic bacterium, Hymenobacter latericoloratus CGMCC 16346, from a water environment. This bacterium can survive for a long time on 1/10,000 diluted nutrient medium and degrade imidacloprid in apotrophic surface water. In addition to bacteria, fungal cultures are also effective tools in the bioremediation of imidacloprid. The effective bioremediation of imidacloprid from contaminated water and environmental samples with Aspergillus terreus YESM3 was reported (Mohammed and Badawy, 2017).
Biodegradation is one of the best techniques that works well under a laboratory environment (Liu et al., 2015; Bhatt et al., 2020b; Lin et al., 2020). Sometimes, the incompatibility of microorganisms with the environment under field conditions reduces their efficiency. Indigenous microorganisms of an environment can easily replace applied cultures because of the difference between the in situ and ex situ environments of the plants, the soil properties, and the micro-ecological conditions (Chen et al., 2012; Bhatt et al., 2020c; Huang et al., 2020; Zhan et al., 2020). Therefore, it is very essential to study the compatibility of isolated strains.
Acetamiprid
Acetamiprid, N-(N-cyano-ethyleneimine)-N-methyl-2-chloropyridine-5-methylamine, is a new kind of broad-spectrum neonicotinoid insecticide with acaricidal activity. It is a systematic insecticide of soil, branches, and leaves, and is widely used to control lepidopteron pests of rice, and planthoppers of vegetables, fruits, and tea. Hussain et al. (2016) summarized the microbial degradation process of neonicotinoids in soil and water environments through bacterial communities. The important environmental factors for the microbial degradation of xenobiotic compounds are temperature and pH (Phugare and Jadhav, 2013).
Several optimization studies of the physicochemical parameters showed that a temperature of 35°C and a pH of 7.0 was optimal for acetamiprid biodegradation. Moreover, the degradation of acetamiprid was studied under different temperatures and pH conditions by strain CS-3. The optimum pH-value for acetamiprid degradation ranged from 5.0 to 7.0, and the degradation value decreased when the pH-value was above 8.0 or below 4.0. Strain CS-3 efficiently degraded acetamiprid between 25 to 30°C, whereas the degradation rate was reduced to half at 20°C and 42°C (Shi et al., 2018).
Acetamiprid rapidly degraded through aerobic soil metabolism with a half-life of 1–8.2 days during various soil studies in the United States and Europe, whereas it took 16–17 days under the field conditions of India (Gupta and Gajbhiye, 2007). Pseudomonos sp. FH2, isolated by Yao and Min (2006), effectively degraded 96.7% acetamiprid in 14 days at pH 7 and 30°C temperature. The transformation rates of acetamiprid by Stenotrophomonas sp. THZ-XP (Tang et al., 2012) and Pigmentiphaga sp. AAP-1 (Wang et al., 2013a) were faster than other strains.
Stenotrophomonas maltophilia CGMCC 11788 had the highest imidacloprid hydroxylation activity and degraded 58.9% acetamiprid within 8 days of incubation and generated the (E)-N-[(6-chloro-3-pyridyl)-methyl]-N-cyano-acetamidine (IM 2-1) intermediate by the demethylation process (Chen et al., 2008). With the production of N-methyl-(6-chloro-3-pyridyl) methylamine, Pigmentiphaga sp. AAP-1 degraded 100 mg⋅L–1 acetamiprid within 2.5 h (Wang et al., 2013a). Yang et al. (2013) reported that 0.22 mM acetamiprid was completely degraded by Pigmentiphaga sp. D-2 after 72 h. Pseudoxanthomonas sp. AAP-7 was reported to hydrolyze or demethylate acetamiprid to 1-(6-chloropyridin-3yl)-N-methylmethanamine as an intermediate (Wang et al., 2013b). Rhodococcus sp. BCH2 was reported to degrade acetamiprid into the intermediate N-methyl (6-chloro-3-pyridyl) methylamine and 6-CNA with glucose and ammonium chloride (Phugare and Jadhav, 2013).
In addition to bacterial strains, fungi also possess better acetamiprid bioremediation potential. Fungal hyphae secrete extracellular enzymes that can penetrate deeper into the soil matrix with a larger surface area than bacterial isolates (Sun et al., 2017; Shi et al., 2018; Guo et al., 2019). Isolating from rotten wood, a white-rot fungus Phanerochaete sordida YK-624 degraded acetamiprid to IM 2-1 over 15 days (Wang et al., 2012). The yeast Rhodotorula mucilaginosa strain IM-2 degraded acetamiprid as well as thiacloprid in both cultures and soil (Dai et al., 2010).
Thiacloprid
Thiacloprid, [3-((6-chloro-3-pyridine) methyl)-1,3-thiazolin-2-subunit] melamine, is a chlorinated nicotinoid insecticide especially used to manage stinging and chewing insects. The mechanism of this pesticide is different from traditional pesticides as it affects the articulation of the posterior nerve membrane in insects. Nicotinic acetylcholine receptors interfere with normal conduction to block nerve channels in the insect nervous system. This results in a substantial accumulation of acetylcholine that severely excites the insect’s body to cause body spasms and paralysis leading to death. It quickly produces strong shock, stomach poison, and internal absorption for a longer duration.
Under the soil, laboratory, or field conditions, the half-life of thiameacloprid ranged from 5 to 27 days, indicating that its transformation was strongly affected by soil microbial activity (Liu et al., 2011). Two bacterial strains, Variovorax boronicumulans CGMCC 4969 and Ensifer meliloti CGMCC 7333, and a yeast, Rhodotorula mucilaginosa IM-2, hydrolyzed thiacloprid to thiacloprid amide with half-lives of 14.3 days, 1.8 days, and 20.9 h, respectively (Zhao et al., 2009; Liu et al., 2011; Zhang et al., 2012; Ge et al., 2014).
Maltophilia oligotrophomonas CGMCC 1.1788 degraded 90.5% of 0.63 mmol⋅L–1 thiacloprid into 4-hydroxyl mercaptoimidacloprid through hydroxylation, within 30 h at a half-life of 9 h. However, at higher concentrations, the rate of transformation was very slow and after 5 days of culture, only 24.2% thiacloprid was converted (Zhao et al., 2009). Microvirga flocculans CGMCC 1.16731, an N2-fixing bacterium, is not only a biofertilizer agent but also effectively degrades thiacloprid and acetamiprid through NHase mediated transformation (Zhao et al., 2019). This bacterium belongs to the genus Proteus, a recently discovered root nodule bacterium, whose environmental functions are still poorly understood (Tomasz et al., 2018).
Thiamethoxam
Thiamethoxam,3-(2-chloro-1,3-thiazol-5-yl-methyl)-5-methyl-1,3,5-oxadiazinan-4-ylidene (nitro) amine, is another neonicotinoid insecticide that shares similar mechanisms with imidacloprid and has no interactive resistance to imidacloprid, idinidine, or alkenididine. The metabolism of microbial degradation of thiamethoxam in soil and liquid culture has only been reported through a few species, including Ensifer adhaerens TMX-23, a nitrogen-fixing and plant-growth-promoting rhizobacterium (Zhou et al., 2013), Pseudomonas sp. (Pandey et al., 2009), Bacillus amyloliquefaciens IN937a, Bacillus pumilus SE34, Sphingomonas sp. TY, and nicotine-degrading bacteria Acinetobacter sp. TW (Wang et al., 2011; Rana et al., 2015). Aerophilus sp. IMBL 4.1 and Pseudomonas putida IMBL 5.2 showed significantly higher thiamethoxam degradation potential and were able to grow under oscillating conditions of temperature and pH (Rana et al., 2015). Ensifer adhaerens TMX-23 possesses bioaugmentation potential and promoted the growth of field crops in soil contaminated with thiamethoxam (Zhou et al., 2013).
Clothianidin
Clothianidin, (E)-1-(2-chloro-1,3-thiazol-5-yl-methyl)-3-methyl2-nitroguanidine, is a kind of new nicotinic insecticide with high efficiency, safety, and selectivity. Its effect was similar to that of the nicotinic acetylcholine receptor and caused shock, gastric toxicity, and internal absorption. Clothianidin is mainly used in rice, vegetables, fruit trees, and other crops to control hemipteran, coleopteran, dipteran, and some lepidopteron pests. The microbial degradation of clothianidin under aerobic and anaerobic conditions was studied. The rate constant (k) and half-life (DT50) of aerobic and anaerobic microorganisms were determined, and enrichment experiments were carried out under different nutritional conditions.
Microbial growth was assessed at different pesticide concentrations and temperatures (Mulligan et al., 2016). Mori et al. (2017) reported the microbial biodegradation of clothianidin. They found that 37% of the clothianidin was degraded by the white-rot fungus Phanerochaete sordida during 20 days of cultivation. This revealed that the degradation process of clothianidin in sordida is similar to that of mice but the microbial metabolism of clothianidin has not been described well. Pseudomonas stutzeri smk aerobically degraded 62% of clothianidin within 14 days at 30°C, which is faster than reported earlier (Parte and Kharat, 2019).
Nitenpyram
Nitenpyram (NIT), [(E)-N-(6-chloro-3-pyridylmethyl)-N-ethyl-N0-methyl-2-nitrovinylidenediamine], is a new nicotinoid insecticide product developed in Japan after imidacloprid and acetamiprid. It shares excellent absorbability, osmotic action, and wide insecticidal spectrum properties. It is less harmful and safer than imidacloprid; thus, it can be frequently used to prevent and control pests of stinging mouthparts, such as whiteflies, aphids, pear psyllids, leafhoppers, thrips, etc (Wang J. et al., 2019). Until now, only one fungal strain belonging to white-rot fungus Phanerochaete sordida YK-624 was reported to degrade nitenpyramm. Under ligninolytic conditions, P. sordida YK-624 completely degraded nitenpyram while there was only a 20% decrease under non-ligninolytic conditions. However, the microbial degradation mechanisms of nitenpyram are not yet explored (Wang J. et al., 2019).
Dinotefuran
Dinotefuran (DIN), N-methyl-N0-nitro-N00-[(tetrahydro-3-furanyl) methyl] guanidine, is another nicotinoid insecticide, with high insecticidal activity at very low doses. Like nitenpyram, it is also very safe for mammals and not well reported for microbial degradation. However, a similar white-rot fungus, P. sordida YK-624, was found capable of degrading 31% of dinotefuran in 20 days under ligninolytic conditions. P. sordida YK-624 did not degrade dinotefuran without ligninolytic conditions. The microbial degradation mechanisms of dinotefuran are not yet understood (Wang J. et al., 2019).
Metabolic Pathways of Neonicotinoid Degradation in Microorganisms
Imidacloprid
Many imidacloprid-degrading microorganisms and their reported metabolic pathways are presented in Figure 1. Oxidation and nitro-reduction are two major microbial biodegradation pathways of imidacloprid (Akoijam and Singh, 2015; Lu et al., 2016; Fusetto et al., 2017). The products produced by light or water degradation and plant metabolisms include imidacloprid urea, 6-chloronicotinic aldehyde, 6-chloro-N-methylnicotinacidamide, 6-chloronicotinic acid, etc. Soil microbial studies indicate that these products may be metabolites of imidacloprid biodegradation (Sabourmoghaddam et al., 2015).
