- 1CAS Key Laboratory of Environmental and Applied Microbiology, Environmental Microbiology Key Laboratory of Sichuan Province, Chengdu Institute of Biology, Chinese Academy of Sciences, Chengdu, China
- 2Key Laboratory of Bio-Resource and Eco-Environment of Ministry of Education, College of Life Sciences, Sichuan University, Chengdu, China
- 3University of Chinese Academy of Sciences, Beijing, China
- 4Sweetpotato Institute, Nanchong Academy of Agricultural Sciences, Nanchong, China
Sweetpotato can be cultivated in the reclaimed rocky soil in Sichuan Basin, China, which benefits from the release of mineral nutrients in the rocky soil by microorganisms. Shortage of nitrogen (N) in the rocky soil limits sweetpotato yield, which can be compensated through N fertilization. Whereas high N fertilization inhibits biological N fixation and induces unintended environmental consequences. However, the effect of low N fertilization on microorganism community and sweetpotato yield in the N-deficient rocky soil is still unclear. We added a low level of 1.5 g urea/m2 to a rocky soil cultivated with sweetpotato, and measured rocky soil physiological and biochemical properties, rhizosphere microbial diversity, sweetpotato physiological properties and transcriptome. When cultivating sweetpotato in the rocky soil, low N fertilization (1.5 g urea/m2) not only improved total N (TN) and available N (AN) in the rocky soil, but also increased available phosphorus (AP), available potassium (AK), and nitrogenase and urease activity. Interestingly, although low N fertilization could reduce bacterial diversity through affecting sweetpotato root exudates and rocky soil properties, the relative abundance of P and K-solubilizing bacteria, N-fixing and urease-producing bacteria increased under low N fertilization, and the relative abundance of plant pathogens decreased. Furthermore, low N fertilization increased the phytohormones, such as zeatin riboside, abscisic acid, and methyl jasmonate contents in sweetpotato root. Those increases were consistent with our transcriptome findings: the inhibition of the lignin synthesis, the promotion of the starch synthesis, and the upregulated expression of Expansin, thus resulting in promoting the formation of tuberous roots and further increasing the sweetpotato yield by half, up to 3.3 kg/m2. This study indicated that low N fertilization in the N-deficient rocky soil improved this soil quality through affecting microorganism community, and further increased sweetpotato yield under regulation of phytohormones pathway.
Introduction
Sweetpotato is the 7th most important food crop, with a global annual production of more than 1 × 1011 kg (Yang et al., 2017). As a rich source of starch, proteins, vitamins, dietary fiber and mineral elements, sweetpotato plays an important role in ensuring food security, especially in sub-Saharan Africa and Asia (Wu S. et al., 2018). With the growing population, global food security is becoming increasingly serious (Food and Agriculture Organization, 2019). Moreover, the global arable land area per capita has decreased from 5.0 × 103 to 2.0 × 103 m2 in the past 70 years (FAO). Therefore, further improving the yield of sweetpotato on the limited available land has become of great significance.
Rocky soil has high potential for agricultural utilization due to rich phosphorus (P), potassium (K), iron and other nutrients (Manning and Theodoro, 2018). For example, in the Sichuan Basin, the largest sweetpotato producing area in China (approximately 1.0 × 1010 m2), more than 70% of the hills (approximately 5.0 × 1010 m2) are covered by rocky soil, which can be reclaimed for sweetpotato cultivation by local farmers (Deng et al., 2014). However, most of the P and K are fixed in the rocky soil, keeping them from being taken up readily by plants (Chatterjee et al., 2014). More seriously, both total nitrogen (TN) and available nitrogen (AN) are inadequate in the rocky soil due to the lack of nitrogen (N) fertilization and other exogenous N input (Zhu et al., 2008).
Microorganisms play a key role in N fixation, elemental transformation, and improving mineral fertility in soil (Pii et al., 2015; Ciccazzo et al., 2016). Biological N fixation is the second largest N source after N fertilizer in farmland, and approximately 24% total N of crops is derived from the non-symbiotic N fixation of microorganisms (Ladha et al., 2016; Feng et al., 2018). Meanwhile, P and K-solubilizing microorganisms transform unavailable P and K to available P and K (AP, AK) through dissolution and/or mineralization (Etesami et al., 2017; Granada et al., 2018). In addition, microorganisms affect soil pH, density and porosity through metabolic activities (Helliwell et al., 2014). However, most microorganisms are in dormant state in natural soils, especially in the low-organic-matter soil such as rocky soil (Joergensen and Wichern, 2018).
As a barren-tolerate crop, sweetpotato can grow in the rocky soil, and provide organic matter for microorganisms. However, the further improvement of sweetpotato yield may be limited by N deficiency in the rocky soil, especially in the early stage of sweetpotato growth (Taranet et al., 2017), even if AP and AK deficiency could be alleviated by P and K-solubilizing microorganisms (Etesami et al., 2017; Granada et al., 2018). N deficiency can be compensated through N fertilization, but high N fertilization inhibits biological N fixation and induces unintended environmental consequences (Wang et al., 2018; Wu H. J. et al., 2018). Thus, it is reasonably to hypothesize that low N fertilization may increase sweetpotato yield, whereas reducing the impact on environment. However, few investigations have been reported regarding N fertilization of sweetpotato in the N-deficient rocky soil, as well as its effect on microorganism community and sweetpotato yield, and their underlying mechanism remains unknown.
In this study, we added a low level of 1.5 g urea/m2 to the N-deficient rocky soil and investigated rocky soil properties, rhizosphere microorganisms, sweetpotato physiological properties and global transcriptional response. The objectives were to (1) investigate the effect of low N fertilization on the bacterial and fungal community in the N-deficient rocky soil, and (2) explore the underlying mechanism by which low N fertilization affects sweetpotato yield in the N-deficient rocky soil.
Materials and Methods
Experimental Design
The experiments were conducted in the N-deficient rocky soil at the Yingxi experimental base of the Nanchong Academy of Agricultural Sciences, Sichuan, China (30°52′N, 106°02′E). The rocky soil is a kind of weathered shale with a diameter less than 1.0 cm, and it is easy to be crushed and ploughed (Supplementary Figure S1A). The soil type is purplish soils according to Classification and codes for Chinese soil (GB/T 17296-2009). The rocky soil had a pH of 7.9, total carbon (TC) content of 11.2 g/kg dry soil, dissolved organic carbon (DOC) of 89.2 mg/kg dry soil, TN content of 0.8 g/kg dry soil, AN content of 7.5 mg/kg dry soil, total P (TP) content of 0.6 g/kg dry soil, AP content of 2.5 mg/kg dry soil, total K (TK) content of 17.5 g/kg dry soil, and AK content of 163.6 g/kg dry soil.
Sweetpotato [Ipomoea batatas (L.) Lam.] cultivar Nanshu 88 was planted by ridging at a height of 40 cm in a 200 m2 field (Luo et al., 2017). The planting density was 6 plants/m2. Sweetpotato was planted on June 12, 2016 and harvested on October 20, 2016. The growth period was 130 days. Based on previous experience, a low level of 1.5 g urea/m2 was added 35 days after planting (DAP) in the treatment group (BF group), while no urea was added in the control group (CK group). Surface irrigation was applied only when the sweetpotato seedlings were first planted. The local weather conditions during the experiment were shown in Supplementary Table S1.
Sampling
Sweetpotato was sampled 60 (corresponding to the expansion of tuberous roots) and 130 DAP (corresponding to harvest). Three scattered plots were randomly selected as three replicates. Five sweetpotatoes were excavated from each sample plot. The vines and tuberous roots of sweetpotato were cut and packed separately.
Rocky soil was sampled 0, 60, and 130 DAP. After a sweetpotato was carefully excavated, the shaken-off soil that was not tightly attached to the sweetpotato root was sampled as bulk soil, while the remaining soil that was attached to the sweetpotato root was sampled as rhizosphere soil (Chaudhary et al., 2015). Root fragments and defoliation were carefully removed.
Thus, each sweetpotato and rocky soil sample had three replicates. The tuberous root and rhizosphere rocky soil samples were snap-frozen immediately in liquid N in the field and were then kept on dry ice. All samples were transferred to the laboratory within 3 h and stored in a −80°C freezer for later analyses.
Chemistry Determination of Sweetpotato
The moisture of sweetpotato tuberous roots and vines was determined by drying at 105°C for 48 h to a constant weight. The sweetpotato root exudates were collected following the method of a previous study (Xu Z. et al., 2015). The carbon (C) and N in root exudates, and TN of sweetpotato tuberous roots and vines was determined by using an elemental analyzer (vario MACRO cube, Elementar, Hanau, Germany). The starch contents of sweetpotato tuberous roots were determined by using a high-performance liquid chromatographer (Thermo 2795, Thermo Corp, Waltham, MA, United States)-evaporative light scattering detector (Alltech ELSD 2000, Alltech Corp, Nicholasville, KY, United States) (Liu et al., 2015). The zeatin riboside (ZR), abscisic acid (ABA), and methyl jasmonate (MeJA) of sweetpotato tuberous roots were extracted and quantified by using an indirect enzyme-linked immunosorbent assay technique (Wang et al., 2012).
The equations for the harvest index and N production efficiency were as follows:
N production efficiency (kg/kg) = Fresh yield of the tuberous root/N absorption of the whole plant (Chen et al., 2017).
Determination of Rocky Soil Properties
The moisture of the rocky soil was determined by drying at 105°C for 48 h to a constant weight. DOC of rocky soil was extracted with distilled water (1:10 w/v, soil/water) and analyzed by a Vario TOC analyzer (Vario MACRO cube, Elementar, Germany) (Xu Z. W. et al., 2015). Bulk soil samples were air-dried for 10 days at room temperature to a constant weight, and sieved (<2 mm) before analyses of soil pH, TC, TN, AN, AP, AK. Rocky soil pH (soil:water, 1:2.5) was determined by using a pH meter (PHS-3C, Fangzhou, China) (Dick et al., 2000). The TC and TN of the rocky soil was determined by using an elemental analyzer (vario MACRO cube, Elementar) (Zhang et al., 2017). The AN of the rocky soil was determined with the micro-Kjeldahl distillation method after digestion with KMNO4 and NaOH solution (Subbiah and Asija, 1956). AP and AK were extracted with 0.5 M sodium bicarbonate and 1 M ammonium acetate, respectively, and then measured with inductively coupled plasma optical emission spectrometry (Optima 8300, PerkinElmer, United States) (He et al., 2016). The bulk density of the rocky soil was determined by using micro-computed tomography (micro-CT) scanning (vivaCT80, Scanco Medical, Bruettisellen, Switzerland), and the obtained radiographic images were reconstructed and analyzed by using Scanco software. Nitrogenase activity was detected with a nitrogenase enzyme-linked immunosorbent assay kit (LB5377B, Liborui, China). Urease activity was assayed following the method of a previous study (Zhang et al., 2011).
Sweetpotato Transcriptome Analysis
The total RNA of sweetpotato root tissue was extracted using TrizolH reagent (Invitrogen, Carlsbad, CA, United States) and then treated with DNase I (Fermentas, Glen Burnie, MD, United States). The RNA concentration, OD260/280 and OD260/230 were measured by using an Agilent 2100 Bioanalyzer (Agilent Technologies, Santa Clara, CA, United States). Qualified libraries were applied for paired-end sequencing (2 × 150 bp) with an Illumina HiSeq 2500 (Illumina, San Diego, CA, United States).
All raw sequencing reads were evaluated with FastQC v0.11.3. The reads containing adapters and low-quality bases were removed. The ribosomal RNA (rRNA) reads were removed by alignment to the rRNA database with Bowtie2 (Langmead and Salzberg, 2012). Approximately 8 Gb of high-quality (Q30 > 92%) clean reads was obtained for each sample after filtering. TopHat2 (version 2.0.3.12) was used to map the clean reads of each sample to the reference genome of Ipomoea batatas (GenBank assembly accession: GCA_002525835.2) (Kim et al., 2013; Yang et al., 2017). The fragments per kilobase of transcript per million mapped reads (FPKM) values were calculated by using Cufflinks (version 2.2.1) (Trapnell et al., 2009).
Microbial 16S and ITS rRNA Sequencing
A PowerSoil DNA Isolation Kit (MOBIO, San Diego, CA, United States) was used to extract total microbial DNA from the rhizosphere rocky soil. The 16S rRNA gene V3−V4 region and ITS2 region were amplified with specific primers (Supplementary Table S2). Qualified libraries were applied for paired-end sequencing (2 × 250 bp) with an Illumina HiSeq 2500 (Illumina).
All raw sequencing reads were evaluated with FastQC v0.11.3. The reads less than 200 bp in length and low-quality reads with a mean quality score below 20 were removed. After filtering, the high-quality reads were assembled into tags (>10 bp overlap and <2% mismatches), and then the tags were filtered using QIIME V1.9.1 (Caporaso et al., 2010). The number of operational taxonomic units (OTUs) was calculated at a distance of 0.03 (97% similarity) by using UPARSE v9.2.64 (Edgar, 2013). The bacterial OTUs were annotated using RDP Classifier v2.2 based on the SILVA database (v128) (Quast et al., 2013) and Greengenes database (July 2010) (DeSantis et al., 2006). Each sample was randomly resampled to 30,000 tags to fairly compare all samples at the same sequencing depth. The fungal OTUs were annotated using RDP Classifier v2.2 based on the UNITE database (version 2016_11_20_ver7) (Abarenkov et al., 2010). Each sample was randomly resampled to 5,000 tags. Alpha diversity indexes (Chao1 index, Sobs, and Shannon index) were calculated using QIIME V1.9.1 (Caporaso et al., 2010).
Tax4Fun was used to predict the bacterial metagenome content based on bacterial composition (Aßhauer et al., 2015), which was calculated with the 16S rRNA gene and annotated with the Greengenes database. The functions were assigned based on the Kyoto Encyclopedia of Gene and Genomes database. FUNGuild was used to parse the ecological guild of the fungal OTUs (Nguyen et al., 2016).
Quantitative Real-Time PCR
Estimation of nifH copy number was performed using quantitative real-time polymerase chain reaction (q-PCR) conducted with a real-time PCR detection system (Bio-Rad Opticon 2, Hercules, CA, United States). The primer pair PolF and PolR was used for q-PCR (Poly et al., 2001). The q-PCR mixtures comprised 12.5 μL of SsoFast EvaGreen Supermix (Bio-Rad), 1 μL each of the forward and reverse primers, 2 μL of template DNA, and 8.5 μL of sterile distilled water for a final volume of 25 μL. The q-PCR conditions comprised 98 °C for 30 s; 40 cycles of 5 s at 95°C, 5 s at 55°C, and 5 s at 72°C; and a final step from 65 to 95°C with an increase of 0.5°C/s to obtain a melting curve. The standard curve was generated by using 10-fold serially diluted plasmids with the nifH gene, and the R2 was 0.996.
Statistical Analyses
The significant differences in sweetpotato and rocky soil properties, nifH copy number and alpha diversity metrics between samples were evaluated using Tukey’s honest significant difference (HSD) test or Student’s two-sample t-test in R 3.5.0. Venn diagram and heatmap analysis were performed in R 3.5.0. Spearman’s correlation coefficients between bacterial communities and rocky soil properties were calculated in R 3.5.0, and network analysis was performed with Cytoscape 3.3.0 (Su et al., 2014).
Results
Rocky Soil Properties
The rocky soil properties were improved after low N fertilization (1.5 g urea/m2). The TC of BF and CK rocky soil increased from 11.2 to 17.2 and 16.4 g/kg, respectively, and the TC of BF rocky soil was higher significantly than that of CK rocky soil 130 DAP (Figure 1A). The DOC of BF and CK rocky soil also increased from 89.2 to 99.6 and 98.6 mg/kg, respectively (Figure 1B). The TN of BF rocky soil increased from 0.8 to 1.0 g/kg, while that of CK rocky soil changed slightly, and TN of BF rocky soil was always higher than that of CK rocky soil (Figure 1C). The AN of BF rocky soil increased significantly from 7.5 to 31.8 mg/kg, and that of CK rocky soil also increased significantly, but the AN of BF rocky soil was always significantly higher than that of CK rocky soil (Figure 1D). The AP of BF and CK rocky soil increased from 0.9 to 2.5 and 2.0 mg/kg respectively, and the AP of BF rocky soil was lower significantly than that of CK rocky soil 60 DAP, but was higher significantly than that of CK rocky soil 130 DAP (Figure 1E). The AK of BF and CK rocky soil increased from 163.6 to 273.9 and 258.2 mg/kg 60 DAP respectively, then decreased 130 DAP, and the AK of BF rocky soil was higher significantly than that of CK rocky soil 60 DAP (Figure 1F).
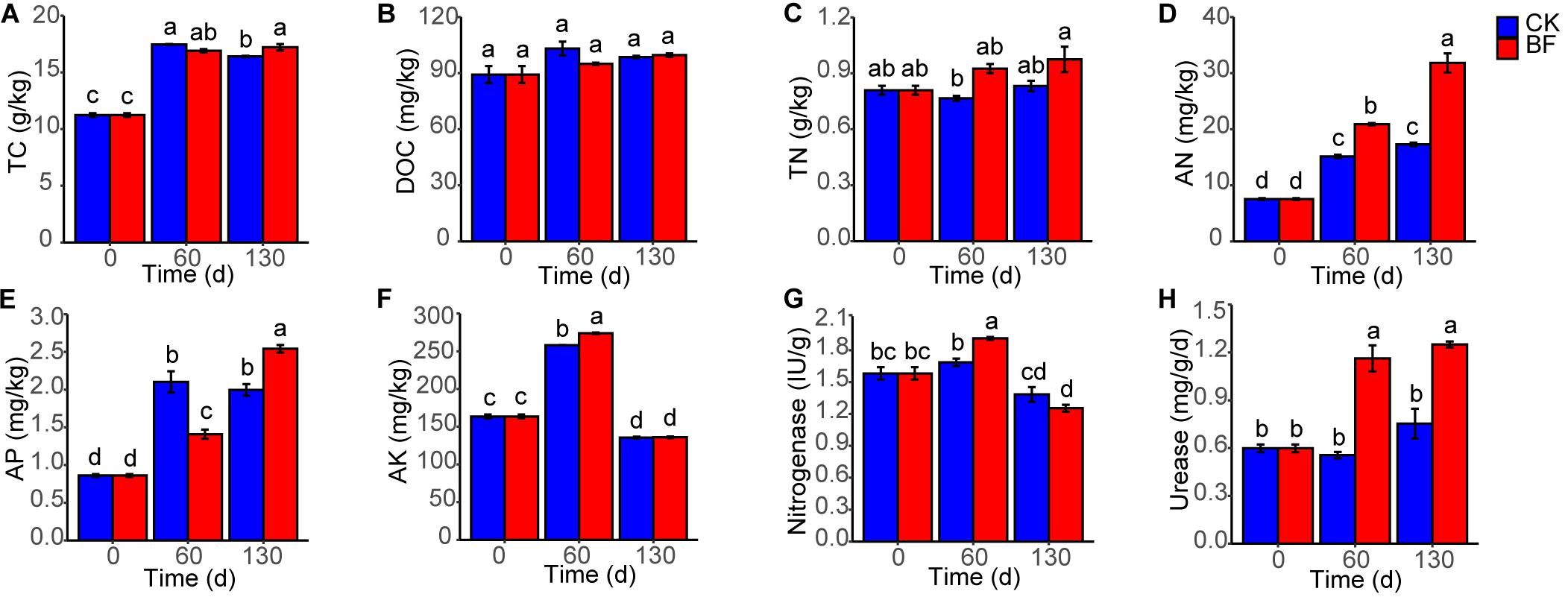
Figure 1. Changes in soil properties. (A) TC, total carbon; (B) DOC, dissolved organic carbon; (C) TN, total nitrogen; (D) AN, available nitrogen; (E) AP, available phosphorus; (F) AK, available potassium; (G) nitrogenase; (H) urease. Different letters denote significant difference from a Tukey’s HSD test (P < 0.05).
The nitrogenase activity of BF rocky soil increased significantly from 1.6 IU (international unit)/g 0 DAP to 1.9 IU/g 60 DAP and was significantly higher than that of CK rocky soil; then, the activity in both of the groups decreased 130 DAP (Figure 1G). The urease activity of BF rocky soil increased from 0.6 to 1.3 mg NH3-N/g/d, while that of CK rocky soil changed slightly, and the urease activity of BF rocky soil was always significantly higher than that of CK rocky soil (Figure 1H).
The bulk density of BF and CK rocky soil decreased from 1,077.5 mg/cm3 (0 DAP) to 1,038.9 and 1,034.0 mg/cm3 (130 DAP), respectively (Supplementary Figure S1). The moisture of BF rocky soil increased from 5.1 to 8.9%, which was almost the same as that of CK rocky soil. The pH of BF rocky soil decreased from 7.9 (0 DAP) to 7.8 (60 DAP) and to 7.6 (130 DAP), while the pH of CK rocky soil decreased to 7.7 (60 DAP) and to 7.8 (130 DAP).
In summary, low N fertilization (1.5 g urea/m2) not only improved TN and AN in the rocky soil, but also increased AP, AK, and nitrogenase and urease activity, indicating the enhanced rocky soil fertility.
Rhizosphere Microorganisms
There were abundant bacteria and fungi in the rhizosphere of sweetpotato, and low N fertilization (1.5 g urea/m2) affected the diversity of microorganisms, especially bacteria. A total of 27,506 bacterial OTUs were identified, in which, 2,379 for BF0 (CK0), 14,673 for CK60, 3,024 for BF60, 6,123 for CK130, and 5,648 for BF130. The number of shared OTUs between samples was just 82, while most OTUs were unique, especially there were 12,873 bacterial unique OTUs in CK60. These bacterial OTUs belong to 40 phyla and 446 genera (Figure 2A). A total of 1,311 fungal OTUs were identified, in which, 448 for BF0 (CK0), 471 for CK60, 459 for BF60, 171 for CK130, and 182 for BF130. Most fungal OTUs were unique, while just 5 fungal OTUs were shared between samples. These fungal OTUs belong to 6 phyla and 175 genera (Figure 2B).