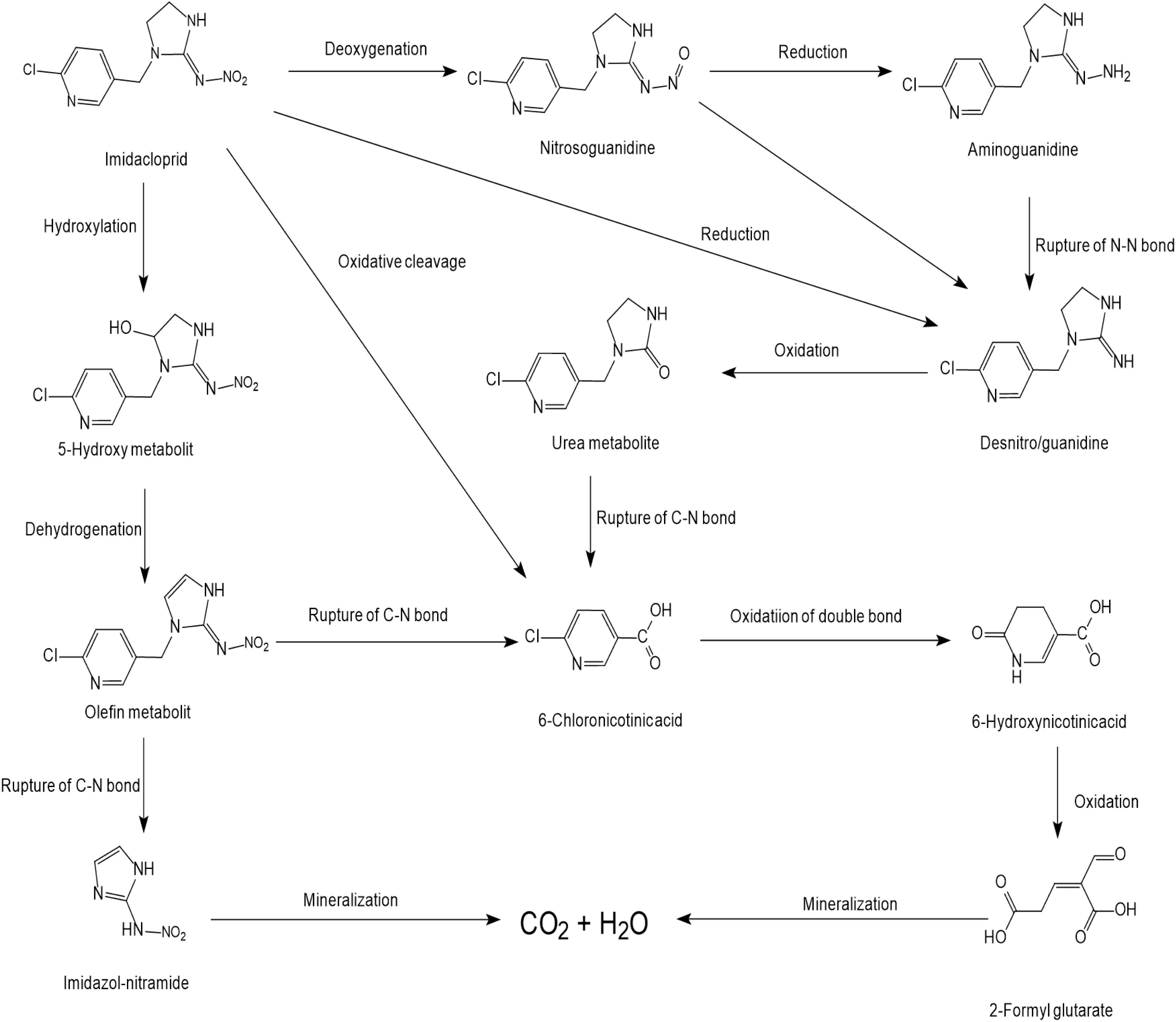
Figure 1. Microbial degradation pathways of imidacloprid (based on Akoijam and Singh, 2014; Sharma et al., 2014; Sabourmoghaddam et al., 2015).
Among the residues of imidacloprid metabolic products in soil, urea is mostly found followed by 6-CNA, 5-hydroxy, olefine, nitrosimine, and nitroguanidine. These residues were not detectable after 60 to 90 days in the second use of imidacloprid (Akoijam and Singh, 2014). Nitro-reduction is the most common and effective approach for imidacloprid bioremediation (Pandey et al., 2009; Sharma et al., 2014).
Initially, two oxygen atoms are removed from imidacloprid to form nitrosoguanidine and aminoguanidine, respectively. The cleavage of the N–N bond produces matter that can form a desnitro/guanidine intermediate with ten times the toxicity to imidacloprid. Intermediate further oxidizes to non-toxic metabolite urea (Pandey et al., 2009; Phugare et al., 2013). In different bacterial systems, matter transforms into 6-chloronicotinic acid due to the cleavage of the C–N bond (Sharma et al., 2014). 6-chloronicotinic acid is easy to decompose organic matter, which generates CO2 and H2O after oxidation.
The oxidation pathway is less responsive in the microbial systems and generates comparatively fewer degradation products. Imidacloprid forms 5-hydroxy metabolites and olefin metabolites via ethylene hydroxylation and the dehydrogenation pathway (Dai et al., 2006; Ma et al., 2014). The carbon atom of the tertiary amine is connected with the 6-chloro-3-pyridinylmethyl moiety of imidacloprid, which is an important active site of hydroxylation (Dai et al., 2010). After the cleavage of the C–N bond, the olefin metabolite is converted to imidazole-nitramide and 6-chloronicotinic acid. Similarly, this mechanism of imidacloprid degradation also exists in animals and several plant species (Ford and Casida, 2006; Thurman et al., 2013). In addition to this, the Cyp6g1 gene found in drosophila plays a crucial part in the degradation of imidacloprid in animals to control and promote the production of metabolites in the oxidation pathway (Fusetto et al., 2017).
Acetamiprid
Several articles have reported the microbial degradation of acetamiprid, as shown in Figure 2 (Tang et al., 2012; Wang et al., 2013a, b; Yang et al., 2013; Shi et al., 2018). Microbial growth mainly depends on the availability of nutrients and their metabolic activity is regulated by the nutrient state of the medium (Phugare and Jadhav, 2013). The insecticidal selectivity of acetaminidine depends on the substituent = NCN and this functional group also initiates the degradation. Generally, the C≡N of acetamiprid is oxidized and fractured to generate N-amidoamide derivative. Due to the asymmetric cleavage, product is degraded to N-methyl-(6-chloro-3-pyridyl) methylamine and (Z)-1-ethylideneurea. The intermediate product quickly generates 6-chloronicotinic acid, which is finally mineralized to H2O and CO2 (Figure 3; Phugare and Jadhav, 2013; Sun S. et al., 2018).
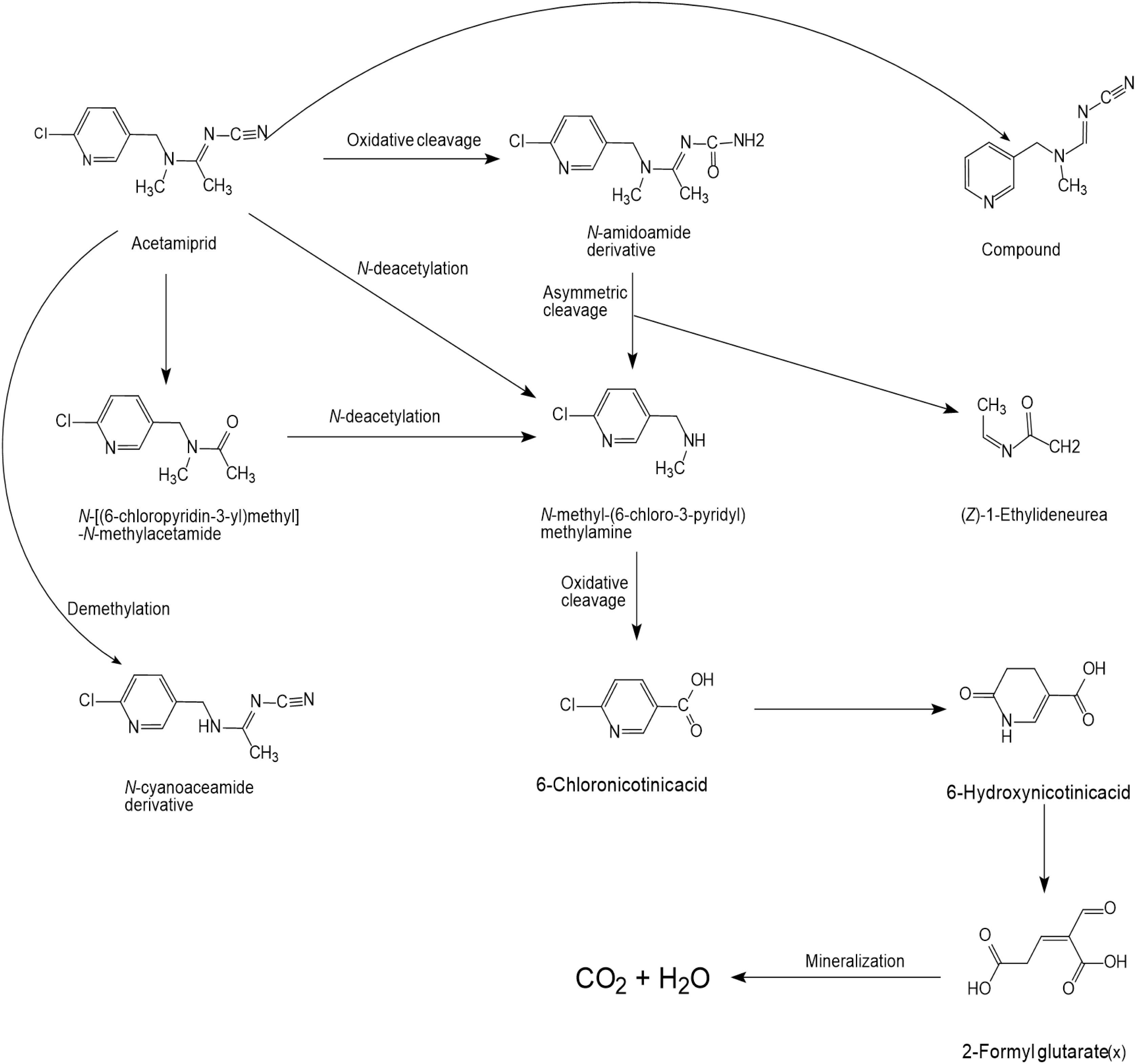
Figure 2. Microbial degradation pathways of acetamiprid (based on Phugare and Jadhav, 2013; Sun S. et al., 2018).
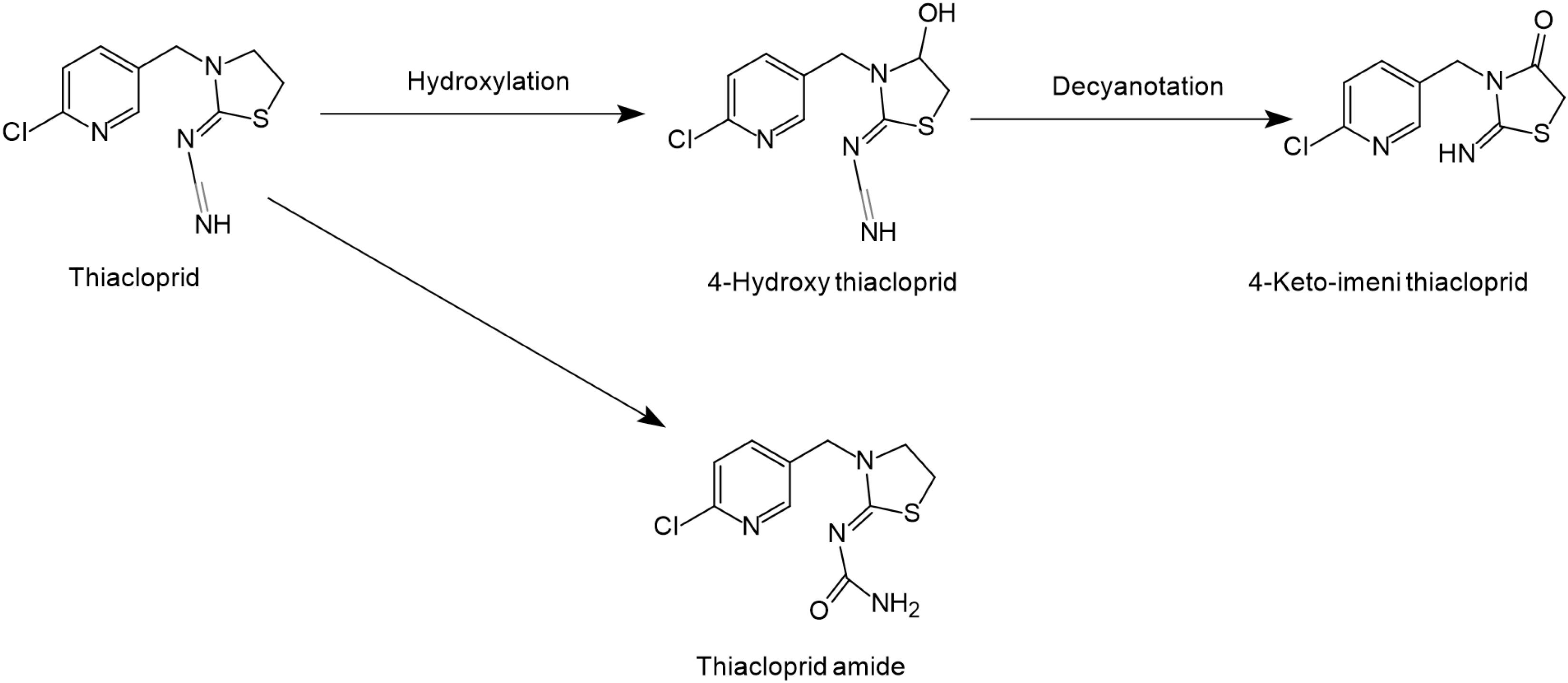
Figure 3. Microbial degradation pathways of thiacloprid (based on Zhao et al., 2009; Thurman et al., 2013).
Tang et al. (2012) found that Stenotrophomonas sp. THZ-XP transformed acetamiprid into N-methyl-(6-chloro-3-pyridyl) methylamine through the intermediate product. Wang et al. (2013a, b) found that acetamiprid can be directly converted into a product without generating any intermediate products. Interestingly, another pathway was reported in Ochrobactrum sp. D-12 to directly dechlorinate and demethylate acetamiprid into the product (Yang et al., 2013).
Stenotrophomonas maltophilia CGMCC 1.1788 transformed acetamiprid to a polar metabolite by reducing its one carbon and two hydrogen atoms. Demethylation of acetamiprid generated a N-cyanoaceamide derivative that has a lower insecticidal activity (Chen et al., 2008). Interestingly, a common inhibitor of cytochrome P450, known as piperonyl butoxide, restrained the N-demethylation of acetamiprid (Chen et al., 2008). However, the microbial system could not transform the N-cyanoaceamide derivative into other products and was only found in animal and plant systems (Brunet et al., 2005).
Acetamiprid metabolism in plants, animals, and soil has been clearly understood along with some examples of microbial degradation. Moreover, ammonase activity, found in many microorganisms, plays an important role in the industrial synthesis of amides. However, the catalytic efficiency and biochemical and structural characteristics of NHase enzymes related to the biodegradation of nitrogenous organic pollutants (especially nitrogenous pesticides) have not been thoroughly studied (Guo et al., 2019). The enzymatic mechanism by which potentially useful environmental microorganisms degrade acetamiprid has not been clearly described (Zhou et al., 2014).
Thiacloprid
The structure of thiacloprid is similar to acetamiprid and therefore very limited literature is available regarding its microbial biodegradation pathways (Figure 3). Moreover, the degradation pathway of acetamiprid can be used as a reference in degradation studies of thiacloprid. Microbial systems release the cyano group of thiacloprid and oxidize hydroxyl group to the carbonyl group to generate 4-hydroxy thiacloprid. 4-hydroxy thiacloprid is rapidly converted into 4-keto-imeni thiacloprid by the decyanotation process. In these two steps of detoxification, thiacloprid loses the cyano and hydroxyl groups (Zhao et al., 2009). Ge et al. (2014) reported that thiacloprid was converted to thiacloprid amide via oxidative cleavage. In addition, a few studies have also revealed that plants and animals can metabolize thiacloprid but subsequent degradation products have not been reported (Thurman et al., 2013).
Thiamethoxam
Thiamethoxam degradation has been reported in many plants and animals but only a few biodegradation studies have been conducted in microbial systems (Nauen et al., 2003; Ford and Casida, 2008). Microorganisms can degrade thiamethoxam via the nitro reduction metabolic pathway to form metabolites, such as nitrosoguanidine/nitrosamine, amino-guanidine, desnitro/guanidine/imine and urea (Pandey et al., 2009; Zhou et al., 2013). Zhou et al. (2014) reported a biodegradation mechanism by Ensifer adhaerens TMX-23 where the cleavage of oxadiazine and hydrogenated methyltriazinone finally converted thiamethoxam into clothianidin-triazinones. Zhou et al. (2014) also reported a biodegradation pathway similar to acetamiprid where thiamethoxam was converted to desmethyl-thiamethoxam through the demethylation pathway. However, the final degradation products were not found in this pathway (Figure 4).
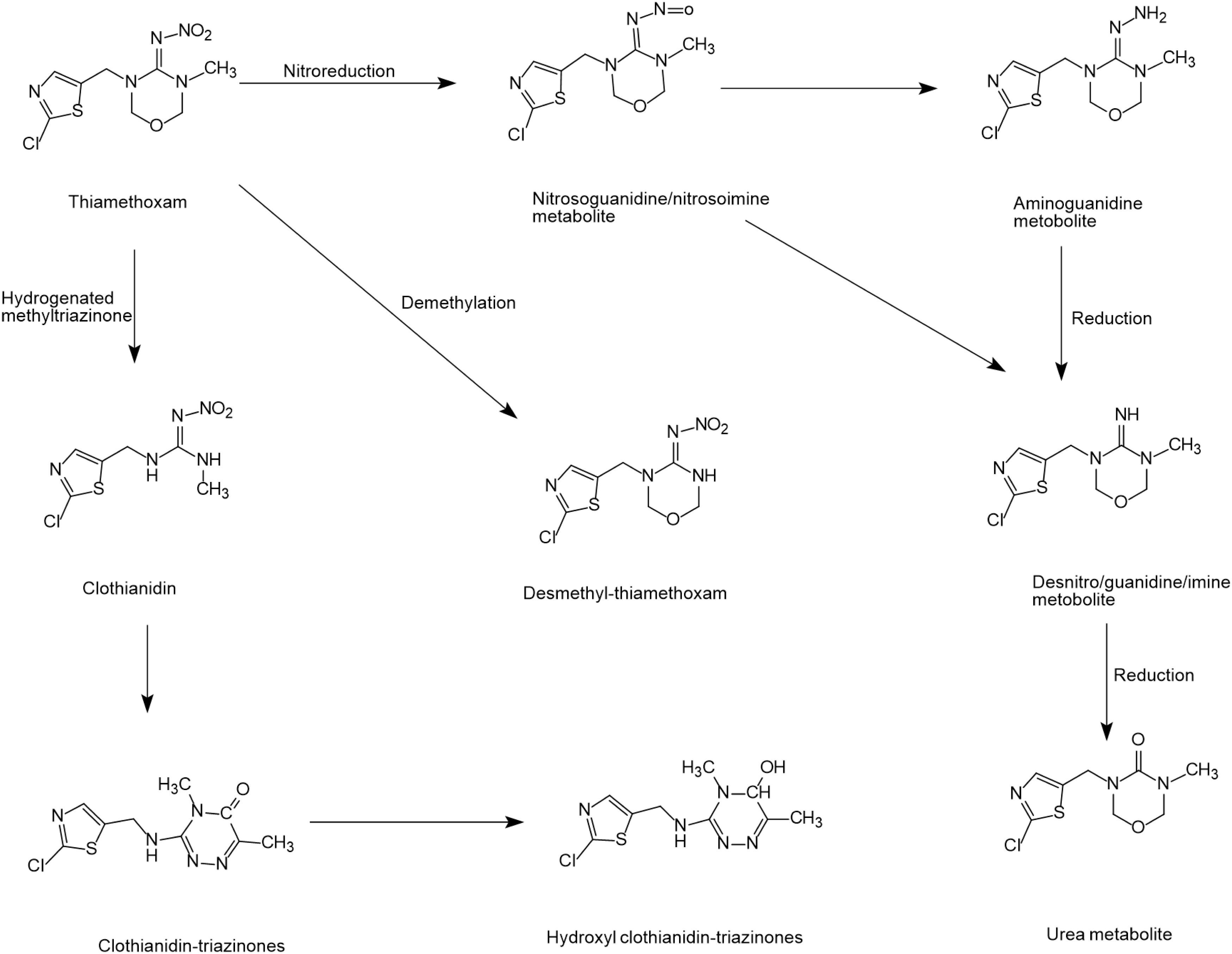
Figure 4. Microbial degradation pathways of thiamethoxam (based on Pandey et al., 2009; Zhou et al., 2014).
Clothianidin
The microbial degradation pathway for clothianidin has only been reported in a few articles, as shown in Figure 5. The cleavage of the C-N bonds between thiazolyl methyl and the guanidine moieties was found to release carbon for the conversion of clothianidin to 2 chloro-5-methyl thiazole and methyl nitroguanidine (Parte and Kharat, 2019). Microbial systems gradually degrade clothianidin to ((2-chlorothiazol-5-yl)methyl)-3-methylguanidine and methyl-3-((thiazol-5-yl) methyl) guanidine through denitrification and dehalogenation. Similar to thiamethoxam and thiacloprid, subsequent degradation products of clothianidin were also not found.