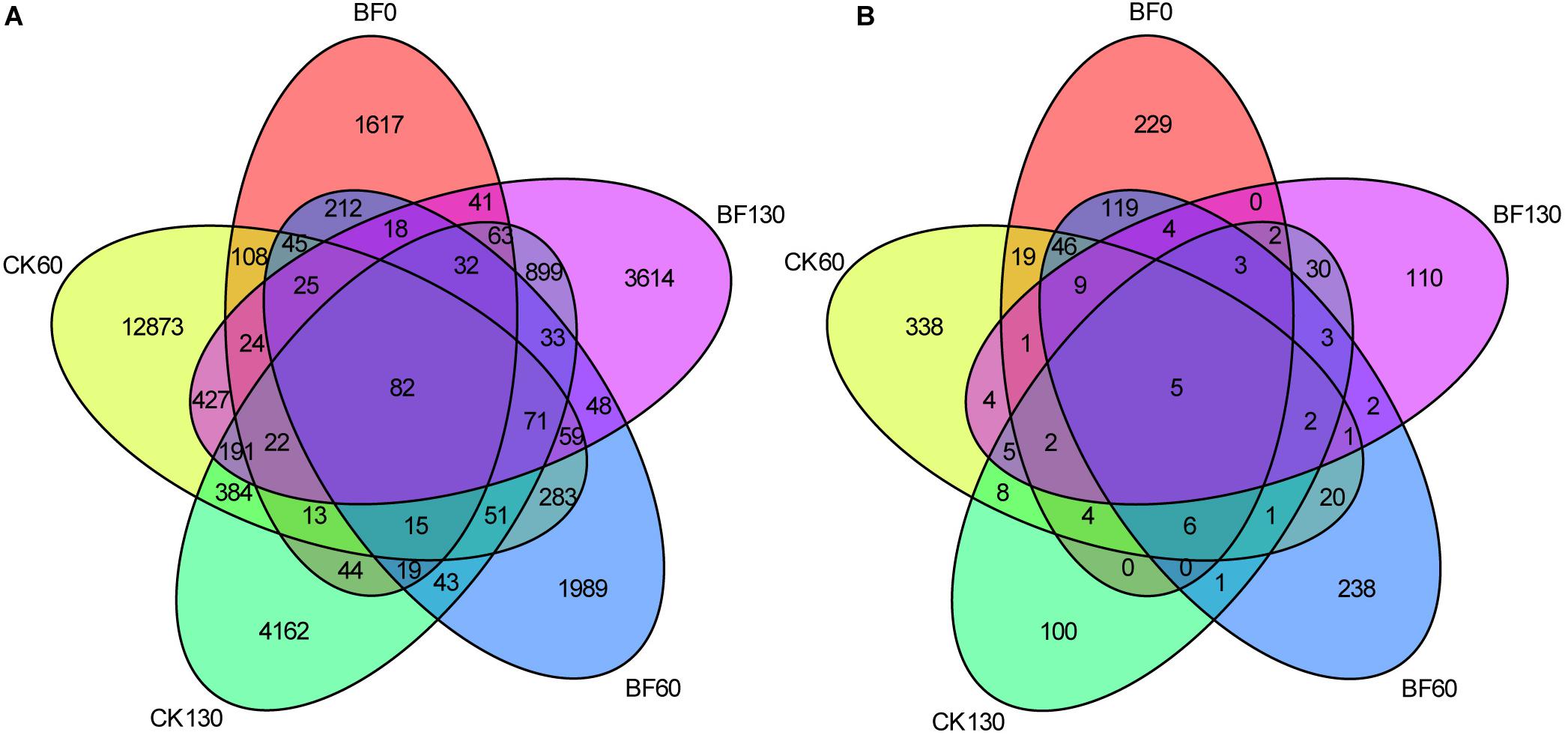
Figure 2. Venn diagram of unique and shared operational taxonomic units (OTUs) among samples. Numbers indicate the number of unique and shared bacterial (A) and fungal OTUs (B).
Low N fertilization (1.5 g urea/m2) reduced rocky soil bacterial alpha diversity in the short term but had little effect in the long term. The bacterial Shannon index of BF rocky soil was 5.8 (60 DAP), which was significantly lower than that (10.7) of CK rocky soil (P = 0.002). However, there was no significant difference between the bacterial Shannon indexes of BF and CK rocky soil 130 DAP (6.6 vs 7.4, respectively; P = 0.218) (Figure 3A). The changes in the bacterial Chao1 and Sobs metrics were similar to the change in the Shannon index (Supplementary Table S3). However, low N fertilization (1.5 g urea/m2) did not reduce rocky soil fungal alpha diversity. There was no significant difference between the fungal Shannon indexes of BF and CK rocky soil 60 DAP (5.0 vs 5.5, respectively; P = 0.267) (Figure 3B). The fungal Chao1 and Sobs metrics of BF rocky soil were even higher than those of CK rocky soil 60 DAP (Supplementary Table S3).
Sweetpotato cultivation and low N fertilization (1.5 g urea/m2) changed the bacterial and fungal community in the rocky soil. For bacteria, the dominant phylum changed from Proteobacteria (37.3% for BF0 (CK0); 62.7% for BF60) to Bacteroidetes (33.8% for BF130). The dominant genus changed from Mycoplasma (13.1%) to Exiguobacterium (14.4% for BF60) and Bacteroides (22.2% for BF130), which were also the dominant genera for CK60 and CK130, respectively (Figure 4A). Heatmap cluster analysis of bacterial genera showed that CK130 and BF130 were grouped together, and BF0 (CK0) and BF60 were grouped together (Figure 4A).
For fungi, the dominant phylum was always Ascomycota during the experiment, while the dominant genus changed from Mortierella (9.6% for BF0 (CK0); 10.7% for BF60) to Cyphellophora (15.4% for BF130). But the dominant genera of CK60 and CK130 were Fusarium and Debaryomyces, respectively (Figure 4B). Heatmap cluster analysis of fungal genera showed that BF0 (CK0) and BF60 were grouped together, and CK60 and CK130 were grouped together (Figure 4B).
More importantly, low N fertilization (1.5 g urea/m2) improved the community of plant growth-promoting bacteria. The relative abundances of phosphorus- and potassium-solubilizing bacteria such as Bradyrhizobium, Agrobacterium and Salmonella increased, and the relative abundance of Bradyrhizobium in BF rocky soil were higher than these in CK rocky soil 60 and 130 DAP (Figure 5A; Sharma et al., 2013). And the relative abundances of N-fixing bacteria such as Enterobacter, Desulfovibrio and Paenibacillus increased in BF rocky soil and were higher than these in CK rocky soil 60 DAP (Figure 5B). Meanwhile, the relative abundances of urease-producing bacteria in BF rocky soil increased 60 DAP, and were always higher than these in CK rocky soil. Especially 60 DAP, the total relative abundance of urease-producing bacteria in group BF rocky soil was 12.2%, while that in CK rocky soil was only 1.6% (Figure 5C).
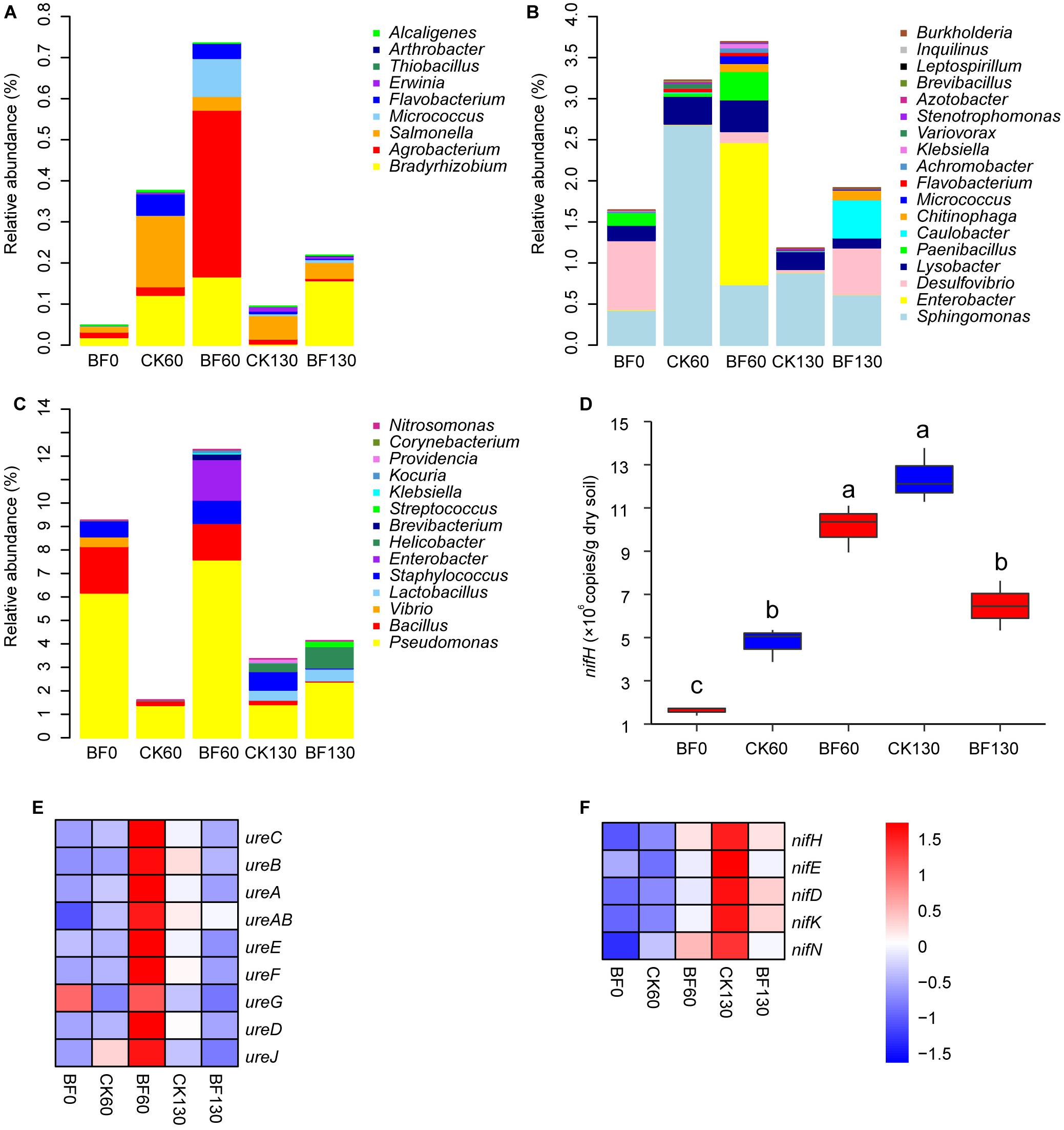
Figure 5. Relative abundance of plant growth-promoting bacteria. (A) phosphorus- and potassium-solubilizing bacterial genera; (B) N fixing bacterial genera; (C) urease-producing bacterial genera; (D) nifH copy number, different letters denote significant difference from a Tukey’s HSD test (P < 0.05); (E) relative abundance of urease coding genes; (F) relative abundance of nitrogenase coding genes.
The nifH copy number was estimated by q-PCR. The nifH copy number of BF rocky soil increased significantly from 1.6 × 106 copies/g dry soil 0 DAP to 1.0 × 107 copies/g dry soil 60 DAP, significantly higher than that (4.8 × 106) in CK rocky soil. Then, the copy number decreased to 6.5 × 106 copies/g dry soil 130 DAP, which was lower than that (1.2 × 107) in CK rocky soil (Figure 5D).