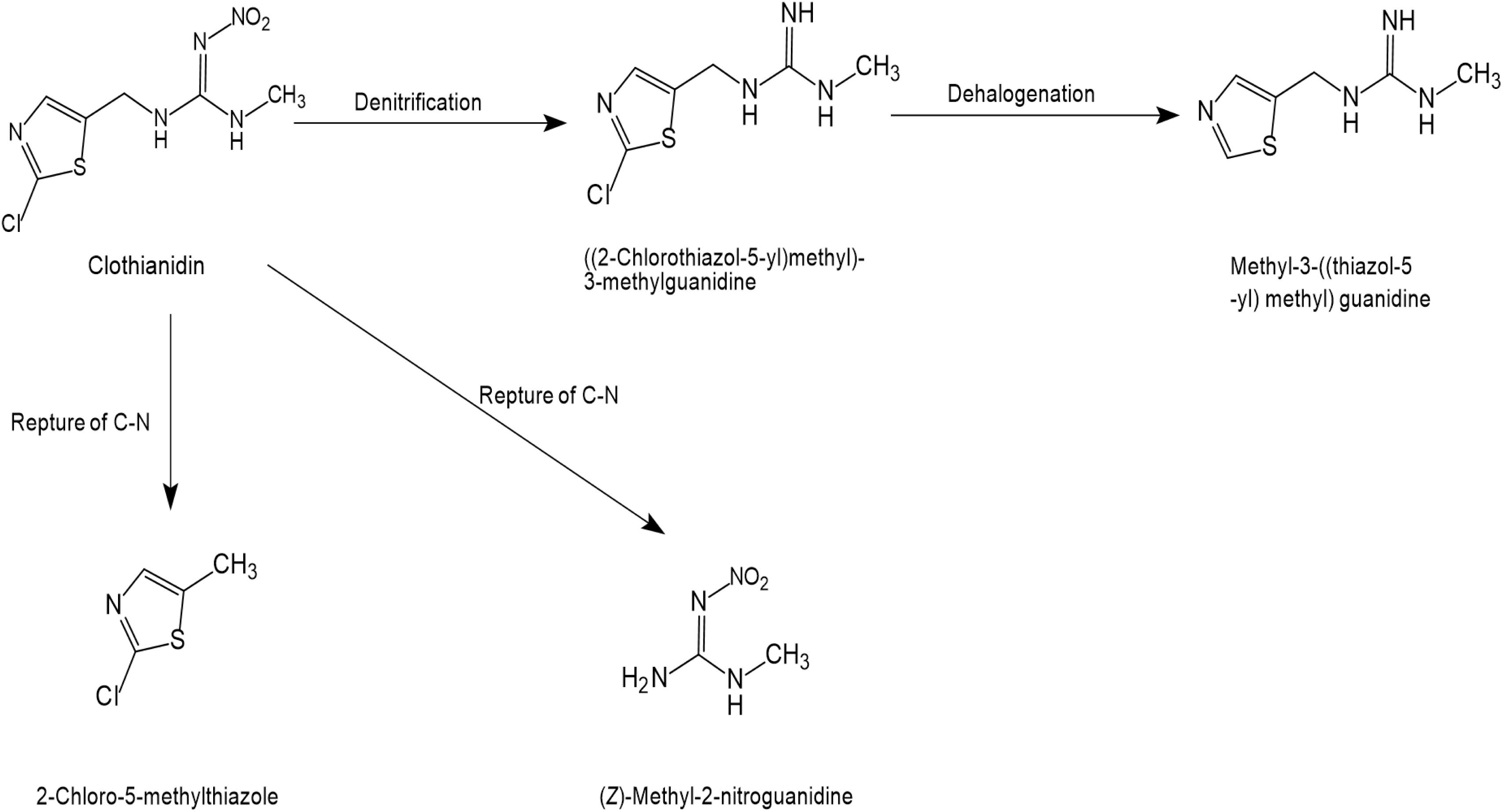
Figure 5. Microbial degradation pathways of clothianidin (based on Parte and Kharat, 2019).
Molecular Biology of Neonicotinoid Degradation
Pesticides are frequently used in crops to protect them from harmful insects and to increase their productivity and yields. However, the excessive use of pesticides can reduce the activity of soil enzymes, which is a key indicator of soil health. Enzymes plays an important role in the biodegradation of natural and man-made organic compounds in soil and are often used to indicate changes in the soil environments under the action of pesticides and fertilizers. Enzyme activity is closely correlated to microbial activity. To evaluate the effects of pesticides, molecular techniques were used to research the changes in microbial community structure and function (Parween et al., 2016).
The use of molecular tools to identify related genes/enzymes and ineffective bacteria/fungi is of great significance for large-scale and effective bioremediation in pesticide contamination sites. By exploring the molecular basis of biodegradation of pesticides by soil microorganisms, the roles of degrading genes and the application of recombinant DNA technology were reported. Molecular biology techniques provide a more comprehensive explanation of in situ microbial communities than standard microbiological methods. At present, the degradation technologies mainly include gel electrophoresis (DGGE), restriction fragment length polymorphism (RFLP), dot blot, Southern blot, PCR amplification, the preparation of metagenomic libraries, the subsequent analysis of bacterial rRNA genes, microarrays, and omics technologies (Parween et al., 2016).
Advances in genomics accelerated the investigation of the novel neonicotinoid degrading gene families. During degradation, the main target for neonicotinoids is the α and β helix proteins (Chen et al., 2017). Many previous researchers explored the details regarding the molecular sites for the neonicotinoids (Matsuda et al., 2020). The biodegradation ability of microorganisms is the key factor in degrading toxic pollutants from the environment. The biodegradation potential of an organism is decided by the genetic content inside individual cells (Feng et al., 2020b). Deoxyribose nucleic acid (DNA) codes the information in the form of mRNA that is further converted to specific degradative enzymes.
During microbial degradation, neonicotinoid degrading enzymes can be up-regulated or down-regulated. To date, the complete degradation of neonicotinoids has not been emphasized. The Hymenobacter latericoloratus CGMCC 16346 has been characterized for the degradation of the imidacloprid. This bacterial strain can degrade the imidacloprid through hydroxylation. The whole genome of this strain has been sequenced and compared with other imidacloprid degrading microbial strains. Both chromosome and plasmid genes were shown to participate in the degradation of imidacloprid (Guo et al., 2020).
In insects, detoxifying enzymes, such as esterase, glutathione S-transferase, and P450s, can catabolize insecticides and toxins. These enzymes, involving point mutations in the target gene to the insecticide, could increase the copy number, mRNA levels, and coding sequence diversity of the response gene (Li et al., 2007). The cytochrome P450 is a large, well-defined family of monooxygenases. Their potential has long been recognized in many industrial processes, particularly because of their ability to utilize molecular oxygen for the oxidation or hydroxylation of substrates (Scott et al., 2008). The P450 enzyme carries out various functions, including biosynthesis and metabolism of alien organisms. The insect genome has been found to contain from 46 to more than 150 P450 genes, and each can translate a different P450 enzyme (Table 4).
At present, the overexpression of one or more P450 enzymes appears to be the main pathway of pest resistance against new toxoid (Nelson, 2009). In insects, resistance genes were noted to be responsible for neonicotinoid degradation, and similar genes carry out the degradation of neonicotinoids in bacteria. Studies regarding neonicotinoid metabolites and the total enzyme activity have revealed that the development of resistance against this insecticide group is due to P450 monooxygenase rather than mutations of nAChR (Rauch and Nauen, 2003). Several P450 genes and enzymes of the CYP6 and CYP3 clade such as P450 CYP6ER1, CYP6G1, CYP6Y3, and CYP353D1v2 have been well reported for their role in neonicotinoid resistance.
P450 CYP6ER1 was connected with the resistance of imidacloprid resistance in Nilaparvata lugens, where amino acid substitutions in the binding site directly contributed to enhancing the metabolism of imidacloprid (Bao et al., 2016; Pang et al., 2016). The CYP6G1 enzyme was used to characterize and quantify the imidacloprid metabolism. It highlights the importance of undetermined transporters in response to imidacloprid by producing toxic metabolites, which were easily excreted (Fusetto et al., 2017). Different from Nilaparvata lugens CYP6ER1, all studied CYP6CM1 variants in Bemisia tabaci yielded similar levels of imidacloprid metabolism (Hamada et al., 2019). Puinean et al. (2010) indicated that CYP6Y3 could confer resistance to neonicotinoid in Myzus persicae (Puinean et al., 2010). CYP353D1v2 was found to over-express in different strains of imidacloprid-resistant whoes striatellus and the RNAi of this gene could significantly suppress the resistance (Elzaki et al., 2017). In the overexpressed P450 gene of the CYP3 clade, successful inhibition of CYP6CY14 transcription by RNAi significantly increased the susceptibility of pesticide-resistant cotton aphids to thiamethoxam (Wu et al., 2018).
Conclusion and Future Prospects
This review comprehensively summarized the microbial degradation and biochemical mechanisms of neonicotinoids. Neonicotinoid pesticides are commonly used in agricultural sectors due to their effective insecticidal properties. However, the deep-rooted negative environmental effects of neonicotinoids should be given serious attention to remove these residues from polluted environments. The application of efficient neonicotinoid-degrading microorganisms in contaminated environments is considered to be the most promising remediation strategy. The toxicity and microbial degradation pathways of imidacloprid, acetamiprid, thiacloprid, thiamethoxam, and clothianidin in neonicotinoids have been clearly understood; however, there are only a few studies regarding nitenpyram and dinotefuran.
The majority of the neonicotinoid degradation intermediates (especially of imidacloprid) are more toxic than their parent compound. Researchers have explored potent microorganisms from pesticide-contaminated agricultural soils, wastewater, rhizospheric soils, and microbial preservation centers. It is noteworthy that none of the single bacterial isolates has been able to fully mineralize imidacloprid, acetamiprid, thiacloprid, thiamethoxam, or clothianidin. Imidacloprid and acetamiprid can be completely co-degraded into carbon dioxide and water by different microorganisms. The isolation or design of such bacteria is critical for the long-term success of biologically mediated environmental degradation of imidacloprid and other neonicotinoids.
To date, only a few reports have conclusively determined the degradation pathways and their associated enzymes. To treat neonicotinoids systematically, the bioremediation potential of degrading microorganisms and enzymes deserves more research. To understand the degradation mechanisms in a contaminated environment, it is very important to study functional genes and enzymes. An immense amount of neonicotinoid-degrading microorganisms have been affirmed; however, few studies have been conducted on their functional genes and enzymes. Therefore, detailed basic work should be carried out before large-scale applications of neonicotinoid-degrading microorganisms for bioremediation. The results of previous studies indicated that high-output sequencing methods may be helpful for the complete annotations of the genes and metabolites produced during microbial degradation.
Author Contributions
SC conceived of the presented idea. SP contributed to the writing and prepared the figures and tables. ZL, WZ, SM, PB, and SC participated in revising the manuscript. All authors approved it for publication.
Funding
We acknowledge the grants from the Key Area Research and Development Program of Guangdong Province (2018B020206001), the National Natural Science Foundation of China (31401763), and the Guangdong Special Branch Plan for Young Talent with Scientific and Technological Innovation (2017TQ04N026).
Conflict of Interest
The authors declare that the research was conducted in the absence of any commercial or financial relationships that could be construed as a potential conflict of interest.
References
Akoijam, R., and Singh, B. (2014). Metabolic degradation of imidacloprid in paddy field soil. Environ. Monit. Assess. 186, 5977–5984. doi: 10.1007/s10661-014-3833-3
Akoijam, R., and Singh, B. (2015). Biodegradation of imidacloprid in sandy loam soil by Bacillus Aerophilus. Intern. J. Environ. Anal. Chem. 95, 730–743. doi: 10.1080/03067319.2015.1055470
Anhalt, J. C., Moorman, T. B., and Koskinen, W. C. (2007). Biodegradation of imidacloprid by an isolated soil microorganism. J. Environ. Sci. Heal. B 42, 509–514. doi: 10.1080/03601230701391401
Arora, P. K., Srivastava, A., Garg, S. K., and Singh, V. P. (2017). Recent advances in degradation of chloronitrophenols. Bioresour. Technol. 250, 902–909. doi: 10.1016/j.biortech.2017.12.007
Babeíová, J., Šefčíková, Z., Čikoš, Š, Špirková, A., Kovaříková, V., Koppel, J., et al. (2017). Exposure to neonicotinoid insecticides induces embryotoxicity in mice and rabbits. Toxicology 392, 71–80. doi: 10.1016/j.tox.2017.10.011
Bao, H., Gao, H., Zhang, Y., Fan, D., Fang, J., and Liu, Z. (2016). The roles of CYP6AY1 and CYP6ER1 in imidacloprid resistance in the brown planthopper: expression levels and detoxification efficiency. Pestic. Biochem. Phys. 129, 70–74. doi: 10.1016/j.pestbp.2015.10.020
Bartlett, A. J., Hedges, A. M., Intini, K. D., Brown, L. R., Maisonneuve, F. J., Robinson, S. A., et al. (2018). Lethal and sublethal toxicity of neonicotinoid and butenolide insecticides to the mayfly, Hexagenia spp. Environ. Pollut. 238, 63–75. doi: 10.1016/j.envpol.2018.03.004
Bartlett, A. J., Hedges, A. M., Intini, K. D., Brown, L. R., Maisonneuve, F. J., Robinson, S. A., et al. (2019). Acute and chronic toxicity of neonicotinoid and butenolide insecticides to the freshwater amphipod, Hyalella azteca. Ecotoxicol. Environ. Saf. 175, 215–223. doi: 10.1016/j.ecoenv.2019.03.038
Bhatt, P., Bhatt, K., Huang, Y., Lin, Z., and Chen, S. (2020a). Esterase is a powerful tool for the biodegradation of pyrethroid insecticides. Chemosphere 244:125507. doi: 10.1016/j.chemosphere.2019.125507
Bhatt, P., Huang, Y., Rene, E. R., Kumar, A. J., and Chen, S. (2020b). Mechanism of allethrin biodegradation by a newly isolated Sphingomonas trueperi strain CW3 from wastewater sludge. Bioresour. Technol. 305:123074. doi: 10.1016/j.biortech.2020.123074
Bhatt, P., Huang, Y., Zhan, H., and Chen, S. (2019). Insight into microbial applications for the biodegradation of pyrethroid insecticides. Front. Microbiol. 10:1778. doi: 10.3389/fmicb.2019.01778
Bhatt, P., Zhang, W., Lin, Z., Pang, S., Huang, Y., and Chen, S. (2020c). Biodegradation of allethrin by a novel fungus Fusarium proliferatum strain CF2, isolated from contaminated soils. Microorganisms 8:593. doi: 10.3390/microorganisms8040593
Birolli, W. G., Vacondio, B., Alvarenga, N., Seleghim, M. H. R., and Porto, A. L. M. (2018). Enantioselective biodegradation of the pyrethroid (±)-lambda-cyhalothrin by marine-derived fungi. Chemosphere 197, 651–660. doi: 10.1016/j.chemosphere.2018.01.054
Bivehed, E., Gustafsson, A., Berglund, A., and Hellman, B. (2019). Evaluation of potential DNA-damaging effects of nitenpyram and imidacloprid in human U937-cells using a new statistical approach to analyse comet data. Expo. Health 305, 90–101. doi: 10.1007/s12403-019-00328-6
Brunet, J., Badiou, A., and Belzunces, L. P. (2005). In vivo metabolic fate of 14C-acetamiprid in six biological compartments of the honeybee, Apis mellifera L. Pest Manag. Sci. 61, 742–748. doi: 10.1002/ps.1046
Calvo-Agudo, M., González-Cabrera, J., Picó, Y., Calatayud-Vernich, P., Urbaneja, A., Dicke, M., et al. (2019). Neonicotinoids in excretion product of phloem-feeding insects kill beneficial insects. Proc. Natl. Acad. Sci. U.S.A. 116, 16817–16822. doi: 10.1073/pnas.1904298116
Caron-Beaudoin, E., Viau, R., Hudon-Thibeault, A., Vaillancourt, C., and Sanderson, J. T. (2017). The use of a unique co-culture model of fetoplacental steroidogenesis as a screening tool for endocrine disruptors: the effects of neonicotinoids on aromatase activity and hormone production. Toxicol. Appl. Pharm. 332, 15–24. doi: 10.1016/j.taap.2017.07.018
Caron-Beaudoin, E., Viau, R., and Sanderson, J. T. (2018). Effects of neonicotinoid pesticides on promoter-specific aromatase (CYP19) expression in Hs578t breast cancer cells and the role of the VEGF pathway. Environ. Heal. Perspect. 126:047014. doi: 10.1289/EHP2698
Casida, J. E. (2017). Why prodrugs and propesticides succeed. Chem. Res. Toxicol. 30, 1117–1126. doi: 10.1021/acs.chemrestox.7b00030
Chen, S., Chang, C., Deng, Y., An, S., Dong, Y. H., Zhou, J., et al. (2014). Fenpropathrin biodegradation pathway in Bacillus sp. DG-02 and its potential for bioremediation of pyrethroid-contaminated soils. J. Agric. Food Chem. 62, 2147–2157. doi: 10.1021/jf404908j
Chen, S., Deng, Y., Chang, C., Lee, J., Cheng, Y., et al. (2015). Pathway and kinetics of cyhalothrin biodegradation by Bacillus thuringiensis strain ZS-19. Sci. Rep. 5:8784. doi: 10.1038/srep08784
Chen, S., Dong, Y. H., Chang, C., Deng, Y., Zhang, X. F., Zhong, G., et al. (2013). Characterization of a novel cyfluthrin-degrading bacterial strain Brevibacterium aureum and its biochemical degradation pathway. Bioresour. Technol. 132, 16–23. doi: 10.1016/j.biortech.2013.01.002
Chen, S., Geng, P., Xiao, Y., and Hu, M. (2012). Bioremediation of β-cypermethrin and 3-phenoxybenzaldehyde contaminated soils using Streptomyces aureus HP-S-01. Appl. Microbiol. Biotechnol. 94, 505–515. doi: 10.1007/s00253-011-3640-5
Chen, S., Hu, M., Liu, J., Zhong, G., Yang, L., Rizwan-ul-Haq, M., et al. (2011). Biodegradation of beta-cypermethrin and 3-phenoxybenzoic acid by a novel Ochrobactrum lupini DG-S-01. J. Hazard. Mater. 187, 433–440. doi: 10.1016/j.jhazmat.2011.01.049
Chen, T., Dai, Y., Ding, J., Yuan, S., and Ni, J. (2008). N-demethylation of neonicotinoid insecticide acetamiprid by bacterium Stenotrophomonas maltophilia CGMCC 1.1788. Biodegradation 19, 651–658. doi: 10.1007/s10532-007-9170-2
Chen, X., Li, F., Chen, A., Ma, K., Liang, P., Liu, Y., et al. (2017). Both point mutations and low expression levels of the nicotinic acetylcholine receptor β1 subunit are associated with imidacloprid resistance in an Aphis gossypii (Glover) population from a Bt cotton field in China. Pestic. Biochem. Phys. 141, 1–8. doi: 10.1016/j.pestbp.2016.11.004
Christen, V., Schirrmann, M., Frey, J. E., and Fent, K. (2018). Global transcriptomic effects of environmentally relevant concentrations of the neonicotinoids clothianidin, imidacloprid, and thiamethoxam in the brain of honey bees (Apis mellifera). Environ. Sci. Technol. 52, 7534–7544. doi: 10.1021/acs.est.8b01801
Christy, A. M., Pierre, M., and James, H. D. (2015). Neonicotinoid contamination of global surface waters and associated risk to aquatic invertebrates: a review. Environ. Int. 74, 291–303. doi: 10.1016/j.envint.2014.10.024
Colin, T., Meikle, W. G., Wu, X., and Barron, A. B. (2019). Traces of a neonicotinoid induce precocious foraging and reduce foraging performance in honey bees. Environ. Sci. Technol. 53, 8252–8261. doi: 10.1021/acs.est.9b02452
Conde-Avila, V., Ortega-Martínez, L. D., Loera, O., El Kassis, E. G., Dávila, J. G., Valenzuela, C. M., et al. (2020). Pesticides degradation by immobilised microorganisms. Int. J. Environ. Anal. Chem. doi: 10.1080/03067319.2020.1715375 [Epub ahead of print].
Cycoñ, M., and Piotrowska-Seget, Z. (2016). Pyrethroid-degrading microorganisms and their potential for the bioremediation of contaminated soils: a review. Front. Microbiol. 7:1463. doi: 10.3389/fmicb.2016.01463
Cycoñ, M., Mrozik, A., and Piotrowska-Seget, Z. (2017). Bioaugmentation as a strategy for the remediation of pesticide-polluted soil: a review. Chemosphere 172, 52–71. doi: 10.1016/j.chemosphere.2016.12.129
Dai, Y., Yuan, S., Ge, F., Chen, T., Xu, S., and Ni, J. (2006). Microbial hydroxylation of imidacloprid for the synthesis of highly insecticidal olefin imidacloprid. Appl. Microbiol. Biot. 71, 927–934. doi: 10.1007/s00253-005-0223-3
Dai, Y., Zhao, Y., Zhang, W., Yu, C., Ji, W., Xu, W., et al. (2010). Biotransformation of thianicotinyl neonicotinoid insecticides: diverse molecular substituents response to metabolism by bacterium Stenotrophomonas maltophilia CGMCC 1.1788. Bioresour. Technol. 101, 3838–3843. doi: 10.1016/j.biortech.2010.01.069
Dankyi, E., Gordon, C., Carboo, D., Apalangya, V. A., and Fomsgaard, I. S. (2018). Sorption and degradation of neonicotinoid insecticides in tropical soils. J. Environ. Sci. Heal. B 53, 587–594. doi: 10.1080/03601234.2018.1473965
Ellis, C., Park, K. J., Whitehorn, P., David, A., and Goulson, D. (2017). The neonicotinoid insecticide thiacloprid impacts upon bumblebee colony development under field conditions. Environ. Sci. Technol. 51, 1727–1732. doi: 10.1021/acs.est.6b04791
Elzaki, M. E. A., Miah, M. A., Wu, M., Zhang, H., Pu, J., Jiang, L., et al. (2017). Imidacloprid is degraded by CYP353D1v2, a cytochrome P450 overexpressed in a resistant strain of laodelphax Striatellus. Pest. Manag. Sci. 73, 1358–1363. doi: 10.1002/ps.4570
Feng, Y., Huang, Y., Zhan, H., Bhatt, H., and Chen, S. (2020a). An overview of strobilurin fungicide degradation: current status and future perspective. Front. Microbiol. 10:389. doi: 10.3389/fmicb.2020.00389
Feng, Y., Zhang, W., Pang, S., Lin, Z., Zhang, Y., Huang, Y., et al. (2020b). Kinetics and new mechanism of azoxystrobin biodegradation by an Ochrobactrum anthropi strain SH14. Microorganisms 8:625. doi: 10.3390/microorganisms8050625