The microbiota’s functions were explored by inferring metagenomes with Tax4Fun. The relative abundances of urease (ureC, ureB, ureA, and ureAB) and urease accessory protein (ureE, ureF, ureG, ureD, and ureJ) increased considerably in BF rocky soil and were higher than those of CK rocky soil 60 DAP. The relative abundance of ureC in BF 0 DAP was 1.8 × 10–4, which increased to 3.5 × 10–4 in BF rocky soil 60 DAP, higher than that (2.0 × 10–4) in CK rocky soil (Figure 5E). The relative abundance of nitrogenase (nifH, nifE, nifD, nifK, and nifN) also increased in BF rocky soil and was higher than that in CK rocky soil 60 DAP. While they were lower than those of CK rocky soil 130 DAP. In particular, the relative abundance of nifH in BF rocky soil 0 DAP was 2.5 × 10–5, and it increased to 5.4 × 10–5 in BF rocky soil 60 DAP, higher than that (3.2 × 10–5) in CK rocky soil (Figure 5F).
The fungal OTUs were assigned to ecological guild with FUNGuild. The relative abundance of plant pathogen and soil saprotroph decreased, while the relative abundance of wood saprotroph and arbuscular mycorrhizal increased. Especially, the relative abundance of plant pathogen in BF rocky soil decreased from 7.8 to 0.8%, which was lower than that of CK130 (6.2%) (Figure 6A). For example, the relative abundance of Gibberella in BF0 (CK0) was 5.2%, which decreased to 2.2% in CK130, however, Gibberella was not detected in BF130 (Figure 6B). While the relative abundance of wood saprotroph of BF rocky soil increased from 0.0008 to 5.9% 130 DAP, which was larger than that of CK130 (5.5%) (Figure 6A).
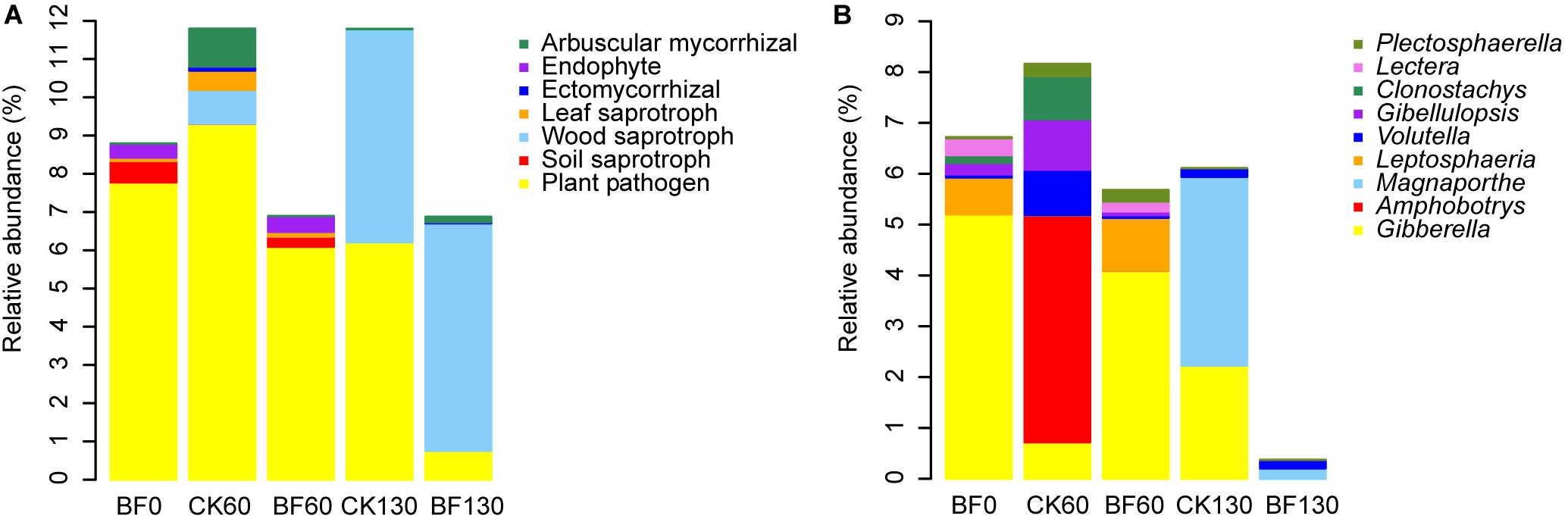
Figure 6. Relative abundance of fungi. (A) fungal ecological guild; (B) the relative abundance of plant pathogen.
Relationships between bacterial community and rocky soil properties were discerned trough network analysis. The result showed that bacteria presented strong relationships with C cycling (invertase, DOC, and TC), mineral element cycling (acid phosphatase, neutral phosphatase, TP, AP, TK, and AK), N cycling (nitrogenase, TN, and AN) and pH (Figure 7). Among those bacterial genera, there were 67 genera presented strong relationships with invertase, and most of which were positive correlations; there were 35, 29, and 29 genera presented strong relationships with AP, acid phosphatase and neutral phosphatase, respectively; and there were 26 genera presented strong relationships with pH (Figure 7).
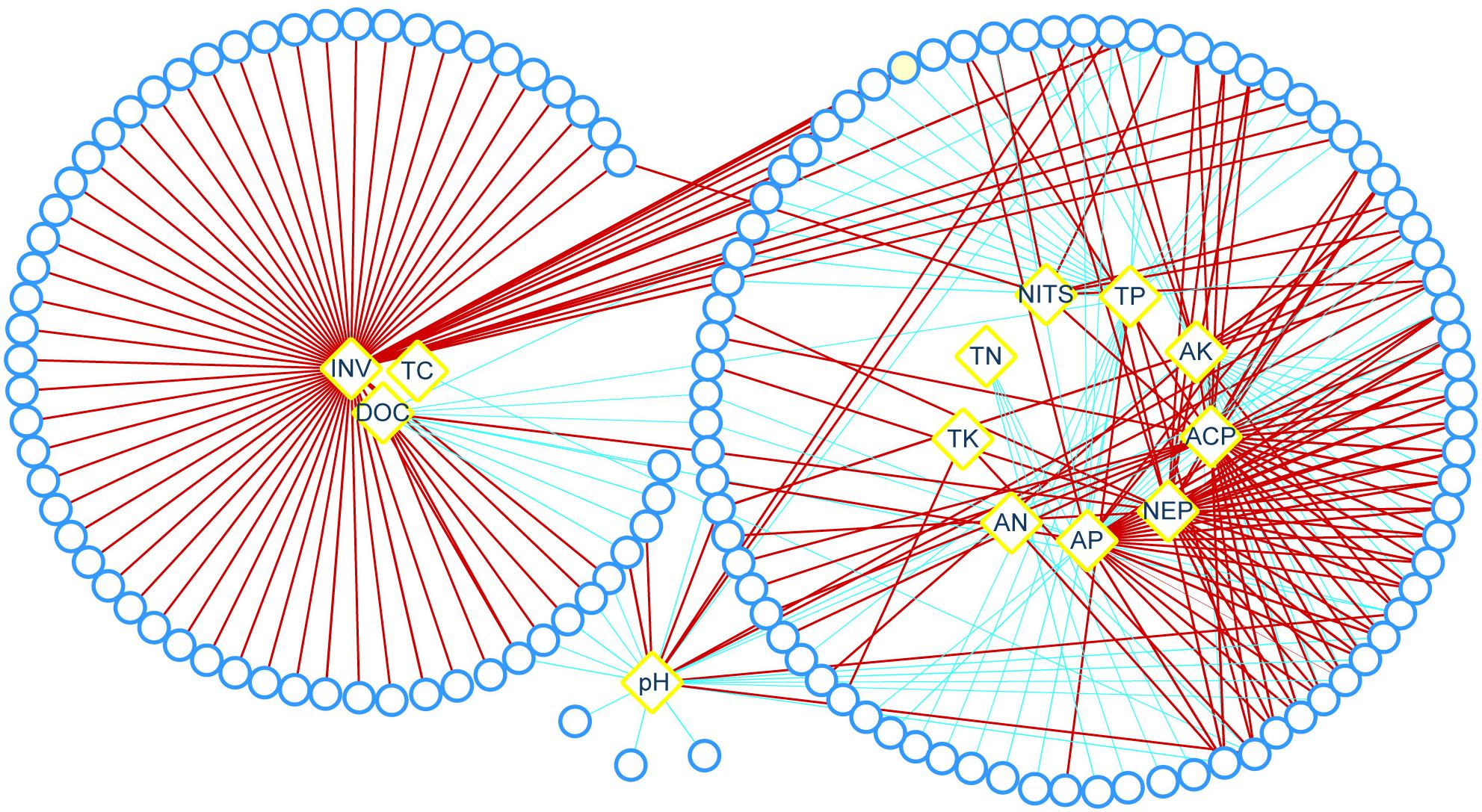
Figure 7. Relationships between bacterial community and soil properties. Red and blue lines represent significant positive and negative correlations, respectively (| Spearman’s ρ| > 0.60; P < 0.05), the width of the lines is proportional to the value of Spearman’s ρ, Round represents bacterial genus, rhombus represents soil property. ACP, acid phosphatase; AK, available potassium; AN, available nitrogen; AP, available phosphorus; DOC, dissolved organic carbon; INV, invertase; NEP, neutral phosphatase; NITS, nitrogenase; TC, total carbon; TK, total potassium; TN, total nitrogen; TP, total phosphorus.
In summary, low N fertilization (1.5 g urea/m2) changed the bacterial and fungal community in the rocky soil, the relative abundance of plant growth-promoting bacteria increased, while that of plant pathogens decreased.
Sweetpotato Tuberous Root Yield, Physiological Properties and Transcriptional Response
After low N fertilization (1.5 g urea/m2), the tuberous root yield of BF sweetpotato increased by half. The tuberous root yields in BF sweetpotato were 0.8 kg/m2 and 3.3 kg/m2 60 DAP and 130 DAP, respectively, and the final yield was higher than that (2.2 kg/m2) of CK sweetpotato. While the vines yields of BF sweetpotato were 0.5 kg/m2 and 1.7 kg/m2 60 DAP and 130 DAP, respectively. And the harvest index (75.9%) of BF sweetpotato was higher than that (70.0%) of CK sweetpotato. The whole-plant N absorption of BF sweetpotato was 8.8 g N/m2, which was higher than that (5.9 g N/m2) in CK sweetpotato and much higher than the amount of N in the urea applied (0.7 g N/m2). The N production efficiency (377.8 kg/kg) of BF sweetpotato was similar to that (387.8 kg/kg) of CK sweetpotato.
The root exudates C and N (15.9 and 4.6 μg/g/h) of BF sweetpotato were lower significantly than those (34.0 and 12.6 μg/g/h) of CK sweetpotato 60 DAP, while there was no significant difference 130 DAP (Figures 8A,B). The starch content of BF sweetpotato was significantly higher than that of CK sweetpotato 60 DAP, while there was no significant difference between BF and CK groups when harvesting occurred 130 DAP (Figure 8C).