Fida, T. T., Moreno-Forero, S. K., Breugelmans, P., Heipieper, H. J., Röling, W. F. M., and Springael, D. (2017). Physiological and transcriptome response of the polycyclic aromatic hydrocarbon degrading Novosphingobium sp. LH128 after inoculation in soil. Environ. Sci. Technol. 51, 1570–1579. doi: 10.1021/acs.est.6b03822
Ford, K. A., and Casida, J. E. (2006). Chloropyridinyl neonicotinoid insecticides: diverse molecular substituents contribute to facile metabolism in mice. Chem. Res. Toxicol. 19, 944–951. doi: 10.1021/tx0600696
Ford, K. A., and Casida, J. E. (2008). Comparative metabolism and pharmacokinetics of seven neonicotinoid insecticides in spinach. J. Agr. Food Chem. 56, 10168–10175. doi: 10.1021/jf8020909
Friedli, A., Williams, G. R., Bruckner, S., Neumann, P., and Straub, L. (2020). The weakest link: haploid honey bees are more susceptible to neonicotinoid insecticides. Chemosphere 242:125145. doi: 10.1016/j.chemosphere.2019.125145
Fusetto, R., Denecke, S., Perry, T., Hair, O., and Batterham, P. (2017). Partitioning the roles of CYP6G1 and gut microbes in the metabolism of the insecticide imidacloprid in drosophila melanogaster. Sci. Rep. 7:11339. doi: 10.1038/s41598-017-09800-2
Gajger, I. T., Sakač, M., and Gregorc, A. (2017). Impact of thiamethoxam on honey bee Queen (Apis mellifera carnica) reproductive morphology and physiology. B. Environ. Contam. Tox. 99, 297–302. doi: 10.1007/s00128-017-2144-0
Ge, F., Zhou, L., Wang, Y., Ma, Y., Zhai, S., Liu, Z., et al. (2014). Hydrolysis of the neonicotinoid insecticide thiacloprid by the N2-fixing bacterium Ensifer meliloti CGMCC 7333. Int. Biodeter. Biodegradat. 93, 10–17. doi: 10.1016/j.ibiod.2014.05.001
Guo, L., Dai, Z., Guo, J., Yang, W., Ge, F., and Dai, Y. (2020). Oligotrophic bacterium Hymenobacter latericoloratus CGMCC 16346 degrades the neonicotinoid imidacloprid in surface water. AMB Express 10:7. doi: 10.1186/s13568-019-0942-y
Guo, L., Fang, W., Guo, L., Yao, C., Zhao, Y., Ge, F., et al. (2019). Biodegradation of the neonicotinoid insecticide acetamiprid by Actinomycetes Streptomyces canus CGMCC 13662 and characterization of the novel nitrile hydratase involved. J. Agr. Food Chem. 67, 5922–5931. doi: 10.1021/acs.jafc.8b06513
Gupta, M., Mathur, S., Sharma, T. K., Rana, M., Gairola, A., Navani, N. K., et al. (2016). A study on metabolic prowess of Pseudomonas sp. RPT 52 to degrade imidacloprid, endosulfan and coragen. J. Hazard. Mater. 301, 250–258. doi: 10.1016/j.jhazmat.2015.08.055
Gupta, S., and Gajbhiye, V. T. (2007). Persistence of acetamiprid in soil. B. Environ. Contam. Tox. 78, 349–352. doi: 10.1007/s00128-007-9097-7
Hamada, A., Wahl, G. D., Nesterov, A., Nakao, T., Kawashima, M., and Banba, S. (2019). Differential metabolism of imidacloprid and dinotefuran by Bemisia Tabaci CYP6CM1 variants. Pestic. Biochem. Phys. 159, 27–33. doi: 10.1016/j.pestbp.2019.05.011
Han, W., Tian, Y., and Shen, X. (2018). Human exposure to neonicotinoid insecticides and the evaluation of their potential toxicity: an overview. Chemosphere 192, 59–65. doi: 10.1016/j.chemosphere.2017.10.149
Hirano, T., Yanai, S., Takada, T., Yoneda, N., Omotehara, T., Kubota, N., et al. (2018). Noael-dose of a neonicotinoid pesticide, clothianidin, acutely induce anxiety-related behavior with human-audible vocalizations in male mice in a novel environment. Toxicol. Lett. 282, 57–63. doi: 10.1016/j.toxlet.2017.10.010
Hladik, M. L., Main, A. R., and Goulson, D. (2018). Environmental risks and challenges associated with neonicotinoid insecticides. Environ. Sci. Technol. 52, 3329–3335. doi: 10.1021/acs.est.7b06388
Hong, X., Zhao, X., Tian, X., Li, J., and Zha, J. (2018). Changes of hematological and biochemical parameters revealed genotoxicity and immunotoxicity of neonicotinoids on Chinese rare minnows (Gobiocypris rarus). Environ. Pollut. 233, 862–871. doi: 10.1016/j.envpol.2017.12.036
Huang, Y., Zhan, H., Bhatt, P., and Chen, S. (2019). Paraquat degradation from contaminated environments: current achievements and perspectives. Front. Microbiol. 10:1754. doi: 10.3389/fmicb.2019.01754
Huang, Y., Lin, Z., Zhang, W., Pang, S., Bhatt, P., Rene, E. R., et al. (2020). New insights into the microbial degradation of D-cyphenothrin in contaminated water/soil environments. Microorganisms 8:473. doi: 10.3390/microorganisms8040473
Hu, G. (2013). Isolation of an indigenous imidacloprid-degrading bacterium and imidacloprid bioremediation under simulated In situ and Ex situ conditions. J. Microbiol. Biotechn. 23, 1617–1626. doi: 10.4014/jmb.1305.05048
Humann-Guilleminot, S., Binkowski, ŁJ., Jenni, L., Hilke, G., Glauser, G., and Helfenstein, F. (2019). A nation−wide survey of neonicotinoid insecticides in agricultural land with implications for agri−environment schemes. J. Appl. Ecol. 56, 1502–1514. doi: 10.1111/1365-2664.13392
Hussain, S., Hartley, C. J., Shettigar, M., and Pandey, G. (2016). Bacterial biodegradation of neonicotinoid pesticides in soil and water systems. FEMS Microbiol. Lett. 363:fnw252. doi: 10.1093/femsle/fnw252
Iturburu, F. G., Simoniello, M. F., Medici, S., Panzeri, A. M., and Menone, M. L. (2018). Imidacloprid causes DNA damage in fish: Clastogenesis as a mechanism of genotoxicity. Bull. Environ. Contam. Toxicol. 100, 760–764. doi: 10.1007/s00128-018-2338-0
Jean-Marc, B., Dominique, A. N., and Heron, M. (2019). A survey and risk assessment of neonicotinoids in water, soil and sediments of belize. Environ. Pollut. 949–958. doi: 10.1016/j.envpol.2019.03.099
Jeschke, P., and Nauen, R. (2008). Neonicotinoids-from zero to hero in insecticide chemistry. Pest Manag. Sci. 64, 1084–1098. doi: 10.1002/ps.1631
Kandil, M. M., Trigo, C., Koskinen, W. C., and Sadowsky, M. J. (2015). Isolation and characterization of a novel imidacloprid-degrading Mycobacterium sp. strain MK6 from an Egyptian Soil. J. Agric. Food Chem. 63, 4721–4727. doi: 10.1021/acs.jafc.5b00754
Lin, Z., Zhang, W., Pang, S., Huang, Y., Mishra, S., Bhatt, P., et al. (2020). Current approaches to and future perspectives on methomyl degradation in contaminated soil/water environments. Molecules 25:738. doi: 10.3390/molecules25030738
Li, X., Schuler, M. A., and Berenbaum, M. R. (2007). Molecular mechanisms of metabolic resistance to synthetic and natural xenobiotics. Annu. Rev. Entomol. 52, 231–253. doi: 10.1146/annurev.ento.51.110104.151104
Li, Z., Yu, T., Chen, Y., Heerman, M., He, J., Huang, J., et al. (2019). Brain transcriptome of honey bees (Apis mellifera) exhibiting impaired olfactory learning induced by a sublethal dose of imidacloprid. Pestic. Biochem. Phys. 156, 36–43. doi: 10.1016/j.pestbp.2019.02.001
Liu, J., Chen, S., Ding, J., Xiao, Y., Han, H., and Zhong, G. (2015). Sugarcane bagasse as support for immobilization of Bacillus pumilus HZ-2 and its use in bioremediation of mesotrione-contaminated soils. Appl. Microbiol. Biotechnol. 99, 10839–10851. doi: 10.1007/s00253-015-6935-0
Liu, S., Liu, Y., He, F., Zhang, H., Li, X., and Tan, H. (2019). Enantioselective olfactory effects of the neonicotinoid dinotefuran on honey bees (Apis mellifera L.). J. Agric. Food Chem. 67, 12105–12116. doi: 10.1021/acs.jafc.9b04851
Liu, T., Wang, X., Xu, J., You, X., Chen, D., Wang, F., et al. (2017). Biochemical and genetic toxicity of dinotefuran on earthworms (Eisenia fetida). Chemosphere 176, 156–164. doi: 10.1016/j.chemosphere.2017.02.113
Liu, Z., Dai, Y., Huang, G., Gu, Y., Ni, J., Wei, H., et al. (2011). Soil microbial degradation of neonicotinoid insecticides imidacloprid, acetamiprid, thiacloprid and imidaclothiz and its effect on the persistence of bioefficacy against horsebean aphid aphis craccivora koch after soil application. Pest Manag. Sci. 67, 1245–1252. doi: 10.1002/ps.2174
Lu, T., Mao, S., Sun, S., Yang, W., Ge, F., and Dai, Y. (2016). Regulation of hydroxylation and nitroreduction pathways during metabolism of the neonicotinoid insecticide imidacloprid by Pseudomonas putida. J. Agric. Food Chem. 64, 4866–4875. doi: 10.1021/acs.jafc.6b01376
Ma, Y., Zhai, S., Mao, S. Y., Sun, S. L., Wang, Y., Liu, Z. H., et al. (2014). Co-metabolic transformation of the neonicotinoid insecticide imidacloprid by the new soil isolate Pseudoxanthomonas Indica CGMCC 6648. J. Environ. Sci. Heal. B 49, 661–670. doi: 10.1080/03601234.2014.922766
Manasi, G., Samarth, M., Tarun, K. S., Manish, R., Ajay, G., and Ranjana, N. K. N. (2016). A study on metabolic prowess of Pseudomonas sp. RPT 52 to degrade imidacloprid, endosulfan and coragen. J. Hazard. Mater. 301, 250–258. doi: 10.1016/j.jhazmat.2015.08.055
Manjon, C., Troczka, B. J., Zaworra, M., Beadle, K., Randall, E., Hertlein, G., et al. (2018). Unravelling the molecular determinants of bee sensitivity to neonicotinoid insecticides. Curr. Biol. 28, 1137–1143.e5. doi: 10.1016/j.cub.2018.02.045
Matsuda, K., Ihara, M., and Sattelle, D. B. (2020). Neonicotinoid insecticides: molecular targets, resistance, and toxicity. Ann. Rev. Pharmacol. Toxicol. 60, 241–255. doi: 10.1146/annurev-pharmtox-010818-021747
Mariusz, C., Anna, M., StAwomir, B., and Marcin, W. (2013). Imidacloprid induces changes in the structure, genetic diversity and catabolic activity of soil microbial communities. J. Environ. Manag. 131, 55–65. doi: 10.1016/j.jenvman.2013.09.041 Z
Mikolić, A., and Karačonji, I. B. (2018). Imidacloprid as reproductive toxicant and endocrine disruptor: investigations in laboratory animals. Arch. Ind. Hyg. Toxicol. 69, 103–108. doi: 10.2478/aiht-2018-69-3144