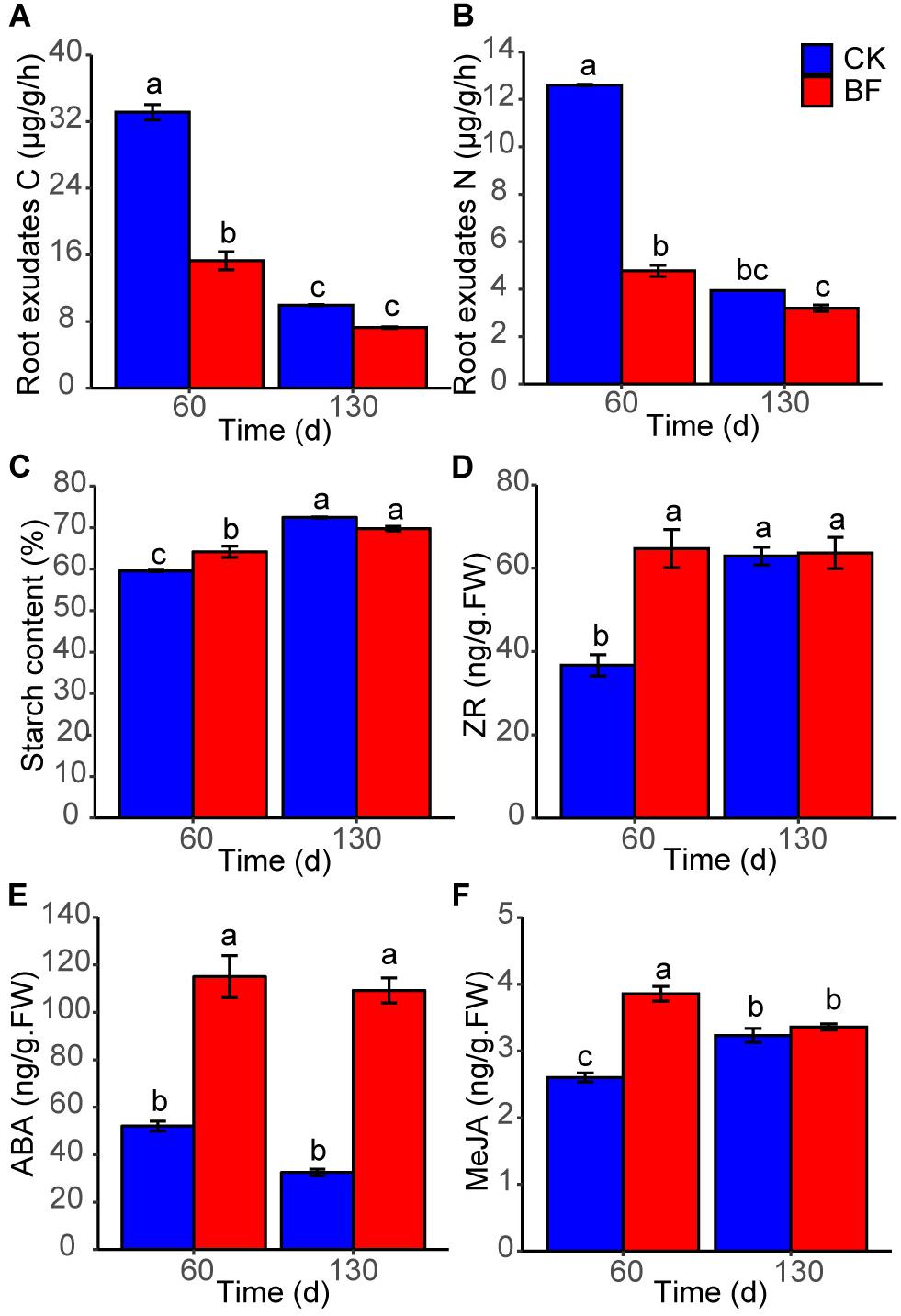
Figure 8. The physiological properties of sweetpotato. (A) root exudates carbon; (B) root exudates nitrogen; (C) starch content; (D) ZR, zeatin riboside; (E) ABA, abscisic acid; (F) MeJA, methyl jasmonate. Different letters denote significant difference from a Tukey’s HSD test (P < 0.05).
The phytohormone content of sweetpotato tuberous roots was measured. The ZR content of BF sweetpotato was 64.7 ng/g (fresh weight, FW) 60 DAP, which was higher than that [51.8 ng/g (FW)] of CK sweetpotato (Figure 8D). The ABA contents of BF sweetpotato were always significantly higher than those of CK sweetpotato (BF vs CK: 115.0 vs 52.0 ng/g (FW), 60 DAP; 109.2 vs 32.5 ng/g (FW), 130 DAP) (Figure 8E). The MeJA content [3.9 ng/g (FW)] of BF sweetpotato was significantly higher than that [2.6 ng/g (FW)] of CK sweetpotato 60 DAP, while there was no significant difference between the two groups 130 DAP (Figure 8F).
After low N fertilization (1.5 g urea/m2), the expression of ZR biosynthesis genes IPT and CYP735A was upregulated, while the expression of ZR degradation gene CKX and transport gene ABCG14 was downregulated in BF sweetpotato compared with that in CK sweetpotato (Figure 9). The expression level of KNOX1 (Homeobox protein knotted-1-like 1) in BF sweetpotato was higher than that in CK sweetpotato 60 DAP, while it was slightly lower 130 DAP. In addition, the expression of lignin biosynthesis genes PAL, C4H, and CCR was downregulated in BF sweetpotato compared with that in CK sweetpotato (Figure 9).
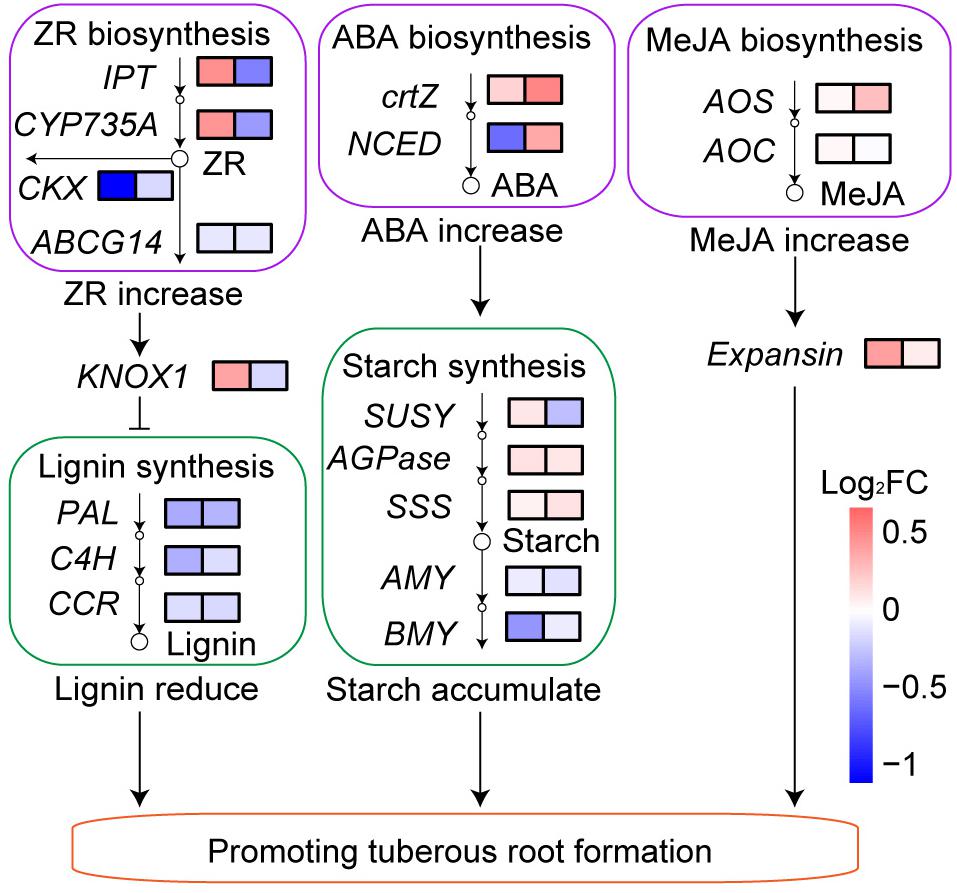
Figure 9. Transcriptional response of sweetpotato to low N fertilization. FC, fold change of the expression level of genes in BF sweetpotato compared with that in CK sweetpotato 60 and 130 DAP.
The expression of ABA biosynthesis genes crtZ and NCED was upregulated in BF sweetpotato compared with that in CK sweetpotato (Figure 9). And the expression of starch biosynthesis genes SUSY, AGPase and SSS was upregulated while the expression of starch degradation genes AMY and BMY was downregulated in BF sweetpotato compared with that in CK sweetpotato (Figure 9).
The expression of MeJA biosynthesis genes AOS and AOC was also upregulated in BF sweetpotato compared with that in CK sweetpotato (Figure 9). The expression of Expansin was upregulated in BF sweetpotato compared with that in CK sweetpotato (Figure 9).
In summary, low N fertilization (1.5 g urea/m2) increased the sweetpotato yield by half, up to 3.3 kg/m2. Physiological and transcriptional data indicated that the phytohormones ZR, ABA, and MeJA contents increased in the sweetpotato root.
Discussion
Sweetpotato Cultivation and Low Nitrogen Fertilization Alter Microorganism Diversity in the Rocky Soil
Most microorganisms are in dormant state in natural soils, especially in the low-organic-matter soil, and these dormant microorganisms are revived immediately and exhibit increased biodiversity if access to organic matter (Joergensen and Wichern, 2018). Sweetpotato provided organic matter for microorganisms in the form of root exudates (Figures 8A,B), and the soil TC, DOC, TN and AN increased (Figures 1A–D), which might induce the increase in microorganism diversity in the rocky soil. We found abundant bacteria and fungi in the rocky soil, and the number of bacterial OTUs (27,506) was larger than that of fungal OTUs (1,311). And the numbers of shared OTUs between samples were just 82 and 5 for bacteria and fungi, respectively, while most OTUs were unique (Figure 2). These results indicated that the microorganism community varied greatly among different samples, which may be affected by sweetpotato cultivation and low N fertilization (1.5 g urea/m2).
Interestingly, low N fertilization (1.5 g urea/m2) reduced rocky soil bacterial diversity in the short term but had little effect in the long term, and had a weaker effect on rocky soil fungal diversity (Figure 3 and Supplementary Table S3). Previous studies found that bacteria were primarily influenced by soil properties, while fungi were primarily influenced by plants (Nielsen et al., 2010; Zhalnina et al., 2015). In our study, network analysis showed that bacteria presented strong relationships with rocky soil properties such as pH, AN, AP, and AK (Figure 7), which might affect rocky soil microorganism community. Moreover, as one of the most important source of C and N nutrients for microorganisms (Sasse et al., 2018), the root exudates C and N of BF sweetpotato were just half of those of CK sweetpotato 60 DAP (Figures 8A,B). This result indicated that applying urea inhibited the secreting of sweetpotato root exudates, which might affect rocky soil microorganism community indirectly. However, urea is a quick-acting N source, and the application amount in this study is low, these might explain the little effect on bacterial diversity in the long term (Figure 3A and Supplementary Table S3).