Mohammed, Y., and Badawy, M. (2017). Biodegradation of imidacloprid in liquid media by an isolated wastewater fungus Aspergillus Terreus YESM3. J. Environ. Sci. Heal. B 52, 752–761. doi: 10.1080/03601234.2017.1356666
Mori, T., Wang, J., Tanaka, Y., Nagai, K., Kawagishi, H., and Hirai, H. (2017). Bioremediation of the neonicotinoid insecticide clothianidin by the white-rot fungus Phanerochaete sordida. J. Hazard. Mater. 321, 586–590. doi: 10.1016/j.jhazmat.2016.09.049
Mulligan, R. A., Tomco, P. L., Howard, M. W., Schempp, T. T., Stewart, D. J., Stacey, P. M., et al. (2016). Aerobic versus anaerobic microbial degradation of clothianidin under simulated california rice field conditions. J. Agric. Food Chem. 64, 7059–7067. doi: 10.1021/acs.jafc.6b02055
Myresiotis, C. K., Vryzas, Z., and Papadopoulou-Mourkidou, E. (2015). Effect of specific plant-growth-promoting rhizobacteria (PGPR) on growth and uptake of neonicotinoid insecticide thiamethoxam in corn (Zea mays L.) seedlings. Pest Manag. Sci. 71, 1258–1266. doi: 10.1002/ps.3919
Nauen, R., Ebbinghaus-Kintscher, U., Salgado, V. L., and Kaussmann, M. (2003). Thiamethoxam is a neonicotinoid precursor converted to clothianidin in insects and plants. Pestic. Biochem. Phys. 76, 55–69. doi: 10.1016/S0048-3575(03)00065-8
Pandey, G., Dorrian, S. J., Russell, R. J., and Oakeshott, J. G. (2009). Biotransformation of the neonicotinoid insecticides imidacloprid and thiamethoxam by Pseudomonas sp. 1G. Biochem. Bioph. Res. Commun. 380, 710–714. doi: 10.1016/j.bbrc.2009.01.156
Pang, R., Chen, M., Liang, Z., Yue, X., Ge, H., and Zhang, W. (2016). Functional analysis of CYP6ER1, a P450 gene associated with imidacloprid resistance in nilaparvata lugens. Sci. Rep. 6:34992. doi: 10.1038/srep34992
Parween, T., Bhandari, P., Jan, S., and Raza, S. K. (2016). Interaction between pesticide and soil microorganisms and their degradation: a molecular approach. Plant Soil Microbes 2, 23–43. doi: 10.1007/978-3-319-29573-2_2
Parte, S. G., and Kharat, A. S. (2019). Aerobic degradation of clothianidin to 2-chloro-methyl thiazole and methyl 3-(thiazole-yl) methyl guanidine produced by Pseudomonas stutzeri smk. J. Environ. Public Health 2019:4807913. doi: 10.1155/2019/4807913
Peter, J., Ralf, N., Michael, S., and Alfred, E. (2010). Overview of the status and global strategy for neonicotinoids. J. Agric. Food Chem. 59, 2897–2908. doi: 10.1021/jf101303g
Phugare, S. S., Kalyani, D. C., Gaikwad, Y. B., and Jadhav, J. P. (2013). Microbial degradation of imidacloprid and toxicological analysis of its biodegradation metabolites in silkworm (Bombyx mori). Chem. Eng. J. 230, 27–35. doi: 10.1016/j.cej.2013.06.042
Phugare, S. S., and Jadhav, J. P. (2013). Biodegradation of acetamiprid by isolated bacterial strain Rhodococcus sp. BCH2 and toxicological analysis of its metabolites in silkworm. Clean Soil Air Water 43, 296–304. doi: 10.1002/clen.201200563
Puinean, A. M., Stephen, P. F., Oliphant, L., Denholm, I., Linda, M. F., Millar, N. S., et al. (2010). Amplification of a cytochrome P450 gene is associated with resistance to neonicotinoid insecticides in the aphid Myzus persicae. PLoS Genet. 6:e1000999. doi: 10.1371/journal.pgen.1000999
Qi, S., Wang, D., Zhu, L., Teng, M., Wang, C., Xue, X., et al. (2018). Effects of a novel neonicotinoid insecticide cycloxaprid on earthworm, eisenia fetida. Environ. Sci. Pollut. Res. Int. 25, 14138–14147. doi: 10.1007/s11356-018-1624-z
Ramamurthy, T., Ghosh, A., Pazhani, G. P., and Shinoda, S. (2014). Current perspectives on viable but non-culturable (VBNC) pathogenic bacteria. Front. Public Health 2:103. doi: 10.3389/fpubh.2014.00103
Rana, S., Jindal, V., Mandal, K., Kaur, G., and Gupta, V. K. (2015). Thiamethoxam degradation by Pseudomonas and Bacillus strains isolated from agricultural soils. Environ. Monit. Assess. 187:300. doi: 10.1007/s10661-015-4532-4
Rauch, N., and Nauen, R. (2003). Identification of biochemical markers linked to neonicotinoid cross resistance in Bemisia tabaci (hemiptera: aleyrodidae). Arch. Insect Biochem. 54, 165–176. doi: 10.1002/arch.10114
Sabourmoghaddam, N., Zakaria, M. P., and Omar, D. (2015). Evidence for the microbial degradation of imidacloprid in soils of cameron highlands. J. Saudi Soc. Agr. Sci. 14, 182–188. doi: 10.1016/j.jssas.2014.03.002
Scott, C., Pandey, G., Hartley, C. J., Jackson, C. J., Cheesman, M. J., Taylor, M. C., et al. (2008). The enzymatic basis for pesticide bioremediation. Indian J. Microbiol. 48, 65–79. doi: 10.1007/s12088-008-0007-4
Seifrtova, M., Halesova, T., Sulcova, K., Riddellova, K., and Erban, T. (2017). Distributions of imidacloprid, imidacloprid-olefin and imidacloprid-urea in green plant tissues and roots of rapeseed (Brassica napus) from artificially contaminated potting soil. Pest Manag. Sci. 73, 1010–1016. doi: 10.1002/ps.4418
Selçuk, Ö, Serdar, A., and Mustafa, Ö (2018). Cypermethrin, chlorpyrifos, deltamethrin, and imidacloprid exposure up-regulates the mRNA and protein levels of bdnf and c-fos in the brain of adult zebrafish (Danio rerio). Chemosphere 203, 318–326. doi: 10.1016/j.Chemosphere.2018.03.190
Şenyildiz, M., Kilinc, A., and Ozden, S. (2018). Investigation of the genotoxic and cytotoxic effects of widely used neonicotinoid insecticides in HepG2 and SH-SY5Y cells. Toxicol. Ind. Health 34, 375–383. doi: 10.1177/0748233718762609
Shaikh, N. S., Kulkarni, S. V., Mulani, S. M., and Baig, U. (2014). Biodegradation of imidacloprid, the new generation neurotoxic insecticide. Biodegradation 3, 16301–16307. doi: 10.15680/IJIRSET.2014.0309071
Sharma, S., Singh, B., and Gupta, V. K. (2014). Biodegradation of imidacloprid by consortium of two soil isolated Bacillus sp. B. Environ. Contam. Tox. 93, 637–642. doi: 10.1007/s00128-014-1386-3
Shettigar, M., Pearce, S., Pandey, R., Khan, F., Dorrian, S. J., Balotra, S., et al. (2012). Cloning of a novel 6-chloronicotinic acid chlorohydrolase from the newly isolated 6-chloronicotinic acid mineralizing Bradyrhizobiaceae Strain SG-6C. PLoS One 7:e51162. doi: 10.1371/journal.pone.0051162
Shi, Z., Dong, W., Xin, F., Liu, J., Zhou, X., Xu, F., et al. (2018). Characteristics and metabolic pathway of acetamiprid biodegradation by Fusarium sp. strain CS-3 isolated from soil. Biodegradation 29, 593–603. doi: 10.1007/s10532-018-9855-8
Simon-Delso, N., Amaral-Rogers, V., Belzunces, L. P., Bonmatin, J. M., Chagnon, M., Downs, C., et al. (2015). Systemic insecticides (neonicotinoids and fipronil): trends, uses, mode of action and metabolites. Environ. Sci. Pollut. Res. Int. 22, 5–34. doi: 10.1007/s11356-014-3470-y
Su, X., Xue, B., Wang, Y., Hashmi, M. Z., Lin, H., Chen, J., et al. (2019). Bacterial community shifts evaluation in the sediments of Puyang River and its nitrogen removal capabilities exploration by resuscitation promoting factor. Ecotoxicol. Environ. Saf. 179, 188–197. doi: 10.1016/j.ecoenv.2019.04.067
Su, X., Wang, Y., Xue, B., Zhang, Y., Mei, R., Zhang, Y., et al. (2018). Resuscitation of functional bacterial community for enhancing biodegradation of phenol under high salinity conditions based on Rpf. Bioresour. Technol. 261, 394–402. doi: 10.1016/j.biortech.2018.04.048
Sumon, K. A., Ritika, A. K., Peeters, E. T. H. M., Rashid, H., Bosma, R. H., Rahman, M. S., et al. (2018). Effects of imidacloprid on the ecology of sub-tropical freshwater microcosms. Environ. Pollut. 236, 432–441. doi: 10.1016/j.envpol.2018.01.102
Sun, S., Fan, Z., Zhao, Y., Guo, L., and Dai, Y. (2018). A novel nutrient deprivation-induced neonicotinoid insecticide acetamiprid degradation by Ensifer adhaerens CGMCC 6315. J. Agric. Food Chem. 67, 63–71. doi: 10.1021/acs.jafc.8b06154
Sun, S., Yang, W., Guo, J., Zhou, Y., and Rui, X. (2017). Biodegradation of the neonicotinoid insecticide acetamiprid in surface water by the bacterium Variovorax boronicumulans CGMCC 4969 and its enzymatic mechanism. RSC Adv. 7, 25387–25397. doi: 10.1039/c7ra01501a
Sun, S.-L., Yang, W.-L., Fang, W.-W., Zhao, Y.-X., Guo, L., and Dai, Y.-J. (2018). The plant growth-promoting rhizobacterium Variovorax boronicumulans CGMCC 4969 regulates the level of indole-3-acetic acid synthesized from indole-3-acetonitrile. Appl. Environ. Microbiol. 84:e00298-18. doi: 10.1128/AEM.00298-18
Taillebois, E., Cartereau, A., Jones, A. K., and Thany, S. H. (2018). Neonicotinoid insecticides mode of action on insect nicotinic acetylcholine receptors using binding studies. Pestic. Biochem. Physiol. 151, 59–66. doi: 10.1016/j.pestbp.2018.04.007
Tang, H., Li, J., Hu, H., and Xu, P. (2012). A newly isolated strain of Stenotrophomonas sp. hydrolyzes acetamiprid, a synthetic insecticide. Process. Biochem. 47, 1820–1825. doi: 10.1016/j.procbio.2012.06.008
Thurman, E. M., Ferrer, I., Zavitsanos, P., and Zweigenbaum, J. A. (2013). Identification of imidacloprid metabolites in onion (Allium cepa L.) using high-resolution mass spectrometry and accurate mass tools. Rapid Commun. Mass Spectrom. 27, 1891–1903. doi: 10.1002/rcm.6637
Tian, X., Yang, W., Wang, D., Zhao, Y., Yao, R., Ma, L., et al. (2018). Chronic brain toxicity response of juvenile Chinese rare minnows (Gobiocypris rarus) to the neonicotinoid insecticides imidacloprid and nitenpyram. Chemosphere 210, 1006–1012. doi: 10.1016/j.chemosphere.2018.06.083
Tomasz, S., Joanna, B., Granada, C. E., Passaglia, M. A., and Passaglia, L. M. P. (2018). Phylogeny and phylogeography of rhizobial symbionts nodulating legumes of the tribe genisteae. Genes (Basel) 3:e163. doi: 10.3390/genes9030163