Microorganism Community Improved Rocky Soil Quality
Microorganisms act as keystone players in improving mineral fertility (such as P and K) and N fixation in soil (Pii et al., 2015; Ciccazzo et al., 2016). The TP and TK in the rocky soil were ample. However, most of the TP and TK combined with minerals and were unavailable for plants (Chatterjee et al., 2014). These unavailable P and K can be transformed to available form by P and K-solubilizing microorganisms through dissolution and/or mineralization (Etesami et al., 2017; Granada et al., 2018). In this study, we found that sweetpotato cultivation and low N fertilization (1.5 g urea/m2) improved the relative abundance of P and K-solubilizing bacteria such as Bradyrhizobium, Agrobacterium and Salmonella (Figure 5A) (Sharma et al., 2013). Moreover, AP of BF130 was larger than that of CK130, and AK of BF60 was larger than that of CK60 (Figures 1E,F). Meanwhile, the total relative abundance of P and K-solubilizing microorganisms in BF rocky soil was larger than that in CK rocky soil (Figure 5A). Those results suggested that low N fertilization (1.5 g urea/m2) might improve rocky soil AP and AK through P- and K-solubilizing microorganisms.
N fixation by microorganisms is one of the most important source of soil N (Ladha et al., 2016). In our study, the whole-plant N absorption of BF sweetpotato was 3.0 g N/m2 higher than that of CK sweetpotato, and the difference was much larger than the amount of N in the urea applied (0.7 g N/m2). In addition, both the TN and AN contents in BF rocky soil were higher than those in CK rocky soil (Figures 1C,D). These results indicated that BF rocky soil had higher levels of other sources of N than CK rocky soil, except for the urea applied. This source might be N fixation by microorganisms.
We found that the relative abundances of N-fixing bacteria such as Enterobacter, Desulfovibrio and Paenibacillus in BF rocky soil were higher than these in CK rocky soil 60 DAP (Figure 5B) (Newman and Postgate, 1968; Roesch et al., 2008). And the copy number of nifH increased in BF rocky soil and was higher than that in CK rocky soil 60 DAP (Figure 5D). The results from Tax4Fun also supported this finding (Figure 5F). In addition, the nitrogenase activity of BF rocky soil increased and was significantly higher than that of CK rocky soil 60 DAP (Figure 1G). These results suggested that biological N fixation in BF rocky soil was stronger than that in CK rocky soil 60 DAP. Our results were consistent with the results of another study in which urea addition (100 mg/kg soil) increased nifH abundance in Antarctic soils, whose TN and AN contents were as low as those in the N-deficient rocky soil in our study (Jung et al., 2011). Our results were also consistent with those from a previous study in which urea fertilization increased nifH abundance in a semiarid grassland on the northern Loess Plateau of China, where the TN content was only half of that in the rocky soil that we studied (Zhang et al., 2019). This finding was inconsistent with the findings of previous studies in which N fertilization (30 g N/m2/year) inhibited biological N fixation (Wang et al., 2017). The reasons for this difference may be that (1) the original N content in the rocky soil and the level of urea applied to the rocky soil were very low, as in the Antarctic soils and semiarid grassland (Jung et al., 2011; Zhang et al., 2019). A previous study also found that nifH gene abundance increased under low N rates (5–15 g N/m2/year) but was suppressed under high N rates (50 g N/m2/year) (Ning et al., 2015). It was suggested that low N fertilization (0.7 g N/m2) may have promoted the growth of N-fixing microorganisms in the N-deficient soil. And (2) the influence on N fixation activity was milder for urea than for nitrate and ammonium (Yamashita et al., 2019).
Urease catalyzes the hydrolysis of urea to ammonia for plant absorption and utilization (Kappaun et al., 2018). Microorganisms are important source of urease in soil (Sun et al., 2019). The relative abundance of urease-producing bacteria in BF rocky soil increased and was greater than that in CK rocky soil (Figure 5C). The Tax4Fun results revealed that the relative abundance of urease and urease accessory protein increased considerably in BF rocky soil after urea was applied (Figure 5E). We also found that the urease activity of BF rocky soil increased to more than 2 times and was always significantly higher than that in CK rocky soil (Figure 1H). These results suggested that applying urea promoted the increase in urease-related microbial relative abundance and further led to the increase in soil urease activity.
Interestingly, low N fertilization (1.5 g urea/m2) inhibited plant pathogenic bacteria such as Mycoplasma and Ralstonia (Figure 4A), which can cause plant yellowing and bacterial wilt, respectively (Whitcomb and Davis, 1970; Genin and Denny, 2012). Moreover, low N fertilization (1.5 g urea/m2) inhibited plant pathogenic fungi (Figure 6). For example, Gibberella (Figure 6B), which is responsible for many plant diseases causing great economic losses, including bakanae disease of Oryza sativa (Nirenberg and O’Donnell, 1998). In addition, sweetpotato cultivation and low N fertilization (1.5 g urea/m2) improved the relative abundance of wood saprotroph (Figure 6A), which promoted degradation of organic matter from sweetpotato.
Increased Phytohormones Promoted the Formation of Sweetpotato Tuberous Roots
Low N fertilization improved soil fertility, especially the AN increased significantly. N is an important nutrient for plant growth, development and production (Leghari et al., 2016). Higher plants have evolved a series of phytohormones such as cytokinins, ABA and MeJA that respond to N signaling and then regulate their physiological and morphological changes (Kiba et al., 2011; Vanstraelen and Benkova, 2012).
Cytokinins are mainly produced in the root and participate in the basic development processes of plants, such as cell division and proliferation, rooting and vascular bundle development (Heyl et al., 2012). N signaling could regulate the growth of each part of the plant through cytokinins (Kiba et al., 2011). In our study, the upregulated expression of ZR (a kind of cytokinins) biosynthesis genes and downregulated expression of ZR degradation and transport genes might led to the higher ZR content of BF sweetpotato than that of CK sweetpotato (Figures 8D, 9). Previous studies found ZR can upregulated the expression level of KNOX1 (Rupp et al., 1999), and KNOX1 is known to inhibit lignin synthesis (Townsley et al., 2013). In our study, the expression of KNOX1 was upregulated, and the expression of lignin biosynthesis genes was downregulated in BF sweetpotato compared with that in CK sweetpotato (Figure 9). Previous study in sweetpotato found that KNOX1 was upregulated during tuberous root formation, and could promote tuberous root formation (Tanaka et al., 2008). In addition, an important sign of sweetpotato tuberous root formation is the reduction in lignin synthesis (Firon et al., 2013; Ponniah et al., 2017). Thus, ZR might inhibit lignin biosynthesis through KNOX1 and further promoted the tuberous root formation of BF sweetpotato.
ABA plays a key role in plant stress resistance and the regulation of storage organ and root development, and is closely linked to N signaling (Kiba et al., 2011; Yu et al., 2019). In our study, the upregulated expression of ABA biosynthesis genes might explain why the ABA content of BF sweetpotato was 2–3 times that of CK sweetpotato (Figures 8E, 9). ABA can promote sucrose uptake and unloading in sink organs and promote starch synthesis by increasing the expression of AGPase (Akihiro et al., 2005; Nagata and Saitou, 2009). We found the expression of the key enzymes in the starch synthesis pathway, AGPase and SSS, was upregulated, while the expression of starch degradation genes was downregulated in BF sweetpotato. All of these factors led to starch accumulation in sweetpotato tuberous roots (Figure 9), which further promoted the tuberous root formation of BF sweetpotato.
MeJA is associated with tuberization, fruit ripening and senescence (Wasternack and Hause, 2013). In our study, the upregulated expression of MeJA biosynthesis genes might explain the higher MeJA content of BF sweetpotato 60 DAP (Figures 8F, 9). MeJA was found to promote the expression of Expansin in previous research (Han et al., 2012; Ochiai et al., 2013). And Expansin causes loosening and extension of plant cell walls, which are instrumental in seed development and yield (Bae et al., 2014). Previous studies also found Expansin involve in the initiating tuberous root of sweetpotato (Firon et al., 2013; Ponniah et al., 2017). In our study, with the increase in MeJA content, the expression of Expansin was upregulated in BF sweetpotato compared with that in CK sweetpotato (Figure 9), and upregulated expression of Expansin promoted the tuberous root formation of BF sweetpotato. All of these factors led to the increase in BF sweetpotato yield.
Conclusion
In this study, low N fertilization (1.5 g urea/m2) in a rocky soil increased the sweetpotato yield by half, up to 3.3 kg/m2. We found low N fertilization reduced rocky soil bacterial diversity in the short term through changes of sweetpotato root exudates and rocky soil properties, but had a weaker effect on rocky soil fungal diversity. Moreover, low N fertilization improved rocky soil quality through increasing relative abundance of P and K-solubilizing bacteria, N-fixing and urease-producing bacteria, and decreasing relative abundance of plant pathogens, besides supplying N directly. Furthermore, improved rocky soil quality, especially AN, induced phytohormonal responses of sweetpotato: the ZR, ABA, and MeJA contents in BF sweetpotato root increased, which promoted the formation of tuberous roots and further increased the sweetpotato yield. Our study explored the effect of low N fertilization on improving microorganism community and sweetpotato yield in the N-deficient rocky soil, which could promote sustainable agricultural development and help mitigate the food crisis. Considering the impact of excessive fertilization on the environment, low N fertilization and plant growth-promoting bacteria provide good options for environment-friendly agriculture. Further research should avoid excessive fertilization through searching for plant growth-promoting bacteria and applying fertilizer according to local soil nutrients.
Data Availability Statement
The raw sequencing data (the transcriptome, 16S and ITS rRNA gene fastq files) are publicly available in the NCBI Sequence Read Archive (SRA) under the SRA accession number SRR10257314–SRR10257319, SRR10257329–SRR10257334, SRR10257376–SRR10257381, SRR9587993–SRR9587998, SRR9588616–SRR9588619, SRR9588634, SRR9588636–SRR9588639, and SRR9588647–SRR9588655.
Author Contributions
HZ, YF, and KH contributed to the study conception and design. Material preparation, data collection and analysis were performed by YD, YJ, ZY, LT, LL, MT, and AD. The first draft of the manuscript was written by YD and all authors commented on previous versions of the manuscript. All authors read and approved the final manuscript.
Funding
This study was supported by the China Agriculture Research System (CARS-10-B22 and CARS-10-05B), the National Key R&D Program of China (2018YFB1501401 and 2018YFF0213505), the National Natural Science Foundation of China (21606218 and 31770395) and Innovation Academy for Seed Design, CAS.
Conflict of Interest
The authors declare that the research was conducted in the absence of any commercial or financial relationships that could be construed as a potential conflict of interest.
Acknowledgments
We thank Sulan He and Mei Wang from the Nanchong Academy of Agricultural Sciences for assistance with sample collection. We thank Professor Yongchun Zhang from the Jiangsu Academy of Agricultural Sciences for providing valuable suggestions regarding soil nutrient research. We also thank Li Chen from Sichuan University for conducting micro-CT scanning.
Supplementary Material
The Supplementary Material for this article can be found online at: https://www.frontiersin.org/articles/10.3389/fmicb.2020.00678/full#supplementary-material
Abbreviations
ABA, abscisic acid; AK, available potassium; AN, available nitrogen; AP, available phosphorus; BF, the treatment group with 1.5 g urea/m2; C, carbon; CK, the control group; DAP, days after planting; DOC, dissolved organic carbon; FPKM, fragments per kilobase of transcript per million mapped reads; FW, fresh weight; IU, international unit; MeJA, methyl jasmonate; N, nitrogen; OTUs, operational taxonomic units; q-PCR, quantitative real-time polymerase chain reaction; rRNA, ribosomal RNA; TC, total carbon; TK, total potassium; TN, total nitrogen; TP, total phosphorous; ZR, zeatin riboside.
References
Abarenkov, K., Nilsson, R. H., Larsson, K. H., Alexander, I. J., Eberhardt, U., Erland, S., et al. (2010). The UNITE database for molecular identification of fungi - recent updates and future perspectives. New Phytol. 186, 281–285. doi: 10.1111/j.1469-8137.2009.03160.x
Akihiro, T., Mizuno, K., and Fujimura, T. (2005). Gene expression of ADP-glucose pyrophosphorylase and starch contents in rice cultured cells are cooperatively regulated by sucrose and ABA. Plant Cell Physiol. 46, 937–946. doi: 10.1093/pcp/pci101
Aßhauer, K. P., Wemheuer, B., Daniel, R., and Meinicke, P. (2015). Tax4Fun: predicting functional profiles from metagenomic 16S rRNA data. Bioinformatics 31, 2882–2884. doi: 10.1093/bioinformatics/btv287
Bae, J. M., Kwak, M. S., Noh, S. A., Oh, M. J., Kim, Y. S., and Shin, J. S. (2014). Overexpression of sweetpotato expansin cDNA (IbEXP1) increases seed yield in Arabidopsis. Transgenic Res. 23, 657–667. doi: 10.1007/s11248-014-9804-1
Caporaso, J. G., Kuczynski, J., Stombaugh, J., Bittinger, K., Bushman, F. D., Costello, E. K., et al. (2010). QIIME allows analysis of high-throughput community sequencing data. Nat. Methods 7, 335–336. doi: 10.1038/nmeth.f.303
Chatterjee, D., Datta, S., and Manjaiah, K. (2014). Fractions, uptake and fixation capacity of phosphorus and potassium in three contrasting soil orders. J. Soil Sci. Plant Nutr. 14, 640–656. doi: 10.4067/S0718-95162014005000051
Chaudhary, D. R., Gautam, R. K., Yousuf, B., Mishra, A., and Jha, B. (2015). Nutrients, microbial community structure and functional gene abundance of rhizosphere and bulk soils of halophytes. Appl. Soil Ecol. 91, 16–26. doi: 10.1016/j.apsoil.2015.02.003
Chen, X., Kou, M., Tang, Z., Zhang, A., and Li, H. (2017). The use of humic acid urea fertilizer for increasing yield and utilization of nitrogen in sweet potato. Plant Soil Environ. 63, 201–206. doi: 10.17221/24/2017-pse
Ciccazzo, S., Esposito, A., Borruso, L., and Brusetti, L. (2016). Microbial communities and primary succession in high altitude mountain environments. Ann. Microbiol. 66, 43–60. doi: 10.1007/s13213-015-1130-1
Deng, X. G., Zhu, F., Li, J. Y., Feng, H., Xi, D. H., and Lin, H. H. (2014). Genetic diversity and phylogentic analysis of sweet potato feathery mottle virus and sweet potato virus G in Sichuan, China. J. Plant Pathol. 96, 215–218. doi: 10.4454/JPP.V96I1.002
DeSantis, T. Z., Hugenholtz, P., Larsen, N., Rojas, M., Brodie, E. L., Keller, K., et al. (2006). Greengenes, a chimera-checked 16S rRNA gene database and workbench compatible with ARB. Appl. Environ. Microbiol. 72, 5069–5072. doi: 10.1128/AEM.03006-05
Dick, W., Cheng, L., and Wang, P. (2000). Soil acid and alkaline phosphatase activity as pH adjustment indicators. Soil Biol. Biochem. 32, 1915–1919. doi: 10.1016/s0038-0717(00)00166-8
Edgar, R. C. (2013). UPARSE: highly accurate OTU sequences from microbial amplicon reads. Nat. Methods 10:996. doi: 10.1038/nmeth.2604
Etesami, H., Emami, S., and Alikhani, H. A. (2017). Potassium solubilizing bacteria (KSB): mechanisms, promotion of plant growth, and future prospects - a review. J. Soil Sci. Plant Nutr. 17, 897–911.
Feng, M. M., Adams, J. M., Fan, K. K., Shi, Y., Sun, R. B., Wang, D. Z., et al. (2018). Long-term fertilization influences community assembly processes of soil diazotrophs. Soil Biol. Biochem. 126, 151–158. doi: 10.1016/j.soilbio.2018.08.021
Firon, N., LaBonte, D., Villordon, A., Kfir, Y., Solis, J., Lapis, E., et al. (2013). Transcriptional profiling of sweetpotato (Ipomoea batatas) roots indicates down-regulation of lignin biosynthesis and up-regulation of starch biosynthesis at an early stage of storage root formation. BMC Genomics 14:460. doi: 10.1186/1471-2164-14-460
Food and Agriculture Organization (2019). Available online at: http://www.fao.org/ (accessed August 15, 2019).
Genin, S., and Denny, T. P. (2012). Pathogenomics of the Ralstonia solanacearum species complex. Annu. Rev. Phytopathol. 50, 67–89. doi: 10.1146/annurev-phyto-081211-173000
Granada, C. E., Passaglia, L. M. P., de Souza, E. M., and Sperotto, R. A. (2018). Is phosphate solubilization the forgotten child of plant growth-promoting rhizobacteria? Front. Microbiol. 9:2054. doi: 10.3389/fmicb.2018.02054
Han, Y. Y., Li, A. X., Li, F., Zhao, M. R., and Wang, W. (2012). Characterization of a wheat (Triticum aestivum L.) expansin gene, TaEXPB23, involved in the abiotic stress response and phytohormone regulation. Plant Physiol. Biochem. 54, 49–58. doi: 10.1016/j.plaphy.2012.02.007
He, F., Zhang, H. Q., and Tang, M. (2016). Aquaporin gene expression and physiological responses of Robinia pseudoacacia L. to the mycorrhizal fungus Rhizophagus irregularis and drought stress. Mycorrhiza 26, 311–323. doi: 10.1007/s00572-015-0670-3
Helliwell, J. R., Miller, A. J., Whalley, W. R., Mooney, S. J., and Sturrock, C. J. (2014). Quantifying the impact of microbes on soil structural development and behaviour in wet soils. Soil Biol. Biochem. 74, 138–147. doi: 10.1016/j.soilbio.2014.03.009
Heyl, A., Riefler, M., Romanov, G. A., and Schmulling, T. (2012). Properties, functions and evolution of cytokinin receptors. Eur. J. Cell Biol. 91, 246–256. doi: 10.1016/j.ejcb.2011.02.009
Joergensen, R. G., and Wichern, F. (2018). Alive and kicking: why dormant soil microorganisms matter. Soil Biol. Biochem. 116, 419–430. doi: 10.1016/j.soilbio.2017.10.022
Jung, J., Yeom, J., Kim, J., Han, J., Lim, H. S., Park, H., et al. (2011). Change in gene abundance in the nitrogen biogeochemical cycle with temperature and nitrogen addition in Antarctic soils. Res. Microbiol. 162, 1018–1026. doi: 10.1016/j.resmic.2011.07.007
Kappaun, K., Piovesan, A. R., Carlini, C. R., and Ligabue-Braun, R. (2018). Ureases: historical aspects, catalytic, and non-catalytic properties - A review. J. Adv. Res. 13, 3–17. doi: 10.1016/j.jare.2018.05.010
Kiba, T., Kudo, T., Kojima, M., and Sakakibara, H. (2011). Hormonal control of nitrogen acquisition: roles of auxin, abscisic acid, and cytokinin. J. Exp. Bot. 62, 1399–1409. doi: 10.1093/jxb/erq410
Kim, D., Pertea, G., Trapnell, C., Pimentel, H., Kelley, R., and Salzberg, S. L. (2013). TopHat2: accurate alignment of transcriptomes in the presence of insertions, deletions and gene fusions. Genome Biol. 14:R36. doi: 10.1186/gb-2013-14-4-r36
Ladha, J. K., Tirol-Padre, A., Reddy, C. K., Cassman, K. G., Verma, S., Powlson, D. S., et al. (2016). Global nitrogen budgets in cereals: a 50-year assessment for maize, rice, and wheat production systems. Sci. Rep. 6:19355. doi: 10.1038/srep19355
Langmead, B., and Salzberg, S. L. (2012). Fast gapped-read alignment with Bowtie 2. Nat. Methods 9, 357–359. doi: 10.1038/nmeth.1923
Leghari, S. J., Wahocho, N. A., Laghari, G. M., HafeezLaghari, A., MustafaBhabhan, G., HussainTalpur, K., et al. (2016). Role of nitrogen for plant growth and development: a review. Adv. Environ. Biol. 10, 209–218.