Tooming, E., Merivee, E., Must, A., Merivee, M., Sibul, I., Nurme, K., et al. (2017). Behavioural effects of the neonicotinoid insecticide thiamethoxam on the predatory insect Platynus assimilis. Ecotoxicology 26, 902–913. doi: 10.1007/s10646-017-1820-5
Tosi, S., Burgio, G., and Nieh, J. C. (2017). A common neonicotinoid pesticide, thiamethoxam, impairs honey bee flight ability. Sci. Rep. 7:1201. doi: 10.1038/s41598-017-01361-8
Tosi, S., and Nieh, J. C. (2017). A common neonicotinoid pesticide, thiamethoxam, alters honey bee activity, motor functions, and movement to light. Sci. Rep. 7:15132. doi: 10.1038/s41598-017-15308-6
van Gestel, C. A. M., de Lima, E., Silva, C., Lam, T., Koekkoek, J. C., Lamoree, M. H., et al. (2017). Multigeneration toxicity of imidacloprid and thiacloprid to Folsomia candida. Ecotoxicology 26, 320–328. doi: 10.1007/s10646-017-1765-8
Vignet, C., Cappello, T., Fu, Q., Lajoie, K., De Marco, G., Clérandeau, C., et al. (2019). Imidacloprid induces adverse effects on fish early life stages that are more severe in Japanese medaka (Oryzias latipes) than in zebrafish (Danio rerio). Chemosphere 225, 470–478. doi: 10.1016/j.Chemosphere.2019.03.002
Wang, G., Yue, W., Liu, Y., Li, F., Xiong, M., and Zhang, H. (2013a). Biodegradation of the neonicotinoid insecticide acetamiprid by bacterium Pigmentiphaga sp. strain AAP-1 isolated from soil. Bioresour. Technol. 138, 359–368. doi: 10.1016/j.biortech.2013.03.193
Wang, G., Zhao, Y., Gao, H., Yue, W., Xiong, M., Li, F., et al. (2013b). Co-metabolic biodegradation of acetamiprid by Pseudoxanthomonas sp. AAP-7 isolated from a long-term acetamiprid-polluted soil. Bioresour. Technol. 150, 259–265. doi: 10.1016/j.biortech.2013.10.008
Wang, J., Hirai, H., and Kawagishi, H. (2012). Biotransformation of acetamiprid by the white-rot fungus Phanerochaete sordida YK-624. Appl. Microbiol. Biotechnol. 93, 831–835. doi: 10.1007/s00253-011-3435-8
Wang, J., Tanaka, Y., Ohno, H., Jia, J., Mori, T., Xiao, T., et al. (2019). Biotransformation and detoxification of the neonicotinoid insecticides nitenpyram and dinotefuran by Phanerochaete sordida YK-624. Environ. Pollut. 252, 856–862. doi: 10.1016/j.envpol.2019.06.022
Wang, M., Yang, G., Wang, X., Yao, Y., Min, H., and Lu, Z. (2011). Nicotine degradation by two novel bacterial isolates of Acinetobacter sp. TW and Sphingomonas sp. TY and their responses in the presence of neonicotinoid insecticides. Microbiol. Biotechnol. 12, 1633–1640. doi: 10.1007/s11274-010-0617-y
Wang, Y., Sun, Q., Tian, C., Gui, W., and Zhu, G. (2016). Degradation properties and identification of metabolites of 6-Cl-PMNI in soil and water. Chemosphere 147, 287–296. doi: 10.1016/j.chemosphere.2015.12.020
Wang, Y., Xu, P., Chang, J., Li, W., Yang, L., and Tian, H. (2020). Unraveling the toxic effects of neonicotinoid insecticides on the thyroid endocrine system of lizards. Environ. Pollut. 258:113731. doi: 10.1016/j.envpol.2019.113731
Wang, Y., Zhang, Y., Li, W., Han, Y., and Guo, B. (2019a). Study on neurotoxicity of dinotefuran, thiamethoxam and imidacloprid against Chinese lizards (Eremias argus). Chemosphere 217, 150–157. doi: 10.1016/j.chemosphere.2018.11.016
Wang, Y., Zhang, Y., Li, W., Yang, L., and Guo, B. (2019b). Distribution, metabolism and hepatotoxicity of neonicotinoids in small farmland lizard and their effects on GH/IGF axis. Sci. Total Environ. 662, 834–841. doi: 10.1016/j.scitotenv.2019.01.277
Wang, Y., Zhang, Y., Xu, P., Guo, B., and Li, W. (2018). Metabolism distribution and effect of thiamethoxam after oral exposure in mongolian racerunner (Eremias argus). J. Agric. Food Chem. 66, 7376–7383. doi: 10.1021/acs.jafc.8b02102
Wang, Y., Zhang, Y., Zeng, T., Li, W., Yang, L., and Guo, B. (2019c). Accumulation and toxicity of thiamethoxam and its metabolite clothianidin to the gonads of Eremias argus. Sci. Total Environ. 667, 586–593. doi: 10.1016/j.scitotenv.2019.02.419
Wondji, C. S., Irving, H., Morgan, J., Lobo, N. F., Collins, F. H., Hunt, R. H., et al. (2008). Two duplicated P450 genes are associated with pyrethroid resistance in Anopheles funestus, a major malaria vector. Genome Res. 19, 452–459. doi: 10.1101/gr.087916.108
Wu, Y., Xu, H., Pan, Y., Gao, J., Xi, J., Zhang, J., et al. (2018). Expression profile changes of cytochrome P450 genes between thiamethoxam susceptible and resistant strains of Aphis gossypii glover. Pestic. Biochem. Phys. 49, 1–7. doi: 10.1016/j.pestbp.2018.05.007
Xiao, Y., Chen, S., Gao, Y., Hu, W., Hu, M., and Zhong, G. (2015). Isolation of a novel beta-cypermethrin degrading strain Bacillus subtilis BSF01 and its biodegradation pathway. Appl. Microbiol. Biotechnol. 99, 2849–2859. doi: 10.1007/s00253-014-6164-y
Yan, S., Wang, J., Zhu, L., Chen, A., and Wang, J. (2015). Toxic effects of nitenpyram on antioxidant enzyme system and DNA in zebrafish (Danio rerio) livers. Ecotoxicol. Environ. Saf. 122, 54–60. doi: 10.1016/j.ecoenv.2015.06.030
Yang, H., Wang, X., Zheng, J., Wang, G., Hong, Q., Li, S., et al. (2013). Biodegradation of acetamiprid by Pigmentiphaga sp. D-2 and the degradation pathway. Int. Biodeter. Biodegr. 85, 95–102. doi: 10.1016/j.ibiod.2013.03.038
Yang, J., Feng, Y., Zhan, H., Liu, J., Yang, F., Zhang, K., et al. (2018). Characterization of a pyrethroid-degrading Pseudomonas fulva strain P31 and biochemical degradation pathway of D-phenothrin. Front. Microbiol. 9:1003. doi: 10.3389/fmicb.2018.01003
Yao, X., and Min, H. (2006). Isolation, characterization and phylogenetic analysis of a bacterial strain capable of degrading acetamiprid. J. Environ. Sci. 18, 141–146.
Zaworra, M., Koehler, H., Schneider, J., Lagojda, A., and Nauen, R. (2018). Pharmacokinetics of three neonicotinoid insecticides upon contact exposure in the western honey bee, Apis mellifera. Chem. Res. Toxicol. 32, 35–37. doi: 10.1021/acs.chemrestox.8b00315
Zhan, H., Feng, Y., Fan, X., and Chen, S. (2018a). Recent advances in glyphosate biodegradation. Appl. Microbiol. Biotechnol. 102, 5033–5043. doi: 10.1007/s00253-018-9035-0
Zhan, H., Wang, H., Liao, L., Feng, Y., Fan, X., Zhang, L., et al. (2018b). Kinetics and novel degradation pathway of permethrin in Acinetobacter baumannii ZH-14. Front. Microbiol. 9:98. doi: 10.3389/fmicb.2018.00098
Zhan, H., Huang, Y., Lin, Z., Bhatt, P., and Chen, S. (2020). New insights into the microbial degradation and catalytic mechanism of synthetic pyrethroids. Environ. Res. 182:109138. doi: 10.1016/j.envres.2020.109138
Zhang, H., Zhou, Q., Zhou, G., Cao, Y., Dai, Y., Ji, W., et al. (2012). Biotransformation of the neonicotinoid insecticide thiacloprid by the bacterium Variovorax boronicumulans strain J1 and mediation of the major metabolic pathway by nitrile hydratase. J. Agric. Food Chem. 60, 153–159. doi: 10.1021/jf203232u
Zhang, P., Ren, C., Sun, H., and Min, L. (2018). Sorption, desorption and degradation of neonicotinoids in four agricultural soils and their effects on soil microorganisms. Sci. Total Environ. 615, 59–69. doi: 10.1016/j.scitotenv.2017.09.097
Zhang, W., Lin, Z., Pang, S., Bhatt, P., and Chen, S. (2020). Insights into the biodegradation of lindane (γ-hexachlorocyclohexane) using a microbial system. Front. Microbiol. 11:522. doi: 10.3389/fmicb.2020.00522
Zhao, Y., Dai, Y., Yu, C., Luo, J., Xu, W., Ni, J., et al. (2009). Hydroxylation of thiacloprid by bacterium Stenotrophomonas Maltophilia CGMCC1.1788. Biodegradation 20, 761–768. doi: 10.1007/s10532-009-9264-0
Zhao, Y., Jiang, H., Cheng, X., Zhu, Y., Fan, Z., Dai, Z., et al. (2019). Neonicotinoid thiacloprid transformation by the N2-fixing bacterium Microvirga Flocculans CGMCC 1.16731 and toxicity of the amide metabolite. Int. Biodeter. Biodegr. 145:104806. doi: 10.1016/j.ibiod.2019.104806
Zhou, G., Wang, Y., Ma, Y., Zhai, S., Zhou, L., Dai, Y., et al. (2014). The metabolism of neonicotinoid insecticide thiamethoxam by soil enrichment cultures, and the bacterial diversity and plant growth-promoting properties of the cultured isolates. J. Environ. Sci. Heal. B. 49, 381–390. doi: 10.1080/03601234.2014.894761
Zhou, G., Wang, Y., Zhai, S., Ge, F., Liu, Z., Dai, Y., et al. (2013). Biodegradation of the neonicotinoid insecticide thiamethoxam by the nitrogen-fixing and plant-growth-promoting Rhizobacterium Ensifer adhaerens strain TMX-23. Appl. Microbiol. Biotechnol. 97, 4065–4074. doi: 10.1007/s00253-012-4638-3
Keywords: neonicotinoids, toxicity, microbial degradation, metabolic pathways, bioremediation, molecular mechanisms
Citation: Pang S, Lin Z, Zhang W, Mishra S, Bhatt P and Chen S (2020) Insights Into the Microbial Degradation and Biochemical Mechanisms of Neonicotinoids. Front. Microbiol. 11:868. doi: 10.3389/fmicb.2020.00868
Received: 13 January 2020; Accepted: 14 April 2020;
Published: 19 May 2020.
Edited by:
Guining Lu, South China University of Technology, ChinaReviewed by:
Xiaomei Su, Zhejiang Normal University, ChinaSantosh Kr. Karn, Sardar Bhagwan Singh Post Graduate Institute of Biomedical Sciences & Research, India
Copyright © 2020 Pang, Lin, Zhang, Mishra, Bhatt and Chen. This is an open-access article distributed under the terms of the Creative Commons Attribution License (CC BY). The use, distribution or reproduction in other forums is permitted, provided the original author(s) and the copyright owner(s) are credited and that the original publication in this journal is cited, in accordance with accepted academic practice. No use, distribution or reproduction is permitted which does not comply with these terms.
*Correspondence: Shaohua Chen, c2hjaGVuQHNjYXUuZWR1LmNu