Liu, Y., Fang, Y., Huang, M., Jin, Y., Sun, J., Tao, X., et al. (2015). Uniconazole-induced starch accumulation in the bioenergy crop duckweed (Landoltia punctata) II: transcriptome alterations of pathways involved in carbohydrate metabolism and endogenous hormone crosstalk. Biotechnol. Biofuels 8:6. doi: 10.1186/s13068-015-0245-8
Luo, Y. L., Wu, X. L., Tang, D. B., Liu, X., Lei, Y. Y., Lv, C. W., et al. (2017). Effect of maize (Zea mays L.) plant-type on yield and photosynthetic characters of sweet potato (Ipomoea batatas L.) in intercropping system. Not. Bot. Horti Agrobo. 45, 245–254. doi: 10.15835/nbha45110421
Manning, D. A. C., and Theodoro, S. H. (2018). Enabling food security through use of local rocks and minerals. Extract. Ind. Soc. doi: 10.1016/j.exis.2018.11.002
Nagata, T., and Saitou, K. (2009). Regulation of expression of D3-type cyclins and ADP-glucose pyrophosphorylase genes by sugar, cytokinin and ABA in sweet potato (Ipomoea batatas Lam.). Plant Prod. Sci. 12, 434–442. doi: 10.1626/pps.12.434
Newman, D. J., and Postgate, J. R. (1968). Rubredoxin from a nitrogen-fixing variety of Desulfovibrio desulfuricans. Eur. J. Biochem. 7, 45–48. doi: 10.1111/j.1432-1033.1968.tb19571.x
Nguyen, N. H., Song, Z. W., Bates, S. T., Branco, S., Tedersoo, L., Menke, J., et al. (2016). FUNGuild: an open annotation tool for parsing fungal community datasets by ecological guild. Fungal Ecol. 20, 241–248. doi: 10.1016/j.funeco.2015.06.006
Nielsen, U. N., Osler, G. H. R., Campbell, C. D., Burslem, D. F. R. P., and Wal, R. V. D. (2010). The influence of vegetation type, soil properties and precipitation on the composition of soil mite and microbial communities at the landscape scale. J. Biogeogr. 37, 1317–1328. doi: 10.1111/j.1365-2699.2010.02281.x
Ning, Q. S., Gu, Q., Shen, J. P., Lv, X. T., Yang, J. J., Zhang, X. M., et al. (2015). Effects of nitrogen deposition rates and frequencies on the abundance of soil nitrogen-related functional genes in temperate grassland of northern China. J. Soils Sed. 15, 694–704. doi: 10.1007/s11368-015-1061-2
Nirenberg, H. I., and O’Donnell, K. (1998). New Fusarium species and combinations within the Gibberella fujikuroi species complex. Mycologia 90, 434–458. doi: 10.2307/3761403
Ochiai, M., Matsumoto, S., and Yamada, K. (2013). Methyl jasmonate treatment promotes flower opening of cut Eustoma by inducing cell wall loosening proteins in petals. Postharvest Biol. Technol. 82, 1–5. doi: 10.1016/j.postharvbio.2013.02.018
Pii, Y., Mimmo, T., Tomasi, N., Terzano, R., Cesco, S., and Crecchio, C. (2015). Microbial interactions in the rhizosphere: beneficial influences of plant growth-promoting rhizobacteria on nutrient acquisition process. A review. Biol. Fertility Soils 51, 403–415. doi: 10.1007/s00374-015-0996-1
Poly, F., Monrozier, L. J., and Bally, R. (2001). Improvement in the RFLP procedure for studying the diversity of nifH genes in communities of nitrogen fixers in soil. Res. Microbiol. 152, 95–103. doi: 10.1016/s0923-2508(00)01172-4
Ponniah, S. K., Thimmapuram, J., Bhide, K., Kalavacharla, V., and Manoharan, M. (2017). Comparative analysis of the root transcriptomes of cultivated sweetpotato(Ipomoea batatas L. Lam) and its wild ancestor (Ipomoea trifida Kunth G. Don). BMC Plant Biol. 17:9. doi: 10.1186/s12870-016-0950-x
Quast, C., Pruesse, E., Yilmaz, P., Gerken, J., Schweer, T., Yarza, P., et al. (2013). The SILVA ribosomal RNA gene database project: improved data processing and web-based tools. Nucleic Acids Res. 41, D590–D596. doi: 10.1093/nar/gks1219
Roesch, L. F. W., Camargo, F. A. O., Bento, F. M., and Triplett, E. W. (2008). Biodiversity of diazotrophic bacteria within the soil, root and stem of field-grown maize. Plant Soil 302, 91–104. doi: 10.1007/s11104-007-9458-3
Rupp, H. M., Frank, M., Werner, T., Strnad, M., and Schmulling, T. (1999). Increased steady state mRNA levels of the STM and KNAT1 homeobox genes in cytokinin overproducing Arabidopsis thaliana indicate a role for cytokinins in the shoot apical meristem. Plant J. 18, 557–563. doi: 10.1046/j.1365-313X.1999.00472.x
Sasse, J., Martinoia, E., and Northen, T. (2018). Feed your friends: do plant exudates shape the root microbiome? Trends Plant Sci. 23, 25–41. doi: 10.1016/j.tplants.2017.09.003
Sharma, S. B., Sayyed, R. Z., Trivedi, M. H., and Gobi, T. A. (2013). Phosphate solubilizing microbes: sustainable approach for managing phosphorus deficiency in agricultural soils. SpringerPlus 2:587. doi: 10.1186/2193-1801-2-587
Su, G., Morris, J. H., Demchak, B., and Bader, G. D. (2014). Biological network exploration with cytoscape 3. Curr. Protoc. Bioinformatics 47, 8.13.1–8.13.24. doi: 10.1002/0471250953.bi0813s47
Subbiah, B. V., and Asija, G. L. (1956). A rapid procedure for estimation of available nitrogen in soils. Curr. Sci. 25, 259–260.
Sun, R., Li, W., Hu, C., and Liu, B. (2019). Long-term urea fertilization alters the composition and increases the abundance of soil ureolytic bacterial communities in an upland soil. FEMS Microbiol. Ecol. 95:fiz044. doi: 10.1093/femsec/fiz044
Tanaka, M., Kato, N., Nakayama, H., Nakatani, M., and Takahata, Y. (2008). Expression of class I knotted1-like homeobox genes in the storage roots of sweetpotato (Ipomoea batatas). J. Plant Physiol. 165, 1726–1735. doi: 10.1016/j.jplph.2007.11.009
Taranet, P., Harper, S., Kirchhof, G., Fujinuma, R., and Menzies, N. (2017). Growth and yield response of glasshouse- and field-grown sweetpotato to nitrogen supply. Nutr. Cycl. Agroecosyst. 108, 309–321. doi: 10.1007/s10705-017-9858-6
Townsley, B., Sinha, N., and Kang, J. (2013). KNOX1 genes regulate lignin deposition and composition in monocots and dicots. Front. Plant Sci. 4:121. doi: 10.3389/fpls.2013.00121
Trapnell, C., Pachter, L., and Salzberg, S. L. (2009). TopHat: discovering splice junctions with RNA-Seq. Bioinformatics 25, 1105–1111. doi: 10.1093/bioinformatics/btp120
Vanstraelen, M., and Benkova, E. (2012). Hormonal interactions in the regulation of plant development. Annu. Rev. Cell. Dev. Biol. 28, 463–487. doi: 10.1146/annurev-cellbio-101011-155741
Wang, C., Zheng, M., Song, W., Wen, S., Wang, B., Zhu, C., et al. (2017). Impact of 25 years of inorganic fertilization on diazotrophic abundance and community structure in an acidic soil in southern China. Soil Biol. Biochem. 113, 240–249. doi: 10.1016/j.soilbio.2017.06.019
Wang, Q., Wang, J., Li, Y., Chen, D., Ao, J., Zhou, W., et al. (2018). Influence of nitrogen and phosphorus additions on N2-fixation activity, abundance, and composition of diazotrophic communities in a Chinese fir plantation. Sci. Total Environ. 619, 1530–1537. doi: 10.1016/j.scitotenv.2017.10.064
Wang, Y., Li, B., Du, M. W., Eneji, A. E., Wang, B. M., Duan, L. S., et al. (2012). Mechanism of phytohormone involvement in feedback regulation of cotton leaf senescence induced by potassium deficiency. J. Exp. Bot. 63, 5887–5901. doi: 10.1093/jxb/ers238
Wasternack, C., and Hause, B. (2013). Jasmonates: biosynthesis, perception, signal transduction and action in plant stress response, growth and development. An update to the 2007 review in Annals of Botany. Ann. Bot. 111, 1021–1058. doi: 10.1093/aob/mct067
Whitcomb, R. F., and Davis, R. E. (1970). Mycoplasma and phytarboviruses as plant pathogens persistently transmitted by insects. Annu. Rev. Entomol. 15:405. doi: 10.1146/annurev.en.15.010170.002201
Wu, H. J., Wang, S., Gao, L. M., Zhang, L., Yuan, Z. W., Fan, T. Y., et al. (2018). Nutrient-derived environmental impacts in Chinese agriculture during 1978-2015. J. Environ. Manage. 217, 762–774. doi: 10.1016/j.jenvman.2018.04.002
Wu, S., Lau, K. H., Cao, Q., Hamilton, J. P., Sun, H., Zhou, C., et al. (2018). Genome sequences of two diploid wild relatives of cultivated sweetpotato reveal targets for genetic improvement. Nat. Commun. 9:4580. doi: 10.1038/s41467-018-06983-8
Xu, Z., Zhao, Y. Q., Yang, D. J., Sun, H. J., Zhang, C. L., and Xie, Y. P. (2015). Attractant and repellent effects of sweet potato root exudates on the potato rot nematode, Ditylenchus destructor. Nematology 17, 117–124. doi: 10.1163/15685411-00002856
Xu, Z. W., Yu, G. R., Zhang, X. Y., Ge, J. P., He, N. P., Wang, Q. F., et al. (2015). The variations in soil microbial communities, enzyme activities and their relationships with soil organic matter decomposition along the northern slope of Changbai Mountain. Appl. Soil Ecol. 86, 19–29. doi: 10.1016/j.apsoil.2014.09.015
Yamashita, N., Tanabata, S., Ohtake, N., Sueyoshi, K., Sato, T., Higuchi, K., et al. (2019). Effects of different chemical forms of nitrogen on the quick and reversible inhibition of soybean nodule growth and nitrogen fixation activity. Front. Plant Sci. 10:131. doi: 10.3389/fpls.2019.00131
Yang, J., Moeinzadeh, M. H., Kuhl, H., Helmuth, J., Xiao, P., Haas, S., et al. (2017). Haplotype-resolved sweet potato genome traces back its hexaploidization history. Nat. Plants 3, 696–703. doi: 10.1038/s41477-017-0002-z
Yu, D., Wildhagen, H., Tylewicz, S., Miskolczi, P. C., Bhalerao, R. P., and Polle, A. (2019). Abscisic acid signalling mediates biomass trade-off and allocation in poplar. New Phytol. 223, 1192–1203. doi: 10.1111/nph.15878
Zhalnina, K., Dias, R., de Quadros, P. D., Davis-Richardson, A., Camargo, F. A. O., Clark, I. M., et al. (2015). Soil pH determines microbial diversity and composition in the park grass experiment. Microb. Ecol. 69, 395–406. doi: 10.1007/s00248-014-0530-2
Zhang, C., Liu, G. B., Xue, S., and Song, Z. L. (2011). Rhizosphere soil microbial activity under different vegetation types on the Loess Plateau, China. Geoderma 161, 115–125. doi: 10.1016/j.geoderma.2010.12.003
Zhang, C., Song, Z., Zhuang, D., Wang, J., Xie, S., and Liu, G. (2019). Urea fertilization decreases soil bacterial diversity, but improves microbial biomass, respiration, and N-cycling potential in a semiarid grassland. Biol. Fertility Soils 55, 229–242. doi: 10.1007/s00374-019-01344-z
Zhang, Z. L., Yuan, Y. S., Zhao, W. Q., He, H. L., Li, D. D., He, W., et al. (2017). Seasonal variations in the soil amino acid pool and flux following the conversion of a natural forest to a pine plantation on the eastern Tibetan Plateau, China. Soil Biol. Biochem. 105, 1–11. doi: 10.1016/j.soilbio.2016.11.002
Keywords: sweetpotato [Ipomoea batatas (L.) Lam.], urea, plant growth-promoting bacteria, biological nitrogen fixation, phytohormone
Citation: Ding Y, Jin Y, He K, Yi Z, Tan L, Liu L, Tang M, Du A, Fang Y and Zhao H (2020) Low Nitrogen Fertilization Alter Rhizosphere Microorganism Community and Improve Sweetpotato Yield in a Nitrogen-Deficient Rocky Soil. Front. Microbiol. 11:678. doi: 10.3389/fmicb.2020.00678
Received: 28 November 2019; Accepted: 24 March 2020;
Published: 15 April 2020.
Edited by:
Camille Eichelberger Granada, Universidade do Vale do Taquari - Univates, BrazilReviewed by:
Vijay Singh Meena, ICAR-Vivekananda Parvatiya Krishi Anusandhan Sansthan, IndiaMarcelo Carvalho Minhoto Teixeira Filho, São Paulo State University, Brazil
Copyright © 2020 Ding, Jin, He, Yi, Tan, Liu, Tang, Du, Fang and Zhao. This is an open-access article distributed under the terms of the Creative Commons Attribution License (CC BY). The use, distribution or reproduction in other forums is permitted, provided the original author(s) and the copyright owner(s) are credited and that the original publication in this journal is cited, in accordance with accepted academic practice. No use, distribution or reproduction is permitted which does not comply with these terms.
*Correspondence: Yang Fang, ZmFuZ3lhbmdAY2liLmFjLmNu; Hai Zhao, emhhb2hhaUBjaWIuYWMuY24=
†These authors have contributed equally to this work and share first authorship